- 1Division of Infectious Disease and HIV Medicine, Department of Medicine, Case Western Reserve University, Cleveland, OH, United States
- 2Cleveland Medical Center, University Hospitals Cleveland Medical Center, Cleveland, OH, United States
- 3Division of Geographic Medicine and Infectious Diseases, Department of Internal Medicine, UT Southwestern Medical Center, Dallas, TX, United States
- 4Department of Immunology, UT Southwestern Medical Center, Dallas, TX, United States
- 5Parkland Health and Hospital System, Dallas, TX, United States
Despite over a century of research, Mycobacterium tuberculosis (Mtb), the causative agent of tuberculosis (TB), continues to kill 1.5 million people annually. Though less than 10% of infected individuals develop active disease, the specific host immune responses that lead to Mtb transmission and death, as well as those that are protective, are not yet fully defined. Recent immune correlative studies demonstrate that the spectrum of infection and disease is more heterogenous than has been classically defined. Moreover, emerging translational and animal model data attribute a diverse immune repertoire to TB outcomes. Thus, protective and detrimental immune responses to Mtb likely encompass a framework that is broader than T helper type 1 (Th1) immunity. Antibodies, Fc receptor interactions and B cells are underexplored host responses to Mtb. Poised at the interface of initial bacterial host interactions and in granulomatous lesions, antibodies and Fc receptors expressed on macrophages, neutrophils, dendritic cells, natural killer cells, T and B cells have the potential to influence local and systemic adaptive immune responses. Broadening the paradigm of protective immunity will offer new paths to improve diagnostics and vaccines to reduce the morbidity and mortality of TB.
The Challenge of Heterogeneity in Clinical Tuberculosis
Every six seconds, an individual is diagnosed with tuberculosis (TB); every twenty seconds an individual dies from active disease (1). This level of morbidity and mortality persists today despite shorter antimicrobial treatment regimens and more sensitive, specific and widely distributed diagnostics. Strategies that prevent active disease are necessary to complement advances in detection and cure. However, defining the targets of prevention is challenged by the heterogeneity of manifestations and outcomes in clinical TB.
Improvements in microbiologic, immunologic and radiographic tools to identify and characterize humans infected with Mycobacterium tuberculosis (Mtb) have expanded the phenotypic spectrum appreciated within and beyond the classical states of latent infection and active disease (Figure 1). Over 90% of human TB is thought to exist as latent infection. This state is defined by the presence of IFNγ secreting T cell response to Mtb antigens after exposure to the bacteria and the absence of signs and symptoms of active disease. The host response is historically captured by the tuberculin skin test (TST), a cell mediated response to intradermal injection of a mixture of Mtb proteins prepared from culture called purified protein derivative (PPD). To overcome false positive responses to non-tuberculous mycobacteria (NTM), including the vaccine strain, Mycobacterium bovis Bacille Calmette-Guérin (BCG), IFNγ release assays (IGRA) were developed as blood tests that measure responses to the Mtb proteins ESAT6, CFP10 and TB7.7. Yet despite enhanced specificity, the rates of false negatives have limited the use of these T cell-based tests in the diagnosis of the remaining 5-10% of TB which is active disease. Instead, active TB disease is defined by the presence of clinical signs and symptoms, radiographic evidence of disease and microbiological evidence of bacteria (detectable by culture, cell wall stain or nucleic acid amplification). Thus, only latent but not active TB is routinely diagnosed using markers of the host immune response. Specific patterns of human immune reactivity that are sterilizing have not yet been discovered but has significant implications for understanding protection from Mtb infection and disease (2).
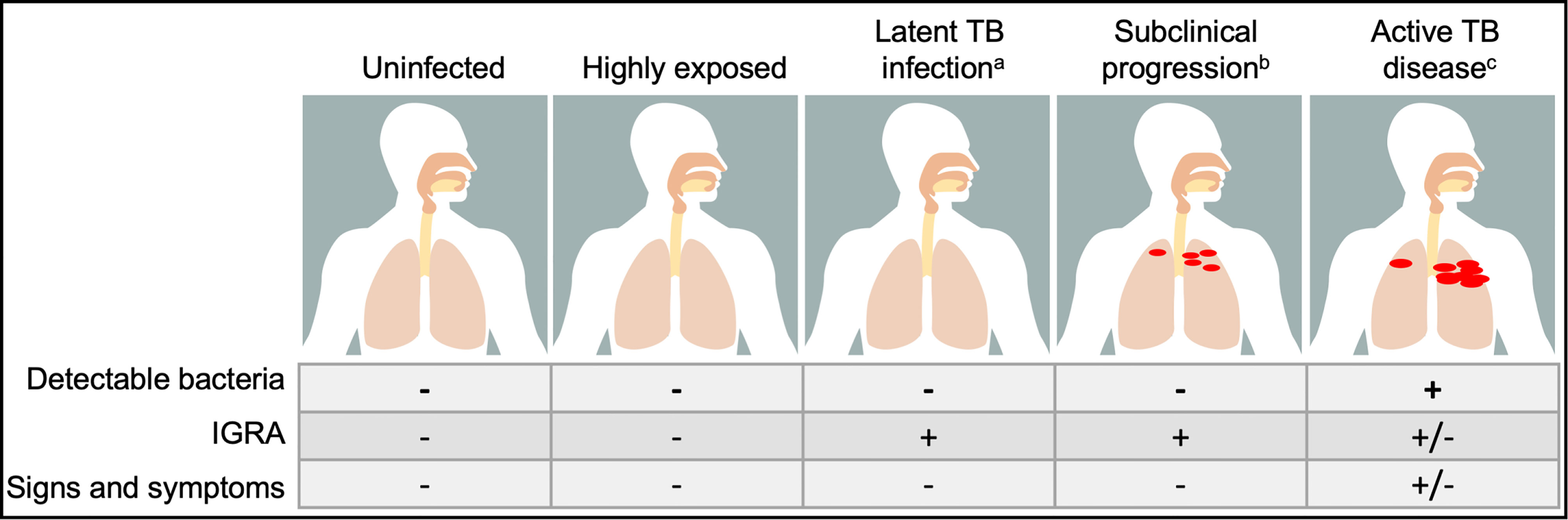
Figure 1 The spectrum of outcomes in human tuberculosis (TB) is heterogenous. Classical clinical states are defines by the presence of detectable bacteria and host response to Mycobacterium tuberculosis antigens by interferon γ release assay (IGRA), with uninfected having neither, latent TB infection having a positive IGRA but no detectable bacteria and active TB disease diagnosed by the capture of Mtb by growth in culture, nucleic acid amplification or cell wall stain. The transition between these states is fluid and poorly captured by these criteria: a. Individuals who have received antibiotic therapy for latent TB infection cannot be differentiated from those who are treatment naïve. b. Individuals who progress subclinically from latent infection to active disease (5-10%) and those who regress or remain asymptomatic (>90%) are indistinguishable. c. After successful treatment with antibiotics for active TB disease, individuals no longer have detectable bacteria as captured by standard assays but may have residual positive IGRA. Moreover, emerging epidemiological and immune correlates data suggest that beyond these classical states, there are groups who are highly exposed to Mtb who potentially represent an alternative state to latent TB infection not yet clearly defined.
Beyond latent and active TB, immunological and imaging modalities point towards states of infection after Mtb exposure that do not fall into the latent versus active TB dichotomy (Figure 1). Waxing and waning T cell and antibody responses to Mtb antigens in a subset of individuals with “latent TB” suggest the existence of states after Mtb exposure outside of the traditional definition but are also not active disease (3). Detection of Mtb RNA and DNA from individuals who meet the definitions of clinical and microbiological cure show bacterial persistence, highlighting the difficulty of conventional immune and microbiological assays to identify the presence of bacteria (4, 5). Finally, highly sensitive computed tomography (CT) and positron emission tomography (PET) imaging has demonstrated different lesions concomitantly regressing and progressing within the same individual, indicating immune response heterogeneity between granulomatous lesions that may underlie clinical outcomes (6–9). These findings advance our understanding of the spectrum of clinical TB, demanding similar innovation in models of disease to expand beyond the current T helper type 1 (Th1)-centric immunological paradigm to capture the diverse spectrum of Mtb infection and disease (10–12).
The Potential of Antibodies and B Cells to Influence Tuberculosis
As a major arm of adaptive immunity, B cells and antibodies have the potential to modulate immune responses to Mtb. In contrast to the dominant roles uncovered in responses to viral and other bacterial infections, antibodies and B cells fill a minor part of the literature on the host response to Mtb. Yet even in this limited evaluation, there is data to suggest that humoral immunity is a complex and promising path towards understanding TB immunology.
Divergent interpretations of antibody and B cell data reflect limitations in contemporary experimental systems. Ablation of B cell (13) and antibody Fc effector functions (14) in mice and non-human primate models (15) impact local bacterial burden and pathology, and antibody Fc features correlate with different human disease states (16–18). Yet, passive transfer of antibodies into mice do not consistently confer protection (19), human deficiencies in immunoglobulins or B cells do not incur increased risk of TB (20, 21) and antibody titers alone are unable to define infection and disease (22). For animal studies, one of the inherent caveats is that models imprecisely phenocopy human Mtb infection and disease. For example, the wildly used inbred C57BL6 mouse model does not form the same granuloma pathology (23) and have different antibody and Fc receptor (FcR) repertoires compared to humans (24). For human studies, power is limited by access to relevant tissues containing Mtb and heterogenous clinical manifestations requiring years in duration for evaluation. For studies of humoral immunity, these difficulties are compounded by the persistence of residual antibodies and B cells despite pharmacologic depletion of plasma and B cells in humans (25, 26). As such, the potential of antibodies and B cells to influence the course of TB is inescapably stitched from studies that identify correlates of protection and disease in humans and evaluate mechanisms in model systems. Here, we extrapolate from the literature to understand the capacity of antibodies and B cells to: (1) modulate initial interactions between Mtb and host cells, (2) guide the development of adaptive immunity, and (3) contribute to protection and disease across the spectrum of clinical TB.
Functional Diversity in Antibodies and B Cells
Antigen Binding Fraction (Fab) Domain
Computational analyses of high throughput sequencing data estimate that the human antibody repertoire has the capacity to bind to 1013 unique molecules via the antigen binding fragment Fab domain (27). Targeted molecules span proteins, glycans, lipids and nucleic acids from self and non-self, contributing to specific and broad recognition patterns. For Mtb, potential antigens recognized by antibodies emanate from the over 4000 open reading frames (28) as well as carbohydrates and lipids that comprise ~60% of the bacterial cell envelope (29). Because of molecular mimicry between microbial and human glyco- and lipo- conjugates, broadly reactive antibodies even at low affinity and avidity have the potential to influence Mtb-host interactions. As such, somatic recombination of V(D)J gene segments and hypermutation in the naïve variable gene region following antigen recognition generate diverse Fab domains with the potential to induce different antibody mediated immune responses and clinical outcomes. Yet efforts to develop antibody-based diagnostics demonstrate that titers poorly capture the spectrum of TB (22). Thus, the search continues for Mtb antigens that induce relevant immune correlates via new combinations of protein, carbohydrate and lipid targets such as lipoarabinomannan (LAM). Moreover, the inability of indirect measures of mycobacterial antigenic burden, such as that by the antibody Fab domain to capture the complexity of Mtb infection and disease, compel the pursuit of immune responses beyond direct target detection.
Crystallizable Fraction (Fc) Domain
Antibody function is determined by the combination of the Fab and crystallizable (Fc) domains. The Fc domain can alter the structure of the Fab domain, impacting antigen recognition. Equally as important as antigen binding, the Fc domain is essential to recruiting innate and adaptive humoral and cellular immune responses. Diversity in the Fc domain is generated through different isotypes (IgM, IgG, IgA, IgE, IgD) and subclasses (IgG1, IgG2, IgG3, IgG4, IgA1, IgA2) (Figure 2A). Studies of polyclonal responses show that all are produced in response to Mtb infection with no one dominating correlate of disease or protection, although there are hints in some cohorts that decreased IgG3 is associated with recurrence (30) and increased IgG4 with active disease (18). Some monoclonal studies have suggested potential differential capacities between IgA, IgG and IgM to induce extra and intracellular host environments that restrict or enhance Mtb (31, 32). If these findings are confirmed by studies in polyclonal responses that influence clinical outcomes, the mechanisms likely involve differential immune complexing. With monomeric and multimeric antibodies, the affinity and avidity for microbial antigens in the Fab domain and host receptors and complement in the Fc domain can vary widely.
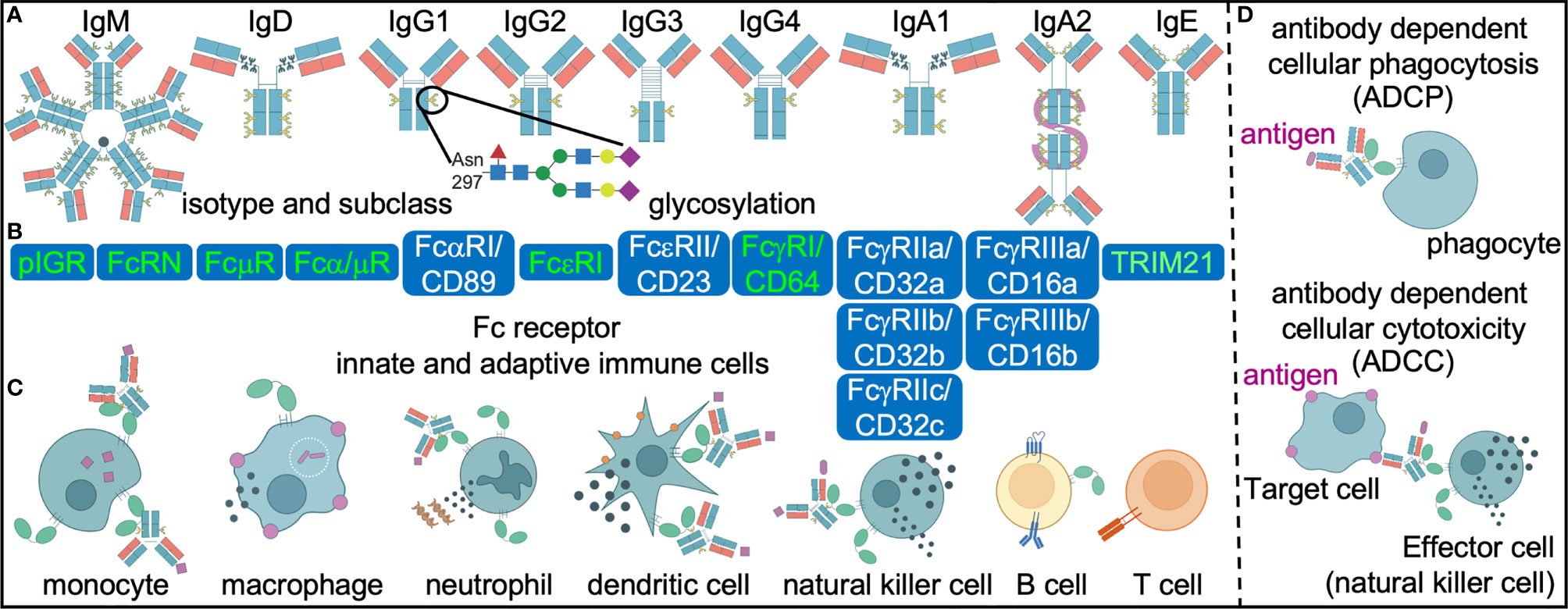
Figure 2 Differential antibody engagement with Fc receptors mediate diverse innate and adaptive immune cell effector functions. Antibody diversity (A) in antigen specificity (theoretical n=1013), isotype (n=5), subclass (n=6) and post-translational glycosylation (theoretical n=36 possible different moieties) influence engagement of Fc receptors. Subsequent differential activation of high (red) and low (white) affinity Fc receptors (B) expressed on innate and adaptive immune cells (C) have the potential to mediate effector functions such as antibody dependent cellular phagocytosis and cytotoxicity (D) that in concert determine the heterogenous outcomes in human TB.
Antibody Post Translational Glycosylation
All antibodies are glycoproteins. These post translational modifications are made by glycosyltransferases and glycosidases during trafficking through the endoplasmic reticulum and Golgi apparatus of B cells. Some data suggest that further modification is possible in a B cell independent manner through glycosyltransferases secreted into the circulatory system (33–36). While all polyclonal IgG have a conserved asparagine residue 297 on the Fc domain that is glycosylated, only 15-20% are modified on the Fab domain (37). These N-linked glycan structures have a complex bi-antennary core composed of N acetylglucosamine and mannose residues (Figure 2A). The further addition and subtraction of N-acetylglucosamine, galactose, sialic acid and fucose extend the structural and functional heterogeneity of the Fc and Fab domains by altering affinity for FcRs and target antigens. O-linked glycosylation of proteins occurs on functional hydroxyl groups of serine and threonine, but site-specific analyses of O-glycans is challenged by the paucity of efficient proteolytic tools. Nevertheless the plethora of data on N-glycans within IgG show distinct and sometimes plastic glycosylation patterns in many physiological states: male versus female, age, pregnancy, infections such as those with HIV and SARS-CoV2, chronic non-infectious diseases such as diabetes and renal disease, autoimmune diseases and exacerbations (38). In some cases such as diabetes, passive transfer of antibodies into mice of differentially glycosylated IgG and the in vivo modification of glycosylation show that altered sialic acid is not merely associative but also contributes to non-immune processes such as glucose intolerance through FcR mediated insulin transport (39, 40).
For latent and active TB, the N-glycan profiles on IgG diverge and vary with antigen specificity (16–18, 41). However, some themes that emerge are that glycans associated with an anti-inflammatory state (galactose and sialic acid) are increased in individuals with latent compared to active TB (16). This is consistent with an overall decreased immune mediated inflammation in latent compared to active TB. In contrast, fucose is decreased in latent compared to active TB. The lack of fucose is generally associated with increased inflammation (42, 43). That afucosylated IgG is enhanced in latent TB indicates that this change is not a biomarker of inflammation but rather potentially reflective of an antibody function. Because studies using polyclonal and monoclonal antibodies show that changes in glycosylation influence FcR affinity and cellular effector functions such as antibody dependent cellular phagocytosis (ADCP) and cytotoxicity, differential modulation of immune responses are implicated for latent and active TB. Afucosylated IgG have increased affinity for FcγRIII/CD16 which mediates antibody dependent cellular cytotoxicity (ADCC). Both are enhanced with IgG purified from latent compared to active TB patients, implicating roles in potential protection for TB (16). If and how antibody glycosylation contributes to immune mediated outcomes in the context of HIV, diabetes and renal disease, the most significant risk factors for TB, remain uncharacterized (44). As biomarkers, direct comparison between Mtb reactive-IgG glycans and titers in a small cohort of individuals show that the former has enhanced ability to distinguish between latent and active TB (17). This is likely due to the ability of IgG glycans to capture the complexity of host immune responses that connect to pathology as compared to microbial burden alone by using antibody titers. As mediators of protection, one study showed that passive transfer of non-Mtb specific polyclonal human IgG into mice after enzymatic removal of glycans increases bacterial burden (45). Whether this applies to Mtb specific IgG and what species of glycans specifically are responsible are not known but could shed light into how differential IgG glycosylation is linked to pathology and protection.
IgA and IgM are also glycosylated, but because far more residues are involved, the deconvolution of these complex post translational modifications lags behind IgG. However, emerging data from the mouse model shows changes in IgM glycans with Mtb infection (46). With further definition, these post translational mechanisms of antibody diversification that contribute to the extent of the B cell antibody repertoire can be clarified across the clinical spectrum within and beyond the latent and active TB spectrum.
Neutralizing and Non-Neutralizing Antibody Functions
For infectious diseases, directly neutralizing and non-neutralizing antibody functions potentially impact microbial infection, replication and immune mediated pathology. Through the Fab domain, antibodies recognize and bind to microbial antigens. This could lead to direct neutralizing activity in which the organism is immediately sequestered after binding. In comparison, non-neutralizing antibody functions involve the Fc domain function to a greater extent. In conjunction with targeting an antigen via the Fab domain, the Fc domain engages complement and FcRs on immune cells that induce host responses to the microbe. Non-neutralizing antibody functions include cellular phagocytosis, cytotoxicity, activation (including NETosis and antigen presentation) and cytokine production, each of which could be protective or pathogenic based on the specific context of the microbe and host. Demonstration of consistent antibody-mediated direct neutralization of Mtb leading to prevention of infection has been elusive to date with monoclonal antibodies. While in vitro studies show different non-neutralizing cell mediated functions with polyclonal IgG isolated from individuals with latent TB, active TB (16), after antibiotic treatment (18), after BCG vaccination (32, 47, 48) and/or high exposure to Mtb (41, 49), it is less clear whether they are protective, inert or pathogenic in vivo.
Fc Receptors (FcR)
Beyond the antibody glycoprotein, FcR variations in copy number and single nucleotide polymorphisms (SNPs) generate diversity. These exist for receptors that have high (FcγRI/CD64, FcRN encoded by FCGRT, FcεRI), intermediate (polymeric immunoglobulin receptor pIGR and Fα/μ receptor) and low affinity (FcγRII/CD32, FcγRIII/CD16, FcαRI/CD89, FcεRII/CD23) for monomeric immunoglobulins (Figure 2B). High variation in copy number has been observed particularly in the FCGR loci. For FcγRII/CD32a, the polymorphism R131H (also described as 167) affects the membrane proximal Ig-like domain of the extracellular region and ablates the ability to bind and phagocytose IgG2 coated particles (50, 51). The R/R131 allotype is associated with more severe outcomes in disseminated meningococcal infection (52) and recurrent bacterial infections (53) in some human populations suggest that these FcR changes could have implications across multiple infectious diseases as well as vaccines. For FcγRIII/CD16a, valine at amino acid 158 (also described as 176) increases the affinity for IgG1 and IgG3 via interactions with the lower hinge region whereas phenylalanine in this same position enhances IgG4 binding (54, 55). The functional implications of this variation are most well-described with ADCC, where individuals with 158V/V compared to 158F/F have higher levels of this classic natural killer cell mediated function. Some human studies link these allelic variants to autoimmune diseases (56) and responses to monoclonal antibody therapies in cancer (57), though the extrapolated mechanisms and impacts may be obscured by the complexity of these conditions. For the only inhibitory FcR, a loss of function variant FcγRIIBT232 is associated with protection against severe malaria in an East African population and susceptibility to the autoimmune disease systemic lupus erythematosus in the Caucasian population (58). These functional impacts are conceptualized in the context of non-neutralizing antibody activity. However, studies in mice show that Fc-FcR binding is critical in enhancing monoclonal antibody mediated direct neutralization of pathogens including HIV (59, 60), influenza (61, 62) and SARS-CoV2 (63–66). Thus, FcR variations have the potential to influence directly neutralizing and non-neutralizing antibody functions.
For TB, studies focused on FCGR genetic variations show a mixed association with outcomes. Increased FCGR1A/CD64 copy number in individuals with active disease indicates an association with poorer outcomes (67). Because the high affinity FcγRI/CD64 is also identified in the non-human primate model as a correlate of disease it could be simply a marker of general inflammation, or induce pathology (68). Beyond FcγRI/CD64, higher FCGR3B copy number in a subpopulation of Ethiopians is associated with the development of TB in people living with HIV, compared to those with HIV alone (69). The data from these two studies suggest that FCGR sequence heterogeneity is involved with inflammation in TB. Studies incorporating a larger clinical spectrum from high Mtb exposure to outcomes of antimicrobial treatment and recurrence could further clarify how FCR genetic variability impacts Mtb infection and disease.
In addition to pathology, data from both mouse models and human studies show that FcRs have the capacity to impart effects that protect the host against bacteria. Knockout of the immunoreceptor tyrosine-based activation motif (ITAM) responsible for activating signaling across FcRs in a low dose aerosol C57BL6 mouse model of Mtb infection leads to increased pulmonary Mtb burden and pathology along with decreased survival (14). Consistent with these findings, mouse knockout of the only the inhibitory FcγRIIb/CD32b leads to decreased pulmonary Mtb burden and pathology (14). Because FcγRIIb/CD32b is the only FcR functionally conserved between mice and humans, these findings could have relevance for translating findings. While in vitro blocking antibody studies show that Mtb-reactive IgG mediated opsonophagocytosis into human monocytes is FcγRII/CD32 dependent, the blocking antibody clone itself does not distinguish between the activating CD32a or inhibitory CD32b in these cells (47). Overcoming this limitation with FcγRIIb/CD32b specific tools could help clarify each respective role (70, 71). Apart from FcγRII/CD32, levels of the activating FcγRIII/CD16 correlate with latent compared to active TB across multiple cohorts (72). In addition, IgG purified from individuals with latent compared to active TB have higher affinity for FcγRIII/CD16 (16). However, the benefit of the activating FcγRIII/CD16 is likely cell type specific as CD16+ monocytes as opposed to macrophages and natural killer cells may be associated with disease severity (73). Finally, data from in vitro whole blood assays with blocking antibodies suggest that decreased bacterial replication mediated by polyclonal IgG from health care workers highly exposed to Mtb is in part due to FcγRIII/CD16 together with FcγRII/CD32 (49). Thus, the combinatorial engagement of low affinity inhibitory and activating FcRs (74) by differential immune complexes containing distinct antibody Fc determines signaling (75) contributes to immune response variability that could influence host outcomes.
Antibody Mediated Cellular Effector Functions
Antigen bound antibodies induce cell surface FcR aggregation and downstream signaling to induce effector functions (76) (Figure 2C). Antibody dependent cellular cytotoxicity (ADCC) is classically mediated by natural killer cells and macrophages that express FcγRIII/CD16 (Figure 2D). Granules containing perforin and granzyme from activated natural killer effector cells as measured by CD107a and IFNγ are released to mediate cytolysis of target cells with antigens present on the surface and recognized by the antibody. Following binding of IgG immune complexes to platelets, the release of serotonin stored in the granules is thought to contribute to systemic shock (77, 78). For FcεR, aggregation can lead to activation of mast cells, eosinophils and basophils with secretion of vasoactive amines and enzymes to generate allergic responses. Thus, the effect of granule release is dependent on the contents.
In addition to FcR aggregation, internalization of the antigen via antibody dependent cellular phagocytosis (ADCP) is an Fc effector function that can occur in neutrophils, monocytes, macrophages and dendritic cells (Figure 2D). Neutrophils express FcRs that engage IgG and IgA with phagocytosis (79), production of reactive oxygen species (80) and neutrophil extracellular traps (NETs) (81, 82) as potential consequences. Monocytes and macrophages can respond to IgG, IgA and, sometimes, IgE mediated antigen uptake (62) with autophagy (83) and vesicular trafficking into the lysosome (84) as well as inflammasome activation (85, 86). In contrast, ADCP in dendritic cells induces antigen presentation, cytokine production and maturation (70, 87–89). Thus, FcR crosslinking induce multiple effector immune functions that are cell type specific.
In TB, antibody cellular effector functions have been described to potentially impact bacteria during initial acquisition of infection and in latent and active TB. In vitro Mtb infection studies show that opsonophagocytosis is influenced by antibodies from BCG vaccination (32, 48, 90). Because extracellular Mtb is thought to exist primarily during initial acquisition of infection and active TB, divergent cellular effector functions resulting from ADCP could be more relevant in these states. Differential macrophage phagolysosomal co-localization and inflammasome activation with intracellular bacteria are reported with polyclonal IgG from latent and active TB (16). Because intracellular Mtb is associated with latent infection, cellular effector functions that target an infected host cell as opposed to bacteria alone could be relevant. This could be ADCC but more broadly speaking, any effector cell function that could impact an infected target cell (for example granule or cytokine release, vesicular trafficking, the production of reactive oxygen species) in cis or trans is plausible. To this point, the discovery of new receptors that bind to antibodies such as the cytoplasmic TRIM21 brings opportunities to expand the repertoire of linked cellular effector functions such as ubiquitination and degradation of intracellular organisms (91). How cytoplasmic receptors, as opposed to cell surface expressing receptors, mediate responses to intracellular Mtb is not known but broadens the potential mechanisms by which antibodies modulate the immune response in TB.
B Cell Functions
T cell-B cell interactions in lymphoid follicles lead to the development of long-lived, antigen-specific humoral responses from plasma cells and plasmablasts (92) (Figure 3A). Expression of the CXCR5 chemokine receptor helps define the structure of B cell follicles by facilitating CD4 T cell trafficking to germinal centers. In secondary lymphoid organs such as lymph nodes and tertiary lymphoid structures in the Mtb-infected lung, CD4+ CXCR5+ T cells are thought to mediate host protective responses (93, 94). CCR7 regulates the trafficking of B cells toward the T cell zone, where they present endocytosed antigen in the form of peptide fragments loaded onto major histocompatibility complex type II (MHC-II) molecules to CD4 T cells (95). In addition, naïve and central memory CD4 T cells express CCR7, enabling circulation through lymphoid organs and interactions with antigen presenting cells (APCs). CCR7 knockout mice have a decreased ability to control Mtb growth after high but not low dose aerosol infection (51, 96). The T cell response is delayed in these knockout mice but whether this is due to a decreased CCR7 mediated B cell trafficking and maturation is less clear. Thus, the co-localization of T cells and B cells in secondary and tertiary lymphoid structures are opportunities for reciprocal interaction and synergy that could influence outcomes in TB (Figures 3B, C).
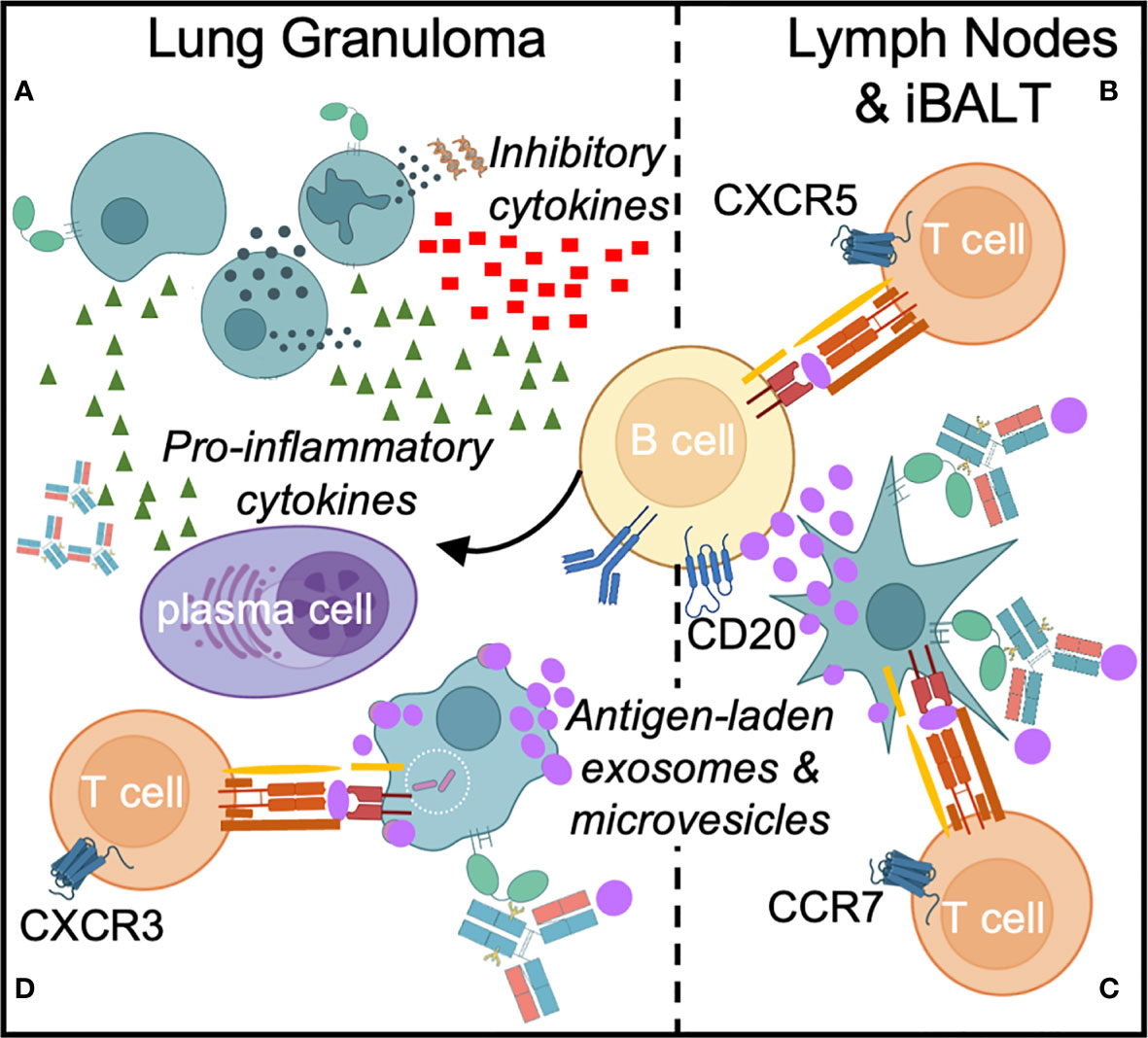
Figure 3 Direct and indirect B cell responses occur in Mtb infection. Direct responses include cytokine production and differentiation of B cells specific into plasma cells that secrete Mtb-specific antibodies (A). In response to Mtb infection in the lung, B cell effectors such as memory B cells, plasmablasts and plasma cells secrete pro-inflammatory cytokines (TNFα, IFNγ, IL2, IL6, GM-CSF and CCL3), while a subset of B cells can secrete inhibitory cytokines, such as IL10, which limits Th17 cell and neutrophil infiltration. In secondary and tertiary lymphoid organs such as lymph nodes and inducible bronchus-associated lymphoid tissue (iBALT), B cells facilitate antigen presentation to T cells (B, C). B cells receive T cell help from Tfh cells to initiate germinal center responses (B) and might also participate in priming T cells through the transfer of Mtb antigens (purple) to dendritic cells via exosomes and extracellular microvesicles (C). Finally, Fc-FcR interactions can enhance Mtb phagocytosis, macrophage and dendritic cell effecror functions, and antigen presentation to T cells in the lung granuloma (D).
The presence of B cells and follicular structures in granulomas and inducible broncho-alveolar associated lymphoid tissue (iBALT) is described in humans, mouse and non-human primate models of TB. These pulmonary lesions are associated with protection against active disease in the non-human primate model (15, 93, 97–99). Furthermore, the identification of Mtb-specific antibody responses and their association with protection in latent infection and in other heavily exposed individuals indicate a protective role for B cells in TB (16, 41, 49), potentially through the activation of Mtb-specific T cell responses and regulation of inflammation (13).
While dendritic cells classically act as APCs to activate T cells in lymphoid organs, early experiments identified a role for B cells in the priming of antigen-specific CD4 T cell responses to vaccine antigens in mice (100). B cell depletion prior to vaccination ablates subsequent T cell proliferation upon ex vivo exposure to APCs and antigen. Interestingly, subcutaneous B cell supplementation proximal to draining lymph nodes at the time of vaccination rescues this muted T cell expansion. Moreover, B cells produce cytokines that regulate T cell responses during infection and persist as antigen-specific memory cells after the resolution of inflammation (101). While patients with active TB have dysfunctional circulating B cells (102–104), the implications for effector functions beyond antibody production and how they impact local (13, 15, 97) and systemic responses remains to be clarified. Because B cell depletion by anti-CD20 antibodies alters T cell activation, cytokine production and Mtb burden in pulmonary lesions (15), evaluation at the lesional level may afford the best appreciation of the diverse spectrum of B cell functions.
B cells were only recently observed to act as natural APCs for T cells, promoting their activation and proliferation when B cells were exposed to T follicular helper (Tfh) cell CD40L and IL4 signaling in contrast to IL21 signaling (105, 106). Colocalization at the T cell-B cell border of lymph nodes or within B cell follicles represent opportunities for B cell antigen presentation to T cells, facilitating T helper functions, B cell activation and clonal expansion. Furthermore, the distinct presence of B cell follicles and germinal centers in the lung as a feature of both granulomas and the adjacent iBALT raises the possibility of roles for B cells in local antigen presentation to T cells, either directly or via exosomes and microvesicles (107) (Figures 3C, D). CD40-activated B cells have been shown to prime at least transient effector CD8 T cell responses to intracellular bacteria such as Listeria monocytogenes (108). Dissecting the impact of B cell mediated antigen presentation for the intracellular Mtb could provide a path towards recognizing alternative mechanisms of T cell activation.
B cells and plasma cells produce pro-inflammatory cytokines (IFNγ, TNFα, IL2, IL6, CCL3, GM-CSF) that are balanced by the ability of certain subsets to produce anti-inflammatory IL10 and IL17 to regulate T cell responses (109). Exploitation of IL10 associated inhibitory properties by intracellular pathogens have been described with Salmonella typhimurium (110) and Listeria (111). In mouse models of these infections, stimulation of TLR2 and TLR4 induces IL10 production from B cells which, when inhibited, improves host control of bacterial burden. In non-human primate models of TB, IL10 is thought to regulate local immune responses to Mtb in lung granulomas where both T and B cells traffick (112). Thus, it is plausible that like Salmonella and Listeria, TLR2 signaling induced by Mtb (113) could modulate IL10 levels through B cells as well as macrophages to locally influence outcomes in TB lesions (114). Similarly, Th17 responses not only modulate B cell function by inducing class switching but B cells, particularly the regulatory subset, can in turn suppress Th17 responses (115, 116). In patients with more severe cavitary TB disease, CD19+ CD1d+ CD5+ B regulatory cells are increased, dampening IL17 (117, 118). Thus, IL10 and IL17 are additional conduits through which B cells can regulate T cell responses and subsequent protection as well as pathology.
At the Interface of Innate Host Mtb Interactions
Antibodies in the Respiratory Tract
Because antibodies are detected at the alveolar epithelia, the site of initial Mtb exposure in aerosol transmission, they are well-positioned to mediate the initial interactions with bacteria. In healthy humans, bronchial sampling by lavage demonstrates that IgG is present in the respiratory tract at levels equivalent to peripheral serum (119). During infection by the SARS-CoV2 virus, IgG levels increase even beyond those of IgA (120). In the widely used C57BL6 mouse model, IgG, IgA, and IgM are detected in the uninfected lung parenchyma with IgG2b levels in bronchoalveolar lavage (BAL) fluid higher than those of IgA (121). Thus, multiple isotypes and subclasses are likely present in the respiratory tract for these early events.
Cross Reactive and Specific Antibodies That Recognize Mtb
The repertoire of Mtb antigens recognized by antibodies in the respiratory tract, as well as systemically, remains a critical gap in knowledge. However, BCG vaccination (32, 47, 48, 122) and exposure to environmentally prevalent non-tuberculous mycobacteria likely introduce at minimum cell wall antigens that elicit antibodies cross-reactive to Mtb (32, 123, 124). As in the gut, symbiotic microbiota from the pulmonary compartment could induce systemic IgG responses that induce protection against pathogens (125). These antibodies arising from non-self antigens complement those from self. Natural IgM and IgG have the ability to engage with carbohydrate and lipid Mtb targets to influence initial host-microbial interactions and subsequent infectious challenge (126). As the products of B-1 cells (127) that arise during immune development independent of exogenous antigens and T cells, natural IgM targeting self-antigens such as phosphorylcholine modulate autoimmunity and responses to virus via antibody mediated induction of complement (128) and efferocytosis of apoptotic cells (129, 130). In murine influenza studies, passive transfer of IgM from uninfected mice into those deficient in B-1 B cells or are unable to secrete IgM enhance survival and microbial specific IgG levels, suggestive of a role in modulating the development of the adaptive immune response in secondary lymphoid organs (131, 132). Beyond IgM, data from mouse studies suggest that natural IgG can aid in FcR mediated phagocytosis of ficolin coated gram negative and positive bacteria to impact susceptibility to infection (133, 134). Whether by specific and or cross-reactive antigen recognition, these studies suggest that localized and systemic antibodies are poised to recognize Mtb and mediate initial interactions within even an uninfected, naïve host.
In individuals who have developed an adaptive immune response to Mtb infection, the over 4000 open reading frames and the plethora of cell membrane and wall lipids and glycolipids provide a spectrum of Mtb targets to which antibodies can direct immune responses (28, 29). Because replicative and non-replicative Mtb states vary in relative abundance of these microbial antigens (135, 136), different specificities may be relevant for antibody functions in latent TB, active TB and with prior antimicrobial treatment. T cell responses to genes encoded by the dormancy regulon of Mtb are enhanced in latent infection (137) but the impact from the antibody standpoint is not known. Moreover, different clinical strains could mean that the Fab domain repertoire vary by geographical region (138). It is presumed that the majority of Mtb targets are expressed by live bacteria and infected host cells including epithelial cells (139) as well as macrophages (140). However, exosomes in the blood and granuloma also contain Mtb antigens that can activate immune cells in vitro and in vivo (141, 142). Thus, antigen specific antibody effector functions can be induced even in the absence of live Mtb.
FcRs in the Respiratory Epithelia
With aerosol transmission, the first encounter between Mtb and host likely occur with non-immune cells in the respiratory tract. At this earliest stage, the conditions that determine if the bacteria infect and cause disease are not known. Antibody Fc domain engagement of receptors aid in translocation across the lumen and induce non-immune cells to secrete local cytokines, activating resident immune cells such as alveolar macrophages. Thus, FcR on epithelial airway cells could be the first to encounter antibody-complexed Mtb to enable bacterial movement and initiate host responses. The two most abundant FcR in the respiratory tract are the neonatal FcR (FcRN) and polymeric immunoglobulin receptor (pIGR) though other FcRs that bind IgG, IgA and IgE are also expressed.
In the respiratory tract, FcRN facilitates transport of IgG across the mucosal surface (143). Its high affinity nature permits binding to monomeric IgG to guide through the endocytic excretion pathway and extend serum half-life by preventing lysosomal degradation. After low dose aerosol Mtb challenge, pulmonary bacterial burden is lower in FcRN knockout compared to wildtype mice at early but not late timepoints. However, there is an absence of differences in initial inoculum (121) and dissemination into the spleen and liver. These findings indicate that though uptake may be similar via non-Mtb specific antibodies, subsequent host responses and bacterial outcomes are transiently affected (121). Monoclonal Mtb specific IgG but not IgA increases opsinophagocytosis in FcRN expressing A549 human lung epithelial cell line (31). Thus, in vivo and in vitro data suggest that Mtb reactive IgG from prior mycobacterial exposure enhances early infection transiently via FcRN at the epithelium. The implications for outcomes in chronic disease and in the presence of Mtb reactive antibodies induced by BCG vaccination, prior Mtb or NTM infection are less clear but likely involve immune as well as epithelial cells.
Similar to FcRN, the polymeric immunoglobulin receptor (pIGR) is widely expressed in the respiratory tract. Unlike FcRN which focuses on IgG, pIGR supports the transport of dimeric IgA and, to a lesser extent, IgM from the interior basolateral surface of the epithelium to the exterior apical side. Interestingly, IgA levels are decreased in saliva but not bronchoalveolar lavage in a total mouse pIGR knockout (144). In these mice, there is an early and unsustained increase in pulmonary bacterial burden and decrease in IFNγ and TNFα after low dose aerosol infection with virulent Mtb strain H37Rv. These data suggest that the effects of non-specific IgA transported to upper respiratory tract by pIGR can be transiently protective in early infection.
Direct neutralization by Mtb specific compared to non-specific IgA in the mucosa that blocks bacterial uptake could mediate a more significant and durable effect. Monoclonal antibodies targeting the mycobacterial glycosylated lipoprotein phosphate transporter subunit PstS1 demonstrate this potential in human lung epithelial cells in vitro (31). Polyclonal antibodies induced after intranasal vaccination with PstS1 shows that in vivo protection in a high dose intranasal BCG challenge model can be generated in a pIGR dependent manner (31). That FcαR/CD89, the primary human IgA FcR, is not detected on human epithelial cells or expressed in mice points towards direct neutralization as one likely mechanism. However, other receptors for IgA including Fcα/μ receptors (145), asialoglycoprotein receptors (146), transferrin receptors (147) and M cell receptors (145, 148) have been reported to be able to bind to the IgA Fc domain. These receptors provide unexplored FcαR/CD89 independent mechanisms of non-neutralization by which Mtb specific IgA that could inhibit bacteria in the respiratory tract.
While the type I FcγRs are commonly described in cells of hematopoietic lineage, which consequently have been the focus of most functional studies, some data suggest that these FcRs can be found in non-immune cells, permitting IgG to induce non-neutralizing functions. The low affinity FcγRIII/CD16 is expressed in primary nasal epithelial cells and in vitro blocking antibody studies demonstrate a role in mediating IgG opsonized bacterial cytokine induction (149). The low affinity inhibitory FcγRIIb/CD32b but not the IgE receptor FcεRIII/CD23 is present in primary human airway smooth muscle cells (150). Understanding non-neutralizing Fc effector functions mediated by non-immune cells in the airway lumen to Mtb could provide insight into the factors that determine whether colonization or deeper infection occurs.
FcRs Mediating Macrophage Environments That Permit and Restrict Mtb Growth
Macrophages represent a quintessential niche for Mtb where bacteria grow and die. In the high dose aerosol murine infection model, tissue resident, long-lived alveolar macrophages act as initial host cell targets for Mtb (151) and contribute to the development of immune conditions that permit Mtb replication and dissemination (151, 152). Bone marrow derived monocytes and macrophages are subsequently recruited to join their embryonic tissue resident counterparts. Further infection of these populations show heterogeneity between and within the groups. Initial studies suggested an M1 and M2 paradigm to describe macrophage restrictiveness and permissiveness to Mtb (153). However, more recent data from single cell mouse and human macrophage transcriptomics suggest that the determinants of bacterial outcomes are far more complex (154, 155). Whether or not FcR mediated signaling contribute to the heterogeneity of macrophage responses to Mtb is not known, but the combinatorial diversity from engagement of the multiple low and high affinity receptors expressed in this immune cell provides this potential.
Macrophages express a plethora of FcRs that enable responsiveness to antibodies found in the blood and tissue, including Mtb lesions such as granulomas (156). High affinity FcγRI/CD64 and FcRN allow monomeric IgG to influence macrophage phenotypes. The low affinity activating FcγRIIIa/CD16a and FcγRIIa/CD32a permit further tuning with IgG immune complexes, counterbalanced by the only inhibitory receptor FcγRIIb/CD32b. In being the only immune cell other than natural killer cells that expresses a significant level of FcγRIIIa/CD16a which classically mediates ADCC, macrophages have the potential to be both effector and target cells. In being like all other immune cells where the cytosolic FcR TRIM21 is expressed, antibodies may also enable the macrophage to target intracellular Mtb. For tissue macrophages including alveolar macrophages the activating low affinity FcγRIIIa/CD16 and high affinity FcγRI/CD64 are particularly highly expressed when compared to blood monocyte derived macrophages (157, 158). Examining the link between FcRs and cell population specific responses to Mtb could clarify how macrophages permit and restrict bacterial replication.
Classic experiments show that extracellular Mtb uptake mediated by live BCG immunized rabbit serum into mouse peritoneal macrophages direct bacteria into phagosomes, though the outcomes for the bacteria and host vary (159). Trafficking of Mtb into the phagolysomal and autophagosomal compartments, induction of pro- and anti- inflammatory cytokines and likely multiple other cellular effector functions collaboratively determine intracellular bacterial fate. Much of the literature has focused on opsonophagocytosis. Thus, classical FcRs at the cell surface involved in ADCP including FcγRI/CD64, FcγRII/CD32 and, to a significantly lesser extent, FcγRIII/CD16a (47) play roles in uptake of extracellular Mtb into monocytes and likely macrophages. For the intracellular bacteria Legionella (160), the initial steps of FcR mediated entry into the macrophage govern intracellular fate, but for Mtb this remains unknown.
As important as extracellular Mtb, in vitro data suggest that antibodies can also impact intracellular bacteria, the predominant state in infection. Addition of polyclonal IgG from latent compared to active TB patients to macrophages already infected with Mtb induce lower intracellular burden (16). That these antibodies have higher affinity for FcγRIIIa/CD16a and induce higher ADCC in vitro with natural killer cells that express FcγRIIIa/CD16a suggests that a similar mechanism could be occurring with Mtb infected macrophages as target and effector cells. An alternative and complementary mechanism could be via the cytosolic E3 ubiquitin ligase TRIM21. This FcR is able to ubiquitinate antibody bound intracellular viruses and bacteria such as Salmonella for degradation (91), though for Mtb this is not yet known. Thus, antibodies through surface and cytoplasmic FcRs can impact intracellular Mtb directly or indirectly to restrict growth.
Whether antibodies induce macrophages to restrict or permit Mtb infection and replication likely results from the sum of engaging multiple high and low affinity activating and inhibitory FcRs, which could differ for extracellular and intracellular bacteria. Additional immune cell signaling pathways could also contribute. For example, Mtb components interact with Toll like receptors (TLR) (161) to induce innate macrophage responses. Cross talk between TLR and FcR signals on macrophages have been described (162). Understanding the interactions of these pathways for Mtb could highlight macrophage diversity in the context of bacteria, antibodies and pathogen associated molecular patterns (PAMPs) that exist in concert throughout infection and disease.
FcR Mediated Neutrophil Inflammation in TB
Like in macrophages, much of the literature on neutrophils in TB have focused on inflammation in active TB (3, 163). Correlations between blood transcriptional signatures with disease suggest that neutrophils mediate or reflect pathogenic inflammation. While expression levels of the high affinity FcγRI/CD64 is consistent with this, additional low affinity receptors capable of binding to IgG and IgA provide the potential for antibody mediated protective effector functions via the peripheral blood and granuloma where neutrophils surround Mtb infected macrophages.
Neutrophils have a partially overlapping FcR repertoire with macrophages. Like macrophages, the low affinity activating FcγRIIa/CD32a, and inhibitory FcγRIIIb/CD32b, as well as high affinity FcRN and TRIM21 are constitutively expressed. Unlike macrophages, high FcαR/CD89 expression characterizes these granulocytes, permitting responses to IgA. The monomeric isoform FcγRIIIb/CD16b is constitutively expressed instead of the heterooligomeric FcγRIIIa/CD16a. FcγRI/CD64 expression is not constitutive but rather induced by IFNγ (164). Thus, baseline and induced FcR permit a spectrum of neutrophil phenotypes in Mtb infection.
Transcriptomics data show that FcγRI/CD64 expression and neutrophil activation are correlates of TB disease progression across multiple human cohorts (3, 165) and mouse and non-human primate models (68). However, despite bearing the same names, murine and human CD64 differ in their binding abilities and expression patterns such that they are not functional homologues. Knockout in mice leads to a transient decrease in pulmonary Mtb at 60 days along with decreased neutrophil but not macrophage recruitment to the lungs, suggesting that FcγRI/CD64 enhances disease (68). Though there are limitations to extrapolating these mouse data to humans, increased FCGR1/CD64 copy number in individuals with active disease shows association with poorer outcomes (67). Neutrophil FcγRI/CD64 expression is a biomarker for sepsis in children and adults with bacterial infections (166). As such, FcγRI/CD64 is likely similarly a correlate of inflammation for TB.
In contrast, the expression of FcγRIIa/CD32a and FcγRIIIb/CD16b on neutrophils suggests the potential existence of an IgG mediated protective function. While ADCC is typically described as a natural killer cell FcγRIIIa/CD16a function, neutrophils can also be effector cells with FcγRIIa/CD32a being the activating receptor, negatively regulated by FcγRIIIb/CD16b as shown in tumor models (167). In TB, higher FCGR3B copy number in a subpopulation of Ethiopians is associated with HIV-TB compared to HIV alone (69), suggesting that decreased neutrophil ADCC could be associated with more severe disease in dually infected individuals.
Beyond IgG, studies using human FcαR CD89 transgenic mice point towards the potential for IgA mediated FcR functions to impact Mtb. Opsonization of H37Rv with human monoclonal IgA targeting the alpha crystallin Mtb protein HspX prior to high dose intranasal challenge of these mice leads to decreased pulmonary bacterial burden early after infection compared to non-transgenic littermates. Thus, beyond direct binding, IgA Fc mediated non-neutralizing functions through FcαR/CD89 can impact events that affect the development of adaptive immunity (168). Whether protection occurs via neutrophils, monocytes/macrophages or other immune cells, the durability of such protection and how much can be translated into humans remain to be clarified (169). Intriguingly, IgA monoclonals can also induce neutrophil-mediated ADCC (170), providing a plausible mechanism by which antibodies can inhibit Mtb. In contrast, there is evidence that both IgA and IgG can induce neutrophil and monocyte mediated trogocytosis (171, 172), a phagocyte nibbling process which can contribute to the spread of intracellular organisms such as Francisella tularensis and Salmonella from infected to uninfected cells (173). Thus, some antibody induced neutrophil functions could contribute to Mtb pathogenesis. Indeed, NETosis is initiated by many receptors, amongst which FcγR (174, 175) and FcαR (81) are members. As NET formation is detected in necrotic lung lesions of TB patients which promote bacterial growth (163), antibody mediated neutrophil activation can be as locally pathologic as it is systemically.
FcRs Influencing Dendritic Cell Antigen Presentation
Dendritic cells are the most efficient professional APCs that prime T cells. As such, enhancement of dendritic cell functions in the context of vaccination can in animal models produce sterilizing protection for Mtb (176). Studies in other infectious disease and cancer models show that antibodies through FcR on dendritic cells bridge the gap between innate and adaptive responses, but how this can confer long lasting immune memory for TB in a physiologically relevant setting continues to be re-evaluated.
Similar to macrophages, dendritic cells express a plethora of receptors that permit responsiveness to antibodies in the lung upon recruitment in Mtb infection. Monocyte derived dendritic cells and macrophages express baseline high levels of activating FcγRs, and conventional and plasmacytoid dendritic cells can also express the only inhibitory FcγR FcγRIIb/CD32b (177). By engaging both activating FcγRIIa/CD32a and inhibitory FcγRIIb/CD32b low affinity signaling, IgG can influence antigen uptake and presentation, maturation and cytokine production. These activities likely direct priming either at the primary pulmonary site of Mtb infection or in the draining lymph nodes as adaptive immunity develops (70, 87–89, 178). Moreover, in addition to its recycling function, the high affinity FcRN in concert with FcγRIIa/CD32a can regulate cross presentation of IgG immune complexes (179). Exactly how this occurs in TB is unclear. FcRN knockout mice have enhanced Mtb infected CD103+ dendritic cells and CD4 T cell priming early at day 14 of infection, with decreased Mtb burden on days 14 and 28. However, it appears that this effect is transient, leading to similar pulmonary burdens on day 56 (121).
An anti-tumor T cell “vaccinal effect” through engagement of FcγRIIa/CD32a on dendritic cells can be induced in a human FCGR transgenic mouse model with monoclonal antibodies. This is in addition to the transient clearance of tumor cell targets by macrophage FcγRIIIa/CD16a mediated ADCC (180). This FcγRIIa/CD32a dendritic cell mediated effect is even more pronounced in the context of influenza infection. Fc engineered antibodies show that binding to FcγRIIa/CD32a on dendritic cells increases CD40, CD80 and CD86 expression that induces protective CD8 T cell activation while FcgRIIIa/CD16a on macrophages had a limited role (181). A direct link between antibody mediated effects on dendritic cell antigen presentation and B and T cell maturation have yet to be shown for Mtb. However, any protective in vitro effect of polyclonal IgG on dendritic cells from humans highly exposed to Mtb is dependent on the presence of MHC-II and CD4 T cells in whole blood (49). This suggests that the interaction is plausible in natural exposure and could be leveraged by vaccines.
Regulation by the Inhibitory FcγRIIb/CD32b
Activating FcR mediated immune responses in the absence of checks and balances lead to autoimmune induced pathology. Though there are multiple regulatory points, FcγRIIb/CD32b is the only inhibitory FcγR and is the only FcγR well conserved between humans and mice (182). The high sequence similarity between the extracellular domains of FCGRIIB/CD32B, FCGRIIA/CD32A and FCGRIIC/CD32C and the paucity of specific monoclonal antibody probes limit experimental designs. However, the knockout mouse model has provided some hints as to the importance of inhibitory FcγR regulation in TB.
In response to low dose Mtb aerosol infection, mice genetically deficient in FcγRIIb/CD32b have increased CD4 T cell IFNγ production, decreased bacterial burden in the lungs and spleen, and decreased neutrophilic inflammation at day 30 (13). Thus, in early infection, activating FcR signaling that enhance CD4 T cells are protective. However, the implications for chronic disease, which is more characteristic of human TB, are unclear as the autoimmune phenotype of these knockout mice confound the interpretation of Mtb survival experiments that last over one year.
Because FcγRIIb/CD32b is detectable on dendritic cells and subpopulations of monocytes, macrophages and neutrophils, it is likely that the cumulative impact of the only inhibitory FcγR for TB reflects functions from all of these immune cells. In human (70) and mouse dendritic cells (88), FcγRIIb/CD32b counter balances FcγRIIa/CD32a activation of maturation, secretion of inflammatory cytokines and MHC-I and MHC-II antigen presentation (87). Thus, loss of FcγRIIb/CD32b in dendritic cells likely promotes the development of a Th1 response able to inhibit Mtb. However, FcγRIIb/CD32b in follicular dendritic cells counterintuitively enhances B cell activation by multimerizing antigens to crosslink multiple B cell receptors (BCRs) (183). The existence of this T cell independent mechanism of antibody induction suggests stark differences within dendritic cell subsets that could have implications for long lasting immunity. For monocytes, macrophages and other granulocytes, the presence of activating FcRs determine the impact of FcγRIIb/CD32b (74, 75, 156). This further argues that FcγRIIb/CD32b functions are likely highlighted primarily in the context of stimulatory factors as opposed to acting in isolation.
In B cells, FcγRIIb/CD32b negatively regulates BCR signaling, expansion and plasma cell differentiation (184), suggesting that antibody production is also inhibited. Thus, Mtb specific antibodies generated in knockout mice could be higher when compared to wildtype. Higher levels of IgG, IgM and IgA could enhance immune cell effector functions, further contributing to anti-Mtb activities in the absence of an inhibitory checkpoint.
Finally, there is emerging evidence from adoptive transfer experiments that FcγRIIb/CD32b can act as a CD8 T cell checkpoint inhibitor involved in anti-tumor immunity (185). Extrapolation for Mtb would implicate an involvement of the inhibitory FcR more directly in the regulation of cytotoxic T cell functions that could be protective.
Influencing Adaptive Immunity
Fc Receptors on T Cells
While MHC-I and MHC-II antigen presentation and expression of co-stimulatory ligands by APCs and B cells represent the primary paths through which CD4 and CD8 T cells are activated, there is some data to suggest that activating FcR can more directly influence T cells. T cell activation and differentiation has been associated with expression of the low affinity FcR, FcγRII/CD32, including both CD4 (186–188) and CD8 T cells (181, 189). On a small subset of CD4 T cells from blood and lymphoid tissues of humans and Rhesus macaques with and without HIV/SIV infection, proliferation, differentiation and cytokine production could be enhanced by immune complex activation of FcγRIIa/CD32a (186, 187). Interestingly, IgM binding to the Fcμ receptor on peripheral human T cells increases T cell receptor (TCR) and CD28 coreceptor expression, thereby lowering the threshold for T cell activation (190). Thus, high levels of IgM such as that induced by intravenous BCG could provide co-stimulation through FcR binding and enhance early T cell responses to lead to protection against Mtb (32). Evaluation of these T cell subsets in TB would provide greater clarity as to whether FcR binding could serve a co-stimulatory function in vivo when T cells are activated naturally through TCR-dependent mechanisms.
B Cells in Antigen Presentation to T Cells
The interactions between naïve T cells and APCs within the T cell zones of secondary lymphoid organs determine the repertoire of TCRs, which is governed by antigen availability and TCR binding characteristics (191). B cells recognize and respond to the structure of 3-dimensional antigens, including the simultaneous binding of non-sequential, distant residues which become juxtaposed upon protein folding. However, T cells recognize sequential amino acids within short peptides only when they are presented in the context of the MHC molecule. Since antigens are bound to the BCR, endocytosed, processed and presented as peptides, antibodies produced by differentiated plasma cells might affect the activation of peptide-specific T cells targeting the same Mtb protein. Thus, B cells could present antigens for the purpose of activating conventional T cells in a manner similar to their presentation of antigens to T follicular helper (Tfh) cells (Figure 3B) (111).
The most well-characterized interactions between B cells and T cells occurs in the context of B cell antigen presentation to Tfh cells in secondary lymphoid organs. This facilitates B cell activation and differentiation into antibody-producing plasma cells. However, the professional antigen-presenting capacity of B cells together with their strategic localization in and around sites of T cell priming in secondary lymphoid organs, granulomas and iBALT after Mtb infection suggests a broader role, including participation in Mtb-specific T cell activation.
Classically, CD4 T cell priming in response to Mtb has been shown to occur when T cells are activated by conventional dendritic cells in lymph nodes after CCR2+ monocyte-derived APCs transport live Mtb from the lung to the draining lymph nodes (178, 192, 193). After priming, effector T cells traffick to the lungs and secrete IFNγ and cytokines in response to Mtb. However, depletion studies in animal models identified a role for B cell antigen presentation in supporting optimal T cell responses in infectious disease and autoimmune models, suggesting that this too could occur in the context of TB. In a mouse model of adjuvanted peptide vaccination, B cell presentation of peptides on MHC-II was found to be necessary to enhance CD4 T cell expansion and IL2 production (106). B cell depletion using an anti-CD20 monoclonal antibody in mice significantly reduces total baseline numbers of naïve, effector, and regulatory CD4 and CD8 T cells. In the context of lymphocytic choriomeningitis virus (LCMV) Armstrong infection, this leads to increased viral load, an effect which is rescued by infusion of LCMV-specific TCR transgenic CD8 T cells (194). Similarly, B cell depletion prior to infection with Trypanosoma cruzi reduces subsequent induction of total and parasite-specific CD8 T cells (195), and B cells are critical in the development of antigen-specific effector and memory CD4 T cell responses to Listeria (196, 197) and Salmonella (198). Finally, at least two studies have shown a supporting role for B cells in priming autoreactive T cell responses in mouse models of autoimmune diabetes, arthritis and lupus independent of plasma cell differentiation and antibody production (197, 199). These data indicate that B cells assist antimicrobial and autoimmune T cell responses either directly or indirectly.
An indirect mechanism of antigen presentation to T cells by B cells is secretion of MHC-II containing exosomes or extracellular vesicles. In this scenario B cells activate T cells in an HLA-DR dependent manner (200–202). T cell stimulation by extracellular vesicles is dependent on expression of the costimulatory receptor CD28 (203). This mechanism of antigen presentation is of particular interest in TB as both extracellular microvesicles and exosomes from Mtb infected macrophages and dendritic cells are sources of antigen that can directly or indirectly activate antigen-specific CD4 and CD8 T cells (204–208). Though not yet studied in TB, B cell exosomes may serve a similar function to provide alternative ways to activate T cells to compensate for Mtb immune evasion in macrophages (209–211). It is also possible that B cell exosomes provide a means of transferring units of MHC class II-peptide complexes between different cells (212) (Figure 3C). Thus, MHC-II provides multiple lines of B cell-T cell communications.
In addition to T and B cells, tingible body macrophages (TBMs) are found in the germinal centers in lymph nodes, spleen and iBALT from the non-human primate model of TB (213). TBMs are specialized macrophages that engulf apoptotic B cells during affinity maturation (214, 215). In this manner, TBMs prevent the loading of self-antigens from cell debris immune complexes onto follicular dendritic cells that present to B cells (216, 217). Moreover, studies using ovalbumin-specific T cell hybridomas show that antigen-laden TBMs suppress the ability of B cells to activate T cells (215), thereby preventing autoimmune responses. In the mouse model of TB, dendritic cells uptake extracellular vesicles from apoptotic macrophages infected with Mtb and stimulate CD8 T cell responses in an MHC-I or CD1-dependent manner (218). These data imply a role for scavenger APCs in presenting Mtb antigens to regulate T cell responses. Cross-priming of CD8 T cells from APCs is likely important for the recognition and killing of many pathogen infected cells. In TB, acquisition of antigens via efferocytosis and uptake of extracellular vesicles enhances the number of antigen specific CD8 T cells that recognize Mtb infected macrophages (130, 219, 220). TBM mediated efferocytosis and T cell cross-priming could explain the association of B cell follicles with protection against active disease in the non-human primate model of TB (213).
B Cell Follicle-Like Structures
Like a granuloma, the iBALT is a tertiary lymphoid structures that enrich and facilitate interaction between myeloid cells, B cells, T cells and non-hematopoietic cells (107, 221). Adjacent to granulomas, the iBALT is a pulmonary structure consisting of B cell follicles and components of the lymph node including high endothelial venules and connection the lymphatic drainage (93, 99, 107, 222–225). Induced early after infection, the follicles contain IgD+ B cells clustered around a network of follicular dendritic cells. These stromal cells produce IL1α, express the lymphotoxin α (LTα) receptor and secrete CXCL13 (225) to help recruit immune cells to form the iBALT (226). The iBALT is associated with protection in the setting of Mtb, serving as both a lymphoid organ and a lung-resident source of locally-activated, antigen-specific T and B cells primed for rapid responses (107, 213).
The iBALT is a feature of type 1 immune responses and is reported to regulate type 2 and type 17 responses. A recent study in a mouse model of asthma found that pre-existing iBALT delayed the onset of Th2 trafficking and related inflammation (227). In a mouse model of influenza infection, the iBALT offers protection through supporting antigen specific CD8 T cells, humoral responses and the formation of immunological memory even in the absence of spleen and lymph nodes (228, 229). The iBALT has also been shown to recruit dendritic cells harboring antigens and naïve T cells, leading to co-localization and T cell priming (230). For TB, the association of iBALT formation with protective phenotypes in human lung tissue as well as in the mouse and non-human primate models point towards a protective feature against active disease (93, 213, 231, 232).
In TB, the extent of T and B cell priming in the iBALT versus lung-draining lymph nodes has been incompletely explored. The priming of naïve antigen specific CD8 T cells after non-replicating viral vector infection has been demonstrated in adoptive cell transfer models in mice (230). However, in the mouse model of TB, priming of naïve T cells in the lung is undetectable (178, 192, 233). Memory T cell priming in the lungs has only been shown after the intratracheal transfer of antigen-laden dendritic cells (234). Yet previously activated T and B cells do traffick through the iBALT in other infectious models (229). Tfh cells which are critical to germinal center formation and B cell differentiation into antibody-producing plasma cells are normally found in secondary lymphoid organs lymph node and spleen and may have the same role in iBALT (107, 235). T cells that express properties of both Th1 and Th17 cells, along with Tfh cells are found in the lung, express CXCR5, produce IFNγ and/or IL17 and are associated with iBALT formation and control of Mtb infection (93, 107). For protection against TB, Tfh cells in the lung support germinal center responses involved in (1) the development of memory B cells and differentiation into antibody producing plasma cells, (2) the regulation of pathologic immune responses such as neutrophilia and cavity formation, (3) the classical priming of T cells locally, and (4) the non-classical activation of CD8 and CD1-restricted T cells by cross-priming and efferocytosis (130, 218, 219).
The formation of iBALT near bronchi or in the lung interstitium is influenced by the infecting microbe and host response (236, 237). The recruitment of dendritic cells, macrophages, and innate lymphoid cells leads to cytokine and chemokine production that enlists B and T cells (223, 230). More specifically, in early Mtb infection, type 3 innate lymphoid cells (ILC3s) (223) and production of IL23, IL17, IL22 and CXCL13 direct B and T cells into the lung of the mouse model (238). Within the iBALT, conventional dendritic cells and T cells localize around B cell follicles into germinal center-like structure. Over time distinct T cell and B cell zones are formed (228, 230). Thus, lymphocytes expressing CCR7 and CXCR5 traffick to and organize the iBALT (93, 239). In addition, CCL19, CCL21 and CXCL13 from follicular dendritic cells are critical to establishing structure (228). These same axes (CCR7-CCL19/CCL21 and CXCL13-CXCR5) are required for T and B cell homing, antigen presentation, T cell activation, IFNγ production and control of Mtb growth (93, 222, 240). Finally, a recent transposon mutagenesis screen in the non-human primate model of TB identified gene associated with production of Mtb cell wall lipids with the formation of iBALT (98). Together, these studies show that the extent of protection offered by the iBALT (241) is likely dependent on both the infecting organism and the host.
Complexity of Immunity in TB
Protection and Sterilizing Immunity
One goal of an effective TB vaccine is to prevent infection or provide sterilizing immunity to the host. However, generating a sufficiently robust and durable immune response that neutralizes and eradicates Mtb could come at a survival cost to the host. To this point, enhancement of CD4 T cell responses at the level of immune checkpoints such as PD-1 counterintuitively enhances disease and decreases survival in mouse studies (242). In some patients, anti-PD-1 monoclonal antibodies used to treat cancer precipitated reactivation TB from latently infected individuals (242). Indeed, post-hoc analyses of the MVA85A clinical trials demonstrate that higher frequency of HLA-DR+ activated CD4 T cells can be associated with increased risk of TB while higher Ag85A IgG titers correlate with protection (243). Thus, strategies that release the break on T cells unilaterally could provide more harm than benefit. Nevertheless, a cytomegalovirus vectored vaccine generating antigen-specific T cell but no antibody responses prevented TB in nearly half of the Mtb-challenged non-human primates (244). Elucidating how immune responses can provide benefit with minimal cost will likely have broad applicability to vaccines across many infectious diseases.
Most TB vaccines in clinical development now incorporate both antibody and T cell responses, though which are correlates of protection and which of disease remain to be fully clarified. These vaccines include but are not limited to the M72-AS01e subunit vaccine containing a recombinant fusion protein of Mtb32a and Mtb39a (245) and BCG in multiple forms (246). While much has been evaluated involving the classic intradermal delivery, revaccination (247, 248) and the use of the recombinant VPM1002 expressing listerolysin-O to enhance CD8 T cell responses (249, 250) are variations currently in clinical trials. In pre-clinical models there is attention paid to delivery route- intravenous (32, 251), intranasal, aerosol, intratracheal and endobronchial (252). For both BCG and MTBVAC, an attenuated Mtb strain lacking the phoP and fadD26 virulence factors, antigen specific IgG are detected in pulmonary but not intradermal vaccinated animals and facilitate Mtb opsonophagocytosis in vitro (253). Thus, independent of what is induced during natural infection and disease, vaccine strategies that incorporate a greater breadth and depth of responses including B cells, antibodies and trained innate immunity (254, 255) could complement T cell responses to more effectively eradicate Mtb (32, 251, 256, 257).
Pathology and Disease
A second goal of an effective vaccine is to prevent TB disease and lung pathology. This, notably, does not necessarily imply eradication of the bacteria. In humans, there is increasing recognition that latent TB may represent a protective state (2, 258). Indeed only 5-10% of individuals progress to active disease. Yet the immune mechanisms by which the transition between latent and active disease occurs in humans and the corollary of how the remaining 90-95% of individuals maintain the asymptomatic latent state – whether this be with dormant or eradicated bacteria (2) – are not known. This phenotype has been difficult to study in animal models. The classic murine model recapitulates active TB but not latent infection. Furthermore, there is a disconnect between Mtb burden, dissemination and survival observed in passive antibody transfer studies of mice (259, 260). Thus, mechanisms by which asymptomatic Mtb latent infection is sustainably induced could be leveraged for vaccine design. To this point, antibodies have the potential to enhance disease whether directly through increased replication such as in Dengue hemorrhagic fever and Leishmania or indirectly through inducing a dysregulated immune response such as in COVID-19 (261). Understanding whether and how antibodies can enhance TB disease by mediating pathological inflammation in immune reconstitution inflammatory syndrome or simply supporting dissemination (262, 263) could provide novel host directed therapeutic targets.
Several candidate subunit TB vaccines aim to prevent not only initial infection but also pathology once infection has occurred. These include, but are not limited, to H56:IC31 that encompasses Ag85B, ESAT6 and Rv2660c (264), and the ID93 + GLA-SE vaccine which contains a TLR4 agonist formulated with a recombinant fusion protein of the Mtb virulence factors (esxV/Rv3619, esxW/Rv3620, PPE42/Rv2608) and Mtb latency associated protein Rv1813 (256, 265, 266). Administration of these vaccines in animal models induces robust antigen specific immune responses that, when combined with antibiotics, is linked to decreased bacterial burden and lung pathology in comparison to antibiotics alone. The highly effective mRNA vaccines BNT162b2 and mRNA-1273 successfully prevents both infection and serious disease with spike specific T cell and antibody responses against SARS-CoV2 (267, 268). As such, mRNA platforms provide promising avenues for TB vaccines in generating immune responses that both protect against Mtb infection and prevent disease pathology.
Challenges in Modeling Tuberculosis
Inherent differences between the widely used inbred mouse models and humans are critical to acknowledge when extrapolating data (269). Antibody translocation and recycling functions of FcRN and pIGR in humans are recapitulated in the murine model. Similarly, the non-classical IgG binding TRIM21, the single IgM receptor (FcμR), the two receptors for IgM and IgA (pIGR and Fcα/μR/CD351) and the two receptors that bind to IgE (FcεRI and FcεRII) in humans are also found in mice. Unique to humans is FcαRI/CD89 which binds only IgA. For the classical IgG receptors (24), the expression of the activating FcγRI/CD64 in mice is limited to monocytes, macrophages and dendritic cells whereas in humans the spectrum also includes neutrophils. Conversely, FcγRIIIa/CD16 in humans is restricted to natural killer cells, monocytes and macrophages while in mice the spectrum also includes neutrophils, dendritic cells, basophils, eosinophils and mast cells. The only inhibitory Fc receptor, FcγRIIb/CD32b, is detected primarily on B cells and other myeloid lineage cells in mice but in a more limited capacity in humans. The activating FcγRIIa/CD32a, FcγRIIc/CD32c and FcγRIIIb/CD16b exist exclusively in humans while FcγRIV/CD16.2 is unique to mice. In addition, there is no clear equivalence between the FcR binding capacity of the human subclasses IgG1, 2, 3 and 4 and mouse IgG1, 2a/c, 2b and 3. Finally, the mouse IgG glycome partially overlaps with that of humans, with the functional implications of the differences not yet defined (36, 270). Mice with human FcR and signaling adaptors (271) as well as immunoglobulin genes (272) provide paths towards bridging the species gap. However, ensuring similarities in expression patterns to recapitulate immune effector functions at baseline and during infection remains to be fully flushed out as levels of each receptor influence each other.
For TB, the widely used low dose aerosol inbred mouse model does not recapitulate latent infection and poorly captures the phenotypic heterogeneity observed in humans (9, 273). The absence of some mechanisms of protection involved in latency may be one reason why results from vaccines studies in mice do not always directly translate to humans. For example, the promising 1-2 fold log reduction in colony forming units in the mouse model with BCG is reflected by reduction of disseminated TB in the pediatric population and variable impact on adult pulmonary TB, the most common form (274). Whether the aforementioned differences in antibody responses between mice and humans could be a factor in this gap is not known. Questions to this point could be addressed with diverse outbred mice and or new models of TB such as ultra-low dose aerosol infection that have the potential to reflect latent infection more closely than the current most widely used approach (275–277). Nevertheless, the canonical (and tractable) inbred mouse model has generated data that has formed the foundations of our understanding of antibody Fc effector functions in autoimmunity and monoclonal antibody fields. Building on this knowledge to address specific questions in the context of Mtb that could be orthogonally tested in non-human primates and or ex vivo work with patient samples provide paths to dissect physiologically relevant mechanisms of disease.
Challenges in Defining Human TB
The development and exploitation of robust imaging, microbiology and immune correlates techniques to characterize the spectrum of TB observed clinically has transformed the classic framework of uninfected, latent infection and active disease. Now it is possible to begin to better identify individuals with high exposure, initial infection, the quiescent bacterial state, subclinical infection, chronic smoldering disease that progresses and regresses, overt active disease, bacterial persistence after treatment and bacterial cure (41, 278–283).
Beyond pulmonary TB, extrapulmonary disease involving hematogenous dissemination to the central nervous system, bone marrow and other organs characterize a subset of cases. The risk of multiorgan involvement increases with immunosuppression. However, the precise mechanisms by which these additional TB states develop are largely unknown but likely involve both bacterial and host protective and pathologic immune factors (284).
Notably, not all immunosuppression leads to TB. Over 90% of TST+ individuals do not develop active TB after receiving anti-TNFα therapy, solid organ or hematopoietic stem cell transplantation (2). Clinical observations in heavily immunosuppressed adults such as these, particularly in the absence of routine antibiotic prophylaxis for TB, indicate that a proportion had elicited sterilizing immunity and cleared the infection. In neonates, accidental delivery of live virulent Mtb contaminating the BCG vaccine in the pre-antibiotic era led to death in only 29% of the cases and the surviving 70% had significant variation in clinical phenotypes (285). These observations demonstrate that there are likely multiple paths to protection that leverage a diverse array of innate and adaptive immune responses. Thus, in addition to CD4 T cell production of IFNγ, the distinct presence of B cells in granulomas in the Mtb infected lung as well as B cell follicles and germinal centers in the adjacent iBALT raise the possibility that their functions influence outcomes. Similarly, the presence of antibodies amidst the FcR bearing monocytes, macrophages, neutrophils, dendritic cells and T cells recruited to an Mtb lesion points towards Fab and Fc domain mediated effector functions that locally coordinate host responses to bacteria. In revisiting the paradigm of protection and disease, linking diverse immune responses that include antibodies, B and T cells will enable the re-examination of the heterogenous spectrum of human TB.
Author Contributions
LL and SC drafted and wrote the manuscript, and approved the submitted version.
Funding
SC is supported by University Hospitals Cleveland Medical Center. LL is supported by the National Institutes of Health under Award Number R01AI158858 and the Disease Oriented Scholars Award at UT Southwestern Medical School.
Conflict of Interest
The authors declare that the research was conducted in the absence of any commercial or financial relationships that could be construed as a potential conflict of interest.
Publisher’s Note
All claims expressed in this article are solely those of the authors and do not necessarily represent those of their affiliated organizations, or those of the publisher, the editors and the reviewers. Any product that may be evaluated in this article, or claim that may be made by its manufacturer, is not guaranteed or endorsed by the publisher.
Acknowledgments
We thank Patricia Grace for her thoughtful review and comments, Taylor Buckholz for help with graphical illustrations and Ann Mcdonald for editorial and reference assistance.
References
2. Behr MA, Edelstein PH, Ramakrishnan L. Is Mycobacterium Tuberculosis Infection Life Long? BMJ (2019) 367:l5770. doi: 10.1136/bmj.l5770
3. Berry MP, Graham CM, McNab FW, Xu Z, Bloch SA, Oni T, et al. An Interferon-Inducible Neutrophil-Driven Blood Transcriptional Signature in Human Tuberculosis. Nature (2010) 466(7309):973–7. doi: 10.1038/nature09247
4. Malherbe ST, Shenai S, Ronacher K, Loxton AG, Dolganov G, Kriel M, et al. Persisting Positron Emission Tomography Lesion Activity and Mycobacterium Tuberculosis mRNA After Tuberculosis Cure. Nat Med (2016) 22(10):1094–100. doi: 10.1038/nm.4177
5. Beltran CGG, Heunis T, Gallant J, Venter R, du Plessis N, Loxton AG, et al. Investigating Non-Sterilizing Cure in TB Patients at the End of Successful Anti-TB Therapy. Front Cell Infect Microbiol (2020) 10:443. doi: 10.3389/fcimb.2020.00443
6. Lin PL, Maiello P, Gideon HP, Coleman MT, Cadena AM, Rodgers MA, et al. PET CT Identifies Reactivation Risk in Cynomolgus Macaques With Latent M. Tuberculosis. PloS Pathog (2016) 12(7):e1005739. doi: 10.1371/journal.ppat.1005739
7. Lin PL, Ford CB, Coleman MT, Myers AJ, Gawande R, Ioerger T, et al. Sterilization of Granulomas is Common in Active and Latent Tuberculosis Despite Within-Host Variability in Bacterial Killing. Nat Med (2014) 20(1):75–9. doi: 10.1038/nm.3412
8. Ordonez AA, Tucker EW, Anderson CJ, Carter CL, Ganatra S, Kaushal D, et al. Visualizing the Dynamics of Tuberculosis Pathology Using Molecular Imaging. J Clin Invest (2021) 131(5):e145107. doi: 10.1172/JCI145107
9. Barry CE 3rd, Boshoff HI, Dartois V, Dick T, Ehrt S, Flynn J, et al. The Spectrum of Latent Tuberculosis: Rethinking the Biology and Intervention Strategies. Nat Rev Microbiol (2009) 7(12):845–55. doi: 10.1038/nrmicro2236
10. Flynn JL, Chan J, Triebold KJ, Dalton DK, Stewart TA, Bloom BR. An Essential Role for Interferon Gamma in Resistance to Mycobacterium Tuberculosis Infection. J Exp Med (1993) 178(6):2249–54. doi: 10.1084/jem.178.6.2249
11. Orme IM, Roberts AD, Griffin JP, Abrams JS. Cytokine Secretion by CD4 T Lymphocytes Acquired in Response to Mycobacterium Tuberculosis Infection. J Immunol (1993) 151(1):518–25.
12. Nunes-Alves C, Booty MG, Carpenter SM, Jayaraman P, Rothchild AC, Behar SM. In Search of a New Paradigm for Protective Immunity to TB. Nat Rev Microbiol (2014) 12(4):289–99. doi: 10.1038/nrmicro3230
13. Maglione PJ, Xu J, Chan J. B Cells Moderate Inflammatory Progression and Enhance Bacterial Containment Upon Pulmonary Challenge With Mycobacterium Tuberculosis. J Immunol (2007) 178(11):7222–34. doi: 10.4049/jimmunol.178.11.7222
14. Maglione PJ, Xu J, Casadevall A, Chan J. Fc Gamma Receptors Regulate Immune Activation and Susceptibility During Mycobacterium Tuberculosis Infection. J Immunol (2008) 180(5):3329–38. doi: 10.4049/jimmunol.180.5.3329
15. Phuah J, Wong EA, Gideon HP, Maiello P, Coleman MT, Hendricks MR, et al. Effects of B Cell Depletion on Early Mycobacterium Tuberculosis Infection in Cynomolgus Macaques. Infect Immun (2016) 84(5):1301–11. doi: 10.1128/IAI.00083-16
16. Lu LL, Chung AW, Rosebrock TR, Ghebremichael M, Yu WH, Grace PS, et al. A Functional Role for Antibodies in Tuberculosis. Cell (2016) 167(2):433–43.e14. doi: 10.1016/j.cell.2016.08.072
17. Lu LL, Das J, Grace PS, Fortune SM, Restrepo BI, Alter G. Antibody Fc Glycosylation Discriminates Between Latent and Active Tuberculosis. J Infect Dis (2020) 222(12):2093–102. doi: 10.1093/infdis/jiz643
18. Grace PS, Dolatshahi S, Lu LL, Cain A, Palmieri F, Petrone L, et al. Antibody Subclass and Glycosylation Shift Following Effective TB Treatment. Front Immunol (2021) 12:679973. doi: 10.3389/fimmu.2021.679973
19. Glatman-Freedman A, Casadevall A. Serum Therapy for Tuberculosis Revisited: Reappraisal of the Role of Antibody-Mediated Immunity Against Mycobacterium Tuberculosis. Clin Microbiol Rev (1998) 11(3):514–32. doi: 10.1128/CMR.11.3.514
20. Kimby E. Tolerability and Safety of Rituximab (MabThera). Cancer Treat Rev (2005) 31(6):456–73. doi: 10.1016/j.ctrv.2005.05.007
21. Janssen LMA, van der Flier M, de Vries E. Lessons Learned From the Clinical Presentation of Common Variable Immunodeficiency Disorders: A Systematic Review and Meta-Analysis. Front Immunol (2021) 12:620709. doi: 10.3389/fimmu.2021.620709
22. Steingart KR, Henry M, Laal S, Hopewell PC, Ramsay A, Menzies D, et al. A Systematic Review of Commercial Serological Antibody Detection Tests for the Diagnosis of Extrapulmonary Tuberculosis. Postgrad Med J (2007) 83(985):705–12. doi: 10.1136/thx.2006.075754
23. Kramnik I, Beamer G. Mouse Models of Human TB Pathology: Roles in the Analysis of Necrosis and the Development of Host-Directed Therapies. Semin Immunopathol (2016) 38(2):221–37. doi: 10.1007/s00281-015-0538-9
24. Bruhns P. Properties of Mouse and Human IgG Receptors and Their Contribution to Disease Models. Blood (2012) 119(24):5640–9. doi: 10.1182/blood-2012-01-380121
25. Marco H, Smith RM, Jones RB, Guerry MJ, Catapano F, Burns S, et al. The Effect of Rituximab Therapy on Immunoglobulin Levels in Patients With Multisystem Autoimmune Disease. BMC Musculoskelet Disord (2014) 15:178. doi: 10.1186/1471-2474-15-178
26. Bergantini L, d'Alessandro M, Cameli P, Vietri L, Vagaggini C, Perrone A, et al. Effects of Rituximab Therapy on B Cell Differentiation and Depletion. Clin Rheumatol (2020) 39(5):1415–21. doi: 10.1007/s10067-020-04996-7
27. Greiff V, Miho E, Menzel U, Reddy ST. Bioinformatic and Statistical Analysis of Adaptive Immune Repertoires. Trends Immunol (2015) 36(11):738–49. doi: 10.1016/j.it.2015.09.006
28. Cole ST, Brosch R, Parkhill J, Garnier T, Churcher C, Harris D, et al. Deciphering the Biology of Mycobacterium Tuberculosis From the Complete Genome Sequence. Nature (1998) 393(6685):537–44. doi: 10.1038/31159
29. Daffe M, Draper P. The Envelope Layers of Mycobacteria With Reference to Their Pathogenicity. Adv Microb Physiol (1998) 39:131–203. doi: 10.1016/S0065-2911(08)60016-8
30. Fischinger S, Cizmeci D, Shin S, Davies L, Grace PS, Sivro A, et al. A Mycobacterium Tuberculosis Specific IgG3 Signature of Recurrent Tuberculosis. Front Immunol (2021) 12:729186. doi: 10.3389/fimmu.2021.729186
31. Zimmermann N, Thormann V, Hu B, Kohler AB, Imai-Matsushima A, Locht C, et al. Human Isotype-Dependent Inhibitory Antibody Responses Against Mycobacterium Tuberculosis. EMBO Mol Med (2016) 8(11):1325–39. doi: 10.15252/emmm.201606330
32. Irvine EB, O'Neil A, Darrah PA, Shin S, Choudhary A, Li W, et al. Robust IgM Responses Following Intravenous Vaccination With Bacille Calmette-Guerin Associate With Prevention of Mycobacterium Tuberculosis Infection in Macaques. Nat Immunol (2021) 22(12):1515–23. doi: 10.1038/s41590-021-01066-1
33. Jones MB, Oswald DM, Joshi S, Whiteheart SW, Orlando R, Cobb BA. B-Cell-Independent Sialylation of IgG. Proc Natl Acad Sci USA (2016) 113(26):7207–12. doi: 10.1073/pnas.1523968113
34. Dougher CWL, Buffone A Jr, Nemeth MJ, Nasirikenari M, Irons EE, Bogner PN, et al. The Blood-Borne Sialyltransferase ST6Gal-1 Is a Negative Systemic Regulator of Granulopoiesis. J Leukoc Biol (2017) 102(2):507–16. doi: 10.1189/jlb.3A1216-538RR
35. Manhardt CT, Punch PR, Dougher CWL, Lau JTY. Extrinsic Sialylation is Dynamically Regulated by Systemic Triggers In Vivo. J Biol Chem (2017) 292(33):13514–20. doi: 10.1074/jbc.C117.795138
36. Schaffert A, Hanic M, Novokmet M, Zaytseva O, Kristic J, Lux A, et al. Minimal B Cell Extrinsic IgG Glycan Modifications of Pro- and Anti-Inflammatory IgG Preparations In Vivo. Front Immunol (2019) 10:3024. doi: 10.3389/fimmu.2019.03024
37. van de Bovenkamp FS, Derksen NIL, Ooijevaar-de Heer P, van Schie KA, Kruithof S, Berkowska MA, et al. Adaptive Antibody Diversification Through N-Linked Glycosylation of the Immunoglobulin Variable Region. Proc Natl Acad Sci USA (2018) 115(8):1901–6. doi: 10.1073/pnas.1711720115
38. Cobb BA. The History of IgG Glycosylation and Where We are Now. Glycobiology (2020) 30(4):202–13. doi: 10.1093/glycob/cwz065
39. Tanigaki K, Sacharidou A, Peng J, Chambliss KL, Yuhanna IS, Ghosh D, et al. Hyposialylated IgG Activates Endothelial IgG Receptor FcgammaRIIB to Promote Obesity-Induced Insulin Resistance. J Clin Invest (2018) 128(1):309–22. doi: 10.1172/JCI89333
40. Peng J, Vongpatanasin W, Sacharidou A, Kifer D, Yuhanna IS, Banerjee S, et al. Supplementation With the Sialic Acid Precursor N-Acetyl-D-Mannosamine Breaks the Link Between Obesity and Hypertension. Circulation (2019) 140(24):2005–18. doi: 10.1161/CIRCULATIONAHA.119.043490
41. Lu LL, Smith MT, Yu KKQ, Luedemann C, Suscovich TJ, Grace PS, et al. IFN-Gamma-Independent Immune Markers of Mycobacterium Tuberculosis Exposure. Nat Med (2019) 25(6):977–87. doi: 10.1038/s41591-019-0441-3
42. Thulin NK, Brewer RC, Sherwood R, Bournazos S, Edwards KG, Ramadoss NS, et al. Maternal Anti-Dengue IgG Fucosylation Predicts Susceptibility to Dengue Disease in Infants. Cell Rep (2020) 31(6):107642. doi: 10.1016/j.celrep.2020.107642
43. Wang TT, Sewatanon J, Memoli MJ, Wrammert J, Bournazos S, Bhaumik SK, et al. IgG Antibodies to Dengue Enhanced for FcgammaRIIIA Binding Determine Disease Severity. Science (2017) 355(6323):395–8. doi: 10.1126/science.aai8128
44. McLean MR, Wragg KM, Lopez E, Kiazyk SA, Ball TB, Bueti J, et al. Serological and Cellular Inflammatory Signatures in End-Stage Kidney Disease and Latent Tuberculosis. Clin Transl Immunol (2021) 10(11):e1355. doi: 10.1002/cti2.1355
45. Olivares N, Marquina B, Mata-Espinoza D, Zatarain-Barron ZL, Pinzon CE, Estrada I, et al. The Protective Effect of Immunoglobulin in Murine Tuberculosis is Dependent on IgG Glycosylation. Pathog Dis (2013) 69(3):176–83. doi: 10.1111/2049-632X.12069
46. Kumagai T, Palacios A, Casadevall A, Garcia MJ, Toro C, Tiemeyer M, et al. Serum IgM Glycosylation Associated With Tuberculosis Infection in Mice. mSphere (2019) 4(2):e00684–18. doi: 10.1128/mSphere.00684-18
47. Chen T, Blanc C, Liu Y, Ishida E, Singer S, Xu J, et al. Capsular Glycan Recognition Provides Antibody-Mediated Immunity Against Tuberculosis. J Clin Invest (2020) 130(4):1808–22. doi: 10.1172/JCI128459
48. de Valliere S, Abate G, Blazevic A, Heuertz RM, Hoft DF. Enhancement of Innate and Cell-Mediated Immunity by Antimycobacterial Antibodies. Infect Immun (2005) 73(10):6711–20. doi: 10.1128/IAI.73.10.6711-6720.2005
49. Li H, Wang XX, Wang B, Fu L, Liu G, Lu Y, et al. Latently and Uninfected Healthcare Workers Exposed to TB Make Protective Antibodies Against Mycobacterium Tuberculosis. Proc Natl Acad Sci USA (2017) 114(19):5023–8. doi: 10.1073/pnas.1611776114
50. Warmerdam PA, van de Winkel JG, Gosselin EJ, Capel PJ. Molecular Basis for a Polymorphism of Human Fc Gamma Receptor II (Cd32). J Exp Med (1990) 172(1):19–25. doi: 10.1084/jem.172.1.19
51. Sanders LA, Feldman RG, Voorhorst-Ogink MM, de Haas M, Rijkers GT, Capel PJ, et al. Human Immunoglobulin G (IgG) Fc Receptor IIA (CD32) Polymorphism and IgG2-Mediated Bacterial Phagocytosis by Neutrophils. Infect Immun (1995) 63(1):73–81. doi: 10.1128/iai.63.1.73-81.1995
52. Platonov AE, Shipulin GA, Vershinina IV, Dankert J, van de Winkel JG, Kuijper EJ. Association of Human Fc Gamma RIIa (CD32) Polymorphism With Susceptibility to and Severity of Meningococcal Disease. Clin Infect Dis (1998) 27(4):746–50. doi: 10.1086/514935
53. Sanders LA, van de Winkel JG, Rijkers GT, Voorhorst-Ogink MM, de Haas M, Capel PJ, et al. Fc Gamma Receptor IIa (CD32) Heterogeneity in Patients With Recurrent Bacterial Respiratory Tract Infections. J Infect Dis (1994) 170(4):854–61. doi: 10.1093/infdis/170.4.854
54. Koene HR, Kleijer M, Algra J, Roos D, von dem Borne AE, de Haas M. Fc gammaRIIIa-158v/F Polymorphism Influences the Binding of IgG by Natural Killer Cell Fc gammaRIIIa, Independently of the Fc gammaRIIIa-48l/R/H Phenotype. Blood (1997) 90(3):1109–14. doi: 10.1182/blood.V90.3.1109
55. Wu J, Edberg JC, Redecha PB, Bansal V, Guyre PM, Coleman K, et al. A Novel Polymorphism of FcgammaRIIIa (CD16) Alters Receptor Function and Predisposes to Autoimmune Disease. J Clin Invest (1997) 100(5):1059–70. doi: 10.1172/JCI119616
56. Bournazos S, Woof JM, Hart SP, Dransfield I. Functional and Clinical Consequences of Fc Receptor Polymorphic and Copy Number Variants. Clin Exp Immunol (2009) 157(2):244–54. doi: 10.1111/j.1365-2249.2009.03980.x
57. Mellor JD, Brown MP, Irving HR, Zalcberg JR, Dobrovic A. A Critical Review of the Role of Fc Gamma Receptor Polymorphisms in the Response to Monoclonal Antibodies in Cancer. J Hematol Oncol (2013) 6:1. doi: 10.1186/1756-8722-6-1
58. Willcocks LC, Carr EJ, Niederer HA, Rayner TF, Williams TN, Yang W, et al. A Defunctioning Polymorphism in FCGR2B is Associated With Protection Against Malaria But Susceptibility to Systemic Lupus Erythematosus. Proc Natl Acad Sci USA (2010) 107(17):7881–5. doi: 10.1073/pnas.0915133107
59. Bournazos S, Klein F, Pietzsch J, Seaman MS, Nussenzweig MC, Ravetch JV. Broadly Neutralizing Anti-HIV-1 Antibodies Require Fc Effector Functions for In Vivo Activity. Cell (2014) 158(6):1243–53. doi: 10.1016/j.cell.2014.08.023
60. Halper-Stromberg A, Lu CL, Klein F, Horwitz JA, Bournazos S, Nogueira L, et al. Broadly Neutralizing Antibodies and Viral Inducers Decrease Rebound From HIV-1 Latent Reservoirs in Humanized Mice. Cell (2014) 158(5):989–99. doi: 10.1016/j.cell.2014.07.043
61. DiLillo DJ, Tan GS, Palese P, Ravetch JV. Broadly Neutralizing Hemagglutinin Stalk-Specific Antibodies Require FcgammaR Interactions for Protection Against Influenza Virus In Vivo. Nat Med (2014) 20(2):143–51. doi: 10.1038/nm.3443
62. He W, Chen CJ, Mullarkey CE, Hamilton JR, Wong CK, Leon PE, et al. Alveolar Macrophages are Critical for Broadly-Reactive Antibody-Mediated Protection Against Influenza A Virus in Mice. Nat Commun (2017) 8(1):846. doi: 10.1038/s41467-017-00928-3
63. Yamin R, Jones AT, Hoffmann HH, Schafer A, Kao KS, Francis RL, et al. Fc-Engineered Antibody Therapeutics With Improved Anti-SARS-CoV-2 Efficacy. Nature (2021) 599(7885):465–70. doi: 10.1038/s41586-021-04017-w
64. Ullah I, Prevost J, Ladinsky MS, Stone H, Lu M, Anand SP, et al. Live Imaging of SARS-CoV-2 Infection in Mice Reveals That Neutralizing Antibodies Require Fc Function for Optimal Efficacy. Immunity (2021) 54(9):2143–58.e15. doi: 10.1016/j.immuni.2021.08.015
65. Suryadevara N, Shrihari S, Gilchuk P, VanBlargan LA, Binshtein E, Zost SJ, et al. Neutralizing and Protective Human Monoclonal Antibodies Recognizing the N-Terminal Domain of the SARS-CoV-2 Spike Protein. Cell (2021) 184(9):2316–31.e15. doi: 10.1016/j.cell.2021.03.029
66. Winkler ES, Gilchuk P, Yu J, Bailey AL, Chen RE, Chong Z, et al. Human Neutralizing Antibodies Against SARS-CoV-2 Require Intact Fc Effector Functions for Optimal Therapeutic Protection. Cell (2021) 184(7):1804–20.e16. doi: 10.1016/j.cell.2021.02.026
67. Sutherland JS, Loxton AG, Haks MC, Kassa D, Ambrose L, Lee JS, et al. Differential Gene Expression of Activating Fcgamma Receptor Classifies Active Tuberculosis Regardless of Human Immunodeficiency Virus Status or Ethnicity. Clin Microbiol Infect (2014) 20(4):O230–8. doi: 10.1111/1469-0691.12383
68. Ahmed M, Thirunavukkarasu S, Rosa BA, Thomas KA, Das S, Rangel-Moreno J, et al. Immune Correlates of Tuberculosis Disease and Risk Translate Across Species. Sci Transl Med (2020) 12(528):eaay0233. doi: 10.1126/scitranslmed.aay0233
69. Machado LR, Bowdrey J, Ngaimisi E, Habtewold A, Minzi O, Makonnen E, et al. Copy Number Variation of Fc Gamma Receptor Genes in HIV-Infected and HIV-Tuberculosis Co-Infected Individuals in Sub-Saharan Africa. PloS One (2013) 8(11):e78165. doi: 10.1371/journal.pone.0078165
70. Boruchov AM, Heller G, Veri MC, Bonvini E, Ravetch JV, Young JW. Activating and Inhibitory IgG Fc Receptors on Human DCs Mediate Opposing Functions. J Clin Invest (2005) 115(10):2914–23. doi: 10.1172/JCI24772
71. Veri MC, Gorlatov S, Li H, Burke S, Johnson S, Stavenhagen J, et al. Monoclonal Antibodies Capable of Discriminating the Human Inhibitory Fcgamma-Receptor IIB (CD32B) From the Activating Fcgamma-Receptor IIA (CD32A): Biochemical, Biological and Functional Characterization. Immunology (2007) 121(3):392–404. doi: 10.1111/j.1365-2567.2007.02588.x
72. Roy Chowdhury R, Vallania F, Yang Q, Lopez Angel CJ, Darboe F, Penn-Nicholson A, et al. A Multi-Cohort Study of the Immune Factors Associated With M. Tuberculosis Infection Outcomes. Nature (2018) 560(7720):644–8. doi: 10.1038/s41586-018-0439-x
73. Lastrucci C, Benard A, Balboa L, Pingris K, Souriant S, Poincloux R, et al. Tuberculosis Is Associated With Expansion of a Motile, Permissive and Immunomodulatory CD16(+) Monocyte Population via the IL-10/STAT3 Axis. Cell Res (2015) 25(12):1333–51. doi: 10.1038/cr.2015.123
74. Nimmerjahn F, Ravetch JV. Divergent Immunoglobulin G Subclass Activity Through Selective Fc Receptor Binding. Science (2005) 310(5753):1510–2. doi: 10.1126/science.1118948
75. Clynes R, Maizes JS, Guinamard R, Ono M, Takai T, Ravetch JV. Modulation of Immune Complex-Induced Inflammation In Vivo by the Coordinate Expression of Activation and Inhibitory Fc Receptors. J Exp Med (1999) 189(1):179–85. doi: 10.1084/jem.189.1.179
76. Lu LL, Suscovich TJ, Fortune SM, Alter G. Beyond Binding: Antibody Effector Functions in Infectious Diseases. Nat Rev Immunol (2018) 18(1):46–61. doi: 10.1038/nri.2017.106
77. Cloutier N, Allaeys I, Marcoux G, Machlus KR, Mailhot B, Zufferey A, et al. Platelets Release Pathogenic Serotonin and Return to Circulation After Immune Complex-Mediated Sequestration. Proc Natl Acad Sci USA (2018) 115(7):E1550–9. doi: 10.1073/pnas.1720553115
78. Cui L, Lee YH, Thein TL, Fang J, Pang J, Ooi EE, et al. Serum Metabolomics Reveals Serotonin as a Predictor of Severe Dengue in the Early Phase of Dengue Fever. PloS Negl Trop Dis (2016) 10(4):e0004607. doi: 10.1371/journal.pntd.0004607
79. Sips M, Krykbaeva M, Diefenbach TJ, Ghebremichael M, Bowman BA, Dugast AS, et al. Fc Receptor-Mediated Phagocytosis in Tissues as a Potent Mechanism for Preventive and Therapeutic HIV Vaccine Strategies. Mucosal Immunol (2016) 9(6):1584–95. doi: 10.1038/mi.2016.12
80. Suh CI, Stull ND, Li XJ, Tian W, Price MO, Grinstein S, et al. The Phosphoinositide-Binding Protein P40phox Activates the NADPH Oxidase During FcgammaIIA Receptor-Induced Phagocytosis. J Exp Med (2006) 203(8):1915–25. doi: 10.1084/jem.20052085
81. Stacey HD, Golubeva D, Posca A, Ang JC, Novakowski KE, Zahoor MA, et al. IgA Potentiates NETosis in Response to Viral Infection. Proc Natl Acad Sci USA (2021) 118(27):e2101497118. doi: 10.1073/pnas.2101497118
82. Jonsson F, de Chaisemartin L, Granger V, Gouel-Cheron A, Gillis CM, Zhu Q, et al. An IgG-Induced Neutrophil Activation Pathway Contributes to Human Drug-Induced Anaphylaxis. Sci Transl Med (2019) 11(500):eaat1479. doi: 10.1126/scitranslmed.aat1479
83. Das M, Karnam A, Stephen-Victor E, Gilardin L, Bhatt B, Kumar Sharma V, et al. Intravenous Immunoglobulin Mediates Anti-Inflammatory Effects in Peripheral Blood Mononuclear Cells by Inducing Autophagy. Cell Death Dis (2020) 11(1):50. doi: 10.1038/s41419-020-2249-y
84. Bonnerot C, Briken V, Brachet V, Lankar D, Cassard S, Jabri B, et al. Syk Protein Tyrosine Kinase Regulates Fc Receptor Gamma-Chain-Mediated Transport to Lysosomes. EMBO J (1998) 17(16):4606–16. doi: 10.1093/emboj/17.16.4606
85. Hirako IC, Gallego-Marin C, Ataide MA, Andrade WA, Gravina H, Rocha BC, et al. DNA-Containing Immunocomplexes Promote Inflammasome Assembly and Release of Pyrogenic Cytokines by CD14+ CD16+ CD64high CD32low Inflammatory Monocytes From Malaria Patients. mBio (2015) 6(6):e01605–15. doi: 10.1128/mBio.01605-15
86. Labzin LI, Bottermann M, Rodriguez-Silvestre P, Foss S, Andersen JT, Vaysburd M, et al. Antibody and DNA Sensing Pathways Converge to Activate the Inflammasome During Primary Human Macrophage Infection. EMBO J (2019) 38(21):e101365. doi: 10.15252/embj.2018101365
87. Dhodapkar KM, Kaufman JL, Ehlers M, Banerjee DK, Bonvini E, Koenig S, et al. Selective Blockade of Inhibitory Fcgamma Receptor Enables Human Dendritic Cell Maturation With IL-12p70 Production and Immunity to Antibody-Coated Tumor Cells. Proc Natl Acad Sci USA (2005) 102(8):2910–5. doi: 10.1073/pnas.0500014102
88. Regnault A, Lankar D, Lacabanne V, Rodriguez A, Thery C, Rescigno M, et al. Fcgamma Receptor-Mediated Induction of Dendritic Cell Maturation and Major Histocompatibility Complex Class I-Restricted Antigen Presentation After Immune Complex Internalization. J Exp Med (1999) 189(2):371–80. doi: 10.1084/jem.189.2.371
89. Hoffmann E, Kotsias F, Visentin G, Bruhns P, Savina A, Amigorena S. Autonomous Phagosomal Degradation and Antigen Presentation in Dendritic Cells. Proc Natl Acad Sci USA (2012) 109(36):14556–61. doi: 10.1073/pnas.1203912109
90. Chen T, Blanc C, Eder AZ, Prados-Rosales R, Souza AC, Kim RS, et al. Association of Human Antibodies to Arabinomannan With Enhanced Mycobacterial Opsonophagocytosis and Intracellular Growth Reduction. J Infect Dis (2016) 214(2):300–10. doi: 10.1093/infdis/jiw141
91. McEwan WA, Tam JC, Watkinson RE, Bidgood SR, Mallery DL, James LC. Intracellular Antibody-Bound Pathogens Stimulate Immune Signaling via the Fc Receptor TRIM21. Nat Immunol (2013) 14(4):327–36. doi: 10.1038/ni.2548
92. MacLennan IC. Germinal Centers. Annu Rev Immunol (1994) 12:117–39. doi: 10.1146/annurev.iy.12.040194.001001
93. Slight SR, Rangel-Moreno J, Gopal R, Lin Y, Fallert Junecko BA, Mehra S, et al. CXCR5(+) T Helper Cells Mediate Protective Immunity Against Tuberculosis. J Clin Invest (2013) 123(2):712–26. doi: 10.1172/JCI65728
94. Walker LS, Gulbranson-Judge A, Flynn S, Brocker T, Raykundalia C, Goodall M, et al. Compromised OX40 Function in CD28-Deficient Mice Is Linked With Failure to Develop CXC Chemokine Receptor 5-Positive CD4 Cells and Germinal Centers. J Exp Med (1999) 190(8):1115–22. doi: 10.1084/jem.190.8.1115
95. Mesin L, Ersching J, Victora GD. Germinal Center B Cell Dynamics. Immunity (2016) 45(3):471–82. doi: 10.1016/j.immuni.2016.09.001
96. Olmos S, Stukes S, Ernst JD. Ectopic Activation of Mycobacterium Tuberculosis-Specific CD4+ T Cells in Lungs of CCR7-/- Mice. J Immunol (2010) 184(2):895–901. doi: 10.4049/jimmunol.0901230
97. Phuah JY, Mattila JT, Lin PL, Flynn JL. Activated B Cells in the Granulomas of Nonhuman Primates Infected With Mycobacterium Tuberculosis. Am J Pathol (2012) 181(2):508–14. doi: 10.1016/j.ajpath.2012.05.009
98. Dunlap MD, Prince OA, Rangel-Moreno J, Thomas KA, Scordo JM, Torrelles JB, et al. Formation of Lung Inducible Bronchus Associated Lymphoid Tissue Is Regulated by Mycobacterium Tuberculosis Expressed Determinants. Front Immunol (2020) 11:1325. doi: 10.3389/fimmu.2020.01325
99. Ulrichs T, Kosmiadi GA, Trusov V, Jorg S, Pradl L, Titukhina M, et al. Human Tuberculous Granulomas Induce Peripheral Lymphoid Follicle-Like Structures to Orchestrate Local Host Defence in the Lung. J Pathol (2004) 204(2):217–28. doi: 10.1002/path.1628
100. Ron Y, Sprent J. T Cell Priming In Vivo: A Major Role for B Cells in Presenting Antigen to T Cells in Lymph Nodes. J Immunol (1987) 138(9):2848–56.
101. Cyster JG, Allen CDC. B Cell Responses: Cell Interaction Dynamics and Decisions. Cell (2019) 177(3):524–40. doi: 10.1016/j.cell.2019.03.016
102. Joosten SA, van Meijgaarden KE, Del Nonno F, Baiocchini A, Petrone L, Vanini V, et al. Patients With Tuberculosis Have a Dysfunctional Circulating B-Cell Compartment, Which Normalizes Following Successful Treatment. PloS Pathog (2016) 12(6):e1005687. doi: 10.1371/journal.ppat.1005687
103. du Plessis WJ, Keyser A, Walzl G, Loxton AG. Phenotypic Analysis of Peripheral B Cell Populations During Mycobacterium Tuberculosis Infection and Disease. J Inflammation (Lond) (2016) 13:23. doi: 10.1186/s12950-016-0133-4
104. Abreu MT, Carvalheiro H, Rodrigues-Sousa T, Domingos A, Segorbe-Luis A, Rodrigues-Santos P, et al. Alterations in the Peripheral Blood B Cell Subpopulations of Multidrug-Resistant Tuberculosis Patients. Clin Exp Med (2014) 14(4):423–9. doi: 10.1007/s10238-013-0258-1
105. Possamai D, Page G, Panes R, Gagnon E, Lapointe R. CD40L-Stimulated B Lymphocytes Are Polarized Toward APC Functions After Exposure to IL-4 and IL-21. J Immunol (2021) 207(1):77–89. doi: 10.4049/jimmunol.2001173
106. Kleindienst P, Brocker T. Concerted Antigen Presentation by Dendritic Cells and B Cells is Necessary for Optimal CD4 T-Cell Immunity In Vivo. Immunology (2005) 115(4):556–64. doi: 10.1111/j.1365-2567.2005.02196.x
107. Marin ND, Dunlap MD, Kaushal D, Khader SA. Friend or Foe: The Protective and Pathological Roles of Inducible Bronchus-Associated Lymphoid Tissue in Pulmonary Diseases. J Immunol (2019) 202(9):2519–26. doi: 10.4049/jimmunol.1801135
108. Mathieu M, Cotta-Grand N, Daudelin JF, Boulet S, Lapointe R, Labrecque N. CD40-Activated B Cells can Efficiently Prime Antigen-Specific Naive CD8+ T Cells to Generate Effector But Not Memory T Cells. PloS One (2012) 7(1):e30139. doi: 10.1371/journal.pone.0030139
109. Shen P, Fillatreau S. Antibody-Independent Functions of B Cells: A Focus on Cytokines. Nat Rev Immunol (2015) 15(7):441–51. doi: 10.1038/nri3857
110. Neves P, Lampropoulou V, Calderon-Gomez E, Roch T, Stervbo U, Shen P, et al. Signaling via the MyD88 Adaptor Protein in B Cells Suppresses Protective Immunity During Salmonella Typhimurium Infection. Immunity (2010) 33(5):777–90. doi: 10.1016/j.immuni.2010.10.016
111. Lee CC, Kung JT. Marginal Zone B Cell Is a Major Source of Il-10 in Listeria Monocytogenes Susceptibility. J Immunol (2012) 189(7):3319–27. doi: 10.4049/jimmunol.1201247
112. Wong EA, Evans S, Kraus CR, Engelman KD, Maiello P, Flores WJ, et al. IL-10 Impairs Local Immune Response in Lung Granulomas and Lymph Nodes During Early Mycobacterium Tuberculosis Infection. J Immunol (2020) 204(3):644–59. doi: 10.4049/jimmunol.1901211
113. Richardson ET, Shukla S, Sweet DR, Wearsch PA, Tsichlis PN, Boom WH, et al. Toll-Like Receptor 2-Dependent Extracellular Signal-Regulated Kinase Signaling in Mycobacterium Tuberculosis-Infected Macrophages Drives Anti-Inflammatory Responses and Inhibits Th1 Polarization of Responding T Cells. Infect Immun (2015) 83(6):2242–54. doi: 10.1128/IAI.00135-15
114. Gideon HP, Phuah J, Myers AJ, Bryson BD, Rodgers MA, Coleman MT, et al. Variability in Tuberculosis Granuloma T Cell Responses Exists, But a Balance of Pro- and Anti-Inflammatory Cytokines Is Associated With Sterilization. PloS Pathog (2015) 11(1):e1004603. doi: 10.1371/journal.ppat.1004603
115. Carter NA, Vasconcellos R, Rosser EC, Tulone C, Munoz-Suano A, Kamanaka M, et al. Mice Lacking Endogenous IL-10-Producing Regulatory B Cells Develop Exacerbated Disease and Present With an Increased Frequency of Th1/Th17 But a Decrease in Regulatory T Cells. J Immunol (2011) 186(10):5569–79. doi: 10.4049/jimmunol.1100284
116. Flores-Borja F, Bosma A, Ng D, Reddy V, Ehrenstein MR, Isenberg DA, et al. CD19+CD24hiCD38hi B Cells Maintain Regulatory T Cells While Limiting TH1 and TH17 Differentiation. Sci Transl Med (2013) 5(173):173ra23. doi: 10.1126/scitranslmed.3005407
117. Zhang M, Zheng X, Zhang J, Zhu Y, Zhu X, Liu H, et al. CD19(+)CD1d(+)CD5(+) B Cell Frequencies are Increased in Patients With Tuberculosis and Suppress Th17 Responses. Cell Immunol (2012) 274(1-2):89–97. doi: 10.1016/j.cellimm.2012.01.007
118. Zhang M, Zeng G, Yang Q, Zhang J, Zhu X, Chen Q, et al. Anti-Tuberculosis Treatment Enhances the Production of IL-22 Through Reducing the Frequencies of Regulatory B Cell. Tuberculosis (Edinb) (2014) 94(3):238–44. doi: 10.1016/j.tube.2013.12.003
119. Reynolds HY. Immunoglobulin G and Its Function in the Human Respiratory Tract. Mayo Clin Proc (1988) 63(2):161–74. doi: 10.1016/S0025-6196(12)64949-0
120. Sterlin D, Mathian A, Miyara M, Mohr A, Anna F, Claer L, et al. IgA Dominates the Early Neutralizing Antibody Response to SARS-CoV-2. Sci Transl Med (2021) 13(577):eabd2223. doi: 10.1126/scitranslmed.abd2223
121. Vogelzang A, Lozza L, Reece ST, Perdomo C, Zedler U, Hahnke K, et al. Neonatal Fc Receptor Regulation of Lung Immunoglobulin and CD103+ Dendritic Cells Confers Transient Susceptibility to Tuberculosis. Infect Immun (2016) 84(10):2914–21. doi: 10.1128/IAI.00533-16
122. Reyes F, Tirado Y, Puig A, Borrero R, Reyes G, Fernandez S, et al. Immunogenicity and Cross-Reactivity Against Mycobacterium Tuberculosis of Proteoliposomes Derived From Mycobacterium Bovis BCG. BMC Immunol (2013) 14 Suppl 1:S7. doi: 10.1186/1471-2172-14-S1-S7
123. Shah JA, Lindestam Arlehamn CS, Horne DJ, Sette A, Hawn TR. Nontuberculous Mycobacteria and Heterologous Immunity to Tuberculosis. J Infect Dis (2019) 220(7):1091–8. doi: 10.1093/infdis/jiz285
124. Perley CC, Frahm M, Click EM, Dobos KM, Ferrari G, Stout JE, et al. The Human Antibody Response to the Surface of Mycobacterium Tuberculosis. PloS One (2014) 9(2):e98938. doi: 10.1371/journal.pone.0098938
125. Zeng MY, Cisalpino D, Varadarajan S, Hellman J, Warren HS, Cascalho M, et al. Gut Microbiota-Induced Immunoglobulin G Controls Systemic Infection by Symbiotic Bacteria and Pathogens. Immunity (2016) 44(3):647–58. doi: 10.1016/j.immuni.2016.02.006
126. Ochsenbein AF, Fehr T, Lutz C, Suter M, Brombacher F, Hengartner H, et al. Control of Early Viral and Bacterial Distribution and Disease by Natural Antibodies. Science (1999) 286(5447):2156–9. doi: 10.1126/science.286.5447.2156
127. Griffin DO, Holodick NE, Rothstein TL. Human B1 Cells in Umbilical Cord and Adult Peripheral Blood Express the Novel Phenotype CD20+ CD27+ CD43+ Cd70. J Exp Med (2011) 208(1):67–80. doi: 10.1084/jem.20101499
128. Jayasekera JP, Moseman EA, Carroll MC. Natural Antibody and Complement Mediate Neutralization of Influenza Virus in the Absence of Prior Immunity. J Virol (2007) 81(7):3487–94. doi: 10.1128/JVI.02128-06
129. Vas J, Gronwall C, Silverman GJ. Fundamental Roles of the Innate-Like Repertoire of Natural Antibodies in Immune Homeostasis. Front Immunol (2013) 4:4. doi: 10.3389/fimmu.2013.00004
130. Martin CJ, Booty MG, Rosebrock TR, Nunes-Alves C, Desjardins DM, Keren I, et al. Efferocytosis is an Innate Antibacterial Mechanism. Cell Host Microbe (2012) 12(3):289–300. doi: 10.1016/j.chom.2012.06.010
131. Baumgarth N, Herman OC, Jager GC, Brown LE, Herzenberg LA, Chen J. B-1 and B-2 Cell-Derived Immunoglobulin M Antibodies are Nonredundant Components of the Protective Response to Influenza Virus Infection. J Exp Med (2000) 192(2):271–80. doi: 10.1084/jem.192.2.271
132. Boes M, Prodeus AP, Schmidt T, Carroll MC, Chen J. A Critical Role of Natural Immunoglobulin M in Immediate Defense Against Systemic Bacterial Infection. J Exp Med (1998) 188(12):2381–6. doi: 10.1084/jem.188.12.2381
133. Panda S, Ding JL. Natural Antibodies Bridge Innate and Adaptive Immunity. J Immunol (2015) 194(1):13–20. doi: 10.4049/jimmunol.1400844
134. Panda S, Zhang J, Tan NS, Ho B, Ding JL. Natural IgG Antibodies Provide Innate Protection Against Ficolin-Opsonized Bacteria. EMBO J (2013) 32(22):2905–19. doi: 10.1038/emboj.2013.199
135. Schubert OT, Ludwig C, Kogadeeva M, Zimmermann M, Rosenberger G, Gengenbacher M, et al. Absolute Proteome Composition and Dynamics During Dormancy and Resuscitation of Mycobacterium Tuberculosis. Cell Host Microbe (2015) 18(1):96–108. doi: 10.1016/j.chom.2015.06.001
136. Gopinath V, Raghunandanan S, Gomez RL, Jose L, Surendran A, Ramachandran R, et al. Profiling the Proteome of Mycobacterium Tuberculosis During Dormancy and Reactivation. Mol Cell Proteomics (2015) 14(8):2160–76. doi: 10.1074/mcp.M115.051151
137. Leyten EM, Lin MY, Franken KL, Friggen AH, Prins C, van Meijgaarden KE, et al. Human T-Cell Responses to 25 Novel Antigens Encoded by Genes of the Dormancy Regulon of Mycobacterium Tuberculosis. Microbes Infect (2006) 8(8):2052–60. doi: 10.1016/j.micinf.2006.03.018
138. Coscolla M, Gagneux S. Consequences of Genomic Diversity in Mycobacterium Tuberculosis. Semin Immunol (2014) 26(6):431–44. doi: 10.1016/j.smim.2014.09.012
139. Cambier CJ, Banik SM, Buonomo JA, Bertozzi CR. Spreading of a Mycobacterial Cell-Surface Lipid Into Host Epithelial Membranes Promotes Infectivity. Elife (2020) 9:e60648. doi: 10.7554/eLife.60648
140. Beatty WL, Rhoades ER, Ullrich HJ, Chatterjee D, Heuser JE, Russell DG. Trafficking and Release of Mycobacterial Lipids From Infected Macrophages. Traffic (2000) 1(3):235–47. doi: 10.1034/j.1600-0854.2000.010306.x
141. Kruh-Garcia NA, Wolfe LM, Chaisson LH, Worodria WO, Nahid P, Schorey JS, et al. Detection of Mycobacterium Tuberculosis Peptides in the Exosomes of Patients With Active and Latent M. Tuberculosis Infection Using MRM-MS. PloS One (2014) 9(7):e103811. doi: 10.1371/journal.pone.0103811
142. Singh PP, Smith VL, Karakousis PC, Schorey JS. Exosomes Isolated From Mycobacteria-Infected Mice or Cultured Macrophages can Recruit and Activate Immune Cells In Vitro and In Vivo. J Immunol (2012) 189(2):777–85. doi: 10.4049/jimmunol.1103638
143. Spiekermann GM, Finn PW, Ward ES, Dumont J, Dickinson BL, Blumberg RS, et al. Receptor-Mediated Immunoglobulin G Transport Across Mucosal Barriers in Adult Life: Functional Expression of FcRn in the Mammalian Lung. J Exp Med (2002) 196(3):303–10. doi: 10.1084/jem.20020400
144. Tjarnlund A, Rodriguez A, Cardona PJ, Guirado E, Ivanyi J, Singh M, et al. Polymeric IgR Knockout Mice Are More Susceptible to Mycobacterial Infections in the Respiratory Tract Than Wild-Type Mice. Int Immunol (2006) 18(5):807–16. doi: 10.1093/intimm/dxl017
145. Shibuya A, Sakamoto N, Shimizu Y, Shibuya K, Osawa M, Hiroyama T, et al. Fc Alpha/Mu Receptor Mediates Endocytosis of IgM-Coated Microbes. Nat Immunol (2000) 1(5):441–6. doi: 10.1038/80886
146. Stockert RJ, Kressner MS, Collins JC, Sternlieb I, Morell AG. IgA Interaction With the Asialoglycoprotein Receptor. Proc Natl Acad Sci USA (1982) 79(20):6229–31. doi: 10.1073/pnas.79.20.6229
147. Moura IC, Centelles MN, Arcos-Fajardo M, Malheiros DM, Collawn JF, Cooper MD, et al. Identification of the Transferrin Receptor as a Novel Immunoglobulin (Ig)A1 Receptor and its Enhanced Expression on Mesangial Cells in IgA Nephropathy. J Exp Med (2001) 194(4):417–25. doi: 10.1084/jem.194.4.417
148. Mantis NJ, Cheung MC, Chintalacharuvu KR, Rey J, Corthesy B, Neutra MR. Selective Adherence of IgA to Murine Peyer's Patch M Cells: Evidence for a Novel IgA Receptor. J Immunol (2002) 169(4):1844–51. doi: 10.4049/jimmunol.169.4.1844
149. Golebski K, Hoepel W, van Egmond D, de Groot EJ, Amatngalim GD, Beekman JM, et al. FcgammaRIII Stimulation Breaks the Tolerance of Human Nasal Epithelial Cells to Bacteria Through Cross-Talk With TLR4. Mucosal Immunol (2019) 12(2):425–33. doi: 10.1038/s41385-018-0129-x
150. Xia YC, Schuliga M, Shepherd M, Powell M, Harris T, Langenbach SY, et al. Functional Expression of IgG-Fc Receptors in Human Airway Smooth Muscle Cells. Am J Respir Cell Mol Biol (2011) 44(5):665–72. doi: 10.1165/rcmb.2009-0371OC
151. Cohen SB, Gern BH, Delahaye JL, Adams KN, Plumlee CR, Winkler JK, et al. Alveolar Macrophages Provide an Early Mycobacterium Tuberculosis Niche and Initiate Dissemination. Cell Host Microbe (2018) 24(3):439–46.e4. doi: 10.1016/j.chom.2018.08.001
152. Papp AC, Azad AK, Pietrzak M, Williams A, Handelman SK, Igo RP Jr, et al. AmpliSeq Transcriptome Analysis of Human Alveolar and Monocyte-Derived Macrophages Over Time in Response to Mycobacterium Tuberculosis Infection. PloS One (2018) 13(5):e0198221. doi: 10.1371/journal.pone.0198221
153. Verreck FA, de Boer T, Langenberg DM, Hoeve MA, Kramer M, Vaisberg E, et al. Human IL-23-Producing Type 1 Macrophages Promote But IL-10-Producing Type 2 Macrophages Subvert Immunity to (Myco)Bacteria. Proc Natl Acad Sci USA (2004) 101(13):4560–5. doi: 10.1073/pnas.0400983101
154. Pisu D, Huang L, Narang V, Theriault M, Le-Bury G, Lee B, et al. Single Cell Analysis of M. Tuberculosis Phenotype and Macrophage Lineages in the Infected Lung. J Exp Med (2021) 218(9):e20210615. doi: 10.1084/jem.20210615
155. Bryson BD, Rosebrock TR, Tafesse FG, Itoh CY, Nibasumba A, Babunovic GH, et al. Heterogeneous GM-CSF Signaling in Macrophages is Associated With Control of Mycobacterium Tuberculosis. Nat Commun (2019) 10(1):2329. doi: 10.1038/s41467-019-10065-8
156. Pincetic A, Bournazos S, DiLillo DJ, Maamary J, Wang TT, Dahan R, et al. Type I and Type II Fc Receptors Regulate Innate and Adaptive Immunity. Nat Immunol (2014) 15(8):707–16. doi: 10.1038/ni.2939
157. Bruggeman CW, Houtzager J, Dierdorp B, Kers J, Pals ST, Lutter R, et al. Tissue-Specific Expression of IgG Receptors by Human Macrophages Ex Vivo. PloS One (2019) 14(10):e0223264. doi: 10.1371/journal.pone.0223264
158. Li Y, Lee PY, Sobel ES, Narain S, Satoh M, Segal MS, et al. Increased Expression of FcgammaRI/CD64 on Circulating Monocytes Parallels Ongoing Inflammation and Nephritis in Lupus. Arthritis Res Ther (2009) 11(1):R6. doi: 10.1186/ar2591
159. Armstrong JA, Hart PD. Phagosome-Lysosome Interactions in Cultured Macrophages Infected With Virulent Tubercle Bacilli. Reversal of the Usual Nonfusion Pattern and Observations on Bacterial Survival. J Exp Med (1975) 142(1):1–16. doi: 10.1084/jem.142.1.1
160. Joller N, Weber SS, Muller AJ, Sporri R, Selchow P, Sander P, et al. Antibodies Protect Against Intracellular Bacteria by Fc Receptor-Mediated Lysosomal Targeting. Proc Natl Acad Sci USA (2010) 107(47):20441–6. doi: 10.1073/pnas.1013827107
161. Faridgohar M, Nikoueinejad H. New Findings of Toll-Like Receptors Involved in Mycobacterium Tuberculosis Infection. Pathog Glob Health (2017) 111(5):256–64. doi: 10.1080/20477724.2017.1351080
162. Vogelpoel LT, Hansen IS, Rispens T, Muller FJ, van Capel TM, Turina MC, et al. Fc Gamma Receptor-TLR Cross-Talk Elicits Pro-Inflammatory Cytokine Production by Human M2 Macrophages. Nat Commun (2014) 5:5444. doi: 10.1038/ncomms6444
163. Moreira-Teixeira L, Stimpson PJ, Stavropoulos E, Hadebe S, Chakravarty P, Ioannou M, et al. Type I IFN Exacerbates Disease in Tuberculosis-Susceptible Mice by Inducing Neutrophil-Mediated Lung Inflammation and NETosis. Nat Commun (2020) 11(1):5566. doi: 10.1038/s41467-020-19412-6
164. Schiff DE, Rae J, Martin TR, Davis BH, Curnutte JT. Increased Phagocyte Fc gammaRI Expression and Improved Fc Gamma-Receptor-Mediated Phagocytosis After In Vivo Recombinant Human Interferon-Gamma Treatment of Normal Human Subjects. Blood (1997) 90(8):3187–94. doi: 10.1182/blood.V90.8.3187
165. Zak DE, Penn-Nicholson A, Scriba TJ, Thompson E, Suliman S, Amon LM, et al. A Blood RNA Signature for Tuberculosis Disease Risk: A Prospective Cohort Study. Lancet (2016) 387(10035):2312–22. doi: 10.1016/S0140-6736(15)01316-1
166. Cid J, Aguinaco R, Sanchez R, Garcia-Pardo G, Llorente A. Neutrophil CD64 Expression as Marker of Bacterial Infection: A Systematic Review and Meta-Analysis. J Infect (2010) 60(5):313–9. doi: 10.1016/j.jinf.2010.02.013
167. Treffers LW, van Houdt M, Bruggeman CW, Heineke MH, Zhao XW, van der Heijden J, et al. FcgammaRIIIb Restricts Antibody-Dependent Destruction of Cancer Cells by Human Neutrophils. Front Immunol (2018) 9:3124. doi: 10.3389/fimmu.2018.03124
168. Balu S, Reljic R, Lewis MJ, Pleass RJ, McIntosh R, van Kooten C, et al. A Novel Human IgA Monoclonal Antibody Protects Against Tuberculosis. J Immunol (2011) 186(5):3113–9. doi: 10.4049/jimmunol.1003189
169. Tran AC, Diogo GR, Paul MJ, Copland A, Hart P, Mehta N, et al. Mucosal Therapy of Multi-Drug Resistant Tuberculosis With IgA and Interferon-Gamma. Front Immunol (2020) 11:582833. doi: 10.3389/fimmu.2020.582833
170. Otten MA, Rudolph E, Dechant M, Tuk CW, Reijmers RM, Beelen RH, et al. Immature Neutrophils Mediate Tumor Cell Killing via IgA But Not IgG Fc Receptors. J Immunol (2005) 174(9):5472–80. doi: 10.4049/jimmunol.174.9.5472
171. Matlung HL, Babes L, Zhao XW, van Houdt M, Treffers LW, van Rees DJ, et al. Neutrophils Kill Antibody-Opsonized Cancer Cells by Trogoptosis. Cell Rep (2018) 23(13):3946–59.e6. doi: 10.1016/j.celrep.2018.05.082
172. Richardson SI, Crowther C, Mkhize NN, Morris L. Measuring the Ability of HIV-Specific Antibodies to Mediate Trogocytosis. J Immunol Methods (2018) 463:71–83. doi: 10.1016/j.jim.2018.09.009
173. Steele S, Radlinski L, Taft-Benz S, Brunton J, Kawula TH. Trogocytosis-Associated Cell to Cell Spread of Intracellular Bacterial Pathogens. Elife (2016) 5:e10625. doi: 10.7554/eLife.10625
174. Chen K, Nishi H, Travers R, Tsuboi N, Martinod K, Wagner DD, et al. Endocytosis of Soluble Immune Complexes Leads to Their Clearance by FcgammaRIIIB But Induces Neutrophil Extracellular Traps via FcgammaRIIA In Vivo. Blood (2012) 120(22):4421–31. doi: 10.1182/blood-2011-12-401133
175. Aleman OR, Mora N, Cortes-Vieyra R, Uribe-Querol E, Rosales C. Differential Use of Human Neutrophil Fcgamma Receptors for Inducing Neutrophil Extracellular Trap Formation. J Immunol Res (2016) 2016:2908034. doi: 10.1155/2016/2908034
176. Griffiths KL, Ahmed M, Das S, Gopal R, Horne W, Connell TD, et al. Targeting Dendritic Cells to Accelerate T-Cell Activation Overcomes a Bottleneck in Tuberculosis Vaccine Efficacy. Nat Commun (2016) 7:13894. doi: 10.1038/ncomms13894
177. Guilliams M, Bruhns P, Saeys Y, Hammad H, Lambrecht BN. The Function of Fcgamma Receptors in Dendritic Cells and Macrophages. Nat Rev Immunol (2014) 14(2):94–108. doi: 10.1038/nri3582
178. Wolf AJ, Desvignes L, Linas B, Banaiee N, Tamura T, Takatsu K, et al. Initiation of the Adaptive Immune Response to Mycobacterium Tuberculosis Depends on Antigen Production in the Local Lymph Node, Not the Lungs. J Exp Med (2008) 205(1):105–15. doi: 10.1084/jem.20071367
179. Liu X, Lu L, Yang Z, Palaniyandi S, Zeng R, Gao LY, et al. The Neonatal FcR-Mediated Presentation of Immune-Complexed Antigen is Associated With Endosomal and Phagosomal pH and Antigen Stability in Macrophages and Dendritic Cells. J Immunol (2011) 186(8):4674–86. doi: 10.4049/jimmunol.1003584
180. DiLillo DJ, Ravetch JV. Differential Fc-Receptor Engagement Drives an Anti-Tumor Vaccinal Effect. Cell (2015) 161(5):1035–45. doi: 10.1016/j.cell.2015.04.016
181. Bournazos S, Ravetch JV, Corti D, Virgin HW. Fc-Optimized Antibodies Elicit CD8 Immunity to Viral Respiratory Infection. Nature (2020) 588(7838):485–90. doi: 10.1038/s41586-020-2838-z
182. Brooks DG, Qiu WQ, Luster AD, Ravetch JV. Structure and Expression of Human IgG FcRII(CD32). Functional Heterogeneity is Encoded by the Alternatively Spliced Products of Multiple Genes. J Exp Med (1989) 170(4):1369–85. doi: 10.1084/jem.170.4.1369
183. El Shikh ME, El Sayed RM, Sukumar S, Szakal AK, Tew JG. Activation of B Cells by Antigens on Follicular Dendritic Cells. Trends Immunol (2010) 31(6):205–11. doi: 10.1016/j.it.2010.03.002
184. Muta T, Kurosaki T, Misulovin Z, Sanchez M, Nussenzweig MC, Ravetch JV. A 13-Amino-Acid Motif in the Cytoplasmic Domain of Fc Gamma RIIB Modulates B-Cell Receptor Signalling. Nature (1994) 368(6466):70–3. doi: 10.1038/368070a0
185. Farley CR, Morris AB, Tariq M, Bennion KB, Potdar S, Kudchadkar R, et al. FcgammaRIIB is a T Cell Checkpoint in Antitumor Immunity. JCI Insight (2021) 6(4):e135623. doi: 10.1172/jci.insight.135623
186. Holgado MP, Sananez I, Raiden S, Geffner JR, Arruvito L. CD32 Ligation Promotes the Activation of CD4(+) T Cells. Front Immunol (2018) 9:2814. doi: 10.3389/fimmu.2018.02814
187. Abdel-Mohsen M, Kuri-Cervantes L, Grau-Exposito J, Spivak AM, Nell RA, Tomescu C, et al. CD32 is Expressed on Cells With Transcriptionally Active HIV But Does Not Enrich for HIV DNA in Resting T Cells. Sci Transl Med (2018) 10(437):eaar6759. doi: 10.1126/scitranslmed.aar6759
188. Engelhardt W, Matzke J, Schmidt RE. Activation-Dependent Expression of Low Affinity IgG Receptors Fc Gamma RII(CD32) and Fc Gamma RIII(CD16) in Subpopulations of Human T Lymphocytes. Immunobiology (1995) 192(5):297–320. doi: 10.1016/S0171-2985(11)80172-5
189. Virdi AK, Wallace J, Barbian H, Richards MH, Ritz EM, Sha B, et al. CD32 Is Enriched on CD4dimCD8bright T Cells. PloS One (2020) 15(9):e0239157. doi: 10.1371/journal.pone.0239157
190. Meryk A, Pangrazzi L, Hagen M, Hatzmann F, Jenewein B, Jakic B, et al. Fcmu Receptor as a Costimulatory Molecule for T Cells. Cell Rep (2019) 26(10):2681–91.e5. doi: 10.1016/j.celrep.2019.02.024
191. Henrickson SE, von Andrian UH. Single-Cell Dynamics of T-Cell Priming. Curr Opin Immunol (2007) 19(3):249–58. doi: 10.1016/j.coi.2007.04.013
192. Carpenter SM, Yang JD, Lee J, Barreira-Silva P, Behar SM. Vaccine-Elicited Memory CD4+ T Cell Expansion is Impaired in the Lungs During Tuberculosis. PloS Pathog (2017) 13(11):e1006704. doi: 10.1371/journal.ppat.1006704
193. Samstein M, Schreiber HA, Leiner IM, Susac B, Glickman MS, Pamer EG. Essential Yet Limited Role for CCR2(+) Inflammatory Monocytes During Mycobacterium Tuberculosis-Specific T Cell Priming. Elife (2013) 2:e01086. doi: 10.7554/eLife.01086.013
194. Lykken JM, DiLillo DJ, Weimer ET, Roser-Page S, Heise MT, Grayson JM, et al. Acute and Chronic B Cell Depletion Disrupts CD4+ and CD8+ T Cell Homeostasis and Expansion During Acute Viral Infection in Mice. J Immunol (2014) 193(2):746–56. doi: 10.4049/jimmunol.1302848
195. Fiocca Vernengo F, Beccaria CG, Araujo Furlan CL, Tosello Boari J, Almada L, Gorosito Serran M, et al. CD8(+) T Cell Immunity Is Compromised by Anti-CD20 Treatment and Rescued by Interleukin-17a. mBio (2020) 11(3):e00447–20. doi: 10.1128/mBio.00447-20
196. Mollo SB, Zajac AJ, Harrington LE. Temporal Requirements for B Cells in the Establishment of CD4 T Cell Memory. J Immunol (2013) 191(12):6052–9. doi: 10.4049/jimmunol.1302033
197. Bouaziz JD, Yanaba K, Venturi GM, Wang Y, Tisch RM, Poe JC, et al. Therapeutic B Cell Depletion Impairs Adaptive and Autoreactive CD4+ T Cell Activation in Mice. Proc Natl Acad Sci USA (2007) 104(52):20878–83. doi: 10.1073/pnas.0709205105
198. Ugrinovic S, Menager N, Goh N, Mastroeni P. Characterization and Development of T-Cell Immune Responses in B-Cell-Deficient (Igh-6(-/-)) Mice With Salmonella Enterica Serovar Typhimurium Infection. Infect Immun (2003) 71(12):6808–19. doi: 10.1128/IAI.71.12.6808-6819.2003
199. Kroeger DR, Rudulier CD, Bretscher PA. Antigen Presenting B Cells Facilitate CD4 T Cell Cooperation Resulting in Enhanced Generation of Effector and Memory CD4 T Cells. PloS One (2013) 8(10):e77346. doi: 10.1371/journal.pone.0077346
200. Raposo G, Nijman HW, Stoorvogel W, Liejendekker R, Harding CV, Melief CJ, et al. B Lymphocytes Secrete Antigen-Presenting Vesicles. J Exp Med (1996) 183(3):1161–72. doi: 10.1084/jem.183.3.1161
201. Muntasell A, Berger AC, Roche PA. T Cell-Induced Secretion of MHC Class II-Peptide Complexes on B Cell Exosomes. EMBO J (2007) 26(19):4263–72. doi: 10.1038/sj.emboj.7601842
202. Saunderson SC, McLellan AD. Role of Lymphocyte Subsets in the Immune Response to Primary B Cell-Derived Exosomes. J Immunol (2017) 199(7):2225–35. doi: 10.4049/jimmunol.1601537
203. Hwang I, Shen X, Sprent J. Direct Stimulation of Naive T Cells by Membrane Vesicles From Antigen-Presenting Cells: Distinct Roles for CD54 and B7 Molecules. Proc Natl Acad Sci U.S.A. (2003) 100(11):6670–5. doi: 10.1073/pnas.1131852100
204. Giri PK, Schorey JS. Exosomes Derived From M. Bovis BCG Infected Macrophages Activate Antigen-Specific CD4+ and CD8+ T Cells In Vitro and In Vivo. PloS One (2008) 3(6):e2461. doi: 10.1371/journal.pone.0002461
205. Ramachandra L, Qu Y, Wang Y, Lewis CJ, Cobb BA, Takatsu K, et al. Mycobacterium Tuberculosis Synergizes With ATP to Induce Release of Microvesicles and Exosomes Containing Major Histocompatibility Complex Class II Molecules Capable of Antigen Presentation. Infect Immun (2010) 78(12):5116–25. doi: 10.1128/IAI.01089-09
206. Bhatnagar S, Shinagawa K, Castellino FJ, Schorey JS. Exosomes Released From Macrophages Infected With Intracellular Pathogens Stimulate a Proinflammatory Response In Vitro and In Vivo. Blood (2007) 110(9):3234–44. doi: 10.1182/blood-2007-03-079152
207. Cheng Y, Schorey JS. Exosomes Carrying Mycobacterial Antigens can Protect Mice Against Mycobacterium Tuberculosis Infection. Eur J Immunol (2013) 43(12):3279–90. doi: 10.1002/eji.201343727
208. Smith VL, Cheng Y, Bryant BR, Schorey JS. Exosomes Function in Antigen Presentation During an In Vivo Mycobacterium Tuberculosis Infection. Sci Rep (2017) 7:43578. doi: 10.1038/srep43578
209. Yang JD, Mott D, Sutiwisesak R, Lu YJ, Raso F, Stowell B, et al. Mycobacterium Tuberculosis-Specific CD4+ and CD8+ T Cells Differ in Their Capacity to Recognize Infected Macrophages. PloS Pathog (2018) 14(5):e1007060. doi: 10.1371/journal.ppat.1007060
210. Srivastava S, Grace PS, Ernst JD. Antigen Export Reduces Antigen Presentation and Limits T Cell Control of M. Tuberculosis. Cell Host Microbe (2016) 19(1):44–54. doi: 10.1016/j.chom.2015.12.003
211. Patankar YR, Sutiwisesak R, Boyce S, Lai R, Lindestam Arlehamn CS, Sette A, et al. Limited Recognition of Mycobacterium Tuberculosis-Infected Macrophages by Polyclonal CD4 and CD8 T Cells From the Lungs of Infected Mice. Mucosal Immunol (2020) 13(1):140–8. doi: 10.1038/s41385-019-0217-6
212. Wakim LM, Bevan MJ. Cross-Dressed Dendritic Cells Drive Memory CD8+ T-Cell Activation After Viral Infection. Nature (2011) 471(7340):629–32. doi: 10.1038/nature09863
213. Foreman TW, Mehra S, LoBato DN, Malek A, Alvarez X, Golden NA, et al. CD4+ T-Cell-Independent Mechanisms Suppress Reactivation of Latent Tuberculosis in a Macaque Model of HIV Coinfection. Proc Natl Acad Sci USA (2016) 113(38):E5636–44. doi: 10.1073/pnas.1611987113
214. Kranich J, Krautler NJ, Heinen E, Polymenidou M, Bridel C, Schildknecht A, et al. Follicular Dendritic Cells Control Engulfment of Apoptotic Bodies by Secreting Mfge8. J Exp Med (2008) 205(6):1293–302. doi: 10.1084/jem.20071019
215. Smith JP, Burton GF, Tew JG, Szakal AK. Tingible Body Macrophages in Regulation of Germinal Center Reactions. Dev Immunol (1998) 6(3-4):285–94. doi: 10.1155/1998/38923
216. Hanayama R, Tanaka M, Miyasaka K, Aozasa K, Koike M, Uchiyama Y, et al. Autoimmune Disease and Impaired Uptake of Apoptotic Cells in MFG-E8-Deficient Mice. Science (2004) 304(5674):1147–50. doi: 10.1126/science.1094359
217. Baumann I, Kolowos W, Voll RE, Manger B, Gaipl U, Neuhuber WL, et al. Impaired Uptake of Apoptotic Cells Into Tingible Body Macrophages in Germinal Centers of Patients With Systemic Lupus Erythematosus. Arthritis Rheum (2002) 46(1):191–201. doi: 10.1002/1529-0131(200201)46:1<191::AID-ART10027>3.0.CO;2-K
218. Schaible UE, Winau F, Sieling PA, Fischer K, Collins HL, Hagens K, et al. Apoptosis Facilitates Antigen Presentation to T Lymphocytes Through MHC-I and CD1 in Tuberculosis. Nat Med (2003) 9(8):1039–46. doi: 10.1038/nm906
219. Lauron EJ, Yang L, Elliott JI, Gainey MD, Fremont DH, Yokoyama WM. Cross-Priming Induces Immunodomination in the Presence of Viral MHC Class I Inhibition. PloS Pathog (2018) 14(2):e1006883. doi: 10.1371/journal.ppat.1006883
220. Sutiwisesak R, Hicks ND, Boyce S, Murphy KC, Papavinasasundaram K, Carpenter SM, et al. A Natural Polymorphism of Mycobacterium Tuberculosis in the esxH Gene Disrupts Immunodomination by the TB10.4-Specific CD8 T Cell Response. PloS Pathog (2020) 16(10):e1009000. doi: 10.1371/journal.ppat.1009000
221. Cadena AM, Fortune SM, Flynn JL. Heterogeneity in Tuberculosis. Nat Rev Immunol (2017) 17(11):691–702. doi: 10.1038/nri.2017.69
222. Kahnert A, Hopken UE, Stein M, Bandermann S, Lipp M, Kaufmann SH. Mycobacterium Tuberculosis Triggers Formation of Lymphoid Structure in Murine Lungs. J Infect Dis (2007) 195(1):46–54. doi: 10.1086/508894
223. Ardain A, Domingo-Gonzalez R, Das S, Kazer SW, Howard NC, Singh A, et al. Group 3 Innate Lymphoid Cells Mediate Early Protective Immunity Against Tuberculosis. Nature (2019) 570(7762):528–32. doi: 10.1038/s41586-019-1276-2
224. Foo SY, Phipps S. Regulation of Inducible BALT Formation and Contribution to Immunity and Pathology. Mucosal Immunol (2010) 3(6):537–44. doi: 10.1038/mi.2010.52
225. Vinuesa CG, Linterman MA, Goodnow CC, Randall KL. T Cells and Follicular Dendritic Cells in Germinal Center B-Cell Formation and Selection. Immunol Rev (2010) 237(1):72–89. doi: 10.1111/j.1600-065X.2010.00937.x
226. Neyt K, GeurtsvanKessel CH, Deswarte K, Hammad H, Lambrecht BN. Early IL-1 Signaling Promotes iBALT Induction After Influenza Virus Infection. Front Immunol (2016) 7:312. doi: 10.3389/fimmu.2016.00312
227. Hwang JY, Silva-Sanchez A, Carragher DM, Garcia-Hernandez ML, Rangel-Moreno J, Randall TD. Inducible Bronchus-Associated Lymphoid Tissue (iBALT) Attenuates Pulmonary Pathology in a Mouse Model of Allergic Airway Disease. Front Immunol (2020) 11:570661. doi: 10.3389/fimmu.2020.570661
228. Moyron-Quiroz JE, Rangel-Moreno J, Kusser K, Hartson L, Sprague F, Goodrich S, et al. Role of Inducible Bronchus Associated Lymphoid Tissue (iBALT) in Respiratory Immunity. Nat Med (2004) 10(9):927–34. doi: 10.1038/nm1091
229. Moyron-Quiroz JE, Rangel-Moreno J, Hartson L, Kusser K, Tighe MP, Klonowski KD, et al. Persistence and Responsiveness of Immunologic Memory in the Absence of Secondary Lymphoid Organs. Immunity (2006) 25(4):643–54. doi: 10.1016/j.immuni.2006.08.022
230. Halle S, Dujardin HC, Bakocevic N, Fleige H, Danzer H, Willenzon S, et al. Induced Bronchus-Associated Lymphoid Tissue Serves as a General Priming Site for T Cells and is Maintained by Dendritic Cells. J Exp Med (2009) 206(12):2593–601. doi: 10.1084/jem.20091472
231. Ulrichs T, Kosmiadi GA, Jorg S, Pradl L, Titukhina M, Mishenko V, et al. Differential Organization of the Local Immune Response in Patients With Active Cavitary Tuberculosis or With Nonprogressive Tuberculoma. J Infect Dis (2005) 192(1):89–97. doi: 10.1086/430621
232. Esaulova E, Das S, Singh DK, Choreno-Parra JA, Swain A, Arthur L, et al. The Immune Landscape in Tuberculosis Reveals Populations Linked to Disease and Latency. Cell Host Microbe (2021) 29(2):165–178 e8. doi: 10.1016/j.chom.2020.11.013
233. Carpenter SM, Nunes-Alves C, Booty MG, Way SS, Behar SM. A Higher Activation Threshold of Memory CD8+ T Cells Has a Fitness Cost That Is Modified by TCR Affinity During Tuberculosis. PloS Pathog (2016) 12(1):e1005380. doi: 10.1371/journal.ppat.1005380
234. Das S, Marin ND, Esaulova E, Ahmed M, Swain A, Rosa BA, et al. Lung Epithelial Signaling Mediates Early Vaccine-Induced CD4(+) T Cell Activation and Mycobacterium Tuberculosis Control. mBio (2021) 12(4):e0146821. doi: 10.1128/mBio.01468-21
235. Qin L, Waseem TC, Sahoo A, Bieerkehazhi S, Zhou H, Galkina EV, et al. Insights Into the Molecular Mechanisms of T Follicular Helper-Mediated Immunity and Pathology. Front Immunol (2018) 9:1884. doi: 10.3389/fimmu.2018.01884
236. Chiavolini D, Rangel-Moreno J, Berg G, Christian K, Oliveira-Nascimento L, Weir S, et al. Bronchus-Associated Lymphoid Tissue (BALT) and Survival in a Vaccine Mouse Model of Tularemia. PloS One (2010) 5(6):e11156. doi: 10.1371/journal.pone.0011156
237. Barone F, Nayar S, Campos J, Cloake T, Withers DR, Toellner KM, et al. IL-22 Regulates Lymphoid Chemokine Production and Assembly of Tertiary Lymphoid Organs. Proc Natl Acad Sci USA (2015) 112(35):11024–9. doi: 10.1073/pnas.1503315112
238. Treerat P, Prince O, Cruz-Lagunas A, Munoz-Torrico M, Salazar-Lezama MA, Selman M, et al. Novel Role for IL-22 in Protection During Chronic Mycobacterium Tuberculosis HN878 Infection. Mucosal Immunol (2017) 10(4):1069–81. doi: 10.1038/mi.2017.15
239. Eddens T, Elsegeiny W, Garcia-Hernadez ML, Castillo P, Trevejo-Nunez G, Serody K, et al. Pneumocystis-Driven Inducible Bronchus-Associated Lymphoid Tissue Formation Requires Th2 and Th17 Immunity. Cell Rep (2017) 18(13):3078–90. doi: 10.1016/j.celrep.2017.03.016
240. Khader SA, Rangel-Moreno J, Fountain JJ, Martino CA, Reiley WW, Pearl JE, et al. In a Murine Tuberculosis Model, the Absence of Homeostatic Chemokines Delays Granuloma Formation and Protective Immunity. J Immunol (2009) 183(12):8004–14. doi: 10.4049/jimmunol.0901937
241. Kaushal D, Mehra S, Didier PJ, Lackner AA. The non-Human Primate Model of Tuberculosis. J Med Primatol (2012) 41(3):191–201. doi: 10.1111/j.1600-0684.2012.00536.x
242. Barber DL, Mayer-Barber KD, Feng CG, Sharpe AH, Sher A. CD4 T Cells Promote Rather Than Control Tuberculosis in the Absence of PD-1-Mediated Inhibition. J Immunol (2011) 186(3):1598–607. doi: 10.4049/jimmunol.1003304
243. Fletcher HA, Snowden MA, Landry B, Rida W, Satti I, Harris SA, et al. T-Cell Activation is an Immune Correlate of Risk in BCG Vaccinated Infants. Nat Commun (2016) 7:11290. doi: 10.1038/ncomms11290
244. Hansen SG, Zak DE, Xu G, Ford JC, Marshall EE, Malouli D, et al. Prevention of Tuberculosis in Rhesus Macaques by a Cytomegalovirus-Based Vaccine. Nat Med (2018) 24(2):130–43. doi: 10.1038/nm.4473
245. Tait DR, Hatherill M, van der Meeren O, Ginsberg AM, Van Brakel E, Salaun B, et al. Final Analysis of a Trial of M72/AS01E Vaccine to Prevent Tuberculosis. N Engl J Med (2019) 381(25):2429–39. doi: 10.1056/NEJMoa1909953
246. Tanner R, Villarreal-Ramos B, Vordermeier HM, McShane H. The Humoral Immune Response to BCG Vaccination. Front Immunol (2019) 10:1317. doi: 10.3389/fimmu.2019.01317
247. Bekker LG, Dintwe O, Fiore-Gartland A, Middelkoop K, Hutter J, Williams A, et al. A Phase 1b Randomized Study of the Safety and Immunological Responses to Vaccination With H4:IC31, H56:IC31, and BCG Revaccination in Mycobacterium Tuberculosis-Uninfected Adolescents in Cape Town, South Africa. EClinicalMedicine (2020) 21:100313. doi: 10.1016/j.eclinm.2020.100313
248. Luabeya AK, Kagina BM, Tameris MD, Geldenhuys H, Hoff ST, Shi Z, et al. First-In-Human Trial of the Post-Exposure Tuberculosis Vaccine H56:IC31 in Mycobacterium Tuberculosis Infected and non-Infected Healthy Adults. Vaccine (2015) 33(33):4130–40. doi: 10.1016/j.vaccine.2015.06.051
249. Loxton AG, Knaul JK, Grode L, Gutschmidt A, Meller C, Eisele B, et al. Safety and Immunogenicity of the Recombinant Mycobacterium Bovis BCG Vaccine VPM1002 in HIV-Unexposed Newborn Infants in South Africa. Clin Vaccine Immunol (2017) 24(2):e00439–16. doi: 10.1128/CVI.00439-16
250. Grode L, Ganoza CA, Brohm C, Weiner J 3rd, Eisele B, Kaufmann SH. Safety and Immunogenicity of the Recombinant BCG Vaccine VPM1002 in a Phase 1 Open-Label Randomized Clinical Trial. Vaccine (2013) 31(9):1340–8. doi: 10.1016/j.vaccine.2012.12.053
251. Darrah PA, Zeppa JJ, Maiello P, Hackney JA, Wadsworth MH 32nd, Hughes TK, et al. Prevention of Tuberculosis in Macaques After Intravenous BCG Immunization. Nature (2020) 577(7788):95–102. doi: 10.1038/s41586-019-1817-8
252. Dijkman K, Sombroek CC, Vervenne RAW, Hofman SO, Boot C, Remarque EJ, et al. Prevention of Tuberculosis Infection and Disease by Local BCG in Repeatedly Exposed Rhesus Macaques. Nat Med (2019) 25(2):255–62. doi: 10.1038/s41591-018-0319-9
253. Dijkman K, Aguilo N, Boot C, Hofman SO, Sombroek CC, Vervenne RAW, et al. Pulmonary MTBVAC Vaccination Induces Immune Signatures Previously Correlated With Prevention of Tuberculosis Infection. Cell Rep Med (2021) 2(1):100187. doi: 10.1016/j.xcrm.2020.100187
254. Divangahi M, Aaby P, Khader SA, Barreiro LB, Bekkering S, Chavakis T, et al. Trained Immunity, Tolerance, Priming and Differentiation: Distinct Immunological Processes. Nat Immunol (2021) 22(1):2–6. doi: 10.1038/s41590-020-00845-6
255. Vierboom MPM, Dijkman K, Sombroek CC, Hofman SO, Boot C, Vervenne RAW, et al. Stronger Induction of Trained Immunity by Mucosal BCG or MTBVAC Vaccination Compared to Standard Intradermal Vaccination. Cell Rep Med (2021) 2(1):100185. doi: 10.1016/j.xcrm.2020.100185
256. Coler RN, Day TA, Ellis R, Piazza FM, Beckmann AM, Vergara J, et al. The TLR-4 Agonist Adjuvant, GLA-SE, Improves Magnitude and Quality of Immune Responses Elicited by the ID93 Tuberculosis Vaccine: First-in-Human Trial. NPJ Vaccines (2018) 3:34. doi: 10.1038/s41541-018-0057-5
257. Prados-Rosales R, Carreno L, Cheng T, Blanc C, Weinrick B, Malek A, et al. Enhanced Control of Mycobacterium Tuberculosis Extrapulmonary Dissemination in Mice by an Arabinomannan-Protein Conjugate Vaccine. PloS Pathog (2017) 13(3):e1006250. doi: 10.1371/journal.ppat.1006250
258. Andrews JR, Noubary F, Walensky RP, Cerda R, Losina E, Horsburgh CR. Risk of Progression to Active Tuberculosis Following Reinfection With Mycobacterium Tuberculosis. Clin Infect Dis (2012) 54(6):784–91. doi: 10.1093/cid/cir951
259. Teitelbaum R, Glatman-Freedman A, Chen B, Robbins JB, Unanue E, Casadevall A, et al. A mAb Recognizing a Surface Antigen of Mycobacterium Tuberculosis Enhances Host Survival. Proc Natl Acad Sci USA (1998) 95(26):15688–93. doi: 10.1073/pnas.95.26.15688
260. Pethe K, Alonso S, Biet F, Delogu G, Brennan MJ, Locht C, et al. The Heparin-Binding Haemagglutinin of M. Tuberculosis is Required for Extrapulmonary Dissemination. Nature (2001) 412(6843):190–4. doi: 10.1038/35084083
261. Bournazos S, Gupta A, Ravetch JV. The Role of IgG Fc Receptors in Antibody-Dependent Enhancement. Nat Rev Immunol (2020) 20(10):633–43. doi: 10.1038/s41577-020-00410-0
262. Lenzini L, Rottoli P, Rottoli L. The Spectrum of Human Tuberculosis. Clin Exp Immunol (1977) 27(2):230–7.
263. Forget A, Benoit JC, Turcotte R, Gusew-Chartrand N. Enhancement Activity of Anti-Mycobacterial Sera in Experimental Mycobacterium Bovis (BCG) Infection in Mice. Infect Immun (1976) 13(5):1301–6. doi: 10.1128/iai.13.5.1301-1306.1976
264. Jenum S, Tonby K, Rueegg CS, Ruhwald M, Kristiansen MP, Bang P, et al. A Phase I/II Randomized Trial of H56:IC31 Vaccination and Adjunctive Cyclooxygenase-2-Inhibitor Treatment in Tuberculosis Patients. Nat Commun (2021) 12(1):6774. doi: 10.1038/s41467-021-27029-6
265. Penn-Nicholson A, Tameris M, Smit E, Day TA, Musvosvi M, Jayashankar L, et al. Safety and Immunogenicity of the Novel Tuberculosis Vaccine ID93 + GLA-SE in BCG-Vaccinated Healthy Adults in South Africa: A Randomised, Double-Blind, Placebo-Controlled Phase 1 Trial. Lancet Respir Med (2018) 6(4):287–98. doi: 10.1016/S2213-2600(18)30077-8
266. Coler RN, Bertholet S, Pine SO, Orr MT, Reese V, Windish HP, et al. Therapeutic Immunization Against Mycobacterium Tuberculosis is an Effective Adjunct to Antibiotic Treatment. J Infect Dis (2013) 207(8):1242–52. doi: 10.1093/infdis/jis425
267. Polack FP, Thomas SJ, Kitchin N, Absalon J, Gurtman A, Lockhart S, et al. Safety and Efficacy of the BNT162b2 mRNA Covid-19 Vaccine. N Engl J Med (2020) 383(27):2603–15. doi: 10.1056/NEJMoa2034577
268. Baden LR, El Sahly HM, Essink B, Kotloff K, Frey S, Novak R, et al. Efficacy and Safety of the mRNA-1273 SARS-CoV-2 Vaccine. N Engl J Med (2021) 384(5):403–16. doi: 10.1056/NEJMoa2035389
269. Bruhns P, Jonsson F. Mouse and Human FcR Effector Functions. Immunol Rev (2015) 268(1):25–51. doi: 10.1111/imr.12350
270. de Haan N, Reiding KR, Kristic J, Hipgrave Ederveen AL, Lauc G, Wuhrer M. The N-Glycosylation of Mouse Immunoglobulin G (IgG)-Fragment Crystallizable Differs Between IgG Subclasses and Strains. Front Immunol (2017) 8:608. doi: 10.3389/fimmu.2017.00608
271. Smith P, DiLillo DJ, Bournazos S, Li F, Ravetch JV. Mouse Model Recapitulating Human Fcgamma Receptor Structural and Functional Diversity. Proc Natl Acad Sci USA (2012) 109(16):6181–6. doi: 10.1073/pnas.1203954109
272. Murphy AJ, Macdonald LE, Stevens S, Karow M, Dore AT, Pobursky K, et al. Mice With Megabase Humanization of Their Immunoglobulin Genes Generate Antibodies as Efficiently as Normal Mice. Proc Natl Acad Sci USA (2014) 111(14):5153–8. doi: 10.1073/pnas.1324022111
273. Flynn JL. Lessons From Experimental Mycobacterium Tuberculosis Infections. Microbes Infect (2006) 8(4):1179–88. doi: 10.1016/j.micinf.2005.10.033
274. Mangtani P, Abubakar I, Ariti C, Beynon R, Pimpin L, Fine PE, et al. Protection by BCG Vaccine Against Tuberculosis: A Systematic Review of Randomized Controlled Trials. Clin Infect Dis (2014) 58(4):470–80. doi: 10.1093/cid/cit790
275. Kurtz SL, Rossi AP, Beamer GL, Gatti DM, Kramnik I, Elkins KL. The Diversity Outbred Mouse Population Is an Improved Animal Model of Vaccination Against Tuberculosis That Reflects Heterogeneity of Protection. mSphere (2020) 5(2):e00097–20. doi: 10.1128/mSphere.00097-20
276. Smith CM, Proulx MK, Olive AJ, Laddy D, Mishra BB, Moss C, et al. Tuberculosis Susceptibility and Vaccine Protection Are Independently Controlled by Host Genotype. mBio (2016) 7(5):e01516–16. doi: 10.1128/mBio.01516-16
277. Plumlee CR, Duffy FJ, Gern BH, Delahaye JL, Cohen SB, Stoltzfus CR, et al. Ultra-Low Dose Aerosol Infection of Mice With Mycobacterium Tuberculosis More Closely Models Human Tuberculosis. Cell Host Microbe (2021) 29(1):68–82 e5. doi: 10.1016/j.chom.2020.10.003
278. Colangeli R, Gupta A, Vinhas SA, Chippada Venkata UD, Kim S, Grady C, et al. Mycobacterium Tuberculosis Progresses Through Two Phases of Latent Infection in Humans. Nat Commun (2020) 11(1):4870. doi: 10.1038/s41467-020-18699-9
279. Warsinske HC, Rao AM, Moreira FMF, Santos PCP, Liu AB, Scott M, et al. Assessment of Validity of a Blood-Based 3-Gene Signature Score for Progression and Diagnosis of Tuberculosis, Disease Severity, and Treatment Response. JAMA Netw Open (2018) 1(6):e183779. doi: 10.1001/jamanetworkopen.2018.3779
280. Mulenga H, Zauchenberger CZ, Bunyasi EW, Mbandi SK, Mendelsohn SC, Kagina B, et al. Performance of Diagnostic and Predictive Host Blood Transcriptomic Signatures for Tuberculosis Disease: A Systematic Review and Meta-Analysis. PloS One (2020) 15(8):e0237574. doi: 10.1371/journal.pone.0237574
281. Mave V, Chandrasekaran P, Chavan A, Shivakumar S, Danasekaran K, Paradkar M, et al. Infection Free "Resisters" Among Household Contacts of Adult Pulmonary Tuberculosis. PloS One (2019) 14(7):e0218034. doi: 10.1371/journal.pone.0218034
282. Seshadri C, Sedaghat N, Campo M, Peterson G, Wells RD, Olson GS, et al. Transcriptional Networks are Associated With Resistance to Mycobacterium Tuberculosis Infection. PloS One (2017) 12(4):e0175844. doi: 10.1371/journal.pone.0175844
283. Kroon EE, Kinnear CJ, Orlova M, Fischinger S, Shin S, Boolay S, et al. An Observational Study Identifying Highly Tuberculosis-Exposed, HIV-1-Positive But Persistently TB, Tuberculin and IGRA Negative Persons With M. Tuberculosis Specific Antibodies in Cape Town, South Africa. EBioMedicine (2020) 61:103053. doi: 10.1016/j.ebiom.2020.103053
284. Jain SK, Tobin DM, Tucker EW, Venketaraman V, Ordonez AA, Jayashankar L, et al. Tuberculous Meningitis: A Roadmap for Advancing Basic and Translational Research. Nat Immunol (2018) 19(6):521–5. doi: 10.1038/s41590-018-0119-x
Keywords: antibody, Fc receptor, B cell, tuberculosis, Fc effector function, iBALT, antigen presentation, T cell
Citation: Carpenter SM and Lu LL (2022) Leveraging Antibody, B Cell and Fc Receptor Interactions to Understand Heterogeneous Immune Responses in Tuberculosis. Front. Immunol. 13:830482. doi: 10.3389/fimmu.2022.830482
Received: 07 December 2021; Accepted: 07 February 2022;
Published: 17 March 2022.
Edited by:
R. Keith Reeves, Duke University, United StatesReviewed by:
Frank Verreck, Biomedical Primate Research Centre (BPRC), NetherlandsAmanda Jezek Martinot, Tufts University, United States
Copyright © 2022 Carpenter and Lu. This is an open-access article distributed under the terms of the Creative Commons Attribution License (CC BY). The use, distribution or reproduction in other forums is permitted, provided the original author(s) and the copyright owner(s) are credited and that the original publication in this journal is cited, in accordance with accepted academic practice. No use, distribution or reproduction is permitted which does not comply with these terms.
*Correspondence: Lenette L. Lu, bGVuZXR0ZS5sdUB1dHNvdXRod2VzdGVybi5lZHU=