- 1Centro de Biotecnología Acuícola, Facultad de Química y Biología, Universidad de Santiago de Chile, Santiago, Chile
- 2Facultad de Medicina Veterinaria y Agronomía, Universidad de Las Américas, Santiago, Chile
- 3Department of Cell Biology, Physiology, and Immunology, Universitat Autònoma de Barcelona, Barcelona, Spain
- 4Departamento de Biología, Facultad de Química y Biología, Universidad de Santiago de Chile, Santiago, Chile
Piscine orthoreovirus (PRV) is a virus in the genus Orthoreovirus of the Reoviridae family, first described in 2010 associated with Heart and Skeletal Muscle Inflammation (HSMI) in Atlantic salmon (Salmo salar). Three phases of PRV infection have been described, the early entry and dissemination, the acute dissemination phase, and the persistence phase. Depending on the PRV genotype and the host, infection can last for life. Mechanisms of immune response to PRV infection have been just beginning to be studied and the knowledge in this matter is here revised. PRV induces a classical antiviral immune response in experimental infection of salmonid erythrocytes, including transcriptional upregulation of ifn-α, rig-i, mx, and pkr. In addition, transcript upregulation of tcra, tcrb, cd2, il-2, cd4-1, ifn-γ, il-12, and il-18 has been observed in Atlantic salmon infected with PRV, indicating that PRV elicited a Th1 type response probably as a host defense strategy. The high expression levels of cd8a, cd8b, and granzyme-A in PRV-infected fish suggest a positive modulatory effect on the CTL-mediated immune response. This is consistent with PRV-dependent upregulation of the genes involved in antigen presentation, including MHC class I, transporters, and proteasome components. We also review the potential immune mechanisms associated with the persistence phenotype of PRV-infected fish and its consequence for the development of a secondary infection. In this scenario, the application of a vaccination strategy is an urgent and challenging task due to the emergence of this viral infection that threatens salmon farming.
Introduction
Piscine orthoreovirus (PRV) is a virus that belongs to the Reoviridae family, Spinareovirinae subfamily, and genus Orthoreovirus. PRV was firstly described in 2010 (1), as it was associated with Heart and Skeletal Muscle Inflammation (HSMI) in Atlantic salmon (Salmo salar) (2). The disease was described in 1999 in fish farms in Norway (3). In Chile, the first report of PRV was published in 2016. Authors found PRV strains in HSMI lesions of farmed Atlantic salmon, and Coho salmon (Oncorhynchus kisutch) (4). HSMI has been diagnosed all the years over the last decade in more than 100 farm centers in Norway (excepting 2017 and 2019) (5), resulting in economic losses estimated at €9 million annually (6). In Chile, 3.7% of the infectious disease mortality of Atlantic salmon, and 14.9% of the infectious disease mortality of Coho salmon were associated with HSMI during 2020 (7). Because of the epidemiological evolution observed in the sanitary surveillance, HSMI was categorized as an emerging disease in Chilean salmon farming (7). The economic impact of PRV infection is associated with mortality and melanized spots in salmon filets (8) that correlate with a pro-inflammatory environment (9). Another concern is that its etiological agent, the PRV, is reportedly spreading from farmed to wild Atlantic salmon with yet undetermined impacts (10). In seawater Atlantic salmon farms, the infection prevalence can reach up to 97% (11), and the etiological agent, PRV, is also present in freshwater Atlantic salmon presmolts with high frequency, found in parr between 30 and 60 grams (4).
PRV and Heart and Skeletal Muscle Inflammation
PRV is a dsRNA virus that has a genome composed of 10 RNA segments that can be classified into three different groups according to sizes: small segments (S1, S2, S3, S4) between 1040 and 1329 bp, medium (M1, M2, M3) between 2179 and 2403 bp, and large (L1, L2, L3) between 3911 and 3935 bp (1, 12, 13). The genome has at least 13 ORFs that encode for at least 11 proteins (14, 15). Eight of these proteins are structural components of the virus particle: segments L1, L3, M1, and S2 encode the inner capsid proteins λ1, λ3, µ2, and σ2, respectively; segments L2, M2, S1, and S4 encode for the outer capsid proteins λ2, µ1, σ3, and σ1, respectively; and segments S3, M3 and S1 encode for the three non-structural proteins σNS, µNS, and p13, respectively (Figure 1) (16, 17). PRV is a non-enveloped virus with an icosahedral structure (13).
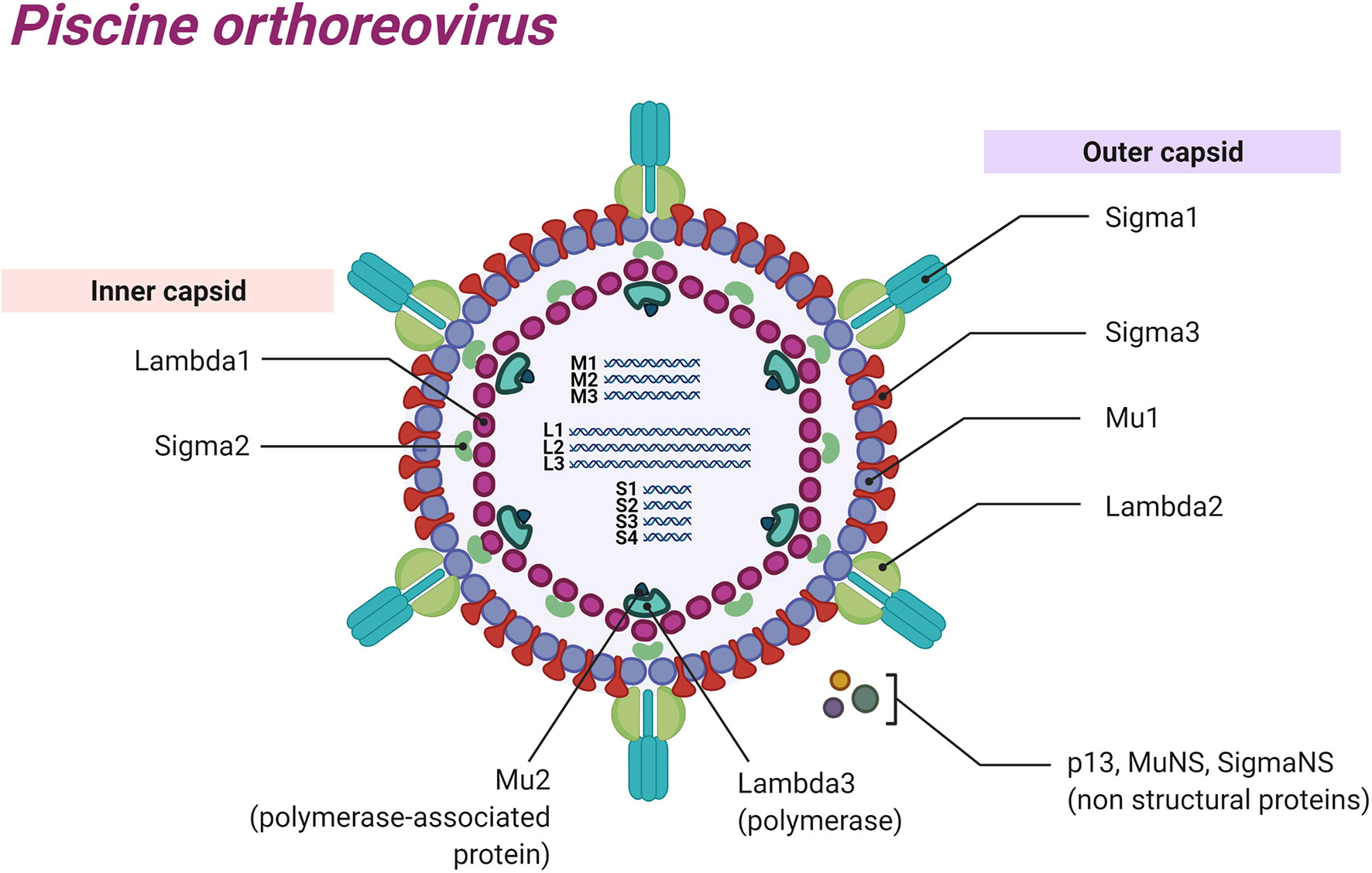
Figure 1 Schematic representation of Piscine orthoreovirus: Structural proteins, dsRNA segments and non-structural proteins are represented.
Three different subtypes of PRV have been described using the coding sequence of PRV segments, denominated as PRV-1, PRV-2 and PRV-3 (18, 19). Phylogenetic analysis focused on the PRV genomic segments S1, differentiates this virus into two major genotypes, I and II, and each of them into two subgenotypes designated as Ia and Ib, and IIa and IIb, respectively. Subgenotypes Ia and Ib make up the PRV-1 subtype and subgenotypes IIb and IIa correspond to the PRV-2 and PRV-3 subtypes, respectively (20).
Recently, with all Gen Bank available PRV sequences (May 2020) and using new PRV S1 and M2 segment sequences was determined that a significant number of the publicly available sequences belong to the PRV-1 subtype (subgenotypes Ia and Ib), less belong to the PRV-3 subtype (subgenotype IIa) and there are few sequences of PRV-2 subtype (subgenotype IIb) (15). PRV is the etiological agent of HSMI in Norway, Canada, Germany, Scotland, Iceland, and Chile. Recently, it was suggested that PRV-1 subgenotype Ib can be responsible for HSMI in Atlantic salmon (15) while the subgenotype Ia was associated with low virulence (12, 15). PRV-2 is a virus found only in Coho salmon in Japan (not associated with HSMI symptoms); while PRV-3 induces a disease similar to HSMI in rainbow trout and salmon coho in Norway, Germany and Chile (12, 15, 21, 22).
Although it is necessary to complement the study using methodologies based on complete genome sequencing, besides segments S1 and M2 of PRV a lower resolution and representativeness of the remaining eight genomic segments for classifications of subgenotypes or subtypes have been observed. Phylogenetic trees support the original classification using the PRV genomic segment S1 (15).
Three phases have been described for PRV infection: i) the early entry and dissemination, ii) the acute phase and iii) persistence (14). Two to three weeks after the host entry, the replication and dissemination of the virus occurs into blood cells (23, 24). In this phase no infection via cohabitation has been described so far (24). To this date, there is no clarity about the mechanism of entry of the virus (14). The acute phase appears after 4 to 6 weeks of exposure to the virus and is characterized by the development of acute inflammation of the heart muscle and skeletal muscle, and substantial PRV replication in the erythrocyte occurs (14, 17, 24, 25). The duration of this phase depends on several factors related to the host immunity and on the PRV genotype causing the infection (26, 27). Cohabitation challenges show a successful infection at this point. This phase lasts between 1 to 2 weeks and then, the load of viral proteins drops dramatically in the erythrocytes, the clinical signs of the disease disappear, and the virus becomes persistent (17, 25). In the persistence phase, the viral RNA is found in erythroid progenitor cells, erythrocytes, macrophages, melano-macrophages, and other uncharacterized cells in the kidney (28). At this phase, poor viral infection is produced by cohabitation, but i.p. injected PRV inoculum prepared from clarified lysed blood cells from persistence phase Atlantic salmon accomplishes successful infection into naive fish (24). The extent of this phase depends on the PRV genotype and the host, but experimental trials have shown that this phase probably can last for life. The early entry and dissemination and acute phases have only been described under laboratory conditions.
Heart and skeletal muscle inflammation was first described in farmed Atlantic salmon in 1999 (3). The disease appears mainly between 5 to 9 months after salmon are transferred to marine water (2) but has also been described even earlier at 14 days of the seawater transfer (3). The clinical signs of the disease may emerge during the peak of the acute phase, but the clinical signs are found usually in the early stages of the persistent phase (24, 29, 30). In the field, the mortality of infected fish is usually low but can also go up to 20% of the infected cages (3). Macroscopic signs are pale heart, pericardial bleeding, ascites, and a pale or stained liver, but hematocrit levels are usually normal. Lesions can occasionally be found in the liver, spleen, gills, and kidney. The main histopathological lesions are in the heart and skeletal muscle. In the heart, necrosis of myocytes, infiltration with mononuclear cells (mainly lymphocytes and macrophages), and a massive inflammatory response are observed associated with myocardial degeneration (26). Pericarditis is usually found in association with myocarditis (2), also, perivasculitis is found in myocardial blood vessels, coronary veins, and grooves. In severe cases, an infiltrative pattern is also found (26). Red muscle inflammation follows the same pattern as seen in the heart but is not a consistent finding (2) and it has been proposed to be attributed to seasonal variation being most prevalent in autumn and winter (26). In experimental conditions, the production of cardiac lesions consistent with HSMI is related to the virulence of the isolate (24, 26, 31). At the same time, the highest virulent isolates correlated with higher plasma viremia, while the low virulent isolates showed a lower amount of virus detected by qPCR (31). There was no correlation between any specific viral gene, protein, or amino acid differences with the virulence of the distinct PRV-1 isolates analyzed although the virus strain and host specific factors are necessary to initiate HSMI (31). For example, in Canada, Pacific-adapted Mowi-McConnell Atlantic salmon infected with PRV shows only mild or non-heart inflammation, even though these fish show high blood viremia (24). A review of the biology, geographic distribution, and host range of PRV has been recently published and is recommended for deeper details of the knowledge on this virus and HSMI (14).
Immune Response Against PRV Infection
PRV generates in ex vivo infected erythrocytes of Atlantic salmon upregulation of Interferon-a (ifn-a), Retinoic acid-inducible gene I (rig-i), Protein kinase R (pkr), and Myxovirus resistance gene (mx), all genes of the innate antiviral immune response (32, 33). In Atlantic salmon, intraperitoneal injected with PRV, ifn-a and mx-α were up-regulated in blood at 4 and 25 days post-challenge (dpc) and in heart, four dpc (34). The peak was in both tissues at four dpc, corresponding to the early infection stage, then the expression decreased to the control level (34). Similar results were reported in seawater adapted post-smolt salmon challenged with PRV by cohabitation. In these fish an increased expression of ifn-a, rig-i, pkr, mx-α, viperin, and Interferon-stimulated gene 15 (isg15) was observed in blood, heart, and in the spleen (35). In addition, a significant upregulation of both β-defensin and hepcidin genes in blood cells was reported at 4 weeks post challenge compared to day 0 (35). These studies show that PRV induces a strong innate immune response in Atlantic salmon, which may induce protection because the induced genes encode a dsRNA receptor like rig-i (35), interferon and interferon induced antiviral proteins such as pkr, mx-α, and viperin, and antiviral peptides like β-defensin and hepcidin (35). However, at this stage, it is unknown whether this response indeed results in protection because viruses have numerous evasion mechanisms against the IFN type I response (36). In fact, immune evasion mechanisms for IFN type I response have been reported for fish viruses such as IPNV, where preVP2, VP3, VP4, and VP5 viral proteins inhibit IFNa1 activation (37). Similarly, s7ORF1 of ISAV inhibits IFN and Mx transcription, while s8ORF2, acting as RNA silencing suppressor, inhibits IFN production (38–40). Therefore, further studies are required to understand whether PRV displays mechanisms to antagonize the IFN type I responses and antiviral peptides observed in infected fish.
Regarding adaptive immunity, transcript upregulation of T lymphocyte related genes such as T cell receptor-a (tcra), tcrb, cluster of differentiation 2 (cd2), and interleukin-2 (il-2) was reported in the head kidney of parr salmon challenged with PRV (41). In addition, cd4-1, the gene encoding the T cell co-receptor, was also upregulated in infected salmon (34), all indicating that PRV elicited the adaptive immune response in Atlantic salmon, which probably involves T cell proliferation. CD4+ CD3+ T cells (T helper) have been in fact identified, isolated and characterized in some fish species, including Japanese Pufferfish (Takifugu rubripes), ginbuna crucian carp (Carassius auratus langsdorfii), zebrafish (Danio rerio), rainbow trout (Oncorhynchus mykiss) (42–47); and rohu (Labeo rohita) (48). Although in Atlantic salmon, T cell isolation and characterization awaits further studies, transcriptional data and the studies in rainbow trout (44, 49–52) support the presence of T lymphocytes in this fish species and its role in response to the pathogens or model antigens. Furthermore, ifn-γ (41) and il-12 were also upregulated in PRV infected salmon (34), indicating that a T helper type-1 response can take place as a host defense strategy. In teleost fish, the differentiation of naive CD4+ T cells into Th1 cells appears to be possible because these cells express both the T-bet master transcription factor and ifn-γ during differentiation (44, 45, 47). Other studies suggest that the differentiation of the CD4+ T cell into Th1, Th2, Th17, Treg lymphocytes can occur in fish, mostly based on the fact that many Th-type cytokine genes have been identified in fish (53) and are upregulated in lymphoid tissues and isolated T cells after antigen stimulation (53). A Th1 response could induce PRV clearance, as in the presence of IFN-γ and IL-12, cellular-mediated immune response can eradicate intracellular pathogens like viruses (54). Furthermore, in HSMI-sick Atlantic salmon hearts, a strong signal of MHC-II in the lesion areas and a moderate signal of CD3+ (55) by immunohistochemistry was detected, suggesting the activation of CD4+ T cells in response to PRV infection.
Following interaction with various bacterial and viral pathogens, macrophages become activated and secrete a wide range of antiviral, pro-inflammatory, and immunomodulatory cytokines (56). Mirroring the Th type differentiation, the macrophages are classified as M1-like or M2-like cells (57, 58) and they reflect the bidirectional macrophage–lymphocyte interaction. Using in situ hybridization to identify double MCSFR and PRV positive cells, it has been found that macrophage-like cells of spleen and kidney, and in melano-macrophages of kidney contain PRV-1, indicating that macrophages might be targets of infections (28). In PRV-infected salmon of a commercial farm, lesions of the white muscle, known as red spots, show abundant iNOS (inducible nitric oxide synthase) in positive M1-polarized macrophages infected with PRV-1. Transformation of red spots into black spots was associated with the presence of arginase-2 expressing M2 melano-macrophages and the reduction of the relative number of PRV-1 in the white skeletal muscle (9). Interestingly, in experimental PRV infection, M1 macrophages does not appear related to the infection damage of the heart in HSMI, although M2 macrophages in heart tissue suggested a role in HSMI recovery (59). Since PRV upregulates IFN-γ and IL-12 during viral infection of Atlantic salmon, it seems plausible that an efficient well-regulated response induces M1 macrophage differentiation for virus clearance in the heart. Furthermore, as IFN-γ can also increase CD80/86, CD83, and MHC-II levels in salmon immune cells, an efficient clearance can also be due to antigen presentation and recognition improvement leading to a rapid immune response (60).
Regarding cellular immunity, recent studies shed light on the potential role of the cytotoxic T lymphocytes (CTL) during PRV infection. First, the high expression levels of cd8a, cd8b, and granzyme-A in the head kidney of PRV-infected fish suggest a positive modulatory effect on the CTL-mediated immune response (34) because fish CTLs express CD8 co-receptor and enzymes able to induce apoptosis of the target cells (61). Recently, a study has shown that Atlantic salmon CD8+ cells appear abundant in areas of the heart that contain PRV-1 infected cells after experimental challenge. Moreover, upregulation of CD8α correlated in time with a moderate decline in PRV-1 RNA levels (59). Interestingly, these results suggest a role of CD8+ cells in virus clearance but direct evidence for the role of functional CTLs (CD8+ T cells) during PRV infection is not available yet. The evaluation of PRV-specific CTL function will require haplotype-matched between the effector and target cells, which can be achieved using clonal teleost fish (62, 63) or infected autologous cells (64, 65). The potential role of CTL in PRV infection of fish is consistent with the Th1 type response observed in salmon because IFN-γ can stimulate the development of CD8+ T cells during viral infection (66). It is also consistent with the fact that PRV infection of salmon erythrocytes induced upregulation of the genes involved in antigen presentation via MHC class I, including transporters like tapasin (tapbp) and proteasome components like proteasome subunit beta type 9 (psmb9a) and proteasome subunit beta type 6 (psmb11) (33), which will positively impact the activation of CD8+ T cells. Activating the antigen-presenting machinery may also be a consequence of IFN-γ upregulation during PRV infection because, in turn, this cytokine upregulates many genes involved in antigen presentation (67).
Finally, regarding humoral immune response against PRV, production of IgM against the PRV μ1 and μNS proteins has been detected in plasma of PRV-1-infected Atlantic Salmon (68). Using a bead based multiplex immunoassays, anti-s1 IgM was also detected in salmon seven weeks after the exposure of PRV shedders. A reduction in HSMI lesions was observed when the specific IgM production reached a maximum level, suggesting a protective effect, even though this humoral immune response has been insufficient to eradicate PRV as viral RNA persisted in the blood of fish with PRV-specific IgM in challenge trials (68). Similarly, in PRV-3-infected rainbow trout a significant increase in -specific IgM in plasma is reported 8 weeks after the exposure (29). Notably, no studies are measuring neutralizing antibodies.
The current knowledge of the innate and adaptive immune response elicited by PRV infection is summarized in Figure 2.
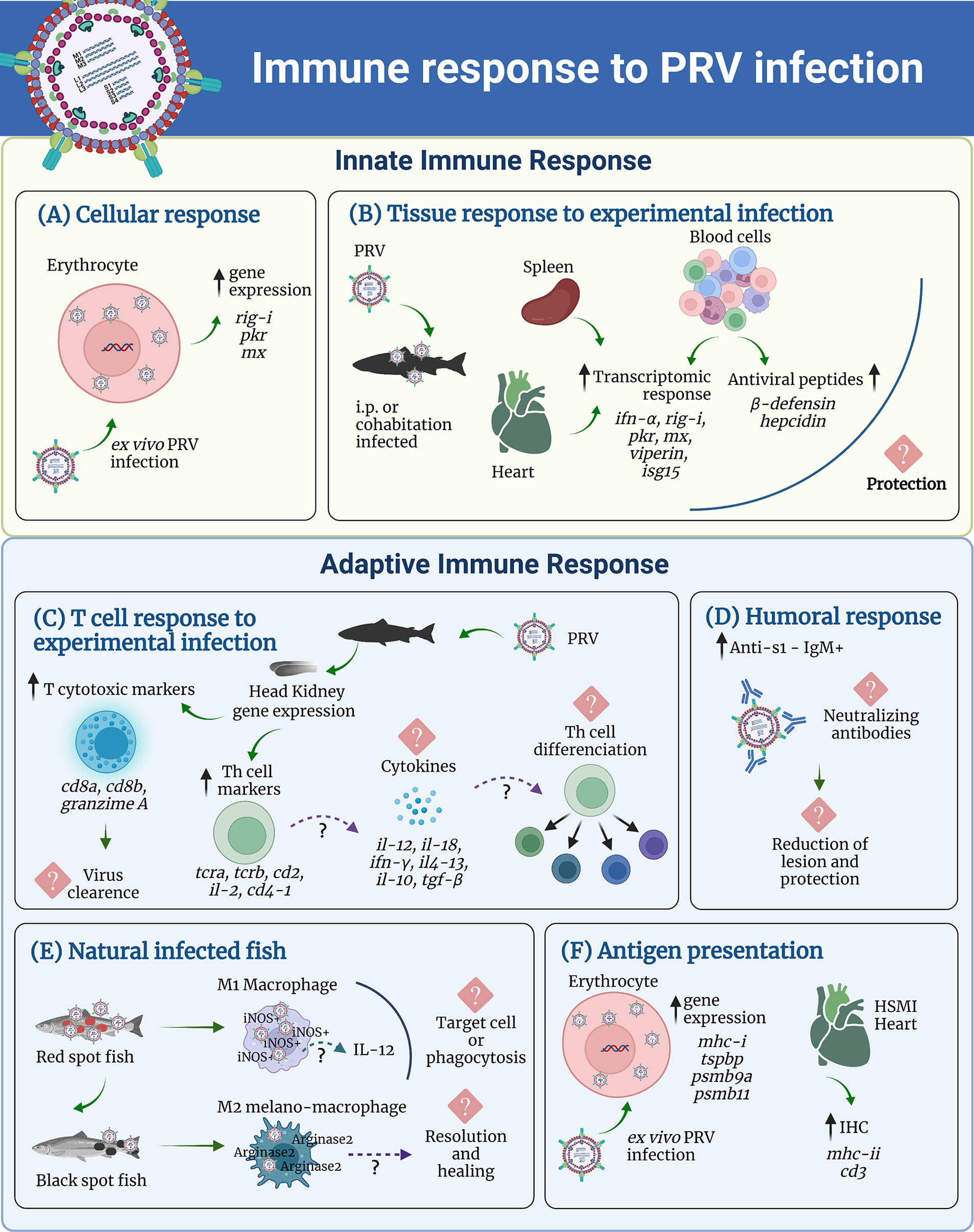
Figure 2 Summary of the current knowledge of the innate and adaptive immune response elicited by PRV infection. In the innate immune response panel, the transcriptome for (A) Cellular response, and (B) Tissue response to experimental infection is represented. For the adaptive immune response panel are shown, (C) T cell response to experimental infection, (D) humoral response, (E) macrophages response in naturally infected fish, and (F) Antigen presentation.
When the Fish Decides to Live With the Enemy: The Phenotype of Persistent Viral Infection in Atlantic Salmon
The persistence is a complex meta-stability exercise involving the overall outcome that favors the coexistence of the viral infection on the host, being considered one of the most successful surveillance strategies in the host-pathogen repertoire (69). In mammals, the phenotype of viral persistence is directly associated with the activity of inhibitory/immunosuppressive cytokines (70). In this process, the modulation of anti-inflammatory cytokines helps establish chronic viral infection (71–73). One of these anti-inflammatory cytokines of the viral persistence phenotype is IL-10, which impairs different immune mechanisms, including antigen recognition, cytokine production, antibody production, and cell proliferation processes, all vital processes for the success of immune response activation and resolution of infection (74). Consistently, viral persistence phenotype in Atlantic salmon infected with the infectious pancreatic necrosis virus (IPNV) is characterized by the upregulation of il-10, the low expression levels of il-1b and il-8 and low levels of total IgM (75). In PRV-infected Atlantic salmon, the persistence phenotype has been recently reported (28). The description of this persistence phenotype is associated with a high level of viral RNA (28) and low levels of viral proteins in the erythrocytes (17), in which an antiviral innate immune response is observed at the transcriptional level (33). Not the cellular or molecular immune mechanisms responsible for or associated with the persistence phenotype of PRV-infected fish are known.
Thus, it seems reasonable that several regulatory mechanisms associated with the recognition and activation of the immune response are activated in the PRV persistence phenotype. They can contribute to establishing a weakened immune status, helping to develop secondary infections with highly prevalent pathogens in salmon farming. Only one study has evaluated the potential effect of PRV on the development of secondary infections, thus centering on the co-occurrence of PRV and salmonid alphavirus (SAV) (35). In co-infected Atlantic salmon (PRV- and then SAV-infected), lower SAV neutralizing titers were observed compared with the controls infected with SAV only (35) suggesting a detrimental effect for immunity. Moreover, a positive correlation between PRV and SAV was observed in moribund or dead salmon (35). These data suggest that PRV infection may affect the infection and susceptibility to other pathogens present in farmed fish. For instance, as far as we know, no articles are reporting the consequences of PRV on the development of secondary bacterial infections of high prevalence in salmon farming. Taking together, it is urgent from the sanitary point of view to elucidating the consequence of the high PRV prevalence upon the risk for the development of a secondary infection that the infection by PRV might produce by itself.
PRV Vaccines
At present, there are no commercially available vaccines against HSMI in the market, and research and evaluation of PRV vaccines are still incipient. Only four studies performed on Atlantic salmon and Coho salmon have been reported (76–79). Wessel et al. (76) evaluated the protective effect of an inactivated vaccine using PRV purified from infected erythrocytes in a vaccination trial against HSMI.
Those immunized fish challenged with PRV by intraperitoneal injection showed a lower PRV load in blood cells and plasma compared both to PBS control group and vaccine control group (vaccinated with ALPHA JECT micro-6; PHARMAQ AS). Differences were observed for all the time-points assessed, i.e., 2-, 4-, 7-, and 10-weeks post-challenge (wpc). However, the PRV load was estimated based on the quantification cycle (Cq) value instead of absolute quantification. Beyond this methodological concern, only differences at 4wpc were registered between the PRV-inactivated vaccine group and the PBS-vaccinated group (76). Furthermore, the same trend was observed from the histopathological evidence on the heart. Thus, data indicate that PRV vaccination substantially reduced the severity of HSMI specific lesions, mainly in the experimental group following an unnatural way of infection (i.e., following an intraperitoneal injection), but did not prevent PRV infection and virus replication. Notably, a different study reported that the inactivated PRV-1 vaccine does not prevent PRV-1 infection and only partially protects against HSMI (78). Regrettably, the applicability of these vaccines is minimal because there are currently no reports of a cell line capable of producing PRV-1 viral progeny (80).
One common and ancient strategy for successful immunization is the cross-protection induced by related low virulent virus variants to cause low-grade disease (78). Particularly for HSMI, the cross-protection has been assessed using PRV-2 and PRV-3 genotypes not associated with disease development in Atlantic salmon. The cross-protection assay showed that the primary infection with intraperitoneally injected PRV-3 genotype completely blocked the infection against PRV-1 and the development of HSMI in Atlantic salmon ten weeks later the immunization with PRV-3 (78), which is in agreement with the fact that PRV-3 induces a disease similar to HSMI in rainbow trout and salmon coho (12, 15, 18, 21, 22, 78). The mechanisms of protection induced by PRV-3 are not known. In fact, the gene expression analysis of cellular immunity indicators (cd8α, ifn-γ, and granzyme-a) indicated that PRV-3 did not trigger spleen upregulation of these genes beyond ten weeks (78). The authors also state that antiviral immune genes viperin, myxovirus resistance gene (Mx), and interferon-stimulated gene (ISG-15) did not change their expression pattern (78).
By contrast, PRV-2 infection did not prevent PRV-1 infection, reducing only the severity of HSMI pathology punctually in some few individuals (78). Since PRV-2 is the etiological agent of a different disease in coho salmon (Oncorhynchus kisutch), named erythrocytic inclusion body syndrome (EIBS) (18), this results may have been expected. Perhaps the protection is associated with the higher amino acid identity of PRV-1 with PRV-3 (90%) than PRV-2 (80%) (81). Importantly, the high identity between PRV-3 and PRV-1 is present in proteins probably involved in the pathogenic effects (82, 83), such as the outer clamp protein σ3 (79.1%) and the non-structural protein p13 (78.2%) (81). Beyond these unknowns, one critical concern on the use of PRV-3 in immunizing Atlantic salmon is the possibility that the RNA segmented of PRV-3 and PRV-1 could reassort if they infect the same cell (84), in which case the consequences are unpredictable. Therefore, the side-effects and potential consequences of this type of immunizing strategy must be carefully analyzed.
In aquaculture, there are DNA vaccines licensed for commercial use for protecting Atlantic salmon against viruses including Infectious Hematopoietic Necrosis Virus (IHNV) (APEX-IHN; Novartis/Elanco) (85), and Salmon Pancreas Disease Virus (SPDV) (86). In this scenario, the vaccine efficacy against HSMI following intramuscular-injected immunization was assessed using pSAV-based replicon vaccines and pcDNA3.1-based expression vaccines. The Atlantic salmon vaccinated with pcDNA3.1 vector expressing µNS, σNS, and σ1 controlled by a CMV promoter showed a substantial reduction in the viral RNA load and the HSMI histopathological changes in epicardium and ventricle (77). By contrast, the pSAV cocktail replicons containing µNS + µ1 + σNS + σ1 + σ3 + λ2, slightly reduced the cardiac histopathological score, but did not reduce the PRV RNA levels in the blood after infection compared to the control, suggesting that the type and number of different expression vectors may influence on such differences (77). The secretion of specific antibodies as an inducer of protection through DNA vaccines (87) was not evaluated. Based on the study of Haatveit et al. (77) it seems that µNS and σ1 are the most promising PRV antigens for a DNA vaccine against HSMI in Atlantic salmon. However, the mechanism of action of these proteins activating the immune response remains to be elucidated. Consequently, the application of DNA vaccine as prophylactic treatment against aquaculture-related viruses, including PRV, must be further assessed.
Conclusions and Perspective
This article revised the current knowledge regarding the immune mechanisms activated in response to PVR infection. There is evidence that PRV activates the antiviral immunity in salmonid erythrocytes, one of its cellular infection targets. Currently, it is unclear whether activating an antiviral environment is enough to induce host protection. Because viruses, including aquatic viruses, show numerous immune evasion mechanisms against the IFN type I host response, understanding PRV-host interaction, which may antagonize the IFN type I response, needs to be addressed. The scope of other immune mechanisms against the PRV and its actual contribution to the resolution of infection, including the role of neutralizing antibodies, still need to be elucidated. Nonetheless, the eradication of virus does not always occur during infection in nature. In fact, for PRV, there are reports of persistent infection promoting host-pathogen coexistence. PRV persistence is characterized by immune modulators´ upregulation mainly associated with anti-inflammatory molecules. Consequently, this lower immune capacity to respond against a threat aggregates complexity to the mechanisms developed by the host for ensuring survival. Importantly, as far as we know, there is a gap in the knowledge concerning the cellular and molecular mechanisms responsible for the promotion of the persistence phenotype on PRV-infected fish.
The generation of further knowledge to understand the immune mechanisms in response to and for protection against PRV will make possible the development of strategies capable of effectively and efficiently facing this viral infection and the negative impact produced in the fish farming industry and the environment. Within the sustainable aquaculture industry framework, all the above is committed to the environment.
Author Contributions
All the authors wrote, read and approved the original draft.
Funding
This work has been supported by DICYT-USACH Postdoctoral fellowship (Nb. 022043IB) and Fondecyt iniciación (Proyect number 11221308).
Conflict of Interest
The authors declare that the research was conducted in the absence of any commercial or financial relationships that could be construed as a potential conflict of interest.
Publisher’s Note
All claims expressed in this article are solely those of the authors and do not necessarily represent those of their affiliated organizations, or those of the publisher, the editors and the reviewers. Any product that may be evaluated in this article, or claim that may be made by its manufacturer, is not guaranteed or endorsed by the publisher.
Acknowledgments
The authors thank the Fondecyt regular (project number 1201664 (MI), and 1211841 (FR-L)), Fondecyt iniciación (project number 11221308; EV-V) grants (ANID, Government of Chile), and DICYT-USACH Postdoctoral fellowship (Nb. 022043IB (MI; EV-V).
References
1. Palacios G, Lovoll M, Tengs T, Hornig M, Hutchison S, Hui J, et al. Heart and Skeletal Muscle Inflammation of Farmed Salmon Is Associated With Infection With a Novel Reovirus. PloS One (2010) 5:3–9. doi: 10.1371/journal.pone.0011487
2. Kongtorp RT, Taksdal T, Lyngøy A. Pathology of Heart and Skeletal Muscle Inflammation (HSMI) in Farmed Atlantic Salmon Salmo Salar. Dis Aquat Organisms (2004) 59:217–24. doi: 10.3354/dao059217
3. Kongtorp RT, Kjerstad A, Taksdal T, Guttvik A, Falk K. Heart and Skeletal Muscle Inflammation in Atlantic Salmon, Salmo Salar L.: A New Infectious Disease. J Fish Dis (2004) 27:351–8. doi: 10.1111/j.1365-2761.2004.00549.x
4. Godoy MG, Kibenge MJT, Wang Y, Suarez R, Leiva C, Vallejos F, et al. First Description of Clinical Presentation of Piscine Orthoreovirus (PRV) Infections in Salmonid Aquaculture in Chile and Identification of a Second Genotype (Genotype II) of PRV. Virol J (2016) 13:98. doi: 10.1186/s12985-016-0554-y
5. Sommerset I, Bang Jensen B, Borno B, Haukaas A, Brun E. The Health Situation in Norwegian Aquaculture 2020 (2021). Available at: www.vetinst.no.
6. Kristoffersen AB, Bang Jensen B, Jansen PA. Risk Mapping of Heart and Skeletal Muscle Inflammation in Salmon Farming. Prev Vet Med (2013) 109:136–43. doi: 10.1016/j.prevetmed.2012.08.012
7. SERNAPESCA. Informe Sanitario De La Salmonicultura En Centros Marinos Año, 2020. Servicio Nacional de Pesca y Acuicultura, Ministerio de Economía, Fomento y Turismo, Gobierno de Chile. Valparaíso (V Región), Chile. (2021).
8. Färber F. Melanin Spots in Atlantic Salmon Fillets-an Investigation of the General Problem, the Frequency and the Economic Implication Based on an Online Survey. Ås, Norway: Norwegian University of Life Sciences. (2017).
9. Malik MS, Bjørgen H, Nyman IB, Wessel Ø, Koppang EO, Dahle MK, et al. PRV-1 Infected Macrophages in Melanized Focal Changes in White Muscle of Atlantic Salmon (Salmo Salar) Correlates With a Pro-Inflammatory Environment. Front Immunol (2021) 12:664624. doi: 10.3389/fimmu.2021.664624
10. Morton A, Routledge R, Hrushowy S, Kibenge M, Kibenge F. The Effect of Exposure to Farmed Salmon on Piscine Orthoreovirus Infection and Fitness in Wild Pacific Salmon in British Columbia, Canada. PloS One (2017) 12:1–18. doi: 10.1371/journal.pone.0188793
11. Mordecai GJ, Miller KM, Bass AL, Bateman AW, Teffer AK, Caleta JM, et al. Aquaculture Mediates Global Transmission of a Viral Pathogen to Wild Salmon (2021). Available at: http://advances.sciencemag.org/.
12. Dhamotharan K, Tengs T, Wessel Ø, Braaen S, Nyman IB, Hansen EF, et al. Evolution of the Piscine Orthoreovirus Genome Linked to Emergence of Heart and Skeletal Muscle Inflammation in Farmed Atlantic Salmon (Salmo Salar). Viruses (2019) 11(5):465. doi: 10.3390/v11050465
13. Markussen T, Dahle MK, Tengs T, Løvoll M, Finstad ØW, Wiik-Nielsen CR, et al. Sequence Analysis of the Genome of Piscine Orthoreovirus (PRV) Associated With Heart and Skeletal Muscle Inflammation (HSMI) in Atlantic Salmon (Salmo Salar). PloS One (2013) 8(7):e70075. doi: 10.1371/journal.pone.0070075
14. Polinski MP, Vendramin N, Cuenca A, Garver KA. Piscine Orthoreovirus: Biology and Distribution in Farmed and Wild Fish. J Fish Dis (2020) 43:1331–52. doi: 10.1111/jfd.13228
15. Godoy M, Medina DA, Suarez R, Valenzuela S, Romero J, Kibenge M, et al. Extensive Phylogenetic Analysis of Piscine Orthoreovirus Genomic Sequences Shows the Robustness of Subgenotype Classification. Pathogens (2021) 10:1–12. doi: 10.3390/pathogens10010041
16. Dryden KA, Wang G, Yeager M, Nibert ML, Coombs KM, Furlong DB, et al. Early Steps in Reovirus Infection Are Associated With Dramatic Changes in Supramolecular Structure and Protein Conformation: Analysis of Virions and Subviral Particles by Cryoelectron Microscopy and Image Reconstruction. J Cell Biol (1993) 122:1023–41. doi: 10.1083/jcb.122.5.1023
17. Haatveit HM, Wessel Ø, Markussen T, Lund M, Thiede B, Nyman IB, et al. Viral Protein Kinetics of Piscine Orthoreovirus Infection in Atlantic Salmon Blood Cells. Viruses (2017) 9(3):49. doi: 10.3390/v9030049
18. Takano T, Nawata A, Sakai T, Matsuyama T, Ito T, Kurita J, et al. Full-Genome Sequencing and Confirmation of the Causative Agent of Erythrocytic Inclusion Body Syndrome in Coho Salmon Identifies a New Type of Piscine Orthoreovirus. PloS One (2016) 11:1–20. doi: 10.1371/journal.pone.0165424
19. Kuehn R, Stoeckle BC, Young M, Popp L, Taeubert JE, Pfaffl MW, et al. Identification of a Piscine Reovirus-Related Pathogen in Proliferative Darkening Syndrome (PDS) Infected Brown Trout (Salmo Trutta Fario) Using a Next-Generation Technology Detection Pipeline. PloS One (2018) 13(10):e0206164. doi: 10.1371/journal.pone.0206164
20. Kibenge FS. Emerging Viruses in Aquaculture. Curr Opin Virol (2019) 34:97–103. doi: 10.1016/j.coviro.2018.12.008
21. Adamek M, Hellmann J, Flamm A, Teitge F, Vendramin N, Fey D, et al. Detection of Piscine Orthoreoviruses (PRV-1 and PRV-3) in Atlantic Salmon and Rainbow Trout Farmed in Germany. Transbound Emerging Dis (2019) 66:14–21. doi: 10.1111/tbed.13018
22. Cartagena J, Tambley C, Sandino AM, Spencer E, Tello M. Detection of Piscine Orthoreovirus in Farmed Rainbow Trout From Chile. Aquaculture (2018) 493:79–84. doi: 10.1016/j.aquaculture.2018.04.044
23. Hauge H, Vendramin N, Taksdal T, Olsen AB, Wessel Ø, Mikkelsen SS, et al. Infection Experiments With Novel Piscine Orthoreovirus From Rainbow Trout (Oncorhynchus Mykiss) in Salmonids. PloS One (2017) 12:1–24. doi: 10.1371/journal.pone.0180293
24. Polinski MP, Marty GD, Snyman HN, Garver KA. Piscine Orthoreovirus Demonstrates High Infectivity But Low Virulence in Atlantic Salmon of Pacific Canada. Sci Rep (2019) 9:1–22. doi: 10.1038/s41598-019-40025-7
25. Finstad ØW, Dahle MK, Lindholm TH, Nyman IB, Løvoll M, Wallace C, et al. Piscine Orthoreovirus (PRV) Infects Atlantic Salmon Erythrocytes. Vet Res (2014) 45:1–13. doi: 10.1186/1297-9716-45-35
26. Di Cicco E, Ferguson HW, Schulze AD, Kaukinen KH, Li S, Vanderstichel R, et al. Heart and Skeletal Muscle Inflammation (HSMI) Disease Diagnosed on a British Columbia Salmon Farm Through a Longitudinal Farm Study. PLoS One (2017) 12(2):e0171471. doi: 10.1371/journal.pone.0171471
27. Lund M, Røsæg MV, Krasnov A, Timmerhaus G, Nyman IB, Aspehaug V, et al. Experimental Piscine Orthoreovirus Infection Mediates Protection Against Pancreas Disease in Atlantic Salmon (Salmo Salar). Vet Res (2016) 47:1–16. doi: 10.1186/s13567-016-0389-y
28. Malik MS, Bjørgen H, Dhamotharan K, Wessel Ø, Koppang EO, di Cicco E, et al. Erythroid Progenitor Cells in Atlantic Salmon (Salmo Salar) may be Persistently and Productively Infected With Piscine Orthoreovirus (PRV). Viruses (2019) 11:824. doi: 10.3390/v11090824
29. Vendramin N, Kannimuthu D, Olsen AB, Cuenca A, Teige LH, Wessel Ø, et al. Piscine Orthoreovirus Subtype 3 (PRV-3) Causes Heart Inflammation in Rainbow Trout (Oncorhynchus Mykiss). Vet Res (2019) 50:1–13. doi: 10.1186/s13567-019-0632-4
30. Wessel Ø, Braaen S, Alarcon M, Haatveit H, Roos N, Markussen T, et al. Infection With Purified Piscine Orthoreovirus Demonstrates a Causal Relationship With Heart and Skeletal Muscle Inflammation in Atlantic Salmon. PloS One (2017) 12:1–24. doi: 10.1371/journal.pone.0183781
31. Wessel Ø, Hansen EF, Dahle MK, Alarcon M, Vatne NA, Nyman IB, et al. Piscine Orthoreovirus-1 Isolates Differ in Their Ability to Induce Heart and Skeletal Muscle Inflammation in Atlantic Salmon (Salmo Salar). Pathogens (2020) 9:1–22. doi: 10.3390/pathogens9121050
32. Wessel Ø, Olsen CM, Rimstad E, Dahle MK. Piscine Orthoreovirus (PRV) Replicates in Atlantic Salmon (Salmo Salar L.) Erythrocytes Ex Vivo. Vet Res (2015) 46:1–11. doi: 10.1186/s13567-015-0154-7
33. Dahle MK, Wessel Ø, Timmerhaus G, Nyman IB, Jørgensen SM, Rimstad E, et al. Transcriptome Analyses of Atlantic Salmon (Salmo Salar L.) Erythrocytes Infected With Piscine Orthoreovirus (PRV). Fish Shellfish Immunol (2015) 45:780–90. doi: 10.1016/j.fsi.2015.05.049
34. Zhang Y, Polinski MP, Morrison PR, Brauner CJ, Farrell AP, Garver KA. High-Load Reovirus Infections do Not Imply Physiological Impairment in Salmon. Front Physiol (2019) 10:114. doi: 10.3389/fphys.2019.00114
35. Røsæg MV, Lund M, Nyman IB, Markussen T, Aspehaug V, Sindre H, et al. Immunological Interactions Between Piscine Orthoreovirus and Salmonid Alphavirus Infections in Atlantic Salmon. Fish Shellfish Immunol (2017) 64:308–19. doi: 10.1016/j.fsi.2017.03.036
36. Beachboard DC, Horner SM. Innate Immune Evasion Strategies of DNA and RNA Viruses. Curr Opin Microbiol (2016) 32:113–9. doi: 10.1016/j.mib.2016.05.015
37. Lauksund S, Greiner-Tollersrud L, Chang CJ, Robertsen B. Infectious Pancreatic Necrosis Virus Proteins VP2, VP3, VP4 and VP5 Antagonize Ifna1 Promoter Activation While VP1 Induces Ifna1. Virus Res (2015) 196:113–21. doi: 10.1016/j.virusres.2014.11.018
38. García-Rosado E, Markussen T, Kileng Ø, Baekkevold ES, Robertsen B, Mjaaland S, et al. Molecular and Functional Characterization of Two Infectious Salmon Anaemia Virus (ISAV) Proteins With Type I Interferon Antagonizing Activity. Virus Res (2008) 133:228–38. doi: 10.1016/j.virusres.2008.01.008
39. McBeath AJA, Collet B, Paley R, Duraffour S, Aspehaug V, Biering E, et al. Identification of an Interferon Antagonist Protein Encoded by Segment 7 of Infectious Salmon Anaemia Virus. Virus Res (2006) 115:176–84. doi: 10.1016/j.virusres.2005.08.005
40. Thukral V, Varshney B, Ramly RB, Ponia SS, Mishra SK, Olsen CM, et al. S8orf2 Protein of Infectious Salmon Anaemia Virus Is a RNA-Silencing Suppressor and Interacts With Salmon Salar Mov10 (Ssmov10) of the Host Rnai Machinery. Virus Genes (2018) 54:199–214. doi: 10.1007/s11262-017-1526-z
41. Johansen LH, Dahle MK, Wessel Ø, Timmerhaus G, Løvoll M, Røsæg M, et al. Differences in Gene Expression in Atlantic Salmon Parr and Smolt After Challenge With Piscine Orthoreovirus (PRV). Mol Immunol (2016) 73:138–50. doi: 10.1016/j.molimm.2016.04.007
42. Toda H, Saito Y, Koike T, Takizawa F, Araki K, Yabu T, et al. Conservation of Characteristics and Functions of CD4 Positive Lymphocytes in a Teleost Fish. Dev Comp Immunol (2011) 35:650–60. doi: 10.1016/j.dci.2011.01.013
43. Bird S, Zou J, Savan R, Kono T, Sakai M, Woo J, et al. Characterisation and Expression Analysis of an Interleukin 6 Homologue in the Japanese Pufferfish, Fugu Rubripes. Dev Comp Immunol (2005) 29:775–89. doi: 10.1016/j.dci.2005.01.002
44. Maisey K, Montero R, Corripio-Miyar Y, Toro-Ascuy D, Valenzuela B, Reyes-Cerpa S, et al. Isolation and Characterization of Salmonid CD4 + T Cells. J Immunol (2016) 196:4150–63. doi: 10.4049/jimmunol.1500439
45. Takizawa F, Magadan S, Parra D, Xu Z, Korytář T, Boudinot P, et al. Novel Teleost CD4-Bearing Cell Populations Provide Insights Into the Evolutionary Origins and Primordial Roles of CD4 Lymphocytes and CD4 Macrophages. J Immunol (2016) 196:4522–35. doi: 10.4049/jimmunol.1600222.Novel
46. Kono T, Korenaga H. Cytokine Gene Expression in CD4 Positive Cells of the Japanese Pufferfish, Takifugu Rubripes. PloS One (2013) 8(6):e66364. doi: 10.1371/journal.pone.0066364
47. Yoon S, Mitra S, Wyse C, Alnabulsi A, Zou J, Weerdenburg EM, et al. First Demonstration of Antigen Induced Cytokine Expression by CD4-1+ Lymphocytes in a Poikilotherm: Studies in Zebrafish (Danio Rerio). PloS One (2015) 10:1–26. doi: 10.1371/journal.pone.0126378
48. Rebello SC, Rathore G, Punia P, Sood N. Development and Characterization of a Monoclonal Antibody Against the Putative T Cells of Labeo Rohita. Cytotechnology (2016) 68:469–80. doi: 10.1007/s10616-014-9800-6
49. Hu Y, Maisey K, Subramani PA, Liu F, Flores-Kossack C, Imarai M, et al. Characterisation of Rainbow Trout Peripheral Blood Leucocytes Prepared by Hypotonic Lysis of Erythrocytes, and Analysis of Their Phagocytic Activity, Proliferation and Response to Pamps and Proinflammatory Cytokines. Dev Comp Immunol (2018) 88:104–13. doi: 10.1016/j.dci.2018.07.010
50. Zhang Y-A, Salinas I, Oriol Sunyer J. Recent Findings on the Structure and Function of Teleost Igt. Fish shellfish Immunol (2011) 31:627–34. doi: 10.1016/j.fsi.2011.03.021
51. Nakanishi T, Shibasaki Y. Matsuura Y. T Cells in Fish. Biol (Basel) (2015) 4:640–63. doi: 10.3390/biology4040640
53. Zou J, Secombes CJ. The Function of Fish Cytokines. Biology (2016) 5(2):23. doi: 10.3390/biology5020023
54. Zhou L, Chong MMW, Littman DR. Plasticity of CD4+ T Cell Lineage Differentiation. Immunity (2009) 30:646–55. doi: 10.1016/j.immuni.2009.05.001
55. Yousaf MN, Koppang EO, Skjødt K, Hordvik I, Zou J, Secombes C, et al. Comparative Cardiac Pathological Changes of Atlantic Salmon (Salmo Salar L.) Affected With Heart and Skeletal Muscle Inflammation (HSMI), Cardiomyopathy Syndrome (CMS) and Pancreas Disease (PD). Vet Immunol Immunopathol (2013) 151:49–62. doi: 10.1016/j.vetimm.2012.10.004
56. Porcheray F, Viaud S, Rimaniol AC, Léone C, Samah B, Dereuddre-Bosquet N, et al. Macrophage Activation Switching: An Asset for the Resolution of Inflammation. Clin Exp Immunol (2005) 142:481–9. doi: 10.1111/j.1365-2249.2005.02934.x
57. Biswas SK, Mantovani A. Macrophage Plasticity and Interaction With Lymphocyte Subsets: Cancer as a Paradigm. Nat Immunol (2010) 11:889–96. doi: 10.1038/ni.1937
58. Nikonova A, Khaitov M, Jackson DJ, Traub S, Trujillo-Torralbo MB, Kudlay DA, et al. M1-Like Macrophages Are Potent Producers of Anti-Viral Interferons and M1-Associated Marker-Positive Lung Macrophages Are Decreased During Rhinovirus-Induced Asthma Exacerbations. EBioMedicine (2020) 54:102734. doi: 10.1016/j.ebiom.2020.102734
59. Malik MS, Nyman IB, Wessel Ø, Dahle MK, Rimstad E. Dynamics of Polarized Macrophages and Activated CD8+ Cells in Heart Tissue of Atlantic Salmon Infected With Piscine Orthoreovirus-1. Front Immunol (2021) 12:729017. doi: 10.3389/fimmu.2021.729017
60. Morales-Lange B, Ramírez-Cepeda F, Schmitt P, Guzmán F, Lagos L, Øverland M, et al. Interferon Gamma Induces the Increase of Cell-Surface Markers (CD80/86, CD83 and MHC-II) in Splenocytes From Atlantic Salmon. Front Immunol (2021) 12:666356. doi: 10.3389/fimmu.2021.666356
61. Somamoto T, Koppang EO, Fischer U. Antiviral Functions of CD8+ Cytotoxic T Cells in Teleost Fish. Dev Comp Immunol (2014) 43:197–204. doi: 10.1016/j.dci.2013.07.014
62. Somamoto T, Nakanishi T, Okamoto N. Specific Cell-Mediated Cytotoxicity Against a Virus-Infected Syngeneic Cell Line in Isogeneic Ginbuna Crucian Carp. Dev Comp Immunol (2000) 24:633–40. doi: 10.1016/S0145-305X(00)00018-5
63. Dijkstra JM, Fischer U, Sawamoto Y, Ototake M, Nakanishi T. Exogenous Antigens and the Stimulation of MHC Class I Restricted Cell-Mediated Cytotoxicity: Possible Strategies for Fish Vaccines. Fish Shellfish Immunol (2001) 11:437–58. doi: 10.1006/fsim.2001.0351
64. Hogan RJ, Stuge TB, Clem LW, Miller NW, Chinchar VG. Anti-Viral Cytotoxic Cells in the Channel Catfish (Ictalurus Punctatus). Dev Comp Immunol (1996) 20:115–27. doi: 10.1016/0145-305X(95)00043-S
65. Chang Y-T, Kai Y-H, Chi S-C, Song Y-L. Cytotoxic CD8α+ Leucocytes Have Heterogeneous Features in Antigen Recognition and Class I MHC Restriction in Grouper. Fish Shellfish Immunol (2011) 30:1283–93. doi: 10.1016/j.fsi.2011.03.018
66. Whitmire JK, Tan JT, Whitton JL. Interferon-γ Acts Directly on CD8+ T Cells to Increase Their Abundance During Virus Infection. J Exp Med (2005) 201:1053–9. doi: 10.1084/jem.20041463
67. Pereiro P, Figueras A, Novoa B. Insights Into Teleost Interferon-Gamma Biology: An Update. Fish Shellfish Immunol (2019) 90:150–64. doi: 10.1016/j.fsi.2019.04.002
68. Teige LH, Lund M, Haatveit HM, Røsæg MV, Wessel Ø, Dahle MK, et al. A Bead Based Multiplex Immunoassay Detects Piscine Orthoreovirus Specific Antibodies in Atlantic Salmon (Salmo Salar). Fish Shellfish Immunol (2017) 63:491–9. doi: 10.1016/j.fsi.2017.02.043
69. Goic B, Saleh MC. Living With the Enemy: Viral Persistent Infections From a Friendly Viewpoint. Curr Opin Microbiol (2012) 15:531–7. doi: 10.1016/j.mib.2012.06.002
70. Alcami A, Koszinowski UH. Viral Mechanisms of Immune Evasion. Trends Microbiol (2000) 8:410–8. doi: 10.1016/S0966-842X(00)01830-8
71. MacDonald AJ, Duffy M, Brady MT, McKiernan S, Hall W, Hegarty J, et al. CD4 T Helper Type 1 and Regulatory T Cells Induced Against the Same Epitopes on the Core Protein in Hepatitis C Virus–Infected Persons. J Infect Dis (2002) 185:720–7. doi: 10.1086/339340
72. Blackburn SD, Wherry EJ. IL-10, T Cell Exhaustion and Viral Persistence. Trends Microbiol (2007) 15:143–6. doi: 10.1016/j.tim.2007.02.006
73. Redpath S, Angulo A, Gascoigne NRJ, Ghazal P. Murine Cytomegalovirus Infection Down-Regulates MHC Class II Expression on Macrophages by Induction of IL-10. J Immunol (1999) 162:6701–7.
74. Ouyang W, O’Garra A. IL-10 Family Cytokines IL-10 and IL-22: From Basic Science to Clinical Translation. Immunity (2019) 50:871–91. doi: 10.1016/j.immuni.2019.03.020
75. Reyes-Cerpa S, Reyes-López F, Toro-Ascuy D, Montero R, Maisey K, Acuña-Castillo C, et al. Induction of Anti-Inflammatory Cytokine Expression by IPNV in Persistent Infection. Fish Shellfish Immunol (2014) 41:172–82. doi: 10.1016/j.fsi.2014.08.029
76. Wessel Ø, Haugland Ø, Rode M, Fredriksen BN, Dahle MK, Rimstad E. Inactivated Piscine Orthoreovirus Vaccine Protects Against Heart and Skeletal Muscle Inflammation in Atlantic Salmon. J Fish Dis (2018) 41:1411–9. doi: 10.1111/jfd.12835
77. Haatveit HM, Hodneland K, Braaen S, Hansen EF, Nyman IB, Dahle MK, et al. DNA Vaccine Expressing the Non-Structural Proteins of Piscine Orthoreovirus Delay the Kinetics of PRV Infection and Induces Moderate Protection Against Heart -and Skeletal Muscle Inflammation in Atlantic Salmon (Salmo Salar). Vaccine (2018) 36:7599–608. doi: 10.1016/j.vaccine.2018.10.094
78. Malik MS, Teige LH, Braaen S, Olsen AB, Nordberg M, Amundsen MM, et al. Piscine Orthoreovirus (Prv)-3, But Not Prv-2, Cross-Protects Against Prv-1 and Heart and Skeletal Muscle Inflammation in Atlantic Salmon. Vaccines (2021) 9:1–20. doi: 10.3390/vaccines9030230
79. Matsuyama T, Takano T, Honjo M, Kikuta T, Nawata A, Kumagai A, et al. Enhancement of Piscine Orthoreovirus-2 DNA Vaccine Potency by Linkage of Antigen Gene to a Trigger Factor Gene or Signal Peptide Genes. Aquaculture (2021) 533:736163. doi: 10.1016/j.aquaculture.2020.736163
80. Pham PH, Misk E, Papazotos F, Jones G, Polinski MP, Contador E, et al. Screening of Fish Cell Lines for Piscine Orthoreovirus-1 (PRV-1) Amplification: Identification of the Non-Supportive PRV-1 Invitrome. Pathogens (2020) 9:1–25. doi: 10.3390/pathogens9100833
81. Dhamotharan K, Vendramin N, Markussen T, Wessel Ø, Cuenca A, Nyman IB, et al. Molecular and Antigenic Characterization of Piscine Orthoreovirus (PRV) From Rainbow Trout (Oncorhynchus Orthoreovirumykiss). Viruses (2018) 10:1–16. doi: 10.3390/v10040170
82. Key T, Read J, Nibert ML, Duncan R. Piscine Reovirus Encodes a Cytotoxic, Non-Fusogenic, Integral Membrane Protein and Previously Unrecognized Virion Outer-Capsid Proteins. J Gen Virol (2013) 94:1039–50. doi: 10.1099/vir.0.048637-0
83. Yue Z, Shatkin AJ. Double-Stranded RNA-Dependent Protein Kinase (PKR) Is Regulated by Reovirus Structural Proteins. Virology (1997) 234:364–71. doi: 10.1006/viro.1997.8664
84. Mcdonald SM, Nelson MI, Turner PE, Patton JT. Reassortment in Segmented RNA Viruses: Mechanisms and Outcomes Outcomes. Nat Rev Microbiol (2016) 14:448–60. doi: 10.1038/nrmicro.2016.46
85. Salonius K, Simard N, Harland R, Ulmer JB. The Road to Licensure of a DNA Vaccine. Curr Opin Invest Drugs (2007) 8:635–41.
86. Collins C, Lorenzen N, Collet B. DNA Vaccination for Finfish Aquaculture. Fish Shellfish Immunol (2019) 85:106–25. doi: 10.1016/j.fsi.2018.07.012
Keywords: piscine orthoreovirus, double strand RNA (dsRNA) virus, heart and skeletal muscle inflammation (HSMI), antiviral immune response, pro-inflammatory cytokines, fish vaccines, aquaculture, emerging diseases
Citation: Vallejos-Vidal E, Reyes-López FE, Sandino AM and Imarai M (2022) Sleeping With the Enemy? The Current Knowledge of Piscine Orthoreovirus (PRV) Immune Response Elicited to Counteract Infection. Front. Immunol. 13:768621. doi: 10.3389/fimmu.2022.768621
Received: 31 August 2021; Accepted: 25 February 2022;
Published: 06 April 2022.
Edited by:
Maria Del Mar Ortega-Villaizan, Miguel Hernández University of Elche, SpainReviewed by:
Espen Rimstad, Norwegian University of Life Sciences, NorwayFrancisca Samsing, The University of Sydney, Australia
Copyright © 2022 Vallejos-Vidal, Reyes-López, Sandino and Imarai. This is an open-access article distributed under the terms of the Creative Commons Attribution License (CC BY). The use, distribution or reproduction in other forums is permitted, provided the original author(s) and the copyright owner(s) are credited and that the original publication in this journal is cited, in accordance with accepted academic practice. No use, distribution or reproduction is permitted which does not comply with these terms.
*Correspondence: Eva Vallejos-Vidal, RXZhLnZhbGxlam9zdkB1c2FjaC5jbA==