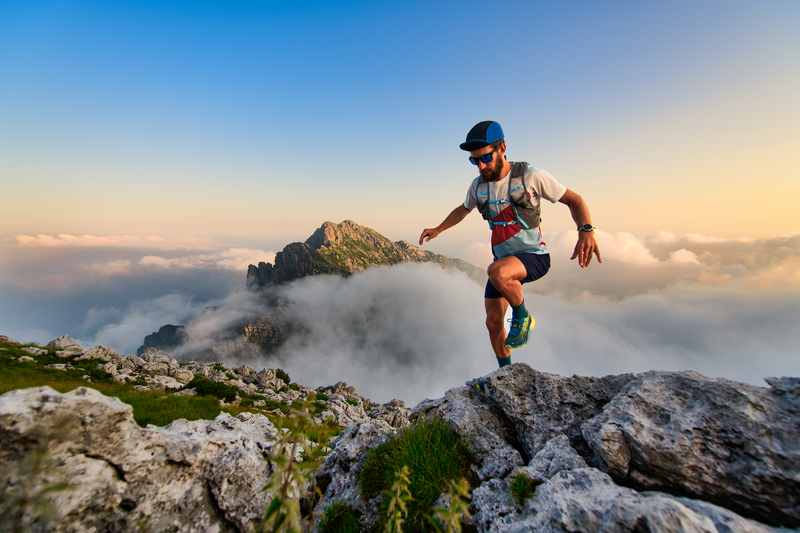
95% of researchers rate our articles as excellent or good
Learn more about the work of our research integrity team to safeguard the quality of each article we publish.
Find out more
MINI REVIEW article
Front. Immunol. , 27 January 2022
Sec. Molecular Innate Immunity
Volume 13 - 2022 | https://doi.org/10.3389/fimmu.2022.827250
This article is part of the Research Topic Insights in Molecular Innate Immunity: 2021 View all 13 articles
Recent evidence shows that innate immune cells, in addition to B and T cells, can retain immunological memory of their encounters and afford long-term resistance against infections in a process known as ‘trained immunity’. However, the duration of the unspecific protection observed in vivo is poorly compatible with the average lifespan of innate immune cells, suggesting the involvement of long-lived cells. Accordingly, recent studies demonstrate that hematopoietic stem and progenitor cells (HSPCs) lay at the foundation of trained immunity, retaining immunological memory of infections and giving rise to a “trained” myeloid progeny for a long time. In this review, we discuss the research demonstrating the involvement of HSPCs in the onset of long-lasting trained immunity. We highlight the roles of specific cytokines and Toll-like receptor ligands in influencing HSPC memory phenotypes and the molecular mechanisms underlying trained immunity HSPCs. Finally, we discuss the potential benefits and drawbacks of the long-lasting trained immune responses, and describe the challenges that the field is facing.
Traditionally, the immune system of vertebrates has been binary classified into the innate and adaptive arms of immunity. While cells of the innate arm promptly recognize and respond to infections through pathogen recognition receptors (PRRs), cells of the adaptive arm rely on the somatic recombination of genes encoding immunoglobulin domains to recognise non-self molecules (1). Although immunological studies on plants and invertebrates – both lacking adaptive immune responses – have suggested that these organisms can become increasingly resistant to reinfections, it was long believed that only B and T cells can confer immunological memory to their host (2–4).
In the last 10 years, a growing body of evidence has demonstrated that innate immune cells can also develop immunological memory in vertebrates – a phenomenon that is termed ‘trained immunity’ or ‘innate immune memory’ (5). In vertebrates, certain cells have been found to develop trained immunity, i.e., monocytes (6), dendritic cells (7), neutrophils (8), and innate lymphoid cells such as NK cells (9) and ILC1s (10). This process mainly relies on the reprogramming of cellular metabolic pathways and the alteration of epigenetic markers regulating the expression of different sets of genes (6, 11–13). While trained immunity generally refers to the induction of an hyperinflammatory phenotype, it was demonstrated that also the generation of opposite phenotypes, such as in the case of ‘endotoxin tolerance’, relies on similar mechanisms (12, 14, 15). Although the molecular basis of trained immunity has mostly been described in vitro using human monocytes and in vivo using murine models of infection, a few studies have demonstrated that the same mechanisms also apply in vivo to humans (16–18). Similarly, epidemiological studies proved that whole-organism vaccinations (such as Bacillus Calmette-Guerin [BCG], smallpox, and measles vaccines) afford unspecific protection against infections not specifically targeted by the vaccines, resulting in a reduction in all-cause mortality (19–22). Recent studies demonstrated that long-lived, self-renewing cells are involved in the onset of trained immunity and, indeed, participate in long-lasting protection against pathogens. For example, alveolar macrophages are able to replenish populations of tissue-resident macrophages in the lungs independently of bone marrow (BM)-derived monocytes and were shown to offer cross-protection against lung pathogens after an initial intranasal challenge with Acinetobacter baumannii (23, 24). However, the duration and extent of the unspecific peripheral protection in vivo (which is measured in years) is poorly compatible with the average lifespan of human monocytes (5 to 7 days), suggesting that other long-lived cells, such as hematopoietic stem and progenitor cells (HSPCs, with lifespans ranging from 10 to 60 months), might be involved in this phenomenon (25–27). In agreement with this hypothesis, several studies published in the last few years suggest that specific signals carried by HSPCs are able to induce trained immunity in these cells, which, in turn, gives rise to a pool of ‘trained’ myeloid progeny.
Here, we present the latest advancements in the field of trained immunity, describe the known mechanisms of trained immunity in HSPCs, and discuss the challenges and remaining controversies that the field is facing.
During infection and inflammation, immune effector cells are rapidly consumed at the sites of infection. As a result, HSPCs need to adapt their proliferation and differentiation rates to meet the increased demand of blood cells. To this end, HSPCs respond to different signals coming either from the BM niche, from distal inflamed sites, or from the infectious agents themselves (28). HSPCs have long been known to respond to multiple cytokines; however, several studies have demonstrated that both murine and human HSPCs express different Toll-like receptors (TLRs), and that direct engagement of these receptors with their ligands can alter the cell differentiation programming and, thus, their cellular output (28–32). HSPCs can also egress from the BM and migrate to tissues, where they can give rise to local populations of immune cells – a process known as ‘extramedullary haematopoiesis’ (33). Interestingly, different studies have reported that HSPCs can home back to the BM after migrating to peripheral tissues, increasing their chance of encountering TLR ligands and building a long-term memory of these encounters (34, 35).
In recent years, several studies have demonstrated that both human and murine HSPC compartments are at the foundation of the long-lasting ‘peripheral’ trained immunity observed in vivo (17). As we will discuss in the next chapters, some of these reports suggest that direct signaling from cytokines and microbial ligands to HSPCs play a central role in the reprogramming of HSPCs and their progeny (Table 1).
Table 1 Experimental studies demonstrating the direct involvement of HSPCs in the induction of innate immune memory.
Cytokines and growth factors play crucial roles in haematopoiesis by regulating HSPC quiescence, proliferation and differentiation (44). Similarly, signaling from many cytokines, such as G-CSF, GM-CSF, M-CSF, IL-6, IL-1β, and Type-I and II IFNs, control the transition to emergency haematopoiesis, i.e., the adaptation of the hematopoietic process during infection (28).
Two recent studies demonstrated that stimulation with β-glucan (a fungal cell wall component known to be a strong inducer of trained immunity in monocytes) also induces long-term trained immunity in murine HSPCs and that IL-1β plays a key role in HSPC reprogramming (6, 36, 37). Mitroulis and colleagues showed that, in a murine model, the intraperitoneal injection of β-glucan acted on CD41+ myeloid-skewed long-term hematopoietic stem cells (LT-HSCs), resulting in the expansion of granulocyte-monocyte progenitors (GMPs) and the reduction of lymphoid-skewed multipotent progenitors (MPP4). These myeloid skews in the HSPCs populations were accompanied by the profound metabolic reprogramming of cKit+ progenitors, which showed increased glycolytic activity and alterations in their cholesterol biosynthesis pathway. Compared to PBS-treated animals, mice primed with β-glucan showed a protective response to a later lipopolysaccharide (LPS) injection, as demonstrated by the enhanced expansion of multipotent progenitors (MPPs) and Lin- Sca1+ cKit+ (LSK) cells in the BM and by the mitigation of DNA damage at the level of LT-HSCs. Interestingly, the authors found that IL-1β signaling was required for the metabolic reprogramming and lineage skewing of LT-HSCs following β-glucan administration, as the pharmacological inhibition of IL-1 by the IL-1 receptor antagonist Anakinra completely abrogated these effects (36). Similarly, in a study by Moorlag and colleagues, β-glucan administration caused the expansion of LT-HSCs, MPPs and GMPs, which was abolished in IL-1R−/− mice as well as wildtype (WT) mice that received Anakinra. Priming with β-glucan also protected the mice against pulmonary infection with virulent Mycobacterium tuberculosis (Mtb), possibly as a result of the enhanced expression of antimicrobial cytokines by myeloid cells. Again, the protective effect provided by β-glucan was completely abolished in IL-1R−/− mice and in Anakinra-treated WT mice, suggesting that IL-1 plays a key role in the induction of protective trained immunity in HSPCs (37).
IL-1 was also found to have a prominent role in the induction of innate immune reprogramming following a western diet regime. This study employed the Ldlr-/- arteriosclerosis mouse model and showed that, when fed a western diet, these animals developed a systemic inflammatory response and long-lasting hyper-responsiveness of myeloid cells that continued after switching back to a chow diet. Mechanistically, the western diet induced the persistent transcriptional and epigenetic reprogramming of BM GMPs, which was mediated by NLRP3 activation and the subsequent release of IL-1. Accordingly, this phenotype was largely blunted in Nlrp3-/- Ldlr-/- mice as well as in animals receiving a recombinant IL-1ra (43).
Type-I and II interferons (IFNs) were also reported to play a key role in the induction of protective or detrimental innate immune memory in murine HSPCs. Two recent studies employed different models of BCG or Mtb infection in mice to determine their effect on the hematopoietic compartment (27, 38). Interestingly, both studies demonstrated that, to affect HSPC biology, the bacteria had to reach the BM, although LSK cells were not directly infected, suggesting an indirect effect was involved. Kaufman and colleagues showed that HSCs isolated from mice exposed to BCG were myeloid-skewed and able to generate an epigenetically reprogrammed myeloid progeny which protected against Mtb infection in vivo (38). The authors suggested that INF-γ signaling by HSCs was required for the generation of this protective response, as mice lacking the IFN-γ receptor failed to generate macrophages with a protective phenotype. Similarly, Khan et al. compared the responses of the hematopoietic compartment to either BCG or virulent Mtb infection (27). They found that Mtb, but not BCG, reduced myelopoiesis by inducing the Ripk3-dependent necroptosis of common myeloid progenitors (CMPs) and GMPs, and impaired HSC engraftment potential. Compared to BCG-exposed HSCs, cells exposed to Mtb showed an upregulation of Stat1 and type-I IFN targets, thus suggesting the involvement of IFN-α or IFN-β in this process. Concordantly, mice lacking the type-I IFN receptor Ifnar1 were afforded more protection against Mtb infection comparted with WT animals. Similarly, the systemic administration of poly(I:C) – a strong inducer of type-I IFNs – recapitulated the negative impact on myelopoiesis observed in Mtb-infected animals, which was completely reverted in Ifnar1-/- mice. Taken together, these studies suggest that the balance between type-I and type-II IFN signaling by HSPCs plays a key role in the induction of a detrimental or protective innate immune memory in vivo. However, different studies demonstrated that chronic exposure of murine and human HSCs to IFN-γ impairs their maintenance and self-renewal, indicating that the route of infection and the duration of the stimulus play key roles in the regulation of HSC fate (45, 46).
Finally, using a murine model of voluntary exercise, Frodermann and colleagues showed that, by diminishing plasma levels of the hormone leptin, exercise reduced HSPC proliferation and lineage commitment, resulting in fewer circulating leukocytes (39). In particular, reduced leptin signaling resulted in the increased production of ‘quiescence-inducing factors’ by LepR+ stromal cells which, in turn, reduced the LSK chromatin accessibility of multiple genes involved in proliferation and myelopoiesis for several weeks after exercise interruption. Surprisingly, compared to sedentary mice, exercised mice were more fully protected against LPS injection and caecal ligation and puncture, as highlighted by their lower mortality and increased cellular output. Although the authors failed to identify the nature of these quiescence-inducing factors, it is likely that cytokines such as SCF are involved in the reprogramming of HSCs in the BM niche (47, 48).
In the last two decades, several studies have demonstrated that both human and murine HSPCs express many PRRs (e.g., TLR-1, -2, -3, -4, -6, -7, -8, NOD2) and are able to respond to their engagement by activating specific downstream signaling pathways (29, 49, 50). TLR ligation on HSPCs, in particular, promotes their exit from quiescence and proliferation and results in a differentiation skew that favours a myeloid over a lymphoid cell fate (31, 32, 49, 50). Moreover, stimulation with TLR ligands induces cytokine secretion in virtually all HSPC subsets (50–52).
More recently, reports suggest that HSPCs stimulated with various TLR-ligands show memory-like traits, particularly those affecting the functionality of their myeloid progeny. For example, several studies from the Gil and Goodridge labs showed that in vitro differentiation of murine Lin-HSPCs exposed to TLR2 and TLR4 ligands (either in vitro or in vivo) generate macrophages with a decreased ability to release proinflammatory cytokines and reactive oxygen species in response to TLR ligation compared to control cells. However, HSPCs differentiated in the presence of Candida albicans yeast generated macrophages with a heightened production of inflammatory cytokines and improved fungicidal activity (52–55). Although they outlined a strong rationale for the involvement of HSPCs in building systemic trained immunity, these studies failed to determine the duration of these memory traits in HSPCs or conclude whether TLR ligation, rather than the production of autocrine soluble factors, is uniquely responsible for the observed phenotypes. A recent study, however, showed that the latter is likely to be the case: while direct TLR2 ligation on Lin-cells was required to promote myeloid differentiation, the differential response of macrophages derived from cells exposed to TLR2 and dectin-1 ligands was completely dependent on HSPC secretome release in response to these ligands (40). Interestingly, as LT-HSC do not express dectin-1, it is possible that myeloid-committed progenitors expressing dectin-1 are responsible for the secretion of training cytokines and factors (56).
However, it was recently reported that the exposure of HSPCs to LPS is sufficient to induce long-lasting innate memory in murine HSPCs (41). Using in vivo models of mixed BM chimaera and LSK cell transplantation, the authors showed that LPS-exposed LT-HSCs provide long-term protection against Pseudomonas aeruginosa infection for more than 12 weeks. This phenomenon relied on the persistent epigenetic reprogramming of HSCs, which showed increased chromatin accessibility, especially in myeloid enhancers, which were later re-activated during reinfection. Interestingly, direct signaling through TLR4 was fundamental to the induction of immune memory in HSCs, as TLR4−/− HSCs almost completely lost the epigenetic signature induced by LPS exposure in the TLR4−/−:WT BM cell chimaera. Furthermore, the authors found that, while not necessary for inducing the initial response to LPS by HSCs, C/EBPβ transcription factor (TF) was required to maintain the long-term accessibility of regulatory elements controlling the expression of myeloid and inflammatory genes during re-infection (41).
C/EBPβ and C/EBPα were also found to play key roles in the differentiation of human CD34+ cells towards the myeloid lineage in response to LPS in vitro. In this case, however, LPS activated a TLR4-MD2-MyD88 pathway that resulted in the expression of IL-6, which in turn, induced C/EBPα and C/EBPβ phosphorylation, finally promoting monocytic-macrophage differentiation (57). Direct TLR4 triggering of HSCs also plays an important role in the maintenance of HSC fitness. Research showed that the TLR4-MyD88 pathway in HSPCs seems to regulate myeloid suppression, while the TLR4-TRIF pathway plays a key role in regulating HSC proliferation (30, 58, 59). Concordantly, in vivo LPS and Salmonella typhimurium infection resulted in the strong proliferation of LT-HSCs through a TRIF-ROS-p38 pathway, which resulted in proliferative stress, DNA damage, and the long-term impairment of their repopulating ability (30).
Among non-canonical TLR4 ligands, heme was recently described to induce trained immunity in human monocytes as well as murine HSPCs (42, 60, 61). In fact, a single injection of heme protected a mouse model of polymicrobial sepsis from death when challenged 7 days post heme-administration. When sepsis was induced 28 days after the initial heme training, this protection completely vanished. A further analysis of BM HSPCs 28 days after heme training revealed that chromatin accessibility in the ST-HSC and MPP3 subsets was substantially different than that in control mice. These differences further reflected the alteration in the chromatin accessibility to TFs that are important for the maintenance of stemness and the promotion of myelopoiesis (e.g., Gata2, Runx1, Nfi) (42).
Finally, it was recently revealed that young and aged LT-HSCs display different memory traits after TLR activation. In aged LT-HSCs, but not in young cells, brief ex vivo exposure to TLR2 and TLR4 ligands induced the expansion of peripheral myeloid cells and a reduction in lymphoid cells 3 months after transplantation. This was possibly the result of the initial expansion of a CD61+ subset of LT-HSCs in the aged mice, which were found to be more proliferative and intrinsically prone to a myeloid output (62).
Taken together, these studies suggest that direct TLR ligation on HSPCs plays an important role in the terminal differentiation of the cells as well as the establishment of innate immune memory. Interestingly, both direct TLR signaling and the indirect signaling autocrinally induced by the production of cytokines seem to be equally involved in this complex phenomenon.
A growing body of evidence, examples of which are presented in this review, highlights the key roles of HSPCs in maintaining the long-lasting aspects of trained immunity. However, several questions remain to be addressed regarding the conundrum of trained immunity and its implications.
Although most of the studies support the idea that trained immunity phenotypes are retained for a very long time, a key remaining aspect of trained immunity that still has to be clearly defined, is the specific duration of such phenotype in vivo in humans. This, as we will discuss later, has important implications on the possible translatability of such evidence to human health. Studies using murine models showed that BM HSPCs can retain an epigenetic memory, affecting the response of their myeloid progeny for over 1 year (27). Similarly, epidemiological studies suggest that BCG vaccination might afford unspecific protection for up to 5 years (63). Although it is still a matter of discussion and an object of ongoing clinical trials, recent epidemiological studies that aimed to assess the protective effects of BCG vaccination against SARS-CoV-2 infections showed that a history of BCG vaccination was associated with a decreased rate of infection and an overall improvement in the symptomatology (64, 65). Furthermore, a recent study suggested that HSCs can establish and retain a persistent epigenetic memory (characterised by drastic differences in DNA methylation and chromatin accessibility) imposed during their development, which eventually direct HSC functions during exposure to stressors (66). It is, therefore, likely that the duration of such an innate immune memory in HSCs can be measured in the context of years and that the additional effects of subsequent stresses and infections on such cells are maintained lifelong. Finally, a breakthrough study recently demonstrated that unspecific protection against bacterial infections afforded by a sublethal infection with C. albicans was transmitted intergenerationally and transgenerationally for up to two generations (67). Strikingly, the progeny of C. albicans-exposed mice inherited an epigenetic signature induced by the fungal insult from the HSPC populations of their parents, resulting in improved myeloid cell output and activation and increased survival following E. coli infection. These results are in agreement with a randomized controlled trial showing that the reduction in mortality observed in BCG-vaccinated infants was significantly improved if the mother also had a history of BGC vaccination (68). Understanding the specific duration of trained immunity induced by different agents (e.g. BCG, β-glucans) will be fundamental to design effective therapies.
Most of the studies performed so far have demonstrated that the typical enhancement of myeloid cell activation afforded by trained immunity leads to the cells having a protective role against unspecific infections, however, less is known about the putative side effects that proinflammatory poising of the myeloid compartment might have in other scenarios (69). The proinflammatory poising of myeloid cells can undoubtedly be useful during infection; nevertheless, there is evidence that it can contribute to inflammatory diseases. For example, NLRP3 activation and IL-1 release during a western diet are known to cause the reprogramming of BM GMPs, resulting in proinflammatory poising of the myeloid compartment (43). Chronic exposure to IL-1 negatively affects the HSC pool, reducing their self-renewing capacity and restricting their lineage output (70). On a similar note, a single LPS injection was found to induce a trained phenotype of murine microglia; this in turn, promoted the neuropathology in a model of Alzheimer’s disease, increasing both amyloid-β levels and the plaque load, and augmented IL-1β levels after brain ischaemia (71).
The hypothesis of a detrimental trained immunity response is also supported by a study on patients who were affected by hyper-IgD syndrome (HIDS), in which recurring attacks of sterile inflammation occur. Compared to healthy donors, the monocytes from these patients showed a trained immunity phenotype characterised by the enhanced production of inflammatory cytokines (both at the basal level and during infection) as well as an H3K27ac signature partially overlapping with that of in vitro trained monocytes. The authors speculated that this phenotype could be induced by the accumulation of mevalonate during HIDS which, in turn, activates the AKT-mTOR pathway (possibly through IGF1-R) and finally amplifies the molecular cascade that controls the epigenetic rewiring of trained monocytes (13). Similar observations linking the proinflammatory phenotype of monocytes to a characteristic epigenetic signature were made on patients affected by symptomatic arteriosclerosis (72) and coronary artery disease (73). Taken together, these studies point to a possible detrimental role of trained immunity, where monocytes (and possibly other myeloid cells) participate in the maintenance and worsening of inflammatory pathologies (Figure 1).
Figure 1 Impact of trained immunity during lifetime. Current studies suggest that trained immunity can have both beneficial and detrimental effects. β-glucan administration is known to induce a long-lasting reprograming of myeloid cells that results in the unspecific protection against different pathogens. BCG vaccination in children is known to afford protection and reduce all-cause mortality; similarly, recent clinical trials suggest that BCG vaccination in elderly can reduce the plasma levels of inflammatory cytokines and mediators, and to afford unspecific protection against respiratory infections. Voluntary exercise also induces analogous effects, decreasing the proliferation and lineage commitment of HSPCs while affording a long-term protection during sepsis. On the other side, western diet was found to reprogram bone marrow granulocyte progenitors, increasing the reactivity of innate immune cells. Similarly, signaling by metabolic intermediates as mevalonate was suggested to be involved in the pathological hyperinflammation affecting patients suffering from hyper-IgD syndrome. Finally, sepsis is also known to induce a long-term immunosuppression in survivors, possibly through mechanisms similar to those inducing trained immunity in hematopoietic progenitors and myeloid cells.
This gains even greater importance in the light of the long-lasting effect exerted on the HSPCs pool. It is, therefore, paramount to fully explore and identify these potential side effects before applying any treatment in the clinical practice. As a matter of fact, the pharmacological control of trained immunity is gaining more and more interest from the scientific community as a tool to boost the immunity of frail patients (74). Two recent clinical trials showed the promising effects of BCG re-vaccination in the elderly (75, 76). However, other researchers have speculated that continuous triggering of the immune system throughout life and, thus, the constant induction of trained immunity on HSPCs, could be the missing link between aging and the persistent low-grade inflammation observed in the elderly that is known as ‘inflammaging’ (77, 78). Therefore, dissecting the molecular mechanisms driving beneficial or detrimental trained immunity in HSPCs will be a fundamental step in the development of novel pharmacological strategies or towards the repurposing of already approved therapies. The induction of trained immunity can be achieved through stimulation with different PRR-ligands (especially ligands for dectin-1 and NOD2), while inhibition of trained immunity can be accomplished by interfering with metabolic pathways or epigenetic regulators (79). As such, great hope is placed on the use of small molecules as regulating epigenetic modifiers (80).
Taken together, the studies presented in this review clearly put the HSPC compartment at the foundation of the long-term effects exerted by trained immunity. Direct induction of trained immunity in HSPCs results in the production of a myeloid progeny with a trained phenotype directly inherited from their progenitors. Although different studies point toward different factors, direct signaling by both cytokines (as IFNs and IL-1) and PRR ligands (as LPS and β-glucans) is fundamental for the induction of the metabolic and epigenetic rewiring in HSPCs responsible for the induction of trained immunity in HSPCs.
As the pharmaceutical control of trained immunity is gaining more and more interest by the scientific community, some aspects of this phenomenon still require thorough investigation in order to design effective therapeutic strategies. In particular, several studies report that trained immunity might participate in the onset and worsening of inflammatory pathologies such as arteriosclerosis. Knowing the derailments that can occur during the induction of trained immunity represent a key step for the development of safe treatments. Secondly, it is still not known the exact and specific duration of trained immunity induced by different factors. This would allow the design of effective ‘training therapies’ aimed to boost the innate response against infections without promoting an undesirable hyperinflammatory bias of the immune system which can lead to the development (or worsening) of inflammatory diseases. Finally, unravelling the common molecular pathways involved in the onset of trained immunity will allow the precise control of the balance between unspecific protection and undesirable hyperinflammation.
We believe that trained immunity represents a unique phenomenon that can be harnesser to design game changing-therapies: the direction is clear and the path paved.
MZ conceived and wrote the manuscript, and secured funding. JF supervised the writing and reviewed the manuscript, and secured funding. Both authors read and approved the final manuscript.
This work was supported by the Ministry of Health of the Czech Republic, grant no. NU21J-05-00056 and DRO (Institute of Haematology and Blood Transfusion—UHKT, 00023736), and by the European Social Fund and European Regional Development Fund—Project ENOCH (no. CZ.02.1.01/0.0/0.0/16_019/0000868). MZ was supported by the European Regional Development Fund—Project Support of MSCA IF fellowships at FNUSA-ICRC (no CZ.02.2.69/0.0/0.0/19_074/0016274).
The authors declare that the research was conducted in the absence of any commercial or financial relationships that could be construed as a potential conflict of interest.
All claims expressed in this article are solely those of the authors and do not necessarily represent those of their affiliated organizations, or those of the publisher, the editors and the reviewers. Any product that may be evaluated in this article, or claim that may be made by its manufacturer, is not guaranteed or endorsed by the publisher.
The authors would like to thank Dr Jessica Tamanini of Insight Editing London for critically reviewing the manuscript before submission.
1. Cooper MD, Alder MN. The Evolution of Adaptive Immune Systems. Cell (2006) 124(4):815–22. doi: 10.1016/j.cell.2006.02.001
2. Gourbal B, Pinaud S, Beckers GJM, van der Meer JWM, Conrath U, Netea MG. Innate Immune Memory: An Evolutionary Perspective. Immunol Rev (2018) 283(1):21–40. doi: 10.1111/imr.12647
3. Melillo D, Marino R, Italiani P, Boraschi D. Innate Immune Memory in Invertebrate Metazoans: A Critical Appraisal. Front Immunol (2018) 9:1915. doi: 10.3389/fimmu.2018.01915
4. Reimer-Michalski EM, Conrath U. Innate Immune Memory in Plants. Semin Immunol (2016) 28(4):319–27. doi: 10.1016/j.smim.2016.05.006
5. Netea MG, Dominguez-Andres J, Barreiro LB, Chavakis T, Divangahi M, Fuchs E, et al. Defining Trained Immunity and Its Role in Health and Disease. Nat Rev Immunol (2020) 20(6):375–88. doi: 10.1038/s41577-020-0285-6
6. Quintin J, Saeed S, Martens JHA, Giamarellos-Bourboulis EJ, Ifrim DC, Logie C, et al. Candida Albicans Infection Affords Protection Against Reinfection via Functional Reprogramming of Monocytes. Cell Host Microbe (2012) 12(2):223–32. doi: 10.1016/j.chom.2012.06.006
7. Hole CR, Wager CML, Castro-Lopez N, Campuzano A, Cai H, Wozniak KL, et al. Induction of Memory-Like Dendritic Cell Responses In Vivo. Nat Commun (2019) 10(1):2955. doi: 10.1038/s41467-019-10486-5
8. Moorlag SJCFM, Rodriguez-Rosales YA, Gillard J, Fanucchi S, Theunissen K, Novakovic B, et al. BCG Vaccination Induces Long-Term Functional Reprogramming of Human Neutrophils. Cell Rep (2020) 33(7):108387. doi: 10.1016/j.celrep.2020.108387
9. Romee R, Rosario M, Berrien-Elliott MM, Wagner JA, Jewell BA, Schappe T, et al. Cytokine-Induced Memory-Like Natural Killer Cells Exhibit Enhanced Responses Against Myeloid Leukemia. Sci Transl Med (2016) 8(357):357ra123. doi: 10.1126/scitranslmed.aaf2341
10. Weizman OE, Song E, Adams NM, Hildreth AD, Riggan L, Krishna C, et al. Mouse Cytomegalovirus-Experienced ILC1s Acquire a Memory Response Dependent on the Viral Glycoprotein M12. Nat Immunol (2019) 20(8):1004–11. doi: 10.1038/s41590-019-0430-1
11. Cheng SC, Quintin J, Cramer RA, Shepardson KM, Saeed S, Kumar V, et al. Mtor- and HIF-1alpha-Mediated Aerobic Glycolysis as Metabolic Basis for Trained Immunity. Science (2014) 345(6204):1250684. doi: 10.1126/science.1250684
12. Saeed S, Quintin J, Kerstens HH, Rao NA, Aghajanirefah A, Matarese F, et al. Epigenetic Programming of Monocyte-to-Macrophage Differentiation and Trained Innate Immunity. Science (2014) 345(6204):1251086. doi: 10.1126/science.1251086
13. Bekkering S, Arts RJW, Novakovic B, Kourtzelis I, van der Heijden C, Li Y, et al. Metabolic Induction of Trained Immunity Through the Mevalonate Pathway. Cell (2018) 172(1-2):135–46.e9. doi: 10.1016/j.cell.2017.11.025
14. Foster SL, Hargreaves DC, Medzhitov R. Gene-Specific Control of Inflammation by TLR-Induced Chromatin Modifications. Nature (2007) 447(7147):972–8. doi: 10.1038/nature05836
15. Novakovic B, Habibi E, Wang SY, Arts RJW, Davar R, Megchelenbrink W, et al. Beta-Glucan Reverses the Epigenetic State of LPS-Induced Immunological Tolerance. Cell (2016) 167(5):1354–68.e14. doi: 10.1016/j.cell.2016.09.034
16. Arts RJW, Moorlag S, Novakovic B, Li Y, Wang SY, Oosting M, et al. BCG Vaccination Protects Against Experimental Viral Infection in Humans Through the Induction of Cytokines Associated With Trained Immunity. Cell Host Microbe (2018) 23(1):89–100.e5. doi: 10.1016/j.chom.2017.12.010
17. Cirovic B, de Bree LCJ, Groh L, Blok BA, Chan J, van der Velden W, et al. BCG Vaccination in Humans Elicits Trained Immunity via the Hematopoietic Progenitor Compartment. Cell Host Microbe (2020) 28(2):322–34.e5. doi: 10.1016/j.chom.2020.05.014
18. Kleinnijenhuis J, Quintin J, Preijers F, Joosten LA, Ifrim DC, Saeed S, et al. Bacille Calmette-Guerin Induces NOD2-Dependent Nonspecific Protection From Reinfection via Epigenetic Reprogramming of Monocytes. Proc Natl Acad Sci USA (2012) 109(43):17537–42. doi: 10.1073/pnas.1202870109
19. Aaby P, Martins CL, Garly ML, Bale C, Andersen A, Rodrigues A, et al. Non-Specific Effects of Standard Measles Vaccine at 4.5 and 9 Months of Age on Childhood Mortality: Randomised Controlled Trial. BMJ (2010) 341:c6495. doi: 10.1136/bmj.c6495
20. Aaby P, Roth A, Ravn H, Napirna BM, Rodrigues A, Lisse IM, et al. Randomized Trial of BCG Vaccination at Birth to Low-Birth-Weight Children: Beneficial Nonspecific Effects in the Neonatal Period? J Infect Dis (2011) 204(2):245–52. doi: 10.1093/infdis/jir240
21. Biering-Sorensen S, Aaby P, Napirna BM, Roth A, Ravn H, Rodrigues A, et al. Small Randomized Trial Among Low-Birth-Weight Children Receiving Bacillus Calmette-Guerin Vaccination at First Health Center Contact. Pediatr Infect Dis J (2012) 31(3):306–8. doi: 10.1097/INF.0b013e3182458289
22. Sorup S, Villumsen M, Ravn H, Benn CS, Sorensen TI, Aaby P, et al. Smallpox Vaccination and All-Cause Infectious Disease Hospitalization: A Danish Register-Based Cohort Study. Int J Epidemiol (2011) 40(4):955–63. doi: 10.1093/ije/dyr063
23. Gu H, Zeng X, Peng L, Xiang C, Zhou Y, Zhang X, et al. Vaccination Induces Rapid Protection Against Bacterial Pneumonia via Training Alveolar Macrophage in Mice. Elife (2021) 10:e69951. doi: 10.7554/eLife.69951
24. Hashimoto D, Chow A, Noizat C, Teo P, Beasley MB, Leboeuf M, et al. Tissue-Resident Macrophages Self-Maintain Locally Throughout Adult Life With Minimal Contribution From Circulating Monocytes. Immunity (2013) 38(4):792–804. doi: 10.1016/j.immuni.2013.04.004
25. Patel AA, Zhang Y, Fullerton JN, Boelen L, Rongvaux A, Maini AA, et al. The Fate and Lifespan of Human Monocyte Subsets in Steady State and Systemic Inflammation. J Exp Med (2017) 214(7):1913–23. doi: 10.1084/jem.20170355
26. Sieburg HB, Rezner BD, Muller-Sieburg CE. Predicting Clonal Self-Renewal and Extinction of Hematopoietic Stem Cells. Proc Natl Acad Sci USA (2011) 108(11):4370–5. doi: 10.1073/pnas.1011414108
27. Khan N, Downey J, Sanz J, Kaufmann E, Blankenhaus B, Pacis A, et al. M. Tuberculosis Reprograms Hematopoietic Stem Cells to Limit Myelopoiesis and Impair Trained Immunity. Cell (2020) 183(3):752–70.e22. doi: 10.1016/j.cell.2020.09.062
28. Boettcher S, Manz MG. Regulation of Inflammation- and Infection-Driven Hematopoiesis. Trends Immunol (2017) 38(5):345–57. doi: 10.1016/j.it.2017.01.004
29. Nagai Y, Garrett KP, Ohta S, Bahrun U, Kouro T, Akira S, et al. Toll-Like Receptors on Hematopoietic Progenitor Cells Stimulate Innate Immune System Replenishment. Immunity (2006) 24(6):801–12. doi: 10.1016/j.immuni.2006.04.008
30. Takizawa H, Fritsch K, Kovtonyuk LV, Saito Y, Yakkala C, Jacobs K, et al. Pathogen-Induced TLR4-TRIF Innate Immune Signaling in Hematopoietic Stem Cells Promotes Proliferation But Reduces Competitive Fitness. Cell Stem Cell (2017) 21(2):225–40.e5. doi: 10.1016/j.stem.2017.06.013
31. Megias J, Yanez A, Moriano S, O’Connor JE, Gozalbo D, Gil ML. Direct Toll-Like Receptor-Mediated Stimulation of Hematopoietic Stem and Progenitor Cells Occurs In Vivo and Promotes Differentiation Toward Macrophages. Stem Cells (2012) 30(7):1486–95. doi: 10.1002/stem.1110
32. Sioud M, Floisand Y, Forfang L, Lund-Johansen F. Signaling Through Toll-Like Receptor 7/8 Induces the Differentiation of Human Bone Marrow CD34+ Progenitor Cells Along the Myeloid Lineage. J Mol Biol (2006) 364(5):945–54. doi: 10.1016/j.jmb.2006.09.054
33. Crane GM, Jeffery E, Morrison SJ. Adult Haematopoietic Stem Cell Niches. Nat Rev Immunol (2017) 17(9):573–90. doi: 10.1038/nri.2017.53
34. Wright DE, Wagers AJ, Gulati AP, Johnson FL, Weissman IL. Physiological Migration of Hematopoietic Stem and Progenitor Cells. Science (2001) 294(5548):1933–6. doi: 10.1126/science.1064081
35. Massberg S, Schaerli P, Knezevic-Maramica I, Kollnberger M, Tubo N, Moseman EA, et al. Immunosurveillance by Hematopoietic Progenitor Cells Trafficking Through Blood, Lymph, and Peripheral Tissues. Cell (2007) 131(5):994–1008. doi: 10.1016/j.cell.2007.09.047
36. Mitroulis I, Ruppova K, Wang B, Chen LS, Grzybek M, Grinenko T, et al. Modulation of Myelopoiesis Progenitors Is an Integral Component of Trained Immunity. Cell (2018) 172(1-2):147–61.e12. doi: 10.1016/j.cell.2017.11.034
37. Moorlag S, Khan N, Novakovic B, Kaufmann E, Jansen T, van Crevel R, et al. Beta-Glucan Induces Protective Trained Immunity Against Mycobacterium Tuberculosis Infection: A Key Role for IL-1. Cell Rep (2020) 31(7):107634. doi: 10.1016/j.celrep.2020.107634
38. Kaufmann E, Sanz J, Dunn JL, Khan N, Mendonca LE, Pacis A, et al. BCG Educates Hematopoietic Stem Cells to Generate Protective Innate Immunity Against Tuberculosis. Cell (2018) 172(1-2):176–90.e19. doi: 10.1016/j.cell.2017.12.031
39. Frodermann V, Rohde D, Courties G, Severe N, Schloss MJ, Amatullah H, et al. Exercise Reduces Inflammatory Cell Production and Cardiovascular Inflammation via Instruction of Hematopoietic Progenitor Cells. Nat Med (2019) 25(11):1761–71. doi: 10.1038/s41591-019-0633-x
40. Bono C, Martinez A, Megias J, Gozalbo D, Yanez A, Gil ML. Dectin-1 Stimulation of Hematopoietic Stem and Progenitor Cells Occurs In Vivo and Promotes Differentiation Toward Trained Macrophages via an Indirect Cell-Autonomous Mechanism. mBio (2020) 11(3):e00781–20. doi: 10.1128/mBio.00781-20
41. de Laval B, Maurizio J, Kandalla PK, Brisou G, Simonnet L, Huber C, et al. C/Ebpbeta-Dependent Epigenetic Memory Induces Trained Immunity in Hematopoietic Stem Cells. Cell Stem Cell (2020) 26(5):657–74.e8. doi: 10.1016/j.stem.2020.01.017
42. Jentho E, Ruiz-Moreno C, Novakovic B, Kourtzelis I, Megchelenbrink WL, Martins R, et al. Trained Innate Immunity, Long-Lasting Epigenetic Modulation, and Skewed Myelopoiesis by Heme. Proc Natl Acad Sci USA (2021) 118(42):e2102698118. doi: 10.1073/pnas.2102698118
43. Christ A, Gunther P, Lauterbach MAR, Duewell P, Biswas D, Pelka K, et al. Western Diet Triggers Nlrp3-Dependent Innate Immune Reprogramming. Cell (2018) 172(1-2):162–75.e14. doi: 10.1016/j.cell.2017.12.013
44. Kaushansky K. Lineage-Specific Hematopoietic Growth Factors. N Engl J Med (2006) 354(19):2034–45. doi: 10.1056/NEJMra052706
45. Yang L, Dybedal I, Bryder D, Nilsson L, Sitnicka E, Sasaki Y, et al. IFN-Gamma Negatively Modulates Self-Renewal of Repopulating Human Hemopoietic Stem Cells. J Immunol (2005) 174(2):752–7. doi: 10.4049/jimmunol.174.2.752
46. de Bruin AM, Demirel O, Hooibrink B, Brandts CH, Nolte MA. Interferon-Gamma Impairs Proliferation of Hematopoietic Stem Cells in Mice. Blood (2013) 121(18):3578–85. doi: 10.1182/blood-2012-05-432906
47. Comazzetto S, Murphy MM, Berto S, Jeffery E, Zhao Z, Morrison SJ. Restricted Hematopoietic Progenitors and Erythropoiesis Require SCF From Leptin Receptor+ Niche Cells in the Bone Marrow. Cell Stem Cell (2019) 24(3):477–86.e6. doi: 10.1016/j.stem.2018.11.022
48. Ding L, Saunders TL, Enikolopov G, Morrison SJ. Endothelial and Perivascular Cells Maintain Haematopoietic Stem Cells. Nature (2012) 481(7382):457–62. doi: 10.1038/nature10783
49. De Luca K, Frances-Duvert V, Asensio MJ, Ihsani R, Debien E, Taillardet M, et al. The TLR1/2 Agonist PAM(3)CSK(4) Instructs Commitment of Human Hematopoietic Stem Cells to a Myeloid Cell Fate. Leukemia (2009) 23(11):2063–74. doi: 10.1038/leu.2009.155
50. Sioud M, Floisand Y. NOD2/CARD15 on Bone Marrow CD34+ Hematopoietic Cells Mediates Induction of Cytokines and Cell Differentiation. J Leukoc Biol (2009) 85(6):939–46. doi: 10.1189/jlb.1008650
51. Zhao JL, Ma C, O’Connell RM, Mehta A, DiLoreto R, Heath JR, et al. Conversion of Danger Signals Into Cytokine Signals by Hematopoietic Stem and Progenitor Cells for Regulation of Stress-Induced Hematopoiesis. Cell Stem Cell (2014) 14(4):445–59. doi: 10.1016/j.stem.2014.01.007
52. Martinez A, Bono C, Megias J, Yanez A, Gozalbo D, Gil ML. Systemic Candidiasis and TLR2 Agonist Exposure Impact the Antifungal Response of Hematopoietic Stem and Progenitor Cells. Front Cell Infect Microbiol (2018) 8:309. doi: 10.3389/fcimb.2018.00309
53. Megias J, Martinez A, Yanez A, Goodridge HS, Gozalbo D, Gil ML. TLR2, TLR4 and Dectin-1 Signalling in Hematopoietic Stem and Progenitor Cells Determines the Antifungal Phenotype of the Macrophages They Produce. Microbes Infect (2016) 18(5):354–63. doi: 10.1016/j.micinf.2016.01.005
54. Martinez A, Bono C, Megias J, Yanez A, Gozalbo D, Gil ML. PRR Signaling During In Vitro Macrophage Differentiation From Progenitors Modulates Their Subsequent Response to Inflammatory Stimuli. Eur Cytokine Netw (2017) 28(3):102–10. doi: 10.1684/ecn.2017.0398
55. Yanez A, Hassanzadeh-Kiabi N, Ng MY, Megias J, Subramanian A, Liu GY, et al. Detection of a TLR2 Agonist by Hematopoietic Stem and Progenitor Cells Impacts the Function of the Macrophages They Produce. Eur J Immunol (2013) 43(8):2114–25. doi: 10.1002/eji.201343403
56. Yanez A, Megias J, O’Connor JE, Gozalbo D, Gil ML. Candida Albicans Induces Selective Development of Macrophages and Monocyte Derived Dendritic Cells by a TLR2 Dependent Signalling. PloS One (2011) 6(9):e24761. doi: 10.1371/journal.pone.0024761
57. Sasaki Y, Guo YM, Goto T, Ubukawa K, Asanuma K, Kobayashi I, et al. IL-6 Generated From Human Hematopoietic Stem and Progenitor Cells Through TLR4 Signaling Promotes Emergency Granulopoiesis by Regulating Transcription Factor Expression. J Immunol (2021) 207(4):1078–86. doi: 10.4049/jimmunol.2100168
58. Zhang H, Rodriguez S, Wang L, Wang S, Serezani H, Kapur R, et al. Sepsis Induces Hematopoietic Stem Cell Exhaustion and Myelosuppression Through Distinct Contributions of TRIF and MYD88. Stem Cell Rep (2016) 6(6):940–56. doi: 10.1016/j.stemcr.2016.05.002
59. Liu A, Wang Y, Ding Y, Baez I, Payne KJ, Borghesi L. Cutting Edge: Hematopoietic Stem Cell Expansion and Common Lymphoid Progenitor Depletion Require Hematopoietic-Derived, Cell-Autonomous TLR4 in a Model of Chronic Endotoxin. J Immunol (2015) 195(6):2524–8. doi: 10.4049/jimmunol.1501231
60. Figueiredo RT, Fernandez PL, Mourao-Sa DS, Porto BN, Dutra FF, Alves LS, et al. Characterization of Heme as Activator of Toll-Like Receptor 4. J Biol Chem (2007) 282(28):20221–9. doi: 10.1074/jbc.M610737200
61. Janciauskiene S, Vijayan V, Immenschuh S. TLR4 Signaling by Heme and the Role of Heme-Binding Blood Proteins. Front Immunol (2020) 11:1964. doi: 10.3389/fimmu.2020.01964
62. Mann M, Mehta A, de Boer CG, Kowalczyk MS, Lee K, Haldeman P, et al. Heterogeneous Responses of Hematopoietic Stem Cells to Inflammatory Stimuli Are Altered With Age. Cell Rep (2018) 25(11):2992–3005.e5. doi: 10.1016/j.celrep.2018.11.056
63. Nankabirwa V, Tumwine JK, Mugaba PM, Tylleskar T, Sommerfelt H, Group P-ES. Child Survival and BCG Vaccination: A Community Based Prospective Cohort Study in Uganda. BMC Public Health (2015) 15:175. doi: 10.1186/s12889-015-1497-8
64. Moorlag S, van Deuren RC, van Werkhoven CH, Jaeger M, Debisarun P, Taks E, et al. Safety and COVID-19 Symptoms in Individuals Recently Vaccinated With BCG: A Retrospective Cohort Study. Cell Rep Med (2020) 1(5):100073. doi: 10.1016/j.xcrm.2020.100073
65. Rivas MN, Ebinger JE, Wu M, Sun N, Braun J, Sobhani K, et al. BCG Vaccination History Associates With Decreased SARS-Cov-2 Seroprevalence Across a Diverse Cohort of Health Care Workers. J Clin Invest (2021) 131(2):e145157. doi: 10.1172/JCI145157
66. Yu VWC, Yusuf RZ, Oki T, Wu J, Saez B, Wang X, et al. Epigenetic Memory Underlies Cell-Autonomous Heterogeneous Behavior of Hematopoietic Stem Cells. Cell (2016) 167(5):1310–22.e17. doi: 10.1016/j.cell.2016.10.045
67. Katzmarski N, Dominguez-Andres J, Cirovic B, Renieris G, Ciarlo E, Le Roy D, et al. Transmission of Trained Immunity and Heterologous Resistance to Infections Across Generations. Nat Immunol (2021) 22(11):1382–90. doi: 10.1038/s41590-021-01052-7
68. Berendsen MLT, Oland CB, Bles P, Jensen AKG, Kofoed PE, Whittle H, et al. Maternal Priming: Bacillus Calmette-Guerin (BCG) Vaccine Scarring in Mothers Enhances the Survival of Their Child With a BCG Vaccine Scar. J Pediatr Infect Dis Soc (2020) 9(2):166–72. doi: 10.1093/jpids/piy142
69. DiNardo AR, Netea MG, Musher DM. Postinfectious Epigenetic Immune Modifications - a Double-Edged Sword. N Engl J Med (2021) 384(3):261–70. doi: 10.1056/NEJMra2028358
70. Pietras EM, Mirantes-Barbeito C, Fong S, Loeffler D, Kovtonyuk LV, Zhang S, et al. Chronic Interleukin-1 Exposure Drives Haematopoietic Stem Cells Towards Precocious Myeloid Differentiation at the Expense of Self-Renewal. Nat Cell Biol (2016) 18(6):607–18. doi: 10.1038/ncb3346
71. Wendeln AC, Degenhardt K, Kaurani L, Gertig M, Ulas T, Jain G, et al. Innate Immune Memory in the Brain Shapes Neurological Disease Hallmarks. Nature (2018) 556(7701):332–8. doi: 10.1038/s41586-018-0023-4
72. Bekkering S, van den Munckhof I, Nielen T, Lamfers E, Dinarello C, Rutten J, et al. Innate Immune Cell Activation and Epigenetic Remodeling in Symptomatic and Asymptomatic Atherosclerosis in Humans In Vivo. Atherosclerosis (2016) 254:228–36. doi: 10.1016/j.atherosclerosis.2016.10.019
73. Shirai T, Nazarewicz RR, Wallis BB, Yanes RE, Watanabe R, Hilhorst M, et al. The Glycolytic Enzyme PKM2 Bridges Metabolic and Inflammatory Dysfunction in Coronary Artery Disease. J Exp Med (2016) 213(3):337–54. doi: 10.1084/jem.20150900
74. Bulut O, Kilic G, Dominguez-Andres J, Netea MG. Overcoming Immune Dysfunction in the Elderly: Trained Immunity as a Novel Approach. Int Immunol (2020) 32(12):741–53. doi: 10.1093/intimm/dxaa052
75. Giamarellos-Bourboulis EJ, Tsilika M, Moorlag S, Antonakos N, Kotsaki A, Dominguez-Andres J, et al. Activate: Randomized Clinical Trial of BCG Vaccination Against Infection in the Elderly. Cell (2020) 183(2):315–23.e9. doi: 10.1016/j.cell.2020.08.051
76. Pavan Kumar N, Padmapriyadarsini C, Rajamanickam A, Marinaik SB, Nancy A, Padmanaban S, et al. Effect of BCG Vaccination on Proinflammatory Responses in Elderly Individuals. Sci Adv (2021) 7(32):eabg7181. doi: 10.1126/sciadv.abg7181
77. Ferrucci L, Fabbri E. Inflammageing: Chronic Inflammation in Ageing, Cardiovascular Disease, and Frailty. Nat Rev Cardiol (2018) 15(9):505–22. doi: 10.1038/s41569-018-0064-2
78. Fulop T, Dupuis G, Baehl S, Le Page A, Bourgade K, Frost E, et al. From Inflamm-Aging to Immune-Paralysis: A Slippery Slope During Aging for Immune-Adaptation. Biogerontology (2016) 17(1):147–57. doi: 10.1007/s10522-015-9615-7
79. Mulder WJM, Ochando J, Joosten LAB, Fayad ZA, Netea MG. Therapeutic Targeting of Trained Immunity. Nat Rev Drug Discovery (2019) 18(7):553–66. doi: 10.1038/s41573-019-0025-4
Keywords: trained immunity, hematopoietic stem cells, HSPCs, innate immunity, myeloid cells, progenitor cells
Citation: De Zuani M and Frič J (2022) Train the Trainer: Hematopoietic Stem Cell Control of Trained Immunity. Front. Immunol. 13:827250. doi: 10.3389/fimmu.2022.827250
Received: 01 December 2021; Accepted: 12 January 2022;
Published: 27 January 2022.
Edited by:
Francesca Granucci, University of Milano-Bicocca, ItalyReviewed by:
María Luisa Gil, University of Valencia, SpainCopyright © 2022 De Zuani and Frič. This is an open-access article distributed under the terms of the Creative Commons Attribution License (CC BY). The use, distribution or reproduction in other forums is permitted, provided the original author(s) and the copyright owner(s) are credited and that the original publication in this journal is cited, in accordance with accepted academic practice. No use, distribution or reproduction is permitted which does not comply with these terms.
*Correspondence: Jan Frič, amFuLmZyaWNAZm51c2EuY3o=
Disclaimer: All claims expressed in this article are solely those of the authors and do not necessarily represent those of their affiliated organizations, or those of the publisher, the editors and the reviewers. Any product that may be evaluated in this article or claim that may be made by its manufacturer is not guaranteed or endorsed by the publisher.
Research integrity at Frontiers
Learn more about the work of our research integrity team to safeguard the quality of each article we publish.