- 1Center of Excellence in Inflammation, Infectious Disease and Immunity, James H. Quillen College of Medicine, East Tennessee State University, Johnson City, TN, United States
- 2Division of Infectious, Inflammatory and Immunologic Diseases, Department of Internal Medicine, Quillen College of Medicine, East Tennessee State University (ETSU), Johnson City, TN, United States
- 3Department of Biochemistry and Cellular and Molecular Biology, University of Tennessee, Knoxville, TN, United States
- 4Hepatitis (HCV/HBV/HIV) Program, James H. Quillen VA Medical Center, Department of Veterans Affairs, Johnson City, TN, United States
Effectively treating infectious diseases often requires a multi-step approach to target different components involved in disease pathogenesis. Similarly, the COVID-19 pandemic has become a global health crisis that requires a comprehensive understanding of Severe Acute Respiratory Syndrome Corona Virus 2 (SARS-CoV-2) infection to develop effective therapeutics. One potential strategy to instill greater immune protection against COVID-19 is boosting the innate immune system. This boosting, termed trained immunity, employs immune system modulators to train innate immune cells to produce an enhanced, non-specific immune response upon reactivation following exposure to pathogens, a process that has been studied in the context of in vitro and in vivo clinical studies prior to the COVID-19 pandemic. Evaluation of the underlying pathways that are essential to inducing protective trained immunity will provide insight into identifying potential therapeutic targets that may alleviate the COVID-19 crisis. Here we review multiple immune training agents, including Bacillus Calmette-Guérin (BCG), β-glucan, and lipopolysaccharide (LPS), and the two most popular cell types involved in trained immunity, monocytes and natural killer (NK) cells, and compare the signaling pathways involved in innate immunity. Additionally, we discuss COVID-19 trained immunity clinical trials, emphasizing the potential of trained immunity to fight SARS-CoV-2 infection. Understanding the mechanisms by which training agents activate innate immune cells to reprogram immune responses may prove beneficial in developing preventive and therapeutic targets against COVID-19.
Introduction
Severe Acute Respiratory Syndrome Corona Virus 2 (SARS-CoV-2), the most recent coronavirus to emerge, has led to the COVID-19 pandemic. As of December 5, 2021, SARS-CoV-2 has infected over 265 million individuals and resulted in over 5.2 million deaths globally (1). Currently, the available mRNA vaccines have been shown to induce and maintain an immune response for at least six months via the production of antibodies against SARS-CoV-2, contributing to reduced viral spread, hospitalization, and mortality (2); however, these vaccines cannot completely control SARS-CoV-2 infection. Moreover, new SARS-CoV-2 variants, such as the delta variant (B 1.617. 2) and the omicron (B. 1. 1. 529), have recently emerged and caused breakthrough infections despite prior vaccinations (3). Many severe cases of COVID-19 progress into acute respiratory distress syndrome (ARDS), mediated by the alteration of the innate immune system that leads to an overproduction of pro-inflammatory cytokines (cytokine storm) (4). Thus, a thorough understanding of innate immune activation during SARS-CoV-2 infection is necessary to develop additional prophylaxis and therapeutics.
There is evidence that innate immune cells are significantly altered during SARS-CoV-2 infection, with severe COVID-19 patients displaying lower levels of total monocytes (5) and a decreased number and activity of natural killer (NK) cells (6). A potential solution for treating coronavirus infections is training of the host’s innate immune system, termed trained immunity, which elicits immune protective effects in several cell types by activating specific signaling pathways. The clear involvement of the innate immune system in COVID-19 immunologic dysregulation necessitates an in-depth characterization of innate immunological mechanisms. Additionally, trained immunity may benefit elderly populations which have a general decreased immune response to vaccines (7). The field of trained immunity has demonstrated many advances in boosting the innate immune system against both bacterial and viral infections, and these may be applied to preventing and/or treating SARS-CoV-2 infection.
To evaluate trained immunity in the era of COVID-19, this review focuses on an overview of trained immunity mechanisms and their impact on COVID-19. Specifically, we describe both individual immune training agents and different innate immune cell types to distinguish specific pathways through which trained immunity can be induced. Furthermore, we relate these pathways to prospective trained immunity COVID-19 therapeutics using clinical trial data. Finally, we summarize the possible implications and future directions of trained immunity in COVID-19. A thorough understanding of these pathways and the subsequent trained immune responses is critical to evaluate how trained innate immunity can be exploited to SARS-CoV-2 infection.
Trained Innate Immunity: A Method to Induce Heterologous Immunological Effects
Host immune responses can be distinguished as innate, which protects in a quick and nonspecific manner, and adaptive, which is slower to act but can induce specific and robust immunological responses via interactions between antigen presenting cells (APCs), such as dendritic cells (DCs) and B cells, and T cells. Although innate immunity is generally considered to be non-specific against invading pathogens, there is evidence that infections and inflammation can induce natural memory in innate immune cells (8). For example, natural killer (NK) cells can undergo expansion, similar to clonal expansion observed in adaptive immune cells, driven by pro-inflammatory cytokines such as IL-12 and IL-18 (9). Innate lymphoid cells (ILCs), which include cytotoxic ILCs (NK cells) and non-cytotoxic ILCs (ILC1, ILC2, and ILC3), are innate immune cells that can confer immunological memory. These ILCs are defined as cells that express cytokine receptors and are capable of transcriptional modifications to regulate tissue damage/repair and induce inflammation. However, unlike T or B lymphocytes, ILCs cannot undergo semantical rearrangement to generate antigen receptors required to confer antigen specificity (10). The presence of ILCs, which provide transcriptionally regulated immunological memory, suggests that the innate immune system is more complex than previously considered, warranting detailed investigations of how the innate immune system may be trained.
Given the recent challenges to the concept that innate immunity is naïve and cannot possess trained capabilities, the field of trained immunity has given rise to novel approaches of mediating robust immune responses. Priming induces alterations to the immune status and functional state of cells, which do not return to basal levels prior to secondary stimulation, allowing for an additive effect of stimuli. Alternately, trained immunity is defined as the capacity of the innate immune system to maintain immunological memory that can protect against secondary challenge following returning to the resting state via a persistent epigenetic signature (11, 12). Due to the identification of numerous training agents able to induce trained immunity, a trained immunity database (TIDB) has been assembled (13). Trained immunity has been utilized to epigenetically program several innate immune cells (monocytes, NKs, myeloid cells, neutrophils, and non-cytotoxic ILCs) to elicit a stronger immune response upon subsequent infection (14). Harnessing the capabilities of trained immunity for COVID-19 first requires an understanding of the pathways responsible for mediating this heterologous immunity and which training agent(s) induce these immunological modifications.
Immune Training Agents Employ Multiple Pathways to Induce Trained Immunity
Trained immunity has been explored as a potential therapeutic against SARS-CoV-2 infection since the beginning of the COVID-19 pandemic. Several immune training agents, such as bacillus Calmette-Guérin (BCG), β-glucan, and lipopolysaccharide (LPS), have been used to stimulate innate immune cells into a protective state against subsequent challenges (Figure 1). Arguably the most notable training agent involved in inducing trained immunity is the live attenuated vaccine, BCG, intended to immunize against M. tuberculosis (15, 16). The BCG vaccine has been hypothesized to provide heterologous immunity against SARS-CoV-2 by epigenetically reprogramming monocytes, conferring an enhanced immune response upon secondary challenge (17). β-glucan, a polysaccharide isolated from Candida albicans, has also been found to possess immunogenic antiviral properties that could help reduce SARS-CoV-2 severity (18). LPS, which is commonly used to restimulate trained cells, can also act as a training agent by inducing persistent modifications in genes responsible for an increased response to secondary infection (19). Collectively, these data provide a foundation implicating these agents as inducers of trained immunity and propose their potential application in treating COVID-19.
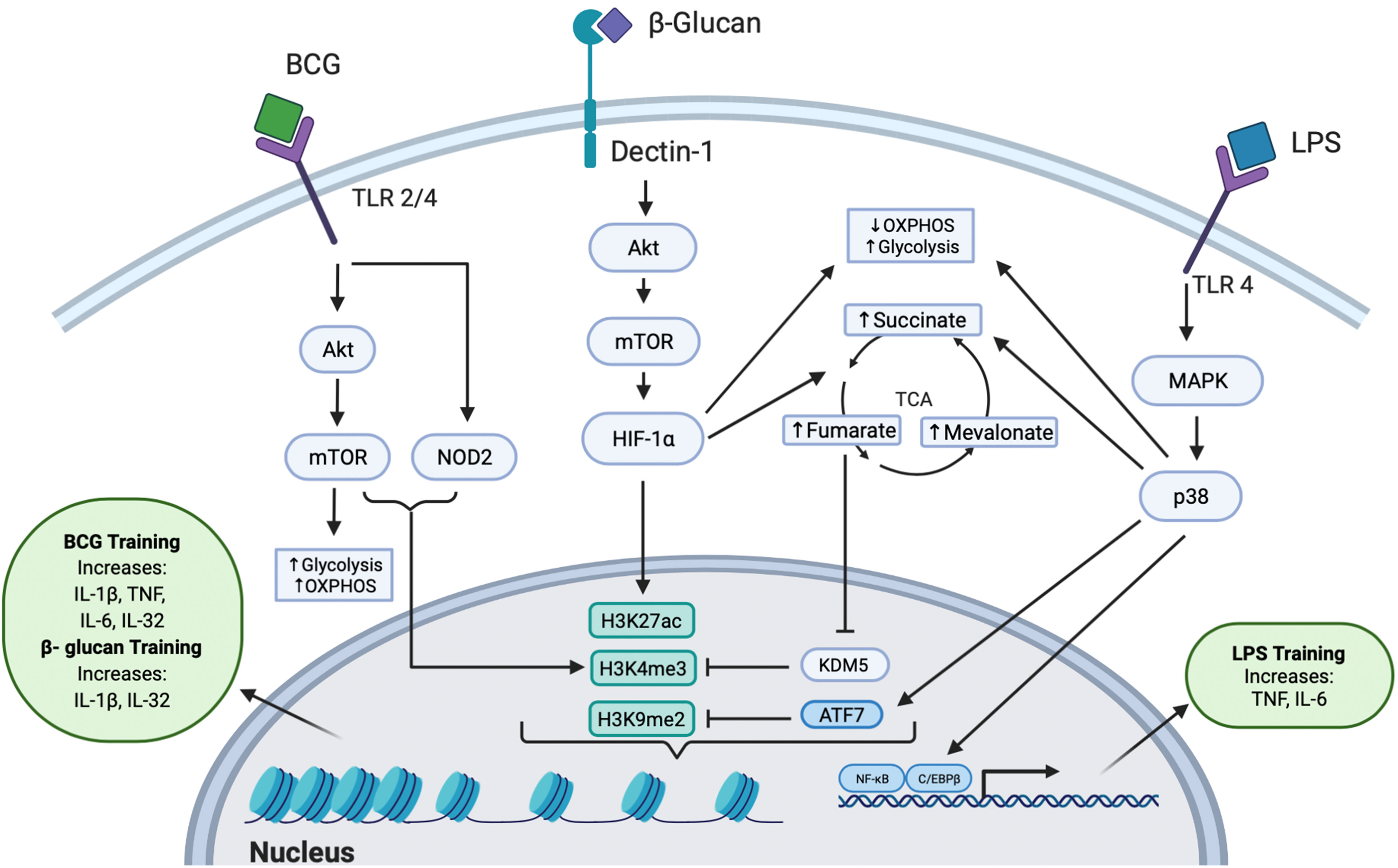
Figure 1 Intracellular Pathways Responsible for Trained Immunity. All three training agents train innate immunity to induce epigenetic modifications through multiple pathways. BCG binds to TLR 2 or TLR 4 and signals via the Akt/mTOR and NOD2 pathways, leading to downstream epigenetic modifications at H3K4me3. Activation of the Akt/mTOR pathway leads to upregulation of OXPHOS and glycolysis. The epigenetic change of H3K4me3 induced an increased production of IL-1β, TNF, IL-6, and IL-32 cytokines. β-glucan binds to the dectin-1 receptor to mediate downstream signaling through the Akt/mTOR/HIF-1α pathway. This signaling leads to decreased OXPHOS, increased glycolysis, and increased production of fumarate, succinate, and mevalonate in the TCA cycle. Fumarate further inhibits the KDM5 histone demethylase which acts at H3K4me3. β-glucan induced trained immunity was also shown to induce dynamic epigenetic changes at H3K27ac. The epigenetic changes further lead to increased IL-1β and IL-32 secretion. LPS can induce trained immunity by binding to TLR4 and signaling through the MAPK/p38 pathway, resulting in reduced OXPHOS, increased glycolysis, increased levels of succinate, and activation of transcription factors C/EBPβ and NF-κB. Additionally, LPS induces the phosphorylation and release of the inhibitory transcription factor ATF7, which inhibits the repressive histone mark H3K9me2. Collectively, these epigenetic changes upregulate the production of TNF and IL-6.
BCG
BCG can be used as a training agent via modulating intracellular signaling pathways by binding to both toll like receptor 2 (TLR2) and TLR4 to induce trained immunity (20). Subsequently, training monocytes with BCG activates the mammalian target of rapamycin (Akt/mTOR) pathway, leading to upregulation of glycolysis, oxidative phosphorylation (OXPHOS), and glutamine metabolism (21). In addition to the Akt/mTOR pathway, the nucleotide-binding oligomerization domain 2 (NOD2) pathway [activated via TLR2/4, which can induce IL-6, IL-1β, and tumor necrosis factor (TNF) (22, 23)], was found to be necessary for BCG-training of monocytes: blocking NOD2 by mutation or chemical inhibition resulted in BCG-trained monocytes that produced significantly lower amounts of TNF upon restimulation (24).
A study of severe combined immunodeficiency (SCID) mice treated with BCG demonstrated enhanced release of cytokines IL-1β and TNF in monocytes in a NOD2-dependent manner upon infection with Candida albicans, which was found to be epigenetically controlled by histone 3 lysine 4 trimethylation (H3K4me3), an open chromatin histone marker (24). Similarly, the Akt pathway was involved in BCG-trained neutrophils, inducing increased H3K4me3 patterns, specifically near promoter regions of genes encoding pro-inflammatory cytokines (CXCL8 and IL-1β) and genes involved in glycolysis (mTOR and PFKB); this lasted at least three months post-vaccination (25). Epigenetic modifications at genes involved in signaling pathways such as AKT1, MAPKs, and PI3K (26) are responsible for inducing trained immunity and have been discovered within the epigenome of specific effector cells such as monocytes (27). This suggests that global epigenetic modifications also directly influence the “trained” functions of specific innate immune cells. Modulations in gene expression result in the increased production of cytokines involved in trained innate immunity. For example, IL-1β appears to be a critical cytokine induced by BCG-training: in BCG-trained monocytes, IL-1β levels increased after restimulation conferring non-specific protection against yellow fever viremia (26). The antimicrobial cytokine IL-32, which is known to be involved in viral defense and capable of modulating cellular metabolism (28), has also been demonstrated to play a role in BCG trained immunity, presumably by activating the NOD2 signaling pathway (29) (Figure 1).
Taken together, signaling pathways and epigenetic modifications induced by BCG-training are responsible for the enhanced innate immune response upon restimulation. Many of these signaling pathways are activated in different cell types within the innate immune system, suggesting that training with BCG may utilize conserved pathways. Since BCG vaccination has been demonstrated to possess therapeutic effects against respiratory infections, it has been hypothesized that BCG may offer heterologous immunity against SARS-CoV-2 infection (17, 30, 31). Therefore, ongoing clinical trials are investigating the relationship between BCG vaccination and protection against COVID-19. Currently, the data suggests that BCG-trained immune responses are present 3 months post BCG-treatment (24); thus, further research should be conducted to evaluate the cellular response of BCG-trained cells for longer times to determine the maximal duration of memory response and protection.
β-Glucan
β-glucan is an immunomodulator that functions as a pathogen associated molecular pattern (PAMP) recognized by pattern recognition receptors (PRRs) expressed on innate immune cells. The immunomodulatory activity of β-glucan not only depends on its physical properties (for example, particle size, molecular weight, conformation, branching frequency, or solubility) but also on the cell type it acts upon, suggesting a highly diverse and targetable approach for β-glucan training (31). β-glucan signaling occurs in monocytes, macrophages, and DCs by the dectin-1 receptor, which activates an intracellular signaling cascade that drives the trained immune response (32–34). Upon β-glucan binding to dectin-1, the downstream Akt/mTOR/HIF1α pathway is activated, leading to subsequent metabolic modifications, including the downregulation of OXPHOS and upregulation of glycolysis (35), resulting in the increased production of tricarboxylic acid (TCA) cycle by-products, including succinate (36), fumarate (37), and mevalonate (a precursor for cholesterol synthesis) (38). In addition to binding to the dectin-1 receptor, β-glucan has been suggested to utilize other receptors to induce trained immunity (39).
Intracellular modifications of the TCA cycle driven through the Akt/mTOR/HIF1α pathway were found to play a key role in β-glucan trained immunity. Training with β-glucan was associated with increased levels of succinate, which has been suggested to have potential therapeutic benefits against sepsis-induced immunoparalysis (36). Fumarate, a metabolite involved in the TCA cycle, was found to increase cytokine production in a dose-dependent manner by inhibiting KDM5 histone demethylases, thereby leading to epigenetic changes (trimethylation, H3K4me3) at promoter regions of pro-inflammatory cytokine genes (37). Interestingly, KDM5B also regulates angiotensin-converting enzyme 2 (ACE2), the receptor involved in SARS-CoV-2 viral entry (40), providing a potential therapeutic target. Fumarate also stabilizes HIF1α by inhibiting hydroxylation, resulting in the induction of 2,688 dynamic H3K27ac changes, as revealed by chromatin immunoprecipitation followed by sequencing (ChIP-seq) (37). Monocyte training with mevalonate, indirectly synthesized from acetyl CoA, increased cytokine production upon restimulation. Importantly, inhibition of mTOR signaling or glycolysis impedes mevalonate training (38). Thus, β-glucan trained immunity is induced through distinct signaling pathways which drive metabolic and subsequent epigenetic modifications to maintain a sustained, non-specific response to invading pathogens (Figure 1).
Epigenetic changes induced by β-glucan in hematopoietic stem and progenitor cells (HSPCs) enable short-lived blood circulating monocytes to respond to secondary challenge, which leads to an increased level of IL-1β in trained monocytes, further inducing the production of IL-32 (41). Similarly, epigenetic reprograming of HSPCs by β-glucan drives trained immunity in an IL-1 dependent manner (42). Thus, β-glucan has a strong potential to be used as a training agent and already has prior applications as an oral supplement, which have been discussed in preventing SARS-CoV-2 infection (43). For example, since IL-1 is involved in COVID-19 dysregulated immune responses, recent studies have determined that blocking its receptor (IL-1R) can be used to treat severe COVID-19 infection (44). Since IL-1β production is greatly increased during COVID-19 (45), β-glucan trained immunity may enable the innate immune system to mount a stronger early immune response to prevent dysregulated cytokine production. This training of innate immune cells to increase production of key cytokines may ultimately improve the immune response upon SARS-CoV-2 infection.
LPS
LPS, a microbial component comprising gram-negative bacterial membranes, binds to TLR4 and has primarily been used to restimulate cells after BCG or β-glucan training, but can also elicit trained immunity under certain conditions (46). Innate immune cells can be primed, resulting in transcriptional changes that provide an increased basal innate immune response (12). Prolonged priming with LPS results in reduced cytokine production and immunosuppression, inducing immune tolerance (47). These effects are due to reduced expression of TLR4, reduced TLR4/MyD88 interaction, and reduced activity of p38 (47). LPS immune tolerance was found to block the accumulation of histone marks at promotors or enhancers (H3K27ac and H3K4me1) of genes that are responsible for lipid metabolism and phagocytic pathways (48). LPS triggers transient silencing of inflammatory genes (associated with methylation at H3K9 and H3K27) as well as inducing antimicrobial effector genes (49). Training with LPS has been demonstrated to reduce inflammation and fibrosis in systemic sclerosis models by downregulating production of proinflammatory cytokines (IL-6, TNF, IL-1β) and instead upregulating production of the anti-inflammatory cytokine IL-10 (50, 51).
Conversely, lower doses of LPS treatment result in an increase of TNF and IL-6 upon restimulation (52), suggesting a role for LPS in trained immunity. LPS can induce trained immunity by activating innate immune cells like macrophages through chromatin and transcriptional modifications via signaling through the MAPK/p38 pathway (Figure 1) (11, 52). These include epigenetic modifications in myeloid cells, where treatment with LPS is associated with chromatin remodeling, such as an increased number of activating marks (53). Evidence suggests that these changes may be long lasting, since LPS stimulation of macrophages induced persistent methylation at H3K4 that corresponded to an enhanced response upon secondary stimulation (54). Additionally, LPS can induce trained immunity through regulation of transcription factors, like ATF7. LPS-treated macrophages were shown to induce phosphorylation and subsequent release of ATF7 via the p38 pathway, resulting in a decrease of repressive H3K9me2, which persists for long periods of time to increase resistance to subsequent reinfection (55). LPS has also been identified to induce epigenetic changes within hematopoietic stem cells (HSCs) that are dependent on the transcription factor C/EBPβ, which likely facilitates the long-lasting transcriptional changes shown to be induced by LPS (19). Similar to β-glucan, LPS treatment has been demonstrated to downregulate OXPHOS and upregulate glycolysis, resulting in increased levels of succinate (56), suggesting that LPS may induce trained immunity through multiple signaling pathways.
Finally, LPS has been demonstrated to indirectly decrease ACE2 expression via NF-κB-dependent upregulation of miR-200c-3p during H5N1 virus infection (57), suggesting that LPS may have additional preventative and therapeutic roles in COVID-19. Taken together, LPS training may provide beneficial effects to impair the ability of viral infectiousness and reduce severe inflammation in COVID-19.
Alternative Immune Training Agents and Antagonists
In addition to BCG, β-glucan, and LPS, alternative immune training agents have been investigated, including aldosterone and oxidized low-density lipoprotein (oxLDL). Aldosterone-trained monocytes were characterized by enriched H3K4me3 at the promoter regions of genes involved in lipid synthesis (58). Similar to BCG treatment, oxLDL training upregulates both OXPHOS and glycolysis (59), which similarly results in increased TCA metabolites (60). Increased levels of proinflammatory cytokines IL-6 and TNF were mediated by the mTOR/HIF-1α pathway (61). These data suggest that oxLDL shares similar yet distinct pathways with BCG and β-glucan training, providing the opportunity to selectively train innate immunity by modifying metabolic pathways.
In contrast, several studies have highlighted that some reagents function as training antagonists, which can function to inhibit cell signaling or alter epigenetic modifications required to induce trained immunity. For example, BCG-incubated monocytes co-incubated with ARTA (a vitamin A metabolite) displayed reduced cytokine production, suggesting the role of ARTA as a trained immunity antagonist. This unique signature was determined to be caused by both increased expression of SUV39H2, a histone methyltransferase that induces the inhibitory histone 3 lysine 9 trimethylation (H3K9me3), and by downregulation of the stimulatory H3K4me3 (62). In addition to ARTA, some cytokines may also function as trained immunity antagonists. The anti-inflammatory cytokines IL-37 and IL-38 as well as the drug Hydroxychloroquine have demonstrated inhibitory effects against trained immunity. Both IL-37 and IL-38 inhibit β-glucan-mediated trained immunity by blocking mTOR signaling (63). IL-37 inhibits β-glucan trained immunity by reversing immunometabolic and histone modifications, thus suppressing production of pro-inflammatory cytokines (64). Likewise, hydroxychloroquine acts through the Akt/mTOR pathway to inhibit β-glucan trained immunity by preventing the production of TNF, IL-6, and IL-1β cytokines via blocking epigenetic modifications (65). Taken together, these trained immunity antagonists may be used with training reagents simultaneously to regulate the level of the immune response.
Diversity of Cell Types That Can Develop Trained Immunity
In addition to evaluating the general intracellular mechanisms associated with trained immunity based on individual immune training agents, it is also important to identify the innate immune cell types capable of being trained. As listed in Table 1, immune training agents and antagonists have been demonstrated to induce or suppress trained immunity in different cell types with varying effects and durations. The innate immune system consists of a variety of different cells, of which monocytes and NK cells have demonstrated adaptive immune characteristics similar to T cells (71–73). Monocytes undergo epigenetic programing, modifying key metabolic pathways that enable an enhanced response to subsequent stimulation (74). Similarly, epigenetic reprograming of NK cells results in clonal expansion and cytokine secretion (11), which may also play a role in activating monocytes during their development (75). Understanding the cell-specific mechanisms through which trained immunity is induced is necessary to selectively target and modify host immunity to induce a stronger innate immune response upon SARS-CoV-2 exposure.
Monocytes in Trained Immunity and COVID-19
Monocytes are critical innate immune cells that have a relatively short lifespan, circulating in the blood stream for 3 to 5 days (76). Despite their short lifespans, monocytes have been effective in presenting trained immune responses, which are driven by epigenetic histone modifications and have been shown to last at least 3 months (24), suggesting that monocyte reprograming may occur within the bone marrow and progenitor cells (11). Recent studies have classified trained immune responses as belonging to central trained immunity (occurring in bone marrow progenitor cells) and peripheral trained immunity (in blood monocytes and tissue macrophages). Monocyte reprogramming occurs at both levels, first by reprograming HSCs in the bone marrow followed by migration and potential differentiation into macrophages within the blood (74).
Epigenetic modifications after monocyte training drive subsequent changes in metabolic signatures. β-glucan trained monocytes exhibit increased glucose consumption and a shift from OXPHOS towards aerobic glycolysis (35), similar to what is observed in cancer metabolism (77). Epigenetic changes may be more dynamic than a few chromatin modifications, as training monocytes with β-glucan caused histone acetylation changes which resulted in approximately 8,000 dynamic regions (51). Taken together, these studies demonstrate that epigenetic modifications are responsible for sustained training effects in monocytes.
Monocytes have been shown to be involved in the innate immune response to COVID-19 (78). Several pro-inflammatory proteins, such as CXCL-10, IL-6, IL-10, IL-12, and TNF, have been identified to be upregulated in severe COVID-19 patients. Upregulation of these chemokine and cytokines were related to increased leptin, a hormone that signals through the NF-κB and STAT3 pathways (79). Similar to the effects of trained immunity, epigenetic changes such as open chromatin marks were present in monocytes from acute-COVID-19 patients and persisted six months after recovery from COVID-19 (80). Peripheral monocytes from COVID-19 patients exhibited mitochondrial dysfunction as evidenced by reduced glycolysis (81). Since trained immunity in monocytes is characterized by an upregulation of glycolysis (35), in vivo training of monocytes may help counteract this reduced glycolysis in COVID-19 patients. Identifying similar monocyte signatures during SARS-CoV-2 infection and innate immune training may help determine a role for trained immunity to prevent or treat COVID-19.
NK Cells in Trained Immunity and COVID-19
Although NK cells are part of the innate immune system, they also share certain memory characteristics of adaptive immune cells (11, 82). NK cells can respond to host infections by recognizing cytokines IL-12 and IL-18 and producing interferon-gamma (IFN-γ) and TNF, as well as excreting perforin and granzymes which penetrate the membrane of an infected cell and induce apoptosis (83). Most innate immune cells are typically considered to be short lived; however, NK cells can live for longer than 6 months in both lymphoid and non-lymphoid organs (84). Similar to cytotoxic T cells, it has been shown that NK cells specific to a cytomegalovirus (CMV) virus receptor have a lifespan of several months (85). Memory NK cells are distinguished by their abilities of enhanced mitochondrial fitness, regulation, and OXPHOS capacity (86).
Although NK cells trained by BCG have been shown to upregulate the production of pro-inflammatory cytokines (IL-1β, IL-6, TNF) (16), the specific mechanism by which trained immunity is induced in NK cells is still poorly understood. The general mechanisms of NK memory and proliferation have been studied in murine models. Not surprisingly, many NK memory-like functions are driven by epigenetic modifications. In CMV infected mice, NK cells were able to mount adaptive immune functions which were subsequently identified to be a result of DNA hypermethylation patterns, similar to cytotoxic T cells (87). In vitro stimulation of NK cells with cytokines IL-12 and IL-18 followed by adoptive transfer into immune deficient mice led to memory-like NK responses that lasted for three months (88). Similar to trained monocytes which are characterized by metabolic reprogramming, recent studies of NK cell metabolism have shown that key metabolic energy pathways such as OXPHOS and glycolysis drive NK anti-viral responses. Moreover, NK cells shift from cytotoxic proliferation to a quiescent state upon resolution of inflammation, indicating that NK cells are highly dynamic which is beneficial for the trained immunity process (86). Metabolic analysis demonstrated that after exposure to a high concentration of IL-15, NK cells became activated via the mTOR pathway; inhibition of this pathway rendered NK cells incapable of proliferating and mounting cytotoxic responses (89). Finally, NK cells may play an indirect role in trained immunity by modulating monocyte function. It has been determined that bone-marrow-resident NK cells promote monocyte effector functions by secreting IFN-γ (75). Alternatively, innate immune crosstalk may also occur in the opposite direction, as macrophages can activate NK cells through secretion of IL-12 and IL-18 or direct ligand interactions (90, 91) Thus, the crosstalk among innate immune cells should be further investigated in trained immunity.
Since NK cells possess memory-like characteristics, training NK cells may allow for a more robust innate response against COVID-19. In hospitalized COVID-19 patients, overall NK cell counts were low; however, the level of adaptive NK cells was increased (92). Furthermore, in severe COVID-19 patients, NK cells were functionally impaired due to elevated IFN-α and TNF signaling (93), suggesting that dysregulation of NK cells is involved in pathogenesis during SARS-CoV-2 infection. Studying the underlying mechanisms by which trained immunity may enhance NK cell function could illustrate the potential application of training NK cells as a COVID-19 therapeutic approach.
Clinical Studies of Trained Immunity in COVID-19
The evidence generated from experimental research regarding the effects of trained immunity has led to its application in translational clinical research. The immune response induced by BCG vaccination in the context of trained immunity has already been thoroughly reviewed (94, 95). Recent studies have evaluated these heterologous effects in relation to respiratory disease. BCG vaccination induced nonspecific effects in reducing infant mortality by 38%, with fewer cases of respiratory infection, fever, and sepsis (96, 97). A cohort of 58,021 children who were vaccinated with BCG showed 17-37% risk reduction for suspected acute lower respiratory tract infections (98). Current opinion suggests that the heterologous effects derived from BCG vaccination may achieve COVID-19 protection, and as such several ongoing clinical trials are investigating the protective effects of BCG in relation to COVID-19 (17, 30, 99–102). Several phase 2/3/4 clinical trials are ongoing in multiple countries to evaluate the efficacy of BCG vaccination as a preventative and therapeutic agent against COVID-19 among health care workers and elderly populations (Table 2).
In addition to BCG, β-glucan has also been demonstrated to strengthen immune responses by targeting macrophages, DCs, and NK cells. A 2014 clinical study of 60 children orally treated with β-glucan showed successful stimulation of mucosal immunity in children with chronic respiratory problems (103, 104). Administration of β-glucan has been shown to correlate with improved respiratory symptoms (105, 106). For example, a study demonstrated that marathon runners who received β-glucan post-marathon reported fewer respiratory symptoms (107). Currently, one clinical trial is investigating ABBC1, a β-glucan dietary supplement, as a potential supplement for improving the efficacy of COVID-19 vaccination (Table 2).
IFN treatments have also demonstrated anti-viral effects and have been proven to be involved in trained immunity responses (108). One study determined that IFN-γ secreted by CD8 T cells can activate alveolar macrophages to induce memory characteristics (109). Type I IFNs have been previously used to treat SARS and MERS (110, 111), suggesting IFNs may be used as a potential COVID-19 therapy (112, 113). A recent clinical trial showed COVID-19 patients treated with IFN β-1a experienced faster recovery (114) and reduced mortality (115, 116). Ongoing clinical trials are using different forms of IFN, including IFN α-2b, IFN β-1a, and IFN β-1b, to investigate their role against SARS-CoV-2 infection (Table 2).
Most clinical trials for trained immunity seek to evaluate the efficacy of immune training by measuring protective immune responses and comparing the number of SARS-CoV-2-infected individuals between experimental and placebo groups. Trained immunity clinical trials can allow for the determination of different protective immune responses in COVID-19 vaccinated or infected individuals. Thus, clinical trials investigating heterologous protective immune responses may lead to discovery of alternative clinical applications of trained immunity.
Future Directions and Conclusions: A Potential Approach to Fighting COVID-19
The field of trained innate immunity has demonstrated that innate immune responses are more complex and adaptive than previously thought. Additionally, the use of different training agents has illustrated a variety of specific intracellular pathways that can be targeted. The past two decades of trained immunity research have provided sufficient preliminary in vitro and in vivo data to merit the ongoing COVID-19 clinical trials.
Although mRNA vaccines have proved effective against the alpha COVID-19 variant, there has been reduced efficacy against the delta and omicron COVID-19 variants (117). Breakthrough infections among vaccinated individuals are beginning to rise (118), likely due to the increased virulence and ability of SARS-CoV-2 variants to escape antibody neutralization (119, 120). These data warrant further research of alternative or supplemental methods to fight COVID-19 infection, such as training innate immunity (121), to prophylactically strengthen the innate immune system against SARS-CoV-2 infection. As shown in Figure 2, we expect individuals treated with training agents to mount a more robust innate immune response upon SARS-CoV-2 infection compared to untrained individuals. Additionally, we also expect that training innate immunity could be beneficial for COVID-19 vaccinated individuals by reducing breakthrough infections, disease severity, and mortality via enhanced immune responses. Furthermore, trained immunity may be beneficial in protecting the elderly against COVID-19 infection. Although aging is characterized by immunosenescence and a general dysregulation of both adaptive and innate immune systems, certain cell types remain preserved, including monocytes and NK cells (122, 123). Studies have highlighted the ability of BCG vaccination to induce the production of pro-inflammatory cytokines and reduce acute upper respiratory tract infections in elderly populations (124). Thus, utilizing trained immunity to prophylactically bolster preserved innate immune cells may improve elderly immune responses to COVID-19. However, immunosenescence may also affect epigenetic modifications such as histone methylation and acetylation (125). DNA hypermethylation at H3K9me3 and H3K27me3 has been associated with aging (126). Data from murine models has also shown that H3K9 acetylation decreased with age, suggesting that the presence of transcriptionally available chromatin decreases with age (127). Thus, further studies are needed to evaluate if epigenetic changes during immunosenescence alter trained immune responses. Data has also suggested changes in the presence and functionality of immune cells with increased age. Some cell types have been shown to have an age-related impairment, including neutrophils and DCs, while other cell types, such as NKs, have been shown to be preserved or even hyper-regulated with age (128, 129). Taken together, these data indicate the possibility that factors such as age may influence epigenetic reprogramming efficiency.
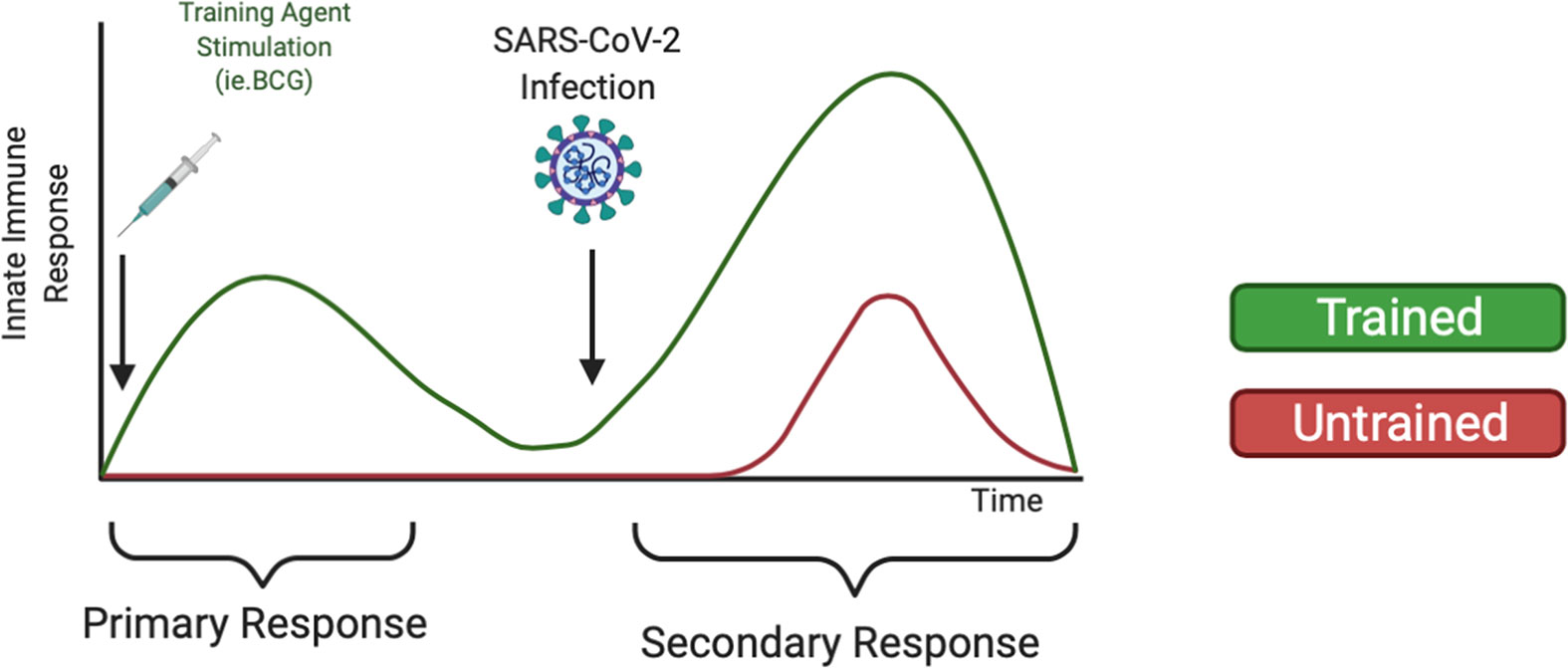
Figure 2 Heterologous Training of Innate Immunity Could Mediate Increased Secondary Response Upon COVID-19 infection. Trained immunity has been demonstrated to non-specifically train innate immune cells such as monocytes and NK cells against pathogens. Using training agents, like the BCG vaccine or β-glucan supplements, could strengthen the innate immune system to mount an increased innate immune response upon SARS-CoV-2 infection.
Recently, a considerable number of SARS-CoV-2-infected individuals have been shown to have “long COVID”, a condition marked by persistent symptoms lasting weeks to months after infection (130); however, the long-term impact of SARS-CoV-2 infection remains unclear. It is possible that persistent symptoms are a side effect of a dysregulated immune reaction during SARS-CoV-2 infection, which could be ameliorated with trained immunity approaches. Ongoing clinical trials assessing the immunogenicity of BCG, β-glucan, and IFNs against COVID-19 (100, 101) will hopefully provide promising evidence for COVID-19 prevention and therapeutics. To fully deploy a multi-faceted approach, it is important to explore all avenues of research, including the application of trained immunity, to investigate the underlying immunological processes that can be targeted for SARS-CoV-2 infection.
Author Contributions
Conceptualization, JB and JM. Literature review, JB. Writing-original draft preparation, JB, JZ, and MS. Writing-review and editing, JB, JZ, MS, ZY, and JM. Supervisions: ZY and JM. All authors have read and agreed to the published version of the manuscript.
Funding
This work was supported by American Diabetes Association award 7-20-COVID-149 (ZY) and Biomedical Laboratory Research and Development Merit Review Award (Department of Veterans Affairs, 5I01BX005428, JM).
Author Disclaimer
The contents in this publication do not represent the views of the Department of Veterans Affairs or the United States Government.
Conflict of Interest
The authors declare that the research was conducted in the absence of any commercial or financial relationships that could be construed as a potential conflict of interest.
Publisher’s Note
All claims expressed in this article are solely those of the authors and do not necessarily represent those of their affiliated organizations, or those of the publisher, the editors and the reviewers. Any product that may be evaluated in this article, or claim that may be made by its manufacturer, is not guaranteed or endorsed by the publisher.
Acknowledgments
This publication is the result of work supported with resources and the use of facilities at the James H. Quillen Veterans Affairs Medical Center.
References
1. World Health Organization. Coronavirus Disease (COVID-2019) Situation Reports 2021. Available at: https://www.who.int/emergencies/diseases/novel-coronavirus-2019/situation-reports.
2. Tartof SY, Slezak JM, Fischer H, Hong V, Ackerson BK, Ranasinghe ON, et al. Effectiveness of mRNA BNT162b2 COVID-19 Vaccine Up to 6 Months in a Large Integrated Health System in the USA: A Retrospective Cohort Study. Lancet (2021) 398(10309):1407–16. doi: 10.1016/S0140-6736(21)02183-8
3. Bergwerk M, Gonen T, Lustig Y, Amit S, Lipsitch M, Cohen C, et al. Covid-19 Breakthrough Infections in Vaccinated Health Care Workers. N Engl J Med (2021) 385(16):1474–84. doi: 10.1056/NEJMoa2109072
4. Prompetchara E, Ketloy C, Palaga T. Immune Responses in COVID-19 and Potential Vaccines: Lessons Learned From SARS and MERS Epidemic. Asian Pac J Allergy Immunol (2020) 38(1):1–9. doi: 10.12932/AP-200220-0772
5. Qin S, Jiang Y, Wei X, Liu X, Guan J, Chen Y, et al. Dynamic Changes in Monocytes Subsets in COVID-19 Patients. Hum Immunol (2021) 82(3):170–6. doi: 10.1016/j.humimm.2020.12.010
6. Osman M, Faridi RM, Sligl W, Shabani-Rad MT, Dharmani-Khan P, Parker A, et al. Impaired Natural Killer Cell Counts and Cytolytic Activity in Patients With Severe COVID-19. Blood Adv (2020) 4(20):5035–9. doi: 10.1182/bloodadvances.2020002650
7. Bulut O, Kilic G, Dominguez-Andres J, Netea MG. Overcoming Immune Dysfunction in the Elderly: Trained Immunity as a Novel Approach. Int Immunol (2020) 32(12):741–53. doi: 10.1093/intimm/dxaa052
8. Netea MG. Immunological Memory in Innate Immunity. J Innate Immun (2014) 6(2):117–8. doi: 10.1159/000357283
9. Sharrock J, Sun JC. Innate Immunological Memory: From Plants to Animals. Curr Opin Immunol (2020) 62:69–78. doi: 10.1016/j.coi.2019.12.001
10. Artis D, Spits H. The Biology of Innate Lymphoid Cells. Nature (2015) 517(7534):293–301. doi: 10.1038/nature14189
11. Netea MG, Joosten LA, Latz E, Mills KH, Natoli G, Stunnenberg HG, et al. Trained Immunity: A Program of Innate Immune Memory in Health and Disease. Science (2016) 352(6284):aaf1098. doi: 10.1126/science.aaf1098
12. Divangahi M, Aaby P, Khader SA, Barreiro LB, Bekkering S, Chavakis T, et al. Trained Immunity, Tolerance, Priming and Differentiation: Distinct Immunological Processes. Nat Immunol (2021) 22(1):2–6. doi: 10.1038/s41590-020-00845-6
13. Cao Y, Dong Q, Wang D, Liu Y, Zhang P, Yu X, et al. TIDB: A Comprehensive Database of Trained Immunity. Database (Oxford) (2021) 2021. doi: 10.1093/database/baab041
14. Bekkering S, Dominguez-Andres J, Joosten LAB, Riksen NP, Netea MG. Trained Immunity: Reprogramming Innate Immunity in Health and Disease. Annu Rev Immunol (2021) 39:667–93. doi: 10.1146/annurev-immunol-102119-073855
16. Kleinnijenhuis J, Quintin J, Preijers F, Joosten LA, Jacobs C, Xavier RJ, et al. BCG-Induced Trained Immunity in NK Cells: Role for Non-Specific Protection to Infection. Clin Immunol (2014) 155(2):213–9. doi: 10.1016/j.clim.2014.10.005
17. O'Neill LAJ, Netea MG. BCG-Induced Trained Immunity: Can it Offer Protection Against COVID-19? Nat Rev Immunol (2020) 20(6):335–7. doi: 10.1038/s41577-020-0337-y
18. Geller A, Yan J. Could the Induction of Trained Immunity by Beta-Glucan Serve as a Defense Against COVID-19? Front Immunol (2020) 11:1782. doi: 10.3389/fimmu.2020.01782
19. de Laval B, Maurizio J, Kandalla PK, Brisou G, Simonnet L, Huber C, et al. C/EBPbeta-Dependent Epigenetic Memory Induces Trained Immunity in Hematopoietic Stem Cells. Cell Stem Cell (2020) 26(5):657–74 e8. doi: 10.1016/j.stem.2020.01.017
20. Heldwein KA, Liang MD, Andresen TK, Thomas KE, Marty AM, Cuesta N, et al. TLR2 and TLR4 Serve Distinct Roles in the Host Immune Response Against Mycobacterium Bovis BCG. J Leukoc Biol (2003) 74(2):277–86. doi: 10.1189/jlb.0103026
21. Arts RJW, Carvalho A, La Rocca C, Palma C, Rodrigues F, Silvestre R, et al. Immunometabolic Pathways in BCG-Induced Trained Immunity. Cell Rep (2016) 17(10):2562–71. doi: 10.1016/j.celrep.2016.11.011
22. Juarez E, Carranza C, Hernandez-Sanchez F, Leon-Contreras JC, Hernandez-Pando R, Escobedo D, et al. NOD2 Enhances the Innate Response of Alveolar Macrophages to Mycobacterium Tuberculosis in Humans. Eur J Immunol (2012) 42(4):880–9. doi: 10.1002/eji.201142105
23. Netea MG, Kullberg BJ, de Jong DJ, Franke B, Sprong T, Naber TH, et al. NOD2 Mediates Anti-Inflammatory Signals Induced by TLR2 Ligands: Implications for Crohn's Disease. Eur J Immunol (2004) 34(7):2052–9. doi: 10.1002/eji.200425229
24. Kleinnijenhuis J, Quintin J, Preijers F, Joosten LA, Ifrim DC, Saeed S, et al. Bacille Calmette-Guerin Induces NOD2-Dependent Nonspecific Protection From Reinfection via Epigenetic Reprogramming of Monocytes. Proc Natl Acad Sci U S A (2012) 109(43):17537–42. doi: 10.1073/pnas.1202870109
25. Moorlag S, Rodriguez-Rosales YA, Gillard J, Fanucchi S, Theunissen K, Novakovic B, et al. BCG Vaccination Induces Long-Term Functional Reprogramming of Human Neutrophils. Cell Rep (2020) 33(7):108387. doi: 10.1016/j.celrep.2020.108387
26. Arts RJW, Moorlag S, Novakovic B, Li Y, Wang SY, Oosting M, et al. BCG Vaccination Protects Against Experimental Viral Infection in Humans Through the Induction of Cytokines Associated With Trained Immunity. Cell Host Microbe (2018) 23(1):89–100.e5. doi: 10.1016/j.chom.2017.12.010
27. Cirovic B, de Bree LCJ, Groh L, Blok BA, Chan J, van der Velden W, et al. BCG Vaccination in Humans Elicits Trained Immunity via the Hematopoietic Progenitor Compartment. Cell Host Microbe (2020) 28(2):322–34. doi: 10.1016/j.chom.2020.05.014
28. Aass KR, Kastnes MH, Standal T. Molecular Interactions and Functions of IL-32. J Leukoc Biol (2021) 109(1):143–59. doi: 10.1002/JLB.3MR0620-550R
29. Silva MVT, Dos Santos JC, Figueiredo AMB, Teufel LU, Pereira JX, Matos GG, et al. The Role of IL-32 in Bacillus Calmette-Guerin (BCG)-Induced Trained Immunity in Infections Caused by Different Leishmania Spp. Microb Pathog (2021) 158:105088. doi: 10.1016/j.micpath.2021.105088
30. Hajjo R, Tropsha A. A Systems Biology Workflow for Drug and Vaccine Repurposing: Identifying Small-Molecule BCG Mimics to Reduce or Prevent COVID-19 Mortality. Pharm Res (2020) 37(11):212. doi: 10.1007/s11095-020-02930-9
31. Han B, Baruah K, Cox E, Vanrompay D, Bossier P. Structure-Functional Activity Relationship of Beta-Glucans From the Perspective of Immunomodulation: A Mini-Review. Front Immunol (2020) 11:658. doi: 10.3389/fimmu.2020.00658
32. Batbayar S, Lee DH, Kim HW. Immunomodulation of Fungal Beta-Glucan in Host Defense Signaling by Dectin-1. Biomol Ther (Seoul) (2012) 20(5):433–45. doi: 10.4062/biomolther.2012.20.5.433
33. Kim HS, Hong JT, Kim Y, Han SB. Stimulatory Effect of Beta-Glucans on Immune Cells. Immune Netw (2011) 11(4):191–5. doi: 10.4110/in.2011.11.4.191
34. Pedro ARV, Lima T, Frois-Martins R, Leal B, Ramos IC, Martins EG, et al. Dectin-1-Mediated Production of Pro-Inflammatory Cytokines Induced by Yeast Beta-Glucans in Bovine Monocytes. Front Immunol (2021) 12:689879. doi: 10.3389/fimmu.2021.689879
35. Cheng SC, Quintin J, Cramer RA, Shepardson KM, Saeed S, Kumar V, et al. mTOR- and HIF-1alpha-Mediated Aerobic Glycolysis as Metabolic Basis for Trained Immunity. Science (2014) 345(6204):1250684. doi: 10.1126/science.1250684
36. Dominguez-Andres J, Novakovic B, Li Y, Scicluna BP, Gresnigt MS, Arts RJW, et al. The Itaconate Pathway Is a Central Regulatory Node Linking Innate Immune Tolerance and Trained Immunity. Cell Metab (2019) 29(1):211–20 e5. doi: 10.1016/j.cmet.2018.09.003
37. Arts RJ, Novakovic B, Ter Horst R, Carvalho A, Bekkering S, Lachmandas E, et al. Glutaminolysis and Fumarate Accumulation Integrate Immunometabolic and Epigenetic Programs in Trained Immunity. Cell Metab (2016) 24(6):807–19. doi: 10.1016/j.cmet.2016.10.008
38. Bekkering S, Arts RJW, Novakovic B, Kourtzelis I, van der Heijden C, Li Y, et al. Metabolic Induction of Trained Immunity Through the Mevalonate Pathway. Cell (2018) 172(1-2):135–46.e9. doi: 10.1016/j.cell.2017.11.025
39. Moerings BGJ, de Graaff P, Furber M, Witkamp RF, Debets R, Mes JJ, et al. Continuous Exposure to Non-Soluble Beta-Glucans Induces Trained Immunity in M-CSF-Differentiated Macrophages. Front Immunol (2021) 12:672796. doi: 10.3389/fimmu.2021.672796
40. Pinto BGG, Oliveira AER, Singh Y, Jimenez L, Goncalves ANA, Ogava RLT, et al. ACE2 Expression Is Increased in the Lungs of Patients With Comorbidities Associated With Severe COVID-19. J Infect Dis (2020) 222(4):556–63. doi: 10.1093/infdis/jiaa332
41. Dos Santos JC, Barroso de Figueiredo AM, Teodoro Silva MV, Cirovic B, de Bree LCJ, Damen M, et al. Beta-Glucan-Induced Trained Immunity Protects Against Leishmania Braziliensis Infection: A Crucial Role for IL-32. Cell Rep (2019) 28(10):2659–72.e6. doi: 10.1016/j.celrep.2019.08.004
42. Moorlag S, Khan N, Novakovic B, Kaufmann E, Jansen T, van Crevel R, et al. Beta-Glucan Induces Protective Trained Immunity Against Mycobacterium Tuberculosis Infection: A Key Role for IL-1. Cell Rep (2020) 31(7):107634. doi: 10.1016/j.celrep.2020.107634
43. Ikewaki N, Iwasaki M, Kurosawa G, Rao KS, Lakey-Beitia J, Preethy S, et al. Beta-Glucans: Wide-Spectrum Immune-Balancing Food-Supplement-Based Enteric (Beta-WIFE) Vaccine Adjuvant Approach to COVID-19. Hum Vaccin Immunother (2021) 17(8):2808–13. doi: 10.1080/21645515.2021.1880210
44. Cauchois R, Koubi M, Delarbre D, Manet C, Carvelli J, Blasco VB, et al. Early IL-1 Receptor Blockade in Severe Inflammatory Respiratory Failure Complicating COVID-19. Proc Natl Acad Sci U S A (2020) 117(32):18951–3. doi: 10.1073/pnas.2009017117
45. de Rivero Vaccari JC, Dietrich WD, Keane RW, de Rivero Vaccari JP. The Inflammasome in Times of COVID-19. Front Immunol (2020) 11:583373. doi: 10.3389/fimmu.2020.583373
46. O'Neill LA, Golenbock D, Bowie AG. The History of Toll-Like Receptors - Redefining Innate Immunity. Nat Rev Immunol (2013) 13(6):453–60. doi: 10.1038/nri3446
47. Seeley JJ, Ghosh S. Molecular Mechanisms of Innate Memory and Tolerance to LPS. J Leukoc Biol (2017) 101(1):107–19. doi: 10.1189/jlb.3MR0316-118RR
48. Novakovic B, Habibi E, Wang SY, Arts RJW, Davar R, Megchelenbrink W, et al. Beta-Glucan Reverses the Epigenetic State of LPS-Induced Immunological Tolerance. Cell (2016) 167(5):1354–68.e14. doi: 10.1016/j.cell.2016.09.034
49. Foster SL, Hargreaves DC, Medzhitov R. Gene-Specific Control of Inflammation by TLR-Induced Chromatin Modifications. Nature (2007) 447(7147):972–8. doi: 10.1038/nature05836
50. Jeljeli M, Riccio LGC, Doridot L, Chene C, Nicco C, Chouzenoux S, et al. Trained Immunity Modulates Inflammation-Induced Fibrosis. Nat Commun (2019) 10(1):5670. doi: 10.1038/s41467-019-13636-x
51. Saeed S, Quintin J, Kerstens HH, Rao NA, Aghajanirefah A, Matarese F, et al. Epigenetic Programming of Monocyte-to-Macrophage Differentiation and Trained Innate Immunity. Science (2014) 345(6204):1251086. doi: 10.1126/science.1251086
52. Ifrim DC, Quintin J, Joosten LA, Jacobs C, Jansen T, Jacobs L, et al. Trained Immunity or Tolerance: Opposing Functional Programs Induced in Human Monocytes After Engagement of Various Pattern Recognition Receptors. Clin Vaccine Immunol (2014) 21(4):534–45. doi: 10.1128/CVI.00688-13
53. Rodriguez RM, Suarez-Alvarez B, Lopez-Larrea C. Therapeutic Epigenetic Reprogramming of Trained Immunity in Myeloid Cells. Trends Immunol (2019) 40(1):66–80. doi: 10.1016/j.it.2018.11.006
54. Ostuni R, Piccolo V, Barozzi I, Polletti S, Termanini A, Bonifacio S, et al. Latent Enhancers Activated by Stimulation in Differentiated Cells. Cell (2013) 152(1-2):157–71. doi: 10.1016/j.cell.2012.12.018
55. Yoshida K, Maekawa T, Zhu Y, Renard-Guillet C, Chatton B, Inoue K, et al. The Transcription Factor ATF7 Mediates Lipopolysaccharide-Induced Epigenetic Changes in Macrophages Involved in Innate Immunological Memory. Nat Immunol (2015) 16(10):1034–43. doi: 10.1038/ni.3257
56. Tannahill GM, Curtis AM, Adamik J, Palsson-McDermott EM, McGettrick AF, Goel G, et al. Succinate Is an Inflammatory Signal That Induces IL-1beta Through HIF-1alpha. Nature (2013) 496(7444):238–42. doi: 10.1038/nature11986
57. Liu Q, Du J, Yu X, Xu J, Huang F, Li X, et al. miRNA-200c-3p Is Crucial in Acute Respiratory Distress Syndrome. Cell Discov (2017) 3:17021. doi: 10.1038/celldisc.2017.21
58. van der Heijden C, Keating ST, Groh L, Joosten LAB, Netea MG, Riksen NP. Aldosterone Induces Trained Immunity: The Role of Fatty Acid Synthesis. Cardiovasc Res (2020) 116(2):317–28. doi: 10.1093/cvr/cvz137
59. Keating ST, Groh L, Thiem K, Bekkering S, Li Y, Matzaraki V, et al. Rewiring of Glucose Metabolism Defines Trained Immunity Induced by Oxidized Low-Density Lipoprotein. J Mol Med (Berl) (2020) 98(6):819–31. doi: 10.1007/s00109-020-01915-w
60. Groh LA, Ferreira AV, Helder L, van der Heijden C, Novakovic B, van de Westerlo E, et al. oxLDL-Induced Trained Immunity Is Dependent on Mitochondrial Metabolic Reprogramming. Immunometabolism (2021) 3(3):e210025. doi: 10.20900/immunometab20210025
61. Sohrabi Y, Lagache SMM, Schnack L, Godfrey R, Kahles F, Bruemmer D, et al. mTOR-Dependent Oxidative Stress Regulates oxLDL-Induced Trained Innate Immunity in Human Monocytes. Front Immunol (2018) 9:3155. doi: 10.3389/fimmu.2018.03155
62. Arts RJ, Blok BA, van Crevel R, Joosten LA, Aaby P, Benn CS, et al. Vitamin A Induces Inhibitory Histone Methylation Modifications and Down-Regulates Trained Immunity in Human Monocytes. J Leukoc Biol (2015) 98(1):129–36. doi: 10.1189/jlb.6AB0914-416R
63. de Graaf DM, Teufel LU, van de Veerdonk FL, Joosten LAB, Netea MG, Dinarello CA, et al. IL-38 Prevents Induction of Trained Immunity by Inhibition of mTOR Signaling. J Leukoc Biol (2021). doi: 10.1002/JLB.3A0220-143RRR
64. Cavalli G, Tengesdal IW, Gresnigt M, Nemkov T, Arts RJW, Dominguez-Andres J, et al. The Anti-Inflammatory Cytokine Interleukin-37 Is an Inhibitor of Trained Immunity. Cell Rep (2021) 35(1):108955. doi: 10.1016/j.celrep.2021.108955
65. Rother N, Yanginlar C, Lindeboom RGH, Bekkering S, van Leent MMT, Buijsers B, et al. Hydroxychloroquine Inhibits the Trained Innate Immune Response to Interferons. Cell Rep Med (2020) 1(9):100146. doi: 10.1016/j.xcrm.2020.100146
66. Arts RJ, Joosten LA, Netea MG. Immunometabolic Circuits in Trained Immunity. Semin Immunol (2016) 28(5):425–30. doi: 10.1016/j.smim.2016.09.002
67. Mitroulis I, Ruppova K, Wang B, Chen LS, Grzybek M, Grinenko T, et al. Modulation of Myelopoiesis Progenitors Is an Integral Component of Trained Immunity. Cell (2018) 172(1-2):147–61.e12. doi: 10.1016/j.cell.2017.11.034
68. van der Heijden C, Groh L, Keating ST, Kaffa C, Noz MP, Kersten S, et al. Catecholamines Induce Trained Immunity in Monocytes In Vitro and In Vivo. Circ Res (2020) 127(2):269–83. doi: 10.1161/CIRCRESAHA.119.315800
69. Bekkering S, Quintin J, Joosten LA, van der Meer JW, Netea MG, Riksen NP. Oxidized Low-Density Lipoprotein Induces Long-Term Proinflammatory Cytokine Production and Foam Cell Formation via Epigenetic Reprogramming of Monocytes. Arterioscler Thromb Vasc Biol (2014) 34(8):1731–8. doi: 10.1161/ATVBAHA.114.303887
70. Schnack L, Sohrabi Y, Lagache SMM, Kahles F, Bruemmer D, Waltenberger J, et al. Mechanisms of Trained Innate Immunity in oxLDL Primed Human Coronary Smooth Muscle Cells. Front Immunol (2019) 10:13. doi: 10.3389/fimmu.2019.00013
71. Narni-Mancinelli E, Vivier E, Kerdiles YM. The 'T-Cell-Ness' of NK Cells: Unexpected Similarities Between NK Cells and T Cells. Int Immunol (2011) 23(7):427–31. doi: 10.1093/intimm/dxr035
72. Marshall JS, Warrington R, Watson W, Kim HL. An Introduction to Immunology and Immunopathology. Allergy Asthma Clin Immunol (2018) 14(Suppl 2):49. doi: 10.1186/s13223-018-0278-1
73. Gasteiger G, D'Osualdo A, Schubert DA, Weber A, Bruscia EM, Hartl D. Cellular Innate Immunity: An Old Game With New Players. J Innate Immun (2017) 9(2):111–25. doi: 10.1159/000453397
74. Netea MG, Dominguez-Andres J, Barreiro LB, Chavakis T, Divangahi M, Fuchs E, et al. Defining Trained Immunity and its Role in Health and Disease. Nat Rev Immunol (2020) 20(6):375–88. doi: 10.1038/s41577-020-0285-6
75. Askenase MH, Han SJ, Byrd AL, Morais da Fonseca D, Bouladoux N, Wilhelm C, et al. Bone-Marrow-Resident NK Cells Prime Monocytes for Regulatory Function During Infection. Immunity (2015) 42(6):1130–42. doi: 10.1016/j.immuni.2015.05.011
76. Auffray C, Sieweke MH, Geissmann F. Blood Monocytes: Development, Heterogeneity, and Relationship With Dendritic Cells. Annu Rev Immunol (2009) 27:669–92. doi: 10.1146/annurev.immunol.021908.132557
77. Bensinger SJ, Christofk HR. New Aspects of the Warburg Effect in Cancer Cell Biology. Semin Cell Dev Biol (2012) 23(4):352–61. doi: 10.1016/j.semcdb.2012.02.003
78. Gomez-Rial J, Rivero-Calle I, Salas A, Martinon-Torres F. Role of Monocytes/Macrophages in Covid-19 Pathogenesis: Implications for Therapy. Infect Drug Resist (2020) 13:2485–93. doi: 10.2147/IDR.S258639
79. Wang J, Xu Y, Zhang X, Wang S, Peng Z, Guo J, et al. Leptin Correlates With Monocytes Activation and Severe Condition in COVID-19 Patients. J Leukoc Biol (2021) 110(1):9–20. doi: 10.1002/JLB.5HI1020-704R
80. Utrero-Rico A, Gonzalez-Cuadrado C, Chivite-Lacaba M, Cabrera-Marante O, Laguna-Goya R, Almendro-Vazquez P, et al. Alterations in Circulating Monocytes Predict COVID-19 Severity and Include Chromatin Modifications Still Detectable Six Months After Recovery. Biomedicines (2021) 9(9). doi: 10.3390/biomedicines9091253
81. Gibellini L, De Biasi S, Paolini A, Borella R, Boraldi F, Mattioli M, et al. Altered Bioenergetics and Mitochondrial Dysfunction of Monocytes in Patients With COVID-19 Pneumonia. EMBO Mol Med (2020) 12(12):e13001. doi: 10.15252/emmm.202013001
82. Biron CA, Nguyen KB, Pien GC, Cousens LP, Salazar-Mather TP. Natural Killer Cells in Antiviral Defense: Function and Regulation by Innate Cytokines. Annu Rev Immunol (1999) 17:189–220. doi: 10.1146/annurev.immunol.17.1.189
83. Marcus A, Raulet DH. Evidence for Natural Killer Cell Memory. Curr Biol (2013) 23(17):R817–20. doi: 10.1016/j.cub.2013.07.015
84. Sun JC, Beilke JN, Bezman NA, Lanier LL. Homeostatic Proliferation Generates Long-Lived Natural Killer Cells That Respond Against Viral Infection. J Exp Med (2011) 208(2):357–68. doi: 10.1084/jem.20100479
85. Sun JC, Beilke JN, Lanier LL. Adaptive Immune Features of Natural Killer Cells. Nature (2009) 457(7229):557–61. doi: 10.1038/nature07665
86. Poznanski SM, Ashkar AA. What Defines NK Cell Functional Fate: Phenotype or Metabolism? Front Immunol (2019) 10:1414. doi: 10.3389/fimmu.2019.01414
87. Schlums H, Cichocki F, Tesi B, Theorell J, Beziat V, Holmes TD, et al. Cytomegalovirus Infection Drives Adaptive Epigenetic Diversification of NK Cells With Altered Signaling and Effector Function. Immunity (2015) 42(3):443–56. doi: 10.1016/j.immuni.2015.02.008
88. Keppel MP, Yang L, Cooper MA. Murine NK Cell Intrinsic Cytokine-Induced Memory-Like Responses are Maintained Following Homeostatic Proliferation. J Immunol (2013) 190(9):4754–62. doi: 10.4049/jimmunol.1201742
89. Marcais A, Cherfils-Vicini J, Viant C, Degouve S, Viel S, Fenis A, et al. The Metabolic Checkpoint Kinase mTOR Is Essential for IL-15 Signaling During the Development and Activation of NK Cells. Nat Immunol (2014) 15(8):749–57. doi: 10.1038/ni.2936
90. Hou X, Zhou R, Wei H, Sun R, Tian Z. NKG2D-Retinoic Acid Early Inducible-1 Recognition Between Natural Killer Cells and Kupffer Cells in a Novel Murine Natural Killer Cell-Dependent Fulminant Hepatitis. Hepatology (2009) 49(3):940–9. doi: 10.1002/hep.22725
91. Michel T, Hentges F, Zimmer J. Consequences of the Crosstalk Between Monocytes/Macrophages and Natural Killer Cells. Front Immunol (2012) 3:403. doi: 10.3389/fimmu.2012.00403
92. Maucourant C, Filipovic I, Ponzetta A, Aleman S, Cornillet M, Hertwig L, et al. Natural Killer Cell Immunotypes Related to COVID-19 Disease Severity. Sci Immunol (2020) 5(50). doi: 10.1126/sciimmunol.abd6832
93. Kramer B, Knoll R, Bonaguro L, ToVinh M, Raabe J, Astaburuaga-Garcia R, et al. Early IFN-Alpha Signatures and Persistent Dysfunction Are Distinguishing Features of NK Cells in Severe COVID-19. Immunity (2021) 54(11):2650–69. doi: 10.1016/j.immuni.2021.09.002
94. Covian C, Fernandez-Fierro A, Retamal-Diaz A, Diaz FE, Vasquez AE, Lay MK, et al. BCG-Induced Cross-Protection and Development of Trained Immunity: Implication for Vaccine Design. Front Immunol (2019) 10:2806. doi: 10.3389/fimmu.2019.02806
95. Kleinnijenhuis J, van Crevel R, Netea MG. Trained Immunity: Consequences for the Heterologous Effects of BCG Vaccination. Trans R Soc Trop Med Hyg (2015) 109(1):29–35. doi: 10.1093/trstmh/tru168
96. Biering-Sorensen S, Aaby P, Lund N, Monteiro I, Jensen KJ, Eriksen HB, et al. Early BCG-Denmark and Neonatal Mortality Among Infants Weighing <2500 G: A Randomized Controlled Trial. Clin Infect Dis (2017) 65(7):1183–90. doi: 10.1093/cid/cix525
97. Aaby P, Roth A, Ravn H, Napirna BM, Rodrigues A, Lisse IM, et al. Randomized Trial of BCG Vaccination at Birth to Low-Birth-Weight Children: Beneficial Nonspecific Effects in the Neonatal Period? J Infect Dis (2011) 204(2):245–52. doi: 10.1093/infdis/jir240
98. Hollm-Delgado MG, Stuart EA, Black RE. Acute Lower Respiratory Infection Among Bacille Calmette-Guerin (BCG)-Vaccinated Children. Pediatrics (2014) 133(1):e73–81. doi: 10.1542/peds.2013-2218
99. Ten Doesschate T, Moorlag S, van der Vaart TW, Taks E, Debisarun P, Ten Oever J, et al. Two Randomized Controlled Trials of Bacillus Calmette-Guerin Vaccination to Reduce Absenteeism Among Health Care Workers and Hospital Admission by Elderly Persons During the COVID-19 Pandemic: A Structured Summary of the Study Protocols for Two Randomised Controlled Trials. Trials (2020) 21(1):481. doi: 10.1186/s13063-020-04389-w
100. Mammucari C, Rizzuto R. Signaling Pathways in Mitochondrial Dysfunction and Aging. Mech Ageing Dev (2010) 131(7-8):536–43. doi: 10.1016/j.mad.2010.07.003
101. BCG Vaccination to Protect Healthcare Workers Against COVID-19 (BRACE) . Available at: https://clinicaltrials.gov/ct2/show/NCT04327206.
102. COVID-19 BC-GBva . Available at: https://www.who.int/news-room/commentaries/detail/bacille-calmette-guérin-(bcg)-vaccination-and-covid-19.
103. Richter J, Svozil V, Kral V, Rajnohova Dobiasova L, Stiborova I, Vetvicka V. Clinical Trials of Yeast-Derived Beta-(1,3) Glucan in Children: Effects on Innate Immunity. Ann Transl Med (2014) 2(2):15. doi: 10.3978/j.issn.2305-5839.2014.02.01
104. Vetvicka V, Richter J, Svozil V, Rajnohova Dobiasova L, Kral V. Placebo-Driven Clinical Trials of Yeast-Derived Beta-(1-3) Glucan in Children With Chronic Respiratory Problems. Ann Transl Med (2013) 1(3):26. doi: 10.3978/j.issn.2305-5839.2013.07.01
105. Talbott SM, Talbott JA. Baker's Yeast Beta-Glucan Supplement Reduces Upper Respiratory Symptoms and Improves Mood State in Stressed Women. J Am Coll Nutr (2012) 31(4):295–300. doi: 10.1080/07315724.2012.10720441
106. Jesenak M, Majtan J, Rennerova Z, Kyselovic J, Banovcin P, Hrubisko M. Immunomodulatory Effect of Pleuran (Beta-Glucan From Pleurotus Ostreatus) in Children With Recurrent Respiratory Tract Infections. Int Immunopharmacol (2013) 15(2):395–9. doi: 10.1016/j.intimp.2012.11.020
107. Talbott S, Talbott J. Effect of BETA 1, 3/1, 6 GLUCAN on Upper Respiratory Tract Infection Symptoms and Mood State in Marathon Athletes. J Sports Sci Med (2009) 8(4):509–15.
108. Ifrim DC, Quintin J, Meerstein-Kessel L, Plantinga TS, Joosten LA, van der Meer JW, et al. Defective Trained Immunity in Patients With STAT-1-Dependent Chronic Mucocutaneaous Candidiasis. Clin Exp Immunol (2015) 181(3):434–40. doi: 10.1111/cei.12642
109. Yao Y, Jeyanathan M, Haddadi S, Barra NG, Vaseghi-Shanjani M, Damjanovic D, et al. Induction of Autonomous Memory Alveolar Macrophages Requires T Cell Help and Is Critical to Trained Immunity. Cell (2018) 175(6):1634–50 e17. doi: 10.1016/j.cell.2018.09.042
110. Tan EL, Ooi EE, Lin CY, Tan HC, Ling AE, Lim B, et al. Inhibition of SARS Coronavirus Infection In Vitro With Clinically Approved Antiviral Drugs. Emerg Infect Dis (2004) 10(4):581–6. doi: 10.3201/eid1004.030458
111. Chan JF, Yao Y, Yeung ML, Deng W, Bao L, Jia L, et al. Treatment With Lopinavir/Ritonavir or Interferon-Beta1b Improves Outcome of MERS-CoV Infection in a Nonhuman Primate Model of Common Marmoset. J Infect Dis (2015) 212(12):1904–13. doi: 10.1093/infdis/jiv392
112. Sallard E, Lescure FX, Yazdanpanah Y, Mentre F, Peiffer-Smadja N. Type 1 Interferons as a Potential Treatment Against COVID-19. Antiviral Res (2020) 178:104791. doi: 10.1016/j.antiviral.2020.104791
113. Schreiber G. The Role of Type I Interferons in the Pathogenesis and Treatment of COVID-19. Front Immunol (2020) 11:595739. doi: 10.3389/fimmu.2020.595739
114. Monk PD, Marsden RJ, Tear VJ, Brookes J, Batten TN, Mankowski M, et al. Safety and Efficacy of Inhaled Nebulised Interferon Beta-1a (SNG001) for Treatment of SARS-CoV-2 Infection: A Randomised, Double-Blind, Placebo-Controlled, Phase 2 Trial. Lancet Respir Med (2021) 9(2):196–206. doi: 10.1016/S2213-2600(20)30511-7
115. Davoudi-Monfared E, Rahmani H, Khalili H, Hajiabdolbaghi M, Salehi M, Abbasian L, et al. A Randomized Clinical Trial of the Efficacy and Safety of Interferon Beta-1a in Treatment of Severe COVID-19. Antimicrob Agents Chemother (2020) 64(9). doi: 10.1128/AAC.01061-20
116. Rahmani H, Davoudi-Monfared E, Nourian A, Khalili H, Hajizadeh N, Jalalabadi NZ, et al. Interferon Beta-1b in Treatment of Severe COVID-19: A Randomized Clinical Trial. Int Immunopharmacol (2020) 88:106903. doi: 10.1016/j.intimp.2020.106903
117. Nanduri S, Pilishvili T, Derado G, Soe MM, Dollard P, Wu H, et al. Effectiveness of Pfizer-BioNTech and Moderna Vaccines in Preventing SARS-CoV-2 Infection Among Nursing Home Residents Before and During Widespread Circulation of the SARS-CoV-2 B.1.617.2 (Delta) Variant - National Healthcare Safety Network, March 1-August 1, 2021. MMWR Morb Mortal Wkly Rep (2021) 70(34):1163–6. doi: 10.15585/mmwr.mm7034e3
118. Hacisuleyman E, Hale C, Saito Y, Blachere NE, Bergh M, Conlon EG, et al. Vaccine Breakthrough Infections With SARS-CoV-2 Variants. N Engl J Med (2021) 384(23):2212–8. doi: 10.1056/NEJMoa2105000
119. Mlcochova P, Kemp S, Dhar MS, Papa G, Meng B, Ferreira I, et al. SARS-CoV-2 B.1.617.2 Delta Variant Replication and Immune Evasion. Nature (2021) 599:114–9. doi: 10.1038/s41586-021-03944-y
120. Planas D, Veyer D, Baidaliuk A, Staropoli I, Guivel-Benhassine F, Rajah MM, et al. Reduced Sensitivity of SARS-CoV-2 Variant Delta to Antibody Neutralization. Nature (2021) 596(7871):276–80. doi: 10.1038/s41586-021-03777-9
121. Schijns V, Lavelle EC. Prevention and Treatment of COVID-19 Disease by Controlled Modulation of Innate Immunity. Eur J Immunol (2020) 50(7):932–8. doi: 10.1002/eji.202048693
122. Solana R, Pawelec G, Tarazona R. Aging and Innate Immunity. Immunity (2006) 24(5):491–4. doi: 10.1016/j.immuni.2006.05.003
123. Panda A, Arjona A, Sapey E, Bai F, Fikrig E, Montgomery RR, et al. Human Innate Immunosenescence: Causes and Consequences for Immunity in Old Age. Trends Immunol (2009) 30(7):325–33. doi: 10.1016/j.it.2009.05.004
124. Wardhana, Datau EA, Sultana A, Mandang VV, Jim E. The Efficacy of Bacillus Calmette-Guerin Vaccinations for the Prevention of Acute Upper Respiratory Tract Infection in the Elderly. Acta Med Indones (2011) 43(3):185–90.
125. Ray D, Yung R. Immune Senescence, Epigenetics and Autoimmunity. Clin Immunol (2018) 196:59–63. doi: 10.1016/j.clim.2018.04.002
126. Montgomery RR, Shaw AC. Paradoxical Changes in Innate Immunity in Aging: Recent Progress and New Directions. J Leukoc Biol (2015) 98(6):937–43. doi: 10.1189/jlb.5MR0315-104R
127. Kawakami K, Nakamura A, Ishigami A, Goto S, Takahashi R. Age-Related Difference of Site-Specific Histone Modifications in Rat Liver. Biogerontology (2009) 10(4):415–21. doi: 10.1007/s10522-008-9176-0
128. Panda A, Qian F, Mohanty S, van Duin D, Newman FK, Zhang L, et al. Age-Associated Decrease in TLR Function in Primary Human Dendritic Cells Predicts Influenza Vaccine Response. J Immunol (2010) 184(5):2518–27. doi: 10.4049/jimmunol.0901022
129. Franceschi C, Salvioli S, Garagnani P, de Eguileor M, Monti D, Capri M. Immunobiography and the Heterogeneity of Immune Responses in the Elderly: A Focus on Inflammaging and Trained Immunity. Front Immunol (2017) 8:982. doi: 10.3389/fimmu.2017.00982
Keywords: trained immunity, BCG, B-glucan, monocytes, natural killer (NK), COVID-19
Citation: Brueggeman JM, Zhao J, Schank M, Yao ZQ and Moorman JP (2022) Trained Immunity: An Overview and the Impact on COVID-19. Front. Immunol. 13:837524. doi: 10.3389/fimmu.2022.837524
Received: 16 December 2021; Accepted: 28 January 2022;
Published: 17 February 2022.
Edited by:
Shailendra K. Saxena, King George’s Medical University, IndiaReviewed by:
Rima Hajjo, Al-Zaytoonah University of Jordan, JordanBoris Novakovic, Royal Children’s Hospital, Australia
Copyright © 2022 Brueggeman, Zhao, Schank, Yao and Moorman. This is an open-access article distributed under the terms of the Creative Commons Attribution License (CC BY). The use, distribution or reproduction in other forums is permitted, provided the original author(s) and the copyright owner(s) are credited and that the original publication in this journal is cited, in accordance with accepted academic practice. No use, distribution or reproduction is permitted which does not comply with these terms.
*Correspondence: Jonathan P. Moorman, bW9vcm1hbkBldHN1LmVkdQ==