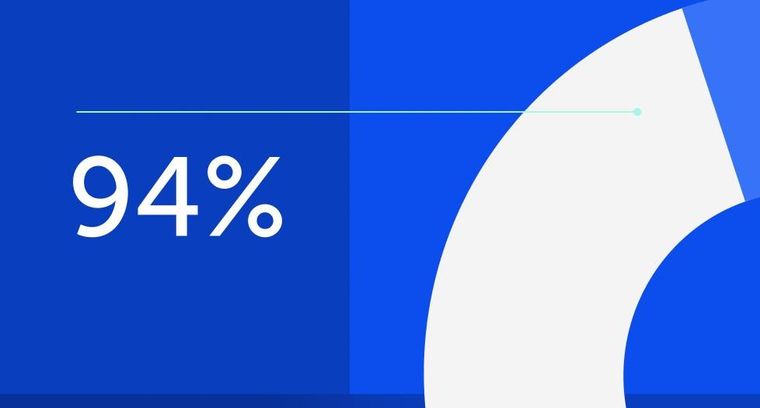
94% of researchers rate our articles as excellent or good
Learn more about the work of our research integrity team to safeguard the quality of each article we publish.
Find out more
REVIEW article
Front. Immunol., 20 April 2022
Sec. Autoimmune and Autoinflammatory Disorders
Volume 13 - 2022 | https://doi.org/10.3389/fimmu.2022.822995
Microparticles (MPs) are small (100 nm – 1 um) extracellular vesicles derived from the plasma membrane of dying or activated cells. MPs are important mediators of intercellular communication, transporting proteins, nucleic acids and lipids from the parent cell to other cells. MPs resemble the state of their parent cells and are easily accessible when released into the blood or urine. MPs also play a role in the pathogenesis of different diseases and are considered as potential biomarkers. MP isolation and characterization is technically challenging and results in different studies are contradictory. Therefore, uniform guidelines to isolate and characterize MPs should be developed. Our understanding of MP biology and how MPs play a role in different pathological mechanisms has greatly advanced in recent years. MPs, especially if derived from apoptotic cells, possess strong immunogenic properties due to the presence of modified proteins and nucleic acids. MPs are often found in patients with autoimmune diseases where MPs for example play a role in the break of immunological tolerance and/or induction of inflammatory conditions. In this review, we describe the main techniques to isolate and characterize MPs, define the characteristics of MPs generated during cell death, illustrate different mechanism of intercellular communication via MPs and summarize the role of MPs in pathological mechanisms with a particular focus on autoimmune diseases.
Microparticles (MPs) are a subclass of extracellular vesicles (EVs) that are a heterogeneous population of vesicles originating from cellular membranes. EVs are important for intercellular communication in a similar manner as cytokines, hormones and neurotransmitters. The release of EVs into the extracellular space occurs mainly via two different mechanisms, by cellular activation or during cell death [apoptosis, necroptosis, pyroptosis, reviewed in (1)] (Figures 1A, B). The smallest EVs are exosomes. Exosomes are formed by exocytosis of multivesicular bodies with size ranges from 50 nm to 100 nm (2). MPs (100 nm-1 µm) and apoptotic bodies (1 µm-4 µm), on the other hand, are formed by membrane shedding (2) and will be the main focus of this review. Formation of MPs by cell activation or apoptosis, is characterized by calcium influx into the cell. Calcium signaling eventually leads to changes in the cytoskeleton and budding of the cell membrane (3). MPs mediate the communication between different cells by carrying a wide range of molecules including receptors, proteins, RNA species such as miRNAs or other nuclear components (Figure 1C). Additionally, MPs can carry mitochondrial proteins, mitochondrial DNA and intact mitochondria [reviewed in (4)]. MPs released into the blood or urine are an easily accessible biomarker to detect differences in cells in response to physiological or pathological changes (5). Circulating MPs used as biomarkers for diagnostic and/or prognostic purposes, may lead to adjustment of therapy based on the individual needs of the patients. However, uncontrolled release of MPs as well as disturbed clearance of MPs, are associated with a wide range of pathologies (including e.g. cardiovascular dysfunction, cancer, autoimmunity) (6–10). In this review, we will focus on experimental techniques to isolate and characterize MPs, the interaction of MPs with immune cells and their pathological roles including induction of different types of cell death and their involvement in autoimmune conditions.
Figure 1 Distinct classes of extracellular vesicles. Upon cell activation (A) exosomes or microparticles are formed by exocytosis or membrane shedding, respectively. Microparticles and apoptotic bodies (B) are released from apoptotic cells. Extracellular vesicles transfer several molecules to target cells (C). miRNA: microRNA, MHC: major histocompatibility complex.
The technique used to isolate and characterize MPs from blood samples can have a great impact on the concentration and composition of the final MP population (11, 12). The variability in methods makes it challenging to compare results from different studies using divergent methods. To improve reproducibility, it is important to use reliable and standardized methods for isolation, quantification and characterization of MPs.
Various pre-analytical factors may influence the outcome of MP analysis. Important parameters to consider when examining MPs are: (i) the type of anticoagulant used in the blood sample; (ii) the time between collecting and processing blood samples; (iii) agitation during transport of blood samples; (iv) the protocol of MP isolation (discussed in more detail below); and (v) the storage of MP samples. The choice of anticoagulant can drastically influence MP counts in blood samples, with citrate being the most widely used and advised anticoagulant (13, 14). Time-delay until processing of blood samples and excessive agitation during transport have been shown to increase numbers of MPs, most likely due to activation of cells releasing MPs ex vivo after blood withdrawal (13, 15). The duration of storage can have an effect on MP integrity, and therefore, counts. Overall, long term storage tends to decrease MP counts (13, 16). Another factor that might influence the analysis of MPs is whether blood is collected pre- or post-prandial. Blood collected post-prandial is enriched in lipoproteins, which could be co-purified with MPs and interfere with analysis (17, 18).
Other body fluids, such as urine, can also be used for MP isolation. Several strategies have been developed for isolation of MPs from urine (19, 20), as urine derived MPs can be used as fluid biopsy reflecting kidney health (21). Especially, miRNA content of urinary MPs reflects different renal pathologies including IgA nephropathy (22). Various pre-analytical factors, such as time of sample collection, temperature and time of storage, influence the size as well as content of MPs isolated from urine (19).
Different MP isolation protocols may result in variations in number and characteristics of isolated MPs. Differential centrifugation offers an easy and relatively unbiased method of isolating MPs and is therefore the most frequently used method for isolation of MPs from different body fluids or cell culture supernatant. To isolate MPs from blood an initial centrifugation at ~300 x g is performed to obtain platelet-poor plasma. The initial centrifugation is followed by a second centrifugation with higher speeds (15,000 – 20,000 x g) to pellet the MPs. The protocol to isolate MPs from cell culture supernatants usually consists of two centrifugation steps: The first centrifugation step at low speed (800 – 1500 x g) aims to eliminate intact cells and/or large particles, whereas the second centrifugation step at high speed (15,000 – 20,000 x g) aims to pellet MPs. By using platelet poor plasma from healthy volunteers, Dey-Hazra et al. showed that a first centrifugation step at higher speed (5000 vs. 1500 x g) leads to more than 10-fold decrease in final concentration of MPs. However, using this method MPs could easier be separated from background noise in flow cytometry experiments (11, 15). The decreased MP counts obtained with an initial high speed centrifugation step can be attributed to reduced contamination by platelets and/or erythrocytes, thereby avoiding formation of MPs from these sources during the isolation process (16). In addition to affecting the total MP count, the isolation strategy can also have an effect on the relative abundance of MP subspecies derived from different cell types, such as platelets or endothelial cells (16). Differential centrifugation does not yield highly pure MP populations and it can typically contain protein aggregates [such as immune complexes (23)], aggregates of smaller vesicles (24) and lipoproteins (25). However, differential centrifugation is able to recover high amounts of MPs from the starting material compared to more stringent isolation methods, such as affinity isolation, and combinations of other techniques (26).
Other methods to isolate MPs from biological fluids include the use of microfluidic systems. These chip-based isolation systems offer the possibility to isolate MPs from very small sample volumes, which is not possible using differential centrifugation. Microfluidic approaches often use size-based filtration to isolate MPs. However, disadvantages of size-based filtration are clogging of the filter and disintegration of MPs thereby making it challenging to isolate sufficient numbers of MPs for further analysis (27, 28). Antibodies recognizing cell surface markers (such as CD41, to capture platelet-derived MPs or CD63, a tetraspanin often found in MPs) may also be applied to isolate MPs, thereby only enriching a subset of MPs (29–31). Development of an affinity-based MP isolation technique with antibodies requires immobilization of antibody molecules on solid media such as magnetic or agarose beads, and ELISA plates. An elution step with appropriate reagents has to be applied, if bead-MP complexes cannot be directly used for the downstream assays, or in case of coated ELISA plates, which may affect the integrity of isolated MPs (32). Elution-induced effects on MPs can be avoided with a recent method developed by Evander et al., which uses microscale acoustic trapping for non-contact capture of MPs and intact MP recovery (33). Other techniques to isolate MPs include density gradient centrifugation, precipitation, size exclusion chromatography and variations on those techniques [reviewed in (26)]. Differential centrifugation will remain the MP isolation technique of choice in the case of large sample volumes. For small sample volumes microfluidics-mediated methods could serve as alternative if future developments succeed in isolating intact MPs in an unbiased manner and with sufficient numbers for downstream analysis.
While the described isolation techniques result in reliable MP preparations, it is important to keep in mind that none of the described isolation techniques will achieve completely pure MP populations. Contaminants present in MP preparations may include platelets, plasmatic protein aggregates and lipoproteins (25, 34, 35). Therefore, potential contaminations need to be evaluated when analyzing MPs.
Flow cytometry is the main technique applied to analyze number and cellular origins of MPs (Figure 2). Flow cytometry analysis is restricted by the detection limit of the instrument allowing to analyze only larger MPs. The detection limit of flow cytometers used to be around 0.4 to 0.5 µm, however, state-of-the-art flow cytometers can analyze particles as small as 0.1 μm. Nevertheless, flow cytometry offers a high throughput technique to count and simultaneously analyze different markers to determine the source of MPs. Analysis of the source of MPs relies on different cell-specific markers present on MPs originating from different cell types. However, there is no consensus on cell markers used to identify MPs from e.g. platelets, endothelial cells or leukocytes (36) (Table 1), which makes it difficult to compare study outcomes. Assessing cell-specific markers on MPs using flow cytometry can be very challenging as the density of markers on small particles can be very low and therefore difficult to detect. Therefore, highly specialized flow cytometers have been developed for MP characterization [compared in (73)]. Another widely used technique to determine vesicular size and concentration is nanoparticle tracking analysis (NTA). NTA determines the size of vesicles by assessing the Brownian motion of vesicles using laser light scattering microscopy (74). Recent advances in NTA include the possibility to (immune)phenotype MPs by the use of fluorescently labelled antibodies, which in part resembles flow cytometric analysis using fluorescent antibodies (75, 76),
Figure 2 Flow cytometry as a technique to quantify and characterize MPs. By combining data on size (forward scatter), granularity (side scatter), and presence of markers of interest (obtained by use of fluorescently labeled antibodies), flow cytometry allows detection of number, cellular origin, and cargo of MPs.
Several groups reported multi-omic analysis of MPs, thereby analyzing the protein and lipid content of MPs (77–80). Kowal and colleagues showed that proteomic analysis of MPs using mass spectrometry led to the identification of proteins defining certain subtypes of vesicles (77). They found proteins uniquely present in bigger MPs (> 150 nm; such as GP96, actinin-4, mitofilin) as well as proteins only present in small MPs (50 – 150 nm; such as syntnein-1, tumor susceptibility 101 (TSG101), a disintegrin and metalloproteinase domain-containing protein 10 (ADAM10), EH domain-containing protein 4 (EHD4) or exosomes. Furthermore, they showed that markers originally used to define exosomes, such as major histocompatibility complex, flotillin and heat shock 70kDa proteins, are also found in larger MPs. In addition to size-based characterization of small MPs and exosomes, tetraspanin-based purification and characterization was applied by Kowal et al. By analyzing the (co)presence and/or (co)absence of CD9, CD63 and CD81, they showed that proteins associated with different stages of the endosomal pathway are enriched in different subgroups of small MPs. Similarly, analysis of CD63+ or CD81+ small MPs and exosomes from B cells revealed different subgroups (81), thereby underscoring the role of tetraspanins in cargo selection and targeting (82). Analysis of MPs by mass spectrometry can also be used to define MP characteristics specific for certain diseases. Ostergaard and colleagues analyzed the proteome of MPs isolated from patients with SLE (78). They found clear differences when comparing MPs from SLE-patients to MPs from healthy controls or to MPs from systemic sclerosis patients. MPs from patients with SLE lack proteins derived from mitochondria, have lower myosin:actin ratios and a high abundance of glycolytic enzymes. These results could lead to further insight into the mechanisms of MP release in SLE or even to more refined diagnostics.
Regulated cell death via apoptosis is essential for the proper functioning of the human body. Cells undergo apoptosis in all parts of the body and this process is normally tightly regulated. Two forms of apoptosis can be differentiated, namely intrinsic or extrinsic apoptosis [reviewed in (83)]. Intrinsic apoptosis is imitated by perturbations of the intracellular homeostasis, whereas extrinsic apoptosis is mostly triggered by the engagement of plasma membrane receptors. The key step to start the intrinsic apoptosis pathway is the permeabilization of the mitochondrial outer membrane by the BCL2 protein family (84, 85). Subsequently, cytochrome c is released from the mitochondria intermembrane space and activated the caspase cascade ultimately leading to the execution of the cellular remodeling observed during apoptosis (86, 87). Extrinsic apoptosis is triggered by the engagement of so-called death receptors (e.g. Fas-receptor) or by dependence receptors, a family of structurally unrelated membrane receptors that are activated as soon as their substrate concentration falls below a certain threshold level such as DCC (deleted in colorectal carcinoma), p75NTR (p75 neurotrophin receptor) (88–90). Again, both receptor types activate downstream signaling leading to caspase activation. During the process of apoptosis, cells shrink and form multiple blebs on the cell surface. Ultimately, these blebs are released from the parent cell and can enter the circulation as MPs (91, 92). Inherent to the mechanism of apoptosis is the redistribution of cellular components. Next to cytoplasmic proteins, especially nuclear components, such as DNA and histones can be found in apoptotic MPs (37, 93).
Apoptosis involves the tight regulation of major structural changes in the cell to ensure proper packaging of cellular constituents and removal of apoptotic cells by phagocytes in an immune silent manner, prior to the formation of MPs. Even though the exact mechanism of how MPs are formed is not completely understood, several regulators of the cytoskeleton seem to play a role in the formation and the sorting of cellular constituents into MPs. The intracellular increase of calcium concentration observed during MP formation, triggers various processes. First, cytoskeletal rearrangements allows the formation of membrane blebs as precursors of MPs. The calcium dependent protease calpain is involved in the cleavage of structural proteins connecting the plasma membrane with the cytoskeleton (3, 94). Other proteins involved in the remodeling of the cytoskeleton are small GTPases, such as ARF6 and RhoA (95–97). Through the remodeling and resorting of the cytoskeleton, cytoskeletal proteins and GTPases might also be involved in the packaging of specific cargo to the MPs (98). Whereas MPs can contain a myriad of different cargoes representing the entire contents of its parent cell, some studies suggest that MPs can also contain only specific constituents of the cell content (99). Finally, to facilitate release of preformed MPs Rho associated protein kinase (ROCK) and extracellular signal–regulated kinases (ERK) activate myosin light chains to induce contraction of the actin-myosin cytoskeleton (100–102).
Besides rearrangements of the cytoskeleton another prominent change during apoptosis is the reorganization of lipids in the plasma membrane of MPs. The best described change is the reorientation of phosphatidylserine (PS) from the inner lipid layer (inner leaflet) to the outer lipid layer (outer leaflet) of the double lipid layer in cellular membrane. Flippases are responsible for the transport of lipids from the outer leaflet to the inner leaflet and prevent the presence of PS in the outer leaflet of healthy cells. During apoptosis the energy dependent flippase mechanism is shut down and PS reaches the outer leaflet by the unspecific transport of lipids facilitated by scramblases (103). Localization of PS in the outer leaflet of apoptotic cells and ultimately also at the surface of apoptotic MPs serve as an ‘eat me’ signal to specialized phagocytes. Therefore, under normal conditions PS exposure is an efficient mechanism to remove apoptotic cells in a quick and immunologically silent manner. However, when early apoptotic cells are not swiftly cleared, they will proceed to late apoptotic cells, thereby releasing MPs. The relative abundance of PS positive and negative MPs can be affected by certain pathologies. For example, PS negative MPs are predominantly present in systemic lupus erythematosus patients while virtually absent in healthy controls (55, 59). To reliably distinguish MPs from large debris (cell remnants) or protein aggregates, many researchers use staining with Annexin V to identify PS on MPs (11, 16). However, the use of Annexin V as a general MP marker remains a matter of debate. For instance, apoptotic endothelial cell-derived MPs show more binding of Annexin V compared to TNF-α activated endothelial cell-derived MPs (38). Overall, PS at the surface of MPs is an important marker to distinguish between apoptotic and non-apoptotic populations of MPs, but PS is unsuited as a general MP marker.
Interestingly, if apoptotic MPs are not cleared properly, they can in turn induce different forms of cell death when interacting with other cells. MPs generated from Jurkat cells induce apoptosis of macrophages, thereby showing a marked increase in Annexin V staining as well as caspase activation in macrophages (104). Previously, we reported that apoptotic MPs generated from endothelial cells, represent MPs found in SLE patients, both in size and by the presence of specific chromatin modifications. These apoptotic MPs were able to induce dose-dependent neutrophil cell death via the formation of neutrophil extracellular traps (NETs) (93). The capability of apoptotic MPs to induce NET formation was found to be dependent on the presence of modified chromatin, especially acetylated histones. Importantly, apoptosis-modified histones within SLE MPs are highly pro-inflammatory, as demonstrated by their potency to activate myeloid and plasmacytoid dendritic cells (37, 93). As macrophages and neutrophils are phagocytotic cells that are specialized in clearing apoptotic material, the observed cell death induction in these cells by apoptotic MPs seems counterintuitive. The cell death of macrophages and neutrophils induced by apoptotic MPs might be explained by the pathologically high concentration of MPs or, in the case of SLE, by the specific pro-inflammatory cargo (e.g. histones) found in SLE-derived MPs.
MPs released from parent cells can have numerous effects on distant cells. MPs have thus been recognized as mediators of intercellular communication. MPs can exert their effects on other cells via different mechanisms (Figure 3): MPs can fuse with the target cell (Figure 3A), MPs can bind to receptors on the target cell by direct interaction (Figure 3B), MPs can be taken up by the target cell via endocytosis (Figure 3C), ultimately ending in the lysosome where its contents are degraded, and MPs can form immune complexes with antibodies (Figure 3D), which can either deposit in the microvasculature or stimulate other cells.
Figure 3 MP induce cellular response via different mechanisms. MPs can fuse with the target cell (A), stimulate receptors on the target cell by direct binding (B), release their content into the endosomal compartment (C), or form immune complexes with autoantibodies (D). miRNA: microRNA, MHC: major histocompatibility complex.
MPs can fuse with other cells to transfer proteins or other molecules from one cell to another. This transport of MPs has been proposed as a communication strategy between distant cells. MPs originating from activated endothelial cells have been described to carry the intercellular adhesion molecule-1 (ICAM-1) to endothelial cells elsewhere in the body (105). ICAM-1 is then incorporated into the plasma membrane of the target cell, thereby increasing its capability of recruiting immune cells. Besides the transfer of membrane associated receptors like ICAM-1, also the delivery of small non-coding RNAs via MPs has been demonstrated. Especially microRNA (miRNA) molecules have been described to be transported between cells (106). miRNAs are capable of regulating the translation of mRNA in the target cell and have been described to be present in MPs released from endothelial cells. These miRNAs can, for example, prevent excessive proliferation of other endothelial cells (107, 108).
MPs have been described to carry different types of immunoglobulins (Igs) (52, 109, 110). Immunoglobulins can be bound to the surface of MPs, but can also recognize components inside MPs (111, 112). MPs found in patients with SLE carry significantly more Ig compared to MPs from healthy controls (110). Additionally, MPs from patients with SLE are covered by depositions of complement proteins C1q and C3, thereby demonstrating that autoantibodies bound to MPs can initiate complement activation (113). A proteomic analysis of MP content in patients with SLE recently confirmed the presence of Ig and complement on MPs (78). MPs could thereby provide a scaffold for immune complex formation in autoimmune diseases (63). Immune complex coated MPs might be more immunogenic compared to MPs without immunoglobulins, since they are more likely to deposit in the microvasculature, thereby causing inflammation and tissue damage (114). However, also in vitro generated MPs without autoantibodies can confer inflammatory effects on other cells (93).
The endocytosis of MPs delivers autoantigens into the endosomal compartments of the target cells. Endocytosis is a rapid (115, 116), energy dependent (117, 118) process and it is facilitated by interactions between proteins of MPs and proteins of recipient cells (117–119). A combination of multiple protein-protein interactions rather than a single protein-protein interaction seems to direct MP uptake (120, 121), which most probably contributes to the specificity of MP delivery. As mentioned earlier, tetraspanins which are highly abundant on MPs such as CD9, CD63, CD81, were shown to be involved in vesicular fusion events contributing to the targeting and uptake of MPs (82, 122–125). Similarly, integrins including ICAM-1, CD11a, CD52, and CD61 contribute to the uptake of MPs (117, 126–128). Proteoglycans such as heparan sulfate proteoglycans (118) and lectins (126, 129) also appear to be involved in MP-recipient cell interaction and MP uptake. Several endocytic pathways including phagocytosis, clathrin- and caveolin-mediated endocytosis facilitate internalization of MPs [reviewed in (130)].
Endosomes containing MPs, might ultimately fuse with lysosomes leading to degradation of the endosomal content. Within the different endosomal compartments, receptors can sense the cargo of the received MPs. Nucleic acids and modified chromatin, especially present within MPs derived from apoptotic cells stimulate pattern-recognition receptors, such as Toll-like receptors (TLRs) (37, 93). The immunogenicity of MPs containing nucleic acids has been recently demonstrated by an elegant study showing the importance of the nuclease DNase1 like-3 (DNase1L3) in the degradation of nucleic acids in MPs. Lack of Dnase1L3 results in the development of lupus-like disease in mice with the characteristic production of high anti-DNA antibody titers (10).
Depending on the type of nucleic acid, either TLR7 (sensing single strand RNA) or TLR 9 (sensing DNA) can be activated (131). TLR ligation leads to the activation of intracellular signaling pathways, thereby leading to changes in gene expression, ultimately resulting in the activation of the target cell (132). Activation of cells can either initiate the production of pro-inflammatory cytokines, trigger the increase of activation markers on the plasma membrane or induce proliferation (64). For example, dendritic cell activation by MPs leads to an increased release of interferon (IFN) type I in SLE (37, 111, 133, 134). Type I IFNs then stimulates the survival and activation of dendritic cells, B-cells and T-cells (135). MPs carrying autoantigens derived from apoptotic cells might also facilitate direct B-cell activation by engagement of the B cell receptor, together with intracellular activation of TLRs (136). Together this potentially leads to the clonal expansion of autoreactive B-cell clones and might result in the break of tolerance in autoimmune diseases.
Upon internalization of MPs by antigen presenting cells, the content of MPs can be presented to T-cells. Alternatively, MPs can transport whole peptide-major histocompatibility complex (MHC) complexes from one cell to another, a process that has been termed ‘cross dressing’ (137, 138). MPs originating from apoptotic cells were shown to induce an alternative maturation of dendritic cells, characterized by down regulation of MHC II, which is in line with the immunologically silent nature of apoptosis (139). This mechanism however was found to be disturbed in patients with systemic lupus erythematosus (SLE), where apoptotic MPs were found to mature dendritic cells without MHC II down regulation (139). This has been explained by the finding that MPs originating from apoptotic cells contain a myriad of modified autoantigens, especially modified chromatin, that can trigger the activation of antigen presenting cells like dendritic cells (37, 93, 111, 140, 141). This difference in dendritic cell maturation might explain part of the autoimmune reaction observed in patients with SLE.
Antigen presenting cells themselves can also release MPs, which may activate T- and B-cells. MPs containing MHC I or MHC II complexes loaded with antigens, together with co-stimulatory molecules can augment the proliferation of specific T-cells as has been shown for dendritic cell derived exosomes (127, 142, 143).
It has become clear that MPs have potent pro-inflammatory effects, however they also can stimulate coagulation and can affect vascular function (144, 145). Owing to this, MPs have been assigned deleterious roles in a plethora of pathologies, predominantly cardiovascular and autoimmune diseases (146). In this and the following section, a brief overview on the role of MPs in various clinical settings is provided.
Exposure of negatively charged PS on apoptotic MPs facilitates the binding of coagulation factors to these MPs and the assembly of coagulation enzyme complexes (147). Coagulation factors become readily activated on MPs as they co-express high levels of membrane-anchored tissue factor (148). Indeed, MPs isolated from cell lines or human plasma readily support thrombin production in vitro (65, 149, 150). Large von Willebrand factor multimers have been detected on endothelial cell-derived MPs, suggesting that MPs can also facilitate the aggregation of platelets during coagulation (39, 50). Besides direct pro-coagulant properties of MPs, they may also indirectly facilitate coagulation. For instance, leukocyte-derived MPs have been shown to contain tissue factor and P-selectin glycoprotein ligand-1 (PSGL-1). Those MPs accumulate at thrombi rich in platelets via the interaction of platelet P-selectin and PSGL-1, bringing tissue factor to the growing thrombus (151).
Experiments with MPs isolated from the blood of women with pre-eclampsia or patients with myocardial infarction showed that MPs can alter vascular function. Notably, these MPs were endowed with the capacity to diminish endothelium-dependent relaxation of arteries ex vivo (152, 153). MPs isolated from healthy donors have been shown to harbor endothelial nitric oxide synthase (eNOS) required for vascular homeostasis, and eNOS-containing MPs appear to be specifically decreased in patients with endothelial dysfunction (154). MPs can also scavenge nitric oxide and thereby cause endothelial dysfunction (155, 156). Furthermore, MP-induced increase in superoxide production in endothelial cells can decrease endothelium-dependent relaxation (157).
Under normal conditions, MPs derived from platelets or megakaryocytes are the most common subpopulation, comprising around 70% of all MPs in the circulation (158). MPs are released in healthy individuals under basal conditions. Various clinical conditions have been associated with alterations in MP counts or changes in specific MP subpopulations. Nevertheless, it is still uncertain whether changes in quantity or characteristics of MPs in autoimmune diseases are the cause or consequence of disease. Although an increased presence of MPs could be explained by disease related pathogenic events, the direct effects of MPs on coagulation, inflammation and endothelial dysfunction strongly suggest that MPs can also be involved in the etiology of the disease. Supporting such a causal role, Sisirak and colleagues showed that a mouse model deficient in DNase1L3 displayed an increased presence of chromatin in MPs, thereby leading to the onset of autoimmunity (10). Owing to their procoagulant and proinflammatory properties, it is not surprising that MP alterations are predominantly connected to cardiovascular and autoimmune diseases. MP alterations in the context of cardiovascular disease is beyond the scope of this review and has been extensively reviewed elsewhere (6, 7, 159, 160).
Antiphospholipid syndrome (APS) is an autoimmune disease that clinically manifests as recurrent thrombotic events and pregnancy-related issues such as stillbirths and (pre)-eclampsia. Laboratory abnormalities include increased levels of autoantibodies against membrane anionic phospholipids, such as anti-cardiolipin and anti-PS autoantibodies. APS can occur in association with other rheumatic disorders, such as SLE, but can also be a clinical entity on its own. The pathogenesis of APS remains poorly understood, but MPs could explain the procoagulant and proinflammatory phenotype of APS (161). Indeed, high levels of endothelial cell-derived tissue factor-expressing MPs have been identified in APS patients (162). Simultaneous analysis of primary APS patients and SLE patients with and without APS showed that especially primary APS patients as well as SLE patients with APS displayed elevated levels of endothelial cell-derived MPs, in contrast to SLE patients without APS (163). Ex vivo experiments with plasma from patients with various autoimmune diseases demonstrated that in particular APS plasma induced endothelial-derived MPs with procoagulant properties (163). Separate analyses of APS patients with only obstetric or only thrombotic complications revealed that endothelial cell-derived MPs are increased in thrombotic but not in obstetric APS patients (48). In addition to endothelial cell-derived MPs, elevated levels of platelet-derived MPs have also been reported (48, 164). Collectively, these data suggest a link between anti-phospholipid antibodies, endothelial cell-derived MPs and the development of thrombosis in APS.
Systemic sclerosis (SSc) is characterized by degenerative changes, diffuse fibrosis and vascular abnormalities in various organs. Raynaud phenomenon, dysphagia, skin tightening and polyarthralgia are amongst its symptoms. Studies report different MP numbers in patients with SSc. Some studies show elevated numbers of MPs (70, 158, 165, 166), whereas other studies report lower MP counts in patients with SSc (40, 60). Nevertheless, MPs have been associated with certain clinical manifestations, such as interstitial pneumonia or perivascular inflammation (40, 165), whereas MP numbers were inversely correlated with skin thickness scores (158). Maugeri et al. showed that platelet derived MPs contain the damage associated molecular pattern high mobility group box 1 (HMGB1), which is able to induce the formation of NETs by neutrophils (70). One study assigned MPs as active players in the pathogenesis of SSc. The inhibition of MP release by endothelial cells using pantethine or by inactivating ATP-binding cassette transporter A1 (ABCA1), thereby inhibiting transport of PS to the outer leaflet, ameliorated skin and lung fibrosis in murine SSc (167). Notably, pantethine had earlier been shown to protect against cerebral malaria by impairing MP release from activated endothelial cells (168). Altogether, these data suggest that MPs play a role in the disease development of SSc.
Primary Sjögren’s syndrome (pSS) is a chronic autoimmune disease in which fluid-secreting glands become inflamed. This primarily results in the development of a dry mouth and dry eyes. Other symptoms can include fatigue, muscle and joint pain and a chronic cough. Genetic, hormonal and environmental factors culminate in the infiltration of lymphocytes into the salivary and lacrimal glands, thereby causing their dysfunction. Increased numbers of endothelial cell-derived MPs have been reported for pSS patients and are considered to reflect the degree of systemic endothelial damage (41). Levels of endothelial cell-derived MPs directly correlate with disease duration (41). In a comparative analysis of patients with pSS, SLE and RA, it was found that all patient cohorts had increased levels of platelet-derived MPs, but that increased levels of leukocyte-derived MPs were exclusive for pSS (169). Exosomes released from salivary gland epithelial cells contain the typical autoantigens that are targeted in pSS, i.e. Ro/SSA, La/SSB and Sm ribonucleoproteins (RNPs), thereby suggesting that the release of such exosomes directly contributes to the autoimmune response in pSS (170). In summary, there is a wide spectrum of elevated MP populations in pSS, that influence key events in its pathogenesis, such as platelet activation, systemic endothelial damage and injury to salivary gland epithelial cells.
Rheumatoid arthritis (RA) is an inflammatory autoimmune disease causing chronic joint inflammation and systemic manifestations, including accelerated forms of cardiovascular disease. Multiple factors, such as genetic background, epigenetic changes and environmental triggers culminate in the production of autoantibodies, predominantly against citrullinated proteins that cause joint inflammation and subsequent bone destruction. MPs are perceived as important mediators of RA disease, both locally in the joints as well as systemically to promote vascular alterations. In particular, elevated levels of platelet-derived MPs have been identified in RA and MP levels correlate with disease activity (71, 171, 172). Platelet-derived MPs are also discovered in synovial fluid of RA patients, in addition to their presence in the circulation (64). Another study claims that MPs derived from granulocytes and monocytes are predominantly found in synovial fluid of RA patients, whereas there was no increase of MPs derived from platelets (173). This discrepancy is most likely explained by the use of different markers to define the origin of MPs. It appears that particularly platelet-derived MPs are active players during inflammatory arthritis, as the depletion of platelets drastically attenuates the severity of arthritis in mouse models (64). Furthermore, MPs isolated from RA joints are capable of activating synovial fibroblasts in vitro (174). Notably, MP-induced fibroblast activation causes the secretion of matrix metalloproteinases and the production of pro-inflammatory cytokines (9). Additionally, MPs in RA patients containing autoantigens can form immune complexes leading to inflammation in the circulation and joints (175). Matrix metalloproteinases degrade extracellular matrices and consequently cause cartilage and bone destruction. Altogether, it appears that there is an important role for MPs, in fueling the local and systemic inflammatory response in RA patients.
Endothelial injury is central in the pathogenesis of vasculitis and is associated with elevated levels of endothelial cell-derived MPs and platelet-derived MPs (176, 177). Endothelial cell-derived MPs are particularly elevated in patients with active disease compared to those in full or partial remission (178). Importantly, levels of endothelial cell-derived MPs correlate with disease activity scores and acute-phase markers (179). In children with vasculitis, platelet-derived MPs are also elevated, but to a lesser extent than those derived from endothelial cells (179). Neutrophil-derived MPs are also reported in vasculitis patients with anti-neutrophil cytoplasmic antibodies (ANCA). Polyclonal ANCA as well as chimeric proteinase 3 (PR3)-ANCA can stimulate neutrophils to release MPs, which in turn express PR3 and myeloperoxidase and bind to endothelial cells in a CD18-dependent manner (51). The latter results in the expression of endothelial adhesion molecules and the secretion of pro-inflammatory cytokines. Thus, neutrophil-derived MPs may connect ANCA with vascular damage in patients with ANCA-associated vasculitis.
SLE is a devastating disease in which autoantibodies develop against nuclear components, such as anti-DNA, anti-histone and anti-RNP autoantibodies. The binding of anti-nuclear autoantibodies to their antigens results in the formation of immune complexes, which deposit in various organs, thereby causing inflammatory tissue destruction due to complement activation and leukocyte recruitment. MPs are perceived as important reservoirs of autoantigens in SLE, as outlined above (37, 109, 180, 181). Central to the pathogenesis of SLE is the failure to adequately clear apoptotic cells and apoptotic MPs (182–184). There are conflicting reports about MP counts in SLE patients compared to healthy controls, ranging from increased numbers of MPs, or no differences to even reduced numbers of MPs (37, 52, 55, 59, 61, 62, 71, 93, 110, 113, 114, 169). A fundamental difference between endothelial cell-derived MPs in SLE versus MPs in RA and SSc is that chromatin is solely present in MPs from SLE patients (37). Chromatin within SLE MPs contains specific modifications to N-terminal histone tails that are associated with apoptosis, as outlined above (140, 185, 186). In contrast, endothelial cell-derived MPs in RA and SSc patients are more likely to reflect endothelial cell activation. As outlined, proteomic analyses of MPs confirmed that apoptotic proteins are specifically present in SLE MPs but not in those from patients with other rheumatic diseases (78, 187). Although MP levels do not seem to directly correlate with disease activity scores, MPs rich in apoptosis-modified chromatin appear to be specifically present in SLE patients with lupus nephritis (93). In summary, SLE MPs are unique compared to MPs in other autoimmune diseases in that they contain high levels of apoptosis-modified chromatin, thereby providing these MPs with potent proinflammatory properties that may enhance the inflammatory response in SLE.
MPs are important players in normal homeostasis as well as in pathological conditions. MPs can serve as mediators of long-distance communication between cells and deliver different kind of cargoes or signals throughout the body. The study of MPs isolated from body fluids, especially plasma, remains challenging since there is no consensus on protocols of how to isolate and analyze number and origin of MPs. To improve comparability between different studies, the International Society for Extracellular Vesicles published consensus papers with guidelines addressing many important issues concerning MP research (26, 36). For the time being differential centrifugation and flow cytometric analyses are the techniques of choice for most researchers in the field. However, new and exciting developments, especially the isolation of MPs by microfluidics and characterization of MPs with mass spectrometry, may change the way of how to isolate and analyze MPs in the future.
MPs released from activated or dying cells can have a pathogenic role in numerous autoimmune diseases by fueling inflammatory processes, coagulation and endothelial dysfunction. Nevertheless, the scientific evidence connecting MPs to autoimmune diseases is predominantly associative in nature and fails to unambiguously answer the question whether MPs are the cause or consequence of disease. To solve this issue, a more thorough understanding of why and how MPs are produced in different disease settings is needed. Additionally, strategies to control the release or the clearance of MPs are needed to investigate the effects of increasing or decreasing MP concentration in health and disease. Alternatively, exogenously administered vesicles could be used to proof that vesicles are causing autoimmunity. Exogenous administration of MPs comes with its own challenges as the source of MPs, as well as the route and dose of administration impacts the final biodistribution (188). Nevertheless, infusion of extracellular vesicles have pathologic effects in different mouse models of preeclampsia or multiple sclerosis (189, 190). In conclusion, findings obtained from research focusing on casual relations rather than associations will facilitate the development of disease-specific therapeutic strategies that can regulate MP release or, alternatively, ensure their immunologically silent clearance; opening new avenues for the treatment of autoimmune diseases in which MPs play a causative role.
NR, CY, and EP wrote the manuscript. NR and CY prepared the figures. LH and JvdV contributed to writing and critically revised the manuscript. All authors contributed to the article and approved the submitted version.
This research was supported by the Radboud Institute for Molecular Life Science (RIMLS) PhD program.
The authors declare that the research was conducted in the absence of any commercial or financial relationships that could be construed as a potential conflict of interest.
All claims expressed in this article are solely those of the authors and do not necessarily represent those of their affiliated organizations, or those of the publisher, the editors and the reviewers. Any product that may be evaluated in this article, or claim that may be made by its manufacturer, is not guaranteed or endorsed by the publisher.
1. Zhao Y, Wei W, Liu ML. Extracellular Vesicles and Lupus Nephritis - New Insights Into Pathophysiology and Clinical Implications. J Autoimmun (2020) 115:102540. doi: 10.1016/j.jaut.2020.102540
2. Stahl AL, Johansson K, Mossberg M, Kahn R, Karpman D. Exosomes and Microvesicles in Normal Physiology, Pathophysiology, and Renal Diseases. Pediatr Nephrol (2019) 34(1):11–30. doi: 10.1007/s00467-017-3816-z
3. Taylor J, Azimi I, Monteith G, Bebawy M. Ca(2+) Mediates Extracellular Vesicle Biogenesis Through Alternate Pathways in Malignancy. J Extracell Vesicles (2020) 9(1):1734326. doi: 10.1080/20013078.2020.1734326
4. Torralba D, Baixauli F, Sánchez-Madrid F. Mitochondria Know No Boundaries: Mechanisms and Functions of Intercellular Mitochondrial Transfer. Front Cell Dev Biol (2016) 4. doi: 10.3389/fcell.2016.00107
5. Merchant ML, Rood IM, Deegens JKJ, Klein JB. Isolation and Characterization of Urinary Extracellular Vesicles: Implications for Biomarker Discovery. Nat Rev Nephrol (2017) 13(12):731–49. doi: 10.1038/nrneph.2017.148
6. Fu S, Zhang Y, Li Y, Luo L, Zhao Y, Yao Y. Extracellular Vesicles in Cardiovascular Diseases. Cell Death Discov (2020) 6:68. doi: 10.1038/s41420-020-00305-y
7. Alexandru N, Costa A, Constantin A, Cochior D, Georgescu A. Microparticles: From Biogenesis to Biomarkers and Diagnostic Tools in Cardiovascular Disease. Curr Stem Cell Res Ther (2017) 12(2):89–102. doi: 10.2174/1574888X11666151203224058
8. Xu R, Rai A, Chen M, Suwakulsiri W, Greening DW, Simpson RJ. Extracellular Vesicles in Cancer - Implications for Future Improvements in Cancer Care. Nat Rev Clin Oncol (2018) 15(10):617–38. doi: 10.1038/s41571-018-0036-9
9. Distler JH, Jungel A, Huber LC, Seemayer CA, Reich CF 3rd, Gay RE, et al. The Induction of Matrix Metalloproteinase and Cytokine Expression in Synovial Fibroblasts Stimulated With Immune Cell Microparticles. Proc Natl Acad Sci U S A (2005) 102(8):2892–7. doi: 10.1073/pnas.0409781102
10. Sisirak V, Sally B, D'Agati V, Martinez-Ortiz W, Ozcakar ZB, David J, et al. Digestion of Chromatin in Apoptotic Cell Microparticles Prevents Autoimmunity. Cell (2016) 166(1):88–101. doi: 10.1016/j.cell.2016.05.034
11. Dey-Hazra E, Hertel B, Kirsch T, Woywodt A, Lovric S, Haller H, et al. Detection of Circulating Microparticles by Flow Cytometry: Influence of Centrifugation, Filtration of Buffer, and Freezing. Vasc Health Risk Manage (2010) 6:1125–33. doi: 10.2147/VHRM.S13236
12. Coumans FAW, Brisson AR, Buzas EI, Dignat-George F, Drees EEE, El-Andaloussi S, et al. Methodological Guidelines to Study Extracellular Vesicles. Circ Res (2017) 120(10):1632–48. doi: 10.1161/CIRCRESAHA.117.309417
13. Lacroix R, Judicone C, Poncelet P, Robert S, Arnaud L, Sampol J, et al. Impact of Pre-Analytical Parameters on the Measurement of Circulating Microparticles: Towards Standardization of Protocol. J Thromb Haemost (2012) 10(3):437–46. doi: 10.1111/j.1538-7836.2011.04610.x
14. Jy W, Horstman LL, Jimenez JJ, Ahn YS, Biro E, Nieuwland R, et al. Measuring Circulating Cell-Derived Microparticles. J Thromb Haemost (2004) 2(10):1842–51. doi: 10.1111/j.1538-7836.2004.00936.x
15. Baek R, Sondergaard EK, Varming K, Jorgensen MM. The Impact of Various Preanalytical Treatments on the Phenotype of Small Extracellular Vesicles in Blood Analyzed by Protein Microarray. J Immunol Methods (2016) 438:11–20. doi: 10.1016/j.jim.2016.08.007
16. Shah MD, Bergeron AL, Dong JF, Lopez JA. Flow Cytometric Measurement of Microparticles: Pitfalls and Protocol Modifications. Platelets (2008) 19(5):365–72. doi: 10.1080/09537100802054107
17. Sodar BW, Kittel A, Paloczi K, Vukman KV, Osteikoetxea X, Szabo-Taylor K, et al. Low-Density Lipoprotein Mimics Blood Plasma-Derived Exosomes and Microvesicles During Isolation and Detection. Sci Rep (2016) 6:24316. doi: 10.1038/srep24316
18. Yuana Y, Levels J, Grootemaat A, Sturk A, Nieuwland R. Co-Isolation of Extracellular Vesicles and High-Density Lipoproteins Using Density Gradient Ultracentrifugation. J Extracell Vesicles (2014) 3(1):23262. doi: 10.3402/jev.v3.23262
19. He L, Zhu D, Wang J, Wu X. A Highly Efficient Method for Isolating Urinary Exosomes. Int J Mol Med (2019) 43(1):83–90. doi: 10.3892/ijmm.2018.3944
20. Navarro-Hernandez IC, Acevedo-Ochoa E, Juárez-Vega G, Meza-Sánchez DE, Hernández-Hernández JM, Maravillas-Montero JL. Size Determination and Phenotypic Analysis of Urinary Extracellular Vesicles Using Flow Cytometry. JoVE (2021) (170):e61695. doi: 10.3791/61695
21. Sun IO, Lerman LO. Urinary Extracellular Vesicles as Biomarkers of Kidney Disease: From Diagnostics to Therapeutics. Diagn (Basel) (2020) 10(5):311. doi: 10.3390/diagnostics10050311
22. Farzamikia N, Baradaran B, Mostafavi S, Ahmadian E, Hosseiniyan Khatibi SM, Zununi Vahed S, et al. Podocyte-Derived Microparticles in IgA Nephropathy. Biomed Pharmacother (2021) 141:111891. doi: 10.1016/j.biopha.2021.111891
23. Gyorgy B, Modos K, Pallinger E, Paloczi K, Pasztoi M, Misjak P, et al. Detection and Isolation of Cell-Derived Microparticles are Compromised by Protein Complexes Resulting From Shared Biophysical Parameters. Blood (2011) 117(4):e39–48. doi: 10.1182/blood-2010-09-307595
24. Linares R, Tan S, Gounou C, Arraud N, Brisson AR. High-Speed Centrifugation Induces Aggregation of Extracellular Vesicles. J Extracell Vesicles (2015) 4:29509. doi: 10.3402/jev.v4.29509
25. Karimi N, Cvjetkovic A, Jang SC, Crescitelli R, Hosseinpour Feizi MA, Nieuwland R, et al. Detailed Analysis of the Plasma Extracellular Vesicle Proteome After Separation From Lipoproteins. Cell Mol Life Sci (2018) 75(15):2873–86. doi: 10.1007/s00018-018-2773-4
26. Thery C, Witwer KW, Aikawa E, Alcaraz MJ, Anderson JD, Andriantsitohaina R, et al. Minimal Information for Studies of Extracellular Vesicles 2018 (MISEV2018): A Position Statement of the International Society for Extracellular Vesicles and Update of the MISEV2014 Guidelines. J Extracell Vesicles (2018) 7(1):1535750. doi: 10.1080/20013078.2018.1535750
27. Davies RT, Kim J, Jang SC, Choi EJ, Gho YS, Park J. Microfluidic Filtration System to Isolate Extracellular Vesicles From Blood. Lab Chip (2012) 12(24):5202–10. doi: 10.1039/c2lc41006k
28. Kuhn P, Eyer K, Robinson T, Schmidt FI, Mercer J, Dittrich PS. A Facile Protocol for the Immobilisation of Vesicles, Virus Particles, Bacteria, and Yeast Cells. Integr Biol (Camb) (2012) 4(12):1550–5. doi: 10.1039/c2ib20181j
29. Chen C, Skog J, Hsu CH, Lessard RT, Balaj L, Wurdinger T, et al. Microfluidic Isolation and Transcriptome Analysis of Serum Microvesicles. Lab Chip (2010) 10(4):505–11. doi: 10.1039/B916199F
30. Ashcroft BA, de Sonneville J, Yuana Y, Osanto S, Bertina R, Kuil ME, et al. Determination of the Size Distribution of Blood Microparticles Directly in Plasma Using Atomic Force Microscopy and Microfluidics. BioMed Microdevices (2012) 14(4):641–9. doi: 10.1007/s10544-012-9642-y
31. Shao H, Chung J, Balaj L, Charest A, Bigner DD, Carter BS, et al. Protein Typing of Circulating Microvesicles Allows Real-Time Monitoring of Glioblastoma Therapy. Nat Med (2012) 18(12):1835–40. doi: 10.1038/nm.2994
32. Liangsupree T, Multia E, Riekkola M-L. Modern Isolation and Separation Techniques for Extracellular Vesicles. J Chromatogr A (2021) 1636:461773. doi: 10.1016/j.chroma.2020.461773
33. Evander M, Gidlof O, Olde B, Erlinge D, Laurell T. Non-Contact Acoustic Capture of Microparticles From Small Plasma Volumes. Lab Chip (2015) 15(12):2588–96. doi: 10.1039/C5LC00290G
34. Brahmer A, Neuberger E, Esch-Heisser L, Haller N, Jorgensen MM, Baek R, et al. Platelets, Endothelial Cells and Leukocytes Contribute to the Exercise-Triggered Release of Extracellular Vesicles Into the Circulation. J Extracell Vesicles (2019) 8(1):1615820. doi: 10.1080/20013078.2019.1615820
35. Yuana Y, Boing AN, Grootemaat AE, van der Pol E, Hau CM, Cizmar P, et al. Handling and Storage of Human Body Fluids for Analysis of Extracellular Vesicles. J Extracell Vesicles (2015) 4:29260. doi: 10.3402/jev.v4.29260
36. Lotvall J, Hill AF, Hochberg F, Buzas EI, Di Vizio D, Gardiner C, et al. Minimal Experimental Requirements for Definition of Extracellular Vesicles and Their Functions: A Position Statement From the International Society for Extracellular Vesicles. J Extracell Vesicles (2014) 3:26913. doi: 10.3402/jev.v3.26913
37. Dieker J, Tel J, Pieterse E, Thielen A, Rother N, Bakker M, et al. Circulating Apoptotic Microparticles in Systemic Lupus Erythematosus Patients Drive the Activation of Dendritic Cell Subsets and Prime Neutrophils for NETosis. Arthritis Rheumatol (2016) 68(2):462–72. doi: 10.1002/art.39417
38. Jimenez JJ, Jy W, Mauro LM, Soderland C, Horstman LL, Ahn YS. Endothelial Cells Release Phenotypically and Quantitatively Distinct Microparticles in Activation and Apoptosis. Thromb Res (2003) 109(4):175–80. doi: 10.1016/S0049-3848(03)00064-1
39. Jimenez JJ, Jy W, Mauro LM, Horstman LL, Soderland C, Ahn YS. Endothelial Microparticles Released in Thrombotic Thrombocytopenic Purpura Express Von Willebrand Factor and Markers of Endothelial Activation. Br J Haematol (2003) 123(5):896–902. doi: 10.1046/j.1365-2141.2003.04716.x
40. Jung C, Drummer K, Oelzner P, Figulla HR, Boettcher J, Franz M, et al. The Association Between Endothelial Microparticles and Inflammation in Patients With Systemic Sclerosis and Raynaud's Phenomenon as Detected by Functional Imaging. Clin Hemorheol Microcirc (2015) 61(3):549–57. doi: 10.3233/CH-151956
41. Bartoloni E, Alunno A, Bistoni O, Caterbi S, Luccioli F, Santoboni G, et al. Characterization of Circulating Endothelial Microparticles and Endothelial Progenitor Cells in Primary Sjogren's Syndrome: New Markers of Chronic Endothelial Damage? Rheumatol (Oxf) (2015) 54(3):536–44. doi: 10.1093/rheumatology/keu320
42. Rousseau M, Belleannee C, Duchez A-C, Cloutier N, Levesque T, Jacques F, et al. Detection and Quantification of Microparticles From Different Cellular Lineages Using Flow Cytometry. Evaluation of the Impact of Secreted Phospholipase A2 on Microparticle Assessment. PloS One (2015) 10(1):e0116812.
43. Li C, Li S, Zhang F, Wu M, Liang H, Song J, et al. Endothelial Microparticles-Mediated Transfer of microRNA-19b Promotes Atherosclerosis via Activating Perivascular Adipose Tissue Inflammation in Apoe(-/-) Mice. Biochem Biophys Res Commun (2018) 495(2):1922–9. doi: 10.1016/j.bbrc.2017.11.195
44. Pasquier J, Thomas B, Hoarau-Vechot J, Odeh T, Robay A, Chidiac O, et al. Circulating Microparticles in Acute Diabetic Charcot Foot Exhibit a High Content of Inflammatory Cytokines, and Support Monocyte-to-Osteoclast Cell Induction. Sci Rep (2017) 7(1):16450. doi: 10.1038/s41598-017-16365-7
45. Bacha NC, Blandinieres A, Rossi E, Gendron N, Nevo N, Lecourt S, et al. Endothelial Microparticles Are Associated to Pathogenesis of Idiopathic Pulmonary Fibrosis. Stem Cell Rev (2018) 14(2):223–35. doi: 10.1007/s12015-017-9778-5
46. McCarthy EM, Moreno-Martinez D, Wilkinson FL, McHugh NJ, Bruce IN, Pauling JD, et al. Microparticle Subpopulations are Potential Markers of Disease Progression and Vascular Dysfunction Across a Spectrum of Connective Tissue Disease. BBA Clin (2017) 7:16–22. doi: 10.1016/j.bbacli.2016.11.003
47. Michalska-Jakubus M, Kowal-Bielecka O, Smith V, Cutolo M, Krasowska D. Plasma Endothelial Microparticles Reflect the Extent of Capillaroscopic Alterations and Correlate With the Severity of Skin Involvement in Systemic Sclerosis. Microvascular Res (2017) 110:24–31. doi: 10.1016/j.mvr.2016.11.006
48. Breen KA, Sanchez K, Kirkman N, Seed PT, Parmar K, Moore GW, et al. Endothelial and Platelet Microparticles in Patients With Antiphospholipid Antibodies. Thromb Res (2015) 135(2):368–74. doi: 10.1016/j.thromres.2014.11.027
49. Cicarini WB, Ferreira KS, Loures CMG, Consoli RV, Neiva CLS, Padua PM, et al. Systemic Lupus Erythematosus: Disease Activity may Influence the Release of Endothelial Microparticles? Blood Coagul Fibrinol (2018) 29(2):189–95. doi: 10.1097/MBC.0000000000000703
50. Jy W, Jimenez JJ, Mauro LM, Horstman LL, Cheng P, Ahn ER, et al. Endothelial Microparticles Induce Formation of Platelet Aggregates via a Von Willebrand Factor/Ristocetin Dependent Pathway, Rendering Them Resistant to Dissociation. J Thromb Haemost (2005) 3(6):1301–8. doi: 10.1111/j.1538-7836.2005.01384.x
51. Hong Y, Eleftheriou D, Hussain AA, Price-Kuehne FE, Savage CO, Jayne D, et al. Anti-Neutrophil Cytoplasmic Antibodies Stimulate Release of Neutrophil Microparticles. J Am Soc Nephrol (2012) 23(1):49–62. doi: 10.1681/ASN.2011030298
52. Burbano C, Villar-Vesga J, Orejuela J, Munoz C, Vanegas A, Vasquez G, et al. Potential Involvement of Platelet-Derived Microparticles and Microparticles Forming Immune Complexes During Monocyte Activation in Patients With Systemic Lupus Erythematosus. Front Immunol (2018) 9:322. doi: 10.3389/fimmu.2018.00322
53. Zinger A, Latham SL, Combes V, Byrne S, Barnett MH, Hawke S, et al. Plasma Levels of Endothelial and B-Cell-Derived Microparticles Are Restored by Fingolimod Treatment in Multiple Sclerosis Patients. Multi Sclerosis (Houndmills Basingstoke Engl) (2016) 22(14):1883–7. doi: 10.1177/1352458516636959
54. Wu YJ, Hua CC, Chen JY, Chang YW, Tseng JC. The Role of Endothelial Microparticles in Autoimmune Disease Patients With Raynaud's Phenomenon. J Microbiol Immunol Infect = Wei mian yu gan ran za zhi (2017) 50(6):857–62. doi: 10.1016/j.jmii.2015.12.010
55. Mobarrez F, Vikerfors A, Gustafsson JT, Gunnarsson I, Zickert A, Larsson A, et al. Microparticles in the Blood of Patients With Systemic Lupus Erythematosus (SLE): Phenotypic Characterization and Clinical Associations. Sci Rep (2016) 6:36025. doi: 10.1038/srep36025
56. Nakaoka H, Hirono K, Yamamoto S, Takasaki I, Takahashi K, Kinoshita K, et al. MicroRNA-145-5p and microRNA-320a Encapsulated in Endothelial Microparticles Contribute to the Progression of Vasculitis in Acute Kawasaki Disease. Sci Rep (2018) 8(1):1016. doi: 10.1038/s41598-018-19310-4
57. Jia L, Fan J, Cui W, Liu S, Li N, Lau WB, et al. Endothelial Cell-Derived Microparticles From Patients With Obstructive Sleep Apnea Hypoxia Syndrome and Coronary Artery Disease Increase Aortic Endothelial Cell Dysfunction. Cell Physiol Biochem (2017) 43(6):2562–70. doi: 10.1159/000484508
58. Martinez GJ, Barraclough JY, Nakhla S, Kienzle V, Robertson S, Mallat Z, et al. Neutrophil-Derived Microparticles are Released Into the Coronary Circulation Following Percutaneous Coronary Intervention in Acute Coronary Syndrome Patients. Biosci Rep (2017) 37(1):BSR20160430. doi: 10.1042/BSR20160430
59. Nielsen CT, Ostergaard O, Johnsen C, Jacobsen S, Heegaard NH. Distinct Features of Circulating Microparticles and Their Relationship to Clinical Manifestations in Systemic Lupus Erythematosus. Arthritis Rheumatol (2011) 63(10):3067–77. doi: 10.1002/art.30499
60. Iversen LV, Ostergaard O, Ullman S, Nielsen CT, Halberg P, Karlsmark T, et al. Circulating Microparticles and Plasma Levels of Soluble E- and P-Selectins in Patients With Systemic Sclerosis. Scand J Rheumatol (2013) 42(6):473–82. doi: 10.3109/03009742.2013.796403
61. Pereira J, Alfaro G, Goycoolea M, Quiroga T, Ocqueteau M, Massardo L, et al. Circulating Platelet-Derived Microparticles in Systemic Lupus Erythematosus. Association With Increased Thrombin Generation and Procoagulant State. Thromb Haemost (2006) 95(1):94–9. doi: 10.1160/TH05-05-0310
62. Lopez P, Rodriguez-Carrio J, Martinez-Zapico A, Caminal-Montero L, Suarez A. Circulating Microparticle Subpopulations in Systemic Lupus Erythematosus are Affected by Disease Activity. Int J Cardiol (2017) 236:138–44. doi: 10.1016/j.ijcard.2017.02.107
63. Cloutier N, Tan S, Boudreau LH, Cramb C, Subbaiah R, Lahey L, et al. The Exposure of Autoantigens by Microparticles Underlies the Formation of Potent Inflammatory Components: The Microparticle-Associated Immune Complexes. EMBO Mol Med (2013) 5(2):235–49. doi: 10.1002/emmm.201201846
64. Boilard E, Nigrovic PA, Larabee K, Watts GF, Coblyn JS, Weinblatt ME, et al. Platelets Amplify Inflammation in Arthritis via Collagen-Dependent Microparticle Production. Science (2010) 327(5965):580–3. doi: 10.1126/science.1181928
65. Aleman MM, Gardiner C, Harrison P, Wolberg AS. Differential Contributions of Monocyte- and Platelet-Derived Microparticles Towards Thrombin Generation and Fibrin Formation and Stability. J Thromb Haemost (2011) 9(11):2251–61. doi: 10.1111/j.1538-7836.2011.04488.x
66. Sionis A, Suades R, Sans-Rosello J, Sanchez-Martinez M, Crespo J, Padro T, et al. Circulating Microparticles Are Associated With Clinical Severity of Persistent ST-Segment Elevation Myocardial Infarction Complicated With Cardiogenic Shock. Int J Cardiol (2018) 258:249–56. doi: 10.1016/j.ijcard.2017.10.044
67. Anene C, Graham AM, Boyne J, Roberts W. Platelet Microparticle Delivered microRNA-Let-7a Promotes the Angiogenic Switch. Biochim Biophys Acta (2018) 1864(8):2633–43. doi: 10.1016/j.bbadis.2018.04.013
68. Laffont B, Corduan A, Plé H, Duchez A-C, Cloutier N, Boilard E, et al. Activated Platelets can Deliver mRNA Regulatory Ago2•microRNA Complexes to Endothelial Cells via Microparticles. Blood (2013) 122(2):253. doi: 10.1182/blood-2013-03-492801
69. Gyorgy B, Szabo TG, Turiak L, Wright M, Herczeg P, Ledeczi Z, et al. Improved Flow Cytometric Assessment Reveals Distinct Microvesicle (Cell-Derived Microparticle) Signatures in Joint Diseases. PloS One (2012) 7(11):e49726. doi: 10.1371/journal.pone.0049726
70. Maugeri N, Capobianco A, Rovere-Querini P, Ramirez GA, Tombetti E, Valle PD, et al. Platelet Microparticles Sustain Autophagy-Associated Activation of Neutrophils in Systemic Sclerosis. Sci Transl Med (2018) 10(451).:eaao3089 doi: 10.1126/scitranslmed.aao3089
71. Knijff-Dutmer EA, Koerts J, Nieuwland R, Kalsbeek-Batenburg EM, van de Laar MA. Elevated Levels of Platelet Microparticles Are Associated With Disease Activity in Rheumatoid Arthritis. Arthritis Rheumatol (2002) 46(6):1498–503. doi: 10.1002/art.10312
72. He Z, Tang Y, Qin C. Increased Circulating Leukocyte-Derived Microparticles in Ischemic Cerebrovascular Disease. Thromb Res (2017) 154:19–25. doi: 10.1016/j.thromres.2017.03.025
73. Botha J, Pugsley HR, Handberg A. Conventional, High-Resolution and Imaging Flow Cytometry: Benchmarking Performance in Characterisation of Extracellular Vesicles. Biomedicine (2021) 9(2):124. doi: 10.3390/biomedicines9020124
74. Vestad B, Llorente A, Neurauter A, Phuyal S, Kierulf B, Kierulf P, et al. Size and Concentration Analyses of Extracellular Vesicles by Nanoparticle Tracking Analysis: A Variation Study. J Extracell Vesicles (2017) 6(1):1344087. doi: 10.1080/20013078.2017.1344087
75. Thane KE, Davis AM, Hoffman AM. Improved Methods for Fluorescent Labeling and Detection of Single Extracellular Vesicles Using Nanoparticle Tracking Analysis. Sci Rep (2019) 9(1):12295. doi: 10.1038/s41598-019-48181-6
76. Desgeorges A, Hollerweger J, Lassacher T, Rohde E, Helmbrecht C, Gimona M. Differential Fluorescence Nanoparticle Tracking Analysis for Enumeration of the Extracellular Vesicle Content in Mixed Particulate Solutions. Methods (2020) 177:67–73. doi: 10.1016/j.ymeth.2020.02.006
77. Kowal J, Arras G, Colombo M, Jouve M, Morath JP, Primdal-Bengtson B, et al. Proteomic Comparison Defines Novel Markers to Characterize Heterogeneous Populations of Extracellular Vesicle Subtypes. Proc Natl Acad Sci USA (2016) 113(8):E968–77. doi: 10.1073/pnas.1521230113
78. Ostergaard O, Nielsen CT, Tanassi JT, Iversen LV, Jacobsen S, Heegaard NHH. Distinct Proteome Pathology of Circulating Microparticles in Systemic Lupus Erythematosus. Clin Proteomics (2017) 14:23. doi: 10.1186/s12014-017-9159-8
79. Cloet T, Momenbeitollahi N, Li H. Recent Advances on Protein-Based Quantification of Extracellular Vesicles. Anal Biochem (2021) 622:114168. doi: 10.1016/j.ab.2021.114168
80. Charest A. Experimental and Biological Insights From Proteomic Analyses of Extracellular Vesicle Cargos in Normalcy and Disease. Adv Biosyst (2020) 4(12):e2000069. doi: 10.1002/adbi.202000069
81. Oksvold MP, Kullmann A, Forfang L, Kierulf B, Li M, Brech A, et al. Expression of B-Cell Surface Antigens in Subpopulations of Exosomes Released From B-Cell Lymphoma Cells. Clin Ther (2014) 36(6):847–62.e1. doi: 10.1016/j.clinthera.2014.05.010
82. Jankovičová J, Sečová P, Michalková K, Antalíková J. Tetraspanins, More Than Markers of Extracellular Vesicles in Reproduction. Int J Mol Sci (2020) 21(20):7568. doi: 10.3390/ijms21207568
83. Galluzzi L, Vitale I, Aaronson SA, Abrams JM, Adam D, Agostinis P, et al. Molecular Mechanisms of Cell Death: Recommendations of the Nomenclature Committee on Cell Death 2018. Cell Death Differ (2018) 25(3):486–541. doi: 10.1038/s41418-017-0012-4
84. Tait SW, Green DR. Mitochondria and Cell Death: Outer Membrane Permeabilization and Beyond. Nat Rev Mol Cell Biol (2010) 11(9):621–32. doi: 10.1038/nrm2952
85. Czabotar PE, Lessene G, Strasser A, Adams JM. Control of Apoptosis by the BCL-2 Protein Family: Implications for Physiology and Therapy. Nat Rev Mol Cell Biol (2014) 15(1):49–63. doi: 10.1038/nrm3722
86. Li P, Nijhawan D, Budihardjo I, Srinivasula SM, Ahmad M, Alnemri ES, et al. Cytochrome C and dATP-Dependent Formation of Apaf-1/Caspase-9 Complex Initiates an Apoptotic Protease Cascade. Cell (1997) 91(4):479–89. doi: 10.1016/S0092-8674(00)80434-1
87. Julien O, Wells JA. Caspases and Their Substrates. Cell Death Differ (2017) 24(8):1380–9. doi: 10.1038/cdd.2017.44
88. Strasser A, Jost PJ, Nagata S. The Many Roles of FAS Receptor Signaling in the Immune System. Immunity (2009) 30(2):180–92. doi: 10.1016/j.immuni.2009.01.001
89. Gibert B, Mehlen P. Dependence Receptors and Cancer: Addiction to Trophic Ligands. Cancer Res (2015) 75(24):5171–5. doi: 10.1158/0008-5472.CAN-14-3652
90. Goldschneider D, Mehlen P. Dependence Receptors: A New Paradigm in Cell Signaling and Cancer Therapy. Oncogene (2010) 29(13):1865–82. doi: 10.1038/onc.2010.13
91. Sebbagh M, Renvoize C, Hamelin J, Riche N, Bertoglio J, Breard J. Caspase-3-Mediated Cleavage of ROCK I Induces MLC Phosphorylation and Apoptotic Membrane Blebbing. Nat Cell Biol (2001) 3(4):346–52. doi: 10.1038/35070019
92. Mills JC, Stone NL, Erhardt J, Pittman RN. Apoptotic Membrane Blebbing is Regulated by Myosin Light Chain Phosphorylation. J Cell Biol (1998) 140(3):627–36. doi: 10.1083/jcb.140.3.627
93. Rother N, Pieterse E, Lubbers J, Hilbrands L, van der Vlag J. Acetylated Histones in Apoptotic Microparticles Drive the Formation of Neutrophil Extracellular Traps in Active Lupus Nephritis. Front Immunol (2017) 8:1136. doi: 10.3389/fimmu.2017.01136
94. Miyoshi H, Umeshita K, Sakon M, Imajoh-Ohmi S, Fujitani K, Gotoh M, et al. Calpain Activation in Plasma Membrane Bleb Formation During Tert-Butyl Hydroperoxide-Induced Rat Hepatocyte Injury. Gastroenterology (1996) 110(6):1897–904. doi: 10.1053/gast.1996.v110.pm8964416
95. D'Souza-Schorey C, Chavrier P. ARF Proteins: Roles in Membrane Traffic and Beyond. Nat Rev Mol Cell Biol (2006) 7(5):347–58. doi: 10.1038/nrm1910
96. Muralidharan-Chari V, Clancy J, Plou C, Romao M, Chavrier P, Raposo G, et al. ARF6-Regulated Shedding of Tumor Cell-Derived Plasma Membrane Microvesicles. Curr Biol (2009) 19(22):1875–85. doi: 10.1016/j.cub.2009.09.059
97. Sedgwick AE, Clancy JW, Olivia Balmert M, D'Souza-Schorey C. Extracellular Microvesicles and Invadopodia Mediate non-Overlapping Modes of Tumor Cell Invasion. Sci Rep (2015) 5:14748. doi: 10.1038/srep14748
98. Zirngibl M, Furnrohr BG, Janko C, Munoz LE, Voll RE, Gregory CD, et al. Loading of Nuclear Autoantigens Prototypically Recognized by Systemic Lupus Erythematosus Sera Into Late Apoptotic Vesicles Requires Intact Microtubules and Myosin Light Chain Kinase Activity. Clin Exp Immunol (2015) 179(1):39–49. doi: 10.1111/cei.12342
99. Clancy JW, Sedgwick A, Rosse C, Muralidharan-Chari V, Raposo G, Method M, et al. Regulated Delivery of Molecular Cargo to Invasive Tumour-Derived Microvesicles. Nat Commun (2015) 6:6919. doi: 10.1038/ncomms7919
100. Coleman ML, Sahai EA, Yeo M, Bosch M, Dewar A, Olson MF. Membrane Blebbing During Apoptosis Results From Caspase-Mediated Activation of ROCK I. Nat Cell Biol (2001) 3(4):339–45. doi: 10.1038/35070009
101. D'Souza-Schorey C, Schorey JS. Regulation and Mechanisms of Extracellular Vesicle Biogenesis and Secretion. Essays Biochem (2018) 62(2):125–33. doi: 10.1042/EBC20170078
102. Das K, Prasad R, Singh A, Bhattacharya A, Roy A, Mallik S, et al. Protease-Activated Receptor 2 Promotes Actomyosin Dependent Transforming Microvesicles Generation From Human Breast Cancer. Mol Carcinog (2018) 57(12):1707–22. doi: 10.1002/mc.22891
103. Nagata S, Sakuragi T, Segawa K. Flippase and Scramblase for Phosphatidylserine Exposure. Curr Opin Immunol (2020) 62:31–8. doi: 10.1016/j.coi.2019.11.009
104. Distler JH, Huber LC, Hueber AJ, Reich CF 3rd, Gay S, Distler O, et al. The Release of Microparticles by Apoptotic Cells and Their Effects on Macrophages. Apoptosis (2005) 10(4):731–41. doi: 10.1007/s10495-005-2941-5
105. Fu Z, Zhou E, Wang X, Tian M, Kong J, Li J, et al. Oxidized Low-Density Lipoprotein-Induced Microparticles Promote Endothelial Monocyte Adhesion via Intercellular Adhesion Molecule 1. Am J Physiol Cell Physiol (2017) 313(5):C567–C74. doi: 10.1152/ajpcell.00158.2016
106. Lee Y, El Andaloussi S, Wood MJ. Exosomes and Microvesicles: Extracellular Vesicles for Genetic Information Transfer and Gene Therapy. Hum Mol Genet (2012) 21(R1):R125–34. doi: 10.1093/hmg/dds317
107. Jansen F, Zietzer A, Stumpf T, Flender A, Schmitz T, Nickenig G, et al. Endothelial Microparticle-Promoted Inhibition of Vascular Remodeling is Abrogated Under Hyperglycaemic Conditions. J Mol Cell Cardiol (2017) 112:91–4. doi: 10.1016/j.yjmcc.2017.09.004
108. Jansen F, Stumpf T, Proebsting S, Franklin BS, Wenzel D, Pfeifer P, et al. Intercellular Transfer of miR-126-3p by Endothelial Microparticles Reduces Vascular Smooth Muscle Cell Proliferation and Limits Neointima Formation by Inhibiting LRP6. J Mol Cell Cardiol (2017) 104:43–52. doi: 10.1016/j.yjmcc.2016.12.005
109. Ullal AJ, Reich CF 3rd, Clowse M, Criscione-Schreiber LG, Tochacek M, Monestier M, et al. Microparticles as Antigenic Targets of Antibodies to DNA and Nucleosomes in Systemic Lupus Erythematosus. J Autoimmun (2011) 36(3-4):173–80. doi: 10.1016/j.jaut.2011.02.001
110. Nielsen CT, Ostergaard O, Stener L, Iversen LV, Truedsson L, Gullstrand B, et al. Increased IgG on Cell-Derived Plasma Microparticles in Systemic Lupus Erythematosus is Associated With Autoantibodies and Complement Activation. Arthritis Rheumatol (2012) 64(4):1227–36. doi: 10.1002/art.34381
111. Dieker J, Hilbrands L, Thielen A, Dijkman H, Berden JH, van der Vlag J. Enhanced Activation of Dendritic Cells by Autologous Apoptotic Microvesicles in MRL/lpr Mice. Arthritis Res Ther (2015) 17:103. doi: 10.1186/s13075-015-0617-2
112. Radic M, Marion T, Monestier M. Nucleosomes are Exposed at the Cell Surface in Apoptosis. J Immunol (2004) 172(11):6692–700. doi: 10.4049/jimmunol.172.11.6692
113. Winberg LK, Nielsen CH, Jacobsen S. Surface Complement C3 Fragments and Cellular Binding of Microparticles in Patients With SLE. Lupus Sci Med (2017) 4(1):e000193. doi: 10.1136/lupus-2016-000193
114. Nielsen CT, Ostergaard O, Rekvig OP, Sturfelt G, Jacobsen S, Heegaard NH. Galectin-3 Binding Protein Links Circulating Microparticles With Electron Dense Glomerular Deposits in Lupus Nephritis. Lupus (2015) 24(11):1150–60. doi: 10.1177/0961203315580146
115. Feng D, Zhao WL, Ye YY, Bai XC, Liu RQ, Chang LF, et al. Cellular Internalization of Exosomes Occurs Through Phagocytosis. Traffic (2010) 11(5):675–87. doi: 10.1111/j.1600-0854.2010.01041.x
116. Fabbri M, Paone A, Calore F, Galli R, Gaudio E, Santhanam R, et al. MicroRNAs Bind to Toll-Like Receptors to Induce Prometastatic Inflammatory Response. Proc Natl Acad Sci USA (2012) 109(31):E2110–6. doi: 10.1073/pnas.1209414109
117. Morelli AE, Larregina AT, Shufesky WJ, Sullivan ML, Stolz DB, Papworth GD, et al. Endocytosis, Intracellular Sorting, and Processing of Exosomes by Dendritic Cells. Blood (2004) 104(10):3257–66. doi: 10.1182/blood-2004-03-0824
118. Christianson HC, Svensson KJ, van Kuppevelt TH, Li JP, Belting M. Cancer Cell Exosomes Depend on Cell-Surface Heparan Sulfate Proteoglycans for Their Internalization and Functional Activity. Proc Natl Acad Sci USA (2013) 110(43):17380–5. doi: 10.1073/pnas.1304266110
119. Svensson KJ, Christianson HC, Wittrup A, Bourseau-Guilmain E, Lindqvist E, Svensson LM, et al. Exosome Uptake Depends on ERK1/2-Heat Shock Protein 27 Signaling and Lipid Raft-Mediated Endocytosis Negatively Regulated by Caveolin-1. J Biol Chem (2013) 288(24):17713–24. doi: 10.1074/jbc.M112.445403
120. Rana S, Zöller M. Exosome Target Cell Selection and the Importance of Exosomal Tetraspanins: A Hypothesis. Biochem Soc Trans (2011) 39(2):559–62. doi: 10.1042/BST0390559
121. Record M, Carayon K, Poirot M, Silvente-Poirot S. Exosomes as New Vesicular Lipid Transporters Involved in Cell-Cell Communication and Various Pathophysiologies. Biochim Biophys Acta (2014) 1841(1):108–20. doi: 10.1016/j.bbalip.2013.10.004
122. Escola JM, Kleijmeer MJ, Stoorvogel W, Griffith JM, Yoshie O, Geuze HJ. Selective Enrichment of Tetraspan Proteins on the Internal Vesicles of Multivesicular Endosomes and on Exosomes Secreted by Human B-Lymphocytes. J Biol Chem (1998) 273(32):20121–7. doi: 10.1074/jbc.273.32.20121
123. Heijnen HF, Schiel AE, Fijnheer R, Geuze HJ, Sixma JJ. Activated Platelets Release Two Types of Membrane Vesicles: Microvesicles by Surface Shedding and Exosomes Derived From Exocytosis of Multivesicular Bodies and Alpha-Granules. Blood (1999) 94(11):3791–9. doi: 10.1182/blood.V94.11.3791
124. Rubinstein E, Ziyyat A, Prenant M, Wrobel E, Wolf JP, Levy S, et al. Reduced Fertility of Female Mice Lacking CD81. Dev Biol (2006) 290(2):351–8. doi: 10.1016/j.ydbio.2005.11.031
125. Zhu GZ, Miller BJ, Boucheix C, Rubinstein E, Liu CC, Hynes RO, et al. Residues SFQ (173-175) in the Large Extracellular Loop of CD9 are Required for Gamete Fusion. Development (2002) 129(8):1995–2002. doi: 10.1242/dev.129.8.1995
126. Hao S, Bai O, Li F, Yuan J, Laferte S, Xiang J. Mature Dendritic Cells Pulsed With Exosomes Stimulate Efficient Cytotoxic T-Lymphocyte Responses and Antitumour Immunity. Immunol (2007) 120(1):90–102. doi: 10.1111/j.1365-2567.2006.02483.x
127. Nolte-'t Hoen EN, Buschow SI, Anderton SM, Stoorvogel W, Wauben MH. Activated T Cells Recruit Exosomes Secreted by Dendritic Cells via LFA-1. Blood (2009) 113(9):1977–81. doi: 10.1182/blood-2008-08-174094
128. Hwang I, Shen X, Sprent J. Direct Stimulation of Naive T Cells by Membrane Vesicles From Antigen-Presenting Cells: Distinct Roles for CD54 and B7 Molecules. Proc Natl Acad Sci USA (2003) 100(11):6670–5. doi: 10.1073/pnas.1131852100
129. Näslund TI, Paquin-Proulx D, Paredes PT, Vallhov H, Sandberg JK, Gabrielsson S. Exosomes From Breast Milk Inhibit HIV-1 Infection of Dendritic Cells and Subsequent Viral Transfer to CD4+ T Cells. Aids (2014) 28(2):171–80. doi: 10.1097/QAD.0000000000000159
130. Mulcahy LA, Pink RC, Carter DRF. Routes and Mechanisms of Extracellular Vesicle Uptake. J Extracell Vesicles (2014) 3(1):24641. doi: 10.3402/jev.v3.24641
131. Zhang Z, Ohto U, Shimizu T. Toward a Structural Understanding of Nucleic Acid-Sensing Toll-Like Receptors in the Innate Immune System. FEBS Lett (2017) 591(20):3167–81. doi: 10.1002/1873-3468.12749
132. Majer O, Liu B, Barton GM. Nucleic Acid-Sensing TLRs: Trafficking and Regulation. Curr Opin Immunol (2017) 44:26–33. doi: 10.1016/j.coi.2016.10.003
133. Fransen JH, Hilbrands LB, Ruben J, Stoffels M, Adema GJ, van der Vlag J, et al. Mouse Dendritic Cells Matured by Ingestion of Apoptotic Blebs Induce T Cells to Produce Interleukin-17. Arthritis rheumatism (2009) 60(8):2304–13. doi: 10.1002/art.24719
134. Schiller M, Parcina M, Heyder P, Foermer S, Ostrop J, Leo A, et al. Induction of Type I IFN is a Physiological Immune Reaction to Apoptotic Cell-Derived Membrane Microparticles. J Immunol (2012) 189(4):1747–56. doi: 10.4049/jimmunol.1100631
135. Crow MK, Olferiev M, Kirou KA. Type I Interferons in Autoimmune Disease. Annu Rev Pathol (2019) 14:369–93. doi: 10.1146/annurev-pathol-020117-043952
136. Pisetsky DS, Lipsky PE. Microparticles as Autoadjuvants in the Pathogenesis of SLE. Nat Rev Rheumatol (2010) 6(6):368–72. doi: 10.1038/nrrheum.2010.66
137. Dolan BP, Gibbs KD Jr, Ostrand-Rosenberg S. Dendritic Cells Cross-Dressed With Peptide MHC Class I Complexes Prime CD8+ T Cells. J Immunol (2006) 177(9):6018–24. doi: 10.4049/jimmunol.177.9.6018
138. Leone DA, Rees AJ, Kain R. Dendritic Cells and Routing Cargo Into Exosomes. Immunol Cell Biol (2018) 96(7):683–93. doi: 10.1111/imcb.12170
139. Fehr EM, Spoerl S, Heyder P, Herrmann M, Bekeredjian-Ding I, Blank N, et al. Apoptotic-Cell-Derived Membrane Vesicles Induce an Alternative Maturation of Human Dendritic Cells Which is Disturbed in SLE. J Autoimmun (2013) 40:86–95. doi: 10.1016/j.jaut.2012.08.003
140. Dieker JW, Fransen JH, van Bavel CC, Briand JP, Jacobs CW, Muller S, et al. Apoptosis-Induced Acetylation of Histones is Pathogenic in Systemic Lupus Erythematosus. Arthritis Rheumatol (2007) 56(6):1921–33. doi: 10.1002/art.22646
141. van der Vlag J, Berden JH. Lupus Nephritis: Role of Antinucleosome Autoantibodies. Semin Nephrol (2011) 31(4):376–89. doi: 10.1016/j.semnephrol.2011.06.009
142. Thery C, Duban L, Segura E, Veron P, Lantz O, Amigorena S. Indirect Activation of Naive CD4+ T Cells by Dendritic Cell-Derived Exosomes. Nat Immunol (2002) 3(12):1156–62. doi: 10.1038/ni854
143. Utsugi-Kobukai S, Fujimaki H, Hotta C, Nakazawa M, Minami M. MHC Class I-Mediated Exogenous Antigen Presentation by Exosomes Secreted From Immature and Mature Bone Marrow Derived Dendritic Cells. Immunol Lett (2003) 89(2-3):125–31. doi: 10.1016/S0165-2478(03)00128-7
144. Enjeti AK, Lincz LF, Seldon M. Microparticles in Health and Disease. Semin Thromb Hemost (2008) 34(7):683–91. doi: 10.1055/s-0028-1104547
145. Herring JM, McMichael MA, Smith SA. Microparticles in Health and Disease. J Vet Intern Med (2013) 27(5):1020–33. doi: 10.1111/jvim.12128
146. Porto I, De Maria GL, Di Vito L, Camaioni C, Gustapane M, Biasucci LM. Microparticles in Health and Disease: Small Mediators, Large Role? Curr Vasc Pharmacol (2011) 9(4):490–500. doi: 10.2174/157016111796197206
147. Owens AP 3rd, Mackman N. Microparticles in Hemostasis and Thrombosis. Circ Res (2011) 108(10):1284–97. doi: 10.1161/CIRCRESAHA.110.233056
148. Zwicker JI, Trenor CC 3rd, Furie BC, Furie B. Tissue Factor-Bearing Microparticles and Thrombus Formation. Arterioscler Thromb Vasc Biol (2011) 31(4):728–33. doi: 10.1161/ATVBAHA.109.200964
149. Bidot L, Jy W, Bidot C Jr, Jimenez JJ, Fontana V, Horstman LL, et al. Microparticle-Mediated Thrombin Generation Assay: Increased Activity in Patients With Recurrent Thrombosis. J Thromb Haemost (2008) 6(6):913–9. doi: 10.1111/j.1538-7836.2008.02963.x
150. Park MS, Xue A, Spears GM, Halling TM, Ferrara MJ, Kuntz MM, et al. Thrombin Generation and Procoagulant Microparticle Profiles After Acute Trauma: A Prospective Cohort Study. J Trauma Acute Care Surg (2015) 79(5):726–31. doi: 10.1097/TA.0000000000000839
151. Furie B, Furie BC. Role of Platelet P-Selectin and Microparticle PSGL-1 in Thrombus Formation. Trends Mol Med (2004) 10(4):171–8. doi: 10.1016/j.molmed.2004.02.008
152. Boulanger CM, Scoazec A, Ebrahimian T, Henry P, Mathieu E, Tedgui A, et al. Circulating Microparticles From Patients With Myocardial Infarction Cause Endothelial Dysfunction. Circulation (2001) 104(22):2649–52. doi: 10.1161/hc4701.100516
153. Vanwijk MJ, Svedas E, Boer K, Nieuwland R, Vanbavel E, Kublickiene KR. Isolated Microparticles, But Not Whole Plasma, From Women With Preeclampsia Impair Endothelium-Dependent Relaxation in Isolated Myometrial Arteries From Healthy Pregnant Women. Am J Obstet Gynecol (2002) 187(6):1686–93. doi: 10.1067/mob.2002.127905
154. Horn P, Cortese-Krott MM, Amabile N, Hundsdorfer C, Kroncke KD, Kelm M, et al. Circulating Microparticles Carry a Functional Endothelial Nitric Oxide Synthase That is Decreased in Patients With Endothelial Dysfunction. J Am Heart Assoc (2012) 2(1):e003764. doi: 10.1161/JAHA.112.003764
155. Donadee C, Raat NJ, Kanias T, Tejero J, Lee JS, Kelley EE, et al. Nitric Oxide Scavenging by Red Blood Cell Microparticles and Cell-Free Hemoglobin as a Mechanism for the Red Cell Storage Lesion. Circulation (2011) 124(4):465–76. doi: 10.1161/CIRCULATIONAHA.110.008698
156. Liu C, Zhao W, Christ GJ, Gladwin MT, Kim-Shapiro DB. Nitric Oxide Scavenging by Red Cell Microparticles. Free Radic Biol Med (2013) 65:1164–73. doi: 10.1016/j.freeradbiomed.2013.09.002
157. Mezentsev A, Merks RM, O'Riordan E, Chen J, Mendelev N, Goligorsky MS, et al. Endothelial Microparticles Affect Angiogenesis In Vitro: Role of Oxidative Stress. Am J Physiol Heart Circ Physiol (2005) 289(3):H1106–14. doi: 10.1152/ajpheart.00265.2005
158. Guiducci S, Distler JH, Jungel A, Huscher D, Huber LC, Michel BA, et al. The Relationship Between Plasma Microparticles and Disease Manifestations in Patients With Systemic Sclerosis. Arthritis Rheumatol (2008) 58(9):2845–53. doi: 10.1002/art.23735
159. Thulin A, Christersson C, Alfredsson J, Siegbahn A. Circulating Cell-Derived Microparticles as Biomarkers in Cardiovascular Disease. biomark Med (2016) 10(9):1009–22. doi: 10.2217/bmm-2016-0035
160. Tushuizen ME, Diamant M, Sturk A, Nieuwland R. Cell-Derived Microparticles in the Pathogenesis of Cardiovascular Disease: Friend or Foe? Arterioscler Thromb Vasc Biol (2011) 31(1):4–9. doi: 10.1161/ATVBAHA.109.200998
161. Stok U, Cucnik S, Sodin-Semrl S, Zigon P. Extracellular Vesicles and Antiphospholipid Syndrome: State-Of-the-Art and Future Challenges. Int J Mol Sci (2021) 22(9):4689. doi: 10.3390/ijms22094689
162. Vikerfors A, Mobarrez F, Bremme K, Holmstrom M, Agren A, Eelde A, et al. Studies of Microparticles in Patients With the Antiphospholipid Syndrome (APS). Lupus (2012) 21(7):802–5. doi: 10.1177/0961203312437809
163. Dignat-George F, Camoin-Jau L, Sabatier F, Arnoux D, Anfosso F, Bardin N, et al. Endothelial Microparticles: A Potential Contribution to the Thrombotic Complications of the Antiphospholipid Syndrome. Thromb Haemost (2004) 91(4):667–73. doi: 10.1160/TH03-07-0487
164. Ford I, Urbaniak S, Greaves M. IgG From Patients With Antiphospholipid Syndrome Binds to Platelets Without Induction of Platelet Activation. Br J Haematol (1998) 102(3):841–9. doi: 10.1046/j.1365-2141.1998.00841.x
165. Nomura S, Inami N, Ozaki Y, Kagawa H, Fukuhara S. Significance of Microparticles in Progressive Systemic Sclerosis With Interstitial Pneumonia. Platelets (2008) 19(3):192–8. doi: 10.1080/09537100701882038
166. Leleu D, Levionnois E, Laurent P, Lazaro E, Richez C, Duffau P, et al. Elevated Circulatory Levels of Microparticles Are Associated to Lung Fibrosis and Vasculopathy During Systemic Sclerosis. Front Immunol (2020) 11:532177. doi: 10.3389/fimmu.2020.532177
167. Kavian N, Marut W, Servettaz A, Nicco C, Chereau C, Lemarechal H, et al. Pantethine Prevents Murine Systemic Sclerosis Through the Inhibition of Microparticle Shedding. Arthritis Rheumatol (2015) 67(7):1881–90. doi: 10.1002/art.39121
168. Penet MF, Abou-Hamdan M, Coltel N, Cornille E, Grau GE, de Reggi M, et al. Protection Against Cerebral Malaria by the Low-Molecular-Weight Thiol Pantethine. Proc Natl Acad Sci USA (2008) 105(4):1321–6. doi: 10.1073/pnas.0706867105
169. Sellam J, Proulle V, Jungel A, Ittah M, Miceli Richard C, Gottenberg JE, et al. Increased Levels of Circulating Microparticles in Primary Sjogren's Syndrome, Systemic Lupus Erythematosus and Rheumatoid Arthritis and Relation With Disease Activity. Arthritis Res Ther (2009) 11(5):R156. doi: 10.1186/ar2833
170. Kapsogeorgou EK, Abu-Helu RF, Moutsopoulos HM, Manoussakis MN. Salivary Gland Epithelial Cell Exosomes: A Source of Autoantigenic Ribonucleoproteins. Arthritis Rheumatol (2005) 52(5):1517–21. doi: 10.1002/art.21005
171. Rodriguez-Carrio J, Alperi-Lopez M, Lopez P, Alonso-Castro S, Carro-Esteban SR, Ballina-Garcia FJ, et al. Altered Profile of Circulating Microparticles in Rheumatoid Arthritis Patients. Clin Sci (Lond) (2015) 128(7):437–48. doi: 10.1042/CS20140675
172. Michael B, Misra D, Chengappa K, Negi V. Relevance of Elevated Microparticles in Peripheral Blood and Synovial Fluid of Patients With Rheumatoid Arthritis. Indian J Rheumatol (2018) 13(4):222–8. doi: 10.4103/injr.injr_101_18
173. Berckmans RJ, Nieuwland R, Tak PP, Boing AN, Romijn FP, Kraan MC, et al. Cell-Derived Microparticles in Synovial Fluid From Inflamed Arthritic Joints Support Coagulation Exclusively via a Factor VII-Dependent Mechanism. Arthritis Rheumatol (2002) 46(11):2857–66. doi: 10.1002/art.10587
174. Berckmans RJ, Nieuwland R, Kraan MC, Schaap MC, Pots D, Smeets TJ, et al. Synovial Microparticles From Arthritic Patients Modulate Chemokine and Cytokine Release by Synoviocytes. Arthritis Res Ther (2005) 7(3):R536–44. doi: 10.1186/ar1706
175. Burbano C, Rojas M, Munoz-Vahos C, Vanegas-Garcia A, Correa LA, Vasquez G, et al. Extracellular Vesicles are Associated With the Systemic Inflammation of Patients With Seropositive Rheumatoid Arthritis. Sci Rep (2018) 8(1):17917. doi: 10.1038/s41598-018-36335-x
176. Brogan PA, Dillon MJ. Endothelial Microparticles and the Diagnosis of the Vasculitides. Intern Med (2004) 43(12):1115–9. doi: 10.2169/internalmedicine.43.1115
177. Miao D, Ma T-T, Chen M, Zhao M-H. Platelets Release Proinflammatory Microparticles in Anti-Neutrophil Cytoplasmic Antibody-Associated Vasculitis. Rheumatology (2019) 58(8):1432–42. doi: 10.1093/rheumatology/kez044
178. Erdbruegger U, Grossheim M, Hertel B, Wyss K, Kirsch T, Woywodt A, et al. Diagnostic Role of Endothelial Microparticles in Vasculitis. Rheumatol (Oxford) (2008) 47(12):1820–5. doi: 10.1093/rheumatology/ken373
179. Brogan PA, Shah V, Brachet C, Harnden A, Mant D, Klein N, et al. Endothelial and Platelet Microparticles in Vasculitis of the Young. Arthritis Rheumatol (2004) 50(3):927–36. doi: 10.1002/art.20199
180. Pisetsky DS, Gauley J, Ullal AJ. Microparticles as a Source of Extracellular DNA. Immunol Res (2011) 49(1-3):227–34. doi: 10.1007/s12026-010-8184-8
181. Ullal AJ, Marion TN, Pisetsky DS. The Role of Antigen Specificity in the Binding of Murine Monoclonal Anti-DNA Antibodies to Microparticles From Apoptotic Cells. Clin Immunol (2014) 154(2):178–87. doi: 10.1016/j.clim.2014.05.007
182. Munoz LE, van Bavel C, Franz S, Berden J, Herrmann M, van der Vlag J. Apoptosis in the Pathogenesis of Systemic Lupus Erythematosus. Lupus (2008) 17(5):371–5. doi: 10.1177/0961203308089990
183. Rekvig OP, van der Vlag J. The Pathogenesis and Diagnosis of Systemic Lupus Erythematosus: Still Not Resolved. Semin Immunopathol (2014) 36(3):301–11. doi: 10.1007/s00281-014-0428-6
184. Pieterse E, van der Vlag J. Breaking Immunological Tolerance in Systemic Lupus Erythematosus. Front Immunol (2014) 5:164. doi: 10.3389/fimmu.2014.00164
185. van Bavel CC, Dieker J, Muller S, Briand JP, Monestier M, Berden JH, et al. Apoptosis-Associated Acetylation on Histone H2B is an Epitope for Lupus Autoantibodies. Mol Immunol (2009) 47(2-3):511–6. doi: 10.1016/j.molimm.2009.08.009
186. van Bavel CC, Dieker JW, Kroeze Y, Tamboer WP, Voll R, Muller S, et al. Apoptosis-Induced Histone H3 Methylation is Targeted by Autoantibodies in Systemic Lupus Erythematosus. Ann Rheum Dis (2011) 70(1):201–7. doi: 10.1136/ard.2010.129320
187. Nielsen CT, Ostergaard O, Rasmussen NS, Jacobsen S, Heegaard NHH. A Review of Studies of the Proteomes of Circulating Microparticles: Key Roles for Galectin-3-Binding Protein-Expressing Microparticles in Vascular Diseases and Systemic Lupus Erythematosus. Clin Proteomics (2017) 14:11. doi: 10.1186/s12014-017-9146-0
188. Wiklander OPB, Nordin JZ, O'Loughlin A, Gustafsson Y, Corso G, Mäger I, et al. Extracellular Vesicle In Vivo Biodistribution is Determined by Cell Source, Route of Administration and Targeting. J Extracell Vesicles (2015) 4:26316. doi: 10.3402/jev.v4.26316
189. Han C, Wang C, Chen Y, Wang J, Xu X, Hilton T, et al. Placenta-Derived Extracellular Vesicles Induce Preeclampsia in Mouse Models. Haematol (2020) 105(6):1686–94. doi: 10.3324/haematol.2019.226209
Keywords: microparticles, autoimmunity, microparticle isolation techniques, cell death, cellular communication
Citation: Rother N, Yanginlar C, Pieterse E, Hilbrands L and van der Vlag J (2022) Microparticles in Autoimmunity: Cause or Consequence of Disease? Front. Immunol. 13:822995. doi: 10.3389/fimmu.2022.822995
Received: 26 November 2021; Accepted: 25 March 2022;
Published: 20 April 2022.
Edited by:
Carmelo Carmona-Rivera, National Institute of Arthritis and Musculoskeletal and Skin Diseases (NIH), United StatesCopyright © 2022 Rother, Yanginlar, Pieterse, Hilbrands and van der Vlag. This is an open-access article distributed under the terms of the Creative Commons Attribution License (CC BY). The use, distribution or reproduction in other forums is permitted, provided the original author(s) and the copyright owner(s) are credited and that the original publication in this journal is cited, in accordance with accepted academic practice. No use, distribution or reproduction is permitted which does not comply with these terms.
*Correspondence: Johan van der Vlag, Sm9oYW4udmFuZGVyVmxhZ0ByYWRib3VkdW1jLm5s
Disclaimer: All claims expressed in this article are solely those of the authors and do not necessarily represent those of their affiliated organizations, or those of the publisher, the editors and the reviewers. Any product that may be evaluated in this article or claim that may be made by its manufacturer is not guaranteed or endorsed by the publisher.
Research integrity at Frontiers
Learn more about the work of our research integrity team to safeguard the quality of each article we publish.