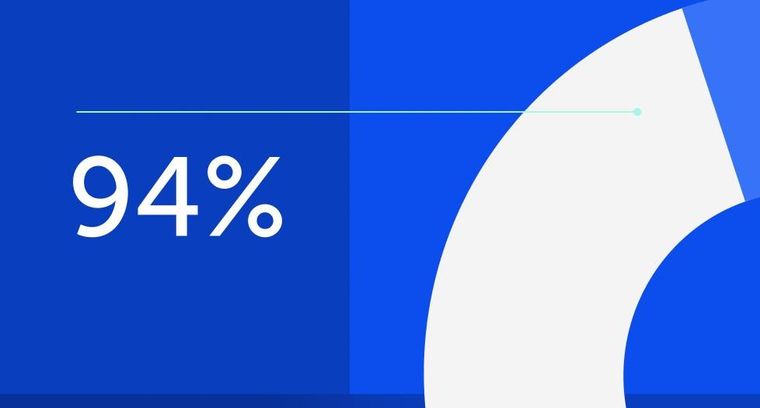
94% of researchers rate our articles as excellent or good
Learn more about the work of our research integrity team to safeguard the quality of each article we publish.
Find out more
REVIEW article
Front. Immunol., 24 March 2022
Sec. Cytokines and Soluble Mediators in Immunity
Volume 13 - 2022 | https://doi.org/10.3389/fimmu.2022.833355
This article is part of the Research TopicImmunometabolic Mechanisms Underlying the Severity of COVID-19View all 25 articles
SARS-CoV-2, which initially emerged in November of 2019, wreaked havoc across the globe by leading to clinical acute respiratory distress syndrome and continues to evade current therapies today due to mutating strains. Diabetes mellitus is considered an important risk factor for progression to severe COVID disease and death, therefore additional research is warranted in this group. Individuals with diabetes at baseline have an underlying inflammatory state with elevated levels of pro-inflammatory cytokines and lower levels of anti-inflammatory cytokines, both of which cause these individuals to have higher susceptibility to SARS- CoV2 infection. The detrimental effects of SARS-CoV-2 has been attributed to its ability to induce a vast cell mediated immune response leading to a surge in the levels of pro-inflammatory cytokines. This paper will be exploring the underlying mechanisms and pathophysiology in individuals with diabetes and insulin resistance making them more prone to have worse outcomes after SARS- CoV2 infection, and to propose an adjunctive therapy to help combat the cytokine surge seen in COVID-19. It will also look at the immunomodulatory effects of glutathione, an antioxidant shown to reduce immune dysregulation in other diseases; Vitamin D, which has been shown to prevent COVID-19 patients from requiring more intensive care time possibly due to its ability to decrease the expression of certain pro-inflammatory cytokines; and steroids, which have been used as immune modulators despite their ability to exacerbate hyperglycemia.
With approximately 251 million confirmed cases, over 5 million deaths documented and only about 3.15 billion of the 7.7 billion total global population fully vaccinated as of November, 2021 (1). The novel strain of coronavirus, which originally appeared in Wuhan, in the People’s Republic of China at the end of 2019 and was found to precipitate acute respiratory distress syndrome (ARDS) is still wreaking havoc across the globe (2).
The genetic similarity of this novel strain to SARS-CoV-1, formerly known as SARS-CoV, and Middle East Respiratory Syndrome Coronavirus (MERS-CoV) lead to it being named Severe Acute Respiratory Syndrome Coronavirus 2 (SARS-CoV-2). SARS-CoV-2 is an enveloped, positive-stranded RNA virus which belongs to a large family of Coronaviridae viruses with noxious capability across many species (3). Prior to COVID-19, Coronaviridae family was well known for being the most common cause of common cold.
Evolution and mutations are an essential part of the viral life cycle of SARS-CoV-2; therefore, the risk of a more virulent strain or decreased vaccine efficacy is a potential outcome. Since the emergence of SARS-CoV-2 there have been a few different variant strains, some more prevalent in certain regions. The World Health Organization (WHO) has separated these variants into four different classes based on their potential for harm, those deemed to be a lower risk were assigned to the class: variant being monitored (VBM), next is variant of interest (VOI), followed by variant of concern (VOC) and finally variant of high consequences (VOHC). The designation of a variant to a certain class differs from country to county (4). Currently, WHO has designated five of the SARS-CoV-2 variants as VOC, these include Alpha, Beta, Gamma, Delta and the newest variant Omicron (5). For a variant to be assigned to VOC this conveys that this strain has shown to be highly transmissible, has increased morbidity and mortality, has shown to have a reduction in neutralization by antibodies, has decreased vaccine or treatment effectiveness as well as interference with diagnostic testing (6). The precise impression is yet to be determined for variant Omicron as this strain materialized very recently and research is ongoing. All of the VOCs have mutations in the spike protein, with variant Alpha having mutations in receptor binding domain along with spontaneous spike mutation and mutation near S1/S2 furin cleavage site, Beta variant was found to have mutations in receptor binding domain of spike protein and Gamma and Delta variants both demonstrated numerous spike protein mutations. As more information becomes available, Omicron was found to have spike protein mutations- a protein essential for viral entry and hence has been a target for vaccine development (7–9).
Over the past year there have been many different mechanisms of virulence, pathophysiology and mechanism of action proposed, each changing as newer information has become available about this novel strain. The mode of infection is via the viral spike S-glycoprotein binding to the ACE2 (Figure 1) on the epithelial surface to mediate viral entry (13). This process is supported by transmembrane protease serine 2 (TMPRSS2) (14), which in COVID-19 leads to a robust immune response resulting in acute inflammation with increased levels of inflammatory mediators including cytokines and chemokines (15). This activation of the immune system provides us with the insight as to why individuals who are immunocompromised at baseline with other comorbidities such as diabetes tend to have a more severe disease progression. Multiple studies have shown that individuals with diabetes are prone to have cytokine dysregulation at baseline with increased levels of pro-inflammatory cytokines and decreased levels of anti-inflammatory cytokines (16).
The difficulties attributed to COVID-19 response is multi-factorial, some of which include new emerging strains, lower socioeconomic status, lack of resources and different co-morbidities with underlying augmented baseline inflammation and immune dysregulation as seen in diabetes. This paper will be divulging into the mechanism and pathophysiology of why individuals with diabetes and insulin resistance are more prone to have worse outcomes after COVID-19 and propose an adjunctive therapy to help combat the cytokine storm as seen in COVID-19 to help alleviate the underlying inflammation (2, 16).
SARS-CoV-2 are RNA viruses which spread via bodily fluid exposure. Viral attachment and entry into host cells happens using the spike protein which sits on the virus’ membrane. Spike protein is composed of two subunits S1 which promotes infectivity via receptor binding and S2 which increase entry via endocytosis. S1 subunit which infects via receptor binding attaches to the host cell via angiotensin-converting enzyme-2 (ACE2) receptors (Figure 1). Here the entire virus enters the cell by being encased in an endosome. Once in the cell, this membrane is then broken-down piece-by-piece by the protease cathepsin. Eventually, this will expose the viral RNA to the cytoplasm. Neuropilin-1 is another host cell receptor for SARS-CoV-2 and facilitates infectivity, the exact mechanism is still debatable, though it is thought to allow for S1 binding as well (16–22).
The second mode of entry requires spike protein cleavage at the S1/S2 site by proteases which includes transmembrane protease serine 2 (TMPRSS2) and cathepsin L (16, 17). This allows for fusion of the S2 component with the cell’s plasma membrane leading to an opening or fusion pore, facilitating the RNA’s entry into cellular cytoplasm (17, 21, 22). Once in the cytoplasm of the cell the RNA genome gets translated into viral proteins. These will eventually form into viruses and be released from the cell (23). Figure 1 demonstrates the steps for entry requiring spike protein cleavage.
As more information about this novel virus is elucidated, another protease’s role in promoting infectivity is becoming more significant. Furin is a proprotein convertase (PC) that has a role in cleaving proteins. It is generally located on the trans-Golgi network and has been found to also cleave at the S1/S2 component of the spike protein. It is thought that this will enhance viral maturity and processing once the virus is already in the cell (23, 24). The spike protein also has a unique furin cleavage site at the S1/S2 junction which facilitates fusion of S2 to the cellular receptor neuropilin-1 (NRP1). This amplifies transmission of SARS-CoV-2 (25). Studies show that patients with diabetes and metabolic syndromes have higher plasma furin levels (26).
It has further been discussed that this elevation in furin may increase the mortality of COVID-19 patients. The augmented furin levels raises susceptibility of host cells, hence boosting viral load. We predict that patients may have worse outcomes secondary to higher viral infiltration of organs with higher furin concentration such as the lungs, kidneys, and atherosclerotic plaques. Respectively, many fatal outcomes from COVID-19 seem to come from acute respiratory distress syndrome, acute renal failure, and acute cardiac injury with heart failure (27).
Diabetes mellitus is a disorder affecting glucose metabolism and can be separated into two types. Type one involves insulin deficiency while the more common type two involves insulin resistance (28, 29).
Insulin resistance is a decreased responsiveness of tissues and cells to circulating insulin. This leads to both increased circulating levels of insulin, as well as higher levels of circulating glucose (30).
Insulin resistance is a metabolic disorder that is not easily explained by a single metabolic pathway. Chronic exposure to energy surplus is postulated to lead to insulin resistance. This usually induces ectopic lipid accumulation in the liver, muscles and adipose tissues leading to reduced glucose uptake by skeletal muscle and decreased glycogen synthesis in the liver (28). Ectopic lipid accumulation is postulated to be one of the main causes of development of insulin resistance. Early studies by Randle suggest that fatty acids impair insulin-mediated glucose uptake by inhibition of pyruvate dehydrogenase, leading to decreased glucose oxidation, which is necessary for glucose metabolism (29).
While plasma lipids play an important role in insulin resistance, other studies have demonstrated that elevated accumulation of intra-myocellular lipid, especially diacylglycerol (DAG) alters the cellular matrix by activating a de-novo protein kinase C (PKC). This impairs insulin signaling by increasing protein phosphatase 2A (PP2A) and sequestrating serine/threonine kinase (Akt2); both of which play an important role in the function of the insulin receptor and it’s signaling pathway as well as in glucose uptake (31–33).
Ceramides are by-products of fatty acids and are believed to play an important role in insulin resistance. As fatty acids enter the cell, they get esterified with sphingosine creating ceramides. These ceramides are membrane bound lipids, found in greater quantities in the muscles and liver cells (34). One-way chronic inflammation can be explained in patients suffering from insulin resistance is by the activation of the innate immune signaling pathway triggered by toll-like receptor 4 (TLR4). TLR4 are an upstream signal required for ceramide biosynthesis. Ceramides are associated with lipid-induced inflammatory pathways and the development of proinflammatory components such as kinase IKβ (35). Patients in septic shock who are suffering from obesity and/or diabetes exhibited an increase in insulin resistance and an elevation in cytokines, this would suggest that insulin resistance plays a role in cytokine production and its downstream complications (36, 37). In turn, cytokines can feed back into this system causing insulin resistance. For example,Tumor necrosis alpha (TNF-α) directly causes insulin resistance through insulin receptor substrate-1 (IRS-1) and insulin receptor substrate-2 (IRS-2 via promoting serine/threonine phosphorylation of the substrates. This leads to an inhibition of the insulin receptor and causes insulin resistance (38).
Diabetes also imparts damage to the body by increasing oxidative stress in DNA, proteins and lipids, which leads to an enhanced cellular response and activation of PKC, transcription factor nuclear factor kappa B (NF-κB) and JNK stress associated kinase (39). Hyperglycemia also leads to microvascular damage, resembling poorly perfused tissue. This in part is referred to as pseudohypoxia which impairs oxidation of NADH to NAD+. Oxidative stress increases reactive oxygen species (ROS) such as superoxide anion and possibly nitric oxide formation and decreases oxidative defenses leading to tissue damage (40).
While there are multiple pathways which lead to oxidative stress such as glucose oxidation, non-enzymatic glycation, and polyol pathways. The polyol pathway appears to have the greatest effect on oxidative stress in an individual (41). The polyol pathway consists of two enzymes, aldose reductase (AR) and sorbitol dehydrogenase (SDH), that aid in the formation of glucose to sorbitol and sorbitol to fructose (42). Therefore, hyperglycemia leads to tissue damage via two pathways, osmotic damage via sorbitol accumulation and oxidative stress by means of oversaturating the polyol pathway and ROS accumulation. The first reaction requires a NADPH for the conversion of glucose into fructose by AR. This in turn, depletes NADPH which is needed by glutathione reductase to generate glutathione (GSH), leading to a depletion of GSH. The second reaction is the conversion of sorbitol to fructose by SDH which causes oxidative stress by increasing the NADH cofactors, a substrate for ROS (43). These are some of the main pathways and reactions believed to cause tissue damage, increase pro inflammatory molecules which eventually leads to insulin resistance.
The pathophysiology and treatment of SARS-CoV-2 has been inexorably linked with our immune system. Severe SARS-CoV-2 infections appear to be largely related to inappropriate and excessive immune system response (15). Meanwhile, humoral and cell mediated immune responses appear to be the cornerstone of the treatment and prevention of SARS-CoV-2. Many attempts at therapeutics have relied on convalescent plasma or monoclonal antibodies, supporting, and amplifying our body’s own natural immune system. Likely more important, highly effective COVID-19 vaccines rely on our bodies own humoral immune system to be effective.
Diabetic patients, making up over ten percent of the population in the United States, and known to have markedly increased mortality rates due to SAR-CoV-2 infections are a group of significant interest regarding COVID-19 infections (4, 44). A meta-analysis concluded that blood glucose level was elevated in severe SARS-CoV-2 when compared to milder disease, and while hemoglobin A1C (HbA1C) was slightly higher in severe disease, it was not significant. This was again seen in a retrospective study by Patel and team which showed that in diabetic patients hospitalized with SARS-CoV-2 there was no significant association between HbA1C level and adverse clinical outcomes (45, 46). Despite there being no significant correlation between HbA1C and adverse outcomes early in the pandemic, a recent meta-analysis by Zheng et al. concluded that uncontrolled hyperglycemia for a prolonged period was associated with adverse prognosis. The mechanistic effect of hyperglycemia on inflammation and cytokine surge has been established in many studies (47).
Glucose reacts non-enzymatically with proteins, forming compounds which undergo a series of irreversible changes over time to become advanced glycation end-products (AGEs). In studies AGEs significantly increase the mRNA expression and production of TNF- α in endothelial cells cultured and grown from the human umbilical vein. TNF- α can then induce the production of inflammatory cytokines such as interleukin-6 (IL-6), interleukin-8 (IL-8), and granulocyte-macrophage colony-stimulating factor (GM-CSF) from endothelial cells (48).
Diabetes, poor glycemic control, and inability to produce adequate insulin are related to several immunosuppressive effects in relation to B and T cell activity, and lead to the disease being considered an immunosuppressive condition. These effects are likely the root of the markedly increased mortality seen in this population, and worth further investigating (44).
Cell-mediated response is assisted and amplified by the activity of CD8+ T cells, leading to the cascade of cytokines that rally other components of the cell-mediated immune response. Prior research has shown that this response is blunted in diabetics (46, 49). For example, research has shown that viral infection can cause states of insulin resistance in skeletal muscle and other sites in the body. This leads to a hyperglycemia and resulting compensatory hyperinsulinemic state. These increased levels of insulin have been shown to be stimulating factors for CD8+ T cells. This hyperinsulinemic response has been shown to be blunted in patients with Type II diabetes (T2DM), leading to decreased CD8+ T cell activity and a state of hyperglycemia in the presence of infection (50, 51). There are also associated effects of uncontrolled hyperglycemia, including glycosylation components of the immune system including IgG and IgM antibodies, as well as inappropriate activation of CD4+, CD8+, and cytotoxic T cells, and decreased production of inflammatory cytokines in immune responses (52). A study comparing patients with impaired glucose tolerance (IGT) to non-diabetic subjects showed that IL-6, C-reactive protein (CRP), serum amyloid A protein, and fibrinogen were more elevated in patients with T2DM (53). Another study looked at type II diabetics who did and did not have an infection present compared to non-diabetic patients with and without infection. Both cohorts of diabetics had higher levels of CRP than the control group of non-diabetic patients with no infection (54, 55).
SARS-CoV-2 infections present somewhat of a paradox regarding cell-mediated immune response. While necessary to control the viral replication and limit infection, it appears most of the serious adverse effects of the infection result from an overactive cytokine cascade. This should raise the question of why immunosuppression is not beneficial in these patients. A similar issue exists regarding steroid use as a therapeutic agent, having been found to have both beneficial and harmful effects in different patient populations (56). Likely the easiest way to parse out the complicated relationships between these competing effects is to look at the result. Diabetics are found to have a higher rate of clinically significant infection, and nearly 3 times the mortality rate from infections (44). This suggests that while there are likely both protective and deleterious effects of the blunted immune response in diabetics- overall it is a detrimental process. The differential effects of glucose on the various immune cell types can be gleaned in Table 1.
Figure 1 SARS-CoV2 infection of host cells (10–12): 1. Attachment of S1(green) subunit of the SARS-CoV-2 (pink) spike protein to the ACE 2 (Orange) receptor on the host cell plasma membrane (blue). 2. Cleavage of S1/S2 (green/purple) complex by either TMPRSS2, or Furin (red) 3. Fusion of the S2 spike protein component with the host cell plasma membrane.
Humoral immunity, and a sustained antibody response to SARS-CoV-2 infections, whether by natural infection or vaccination appear to be the definitive method of control of the pandemic. The question of sustained immune response will likely be the deciding factor of whether COVID-19 becomes a seasonal infection, or eventually sputters out as the number of potential infected dwindles. One of the current areas of interest is how long the antibody response to COVID-19 infections lasts from a natural infection, how long it provides effective resistance, and how the response from different vaccines compares to this (60). Diabetics are again a group of interest because of impaired B-cell response. Clinically, diabetics have shown impaired humoral immune responses to both infections and vaccinations in the past (significant examples being influenza and hepatitis B vaccines) (61). The effects of diabetes on humoral immunity are complex and interconnected. Factors limiting cell mediated immune responses mentioned above contribute to a less robust activation of the humoral immune system, while glycosylation of antibodies directly affects the effectiveness of these responses (52, 61). All of this inflammatory dysregulation present in diabetics is a recipe for disaster if mixed with COVID-19. Both disease states have many similar pro-inflammatory mechanisms that can potentiate a very sick patient (50). Despite this, clinical trials have shown mixed, but promising, results of immune response to COVID-19 (measured in antibody titers) that appear to be appropriate and sustained (61, 62). Giving some hope that this population will have an effective response to vaccination programs going forward. Table 2 Summarizes the main factors of diabetes that contribute to SARS-CoV-2 pathophysiology as discussed in this paper.
Table 2 Summary of the main factors of diabetes that contribute to SARS-CoV-2 pathophysiology as discussed in this paper.
Glutathione (GSH) is an endogenous compound which continuously cycles between oxidative states in order to regulate the body’s oxidation status (63). Reduced GSH is oxidized to form Glutathione disulfide (GSSG) upon reduction of reactive oxygen species (ROS)-scavenger enzymes (64). Thus, GSH ultimately neutralizes oxidative compounds via a series of enzymatic reactions, combating the oxidative stress induced pro-inflammatory cytokine expression classically seen in diabetic patients (63, 64). Certain agents can upregulate or downregulate glutathione activity. For instance, N-acetyl-cysteine (NAC) and glutathione ether ester (GEE) increase intracellular GSH levels whereas Diamide and Buthionine Sulfoxime (BSO) deplete intracellular GSH (64–66). In lymphoma B cells, BSO treatment and consequent GSH depletion increased ROS production, ultimately inducing apoptosis (65).
GSH affects the expression of cytokines, this has been established by many studies which show an imbalance between pro and anti-inflammatory cytokines in disease states such as diabetes and human immunodeficiency virus (67). In a study analyzing heart failure among rabbits, those treated with NAC showed greater levels of GSH and consequently decreased expression of the transcription factor NF-κB when compared to controls. NF-κB activity upregulates expression of IL-6, interleukin-1(IL-1), and TNF-α (66). Furthermore, lower total GSH levels were correlated with increased disease severity and lung damage, highlighting glutathione’s potential role in COVID-19 management (68). Essentially, GSH reduces oxidative stress and the expression of cytokines pertinent to the diabetes related exacerbation of SARS-CoV-2 pathogenesis and thus could be a potential therapeutic target for reducing disease severity. Several clinical trials, as listed in Table 3, investigate the therapeutic potential of glutathione in COVID-19 pathogenesis; however, diabetic-specific trials have not yet been commenced (69–73).
Table 3 Current glutathione-relevant clinical trials in COVID-19 patients and their corresponding clinicaltrials.gov identification numbers, treatments, measured parameters, and clinical phases.
In addition to GSH, Vitamin D can also be implicated in the therapeutic barrage against COVID-19. In a randomized clinical study, patients with COVID-19 who were administered 25-Hydroxyvitamin-D3 (calcifediol) showed a significantly lower need for Intensive care unit (ICU) treatment when compared to non-administered COVID-19 patients (74). In another randomized clinical trial consisting of 130 COVID-19 patients with hypovitaminosis D, high dose (60,000 IU) daily oral supplementation of Vitamin D significantly increased systemic Vitamin D levels and decreased the levels of the following inflammatory markers: C-reactive peptide (CRP), IL-6, and Neutrophil/lymphocyte ratio (75). Information on clinical trials involving Vitamin D supplementation in COVID-19 patients can be gleaned from Table 4 (76, 77). No clinical trials involving vitamin D supplementation in diabetic COVID-19 patients have been undertaken.
Table 4 Current Vitamin D-relevant clinical trials in COVID-19 patients and their corresponding clinicaltrials.gov identification numbers, treatments, measured parameters, and clinical phases.
Both 25-Hydroxyvitamin-D3 and 1,25-Dihydroxyvitamin-D3 (calcitriol) inhibit the expression of TNF-α, IL-6, Monocyte chemoattractant protein-1(MCP-1) and NF-κB in lipopolysaccharide-stimulated and unstimulated macrophages (78). They also significantly reduce the expression of IL-6, MCP-1, and IL-8 in a dose-dependent manner among human periodontal ligament fibroblasts as well as the expression of MCP-1 and IL-8 in primary human periodontal ligament cells (79). These anti-inflammatory outcomes of vitamin D administration may be explained by its effects on TNF-α. In vascular smooth muscle cells (VSMCs) TNF-α activates NF-κB via TNF receptor binding, eventually inducing the expression of pro-inflammatory genes such as MCP-1 and Interleukin -1 beta (IL-1β) (80). However, administration of both calcitriol and paricalcitol attenuated TNF-α-dependent NF-κB binding to DNA, decreasing nuclear translocation of NF-κB and consequent NF-κB-induced gene transcription (81). Thus, vitamin D inhibits the NF-κB-induced transcription of cytokines upregulated during hyperglycemia and the cytokine storm seen in severe SARS-CoV-2 infection. Vitamin D may also alleviate heightened oxidative stress characteristic of hyperglycemia, subsequently alleviating SARS-CoV-2’s pathogenesis in diabetic patients. According to a meta-analysis and systematic review by Sepidarkish et al., Vitamin D supplementation increases serum levels of glutathione (GSH) and significantly depletes malondialdehyde (MDA), indicating decreased oxidative stress (82). Furthermore, treatment with Vitamin D increases glucose tolerance and decreases insulin resistance. The proposed mechanism involves a direct stimulation of insulin-producing pancreatic cells via their vitamin D receptors and an indirect stimulation via normalization of systemic calcium levels, allowing appropriate calcium-induced insulin secretion (83). Despite there being no clinical trials demonstrating that low Vitamin D levels lead to higher SARS-CoV-2 susceptibility, there have been numerous studies showing that the pre-infection Vitamin D deficiency is associated with increased morbidity and mortality and hence lower Vitamin D levels are likely a good predictor for disease progression (84).
A study by Demir et al. validates the impact of Vitamin D on inflammation. This study showed that as Vitamin D levels increased, the risk of SARS-CoV-2 infections decreased along with its downstream effects. It proved that if an individual is already positive for COVID-19, as their Vitamin D levels increased, the levels of inflammatory markers such as D-Dimer and C-reactive protein decreased along with the extent of lung injury (85).
Numerous studies have displayed that having baseline levels of Vitamin D deficiency is a common risk factor for multiple inflammatory diseases including obesity, diabetes, insulin resistance and atherosclerosis (86, 87).
A study conducted by Jain and team looked at the link between Vitamin D deficiency and a lower GSH level, where they showed that supplementation using a combination of Vitamin D and L-cysteine significantly raised GSH levels while lowering oxidative stress, TNF-α level, and insulin resistance levels in blood (86).
Thus, Vitamin D supplementation not only reduces oxidative stress, but it also augments GSH and contributes to improving diabetic hyperglycemia and its associated inflammation.
Corticosteroids which include glucocorticoids and mineralocorticoids are a class of stress hormones released in a circadian manner by the adrenal cortex. It plays a role in regulating physiologic processes essential for life (88). Today corticosteroids are at the forefront of immunosuppressive and anti-inflammatory therapy and hence are a critical part in the fight to combat the detrimental impact of SARS-CoV-2 (89). The release of glucocorticoids is regulated by hypothalamic-pituitary-adrenal (HPA) axis (88, 90). The downstream effects of glucocorticoids are mediated via two major pathways; first by its direct effect on gene transcription via glucocorticoid responsive elements and transcription factors, both of which inhibit cytokine synthesis, obstruct promoter sites of pro-inflammatory cytokines and impede nuclear factor kappa B. Nuclear factor kappa B which acts to activate immunoregulatory genes in inflammation, therefore when inhibited by steroids, leading to a decrease in inflammation (91, 92). Secondly corticosteroids modulate cytokine function via post translation events by affecting the stability of messenger RNAs which encodes for certain cytokines (93, 94).
Corticosteroids are immunosuppressants and hence will function to decrease the cytokine production, but concurrently would also hinder the defensive properties of the immune system by blocking T-cells and decreasing the ability of B-cells to make antibodies. They also subdue the function of macrophages and natural killer cells, hence, impeding the body from fighting against superimposed infections (95). A study by Lee et al. showed that early corticosteroid use was associated with higher continuous plasma viral load (96). The recovery trial, one of the more prominent studies supporting corticosteroids for SARS-CoV-2 concluded that dexamethasone for 10 days reduced 28-day mortality in patients receiving some form of respiratory support but found no benefits and possibly harm in patients not requiring oxygen supplementation. This was also seen documented in the meta-analysis by WHO prior to their recommendation for corticosteroid use along with many other studies, all of which recommended corticosteroids for severely ill patients only (10, 56, 95, 97, 98).
As SARS-CoV-2 lead to devastation across the globe, the impact of different co-morbidities became more apparent, and the different consequences became evident. Diabetes is one of the co-morbidities that has been shown to be an independent risk factor for infections and for severe disease progression of Covid-19 (11, 12). There have been many emerging reports and studies showing that there is a high rate of mucormycosis infection in patients with SARS-CoV-2 in India. Hyperglycemia, becoming worse in the setting of corticosteroid supplementation, provides an ideal environment for fungal infections (11). In a systemic review of cases looking at 101 patients with mucormycosis it was found that 80% were diabetic patients and 76.3% had received corticosteroids, as there was no specific documentation of the prevalence of infections in diabetic and non-diabetic patients’ definitive conclusions could not be drawn (11).
As noted above, steroids are undoubtfully beneficial and most definitely contributed to keeping our mortality rate lower, but are not without risk, and these risks are compounded when coupled with certain co-morbidities such as diabetes. Therefore, it is crucial that the usage of corticosteroids is done cautiously in certain population, such as the immunocompromised community.
Diabetes is currently one of the top ten causes of death worldwide, the number of individuals with diabetes rose from 108 million in 1980 to 422 million in 2014 and in 2019, an estimated 1.5 million deaths were directly caused by diabetes (1). The underlying immune system dysregulation became an imminent point of interest as the COVID-19 pandemic swept through the globe. SARS-CoV-2 by itself had exhausted medical resources as there was a rush to analyze the pathogenesis of the novel virus. Nowadays, there are multiple vaccines available but only a fraction of the world’s population has been vaccinated. As we see newer strains emerging it begs the question if the current vaccines provide appropriate protection from these evolving viruses.
In the executive summary published by the Centers for Disease Control and Prevention (CDC), reviewing the meta-analysis of clinical trials, it was suggested that vaccination is effective in preventing severe infection and provided protection from variants. This analysis also showed that various immune markers including neutralizing antibodies, including CD 4+ and CD8+ T cells, have a half-life, and see a decline after a period of time. Memory B cells on the other hand was elevated after an infection/vaccination and this level was sustained (99, 100).
CDC’s weekly report showed that vaccine effectiveness was significantly reduced in patients 4-6 months after vaccination, showing increased morbidity and mortality among nursing home residents who had already received the full vaccination series when the Delta variant predominated, hence reenforcing the need for boosters (101).
Here we presented GSH, an antioxidant that has been shown to normalize pro and anti-inflammatory cytokines in other disease states such as diabetes and HIV, as a potential therapeutic pathway for COVID-19 infections. We also discussed the risks and benefits of corticosteroids and recommend that physicians use them sparingly when dealing with patients who do not require respiratory support. Immunomodulation with steroids, GSH, and Vitamin D have shown beneficial effects in prior studies related to similar inflammatory conditions and warrant further research in the pathophysiology and treatment of COVID-19.
VV and MS conceived this work. All the authors contributed to draft of the manuscript. OB, MN, and JR contributed equally to this manuscript. All authors contributed to the article and approved the submitted version.
The authors declare that the research was conducted in the absence of any commercial or financial relationships that could be construed as a potential conflict of interest.
All claims expressed in this article are solely those of the authors and do not necessarily represent those of their affiliated organizations, or those of the publisher, the editors and the reviewers. Any product that may be evaluated in this article, or claim that may be made by its manufacturer, is not guaranteed or endorsed by the publisher.
1. World Health Organization. Coronavirus Disease (COVID-19) Pandemic. (2021). Available at: https://www.who.int/emergencies/diseases/novel-coronavirus-2019. Accessed December 1, 2021.
2. Guloyan V, Oganesian B, Baghdasaryan N, Yeh C, Singh M, Guilford F, et al. Glutathione Supplementation as an Adjunctive Therapy in COVID-19. Antioxid (Basel) (2020) 9(10):1–2. doi: 10.3390/antiox9100914
3. Parasher A. COVID-19: Current Understanding of its Pathophysiology, Clinical Presentation and Treatment. Postgrad Med J May (1147) 2021:97. doi: 10.1136/postgradmedj-2020-138577
4. Centers for Disease Control and Prevention. National Diabetes Statistics Report. In: Estimates of Diabetes and its Burden in the United States (2020). Available at: https://www.cdc.gov/diabetes/data/statistics-report/index.html. Accessed November 28, 2021.
5. WHO. Tracking SARS-CoV2 Variants. (2021). Available at: https://www.who.int/en/activities/tracking-SARS-CoV-2-variants/. Accessed December 1, 2021
6. National Center for Immunization and Respiratory Diseases (NCIRD) DoVD. SARS-CoV-2 Variant Classifications and Definitions. In: Centers for Disease Control and Prevention (2021). Available at: https://www.cdc.gov/coronavirus/2019-ncov/variants/variant-classifications.html. Accessed December 2, 2021.
7. Lauring AS, Hodcroft EB. Genetic Variants of SARS-CoV-2-What Do They Mean? JAMA (2021) 325(6):529–31. doi: 10.1001/jama.2020.27124
8. Tao K, Tzou PL, Nouhin J, Gupta RK, de Oliveira T, Kosakovsky Pond SL, et al. The Biological and Clinical Significance of Emerging SARS-CoV-2 Variants. Nat Rev Genet (2021) 22(12):757–73. doi: 10.1038/s41576-021-00408-x
9. Deb P, Molla MA, Saif-Ur-Rahman KM, Chandra Das M, Das D. A Review of Epidemiology, Clinical Features and Disease Course, Transmission Dynamics, and Neutralization Efficacy of SARS-CoV-2 Variants. Egyptian J Bronchol (2021) 49:2–3. doi: 10.1186/s43168-021-00090-x
10. Cano EJ, Fonseca Fuentes X, Corsini Campioli C, O’Horo JC, Saleh OA, Odeyemi Y, et al. Impact of Corticosteroids in Coronavirus Disease 2019 Outcomes: Systematic Review and Meta-Analysis. Chest (2021) 159(3):1019–40. doi: 10.1016/j.chest.2020.10.054
11. Singh AK. Mucormycosis in COVID-19: A Systematic Review of Cases Reported Worldwide and in India. In: Ritu Singh SRJ, Anoop M, editors. Science Direct: Diabetes & Metabolic Syndrome: Clinical Research & Reviews (2021).
12. Erener S. Diabetes, Infection Risk and COVID-19. Mol Metab (2020) 39:101044. doi: 10.1016/j.molmet.2020.101044
13. Osuchowski MF, Winkler MS, Skirecki T, Cajander S, Shankar-Hari M, Lanchmann G, et al. The COVID-19 Puzzle: Deciphering Pathophysiology and Phenotypes of a New Disease Entity. Lancet Respir Med (2021) 9(6):622–42. doi: 10.1016/S2213-2600(21)00218-6
14. Johnson BA, Xie X, Kalveram B, Lokugamage KG, Muruato A, Zou J, et al. Furin Cleavage Site Is Key to SARS-CoV-2 Pathogenesis. bioRxiv (2020) 14. doi: 10.1101/2020.08.26.268854
15. Hojyo S, Uchida M, Tanaka K, Hasebe R, Tanaka Y, Murukami M, et al. How COVID-19 Induces Cytokine Storm With High Mortality. Inflammation Regen (2020) 40:37. doi: 10.1186/s41232-020-00146-3
16. Lagman M, Ly J, Saing T, Singh MK, Vera Tuleda E, Morris D, et al. Investigating the Causes for Decreased Levels of Glutathione in Individuals With Type II Diabetes. PloS One (2015) 10(3):e0118436. doi: 10.1371/journal.pone.0118436
17. Chen J, Wang R, Wang M, Wei GW. Mutations Strengthened SARS-CoV-2 Infectivity. J Mol Biol (2020) 432(19):5212–26. doi: 10.1016/j.jmb.2020.07.009
18. Tang T, Bidon M, Jaimes JA, Whittaker GR, Daniel S. Coronavirus Membrane Fusion Mechanism Offers a Potential Target for Antiviral Development. Antiviral Res (2020) 178:104792. doi: 10.1016/j.antiviral.2020.104792
19. Shang J, Wan Y, Luo C, Ye G, Geng Q, Auerback A, et al. Cell Entry Mechanisms of SARS-CoV-2. Proc Natl Acad Sci U S A (2020) 117(21):11727–34. doi: 10.1073/pnas.2003138117
20. He J, Tao H, Yan Y, Huang SY, Xiao Y. Molecular Mechanism of Evolution and Human Infection With SARS-CoV-2. Viruses (2020) 12(4):2. doi: 10.3390/v12040428
21. Wu A, Peng Y, Huang B, Ding X, Wang X, Niu P, et al. Genome Composition and Divergence of the Novel Coronavirus (2019-Ncov) Originating in China. Cell Host Microbe (2020) 27(3):325–8. doi: 10.1016/j.chom.2020.02.001
22. Bayati A, Kumar R, Francis V, McPherson PS. SARS-CoV-2 Infects Cells Following Viral Entry via Clathrin-Mediated Endocytosis. J Biol Chem (2021) 296:100306. doi: 10.1016/j.jbc.2021.100306
23. Lu G, Hu Y, Wang Q, Qi J, Gao F, Li Y, et al. Molecular Basis of Binding Between Novel Human Coronavirus MERS-CoV and its Receptor CD26. Nature Aug (2013) 500(7461):227–31. doi: 10.1038/nature12328
24. Harrison AG, Lin T, Wang P. Mechanisms of SARS-CoV-2 Transmission and Pathogenesis. Trends Immunol (2020) 41(12):1100–15. doi: 10.1016/j.it.2020.10.004
25. Papa G, Mallery DL, Albecka A, Welch LG, Cattin-Ortola J, Luptak J, et al. Furin Cleavage of SARS-CoV-2 Spike Promotes But is Not Essential for Infection and Cell-Cell Fusion. PloS Pathog (2021) 17(1):e1009246. doi: 10.1371/journal.ppat.1009246
26. Cantuti-Castelvetri L, Ojha R, Pedro LD, Djannatian M, Franz J, Kuivanen S, et al. Neuropilin-1 Facilitates SARS-CoV-2 Cell Entry and Infectivity. Science (2020) 370(6518):856–60. doi: 10.1126/science.abd2985
27. Fernandez C, Rysä J, Almgren P, Nilsson J, Engstrom G, Orho-Melander M, et al. Plasma Levels of the Proprotein Convertase Furin and Incidence of Diabetes and Mortality. J Intern Med (2018) 284(4):377–87. doi: 10.1111/joim.12783
28. Samuel VT, Shulman GI. The Pathogenesis of Insulin Resistance: Integrating Signaling Pathways and Substrate Flux. J Clin Invest (2016) 126(1):12–22. doi: 10.1172/JCI77812
29. Randle PJ, Garland PB, Hales CN, Newsholme EA. The Glucose Fatty-Acid Cycle. Its Role in Insulin Sensitivity and the Metabolic Disturbances of Diabetes Mellitus. Lancet Apr (1963) 1(7285):785–9. doi: 10.1016/s0140-6736(63)91500-9
31. Krssak M, Falk Petersen K, Dresner A, DiPietro L, Vogel SM, Rothman DL, et al. Intramyocellular Lipid Concentrations are Correlated With Insulin Sensitivity in Humans: A 1H NMR Spectroscopy Study. Diabetologia (1999) 42(1):113–6. doi: 10.1007/s001250051123
32. Summers SA, Garza LA, Zhou H, Birnbaum MJ. Regulation of Insulin-Stimulated Glucose Transporter GLUT4 Translocation and Akt Kinase Activity by Ceramide. Mol Cell Biol (1998) 18(9):5457–64. doi: 10.1128/MCB.18.9.5457
33. Itani SI, Ruderman NB, Schmieder F, Boden G. Lipid-Induced Insulin Resistance in Human Muscle is Associated With Changes in Diacylglycerol, Protein Kinase C, and IkappaB-Alpha. Diabetes (2002) 51(7):2005–11. doi: 10.2337/diabetes.51.7.2005
34. Turinsky J, O’Sullivan DM, Bayly BP. 1,2-Diacylglycerol and Ceramide Levels in Insulin-Resistant Tissues of the Rat In Vivo. J Biol Chem (1990) 265(28):16880–5. doi: 10.1016/S0021-9258(17)44844-7
35. Holland WL, Bikman BT, Wang LP, Yuguang G, Sargent KM, Bulchand S, et al. Lipid-Induced Insulin Resistance Mediated by the Proinflammatory Receptor TLR4 Requires Saturated Fatty Acid-Induced Ceramide Biosynthesis in Mice. J Clin Invest (2011) 121(5):1858–70. doi: 10.1172/JCI43378
36. Samuel VT, Shulman GI. Mechanisms for Insulin Resistance: Common Threads and Missing Links. Cell (2012) 148(5):852–71. doi: 10.1016/j.cell.2012.02.017
37. Hotamisligil GS. Inflammatory Pathways and Insulin Action. Int J Obes Relat Metab Disord (2003) 27 Suppl 3:S53–5. doi: 10.1038/sj.ijo.0802502
38. Rui L, Aguirre V, Kim JK, Shulman GI, Lee A, Corbould A, et al. Insulin/IGF-1 and TNF-Alpha Stimulate Phosphorylation of IRS-1 at Inhibitory Ser307 via Distinct Pathways. J Clin Invest (2001) 107(2):181–9. doi: 10.1172/JCI10934
39. Chung SS, Ho EC, Lam KS, Chung SK. Contribution of Polyol Pathway to Diabetes-Induced Oxidative Stress. J Am Soc Nephrol (2003) 14(8 Suppl 3):S233–6. doi: 10.1097/01.asn.0000077408.15865.06
40. Williamson JR, Chang K, Frangos M, Hasan KS, Ido Y, Kawamura T, et al. Hyperglycemic Pseudohypoxia and Diabetic Complications. Diabetes (1993) 42(6):801–13. doi: 10.2337/diab.42.6.801
41. Cheng HM, González RG. The Effect of High Glucose and Oxidative Stress on Lens Metabolism, Aldose Reductase, and Senile Cataractogenesis. Metabolism (1986) 35(4 Suppl 1):10–4. doi: 10.1016/0026-0495(86)90180-0
42. Oates PJ, Mylari BL. Aldose Reductase Inhibitors: Therapeutic Implications for Diabetic Complications. Expert Opin Investig Drugs (1999) 8(12):2095–119. doi: 10.1517/13543784.8.12.2095
43. Lee AY, Chung SS. Contributions of Polyol Pathway to Oxidative Stress in Diabetic Cataract. FASEB J (1999) 13(1):23–30. doi: 10.1096/fasebj.13.1.23
44. Riddle MC, Buse JB, Franks PW, Knowler WC, Ratner RE, Selvin E, et al. COVID-19 in People With Diabetes: Urgently Needed Lessons From Early Reports. Diabetes Care (2020) 43(7):1378–81. doi: 10.2337/dci20-0024
45. Chen J, Wu C, Wang X, Yu J, Sun Z. The Impact of COVID-19 on Blood Glucose: A Systematic Review and Meta-Analysis. Front Endocrinol (Lausanne) (2020) 11:574541. doi: 10.3389/fendo.2020.574541
46. Patel AJ, Klek SP, Peragallo-Dittko V, Goldstein M, Burdge E, Nadile V, et al. Correlation of Hemoglobin A1C and Outcomes in Patients Hospitalized With COVID-19. Endocr Pract (2021) 27(10):1046–51. doi: 10.1016/j.eprac.2021.07.008
47. Zhu Z, Mao Y, Chen G. Predictive Value of HbA1c for in-Hospital Adverse Prognosis in COVID-19: A Systematic Review and Meta-Analysis. Prim Care Diabetes (2021) 15(6):910–7. doi: 10.1016/j.pcd.2021.07.013
48. Rashid G, Benchetrit S, Fishman D, Bernheim J. Effect of Advanced Glycation End-Products on Gene Expression and Synthesis of TNF-α and Endothelial Nitric Oxide Synthase by Endothelial Cells. Kidney Int (2004) 66(3):1099–106. doi: 10.1111/j.1523-1755.2004.00860.x
49. Berbudi A, Rahmadika N, Tjahjadi AI, Ruslami R. Type 2 Diabetes and its Impact on the Immune System. Curr Diabetes Rev (2020) 16(5):442–9. doi: 10.2174/1573399815666191024085838
50. Lim S, Bae JH, Kwon HS, Nauck MA. COVID-19 and Diabetes Mellitus: From Pathophysiology to Clinical Management. Nat Rev Endocrinol (2021) 17(1):11–30. doi: 10.1038/s41574-020-00435-4
51. Šestan M, Marinović S, Kavazović I, Cekinović Đ, Wueest S, Turk Wensveen T, et al. Virus-Induced Interferon-γ Causes Insulin Resistance in Skeletal Muscle and Derails Glycemic Control in Obesity. Immunty (2018) 49(1):164–77.e6. doi: 10.1016/j.immuni.2018.05.005
52. Fournet M, Bonté F, Desmoulière A. Glycation Damage: A Possible Hub for Major Pathophysiological Disorders and Aging. Aging Dis Oct (2018) 9(5):880–900. doi: 10.14336/AD.2017.1121
53. Müller S, Martin S, Koenig W, Hanifi-Moghaddam P, Rathmann W, Haastert B, et al. Impaired Glucose Tolerance is Associated With Increased Serum Concentrations of Interleukin 6 and Co-Regulated Acute-Phase Proteins But Not TNF-Alpha or its Receptors. Diabetol Jun (2002) 45(6):805–12. doi: 10.1007/s00125-002-0829-2
54. Rodríguez-Morán M, Guerrero-Romero F. Increased Levels of C-Reactive Protein in Noncontrolled Type II Diabetic Subjects. J Diabetes Complications (1999) 13(4):211–5. doi: 10.1016/s1056-8727(99)00047-1
55. Randeria SN, Thomson GJA, Nell TA, Roberts T, Pretorius E. Inflammatory Cytokines in Type 2 Diabetes Mellitus as Facilitators of Hypercoagulation and Abnormal Clot Formation. Cardiovasc Diabetol (2019) 18(1):72. doi: 10.1186/s12933-019-0870-9
56. Calzetta L, Aiello M, Frizzelli A, Rogliani P, Chetta A. Dexamethasone in Patients Hospitalized With COVID-19: Whether, When and to Whom. J Clin Med (2021) 10(8):4–5. doi: 10.3390/jcm10081607
57. Morohoshi M, Fujisawa K, Uchimura I, Numano F. The Effect of Glucose and Advanced Glycosylation End Products on IL-6 Production by Human Monocytes. Ann N Y Acad Sci (1995) 748:562–70. doi: 10.1111/j.1749-6632.1994.tb17362.x
58. Jafar N, Edriss H, Nugent K. The Effect of Short-Term Hyperglycemia on the Innate Immune System. Am J Med Sci (2016) 351(2):201–11. doi: 10.1016/j.amjms.2015.11.011
59. Shomali N, Mahmoudi J, Mahmoodpoor A, Zamiri RE, Akbari M, Xu H, et al. Harmful Effects of High Amounts of Glucose on the Immune System: An Updated Review. Biotechnol Appl Biochem (2021) 68(2):404–10. doi: 10.1002/bab.1938
60. Cromer D, Juno JA, Khoury D, Reynaldi A, Wheatley AD, Kent SJ, et al. Prospects for Durable Immune Control of SARS-CoV-2 and Prevention of Reinfection. Nat Rev Immunol (2021) 21(6):395–404. doi: 10.1038/s41577-021-00550-x
61. Pal R, Sachdeva N, Mukherjee S, Suri V, Zohmangaihi D, Ram S, et al. Impaired Anti-SARS-CoV-2 Antibody Response in non-Severe COVID-19 Patients With Diabetes Mellitus: A Preliminary Report. Diabetes Metab Syndr (2021) 15(1):193–6. doi: 10.1016/j.dsx.2020.12.035
62. Lampasona V, Secchi M, Scavini M, Bazzigaluppi E, Bragatti C, Marzinotto I, et al. Antibody Response to Multiple Antigens of SARS-CoV-2 in Patients With Diabetes: An Observational Cohort Study. Diabetologia (2020) 63(12):2548–58. doi: 10.1007/s00125-020-05284-4
63. Aquilano K, Baldelli S, Ciriolo MR. Glutathione: New Roles in Redox Signaling for an Old Antioxidant. Front Pharmacol (2014) 5:196. doi: 10.3389/fphar.2014.00196
64. Johnson WM, Wilson-Delfosse AL, Mieyal JJ. Dysregulation of Glutathione Homeostasis in Neurodegenerative Diseases. Nutrients (2012) 4(10):1399–440. doi: 10.3390/nu4101399
65. Armstrong JS, Steinauer KK, Hornung B, Irish JM, Lecane P, Birrell GW, et al. Role of Glutathione Depletion and Reactive Oxygen Species Generation in Apoptotic Signaling in a Human B Lymphoma Cell Line. Cell Death Differ (2002) 9(3):252–63. doi: 10.1038/sj.cdd.4400959
66. Wu XY, Luo AY, Zhou YR, Ren JH. N-Acetylcysteine Reduces Oxidative Stress, Nuclear Factor−κb Activity and Cardiomyocyte Apoptosis in Heart Failure. Mol Med Rep (2014) 10(2):615–24. doi: 10.3892/mmr.2014.2292
67. Khatchadourian C, Sisliyan C, Nguyen K, Poladian N, Tian Q, Tamjidi F, et al. Hyperlipidemia and Obesity’s Role in Immune Dysregulation Underlying the Severity of COVID-19 Infection. Clin Pract (2021) 11(4):694–707. doi: 10.3390/clinpract11040085
68. Kryukov EV, Ivanov AV, Karpov VO, Alexandrin VV, Dygai AM, Kruglova MP, et al. Association of Low Molecular Weight Plasma Aminothiols With the Severity of Coronavirus Disease 2019. Oxid Med Cell Longev (2021) 2021:9221693. doi: 10.1155/2021/9221693
70. ClinicalTrial.gov. A Study to Evaluate the Efficacy and Safety of Prothione Capsule for Mild to Moderate Coronavirus Disease. (2019).
73. ClinicalTrials.gov. Efficacy of N-Acetylcysteine (NAC) in Preventing Covid-19 From Progressing to Severe Disease.
74. Entrenas Castillo M, Entrenas Costa LM, Vaquero Barrios JM, Alcalá Diaz JF, López Miranda J, Bouillon R, et al. “Effect of Calcifediol Treatment and Best Available Therapy Versus Best Available Therapy on Intensive Care Unit Admission and Mortality Among Patients Hospitalized for COVID-19: A Pilot Randomized Clinical Study”. J Steroid Biochem Mol Biol (2020) 203:105751. doi: 10.1016/j.jsbmb.2020.105751
75. Lakkireddy M, Gadiga SG, Malathi RD, Karra ML, Murthy Raju P I.S.S.V, Ragini, et al. Impact of Daily High Dose Oral Vitamin D Therapy on the Inflammatory Markers in Patients With COVID 19 Disease. Sci Rep (2021) 11(1):10641. doi: 10.1038/s41598-021-90189-4
76. ClinicalTrials.gov. Clincal Outcomes of High Dose Vitamin D Versus Standard Dose in Covid-19 Egyptian Patients.
77. ClinicalTrials.gov. Effect of Vitamin D Therapy on Morbidity and Mortality in Patients With SARS-CoV2 Infection.
78. Rafique A, Rejnmark L, Heickendorff L, Moller HJ. 25(OH)D3 and 1.25(OH)2D3 Inhibits TNF-α Expression in Human Monocyte Derived Macrophages. PloS One (2019) 14(4):e0215383–e0215383. doi: 10.1371/journal.pone.0215383
79. Andrukhov O, Andrukhova O, Hulan U, Tang Y, Bantleon HP, Rausch-Fan X. Both 25-Hydroxyvitamin-D3 and 1,25-Dihydroxyvitamin-D3 Reduces Inflammatory Response in Human Periodontal Ligament Cells. PloS One (2014) 9(2):e90301–1. doi: 10.1371/journal.pone.0090301
80. Mussbacher M, Salzmann M, Brostjan C, Hoesel B, Schoergenhofer C, Datler H, et al. Cell Type-Specific Roles of NF-κb Linking Inflammation and Thrombosis. Front Immunol (2019) 10:85–5. doi: 10.3389/fimmu.2019.00085
81. Martinez-Moreno JM, Herencia C, Montes de Oca A, Munoz-Castaneda JR, Rodriguez-Ortiz ME, Diaz-Tocados JM, et al. Vitamin D Modulates Tissue Factor and Protease-Activated Receptor 2 Expression in Vascular Smooth Muscle Cells. FASEB J (2016) 30(3):1367–76. doi: 10.1096/fj.15-272872
82. Sepidarkish M, Farsi F, Akbari-Fakhrabadi M, Namazi N, Almasi-Hashiani A, Hagiagha AM, et al. The Effect of Vitamin D Supplementation on Oxidative Stress Parameters: A Systematic Review and Meta-Analysis of Clinical Trials. Pharmacol Res (2019) 139:141–52. doi: 10.1016/j.phrs.2018.11.011
83. Martin TRKC. Vitamin D and Diabetes. Diabetes Spectr (2011) p:113–8. doi: 10.2337/diaspect.24.2.113
84. Dror AA, Morozov N, Daoud A, Namir Y, Yakir O, Shachar Y, et al. Pre-Infection 25-Hydroxyvitamin D3 Levels and Association With Severity of COVID-19 Illness. PloS One (2022) 17(2):e0263069. doi: 10.1371/journal.pone.0263069
85. Demir M, Demir F, Aygun H. Vitamin D Deficiency is Associated With Covid-19 Positivity and Severity of the Disease. J Med Virol (2021) 93(5):2992–9. doi: 10.1002/jmv.26832
86. Jain SK, Parsanathan R, Achari AE, Kanikarla-Marie P, Bocchini JA. Glutathione Stimulates Vitamin D Regulatory and Glucose-Metabolism Genes, Lowers Oxidative Stress and Inflammation, and Increases 25-Hydroxy-Vitamin D Levels in Blood: A Novel Approach to Treat 25-Hydroxyvitamin D Deficiency. Antioxid Redox Signal (2018) 29(17):1792–807. doi: 10.1089/ars.2017.7462
87. Parsanathan R, Jain SK. Glutathione Deficiency Induces Epigenetic Alterations of Vitamin D Metabolism Genes in the Livers of High-Fat Diet-Fed Obese Mice. Sci Rep (2019) 9(1):14784. doi: 10.1038/s41598-019-51377-5
88. Ramamoorthy S, Cidlowski JA. Corticosteroids: Mechanisms of Action in Health and Disease. Rheum Dis Clin North Am (2016) 42(1):15–31. doi: 10.1016/j.rdc.2015.08.002
89. Coutinho AE, Chapman KE. The Anti-Inflammatory and Immunosuppressive Effects of Glucocorticoids, Recent Developments and Mechanistic Insights. Mol Cell Endocrinol (2011) 335(1):2–13. doi: 10.1016/j.mce.2010.04.005
90. Yeager MP, Guyre PM, Munck AU. Glucocorticoid Regulation of the Inflammatory Response to Injury. Acta Anaesthesiol Scand (2004) 48(7):799–813. doi: 10.1111/j.1399-6576.2004.00434.x
91. Auphan N, DiDonato JA, Rosette C, Helmberg A, Karin M. Immunosuppression by Glucocorticoids: Inhibition of NF-Kappa B Activity Through Induction of I Kappa B Synthesis. Science (1995) 270(5234):286–90. doi: 10.1126/science.270.5234.286
92. Scheinman RI, Cogswell PC, Lofquist AK, Baldwin AS. Role of Transcriptional Activation of I Kappa B Alpha in Mediation of Immunosuppression by Glucocorticoids. Science (1995) 270(5234):283–6. doi: 10.1126/science.270.5234.283
93. Rhen T, Cidlowski JA. Antiinflammatory Action of Glucocorticoids–New Mechanisms for Old Drugs. N Engl J Med (2005) 353(16):1711–23. doi: 10.1056/NEJMra050541
94. Tobler A, Meier R, Seitz M, Dewald B, Baggiolini M, Fey MF. Glucocorticoids Downregulate Gene Expression of GM-CSF, NAP-1/IL-8, and IL-6, But Not of M-CSF in Human Fibroblasts. Blood (1992) 79(1):45–51. doi: 10.1182/blood.V79.1.45.bloodjournal79145
95. Theoharides TC, Conti P. Dexamethasone for COVID-19? Not So Fast. J Biol Regul Homeost Agents 2020 (2020) 34(3):1241–3. doi: 10.23812/20-EDITORIAL_1-5
96. Lee N, Allen Chan KC, Hui DS, Ng EKO, Wu A, Chiu RWK, et al. Effects of Early Corticosteroid Treatment on Plasma SARS-Associated Coronavirus RNA Concentrations in Adult Patients. J Clin Virol (2004) 31(4):304–9. doi: 10.1016/j.jcv.2004.07.006
97. Pasin L, Navalesi P, Zangrillo A, Kuzovlev A, Likhvantsev V, Hajjar LA, et al. Corticosteroids for Patients With Coronavirus Disease 2019 (COVID-19) With Different Disease Severity: A Meta-Analysis of Randomized Clinical Trials. J Cardiothorac Vasc Anesth (2021) 35(2):578–84. doi: 10.1053/j.jvca.2020.11.057
98. Health Care Readiness WHH. Corticosteriods for COVID-19. World Health Organization (2020). Available at: https://www.who.int/publications/i/item/WHO-2019-nCoV-Corticosteroids-2020.1. Accessed December 1, 2021.
99. CDC. Science Brief: SARS-CoV-2 Infection Induced and Vaccine-Induced Immunity. (2021) [cited 2021 November 1], Available at: https://www.cdc.gov/coronavirus/2019-ncov/science/science-briefs/vaccine-induced-immunity.html#print.
100. Dan JM, Mateus J, Kato Y, Hastie KM, Yu ED, Faliti CE, et al. Immunological Memory to SARS-CoV-2 Assessed for Up to 8 Months After Infection. Science (2021) 371(6529):1, 3–4. doi: 10.1126/science.abf406399
101. Nanduri S, Pilishvili T, Derado G, Soe MM, Dollard P, Wu H, et al. Effectiveness of Pfizer-BioNTech and Moderna Vaccines in Preventing SARS-CoV-2 Infection Among Nursing Home Residents Before and During Widespread Circulation of the SARS-CoV-2 B.1.617.2 (Delta) Variant - National Healthcare Safety Network, March 1-August 1, 2021. MMWR Morb Mortal Wkly Rep (2021) 70(34):1163–6. doi: 10.15585/mmwr.mm7034e3
Keywords: type 2 diabetes, SARS-COV-2 infection, immune response, inflammation, oxidative stress
Citation: Singh M, Barrera Adame O, Nickas M, Robison J, Khatchadourian C and Venketaraman V (2022) Type 2 Diabetes Contributes to Altered Adaptive Immune Responses and Vascular Inflammation in Patients With SARS-CoV-2 Infection. Front. Immunol. 13:833355. doi: 10.3389/fimmu.2022.833355
Received: 11 December 2021; Accepted: 28 February 2022;
Published: 24 March 2022.
Edited by:
Esmaeil Mortaz, National Research Institute of Tuberculosis and Lung Diseases (NRITLD), IranReviewed by:
Galileo Escobedo, General Hospital of Mexico, MexicoCopyright © 2022 Singh, Barrera Adame, Nickas, Robison, Khatchadourian and Venketaraman. This is an open-access article distributed under the terms of the Creative Commons Attribution License (CC BY). The use, distribution or reproduction in other forums is permitted, provided the original author(s) and the copyright owner(s) are credited and that the original publication in this journal is cited, in accordance with accepted academic practice. No use, distribution or reproduction is permitted which does not comply with these terms.
*Correspondence: Vishwanath Venketaraman, dnZlbmtldGFyYW1hbkB3ZXN0ZXJudS5lZHU=
†These authors have contributed equally to this work
Disclaimer: All claims expressed in this article are solely those of the authors and do not necessarily represent those of their affiliated organizations, or those of the publisher, the editors and the reviewers. Any product that may be evaluated in this article or claim that may be made by its manufacturer is not guaranteed or endorsed by the publisher.
Research integrity at Frontiers
Learn more about the work of our research integrity team to safeguard the quality of each article we publish.