- 1Department of Biosciences and Bioengineering, Indian Institute of Technology, Roorkee, India
- 2Center for Global Infectious Disease Research, Seattle Children’s Research Institute, Seattle, WA, United States
Malaria is a global infectious disease that remains a leading cause of morbidity and mortality in the developing world. Multiple environmental and host and parasite factors govern the clinical outcomes of malaria. The host immune response against the Plasmodium parasite is heterogenous and stage-specific both in the human host and mosquito vector. The Plasmodium parasite virulence is predominantly associated with its ability to evade the host’s immune response. Despite the availability of drug-based therapies, Plasmodium parasites can acquire drug resistance due to high antigenic variations and allelic polymorphisms. The lack of licensed vaccines against Plasmodium infection necessitates the development of effective, safe and successful therapeutics. To design an effective vaccine, it is important to study the immune evasion strategies and stage-specific Plasmodium proteins, which are targets of the host immune response. This review provides an overview of the host immune defense mechanisms and parasite immune evasion strategies during Plasmodium infection. Furthermore, we also summarize and discuss the current progress in various anti-malarial vaccine approaches, along with antibody-based therapy involving monoclonal antibodies, and research advancements in host-directed therapy, which can together open new avenues for developing novel immunotherapies against malaria infection and transmission.
1. Introduction
Plasmodium is a genus of unicellular eukaryotes that are obligate parasites of vertebrates and insects. Protozoan parasites belonging to the genus Plasmodium, mainly cause malaria, which is prevalent mainly in tropical and subtropical regions, and is a major global health problem (1). Malaria is a life-threatening disease, which is transmitted to humans via the female Anopheles mosquito. Although there are more than 100 species of Plasmodium which can infect many animal species, five species of Plasmodium (P. falciparum, P. vivax, P. malariae, P. ovale and P. knowlesi) have long been recognized to infect humans and cause illness (2). Among these five Plasmodium species, infection with P. falciparum accounts for more than 90% of the world’s malaria mortality and P. falciparum and P. vivax are involved in causing high disease burden in Sub-Saharan and Asian regions (3, 4). According to the latest statistics, there were approximately 241 million cases of malaria globally with nearly 627,000 deaths in 2020 (5). A high incidence of malaria has been reported in the African region which contributes to about 95% of cases resulting in 96% of malaria deaths; out of which, children under the age of five accounted for 80% of malaria deaths (5). Plasmodium life cycle alternates between the primary host (mosquito) and secondary host (human). Plasmodium completes asexual development inside human hepatocytes and erythrocytes. Inside hepatocytes, the parasite undergoes differentiation into trophozoite and schizont stages to form first generation of merozoites (6). Merozoites invade human red blood cells (RBCs) and undergo erythrocytic schizogony to develop through ring, trophozoite and schizont stages. Schizonts release merozoites that continue to infect erythrocytes to initiate the erythrocytic cycle. Some of the asexually replicating parasites commit and differentiate into gametocytes. Gametocytes develop through stages I-V over two weeks inside erythrocytes and erythroblasts (7). Stage V gametocytes are taken up in blood meal and they rapidly differentiate into gametes. A male gametocyte undergoes three rounds of rapid DNA replication to form eight flagellated male gametes (microgametes). On the other hand, a female gametocyte forms a single female gamete (macrogamete). Male and female gametes undergo fertilization to form a short-lived zygote. This short-lived zygote differentiates into motile ookinete. The ookinete ultimately develops into oocysts. Sporozoites forms inside oocysts which migrate to the salivary glands of mosquito. Sporozoites stay in the salivary glands for initiation of the next infection cycle (6, 7). The Plasmodium life-cycle thus represents a series of differentiation stages, which are characterized by the expression of stage-specific proteins, some of which are targets of host immune response.
In this review, we have discussed various host defence mechanisms and counter mechanisms employed by Plasmodium when it undergoes multiple stages of development inside a human host. Various anti-malarial drugs, such as chloroquine and primaquine are associated with adverse side effects. Additionally, malarial parasite can acquire drug resistance, which necessitates the development of alternative immunotherapeutics (8, 9). Despite numerous studies on vaccine candidates, there is no licensed vaccine against Plasmodium infection. The major obstacle in anti-malaria vaccine development is antigenic variants, therefore identification of promiscuous T-cell and B-cell epitopes may improve vaccine development strategies. This review provides current and updated information regarding various anti-malarial vaccine candidates. Since humoral immune responses and antibody effector functions largely contribute to anti-malaria immunity, this review also details various monoclonal antibodies developed and their efficacy against multiple stages of Plasmodium parasite. Furthermore, we discuss the development of host-directed therapy which can block the transmission of the parasite and may prove to be effective in the management of severe malaria infections.
2. Parasite survival or immune evasion strategies in mammalian and mosquito hosts
Although the host immune system can reduce the parasite burden, malarial parasites have a variety of efficient immune evasion mechanisms. These immune evasion mechanisms make the host immune system ineffective to prevent the parasite’s development and progression through the skin, liver, blood, and spleen at various stages.
2.1. Parasite survival or immune evasion strategies in mammalian host
During vector transmission to humans, Plasmodium sporozoites are injected into the dermis. The sporozoites migrate from the dermis to the liver and proceed to the liver stage and blood stage cycle. Plasmodium parasite undergoes a complex infection cycle where it interacts with various host cells and modulates their functions (10). Early clearance of parasites by the innate immune system is inefficient due to several strategies employed by Plasmodium to evade the host immune system. Skin is a physical barrier that sporozoites encounter after transmission into the human host (11). Sporozoites employ strategies such as cell traversal and motility to pass this physical barrier. Cell traversal proteins such as SPECT1 (sporozoite microneme protein essential for cell traversal) and SPECT2 are utilized by sporozoites to achieve successful migration to the liver (12). Another sporozoite surface protein, TRAP (thrombospondin-related anonymous protein) is responsible for sporozoite motility through the dermis. TRAP also interacts with host cells through binding to sulfated glycoconjugates motifs which results in cell surface recognition and entry to liver cells (13). Upon mosquito bite, neutrophils are the first to be recruited at the site of infection. Neutrophils and monocytes can phagocytose sporozoites. However, upregulation of the Agaphelin protein can have negative effects on neutrophil chemotaxis and NET development (14). Monocytes can inhibit the growth of parasites by antibody-dependent cellular inhibition (ADCI) (15). However, ingestion of hemozoin (parasite pigment) impairs the function of monocytes and macrophages and represses their ability to produce inflammatory cytokines (16).
2.1.1. Parasite survival or immune evasion strategies during the liver stage
To establish a successful infection in hepatocytes, the sporozoites need to cross the barrier of specialized phagocytic cells in the liver, also known as Kupffer cells (KCs) (17). Although KCs can kill most invading microorganisms, sporozoites have various strategies to evade KC-mediated defence response. The interactions of sporozoites are mediated by circumsporozoite protein (CSP) which binds to heparin sulfate proteoglycans present on the surface of KCs (18). CSP also interacts with LRP-1 (low-density lipoprotein-related protein), which upregulates the intracellular levels of cAMP/EPAC and prevents ROS formation. Prevention of ROS formation contributes to parasite survival (19). In the rodent malaria model involving P. yoelii, it has been reported that sporozoites can modulate the cytokine response via upregulation of Th2 cytokines and downregulation of Th1 cytokines, which aids in sporozoite survival and invasion through liver cells (20). CSP protein has been shown to inhibit IL-12, IL-6 and TNF-α secretion, and increase IL-10 and TGF-β levels, which can aid in immune invasion (21, 22). Furthermore, sporozoites can also manipulate the key functions of KCs by impairing their antigen presentation capacity and inducing forceful apoptosis (23). Plasmodium parasite is also known to produce MIF (macrophage inhibitory factor) cytokine. MIF inhibits the migration and activation of phagocytes. It can also manipulate T-cell differentiation resulting in reduced anti-Plasmodium CD4+ T-cell response (24, 25).
Antibodies against free sporozoites and CSP are the first line of defence to prevent the invasion of hepatocytes (26). Antibody-effector functions such as neutralization, complement activation phagocytosis and antibody-dependent cellular cytotoxicity (ADCC) play an important role in eliminating sporozoites (27). However, the parasite can shed CSP during cell traversal in the liver and evade antibody-mediated clearance. Furthermore, CSP has multiple tandem repeats which can downregulate antibody isotype maturation. Sporozoites are known to modulate hepatocyte functions which contribute to their intra-hepatocytic proliferation and survival. Release of CSP by sporozoites causes suppression of the NF-kB signalling which negatively affects the host immune mechanisms (28). Sporozoites alter host inflammatory responses via upregulation of host heme oxygenase-1 protein (HO-1) (29). Furthermore, sporozoite infection of hepatocytes affects the mTOR pathway, which leads to an alteration of intracellular proteins involved in cell growth, proliferation, and survival (30). After hepatocyte invasion, sporozoites develop a membrane called parasitophorous vacuolar membrane (PVM) around their cell surface which protects them from selective autophagy and apoptosis. This membrane-enclosed structure helps the parasite to overcome its intracellular degradation while residing inside the host cells (31). A parasite-derived PVM-resident protein upregulated in infectious sporozoites 4 (UIS4), interacts with the host cell actin and by suppressing filamentous actin formation, UIS4 avoids parasite elimination (32). Hepatic Merozoites employ various immune evasion strategies to overcome the role of liver phagocytic cells in their development. Merozoites protect themselves from the liver phagocytic cells by getting released inside merosomes (33). These immune evasion strategies employed by merozoites during liver stages further clear their path for entering to blood stage. Each hepatic merozoite can subsequently invade RBCs and initiate blood stage development. Since RBCs do not express MHC molecules on their surface, erythrocytic merozoites escape recognition by CD8+ T-cells (34).
2.1.2. Parasite survival or immune evasion strategies during blood stage
During the blood stage of infection, Plasmodium employs various immune evasion strategies to evade the host’s immune response. Plasmodium manipulates the NF-kB and Type 1 interferon pathway to drive inflammation responsible for malaria pathogenesis (35). Intracellular parasitism is responsible for the immune escape of the parasite from antibodies. As antibodies can only bind extracellular/free sporozoites or merozoites, therefore when parasites invade host cells, antibodies cannot cross the cell membrane, preventing the antibody function (36). Antigenic diversity/polymorphism and expression of antigenic variants at different stages of infection are two major immune evasion strategies which promote parasite survival and contribute to long-lasting parasite infections (36). To invade RBCs, merozoites express a variety of surface proteins like MSP-1 (merozoite surface protein). MSP-1 interacts with glycosylphosphatidylinositol (GPI) anchors present on RBCs (37). Antigenic diversity involves the expression of antigenically different alleles of a gene in different parasite populations. For example, msp1 has many alleles and antibodies to one msp1 allele cannot recognize others. Another class of merozoite proteins namely erythrocyte binding-like (EBL) proteins promote immune evasion. Both MSPs and EBLs are present as multiple alleles, thereby showing a high degree of polymorphism (38, 39).
2.1.3. A mechanism of antigenic variation during blood stage
The most prominent immune escape strategy which is employed by Plasmodium is the expression of antigenic variants during its blood stage. Antigenic variation is maintained by variant surface antigens (VSAs). VSAs consist primarily of an immunodominant molecule known as P. falciparum erythrocyte membrane protein 1 (PfEMP1) encoded by the var multigene family (40, 41). PfEMP1 protein expression on infected RBCs (iRBCs) is responsible for adhesion to endothelial cells (40). Adherence of parasitic forms to endothelial cells aid in immune evasion, preventing their entry into the spleen and liver, which may lead to severe forms of cerebral malaria (42). Antibodies to PfEMP1 on the surface of iRBCs interfere with its binding to endothelial cells. Antigenic variation helps the parasite to escape the host antibody response. The genome of P. falciparum contains about 60 var genes, encoding a different variant of PfEMP1. The gene expression of PfEMP1 is highly regulated and only one var gene express at a time. Although antibody-mediated response against a single PfEMP1 variant can reduce the parasite burden to some extent. However, a small fraction of parasites switch the var gene expression, encoding a different PfEMP1 variant which results in immune evasion from antibody-mediated response (43). PfEMP1 encoding region of var gene contains two exons and one conserved intron. Each var gene contains two promoters, one promotor gives rise to PfEMP1-encoding mRNA which contributes to mutually exclusive expression of PfEMP1 variants. The other bidirectional promotor found within the intron region drives the expression of chromatin-associated sense and anti-sense, long non-coding RNAs (lncRNAs) (44). Regulatory elements such as lncRNAs may have transcriptional control over var gene expression. While sense lncRNAs are expressed during later stages of parasite development, the antisense lncRNA is expressed only from the single active var gene at the early stages of parasite development in RBCs, when var mRNA is transcribed (45). Anti-sense lncRNA recruits the proteins required for chromatin modifications and transcriptional activation. They are majorly involved in the mutually exclusive expression of PfEMP1 variants which contribute to antigenic variation and host immune evasion by parasite (46). Recently, one group of researchers have identified an anti-sense lncRNA-associated protein, PfTPx-1 which localizes to specific nuclear subcompartment and creates a redox-controlled microenvironment essential for the active transcription of var genes. Furthermore, alterations in PfTPx-1 expression influence both gene switching as well as transcriptional activation of var genes (47). Although var genes are involved in PfEMP1 expression which is a key to parasite survival in their host, the mechanism of mutually exclusive expression of var genes is not completely understood. The histone modifications is involved in the epigenetic regulation of var gene expression (48). In a study, Volz et al. identified the role of histone methyltransferase, PfSET10 in antigenic variation of malaria parasite. They concluded that PfSET10 is not only required for var gene expression but it also plays an important role in parasite viability (49). However, more recently, Ngwa et al. reported that the disruption of PfSET10 causes no effect on var gene expression (50). Furthermore, there is a lot of uncertainty and contradiction in the role of some histone deacetylase genes, PfSir2a and PfSir2b (51, 52). Various mechanisms such as changes in subnuclear localization and enzymatic activity of proteins involved in epigenetic regulation can be responsible for such huge differences/variations in experimental results. Therefore, it warrants considerable caution to interpret the results of such experiments. Notably, knockouts of PfRecQ helicases cause dysregulation of var gene expression suggesting their role in var gene regulation (53, 54). In a recent study, CRISPR/dCas9 has been used to explore the role of other var gene regulatory elements. A complex of chromatin remodeler proteins, PfISWI has been identified which may have a role in transcriptional activation of var genes. Further, functional characterization of PfISWI may provide insights into transcription control of var genes (55). Future research is needed for the molecular and functional characterization of more epigenetic regulators which can reveal the underlying mechanisms of antigenic variation. Moreover, the inhibitors of epigenetic regulator can be employed as potent anti-malarial drugs (50). Apart from PfEMP1, variant proteins such as RIFIN (early trophozoite) and STEVOR (mature trophozoite), belonging to other multigene families (rif and stevor) also contribute to the adherence of iRBCs to endothelial cells, leading to their sequestration in the microvascular system of host organs, preventing splenic elimination (42, 56). Both trophozoites and schizonts employ sequestration as another strategy for immune evasion. Interestingly, PfEMP1 also induces direct immunosuppressive effects on various types of immune cells (57, 58). Recent studies using humanized mice demonstrated that parasites adapted to thrive in the humanized mice showed enhanced expression of specific PfEMP1s such as VAR2CSA. Expression of VAR2CSA protected the parasites from macrophage phagocytosis and also reduced NK cell-mediated killing through interaction with the immune inhibitory receptor, LILRB1 (59, 60). Of note, the role of neutrophil mediated innate immune response against iRBCs has been examined in a recent study. The neutrophil expresses ICAM-1 which can interact with PfEMP1 resulting in killing of iRBCs (61). Moreover, RIFIN proteins aid in host immune evasion via targeting LILRB1. They can inhibit the activation of LILRB1-expressing NK cells and B-cells. Further studies are required to understand the interactions between polymorphic proteins and host immune inhibitory receptors which may prove crucial for the regulation of malaria infection (62).
2.2. Parasite survival or immune evasion strategies in mosquito host
Mosquitoes become infected when they ingest human blood containing gametocytes. The gametocytes complete their maturation in the midgut lumen. The gametocytes differentiate into gametes, which undergo fertilization to form zygote. The Plasmodium zygote matures into an ookinete. Physical barriers such as peritrophic membrane (PM) of the midgut, acts as a first line of defense of Anopheles mosquito against ookinetes (63). Ookinetes secretes chitinase enzyme which helps to clear their way through PM (64). Ookinetes are also exposed to the midgut proteases. To evade the midgut proteases, ookinetes express surface proteins P25 and P28 which plays an important role in midgut invasion (65). The most important parasite factor, P47 which is encoded by high polymorphic Pf47 gene, is involved in mosquito immune evasion in P. falciparum. P47 interfere with the complement-like immune responses of mosquito (66, 67). Moreover, P47 also inhibits JNK pathway-mediated apoptosis of P. falciparum (68). In P. berghei, P47 is also essential for ookinete protection from the Anopheles complement-like response (68). Another parasite protein, PIMMS43 (Plasmodium Infection of the Mosquito Midgut Screen 43) expressed on the surface of ookinete and sporozoites is required for parasite evasion from mosquito complement-like response (69). The host-parasite interactions have immensely contributed to our understanding of parasite survival strategies and host immune evasion mechanisms. During the past few decades, most of the host immune evasion proteins such as CSP, TRAP, MSP, PfEMP1, P28, P47 etc. have been assessed in experimental setting. These proteins have been assessed as potential vaccine candidates against different life stages of Plasmodium. A list of Plasmodium proteins involved in host immune evasion is presented in Table 1.
3. Host defence mechanisms against Plasmodium in mammalian and mosquito hosts
3.1. Host defence mechanisms against Plasmodium in mammalian host
3.1.1. Role of innate immunity in host defence in mammalian host
The complement system acts as the first line of defence against parasites and is considered a major player during innate immunity. Malarial parasite evades the host complement system at different stages. Surface molecules of P. falciparum are involved in capturing host complement regulator proteins which inhibits complement activities. It has been suggested that sporozoites are resistant to complement-mediated cell lysis (77). During the blood stage, free merozoites and intracellular schizonts bind to complement proteins which contributes to parasite survival. For instance, interaction of Pf92 and GAP50 proteins with complement regulator proteins, FH and FHL-1 leads to the inactivation of C3b (70–72). Additionally, knob-like protrusions of PfEMP1 on the surface of iRBCs have been shown to prevent complement fixation (74). Of note, Plasmodium can hijack complement receptor 1 (CR1) as an entry receptor for invading RBCs using parasite ligand PfRh4 (73). Furthermore, PfEMP1 variants can interact with various RBC receptors such as CR1 and alpha2-macroglobulin to mediate rosetting/rosette formation (75, 76). Rosette formation is another strategy employed by Plasmodium to evade the host immune response, wherein iRBCs form clusters with uninfected RBCs. It interferes with immune recognition and enhances parasite virulence (78). It has been reported that the release of complement-deposited digestive vacuoles by iRBCs leads to macrophage exhaustion. Furthermore, it can induce the lysis of adjacent RBCs and erythrophagocytosis, contributing to anaemia (79). A recent study showed that the acquisition of human plasminogen facilitates complement evasion by Plasmodium. It has been shown that the plasminogen promotes C3b inactivation and prevents terminal complement complex formation (80). Moreover, in severe malaria cases, P. falciparum inhibits the membrane attack complex which results in complement evasion (81).
3.1.2. Role of humoral immunity in host defence in mammalian host
Humoral immunity plays a crucial role against Plasmodium. Antibody-mediated responses largely contribute to host’s anti-malarial immunity. The major antibody functional activities include ADCC, ADCI, growth inhibition and inhibition of host cell invasion (3, 82). Plasmodium parasite expresses a wide variety of parasitic factors/proteins at multiple stages. Antibodies targeting these parasitic factors have revealed the importance of stage-specific functional antibody responses in malaria. The antibody effector functions against Plasmodium may vary with parasite stage (4). Host antibodies generated against sporozoites can inhibit their motility, traversal and invasion to hepatocytes. Further, antibodies can enhance complement-mediated lysis of sporozoites and inhibition of hepatocyte traversal (26, 27). During blood stage, they promote phagocytosis and complement-mediated lysis of merozoites. Moreover, antibodies targeting merozoites can directly inhibit their invasion of RBCs. Furthermore, antibodies bind to the surface of the iRBC and promote their agglutination and phagocytosis (3, 11). Antibodies towards iRBCs can block the schizont egress, rosette formation and their sequestration to host endothelium and epithelium (11). More research on antibody-mediated effector functions can contribute to our understanding of host-parasite interaction which may improve the anti-malaria vaccine development strategies.
3.1.3. Role of cellular immunity in host defence in mammalian host
Along with phagocytic cells, NK cells are known to mediate innate immune functions by secreting IFN-γ enabling parasite clearance, and directly killing infected cells by cytotoxicity (16). Additionally, NK cells are also involved in killing P. falciparum-infected RBCs by producing perforins, IFN-γ and granzymes (83). Plasmodium is known to interact with dendritic cells (DCs) at every stage of their life cycle. DCs can phagocytose sporozoites and prime antigen-specific T-cell responses (84). However, Plasmodium inhibits DC activation and functioning which interferes with the development of protective immune responses (85). In addition, Plasmodium infection can lead to reduced DC numbers due to increased DC apoptosis (86). T-cells via their cell surface receptors can recognize parasite-generated epitopes which interact with MHC molecules present on the cell surface of antigen-presenting cells (APCs). P. falciparum has been shown to inhibit the maturation of APCs, resulting in impaired T-cell responses (87). Among the CD4+ T-cell population, regulatory T-cells play an important role in parasite immune responses. It has been shown that malarial parasites exhibit a novel immune mechanism via preferentially activating T-reg cells with enhanced suppressive activity (88). Proinflammatory cytokine response mediated by helper CD4+ T-cells activates macrophages which helps to control merozoites via phagocytosis (89). Further, CD4+ T-cells activate specific B-cell clones which contribute to antibody-mediated effector functions against merozoites (90). CD8+ T-cells can kill parasite-infected hepatocytes using perforin and granzymes, through MHC I-associated recognition (83). Further, cytotoxic CD8+ T-cells produce IFN-γ which plays an important role in the killing of intrahepatic sporozoites and is associated with protection from malaria (91). However, the role of CD8+ T-cells in the blood stage is negligible because RBCs lack MHC molecules which prevent immune recognition of the parasite and help the parasite to escape CD8+ T-cell response (3, 92). It has been speculated that Plasmodium utilizes a variety of cryptic T-cell epitopes to evade immune responses (93). Additionally, high levels of polymorphisms in the parasite epitopes can lead to immune evasion of the CTL response and alter memory T-cell effector functions (94).
3.2. Host defence mechanisms against Plasmodium in mosquito host
Complement-like or thioester-containing protein 1 (TEP1) is the major protein involved in the humoral immune response against Plasmodium. TEP1 gets accumulated on the ookinete surface for parasite killing and lysis. However, silencing TEP1 increases oocyst counts. Furthermore, TEP1 melanize the parasite and blocking TEP1 expression significantly reduces melanization of Plasmodium (95). Plasmodium utilizes two C-type lectins (CTL4 and CTLMA2) from the mosquito to escape from the immune system. Silencing of CTL4 and CTLMA2 in susceptible mosquitoes triggered melanization and reduced oocyst formation (96). Recently, Kolli et al. reported that glutaminyl cyclase (QC) mediated post-translational modifications of Plasmodium surface proteins can contribute to parasite evasion by disrupting mosquito immune responses such as melanization or hemocytes-mediated phagocytosis (97). The primary immune cells involved in mosquito innate immune response are hemocytes. Hemocytes such as prohemocytes, granulocytes, and oenocytoids are involved in various innate immune mechanisms against Plasmodium (98). Hemocytes along with fat bodies of hemolymph secrete immune factors which trigger secretion of antimicrobial peptides and induce phagocytosis, agglutination, melanization and encapsulation of parasites (99). Furthermore, reactive oxygen species (ROS) produced by hemocytes are also involved in mosquito immunity against P. falciparum (100). Mosquito midgut epithelial cells secrete immune-modulatory peroxidase (IMPer) which is crucial in the formation of dityrosine network. The dityrosine network is utilized by parasites to evade midgut immune response via inactivating NOS (Nitric oxide synthase) expression (101). Inside mosquito midgut, Plasmodium gametocytes differentiate into gametes, which fertilize to form zygote and subsequently progress to ookinetes. When ookinetes reaches to basal lamina, they differentiate into oocysts. Antibodies can prevent the Plasmodium development during mosquito stage by preventing gamete fusion and inducing complement-mediated killing of gametes/ookinetes. Antibodies can also prevent penetration and motility of ookinete through midgut wall and formation of oocysts (11). Oocysts mature and release sporozoites into mosquito haemocoel. A malaria scavenger-like (SR) protein is necessary for sporozoite development. Disruption of PbSR protein inhibits sporozoite formation (102). Sporozoites show positive chemotaxis toward salivary glands. At this stage sporozoites uniformly express CSP proteins which are essential for salivary gland invasion (103). Sporozoites are accumulated in the salivary duct of Anopheles mosquito and are ready to complete the malaria transmission cycle.
4. Vaccine candidates against Plasmodium
Plasmodium expresses a variety of surface antigens during its developmental stages- pre-erythrocytic stage, erythrocytic stage, gametocyte/sexual stage and mosquito stage. Over the past few decades, various anti-malaria vaccine candidates have been assessed from different parasite stages (Figure 1).
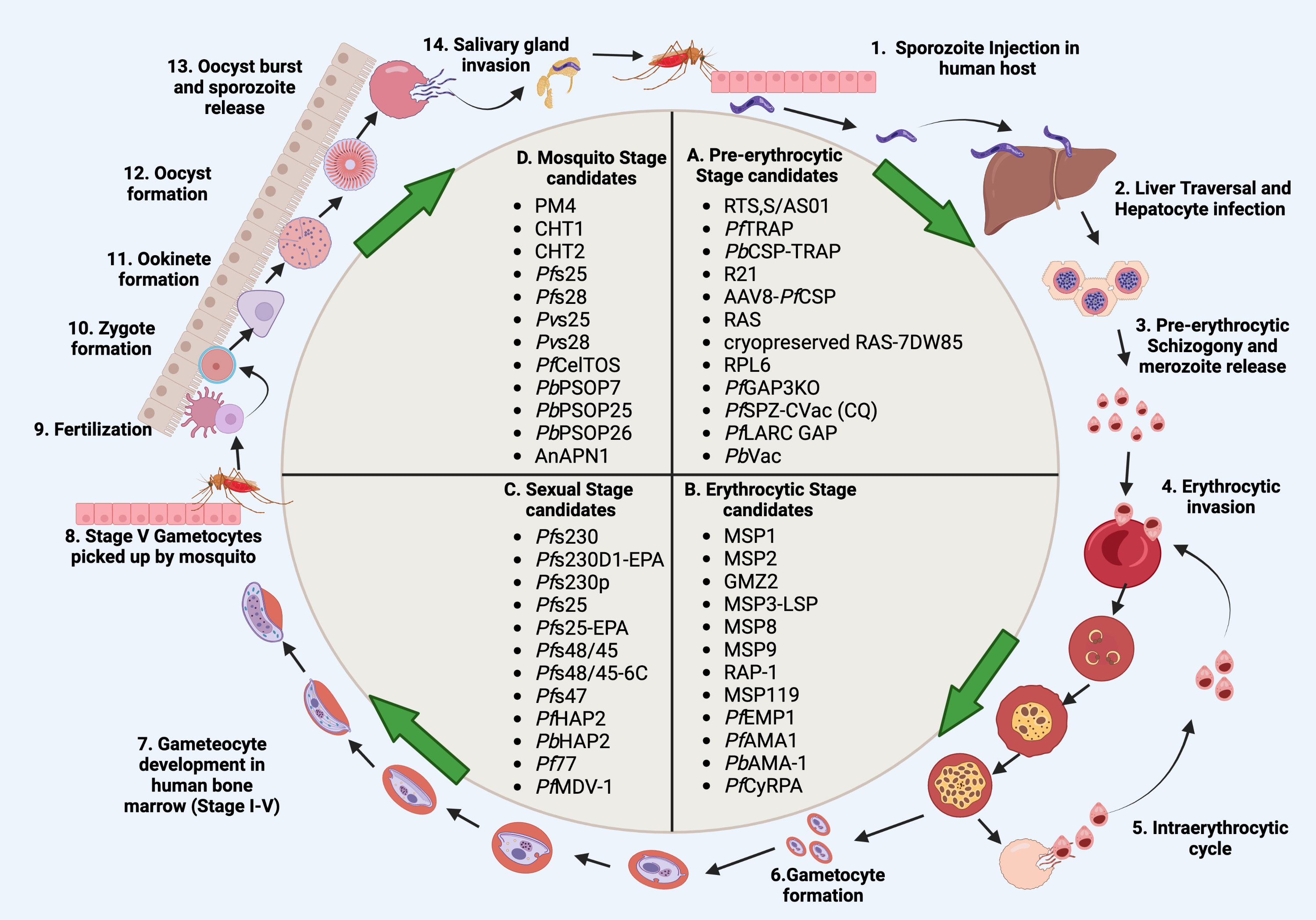
Figure 1 Schematic representation of malaria vaccine candidates during different developmental stages. (A) Pre-erythrocytic candidates (RTS,S/AS01, PfTRAP, PbCSP-TRAP, R21, AAV8-PfCSP, RAS, cryopreserved RAS-7DW85, RPL6, PfGAP3KO, PfSPZ-CVac (CQ), PfLARC GAP, PbVac). (B) Erythrocytic candidates MSP1, MSP2, GMZ2, MSP3-LSP, MSP8, MSP9, RAP-1, MSP119, PfEMP1, PfAMA1, PbAMA-1, PfCyRPA). (C), Sexual stage candidates (Pfs230, Pfs230D1-EPA, Pfs230p, Pfs25, Pfs25-EPA, Pfs48/45, Pfs48/45-6C, Pfs47, PfHAP2, PfHAP2p, PbHAP2, Pf77, PfMDV-1). (D) Mosquito stage candidates PM4, CHT1, CHT2, Pfs25, Pfs28, Pvs25, Pvs28, PfCelTOS, PbPSOP7, PbPSOP25, PbPSOP26, AnAPN1). Steps 1-14 show the malaria parasite life cycle which completes in four stages; pre-erythrocytic, erythrocytic, sexual, and mosquito stages. During the pre-erythrocytic stage, sporozoites are injected by an infected mosquito into the human host which then migrates to the liver and infects hepatocytes. Sporozoites start pre-erythrocytic schizogony by forming schizonts. Schizonts rupture and release merozoites into blood circulation. Merozoites invade erythrocytes which initiates the erythrocytic stage. Merozoites differentiate into different forms such as ring, trophozoite and schizont forms. Schizonts rupture and release either merozoites or gametocytes. Merozoites start the intraerythrocytic cycle while gametocytes undergo further development in the bone marrow. While inside bone marrow, the gametocytes differentiate into sequential gametocyte stages (Stage I-V). Stage V gametocytes move to peripheral circulation and are then picked up by the mosquito. Gametocytes develop in the mosquito midgut and differentiate into microgametes (male gametes) and macrogametes (female gametes). Fertilization takes place in the mosquito midgut which forms a short-lived zygote which transforms into a motile zygote, ookinete. The ookinete develops into an oocyst and sporozoite development starts within the oocyst. The oocyst ruptures and releases the sporozoites, which then invade the salivary glands of the mosquito. The life cycle of the malaria parasite restarts when the mosquitoes bite another human host. Created with BioRender.com.
4.1. Pre-erythrocytic stage vaccine candidates
When an infected mosquito bites the human host, sporozoites are injected through the skin. Sporozoites contain surface antigens which are involved in Plasmodium development in the human host. The sporozoite surface antigens act as putative vaccine antigens which can induce protective humoral immune responses and are currently under clinical trials (104). One of the most potent sporozoite surface proteins is CSP. CSP protein is required by Plasmodium during developmental stages in both the primary mosquito host (mosquito stage) and secondary human host (pre-erythrocytic stage). CSP protein of P. falciparum sporozoites contains highly conserved protein domains structures which have been characterized by repeating amino acid, asparagine-alanine-asparagine-proline (NANP) motifs (105). CSP has been shown to induce high antibody titres indicating their role in conferring protection in animal models (106). Currently, there is only one anti-malaria vaccine which has reached phase 3 trial, namely, RTS,S, which targets PfCSP protein (107). However, when RTS,S was administered with a liposome-based adjuvant, AS01, it showed limited efficacy and short-lived protection (107). RTS,S/AS01 (trade name Mosquirix) has been recently approved by WHO for broad use in children (108). Another protein antigen TRAP, which is critical for sporozoite motility is considered a promising vaccine candidate. In one study, BALB/c mice were immunized with recombinant P. falciparum TRAP (PfTRAP) along with poly (I:C) adjuvant. Vaccination with PfTRAP induced Th1 immune response and high titers of protective IgG antibodies (109). In another study, a vaccine formulation was prepared by fusion of P. berghei CSP and TRAP antigen along with Addavax adjuvant. The mice were immunized with P. berghei CSP-TRAP which elicited higher antibody titers (110). Recent studies have shown that co-immunization with several other pre-erythrocytic vaccine antigens can confer sterile protection in rodent malaria models (111), necessitating replication of these studies using human malaria parasite pre-erythrocytic vaccine antigens. Recently, R21, a malaria vaccine, which targets PfCSP has been developed. The administration of R21 with matrix M (a lipid-based adjuvant) has been shown to improve immunogenicity and enhance protection. R21 is an emerging vaccine formulation which is under phase II field trials and needs further investigations (112). In another study, intravenous administration of an Adeno-associated virus serotype 8 (AAV8) vector-based anti-sporozoite vaccine containing PfCSP (AAV8-PfCSP) generated protective humoral and cellular immune responses by inducing high antibody titres and recruiting liver-resident memory CD8+ T (TRM) cells in a mice model (113). In addition, immunization with peptides or protein fragments from a sporozoite, liver stage tryptophan-rich protein (SLTRiP) showed significant reduction in parasite numbers during liver stage by inducing a long lasting and protective CD8+ T memory response (114, 115).
Intravenous administration of radiation-attenuated sporozoite (RAS) vaccines induces Plasmodium-specific TRM cells which confer protection in mice against wild-type sporozoite challenge. RAS vaccination strategy has been improved by prime and trap strategy which involves epidermal priming of CSP antigen. A single intravenous dose of RAS aid in the activation of TRM in the spleen, along with trapping and expansion of CD8+ T-cells in the liver region of BALB/c mice (116). Further, cryopreserved RAS vaccination induced similar levels of CD8+ T-cell responses in mouse liver and protected mice against wild-type sporozoite challenge (116). Ribosomal protein RPL6 is a natural peptide antigen which is expressed by Plasmodium during pre-erythrocytic stage infection. Prime and trap vaccination strategy targeting RPL6 was used for the elimination of Plasmodium infection in mouse liver. RPL6 induced effective protection by inducing liver TRM cell response against P. berghei sporozoites challenge in mice (117).
Some vaccine development approaches such as genetically attenuated parasites (GAP), utilize genetic attenuation/deletion of genes essential for the completion of liver stage development (118). Sanaria® PfSPZ-GA1 is a genetically attenuated whole sporozoite vaccine. It was generated by knocking out B9 and SLARP genes to halt the development of sporozoites in the early liver stages (119). Another GAP vaccine, PfGAP3KO vaccine was generated by knocking out three genes, P. falciparum p52−/p36−/sap1− expressed in the pre-erythrocytic stage (120). The PfGAP3KO vaccine was administered to humanized mice model transplanted with human hepatocytes and RBCs. PfGAP3KO was unable to complete its development from the liver stage to the blood stage, thereby protecting against the sporozoite challenge (120). Another study tested the safety and immunogenicity of the PfGAP3KO vaccine in human volunteers and a single dose administration of the PfGAP3KO vaccine elicited a protective antibody-mediated immune response against sporozoite infection (121). In addition, PfGAP3KO protected malaria-naïve subjects from controlled human malaria infection (122). Recently, a late liver stage arresting replication-competent (PfLARC) GAP was generated against the human malaria parasite. Specifically, a LARC GAP for P. falciparum was generated by deleting the Mei2 (Meiosis inhibited 2) gene. The Mei2 gene is expressed by the late liver-stage parasite. PfMei2- liver stages failed to complete their intra-hepatic development and do not form infectious exoerythrocytic merozoites (123). Another immunization approach which is simple, efficacious, safe and highly immunogenic during malaria vaccination is P. falciparum sporozoites under chemoprophylaxis vaccination (PfSPZ-CVac). In this approach, human volunteers are immunized with cryopreserved PfSPZ along with a 10 mg/kg chloroquine base. PfSPZ-CVac immunization conferred protection in malaria-naive volunteers by inducing high levels of anti-PfCSP antibodies (124). While PfSPZ-CVac (CQ) was safe and conferred protection to malaria-naïve participants in controlled human malaria infection, this vaccine was unable to protect against P. falciparum infection in a very high transmission setting (125).
It has been shown that P. berghei-based vaccination (PbVac) confers cross-species protection against P. falciparum malaria (126). P. berghei is highly amenable to a genetic modification that enables the gene insertion of other human Plasmodium species antigens (such as CSP) into its genome loci, which may aid in the expression of heterologous Plasmodium antigens (127). Immunization with such chimeric P. berghei sporozoites derived from heterologous immunogens is expected to elicit both cross-species immune responses as well as targeted immunity against human Plasmodium parasites (128). P. berghei-based vaccines expressing both the protein, PbCSP and PfCSP at the surface of sporozoites were administered in rabbits via bites of PbVac-infected mosquitoes. This immunization elicited PfCSP-specific immune responses which inhibited both in vitro and in vivo P. falciparum infection of human hepatocytes (128). Although PbVac was not able to confer sterile protection in phase 1/2a clinical trials, it elicited dose-dependent humoral and cellular immune responses, thereby reducing the liver parasite burden (129). Further exploration is required for the assessment of such vaccination approaches against P. falciparum malaria.
4.2. Erythrocytic stage vaccine candidates
Induction of protective humoral, as well as cellular immune responses against Plasmodium, is the primary goal in the development of malaria vaccines. The vaccine antigens from the erythrocytic stage can be utilized in reducing the parasite burden. The protective antibodies generated against these antigens can either block the merozoite invasion of erythrocytes or lead to phagocytosis of merozoites (130). A variety of MSPs and invasion complex proteins are responsible for erythrocyte invasion. It has been reported that msp1 and msp2 show high levels of genetic polymorphism which may complicate the malaria vaccine development (131). However, another study reported that MSP1 contains conserved B-cell epitopes indicating that MSP1 could serve as a promising vaccine candidate against P. vivax malaria (132). In another study, the engraftment of MSP2 proteins obtained from P. falciparum with liposomes and supplemented with TLR4/2 antigen resulted in a strong immune response in a murine model. Briefly, immunization of mice with this MSP2 vaccine formulation generated a protective antibody response against conserved C-terminal domains of MSP2 (133). Among MSPs, the MSP3 antigen has been reported as a highly immunogenic vaccine candidate which can induce protective immune responses. MSP3 vaccine formulations such as GMZ2 (a recombinant protein fusion of GLURP (Glutamate-rich protein) and MSP3) and MSP3-LSP (a combination of MSP3 and LSP1 (Long synthetic peptide)) are under phase II clinical trials (134, 135).
VLP (virus-like particles) based vaccination strategies are considered an efficacious vaccine delivery platform for multiple antigens. Three VLPs, MSP8, MSP9 and RAP1 (Rhoptry-associated protein) were complexed with influenza virus matrix protein. Mice were immunized with a mixture of these VLPs and challenged with P. berghei infection later (136). VLP vaccination induced protective CD4+ and CD8+ T-cell responses and alleviated TNF-α and IFN-γ levels in mice sera and spleen. VLP vaccination enhanced the mice survival rate and reduced the parasite burden in peripheral blood (136). Based on genetic diversity analysis, low genetic diversity and highly conserved sequences have been reported in P. vivax leading vaccine candidate antigen MSP119. It has been speculated that MSP119 could be used in multivalent vaccine formulations against P. vivax infection (137). Another candidate malaria vaccine antigen AMA1 (apical membrane antigen) is expressed on the merozoite cell surface. P. falciparum AMA1 shows a high level of genetic polymorphism. To reduce the genetic polymorphism, three diversity-covering (DiCo) protein sequences were designed. Administration of PfAMA1-DiCo along with Alhydrogel to malaria-exposed adults resulted in a significantly higher antibody response against DiCo variants (138). Although vaccine antigens from Plasmodium species have been used in generating a variety of vaccine formulations, there is no vaccine against P. knowlesi to date. In a recent study, using bioinformatic analysis, two potential immunogenic B-cell and T-cell epitopes of PfAMA1 protein were reported, which could be used in the development of multi-epitope-based vaccines against P. knowlesi infection (139). In a recent study, using a heterologous prime-boost immunization strategy, three vaccine formulations namely recombinant baculovirus, VLP and recombinant vaccinia virus, each of them expressing P. berghei AMA1 protein were prepared. The sequential administration of these vaccine formulations in a mice model induced protective IgG antibodies and CD4+ and CD8+ T-cell immune responses against P. berghei infection providing evidence for the implementation of AMA1-based vaccination approaches (140).
4.3. Sexual stage vaccine candidates
Some of the asexually replicating merozoites commit and differentiate into gametocytes which initiate the sexual stage of Plasmodium. Several parasite proteins are expressed exclusively by gametocytes and constitute targets for malaria transmission-blocking vaccines (TBVs) (141). These candidates elicit human antibodies that inhibit the development of Plasmodium in mosquitoes, thereby preventing its further transmission. There are several TBV antigens which includes Pfs230, Pfs230p, Pfs25, Pfs48/45, Pfs47, HAP2 and HAP2p, Pf77, and PfMDV-1 (141). Among TBV vaccine candidates, only two candidates: Pfs230 and Pfs25 have reached Phase 1/2 clinical trials. Pfs25 and Pfs230 are gametocyte surface proteins expressed by P. falciparum during the sexual stage. These proteins are essential for gamete fertility. Pfs25 is a female-specific protein while Pfs230 is expressed by both male and female gametocytes/gametes. Pfs230p is a paralog of Pfs230. Pf230p plays a crucial role in P. falciparum male fertility and zygote formation and can be investigated further as a TBV candidate (142). The administration of Exoprotein A (EPA) and Pfs25 conjugated vaccine in Alhydrogel®, was reported safe and immunogenic in Malian adults which induced significant serum activity after four doses. In a laboratory assay, serum activity was assessed in reducing parasite transmission to mosquitoes. However, transmission-blocking activity was not enough, and Pfs25-specific antibody titers declined rapidly with time (143). The effect of ALFQ, a liposomal adjuvant, on the immunogenicity of Pfs230D1-EPA and Pfs25-EPA was assessed in a Rhesus macaque model. Both vaccine conjugates generated strong antibody responses after two vaccinations. Although functional activity declined rapidly, a third vaccination of Pfs230D1-EPA induced functional activity which lasted for a few months (144).
In a recent clinical trial, a vaccine formulation was prepared by conjugating Pfs230 or Pfs25 antigens with EPA along with Alhydrogel. As compared to Pfs25, the Pfs230 vaccine induced a much greater complement-dependent transmission-blocking activity in humans (145). Furthermore, the limited polymorphism in P230 and conservation of sequence among Pf230 and Pv230 may aid in the development of a TBV vaccine against P. vivax (146). Pfs48/45, a cysteine-rich P. falciparum sexual stage surface protein is a leading clinical TBV candidate antigen (147). Pfs48/45 protein contains multiple disulfide bonds which are critical for its proper folding and induction of transmission-blocking antibodies. Pfs48/45 antigen is recognized by the most potent transmission-blocking monoclonal antibody. The functional conservation of P48/45 in P. berghei and P. vivax may provide an effective in vivo model to test P. vivax-based TBVs (148). However, clinical development of Pfs48/45 antigens as a vaccine candidate has been hindered, due to its poor biochemical characteristics. In a recent study, bioinformatics approaches has been used to design nanoparticle-based, stabilized Pfs48/45 vaccines which were then administered in mice model. These multimeric Pfs48/45-6C vaccines elicited antibodies that drive potent transmission-reducing activity (149). P. falciparum protein, P47 is a paralog of Pfs48/45. Pfs47 plays an important role in protecting ookinetes from mosquito’s immune system, Pfs47 could be a potential TBV candidate (93). The Hapless 2 (HAP2) family of proteins play a critical role in gamete fusion, and immunization with protein fragments of PfHAP2, PfHAP2p and PbHAP2 generated transmission-blocking activity (150, 151). Recombinant PbHAP2 protein administered in rabbits showed high immunogenicity by inducing HAP2-specific antibodies which inhibited in vitro ookinete formation and oocyst formation in Anopheles midgut (151). Targeting conserved fusion loops of HAP2 inhibits transmission of P. berghei and P. falciparum, which offers an opportunity for designing effective TBV vaccines (152). Other TBV vaccine candidates, such as Pf77 and male development gene 1 (PfMDV-1) induce antibodies which show transmission-reducing activity against Plasmodium. Both Pf77 and PfMDV-1 display less antigenic polymorphism and are known to induce naturally occurring antibodies in individuals living in endemic areas of Africa. These antigens are highly immunogenic and can induce transmission-reducing antibodies which may aid in the reduction of oocyst counts in Anopheles mosquito midgut (153).
4.4. Mosquito stage vaccine candidates
Inside the mosquito, Plasmodium ookinetes invade the midgut epithelium of mosquito host to transform into oocysts. During this stage, ookinetes encounter multiple barriers such as extracellular matrix (ECM) and innate immune responses of the mosquito midgut. There are some protein antigens such as PM4 (aspartic protease plasmepsin 4) and CHT1/CHT2 (chitinase) which may prove to be transmission-blocking targets of Plasmodium ookinete. Antibodies against both PM4 and CHT1 block the passage of ookinetes through ECM, thereby reducing oocyst counts and infectivity of malaria (154, 155). Further, P. berghei ookinete surface proteins such as P25 and P28 contribute to midgut invasion. Antibodies targeting proteins P25 and P28 have been shown to affect oocyst formation (156).
The most potent TBV antigens Pfs25 and Pfs28 are expressed on the surface of ookinetes (157). Both Pfs28 and Pfs25 have limited antigen diversity, are immunogenic and show structural similarities. It has been reported that Pfs28-specific antibodies can block P. falciparum transmission and also show synergism in blocking transmission when combined with Pfs25-specific antibodies. Therefore, Pfs28 and Pfs25 may prove to be effective TBV (158). A Pfs25-EPA-based TBV vaccine formulated with alum has been tested in adults in a phase I trial in the USA recently. Although the vaccine was safe and well-tolerated, the functional activity of the anti-Pfs25 antibodies was less and reduced rapidly (159). Furthermore, P. vivax TBV antigens, Pvs25 and Pvs28 have been reported to induce anti-parasite response and antibodies generated against Pvs25 and Pvs28 were able to completely block the P. vivax infection in mosquitoes (160). Another class of P. berghei-secreted ookinete proteins, PbPSOP7, PbPSOP25, and PbPSOP26 show transmission-blocking activity. Mice immunization with recombinant PbPSOP7, PbPSOP25, and PbPSOP26 proteins induced specific antibodies which recognized the ookinete surface, and mosquitoes fed on these immunized mice showed transmission-reducing activity (161). Vaccination of mice with recombinant P. falciparum cell-traversal protein for ookinetes and sporozoites, PfCelTOS (a P. falciparum TBV candidate) along with TLR-based adjuvant, elicited specific anti-PfCelTOS antibody-mediated immune response, which has been shown to induce transmission-reducing activity in mosquito (162). Recently, a mosquito midgut protein, namely anopheline alanyl aminopeptidase N 1 (AnAPN1), has been shown to induce potent transmission-blocking antibodies and may prove to be a potential TBV candidate (163). Moreover, Bender et al. designed a vaccine construct, UF6B, from AnAPN1 protein. The immunogenicity of UF6B was evaluated in mice, wherein mice were immunized with UF6B along with human safe adjuvant, GLA-LSQ. Vaccination with UF6b:GLA-LSQ induced humoral immune response against a potent transmission-blocking epitope indicating that UF6b vaccine construct could be a TBV candidate for malaria elimination (163). A list of various stage specific malaria vaccine candidates is presented in Table 2.
4.5. Current vaccine approaches
Despite the availability of multiple vaccine candidates, it has been difficult to develop a highly effective vaccine against Malaria, probably due to the high polymorphism associated with proposed vaccine candidates and their limited efficacy. Novel nanoparticle-based vaccination approaches seem promising due to their safety, biocompatibility, and efficacy in generating efficient anti-malaria vaccines (164). Recently, a trimethyl chitosan-based vaccine containing multiple malaria antigens from different developmental stages was prepared by using a layer-by-layer (LbL) antigen delivery platform. LbL NP vaccine administration in mice induced the highest T-cell response against PfCSP indicating that it could be a potent vaccine candidate against malaria (164). P. falciparum cysteine-rich protective antigen (CyRPA) is a merozoite surface antigen involved in RBC invasion. In one pre-clinical study, it was found that vaccine formulation containing CyRPA along with Alhydrogel elicit neutralizing antibody and anti-parasite cytokine response in mice. Therefore, it could be a potential vaccine candidate against blood stages of P. falciparum infection (165). Another powerful approach, insect cell culture coupled with baculovirus expression vector systems (IC BEVS), has been utilized for high-yield expression of recombinant PfCyRPA protein (166). The purified PfCyRPA protein was formulated with lipid-based virosome nanoparticles and used for the immunization of rabbits. Immunization resulted in the production of anti-PfCyRPA specific antibodies which inhibited the multiplication of P. falciparum in vitro (166).
Due to HLA polymorphism in human populations, it has been difficult to generate highly efficacious vaccines against malaria. Epitope-based vaccination approaches are more promising due to the selection of epitope regions present on antigenic molecules which may further enhance the vaccine efficacy. In one study, the VLP-based approach was used to prepare an epitope-based vaccine against the blood stage of malaria. P. falciparum CSP protein contains a highly vulnerable L9 epitope at N-terminus central repeat region. L9 VLP vaccination confers antibody-mediated protection against the blood stage malaria in mice (167)). Another in silico immunoinformatics-based study was conducted to predict T-cell and B-cell epitopes in P. vivax PPPK-DHPS and DHFR-TS proteins (168). Since the number of predicted promiscuous epitopes in selected proteins was higher, these predicted epitopes could be considered major vaccine targets against P. vivax malaria and may aid in the development of effective vaccines (168). A multi-epitope vaccine was designed against the blood stage of P. falciparum by selecting multiple epitopes of P. falciparum glutamic acid-rich protein (PfGARP) protein. A total of 10 epitopes (5 B and 5 HTL epitopes) were linked by suitable linkers along with flagellin adjuvant to enhance the immunogenicity of the vaccine construct (169). While in silico immune simulation resulted in an elevated humoral and cellular immune response against malaria, such in silico studies need further in vitro and in vivo evaluations (169).
Multistage chimeric vaccine-based approaches against malaria have gained attention due to their enhanced efficacy. A vaccine candidate GMZ2.6c has been designed by genetically fusion of Pfs48/45-6C protein with GMZ2 (a fusion protein of GLURP and MSP-3). GMZ2.6c vaccine efficacy can be enhanced by using TLR4 agonists which have been reported to induce parasite-specific antibodies and T-cell-mediated immunity in mice models (170). Recently, one study reported that the GMZ2.6c vaccine is recognized by naturally acquired antibodies in individuals living in malaria-endemic regions of Brazil with different levels of transmission (171). Another chimeric multistage TBV, ProC6C was prepared by combining Pfs230-Pfs48/45 fusion protein with the PfCSP linker sequence. The ProC6C long with adjuvant Alhydrogel was administered in mice which elicited a strong antibody response which helped in reducing transmission to mosquitoes and limited sporozoites invasion to human hepatocytes (147). VAR2CSA is considered a potential vaccine candidate against placental malaria. P. falciparum VAR2CSA protein binds to chondroitin sulphate-A (CSA) present on the surface of the syncytiotrophoblast of the placenta (172, 173). Two vaccine formulations based on PfVAR2CSA, PAMVAC and PRIMVAC are currently in Phase I clinical trials. However, VAR2CSA shows a high level of antigenic polymorphism which is a major obstacle in the development of a vaccine against placental malaria (174).
Genetic manipulation of Plasmodium genes is a time-consuming process, therefore lyse-reseal erythrocytes for delivery (LyRED) of miRNA are more advanced, fast and effective methods for studying novel malaria vaccine antigens. The miRNA-based translational repression can be monitored within a few days. It can be used for the characterization and identification of malaria vaccine antigens from different developmental stages which may contribute to the development of effective subunit vaccines (175). P. vivax merozoites contain Duffy binding protein (PvDBP) which is involved in reticulocyte invasion via interaction with DARC (Duffy antigen receptor for chemokines) receptors present on host reticulocytes (176, 177). Although DBP shows high levels of polymorphism, the amino-terminal cysteine-rich region II found in PvDBP (PvDBPII) serves as an attractive target. However, the generation of a DBP-based vaccine is still a distant dream and further investigations are required to prove its efficacy against P. vivax malaria (178).
Chemoprophylaxis with P. falciparum sporozoites (CPS) is a whole sporozoite based vaccination approach. CPS immunization has been shown to induce sterile immunity in human volunteers against pre erythrocytic stage of P. falciparum (179). Combination of CPS with various anti-malaria drugs has been reported to improve the efficacy of such vaccines. For instance, a single dose piperaquine-tetraphosphate (PPQ) along with CPS resulted in expansion of hepatic and splenic memory CD8+ T-cells in rodent malaria model (180). The efficacy of CPS immunization has been assessed in a human liver-chimeric mice model. CPS immunization induced functional IgG antibodies against P. falciparum sporozoites. These functional antibodies interfered with host-parasite interaction and reduced the sporozoite traversal during liver stage (181). In experimental swiss mice, CPS immunization under chemoprophylactic cover of Artether, Mefloquine/Azithromycin, Lumifentarine, and halofantrine conferred strong and long-lasting protection against P. yoelli sporozoite infection (182–184). Another study identified the correlates of protection for CPS vaccination by transcriptome analysis of PBMCs from CPS immunized individuals. Various correlates of protection such as interferons, Toll-like receptor (TLR), NF-kB, and monocyte-related signatures were found associated with protection. Such transcriptional analysis of post-vaccination protection signatures may prove useful for assessing vaccine efficacy during clinical trials (185). While RTS/S/AS01E induce moderate protection in African children, CPS immunization induced 100% sterile protection in naive adults (107, 179). Overall, whole sporozoite based alternative vaccination approaches seem promising for the development of safe, effective, and potent anti-malaria vaccines.
5. Antibody-mediated therapy
Since Plasmodium parasites are increasingly becoming resistant to conventional anti-malarial drug-based therapy, novel antibody-based therapies can prove beneficial to prevent malaria. Antibody based therapy are highly effective and can be used in patients, non-responsive to conventional anti-malarial drug regimens (186). Studies have shown that passively transferred antibodies reduce parasitemia associated with Plasmodium (187–189). Multiple antibody effector functions are involved in immunity to malaria, which includes direct inhibition or neutralization, complement fixation and activation, and opsonic phagocytosis or cellular cytotoxicity by immune cells through interactions with Fc-receptors (190, 191) (Figure 2). Protective immunity to malaria is mainly associated with IgG1 and IgG3 subclasses, with IgG2 and IgG4 being associated with a decrease in opsonization (192). Humoral immune responses attack different parasite stages, and antibody-based therapy may prevent malaria infection or transmission.
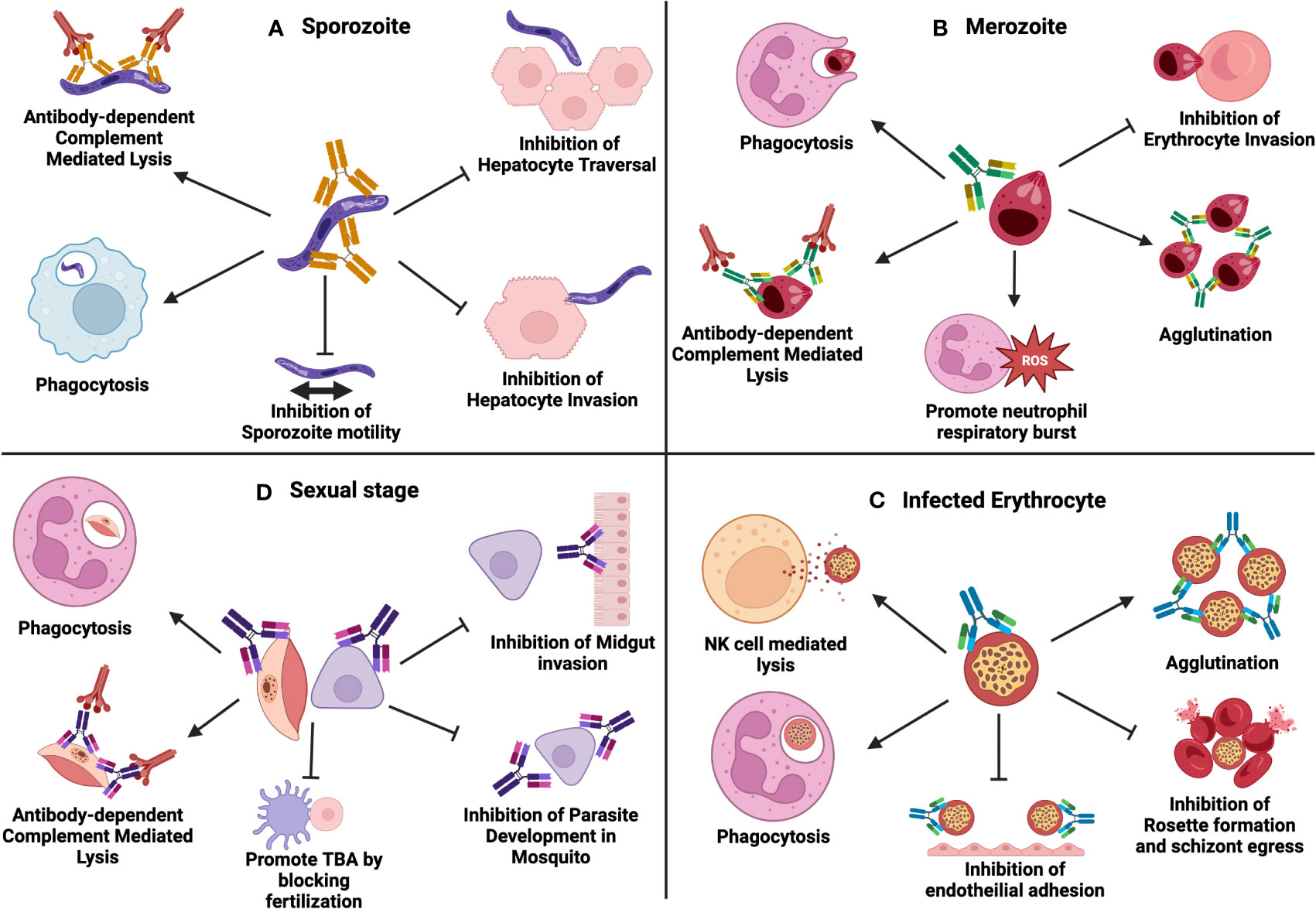
Figure 2 Multiple antibody effector functions involved in immunity to malaria: (A) Antibodies to sporozoites can function through phagocytosis, complement activation, inhibition of sporozoite motility, inhibition of hepatocyte traversal and inhibition of hepatocyte invasion. (B) Antibodies to merozoites can function through phagocytosis, complement activation, promoting neutrophil respiratory burst, agglutination and inhibition of erythrocyte invasion. (C) Antibodies against infected erythrocytes function through phagocytosis, NK-cell mediated antibody-dependent cellular cytotoxicity (ADCC), agglutination, inhibition of endothelial invasion, inhibition of rosette formation, and schizont egress. (D) Antibodies during parasite sexual stages function through phagocytosis, complement activation, promoting TBA by blocking fertilization, inhibition of midgut invasion and inhibition of parasite development in the mosquito. Created with BioRender.com.
5.1. Antibodies to sporozoites
Blocking sporozoite motility, dermal exit, hepatocyte traversal, and eventual invasion of hepatocytes are only a few of the sporozoite-targeting strategies used by antibodies (4). Through the activation of the complement system, phagocytosis, and Fc-mediated innate cell activities, antibodies also assist in the killing of sporozoites (191). Some mechanisms such as in vitro parasite neutralisation and in vivo protection are employed by monoclonal antibodies against the PfCSP (193). Also, monoclonal antibodies against the repeat region have been shown to inhibit sporozoites (194). The testing of the CSP-based RTS,S vaccine provides the strongest support for the idea that anti-CSP antibodies can protect against malaria (195). RTS,S is a VLP consisting of a central tandem repeat of 19 NANP repeats (R) and C terminal domain of the CSP (containing T-cell epitopes) fused to the Hepatitis B Surface antigen (S). The ‘RTS’ fusion protein and free ‘S’ protein spontaneously assemble in ‘RTS,S’ particles. A formulation of RTS,S is undergoing Phase III clinical studies using AS01, a unique adjuvant made up of a combination of liposomes, saponin and monophosphoryl lipid A (196). Studies with RTS,S vaccine showed that antibodies can mediate sterilizing immunity, and antibodies against the sporozoite can be efficient mediators of protection against pre-erythrocytic stage malaria (197). Few human monoclonal antibodies isolated from naturally infected individuals or individuals vaccinated with RTS,S, PfSPZ Vaccine, or PfSPZ-CVac can inhibit sporozoite invasion in animal models (27, 198, 199). In animal models, several anti-PfCSP monoclonal antibodies have been shown to be protective. Monoclonal antibodies (MAL1C, MAL2A, and MAL3B) isolated from an RTS, S-immunized individuals, imparted sterilizing immunity (197, 200). Another PfCSP monoclonal antibody (2A10), isolated from the whole sporozoite immunized mice (201), was protective in vectored prophylaxis and passive infusion studies (202). Furthermore, passive transfer of a P. yoelii CSP monoclonal antibody (2F6) showed inhibition of liver infection when mice were challenged with sporozoites (203). Moreover, in a recent human clinical trial (Phase I) with malaria-naive volunteers, 40 mg/kg of an anti-malaria monoclonal antibody known as “CIS43LS” (directed against PfCSP), was intravenously administered to patients which protected against controlled malaria challenge (204, 205). A phase I clinical trial of another CSP-specific monoclonal antibody (L9LS) was recently conducted by Wu et al. and intravenous or subcutaneous administration of L9LS, protected the recipients against malaria after controlled infection (198, 206). In addition to CSP, monoclonal antibodies against TRAP, also known as sporozoite surface protein 2 or SSP2) have been shown to prevent parasite infection of hepatocytes in both in vitro and in vivo models (207). Although people who have higher levels of antibodies against sporozoite antigens are better protected against infection, studies on the malaria vaccine have generally had unsatisfactory results using antibody titers as correlates of protection (208). The limited effectiveness of RTS,S in areas where malaria is endemic, indicates that the functioning and avidity of the antibodies, rather than the antibody titers, are better correlates of immunological protection against malaria (209, 210).
5.2. Antibodies to merozoites
It has been demonstrated that antibodies against several merozoite antigens function through neutralization (211). Antibodies can inhibit the invasion of red blood cells (RBCs) through binding to merozoite antigens and can inhibit P. falciparum growth and multiplication in vitro (212). Antibodies can bind to merozoite surface and cause merozoite agglutination, destruction of merozoites by complement-mediated damage, phagocytosis, antibody-dependent cellular cytotoxicity, and antibody-dependent respiratory burst by neutrophils (213). Merozoite surface antigens like Plasmodium reticulocyte-binding homologues (PfRH) and erythrocyte-binding antigens (EBA) are also targets of antibody response (214, 215). Anti-PfRH5 antibodies are highly effective at preventing P. falciparum merozoites from invading erythrocytes (216). Recombinant monoclonal antibodies against both PfRh5 and PfCyRPA have been shown to block invasion (217). Interestingly, both non-neutralizing and neutralizing monoclonal antibodies against PfRh5 can synergize to reduce parasite invasion of RBCs (216). Anti-EBA-175 monoclonal antibodies (R217 and R218) have been described as inhibitory for P. falciparum invasion in RBCs (218). Human monoclonal antibodies against various merozoite antigens have been isolated (PfMSP1, PfMSP2, PfMSP3, PfRH5, PfAMA1), and some of these antibodies were seen to exhibit anti-parasitic activity in vitro (219). Monoclonal antibodies to MSP1 paralog in P. vivax (PvMSP1P) can also reduce parasite invasion (220). Anti-MSP3 antibodies were shown to have anti-malaria activity via antibody-dependent cellular suppression of P. falciparum (221). The DBP is a vital ligand for P. vivax blood-stage merozoite invasion and monoclonal antibodies against DBP inhibited parasite binding to RBCs (222). Human monoclonal antibodies (053054 and 092096) have been shown to neutralize P. vivax in ex vivo experiments (223). Monoclonal antibodies to P. vivax reticulocyte binding protein 2b (PvRbp2) can inhibit parasite invasion into reticulocytes (224). An antibody against AMA1 exhibits significant inhibitory activity against different Plasmodium strains, providing a basis for its therapeutic application (225). Rhoptry (apical organelles involved in erythrocyte invasion) proteins participate in the invasion of red blood cells by merozoites and monoclonal antibodies specific to RAP1 inhibit P. falciparum growth in vitro (226). A monoclonal antibody (RAM1.25) developed against rhoptry‐associated membrane antigen (PfRAMA) exhibited both the growth inhibitory and neutralizing activity against the Plasmodium parasite (227).
5.3. Antibodies to iRBCs
The role of antibodies to Plasmodium parasite-infected erythrocyte surface antigens (including PfEMP1) in naturally acquired immunity to malaria is still unclear (228). Antibodies targeting VSAs such as PfEMP1, RIFINs and STEVORs proteins expressed during the infected erythrocyte stage are key components of natural immunity to malaria (40) The antibodies against VSAs work by preventing the parasite’s attempts to evade the immune system (229). Antibodies attaching to the surface of the iRBCs can promote phagocytosis and agglutination of iRBCs. Further, antibodies directed against iRBCs can inhibit rosette formation, or schizont outflow and adhesion of the iRBCs to endothelium and epithelium (sequestration) (4). PfEMP1 expressed on the surface of iRBCs is a major target of protective antibodies in malaria (230) and it has been hypothesized that repeated infections are required to elicit a protective repertoire of PfEMP1-specific antibodies (231, 232). Additionally, in pregnancy-associated malaria, antibodies against VAR2CSA (a variant of PfEMP1, which binds to CSA in the placenta) have been linked to protection against malaria (233, 234). The binding of PfEMP1 to CSA receptors allows the sequestration of iRBCs in the placenta and VAR2CSA antibodies function mainly by inhibiting parasite adhesion to RBCs and sequestration along with other effector mechanisms (235). In addition, monoclonal antibodies against PfEMP1 inhibited the formation of rosettes (236). Interestingly, a new class of receptor-based monoclonal antibodies generated by the insertion of a host receptor (collagen-binding inhibitory receptor, LAIR1) into an antibody gene have been shown to agglutinate iRBCs and opsonize them for phagocytosis by monocytes, thereby aiding parasite clearance (237). Monoclonal antibodies to Plasmodium schizont egress antigen-1 (PfSEA-1) (expressed in schizont-infected red blood cells), decreased parasite replication by arresting schizont rupture, and maternal antibodies to PfSEA-1 protected infants from severe malaria (238, 239).
5.4. Antibodies to gametocytes
Antibodies against gametocytes can affect the maturation and sequestration of early gametocytes and circulating gametocytes respectively. Additionally, antibodies target gametes that develop in the midgut of mosquitoes (240). Antibodies targeting gametocyte antigens Pfs230 and Pfs48/45 can show transmission-blocking activity (TBA) by inducing complement-mediated lysis or promoting phagocytosis (240–242). A humanized monoclonal antibody (TB31F) against Pfs48/45 which binds to gametocytes and inhibits fertilization. TB31F was capable of completely blocking the transmission of P. falciparum parasites from humans to mosquitoes in a phase 1 clinical trial (243). Antibodies to macrogametes and/or zygotes can inhibit parasite development within the mosquito (244, 245). Antibodies to female gamete antigen Pfs47 also have TBA and may function by inhibiting ookinete development and fertilization (246). Neutralizing antibodies to Pfs25, a zygote antigen, can reduce transmission independently of complement (247). Recently, it has been reported that monoclonal antibodies generated against Anopheles gambiae mosquito saliva protein TRIO (AgTRIO) markedly reduced early Plasmodium infection in a murine model (248). Human monoclonal antibodies to Pfs25, (a gametocyte antigen) can block malaria transmission. Membrane-associated erythrocytic binding protein (MAEBL) is expressed in the liver stages. It is required for sporozoite infection of mosquito salivary glands and antibodies against MAEBL partially inhibit hepatocyte invasion by sporozoites and/or liver-stage development (249, 250). Monoclonal antibodies to Plasmodium protein CelTOS strongly inhibited the oocyst development of P. falciparum in mosquitoes and neutralized sporozoite hepatocyte infection in vivo (251).
Neutralizing monoclonal antibodies raised against the GPI toxin of P. falciparum can inhibit the induction of TNF-α. They can also modify the clinical course of infection in animal models of severe disease (252). During P. yoelii infection, treatment of mice with anti-IL-10 monoclonal antibodies resulted in substantial prolongation of survival, whereas treatment of mice with anti-IFN-γ monoclonal antibodies exacerbated infection (253). Exported protein 1 (EXP-1) found in the parasitophorous vacuolar membrane seen during the liver and blood stage, contains a defined epitope. This defined epitope is recognized by a parasite inhibitory monoclonal antibody (8E7/55) (254). The GLURP is an exoantigen expressed in all stages of the P. falciparum life cycle in humans. It is a target for antibody-dependent monocyte-mediated inhibition of parasite growth, and affinity-purified human IgG antibodies to GLURP can promote a strong ADCI effect in vitro (255). A monoclonal antibody directed against EWGWS epitope of Enolase (PfEno) was found to slow blood-stage malarial parasite growth. It may protect against dual-stage, species and strain-transcending malaria (256). Monoclonal antibodies against pre-erythrocytic stage antigens and erythrocytic stage antigens are currently being explored for therapeutic use. Notably, monoclonal antibodies targeting the sexual stage antigens in mosquitoes can abrogate transmission. Although gametocyte antibodies are largely responsible for reducing malaria transmission, it has been hypothesized that some antibodies can mediate antibody enhancement of malaria transmission (257). While the protective nature of Plasmodium-specific antibodies has been demonstrated in multiple studies, few reports have also identified non-protective antibodies (258, 259). Furthermore, the identification of protective antibody epitopes can be useful in developing antibody-guided vaccine designs against malaria. Recently, Murugan et al. identified a conserved core epitope by characterizing 200 human monoclonal PfCSP antibodies induced by sporozoite immunization. This epitope-based approach can be used for rational designing of a next-generation PfCSP vaccine, which can elicit high-affinity antibody responses (260).
6. Host-directed therapies
Host-directed therapy can be implemented during multiple stages of malaria infection by targeting host cell functions which are required for parasite survival and proliferation. Host-directed therapy does not put selection pressure on Plasmodium which prevents the selection of specific genetic variants involved in conferring drug resistance. Therefore, by targeting specific host molecules, the problem of anti-malaria drug resistance can be resolved.
6.1. Host-directed therapy against liver stage
During liver stage infection, the host-directed therapy may prove crucial, as blocking malaria infection during the early liver stage could prevent the progression of sporozoites to merozoites. After invading hepatocytes, P. vivax sporozoites transform either into schizonts or hypnozoites. Schizonts are dividing forms while hypnozoites are non-diving or dormant forms. The size of hypnozoites increases slightly with time and are considered to be metabolically active forms (261). Currently, no biomarkers are available to detect hypnozoite infection in humans which makes its early diagnosis challenging. However, one study reported that hypnozoites-infected liver-chimeric humanized mice hepatocytes secrete parasite protein-loaded exosomes in plasma indicating the presence of P. vivax infection (262). It has been suggested that the elimination of even a small fraction of hypnozoites could prove to be beneficial for tackling the increasing incidence of relapsing malaria (263). Currently, there are very few approved drugs such as primaquine and tafenoquine which acts against hypnozoites. However, these drugs are associated with complications in glucose-6-phosphate dehydrogenase (G6PD)-deficient individuals (264, 265).
The host factor CD68 is thought to facilitate the traversal of sporozoites through liver-resident KCs making it an attractive target for host-based therapy (18). In one study, it has been reported that monensin, an antibiotic conferred protection against sporozoite infection in a mouse model. Monensin renders host cells resistant to sporozoite infection by inhibiting sporozoite invasion to hepatocytes (266). Recent studies have revealed that a series of host cell endocytic vesicles are sequestered towards intracellular sporozoites which aid in their development during the late liver stage (267). Various host cell proteins, such as COPB2 (coatomer protein complex subunit beta 2), COPG1 (coatomer protein complex subunit gamma 1) or the adaptor protein GGA1 (Golgi associated, gamma adaptin ear containing, ARF-binding protein 1), are involved in trafficking of vesicles toward intracellular parasite (268). Targeting these cellular proteins can impair parasite development in hepatocytes. It has been shown that targeting aquaporin-3 (AQP3), which is a water channel protein contributing to the development of Plasmodium during multiple stages of its life cycle, can lead to successful impairment of P. vivax liver stage (269, 270). Therefore, the development of AQP3 inhibitors may have an anti-hypnozoite effect which may decrease the prevalence of relapsing malaria. Furthermore, p53, a tumour repressor gene is involved in altering lipid peroxidation in the hepatocytes which negatively impacts the liver stage development (271). Upregulating the levels of p53 leads to a dramatically reduced number of liver-stage parasites (272). Plasmodium-infected hepatocytes are thought to be more susceptible to mitochondria-initiated apoptosis. Treatment with a chemical inhibitor which inhibits B-cell lymphoma 2 (Bcl-2) family proteins can result in enhanced apoptosis of infected hepatocytes (272). Another protein of the Bcl-2 family, BCL-xL contribute to P. falciparum development in iRBCs. BCL-xL inhibitors impaired parasite growth in vitro and induced apoptosis of iRBCs (273). Furthermore, various cellular inhibitor of apoptosis proteins (cIAPs) gets upregulated during Plasmodium infection. Liver stage malaria parasite can be controlled by the inactivation of cIAPs which results in TNF-mediated apoptosis of infected hepatocytes (274).
6.2. Host-directed therapy against erythrocytic stage
During blood-stage infection, erythrocyte receptors such as basigin (BSG) and CD55 facilitate merozoite invasion to erythrocytes (275, 276). These erythrocytic receptors could prove to be potential therapeutic targets. Moreover, merozoites can also invade erythrocytes via cell surface receptor, ICAM-4 (intercellular adhesion molecule-4) (277). Treatment with ICAM-4 inhibitors could be used to block the entry of merozoites to erythrocytes. Furthermore, several host protein kinases are involved in blood stage development and targeting these kinases via kinase inhibitors could prove to be an essential approach against malaria. During blood-stage infection when merozoites invade erythrocytes various protein kinase gets activated (278). Blood stage infection has been shown to activate downstream cell signalling pathways which involve activation of PAK-MEK kinase in host erythrocytes. Although protein kinase inhibitors such as U0126, a MEK1 (MAP/ERK kinase-1) inhibitor, are candidates for host-directed therapy against parasite proliferation in erythrocytes (279), MEK1 inhibitors are associated with cell toxicity. Therefore, further research is required in the development of strategies for reducing toxicity. In erythrocytes, ferrochelatase is an enzyme involved in heme biosynthesis. Ferrochelatase inhibitors have been shown to restrict Plasmodium growth inside healthy human erythrocytes in vitro (280). Therefore, desferrioxamine, an inhibitor of ferrochelatase could be used in targeted therapy against malaria. Human erythrocytes contain Peroxiredoxin-2 (Prx2), a thiol-dependant peroxidase which protects the erythrocytic cells from the oxidative environment encountered by erythrocytes during malaria infection (281). Plasmodium utilizes these peroxidases for haemoglobin digestion which contributes to its development inside erythrocytes. Treatment with Prx2 inhibitor, Conoidin A renders erythrocytes resistant to P. falciparum infection (281). One recent study has shown that selective inhibition of the glycolysis process in iRBCs by Enolase inhibitors (HEX and DeoxySF-2312) could be a novel host-directed therapy against malaria (282).
6.3. Host-directed therapy against cerebral malaria
Aptamers are ss-oligonucleotides (ssDNA or RNA) which can recognize, bind and alter the activity of targeted molecules. It has been suggested that aptamers targeted against host cell-matrix receptors could be used in blocking the interactions between parasites and host cells (283). A combination therapy containing antimalarial drugs and host-directed anti-inflammatory innate defence regulator peptides (IDR-1018) increased the survival rates of malaria-infected mice. Therefore, IDRs along with antimalarial drugs could be a promising adjunctive host-directed therapy against severe malaria (284). Currently, artemisinin is the drug of choice for cerebral malaria and the development of host-directed therapy is underway. In one study, it has been shown that inhaled form of NO (nitric oxide) along with its derivative can be used as an adjunctive treatment against cerebral malaria (285). PfGPI-induced host inflammatory responses play an important role in the pathogenesis of severe cerebral malaria. PfGPI stimulates host macrophages and induces TNF-α secretion via activating MAPK pathways, including JNK2. Therefore, treatment with JNK2 inhibitors can decrease TNF-α secretion, thereby reducing inflammation in mice models of cerebral malaria (286). Interestingly, treatment of infected mice with NRG1 (neuregulin1), a neuronal growth factor, reduced tissue damage during experimental cerebral malaria (287). The brain microvascular endothelium plays a major role in the pathogenesis of cerebral malaria and molecules, modulators/inhibitors targeting its regulatory pathways are promising candidates in the treatment of cerebral malaria. Repurposing of current therapeutics which modulate the endothelium of the blood-brain barrier such as S1P modulators (neurologic disease) and VEGFR2 tyrosine kinase inhibitors (cancer) could confer neuroprotective activity against cerebral malaria (288).
Furthermore, AQP3 is required by both P. vivax and P. falciparum for their development during blood stages, therefore, AQP3 inhibitors may contribute to pan anti-malaria activity (289, 290). Although little is known about the role of host-directed therapy for gametocyte stages, host-targeted therapy that reduces gametocyte development and differentiation into male and female gametes could limit their transmission (289, 290). An added advantage of host-directed therapy is that it could be employed against host cellular pathways involved in the production of erythrocytic components that are scavenged by the parasites for their development. These therapies would act by depriving the parasite of these essential components. Since host-directed therapies control host pathways and the parasite has no genetic control over the host proteins, therefore it is less likely that the parasite would develop resistance against these therapeutics (291).
7. Conclusion and future directions
The parasite immune evasion strategies contribute to parasite survival and are considered a big obstacle in developing effective therapeutics against malaria. Research gaps yet remain in our understanding of host-parasite interactions and insights into these mechanisms are of utmost importance for developing effective vaccines and immunotherapies that can overcome immune evasion mechanisms and induce long-lasting immunity against malaria.
RTS,S is the most promising anti-malaria vaccine to date which has completed phase III clinical trials. However, its limited efficacy and geographically regional effect have been seen in many studies. Compared to RTS,S and other subunit vaccines, the whole sporozoites-based vaccine has had more success (292). Despite significant progress in whole sporozoite-based vaccines, the lack of an effective system for in vitro production of P. falciparum sporozoites warrants more research for malaria vaccine development. Moreover, most of the vaccination studies are based on P. falciparum and vaccine research on the second most malaria-causing strain P. vivax is lagging far behind. Furthermore, research on P. vivax candidates is limited due to difficulties associated with in vitro continuous culturing of P. vivax and only a few P. vivax vaccine candidates have reached to clinical development stages. Therefore, there is an emergent need to expand the repository of P. vivax vaccine candidates for demonstrating heterologous protection (293). Although a variety of anti-malaria vaccine candidates have been identified, associated limitations such as poor immunogenicity with limited efficacy impede their success. Additional research is needed for the development of an effective and safe vaccine which can generate long-lasting and strain-transcending immunity in people of all age groups. Using novel immunoinformatics and/or in silico-based approaches, a combination of different vaccine antigens from multiple stages of the Plasmodium life cycle may prove beneficial for developing a multi-antigen or multi-stage vaccine against malaria. Additionally, improving vaccine protection utilizing a staggered, segmented dosage regimen and other alternative adjuvants along with novel delivery systems must be explored. Development of a variety of vaccine platforms including VLP-based, multi-stage chimeric, GAPs, LARC GAP, mRNA vector-based, CPS-based and nanoparticle-based vaccines along with immunogenic adjuvants that can elicit robust immune responses are currently underway (Figure 1). To improve the affinity and longevity of vaccine-induced protective antibodies, novel target epitopes should be identified which can induce long-lived protective humoral responses. Vaccine strategies should not only include optimized antibody epitopes, but T-cell epitopes as well for mediating effective Th1 and Th2 responses. More efforts are needed to develop and refine existing animal models for investigating protection mechanisms. Determining immune correlates of protection will accelerate the development of an efficacious malaria vaccine in future.
Since humoral immunity contributes to immune defence mechanisms against malaria, antibody-based therapeutics may prove beneficial in the prevention or treatment of malaria. Monoclonal antibody-based therapy is of particular interest in containment and/or outbreak zones where active malaria transmission is confined to a particular area, season and travellers. Development of human monoclonal antibodies against key vaccine targets of P. falciparum and P. vivax helps to identify conserved epitopes that aid in vaccine development. Such approaches can also be extended to various stage-specific antigens of Plasmodium. Combination therapy with bispecific antibodies or cocktails of antibodies can be prepared that can target different antigens of parasite stages. Various antibody effector functions such as promoting neutrophil burst, NK cell-mediated killing, phagocytosis, agglutination, schizont egress inhibition, rosette inhibition, complement fixation, antibody-dependent complement-mediated lysis, inhibition of adhesion of infected erythrocyte and inhibition of merozoite invasion, have been investigated in various studies (Figure 2). The roles of variation in immunoglobulin allotype, antibody glycosylation and Fc sequence have been less explored. Further studies may help in understanding the immune responses which are necessary for protection from malaria.
Host-directed therapy, which is another major area of therapeutics, has emerged recently. Malaria parasite relies on a network of host pathways which contribute to its development. A wide variety of host factors that are involved in the development of the parasite present novel opportunities for host-directed therapies in malaria. Host-directed therapy particularly targets the host factors which makes parasites deprived of these essential factors needed for parasite invasion, multiplication, and survival inside host cells. Host-directed therapy acts synergistically with anti-malarial drugs and could also be used as novel adjunctive therapy in treating malaria. Furthermore, combining antimalarial drugs with vaccine/s or antibodies and using them as an adjunct therapy show potential to reduce the prevalence and transmission of malaria. It is expected that these combined approaches including antibody-based therapy, host-directed therapy and the development of novel and efficacious vaccines can contribute to the current goal of WHO for a malaria-free world. In summary, a thorough comprehension of the equilibrium existing between the host immune system and parasite immune evasion mechanisms is extremely important for the development of efficient immunological therapeutics.
Author contributions
PC: original draft preparation, reference collection, and manuscript writing; RR: manuscript editing; SK: manuscript editing; SR: conceptualization, supervision, manuscript editing, and proofreading. All authors contributed to the article and approved the submitted version.
Funding
The authors acknowledge the ICMR-JRF Fellowship grant to PC.
Acknowledgments
The authors acknowledge Mohd. Faraz Saifi for assistance in reference collection and all the facilities at IIT-Roorkee.
Conflict of interest
The authors declare that the research was conducted in the absence of any commercial or financial relationships that could be construed as a potential conflict of interest.
Publisher’s note
All claims expressed in this article are solely those of the authors and do not necessarily represent those of their affiliated organizations, or those of the publisher, the editors and the reviewers. Any product that may be evaluated in this article, or claim that may be made by its manufacturer, is not guaranteed or endorsed by the publisher.
References
1. Cowman AF, Healer J, Marapana D, Marsh K. Malaria: Biology and disease. Cell (2016) 167:610–24. doi: 10.1016/j.cell.2016.07.055
2. Sato S. Plasmodium–a brief introduction to the parasites causing human malaria and their basic biology. J Physiol Anthropol (2021) 40:1–3. doi: 10.1186/s40101-020-00251-9
3. Gomes PS, Bhardwaj J, Rivera-Correa J, Freire-De-Lima CG, Morrot A. Immune escape strategies of malaria parasites. Front Microbiol (2016) 7:1617. doi: 10.3389/fmicb.2016.01617
4. Aitken HE, Mahanty S, Rogerson JS. Antibody effector functions in malaria and other parasitic diseases: A few needles and many haystacks. Immunol Cell Biol (2020) 98:264–75. doi: 10.1111/imcb.12320
5. Global Malaria Programme, WHO, World Malaria Report. (2021). Available at: https://www.who.int/teams/global-malaria-programme/reports/world-malaria-report-2021.
6. Shears MJ, Sekhar Nirujogi R, Swearingen KE, Renuse S, Mishra S, Jaipal Reddy P, et al. Proteomic analysis of plasmodium merosomes: The link between liver and blood stages in malaria. J Proteome Res (2019) 18:3404–18. doi: 10.1021/acs.jproteome.9b00324
7. Venugopal K, Hentzschel F, Valkiūnas G, Marti M. Plasmodium asexual growth and sexual development in the haematopoietic niche of the host. Nat Rev Microbiol (2020) 18:177–89. doi: 10.1038/s41579-019-0306-2
8. Martins AC, Cayotopa AD, Klein WW, Schlosser AR, Silva AF, Souza MN, et al. Side effects of chloroquine and primaquine and symptom reduction in malaria endemic area (Mâncio Lima, acre, Brazil). Interdiscip Perspect Infect Dis (2015) 2015:346853. doi: 10.1155/2015/346853
9. Chaudhry HE, Khan S, Jamil S, Shaik TA, Ullah SE, Bseiso A, et al. Chloroquine-induced psychosis: A case report. Cureus. (2022) 14:e30498. doi: 10.7759/cureus.30498
10. Real E, Howick VM, Dahalan FA, Witmer K, Cudini J, Andradi-Brown C, et al. A single-cell atlas of plasmodium falciparum transmission through the mosquito. Nat Commun (2021) 12:1–3. doi: 10.1038/s41467-021-23434-z
11. Rénia L, Goh YS. Malaria parasites: the great escape. Front Immunol (2016) 7:463. doi: 10.3389/fimmu.2016.00463
12. Patarroyo ME, Alba MP, Curtidor H. Biological and structural characteristics of the binding peptides from the sporozoite proteins essential for cell traversal (SPECT)-1 and-2. Peptides (2011) 32:154–60. doi: 10.1016/j.peptides.2010.09.026
13. Müller HM, Reckmann I, Hollingdale MR, Bujard H, Robson KJ, Crisanti A. Thrombospondin related anonymous protein (TRAP) of plasmodium falciparum binds specifically to sulfated glycoconjugates and to HepG2 hepatoma cells suggesting a role for this molecule in sporozoite invasion of hepatocytes. EMBO J (1993) 12:2881–9. doi: 10.1002/j.1460-2075.1993.tb05950.x
14. Waisberg M, Molina-Cruz A, Mizurini DM, Gera N, Sousa BC, Ma D, et al. Plasmodium falciparum infection induces expression of a mosquito salivary protein (Agaphelin) that targets neutrophil function and inhibits thrombosis without impairing hemostasis. PLos Pathog (2014) 10:e1004338. doi: 10.1371/journal.ppat.1004338
15. Roussilhon C, Bang G, Bastaert F, Solhonne B, Garcia-Verdugo I, Peronet R, et al. The antimicrobial molecule trappin-2/elafin has anti-parasitic properties and is protective in vivo in a murine model of cerebral malaria. Sci Rep (2017) 7:1–6. doi: 10.1038/srep42243
16. Stegmann KA, De Souza JB, Riley EM. IL-18-induced expression of high-affinity IL-2R on murine NK cells is essential for NK-cell IFN-γ production during murine plasmodium yoelii infection. Eur J Immunol (2015) 45:3431–40. doi: 10.1002/eji.201546018
17. Tavares J, Formaglio P, Thiberge S, Mordelet E, Van Rooijen N, Medvinsky A, et al. Role of host cell traversal by the malaria sporozoite during liver infection. J Exp Med (2013) 210:905–15. doi: 10.1084/jem.20121130
18. Cha SJ, Srinivasan P, Schindler CW, van Rooijen N, van Rooijen N, Stins M, et al. CD68 acts as a major gateway for malaria sporozoite liver infection. J Exp Med (2015) 212:1391–403. doi: 10.1084/jem.20110575
19. Ikarashi M, Nakashima H, Kinoshita M, Sato A, Nakashima M, Miyazaki H, et al. Distinct development and functions of resident and recruited liver kupffer cells/macrophages. J Leukoc Biol (2013) 94:1325–36. doi: 10.1189/jlb.0313144
20. Klotz C, Frevert U. Plasmodium yoelii sporozoites modulate cytokine profile and induce apoptosis in murine kupffer cells. Int J Parasitol (2008) 38:1639–50. doi: 10.1016/j.ijpara.2008.05.018
21. Zheng H, Tan Z, Xu W. Immune evasion strategies of pre-erythrocytic malaria parasites. Mediators Inflammation (2014) 2014:362605. doi: 10.1155/2014/362605
22. Bertolino P, Bowen DG. Malaria and the liver: Immunological hide-and seek or subversion of immunity from within? Front Microbiol (2015) 6:41. doi: 10.3389/fmicb.2015.00041
23. Steers N, Schwenk R, Bacon DJ, Berenzon D, Williams J, Krzych U. The immune status of kupffer cells profoundly influences their responses to infectious plasmodium berghei sporozoites. Eur J Immunol (2005) 35:2335–46. doi: 10.1002/eji.200425680
24. Sun T, Holowka T, Song Y, Zierow S, Leng L, Chen Y, et al. A plasmodium encoded cytokine suppresses T-cell immunity during malaria. Proc Natl Acad Sci USA (2012) 109:E2117–26. doi: 10.1073/pnas.1206573109
25. Baeza Garcia A, Siu E, Sun T, Exler V, Brito L, Hekele A, et al. Neutralization of the plasmodium-encoded MIF ortholog confers protective immunity against malaria infection. Nat Commun (2018) 9:2714. doi: 10.1038/s41467-018-05041-7
26. Casares S, Richie TL. Immune evasion by malaria parasites: A challenge for vaccine development. Curr Opin Immunol (2009) 21:321–30. doi: 10.1016/j.coi.2009.05.015
27. Livingstone MC, Bitzer AA, Giri A, Luo K, Sankhala RS, Choe M, et al. In vitro and in vivo inhibition of malaria parasite infection by monoclonal antibodies against plasmodium falciparum circumsporozoite protein (CSP). Sci Rep (2021) 11:1–5. doi: 10.1038/s41598-021-84622-x
28. Ding Y, Huang X, Liu T, Fu Y, Tan Z, Zheng H, et al. The plasmodium circumsporozoite protein, a novel NF-κB inhibitor, suppresses the growth of SW480. Pathol Oncol Res (2012) 18:895–902. doi: 10.1007/s12253-012-9519-7
29. Pamplona A, Ferreira A, Balla J, Jeney V, Balla G, Epiphanio S, et al. Heme oxygenase-1 and carbon monoxide suppress the pathogenesis of experimental cerebral malaria. Nat Med (2007) 13:703–10. doi: 10.1038/nm1586
30. Hanson KK, Ressurreição AS, Buchholz K, Prudêncio M, Herman-Ornelas JD, Rebelo M, et al. Torins are potent antimalarials that block replenishment of plasmodium liver stage parasitophorous vacuole membrane proteins. Proc Natl Acad Sci (2013) 110:E2838–47. doi: 10.1073/pnas.1306097110
31. Thieleke-Matos C, Lopes da Silva M, Cabrita-Santos L, Portal MD, Rodrigues IP, Zuzarte-Luis V, et al. Host cell autophagy contributes to plasmodium liver development. Cell Microbiol (2016) 18:437–50. doi: 10.1111/cmi.12524
32. M'Bana V, Lahree A, Marques S, Slavic K, Mota MM. Plasmodium parasitophorous vacuole membrane-resident protein UIS4 manipulates host cell actin to avoid parasite elimination. iScience (2022) 25:104281. doi: 10.1016/j.isci.2022.104281
33. Garg S, Agarwal S, Kumar S, Yazdani SS, Chitnis CE, Singh S. Calcium-dependent permeabilization of erythrocytes by a perforin-like protein during egress of malaria parasites. Nat Commun (2013) 4:1736. doi: 10.1038/ncomms2725
34. Bowen DG, Walker CM. Mutational escape from CD8+ T cell immunity: HCV evolution, from chimpanzees to man. J Exp Med (2005) 201:1709–14. doi: 10.1084/jem.20050808
35. Tripathi AK, Sha W, Shulaev V, Stins MF, Sullivan DJ Jr. Plasmodium falciparum–infected erythrocytes induce NF-κB regulated inflammatory pathways in human cerebral endothelium. Blood J Am Soc Hematol (2009) 114:4243–52. doi: 10.1182/blood-2009-06-226415
36. Wilson KL, Xiang SD, Plebanski M. A model to study the impact of polymorphism driven liver-stage immune evasion by malaria parasites, to help design effective cross-reactive vaccines. Front Microbiol (2016) 7:303. doi: 10.3389/fmicb.2016.00303
37. Nosjean O, Briolay A, Roux B. Mammalian GPI proteins: Sorting, membrane residence and functions. Biochim Biophys Acta (1997) 1331:153–86. doi: 10.1016/S0304-4157(97)00005-1
38. Souza-Silva FA, Torres LM, Santos-Alves JR, Tang ML, Sanchez BA, Sousa TN, et al. Duffy Antigen receptor for chemokine (DARC) polymorphisms and its involvement in acquisition of inhibitory anti-duffy binding protein II (DBPII) immunity. PLos One (2014) 9:e93782. doi: 10.1371/journal.pone.0093782
39. Boyle MJ, Langer C, Chan J, Hodder AN, Coppel RL, Anders RF, et al. Sequential processing of merozoite surface proteins during and after erythrocyte invasion by plasmodium falciparum. Infect Immun (2014) 82:924–36. doi: 10.1128/IAI.00866-13
40. Chan JA, Howell KB, Reiling L, Ataide R, Mackintosh CL, Fowkes FJI, et al. Targets of antibodies against plasmodium falciparum–infected erythrocytes in malaria immunity. J Clin Invest (2012) 129:3227–38. doi: 10.1172/JCI62182
41. Wahlgren M, Goel S, Akhouri RR. Variant surface antigens of plasmodium falciparum and their roles in severe malaria. Nat Rev Microbiol (2017) 15:479–91. doi: 10.1038/nrmicro.2017.47
42. Pasternak ND, Dzikowski R. PfEMP1: An antigen that plays a key role in the pathogenicity and immune evasion of the malaria parasite plasmodium falciparum. Int J Biochem Cell Biol (2009) 41:1463–6. doi: 10.1016/j.biocel.2008.12.012
43. Miller LH, Baruch DI, Marsh K, Doumbo OK. The pathogenic basis of malaria. Nature (2002) 415:673–79. doi: 10.1038/415673a
44. Epp C, Li F, Howitt CA, Chookajorn T, Deitsch KW. Chromatin associated sense and antisense noncoding RNAs are transcribed from the var gene family of virulence genes of the malaria parasite plasmodium falciparum. RNA (2009) 15:116–27. doi: 10.1261/rna.1080109
45. Deitsch KW, Dzikowski R. Variant gene expression and antigenic variation by malaria parasites. Annu Rev Microbiol (2017) 71:625–41. doi: 10.1146/annurev-micro-090816-093841
46. Simantov K, Goyal M, Dzikowski R. Emerging biology of noncoding RNAs in malaria parasites. PLos Pathogens (2022) 18:e1010600. doi: 10.1371/journal.ppat.1010600
47. Heinberg A, Amit-Avraham I, Mitesser V, Simantov K, Goyal M, Nevo Y, et al. A nuclear redox sensor modulates gene activation and var switching in plasmodium falciparum. Proc Natl Acad Sci (2022) 119:e2201247119. doi: 10.1073/pnas.2201247119
48. Dzikowski R, Li F, Amulic B, Eisberg A, Frank M, Patel S, et al. Mechanisms underlying mutually exclusive expression of virulence genes by malaria parasites. EMBO Rep (2007) 8:959–65. doi: 10.1038/sj.embor.7401063
49. Volz JC, Bártfai R, Petter M, Langer C, Josling GA, Tsuboi T, et al. PfSET10, a plasmodium falciparum methyltransferase, maintains the active var gene in a poised state during parasite division. Cell Host Microbe (2012) 11:7–18. doi: 10.1016/j.chom.2011.11.011
50. Ngwa CJ, Gross MR, Musabyimana JP, Pradel G, Deitsch KW. The role of the histone methyltransferase PfSET10 in antigenic variation by malaria parasites: A cautionary tale. Msphere. (2021) 6:e01217-20. doi: 10.1128/mSphere.01217-20
51. Tonkin CJ, Carret CK, Duraisingh MT, Voss TS, Ralph SA, Hommel M, et al. Sir2 paralogues cooperate to regulate virulence genes and antigenic variation in plasmodium falciparum. PLos Biol (2009) 7:e84. doi: 10.1371/journal.pbio.1000084
52. Merrick CJ, Jiang RH, Skillman KM, Samarakoon U, Moore RM, Dzikowski R, et al. Functional analysis of sirtuin genes in multiple plasmodium falciparum strains. PLos One (2015) 10:e0118865. doi: 10.1371/journal.pone.0118865
53. Claessens A, Harris LM, Stanojcic S, Chappell L, Stanton A, Kuk N, et al. RecQ helicases in the malaria parasite plasmodium falciparum affect genome stability, gene expression patterns and DNA replication dynamics. PLos Genet (2018) 14:e1007490. doi: 10.1371/journal.pgen.1007490
54. Li Z, Yin S, Sun M, Cheng X, Wei J, Gilbert N, et al. DNA Helicase RecQ1 regulates mutually exclusive expression of virulence genes in plasmodium falciparum via heterochromatin alteration. Proc Natl Acad Sci USA (2019) 116:3177–82. doi: 10.1073/pnas.1811766116
55. Bryant JM, Baumgarten S, Dingli F, Loew D, Sinha A, Claës A, et al. Exploring the virulence gene interactome with CRISPR/dCas9 in the human malaria parasite. Mol Syst Biol (2020) 16:e9569. doi: 10.15252/msb.20209569
56. Jensen AR, Adams Y, Hviid L. Cerebral plasmodium falciparum malaria: The role of PfEMP1 in its pathogenesis and immunity, and PfEMP1-based vaccines to prevent it. Immunol Rev (2020) 293:230–52. doi: 10.1111/imr.12807
57. Niang M, Bei AK, Madnani KG, Pelly S, Dankwa S, Kanjee U, et al. STEVOR is a plasmodium falciparum erythrocyte binding protein that mediates merozoite invasion and rosetting. Cell Host Microbe (2014) 16:81–93. doi: 10.1016/j.chom.2014.06.004
58. Goel S, Palmkvist M, Moll K, Joannin N, Lara P, Akhouri RR, et al. RIFINs are adhesins implicated in severe plasmodium falciparum malaria. Nat Med (2015) 21:314–7. doi: 10.1038/nm.3812
59. D'Ombrain MC, Voss TS, Maier AG, Pearce JA, Hansen DS, Cowman AF, et al. Plasmodium falciparum erythrocyte membrane protein-1 specifically suppresses early production of host interferon-gamma. Cell Host Microbe (2007) 2:130–8. doi: 10.1016/j.chom.2007.06.012
60. Chew M, Ye W, Omelianczyk RI, Pasaje CF, Hoo R, Chen Q, et al. Selective expression of variant surface antigens enables plasmodium falciparum to evade immune clearance. vivo. Nat Commun (2022) 13:4067. doi: 10.1038/s41467-022-31741-2
61. Zelter T, Strahilevitz J, Simantov K, Yajuk O, Adams Y, Ramstedt Jensen A, et al. Neutrophils impose strong immune pressure against PfEMP1 variants implicated in cerebral malaria. EMBO Rep (2022) 13:e53641. doi: 10.15252/embr.202153641
62. Saito F, Hirayasu K, Satoh T, Wang CW, Lusingu J, Arimori T, et al. Immune evasion of plasmodium falciparum by RIFIN via inhibitory receptors. Nature. (2017) 552:101–5. doi: 10.1038/nature24994
63. Devenport M, Fujioka H, Donnelly-Doman M, Shen Z, Jacobs-Lorena M. Storage and secretion of Ag-Aper14, a novel peritrophic matrix protein, and Ag-Muc1 from the mosquito anopheles gambiae. Cell Tissue Res (2005) 320:175–85. doi: 10.1007/s00441-004-1067-3
64. Shahabuddin M, Kaslow DC. Plasmodium: Parasite chitinase and its role in malaria transmission. Exp parasitol (1994) 79:85–8. doi: 10.1006/expr.1994.1066
65. Tomas AM, Margos G, Dimopoulos G, Van Lin LH, de Koning-Ward TF, Sinha R, et al. P25 and P28 proteins of the malaria ookinete surface have multiple and partially redundant functions. EMBO J (2001) 20:3975–83. doi: 10.1093/emboj/20.15.3975
66. Molina-Cruz A, Garver LS, Alabaster A, Bangiolo L, Haile A, Winikor J, et al. The human malaria parasite Pfs47 gene mediates evasion of the mosquito immune system. Science. (2013) 340:984–7. doi: 10.1126/science.1235264
67. Molina-Cruz A, Canepa GE, Alves E Silva TL, Williams AE, Nagyal S, Yenkoidiok-Douti L, et al. Plasmodium falciparum evades immunity of anopheline mosquitoes by interacting with a Pfs47 midgut receptor. Proc Natl Acad Sci U S A. (2020) 117:2597–605. doi: 10.1073/pnas.1917042117
68. Ramphul UN, Garver LS, Molina-Cruz A, Canepa GE, Barillas-Mury C. Plasmodium falciparum evades mosquito immunity by disrupting JNK-mediated apoptosis of invaded midgut cells. Proc Natl Acad Sci U S A. (2015) 112:1273–80. doi: 10.1073/pnas.1423586112
69. Ukegbu CV, Giorgalli M, Tapanelli S, Rona LDP, Jaye A, Wyer C, et al. PIMMS43 is required for malaria parasite immune evasion and sporogonic development in the mosquito vector. Proc Natl Acad Sci USA (2020) 117:7363–73. doi: 10.1073/pnas.1919709117
70. Simon N, Lasonder E, Scheuermayer M, Kuehn A, Tews S, Fischer R, et al. Malaria parasites co-opt human factor h to prevent complement-mediated lysis in the mosquito midgut. Cell Host Microbe (2013) 13:29–41. doi: 10.1016/j.chom.2012.11.013
71. Kennedy AT, Schmidt CQ, Thompson JK, Weiss GE, Taechalertpaisarn T, Gilson PR, et al. Recruitment of factor h as a novel complement evasion strategy for blood-stage plasmodium falciparum infection. J Immunol (2015) 196:1239–48. doi: 10.4049/jimmunol.1501581
72. Rosa TFA, Flammersfeld A, Ngwa CJ, Kiesow M, Fischer R, Zipfel PF, et al. The plasmodium falciparum blood stages acquire factor h family proteins to evade destruction by human complement. Cell Microbiol (2015) 18:573–90. doi: 10.1111/cmi.12535
73. Tham WH, Wilson DW, Lopaticki S, Schmidt CQ, Tetteh-Quarcoo PB, Barlow PN, et al. Complement receptor 1 is the host erythrocyte receptor for plasmodium falciparum PfRh4 invasion ligand. Proc Natl Acad Sci (2010) 107:17327–32. doi: 10.1073/pnas.1008151107
74. Larsen MD, Quintana MDP, Ditlev SB, Bayarri Olmos R, Ofori MF, Hviid L, et al. Evasion of classical complement pathway activation on plasmodium falciparum-infected erythrocytes opsonized by PfEMP1-specific IgG. Front Immunol (2019) 9:3088. doi: 10.3389/fimmu.2018.03088
75. Vigan-Womas I, Guillotte M, Juillerat A, Vallieres C, Lewit-Bentley A, Tall A, et al. Allelic diversity of the plasmodium falciparum erythrocyte membrane protein 1 entails variant-specific red cell surface epitopes. PLos One (2011) 6:e16544. doi: 10.1371/journal.pone.0016544
76. Stevenson L, Laursen E, Cowan GJ, Bandoh B, Barfod L, Cavanagh DR, et al. α2-macroglobulin can crosslink multiple plasmodium falciparum erythrocyte membrane protein 1 (PfEMP1) molecules and may facilitate adhesion of parasitized erythrocytes. PLos pathogens (2015) 11:e1005022. doi: 10.1371/journal.ppat.1005022
77. Kiyuka PK, Meri S, Khattab A. Complement in malaria: immune evasion strategies and role in protective immunity. FEBS letters (2020) 594:2502–17. doi: 10.1002/1873-3468.13772
78. Yam XY, Preiser PR. Host immune evasion strategies of malaria blood stage parasite. Mol BioSystems (2017) 13:2498–508. doi: 10.1039/C7MB00502D
79. Dasari P, Fries A, Heber SD, Salama A, Blau IW, Lingelbach K, et al. Malarial anemia: Digestive vacuole of plasmodium falciparum mediates complement deposition on bystander cells to provoke hemophagocytosis. Med Microbiol Immunol (2014) 203:383–93. doi: 10.1007/s00430-014-0347-0
80. Reiss T, Theis HI, Gonzalez-Delgado A, Vega-Rodriguez J, Zipfel PF, Skerka C, et al. Acquisition of human plasminogen facilitates complement evasion by the malaria parasite plasmodium falciparum. Eur J Immunol (2021) 51:490–3. doi: 10.1002/eji.202048718
81. Schmidt CQ, Kennedy AT, Tham WH. More than just immune evasion: Hijacking complement by plasmodium falciparum. Mol Immunol (2015) 67:71–84. doi: 10.1016/j.molimm.2015.03.006
82. Shukla M, Chandley P, Rohatgi S. The role of b-cells and antibodies against candida vaccine antigens in invasive candidiasis. Vaccines. (2021) 9:1159. doi: 10.3390/vaccines9101159
83. Chen Q, Amaladoss A, Ye W, Liu M, Dummler S, Kong F, et al. Human natural killer cells control plasmodium falciparum infection by eliminating infected red blood cells. Proc Natl Acad Sci (2014) 111:1479–84. doi: 10.1073/pnas.1323318111
84. Yap XZ, Lundie RJ, Beeson JG, O'Keeffe M. Dendritic cell responses and function in malaria. Front Immunol (2019) 10:357. doi: 10.3389/fimmu.2019.00357
85. Yap XZ, Lundie RJ, Feng G, Pooley J, Beeson JG, O'Keeffe M. Different life cycle stages of plasmodium falciparum induce contrasting responses in dendritic cells. Front Immunol (2019) 10:32. doi: 10.3389/fimmu.2019.00032
86. Woodberry T, Minigo G, Piera K, Amante FH, Pinzon-Charry A, Good MF, et al. Low-level plasmodium falciparum blood-stage infection causes dendritic cell apoptosis and dysfunction in healthy volunteers. J Infect Dis (2012) 206:333–40. doi: 10.1093/infdis/jis366
87. Kurup SP, Butler NS, Harty JT. T Cell-mediated immunity to malaria. Nat Rev Immunol (2019) 19:457–71. doi: 10.1038/s41577-019-0158-z
88. Hisaeda H, Maekawa Y, Iwakawa D, Okada H, Himeno K, Kishihara K, et al. Escape of malaria parasites from host immunity requires CD4+ CD25+ regulatory T cells. Nat Med (2004) 10:29–30. doi: 10.1038/nm975
89. Wykes MN, Horne-Debets JM, Leow CY, Karunarathne DS. Malaria drives T cells to exhaustion. Front Microbiol (2014) 5:249. doi: 10.3389/fmicb.2014.00249
90. Kafuye-Mlwilo MY, Mukherjee P, Chauhan VS. Kinetics of humoral and memory b cell response induced by the plasmodium falciparum 19-kilodalton merozoite surface protein 1 in mice. Infection immunity (2012) 80:633–42. doi: 10.1128/IAI.05188-11
91. Risco-Castillo V, Topçu S, Marinach C, Manzoni G, Bigorgne AE, Briquet S, et al. Malaria sporozoites traverse host cells within transient vacuoles. Cell Host Microbe (2015) 18:593–603. doi: 10.1016/j.chom.2015.10.006
92. Stanisic DI, Barry AE, Good MF. Escaping the immune system: How the malaria parasite makes vaccine development a challenge. Trends Parasitol (2013) 29:612–22. doi: 10.1016/j.pt.2013.10.001
93. Kotraiah V, Phares TW, Terry FE, Hindocha P, Silk SE, Nielsen CM, et al. Identification and immune assessment of T cell epitopes in five plasmodium falciparum blood stage antigens to facilitate vaccine candidate selection and optimization. Front Immunol (2021) 12:690348. doi: 10.3389/fimmu.2021.690348
94. Templeton TJ. The varieties of gene amplification, diversification and hypervariability in the human malaria parasite, plasmodium falciparum. Mol Biochem Parasitol (2009) 166:109–16. doi: 10.1016/j.molbiopara.2009.04.003
95. Blandin S, Shiao SH, Moita LF, Janse CJ, Waters AP, Kafatos FC, et al. Complement-like protein TEP1 is a determinant of vectorial capacity in the malaria vector anopheles gambiae. Cell. (2004) 116:661–70. doi: 10.1016/S0092-8674(04)00173-4
96. Osta MA, Christophides GK, Kafatos FC. Effects of mosquito genes on plasmodium development. Science (2004) 303:2030–2. doi: 10.1126/science.1091789
97. Kolli SK, Molina-Cruz A, Araki T, Geurten FJA, Ramesar J, Chevalley-Maurel S, et al. Malaria parasite evades mosquito immunity by glutaminyl cyclase-mediated posttranslational protein modification. Proc Natl Acad Sci U S A. (2022) 119:e2209729119. doi: 10.1073/pnas.2209729119
98. Clayton AM, Dong Y, Dimopoulos G. The anopheles innate immune system in the defense against malaria infection. J innate immunity (2014) 6:169–81. doi: 10.1159/000353602
99. Osta MA, Christophides GK, Vlachou D, Kafatos FC. Innate immunity in the malaria vector anopheles gambiae: comparative and functional genomics. J Exp Biol (2004) 207:2551–63. doi: 10.1242/jeb.01066
100. Molina-Cruz A, DeJong RJ, Charles B, Gupta L, Kumar S, Jaramillo-Gutierrez G, et al. Reactive oxygen species modulate anopheles gambiae immunity against bacteria and plasmodium. J Biol Chem (2008) 283:3217–23. doi: 10.1074/jbc.M705873200
101. Crompton PD, Moebius J, Portugal S, Waisberg M, Hart G, Garver LS, et al. Malaria immunity in man and mosquito: insights into unsolved mysteries of a deadly infectious disease. Annu Rev Immunol (2014) 32:157–87. doi: 10.1146/annurev-immunol-032713-120220
102. Claudianos C, Dessens JT, Trueman HE, Arai M, Mendoza J, Butcher GA, et al. A malaria scavenger receptor-like protein essential for parasite development. Mol Microbiol (2002) 45:1473–84. doi: 10.1046/j.1365-2958.2002.03118.x
103. Tewari R, Rathore D, Crisanti A. Motility and infectivity of plasmodium berghei sporozoites expressing avian plasmodium gallinaceum circumsporozoite protein. Cell Microbiol (2005) 7:699–707. doi: 10.1111/j.1462-5822.2005.00503.x
104. Bettencourt P. Current challenges in the identification of preerythrocytic malaria vaccine candidate antigens. Front Immunol (2020) 11:190. doi: 10.3389/fimmu.2020.00190
105. Cohen J, Nussenzweig V, Vekemans J, Leach A. From the circumsporozoite protein to the RTS, S/AS candidate vaccine. Hum Vaccines (2010) 6:90–6. doi: 10.4161/hv.6.1.9677
106. McCall MB, Kremsner PG, Mordmüller B. Correlating efficacy and immunogenicity in malaria vaccine trials. InSeminars Immunol (2018) 39:52–64. doi: 10.1016/j.smim.2018.08.002
107. Agnandji ST, Lell B, Fernandes JF, Abossolo BP, Methogo BG, Kabwende AL, et al. RTS,S clinical trials partnership. 2012. a phase 3 trial of RTS,S/AS01 malaria vaccine in African infants. N Engl J Med (2012) 367:2284–95. doi: 10.1056/NEJMoa1208394
108. Nadeem AY, Shehzad A, Islam SU, Al-Suhaimi EA, Lee YS. Mosquirix™ RTS, S/AS01 vaccine development, immunogenicity, and efficacy. Vaccines (Basel) (2022) 10:713. doi: 10.3390/vaccines10050713
109. Mehrizi AA, Ameri Torzani M, Zakeri S, Jafary Zadeh A, Babaeekhou L. Th1 immune response to plasmodium falciparum recombinant thrombospondin-related adhesive protein (TRAP) antigen is enhanced by TLR3-specific adjuvant, poly(I:C) in BALB/c mice. Parasite Immunol (2018) 40:e12538. doi: 10.1111/pim.12538
110. Lu C, Song G, Beale K, Yan J, Garst E, Feng J, et al. Design and assessment of TRAP-CSP fusion antigens as effective malaria vaccines. PLos One (2020) 15:e0216260. doi: 10.1371/journal.pone.0216260
111. Daniel S, Pichugin A, Torano H, Renn JP, Kwan J, Cowles MV, et al. Plasmodium preerythrocytic vaccine antigens enhance sterile protection in mice induced by circumsporozoite protein. Infect Immun (2021) 89:e0016521. doi: 10.1128/IAI.00165-21
112. Datoo MS, Natama MH, Somé A, Traoré O, Rouamba T, Bellamy D, et al. Efficacy of a low-dose candidate malaria vaccine, R21 in adjuvant matrix-m, with seasonal administration to children in Burkina Faso: a randomised controlled trial. Lancet. (2021) 397:1809–18. doi: 10.1016/S0140-6736(21)00943-0
113. Shahnaij M, Iyori M, Mizukami H, Kajino M, Yamagoshi I, Syafira I, et al. Liver-directed AAV8 booster vaccine expressing plasmodium falciparum antigen following adenovirus vaccine priming elicits sterile protection in a murine model. Front Immunol (2021) 12:612910. doi: 10.3389/fimmu.2021.612910
114. Quadiri A, Kalia I, Kashif M, Singh AP. Identification and characterization of protective CD8+ T-epitopes in a malaria vaccine candidate SLTRiP. Immunity Inflammation Disease (2020) 8(1):50–61. doi: 10.1002/iid3.283
115. Quadiri A, Kashif M, Kalia I, Singh AP. SLTRiP induces long lasting and protective T-cell memory response. bioRxiv. (2021) 1:1–20. doi: 10.1101/2021.01.07.425694
116. Watson F, Shears M, Matsubara J, Kalata A, Seilie A, Talavera IC, et al. Cryopreserved sporozoites with and without the glycolipid adjuvant 7DW8-5 protect in prime-and-Trap malaria vaccination. Am J Trop Med Hyg (2022) 106:1227–36. doi: 10.4269/ajtmh.21-1084
117. Valencia-Hernandez AM, Ng WY, Ghazanfari N, Ghilas S, de Menezes MN, Holz LE, et al. A natural peptide antigen within the plasmodium ribosomal protein RPL6 confers liver TRM cell-mediated immunity against malaria in mice. Cell Host Microbe (2020) 27:950–962.e7. doi: 10.1016/j.chom.2020.04.010
118. Mueller AK, Labaied M, Kappe SH, Matuschewski K. Genetically modified plasmodium parasites as a protective experimental malaria vaccine. Nature. (2005) 433:164–7. doi: 10.1038/nature03188
119. Richie TL, Billingsley PF, Sim BK, James ER, Chakravarty S, Epstein JE, et al. Progress with plasmodium falciparum sporozoite (PfSPZ)-based malaria vaccines. Vaccine. (2015) 33:7452–61. doi: 10.1016/j.vaccine.2015.09.096
120. Mikolajczak SA, Lakshmanan V, Fishbaugher M, Camargo N, Harupa A, Kaushansky A, et al. A next-generation genetically attenuated plasmodium falciparum parasite created by triple gene deletion. Mol Ther (2014) 22:1707–15. doi: 10.1038/mt.2014.85
121. Kublin JG, Mikolajczak SA, Sack BK, Fishbaugher ME, Seilie A, Shelton L, et al. Complete attenuation of genetically engineered plasmodium falciparum sporozoites in human subjects. Sci Transl Med (2017) 9:eaad9099. doi: 10.1126/scitranslmed.aad9099
122. Murphy SC, Vaughan AM, Kublin JG, Fishbauger M, Seilie AM, Cruz KP, et al. A genetically engineered plasmodium falciparum parasite vaccine provides protection from controlled human malaria infection. Sci Transl Med (2022) 14:eabn9709. doi: 10.1126/scitranslmed.abn9709
123. Goswami D, Betz W, Locham NK, Parthiban C, Brager C, Schäfer C, et al. A replication-competent late liver stage-attenuated human malaria parasite. JCI Insight (2020) 5:e135589. doi: 10.1172/jci.insight.135589
124. Sulyok Z, Fendel R, Eder B, Lorenz FR, Kc N, Karnahl M, et al. Heterologous protection against malaria by a simple chemoattenuated PfSPZ vaccine regimen in a randomized trial. Nat Commun (2021) 12:2518. doi: 10.1038/s41467-021-22740-w
125. Coulibaly D, Kone AK, Traore K, Niangaly A, Kouriba B, Arama C, et al. PfSPZ-CVac malaria vaccine demonstrates safety among malaria-experienced adults: A randomized, controlled phase 1 trial. E Clin Med (2022) 52:101579. doi: 10.1016/j.eclinm.2022.101579
126. Nunes-Cabaço H, Moita D, Prudêncio M. Five decades of clinical assessment of whole-sporozoite malaria vaccines. Front Immunol (2022) 13. doi: 10.3389/fimmu.2022.977472
127. Lin JW, Annoura T, Sajid M, Chevalley-Maurel S, Ramesar J, Klop O, et al. A novel ‘Gene Insertion/Marker out’ (Gimo) method for transgene expression and gene complementation in rodent malaria parasites. PLos One (2011) 6:e29289. doi: 10.1371/journal.pone.0029289
128. Mendes AM, Machado M, Goncalves-Rosa N, Reuling IJ, Foquet L, Marques C, et al. A plasmodium berghei sporozoite-based vaccination platform against human malaria. NPJ Vaccines (2018) 3:33. doi: 10.1038/s41541-018-0068-2
129. Reuling IJ, Mendes AM, de Jong GM, Fabra-Garcia A, Nunes-Cabaco H, van Gemert GJ, et al. An open-label phase 1/2a trial of a genetically modified rodent malaria parasite for immunization against plasmodium falciparum malaria. Sci Transl Med (2020) 12:1–12. doi: 10.1126/scitranslmed.aay2578
130. Deshmukh A, Chourasia BK, Mehrotra S, Kana IH, Paul G, Panda A, et al. Plasmodium falciparum MSP3 exists in a complex on the merozoite surface and generates antibody response during natural infection. Infect Immun (2018) 86:e00067–18. doi: 10.1128/IAI.00067-18
131. Sathishkumar V, Nirmolia T, Bhattacharyya DR, Patgiri SJ. Genetic polymorphism of plasmodium falciparum msp-1, msp-2 and glurp vaccine candidate genes in pre-artemisinin era clinical isolates from lakhimpur district in Assam, northeast India. Access Microbiol (2022) 4:000350. doi: 10.1099/acmi.0.000350
132. Ghoshal S, Datta Kanjilal S, Sengupta S. Plasmodium vivax vaccine candidate MSP1 displays conserved b-cell epitope despite high genetic diversity. Infect Genet Evol (2021) 93:104929. doi: 10.1016/j.meegid.2021.104929
133. Das SC, Price JD, Gosling K, MacLennan N, Ataíde R, Seow J, et al. Liposome engraftment and antigen combination potentiate the immune response towards conserved epitopes of the malaria vaccine candidate MSP2. Vaccine. (2021) 39:1746–57. doi: 10.1016/j.vaccine.2021.02.010
134. Dejon-Agobe JC, Ateba-Ngoa U, Lalremruata A, Homoet A, Engelhorn J, Nouatin OP, et al. Controlled human malaria infection of healthy adults with lifelong malaria exposure to assess safety, immunogenicity, and efficacy of the asexual blood stage malaria vaccine candidate GMZ2. Clin Infect Dis (2019) 69:1377–84. doi: 10.1093/cid/ciy1087
135. Sirima SB, Tiono AB, Ouédraogo A, Diarra A, Ouédraogo AL, Yaro JB, et al. Safety and immunogenicity of the malaria vaccine candidate MSP3 long synthetic peptide in 12-24 months-old burkinabe children. PLos One (2009) 4:e7549. doi: 10.1371/journal.pone.0007549
136. Lee SH, Chu KB, Kang HJ, Quan FS. Protection and alleviated inflammation induced by virus-like particle vaccines containing plasmodium berghei MSP-8, MSP-9 and RAP1. Vaccines (Basel) (2022) 10:203. doi: 10.3390/vaccines10020203
137. Kale S, Pande V, Singh OP, Carlton JM, Mallick PK. Genetic diversity in two leading plasmodium vivax malaria vaccine candidates AMA1 and MSP119 at three sites in India. PLos Negl Trop Dis (2021) 15:e0009652. doi: 10.1371/journal.pntd.0009652
138. Remarque EJ, Faber BW, Rodriguez Garcia R, Oostermeijer H, Sirima SB, Nebie Ouedraogo I, et al. Accelerated phase ia/b evaluation of the malaria vaccine candidate PfAMA1 DiCo demonstrates broadening of humoral immune responses. NPJ Vaccines (2021) 6:55. doi: 10.1038/s41541-021-00319-2
139. Azazi A, Haron FN, Chua KH, Lim YAL, Lee PC, Chew CH. Bioinformatics characterization of plasmodium knowlesi apical membrane antigen 1 (PkAMA1) for multi-epitope vaccine design. Trop Biomed (2021) 38:265–75. doi: 10.47665/tb.38.3.067
140. Kim MJ, Chu KB, Kang HJ, Yoon KW, Eom GD, Mao J, et al. Protective immunity induced by immunization with baculovirus, virus-like particle, and vaccinia virus expressing the AMA1 of plasmodium berghei. Biomedicines. (2022) 10:2289. doi: 10.3390/biomedicines10092289
141. Delves MJ, Angrisano F, Blagborough AM. Antimalarial transmission-blocking interventions: Past, present, and future. Trends Parasitol (2018) 34:735–46. doi: 10.1016/j.pt.2018.07.001
142. Marin-Mogollon C, van de Vegte-Bolmer M, van Gemert GJ, van Pul FJA, Ramesar J, Othman AS, et al. The plasmodium falciparum male gametocyte protein P230p, a paralog of P230, is vital for ookinete formation and mosquito transmission. Sci Rep (2018) 8:14902. doi: 10.1038/s41598-018-33236-x
143. Sagara I, Healy SA, Assadou MH, Gabriel EE, Kone M, Sissoko K, et al. Safety and immunogenicity of Pfs25H-EPA/Alhydrogel, a transmission-blocking vaccine against plasmodium falciparum: a randomised, double-blind, comparator-controlled, dose-escalation study in healthy malian adults. Lancet Infect Dis (2018) 18:969–82. doi: 10.1016/S1473-3099(18)30344-X
144. Scaria PV, Anderson C, Muratova O, Alani N, Trinh HV, Nadakal ST, et al. Malaria transmission-blocking conjugate vaccine in ALFQ adjuvant induces durable functional immune responses in rhesus macaques. NPJ Vaccines (2021) 6:1–10. doi: 10.1038/s41541-021-00407-3
145. Healy SA, Anderson C, Swihart BJ, Mwakingwe A, Gabriel EE, Decederfelt H, et al. Pfs230 yields higher malaria transmission-blocking vaccine activity than Pfs25 in humans but not mice. J Clin Invest (2021) 131:e146221. doi: 10.1172/JCI146221
146. Doi M, Tanabe K, Tachibana S, Hamai M, Tachibana M, Mita T, et al. Worldwide sequence conservation of transmission-blocking vaccine candidate Pvs230 in plasmodium vivax. Vaccine. (2011) 29:4308–15. doi: 10.1016/j.vaccine.2011.04.028
147. Singh SK, Plieskatt J, Chourasia BK, Singh V, Bengtsson KL, Reimer JM, et al. Preclinical development of a Pfs230-Pfs48/45 chimeric malaria transmission-blocking vaccine. NPJ Vaccines (2021) 6:120. doi: 10.1038/s41541-021-00383-8
148. Cao Y, Hart RJ, Bansal GP, Kumar N. Functional conservation of P48/45 proteins in the transmission stages of plasmodium vivax (Human malaria parasite) and p. berghei (Murine Malaria Parasite) mBio (2018) 9:e01627–18. doi: 10.1128/mBio.01627-18
149. McLeod B, Mabrouk MT, Miura K, Ravichandran R, Kephart S, Hailemariam S, et al. Vaccination with a structure-based stabilized version of malarial antigen Pfs48/45 elicits ultra-potent transmission-blocking antibody responses. Immunity. (2022) 55:1680–1692.e8. doi: 10.1016/j.immuni.2022.07.015
150. Kumar S, Valansi C, Haile MT, Li X, Flyak K, Dwivedy A, et al. Malaria parasites utilize two essential plasma membrane fusogens for gamete fertilization. Cell Mol Life Sci (2022) 79:549. doi: 10.1007/s00018-022-04583-w
151. Blagborough AM, Sinden RE. Plasmodium berghei HAP2 induces strong malaria transmission-blocking immunity in vivo and in vitro. Vaccine. (2009) 27:5187–94. doi: 10.1016/j.vaccine.2009.06.069
152. Angrisano F, Sala KA, Da DF, Liu Y, Pei J, Grishin NV, et al. Targeting the conserved fusion loop of HAP2 inhibits the transmission of plasmodium berghei and falciparum. Cell Rep (2017) 21:2868–78. doi: 10.1016/j.celrep.2017.11.024
153. Tripathi AK, Oakley MS, Verma N, Mlambo G, Zheng H, Meredith SM, et al. Plasmodium falciparum Pf77 and male development gene 1 as vaccine antigens that induce potent transmission-reducing antibodies. Sci Transl Med (2021) 13:eabg2112. doi: 10.1126/scitranslmed.abg2112
154. Li F, Patra KP, Yowell CA, Dame JB, Chin K, Vinetz JM. Apical surface expression of aspartic protease plasmepsin 4, a potential transmission-blocking target of the plasmodium ookinete. J Biol Chem (2010) 285:8076–83. doi: 10.1074/jbc.M109.063388
155. Li F, Patra KP, Vinetz JM. An anti-chitinase malaria transmission–blocking single-chain antibody as an effector molecule for creating a plasmodium falciparum–refractory mosquito. J Infect diseases (2005) 192:878–87. doi: 10.1086/432552
156. Baton LA, Ranford-Cartwright LC. Do malaria ookinete surface proteins P25 and P28 mediate parasite entry into mosquito midgut epithelial cells? Malaria J (2005) 4:1–8. doi: 10.1186/1475-2875-4-15
157. Kaslow DC, Bathurst IC, Lensen T, Ponnudurai T, Barr PJ, Keister DB. Saccharomyces cerevisiae recombinant Pfs25 adsorbed to alum elicits antibodies that block transmission of plasmodium falciparum. Infection immunity (1994) 62:5576–80. doi: 10.1128/iai.62.12.5576-5580.1994
158. Duffy PE, Kaslow DC. A novel malaria protein, Pfs28, and Pfs25 are genetically linked and synergistic as falciparum malaria transmission-blocking vaccines. Infection immunity (1997) 65:1109–13. doi: 10.1128/iai.65.3.1109-1113.1997
159. Talaat KR, Ellis RD, Hurd J, Hentrich A, Gabriel E, Hynes NA, et al. Safety and immunogenicity of Pfs25-EPA/Alhydrogel®, a transmission blocking vaccine against plasmodium falciparum: an open label study in malaria naïve adults. PLos One (2016) 11:e0163144. doi: 10.1371/journal.pone.0163144
160. Hisaeda H, Stowers AW, Tsuboi T, Collins WE, Sattabongkot JS, Suwanabun N, et al. Antibodies to malaria vaccine candidates Pvs25 and Pvs28 completely block the ability of plasmodium vivax to infect mosquitoes. Infection immunity (2000) 68:6618–23. doi: 10.1128/IAI.68.12.6618-6623.2000
161. Zheng W, Kou X, Du Y, Liu F, Yu C, Tsuboi T, et al. Identification of three ookinete-specific genes and evaluation of their transmission-blocking potentials in plasmodium berghei. Vaccine. (2016) 34:2570–8. doi: 10.1016/j.vaccine.2016.04.011
162. Pirahmadi S, Zakeri S A, Mehrizi A, Djadid N D, AA R, Sani J J, et al. Cell-traversal protein for ookinetes and sporozoites (CelTOS) formulated with potent TLR adjuvants induces high-affinity antibodies that inhibit plasmodium falciparum infection in anopheles stephensi. Malar J (2019) 18:146. doi: 10.1186/s12936-019-2773-3
163. Bender NG, Khare P, Martinez J, Tweedell RE, Nyasembe VO, López-Gutiérrez B, et al. Immunofocusing humoral immunity potentiates the functional efficacy of the AnAPN1 malaria transmission-blocking vaccine antigen. NPJ Vaccines (2021) 6:1–10. doi: 10.1038/s41541-021-00309-4
164. Xu Y, Zhou Z, Brooks B, Ferguson T, Obliosca J, Huang J, et al. Layer-by-Layer delivery of multiple antigens using trimethyl chitosan nanoparticles as a malaria vaccine candidate. Front Immunol (2022) 13:900080. doi: 10.3389/fimmu.2022.900080
165. Somanathan A, Mian SY, Chaddha K, Uchoi S, Bharti PK, Tandon R, et al. Process development and preclinical evaluation of a major plasmodium falciparum blood stage vaccine candidate, cysteine-rich protective antigen (CyRPA). Front Immunol (2022) 13:1005332. doi: 10.3389/fimmu.2022.1005332
166. Fernandes B, Sousa M, Castro R, Schäfer A, Hauser J, Schulze K, et al. Scalable process for high-yield production of PfCyRPA using insect cells for inclusion in a malaria virosome-based vaccine candidate. Front Bioeng Biotechnol (2022) 10:879078. doi: 10.3389/fbioe.2022.879078
167. Jelínková L, Flores-Garcia Y, Shapiro S, Roberts BT, Petrovsky N, Zavala F, et al. A vaccine targeting the L9 epitope of the malaria circumsporozoite protein confers protection from blood-stage infection in a mouse challenge model. NPJ Vaccines (2022) 7:34. doi: 10.1038/s41541-022-00457-1
168. Khan N, Bin-Mwena MN, Alruways MW, Allehyani NMM, Alanzi MO, Shahzad, Khan A, et al. In silico study to predict promiscuous T cell and b cell-epitopes derived from the vaccine candidate antigens of plasmodium vivax binding to MHC class-II alleles. J Vector Borne Dis (2022) 59:154–62. doi: 10.4103/0972-9062.335726
169. Atapour A, Vosough P, Jafari S, Sarab GA. A multi-epitope vaccine designed against blood-stage of malaria: An immunoinformatic and structural approach. Sci Rep (2022) 12:11683. doi: 10.1038/s41598-022-15956-3
170. Baldwin SL, Roeffen W, Singh SK, Tiendrebeogo RW, Christiansen M, Beebe E, et al. Synthetic TLR4 agonists enhance functional antibodies and CD4+ T-cell responses against the plasmodium falciparum GMZ2.6C multi-stage vaccine antigen. Vaccine. (2016) 34:2207–15. doi: 10.1016/j.vaccine.2016.03.016
171. Baptista BO, de Souza ABL, Riccio EKP, Bianco-Junior C, Totino PRR, Martins da Silva JH, et al. Naturally acquired antibody response to a plasmodium falciparum chimeric vaccine candidate GMZ2.6c and its components (MSP-3, GLURP, and Pfs48/45) in individuals living in Brazilian malaria-endemic areas. Malar J (2022) 21:6. doi: 10.1186/s12936-021-04020-6
172. Duffy MF, Maier AG, Byrne TJ, Marty AJ, Elliott SR, O'Neill MT, et al. VAR2CSA is the principal ligand for chondroitin sulfate a in two allogeneic isolates of plasmodium falciparum. Mol Biochem Parasitol (2006) 148:117–24. doi: 10.1016/j.molbiopara.2006.03.006
173. Dara A, Travassos MA, Adams M, Schaffer DeRoo S, Drábek EF, Agrawal S, et al. A new method for sequencing the hypervariable plasmodium falciparum gene var2csa from clinical samples. Malar J (2017) 16:343. doi: 10.1186/s12936-017-1976-8
174. Doritchamou JYA, Suurbaar J, Tuikue Ndam N. Progress and new horizons toward a VAR2CSA-based placental malaria vaccine. Expert Rev Vaccines (2021) 20:215–26. doi: 10.1080/14760584.2021.1878029
175. Chakrabarti M, Garg S, Munjal A, Karan S, Pati S, Garg LC, et al. A fast-track phenotypic characterization of plasmodium falciparum vaccine antigens through lyse-reseal erythrocytes mediated delivery (LyRED) of RNA interference for targeted translational repression. Methods Mol Biol (2022) 2410:539–53. doi: 10.1007/978-1-0716-1884-4_27
176. Van Buskirk KM, Sevova E, Adams JH. Conserved residues in the plasmodium vivax Duffy-binding protein ligand domain are critical for erythrocyte receptor recognition. Proc Natl Acad Sci U S A. (2004) 101:15754–9. doi: 10.1073/pnas.0405421101
177. Apostolakis S, Chalikias GK, Tziakas DN, Konstantinides S. Erythrocyte Duffy antigen receptor for chemokines (DARC): Diagnostic and therapeutic implications in atherosclerotic cardiovascular disease. Acta Pharmacol Sin (2011) 32:417–24. doi: 10.1038/aps.2011.13
178. Kar S, Sinha A. Plasmodium vivax Duffy binding protein-based vaccine: A distant dream. Front Cell Infect Microbiol (2022) 12:916702. doi: 10.3389/fcimb.2022.916702
179. Bijker EM, Bastiaens GJ, Teirlinck AC, van Gemert GJ, Graumans W, van de Vegte-Bolmer M, et al. Protection against malaria after immunization by chloroquine prophylaxis and sporozoites is mediated by preerythrocytic immunity. Proc Natl Acad Sci (2013) 110:7862–7. doi: 10.1073/pnas.1220360110
180. Pfeil J, Sepp KJ, Heiss K, Meister M, Mueller AK, Borrmann S. Protection against malaria by immunization with non-attenuated sporozoites under single-dose piperaquine-tetraphosphate chemoprophylaxis. Vaccine. (2014) 32:6005–11. doi: 10.1016/j.vaccine.2014.07.112
181. Behet MC, Foquet L, van Gemert GJ, Bijker EM, Meuleman P, Leroux-Roels G, et al. Sporozoite immunization of human volunteers under chemoprophylaxis induces functional antibodies against pre-erythrocytic stages of plasmodium falciparum. Malaria J (2014) 13(1):1–2. doi: 10.1186/1475-2875-13-136
182. Bhardwaj J, Siddiqui AJ, Goyal M, Prakash K, Soni A, Puri SK. Repetitive live sporozoites inoculation under arteether chemoprophylaxis confers protection against subsequent sporozoite challenge in rodent malaria model. Acta Tropica (2016) 158:130–8. doi: 10.1016/j.actatropica.2016.02.016
183. Siddiqui AJ, Bhardwaj J, Hamadou WS, Goyal M, Ashraf SA, Jahan S, et al. Chemoprophylaxis under sporozoites-lumefantrine (CPS-LMF) immunization induce protective immune responses against plasmodium yoelii sporozoites infection in mice. 3 Biotech (2021) 11:1–5. doi: 10.1007/s13205-021-03022-0
184. Siddiqui AJ, Bhardwaj J, Hamadou WS, Goyái M, Jahan S, Ashraf SA, et al. Impact of chemoprophylaxis immunisation under halofantrine (CPS-HF) drug cover in plasmodium yoelii Swiss mice malaria model. Folia Parasitologica (2022) 69:1–0. doi: 10.14411/fp.2022.003
185. Moncunill G, Scholzen A, Mpina M, Nhabomba A, Hounkpatin AB, Osaba L, et al. Antigen-stimulated PBMC transcriptional protective signatures for malaria immunization. Sci Trans Med (2020) 12:eaay8924. doi: 10.1126/scitranslmed.aay8924
186. Pleass RJ, Holder AA. Antibody-based therapies for malaria. Nat Rev Microbiol (2005) 3:893–9. doi: 10.1038/nrmicro1267
187. Cohen S, Mc GI, Carrington S. Gamma-globulin and acquired immunity to human malaria. Nature (1961) 192:733–7. doi: 10.1038/192733a0
188. Spencer Valero LM, Ogun SA, Fleck SL, Ling IT, Scott-Finnigan TJ, Blackman MJ, et al. Passive immunization with antibodies against three distinct epitopes on plasmodium yoelii merozoite surface protein 1 suppresses parasitemia. Infect Immun (1998) 66:3925–30. doi: 10.1128/IAI.66.8.3925-3930.1998
189. Akter J, Khoury DS, Aogo R, Lansink LIM, SheelaNair A, Thomas BS, et al. Plasmodium-specific antibodies block in vivo parasite growth without clearing infected red blood cells. PLos Pathog (2019) 15:e1007599. doi: 10.1371/journal.ppat.1007599
190. Kurtovic L, Boyle MJ, Opi DH, Kennedy AT, Tham WH, Reiling L, et al. Complement in malaria immunity and vaccines. Immunol Rev (2020) 293:38–56. doi: 10.1111/imr.12802
191. Opi DH, Kurtovic L, Chan JA, Horton JL, Feng G, Beeson JG. Multi-functional antibody profiling for malaria vaccine development and evaluation. Expert Rev Vaccines (2021) 20:1257–72. doi: 10.1080/14760584.2021.1981864
192. Dobano C, Santano R, Vidal M, Jiménez A, Jairoce C, Ubillos I, et al. Differential patterns of IgG subclass responses to plasmodium falciparum antigens in relation to malaria protection and RTS,S vaccination. Front Immunol (2019) 10:439. doi: 10.3389/fimmu.2019.00439
193. Zhang M, Mandraju R, Rai U, Shiratsuchi T, Tsuji M. Monoclonal antibodies against plasmodium falciparum circumsporozoite protein. Antibodies (Basel) (2017) 6:11. doi: 10.3390/antib6030011
194. Wang LT, Pereira LS, Kiyuka PK, Schön A, Kisalu NK, Vistein R, et al. Protective effects of combining monoclonal antibodies and vaccines against the plasmodium falciparum circumsporozoite protein. PLos Pathog (2021) 17:e1010133. doi: 10.1371/journal.ppat.1010133
195. Laurens MB. RTS,S/AS01 vaccine (Mosquirix™): an overview. Hum Vaccin Immunother (2020) 16:480–9. doi: 10.1080/21645515.2019.1669415
196. Casares S, Brumeanu TD, Richie TL. The RTS,S malaria vaccine. Vaccine. (2010) 28:4880–94. doi: 10.1016/j.vaccine.2010.05.033
197. Foquet L, Hermsen CC, van Gemert GJ, Van Braeckel E, Weening KE, Sauerwein R, et al. Vaccine-induced monoclonal antibodies targeting circumsporozoite protein prevent plasmodium falciparum infection. J Clin Invest (2014) 124:140–4. doi: 10.1172/JCI70349
198. Wang LT, Pereira LS, Flores-Garcia Y, O'Connor J, Flynn BJ, Schön A, et al. A potent anti-malarial human monoclonal antibody targets circumsporozoite protein minor repeats and neutralizes sporozoites in the liver. Immunity. (2020) 53:733–744.e8. doi: 10.1016/j.immuni.2020.08.014
199. Flores-Garcia Y, Wang LT, Park M, Asady B, Idris AH, Kisalu NK, et al. The p. falciparum CSP repeat region contains three distinct epitopes required for protection by antibodies in vivo. PLos Pathog (2021) 17:e1010042. doi: 10.1371/journal.ppat.1010042
200. Clement F, Dewar V, Van Braeckel E, Desombere I, Dewerchin M, Swysen C, et al. Validation of an enzyme-linked immunosorbent assay for the quantification of human IgG directed against the repeat region of the circumsporozoite protein of the parasite plasmodium falciparum. Malar J (2012) 11:384. doi: 10.1186/1475-2875-11-384
201. Burkot TR, Da ZW, Geysen HM, Wirtz RA, Saul A. Fine specificities of monoclonal antibodies against the plasmodium falciparum circumsporozoite protein: recognition of both repetitive and non-repetitive regions. Parasite Immunol (1991) 13:161–70. doi: 10.1111/j.1365-3024.1991.tb00272.x
202. Deal C, Balazs AB, Espinosa DA, Zavala F, Baltimore D, Ketner G. Vectored antibody gene delivery protects against plasmodium falciparum sporozoite challenge in mice. Proc Natl Acad Sci U S A. (2014) 111:12528–32. doi: 10.1073/pnas.1407362111
203. Sack BK, Miller JL, Vaughan AM, Douglass A, Kaushansky A, Mikolajczak S, et al. Model for in vivo assessment of humoral protection against malaria sporozoite challenge by passive transfer of monoclonal antibodies and immune serum. Infect Immun (2014) 82:808–17. doi: 10.1128/IAI.01249-13
204. Gaudinski MR, Berkowitz NM, Idris AH, Coates EE, Holman LA, Mendoza F, et al. A monoclonal antibody for malaria prevention. N Engl J Med (2021) 385:803–14. doi: 10.1056/NEJMoa2034031
205. Kisalu NK, Idris AH, Weidle C, Flores-Garcia Y, Flynn BJ, Sack BK, et al. A human monoclonal antibody prevents malaria infection by targeting a new site of vulnerability on the parasite. Nat Med (2018) 24:408–16. doi: 10.1038/nm.4512
206. Wu RL, Idris AH, Berkowitz NM, Happe M, Gaudinski MR, Buettner C, et al. Low-dose subcutaneous or intravenous monoclonal antibody to prevent malaria. N Engl J Med (2022) 387:397–407. doi: 10.1056/NEJMoa2203067
207. Wilder BK, Vigdorovich V, Carbonetti S, Minkah N, Hertoghs N, Raappana A, et al. Anti-TRAP/SSP2 monoclonal antibodies can inhibit sporozoite infection and may enhance protection of anti-CSP monoclonal antibodies. NPJ Vaccines (2022) 7:58. doi: 10.1038/s41541-022-00480-2
208. Hamre KES, Ondigo BN, Hodges JS, Dutta S, Theisen M, Ayodo G, et al. Antibody correlates of protection from clinical plasmodium falciparum malaria in an area of low and unstable malaria transmission. Am J Trop Med Hyg (2020) 103:2174–82. doi: 10.4269/ajtmh.18-0805
209. Osier FH, Feng G, Boyle MJ, Langer C, Zhou J, Richards JS, et al. Opsonic phagocytosis of plasmodium falciparum merozoites: mechanism in human immunity and a correlate of protection against malaria. BMC Med (2014) 12:108. doi: 10.1186/1741-7015-12-108
210. Ssewanyana I, Arinaitwe E, Nankabirwa JI, Yeka A, Sullivan R, Kamya MR, et al. Avidity of anti-malarial antibodies inversely related to transmission intensity at three sites in Uganda. Malar J (2017) 16:67. doi: 10.1186/s12936-017-1721-3
211. Douglas AD, Williams AR, Knuepfer E, Illingworth JJ, Furze JM, Crosnier C, et al. Neutralization of plasmodium falciparum merozoites by antibodies against PfRH5. J Immunol (2014) 192:245–58. doi: 10.4049/jimmunol.1302045
212. Sakamoto H, Takeo S, Maier AG, Sattabongkot J, Cowman AF, Tsuboi T. Antibodies against a plasmodium falciparum antigen PfMSPDBL1 inhibit merozoite invasion into human erythrocytes. Vaccine. (2012) 30:1972–80. doi: 10.1016/j.vaccine.2012.01.010
213. Perraut R, Varela ML, Joos C, Diouf B, Sokhna C, Mbengue B, et al. Association of antibodies to plasmodium falciparum merozoite surface protein-4 with protection against clinical malaria. Vaccine. (2017) 35:6720–6. doi: 10.1016/j.vaccine.2017.10.012
214. Baum J, Thomas AW, Conway DJ. Evidence for diversifying selection on erythrocyte-binding antigens of plasmodium falciparum and p. vivax Genet (2003) 163:1327–36. doi: 10.1093/genetics/163.4.1327
215. Gao X, Yeo KP, Aw SS, Kuss C, Iyer JK, Genesan S, et al. Antibodies targeting the PfRH1 binding domain inhibit invasion of plasmodium falciparum merozoites. PLos Pathog (2008) 4:e1000104. doi: 10.1371/journal.ppat.1000104
216. Alanine DGW, Quinkert D, Kumarasingha R, Mehmood S, Donnellan FR, Minkah NK, et al. Human antibodies that slow erythrocyte invasion potentiate malaria-neutralizing antibodies. Cell (2019) 178:216–228.e21. doi: 10.1016/j.cell.2019.05.025
217. Nacer A, Kivi G, Pert R, Juronen E, Holenya P, Aliprandini E, et al. Expanding the malaria antibody toolkit: Development and characterisation of plasmodium falciparum RH5, CyRPA, and CSP recombinant human monoclonal antibodies. Front Cell Infect Microbiol (2022) 12:901253. doi: 10.3389/fcimb.2022.901253
218. Chen E, Paing MM, Salinas N, Sim BK, Tolia NH. Structural and functional basis for inhibition of erythrocyte invasion by antibodies that target plasmodium falciparum EBA-175. PLos Pathog (2013) 9:e1003390. doi: 10.1371/journal.ppat.1003390
219. Woehlbier U, Epp C, Hackett F, Blackman MJ, Bujard H. Antibodies against multiple merozoite surface antigens of the human malaria parasite plasmodium falciparum inhibit parasite maturation and red blood cell invasion. Malar J (2010) 9:77. doi: 10.1186/1475-2875-9-77
220. Han JH, Cheng Y, Muh F, Ahmed MA, Cho JS, Nyunt MH, et al. Inhibition of parasite invasion by monoclonal antibody against epidermal growth factor-like domain of plasmodium vivax merozoite surface protein 1 paralog. Sci Rep (2019) 9:3906. doi: 10.1038/s41598-019-40321-2
221. Lundquist R, Nielsen LK, Jafarshad A, Soesoe D, Christensen LH, Druilhe P, et al. Human recombinant antibodies against plasmodium falciparum merozoite surface protein 3 cloned from peripheral blood leukocytes of individuals with immunity to malaria demonstrate antiparasitic properties. Infect Immun (2006) 74:3222–31. doi: 10.1128/IAI.00928-05
222. Rawlinson TA, Barber NM, Mohring F, Cho JS, Kosaisavee V, Gérard SF, et al. Structural basis for inhibition of plasmodium vivax invasion by a broadly neutralizing vaccine-induced human antibody. Nat Microbiol (2019) 4:1497–507. doi: 10.1038/s41564-019-0462-1
223. Urusova D, Carias L, Huang Y, Nicolete VC, Popovici J, Roesch C, et al. Structural basis for neutralization of plasmodium vivax by naturally acquired human antibodies that target DBP. Nat Microbiol (2019) 4:1486–96. doi: 10.1038/s41564-019-0461-2
224. Chan LJ, Gandhirajan A, Carias LL, Dietrich MH, Vadas O, Visentin R, et al. Naturally acquired blocking human monoclonal antibodies to plasmodium vivax reticulocyte binding protein 2b. Nat Commun (2021) 12:1538. doi: 10.1038/s41467-021-21811-2
225. Hviid L, Lopez-Perez M, Larsen MD, Vidarsson G. No sweet deal: the antibody-mediated immune response to malaria. Trends Parasitol (2022) 38:428–34. doi: 10.1016/j.pt.2022.02.008
226. Maskus DJ, Królik M, Bethke S, Spiegel H, Kapelski S, Seidel M, et al. Characterization of a novel inhibitory human monoclonal antibody directed against plasmodium falciparum apical membrane antigen 1. Sci Rep (2016) 6:39462. doi: 10.1038/srep39462
227. Moreno R, Pöltl-Frank F, Stüber D, Matile H, Mutz M, Weiss NA, et al. Rhoptry-associated protein 1-binding monoclonal antibody raised against a heterologous peptide sequence inhibits plasmodium falciparum growth in vitro. Infect Immun (2001) 69:2558–68. doi: 10.1128/IAI.69.4.2558-2568.2001
228. Knudsen AS, Walker MR, Agullet JP, Björnsson KH, Bassi MR, Barfod L. Enhancing neutralization of plasmodium falciparum using a novel monoclonal antibody against the rhoptry-associated membrane antigen. Sci Rep (2022) 12:3040. doi: 10.1038/s41598-022-06921-1
229. Bull PC, Abdi AI. The role of PfEMP1 as targets of naturally acquired immunity to childhood malaria: prospects for a vaccine. Parasitology (2016) 143:171–86. doi: 10.1017/S0031182015001274
230. Severins M, Klinkenberg D, Heesterbeek H. How selection forces dictate the variant surface antigens used by malaria parasites. J R Soc Interface (2012) 9:246–60. doi: 10.1098/rsif.2011.0239
231. Giha HA, Staalsoe T, Dodoo D, Roper C, Satti GM, Arnot DE, et al. Antibodies to variable plasmodium falciparum-infected erythrocyte surface antigens are associated with protection from novel malaria infections. Immunol Lett (2000) 71:117–26. doi: 10.1016/S0165-2478(99)00173-X
232. Bull PC, Lowe BS, Kortok M, Molyneux CS, Newbold CI, Marsh K. Parasite antigens on the infected red cell surface are targets for naturally acquired immunity to malaria. Nat Med (1998) 4:358–60. doi: 10.1038/nm0398-358
233. Hviid L, Salanti A. VAR2CSA and protective immunity against pregnancy-associated plasmodium falciparum malaria. Parasitology. (2007) 134:1871–6. doi: 10.1017/S0031182007000121
234. Beeson JG, Ndungu F, Persson KE, Chesson JM, Kelly GL, Uyoga S, et al. Antibodies among men and children to placental-binding plasmodium falciparum-infected erythrocytes that express var2csa. Am J Trop Med Hyg (2007) 77:22–8. doi: 10.4269/ajtmh.2007.77.22
235. Ayres Pereira M, Mandel Clausen T, Pehrson C, Mao Y, Resende M, Daugaard M, et al. Placental sequestration of plasmodium falciparum malaria parasites is mediated by the interaction between VAR2CSA and chondroitin sulfate a on syndecan-1. PLos Pathog (2016) 12:e1005831. doi: 10.1371/journal.ppat.1005831
236. Guillotte M, Nato F, Juillerat A, Hessel A, Marchand F, Lewit-Bentley A, et al. Functional analysis of monoclonal antibodies against the plasmodium falciparum PfEMP1-VarO adhesin. Malar J (2016) 15:28. doi: 10.1186/s12936-015-1016-5
237. Tan J, Pieper K, Piccoli L, Abdi A, Perez MF, Geiger R, et al. A LAIR1 insertion generates broadly reactive antibodies against malaria variant antigens. Nature. (2016) 529:105–9. doi: 10.1038/nature16450
238. Raj DK, Nixon CP, Nixon CE, Dvorin JD, DiPetrillo CG, Pond-Tor S, et al. Antibodies to PfSEA-1 block parasite egress from RBCs and protect against malaria infection. Science. (2014) 344:871–7. doi: 10.1126/science.1254417
239. Kurtis JD, Raj DK, Michelow IC, Park S, Nixon CE, McDonald EA, et al. Maternally-derived antibodies to schizont egress antigen-1 and protection of infants from severe malaria. Clin Infect Dis (2019) 68:1718–24. doi: 10.1093/cid/ciy728
240. de Jong RM, Tebeje SK, Meerstein-Kessel L, Tadesse FG, Jore MM, Stone W, et al. Immunity against sexual stage plasmodium falciparum and plasmodium vivax parasites. Immunol Rev (2020) 293:190–215. doi: 10.1111/imr.12828
241. Jones S, Grignard L, Nebie I, Chilongola J, Dodoo D, Sauerwein R, et al. Naturally acquired antibody responses to recombinant Pfs230 and Pfs48/45 transmission blocking vaccine candidates. J Infect (2015) 71:117–27. doi: 10.1016/j.jinf.2015.03.007
242. Coelho CH, Tang WK, Burkhardt M, Galson JD, Muratova O, Salinas ND, et al. A human monoclonal antibody blocks malaria transmission and defines a highly conserved neutralizing epitope on gametes. Nat Commun (2021) 12:1750. doi: 10.1038/s41467-021-21955-1
243. de Jong RM, Meerstein-Kessel L, Da DF, Nsango S, Challenger JD, van de Vegte-Bolmer M, et al. Monoclonal antibodies block transmission of genetically diverse plasmodium falciparum strains to mosquitoes. NPJ Vaccines (2021) 6:101. doi: 10.1038/s41541-021-00366-9
244. van der Boor SC, Smit MJ, van Beek SW, Ramjith J, Teelen K, van de Vegte-Bolmer M, et al. Safety, tolerability, and plasmodium falciparum transmission-reducing activity of monoclonal antibody TB31F: a single-centre, open-label, first-in-human, dose-escalation, phase 1 trial in healthy malaria-naive adults. Lancet Infect Dis (2022) 10:S1473–3099(22)00428-5. doi: 10.1016/S1473-3099(22)00428-5
245. Canepa GE, Molina-Cruz A, Yenkoidiok-Douti L, Calvo E, Williams AE, Burkhardt M, et al. Antibody targeting of a specific region of Pfs47 blocks plasmodium falciparum malaria transmission. NPJ Vaccines (2018) 3:26. doi: 10.1038/s41541-018-0065-5
246. MacDonald NJ, Nguyen V, Shimp R, Reiter K, Herrera R, Burkhardt M, et al. Structural and immunological characterization of recombinant 6-cysteine domains of the plasmodium falciparum sexual stage protein Pfs230. J Biol Chem (2016) 291:19913–22. doi: 10.1074/jbc.M116.732305
247. Chuang YM, Tang XD, Fikrig E. A mosquito AgTRIO monoclonal antibody reduces early plasmodium infection of mice. Infect Immun (2022) 90:e0035921. doi: 10.1128/IAI.00359-21
248. Preiser P, Rénia L, Singh N, Balu B, Jarra W, Voza T, et al. Antibodies against MAEBL ligand domains M1 and M2 inhibit sporozoite development in vitro. Infect Immun (2004) 72:3604–8. doi: 10.1128/IAI.72.6.3604-3608.2004
249. Leite JA, Bargieri DY, Carvalho BO, Albrecht L, Lopes SC, Kayano AC, et al. Immunization with the MAEBL M2 domain protects against lethal plasmodium yoelii infection. Infect Immun (2015) 83:3781–92. doi: 10.1128/IAI.00262-15
250. Espinosa DA, Vega-Rodriguez J, Flores-Garcia Y, Noe AR, Muñoz C, Coleman R, et al. The plasmodium falciparum cell-traversal protein for ookinetes and sporozoites as a candidate for preerythrocytic and transmission-blocking vaccines. Infect Immun (2017) 85:e00498-16. doi: 10.1128/IAI.00498-16
251. Schofield L, Hackett F. Signal transduction in host cells by a glycosylphosphatidylinositol toxin of malaria parasites. J Exp Med (1993) 177:145–53. doi: 10.1084/jem.177.1.145
252. Kobayashi F, Ishida H, Matsui T, Tsuji M. Effects of in vivo administration of anti-IL-10 or anti-IFN-gamma monoclonal antibody on the host defense mechanism against plasmodium yoelii yoelii infection. J Vet Med Sci (2000) 62:583–7. doi: 10.1292/jvms.62.583
253. Lord R, Jones GL, Spencer L, Saul A. Mice immunized with a synthetic peptide construct corresponding to an epitope present on a plasmodium falciparum antigen are protected against plasmodium chabaudi challenge. Parasite Immunol (1993) 15:613–8. doi: 10.1111/j.1365-3024.1993.tb00574.x
254. Theisen M, Soe S, Jessing SG, Okkels LM, Danielsen S, Oeuvray C, et al. Identification of a major b-cell epitope of the plasmodium falciparum glutamate-rich protein (GLURP), targeted by human antibodies mediating parasite killing. Vaccine. (2000) 19:204–12. doi: 10.1016/S0264-410X(00)00181-X
255. Hermsen CC, Verhage DF, Telgt DS, Teelen K, Bousema JT, Roestenberg M, et al. Glutamate-rich protein (GLURP) induces antibodies that inhibit in vitro growth of plasmodium falciparum in a phase 1 malaria vaccine trial. Vaccine. (2007) 25:2930–40. doi: 10.1016/j.vaccine.2006.06.081
256. Dutta S, Tewari A, Balaji C, Verma R, Moitra A, Yadav M, et al. Strain-transcending neutralization of malaria parasite by antibodies against plasmodium falciparum enolase. Malar J (2018) 17:304. doi: 10.1186/s12936-018-2455-6
257. Stone W, Bousema T, Sauerwein R, Drakeley C. Two-faced immunity? the evidence for antibody enhancement of malaria transmission. Trends Parasitol (2019) 35:140–53. doi: 10.1016/j.pt.2018.11.003
258. Scally SW, Murugan R, Bosch A, Triller G, Costa G, Mordmüller B, et al. Rare PfCSP c-terminal antibodies induced by live sporozoite vaccination are ineffective against malaria infection. J Exp Med (2018) 215:63–75. doi: 10.1084/jem.20170869
259. Thai E, Costa G, Weyrich A, Murugan R, Oyen D, Flores-Garcia Y, et al. A high-affinity antibody against the CSP n-terminal domain lacks plasmodium falciparum inhibitory activity. J Exp Med (2020) 217:e20200061. doi: 10.1084/jem.20200061
260. Murugan R, Scally SW, Costa G, Mustafa G, Thai E, Decker T, et al. Evolution of protective human antibodies against plasmodium falciparum circumsporozoite protein repeat motifs. Nat Med (2020) 26:1135–45. doi: 10.1038/s41591-020-0881-9
261. Mikolajczak SA, Vaughan AM, Kangwanrangsan N, Roobsoong W, Fishbaugher M, Yimamnuaychok N, et al. Plasmodium vivax liver stage development and hypnozoite persistence in human liver-chimeric mice. Cell Host Microbe (2015) 17:526–35. doi: 10.1016/j.chom.2015.02.011
262. Gualdrón-López M, Flannery EL, Kangwanrangsan N, Chuenchob V, Fernandez-Orth D, Segui-Barber J, et al. Characterization of plasmodium vivax proteins in plasma-derived exosomes from malaria-infected liver-chimeric humanized mice. Front Microbiol (2018) 9:1271. doi: 10.3389/fmicb.2018.01271
263. White M, Amino R, Mueller I. Theoretical implications of a pre-erythrocytic plasmodium vivax vaccine for preventing relapses. Trends parasitol (2017) 33:260–3. doi: 10.1016/j.pt.2016.12.011
264. Baird JK. 8-aminoquinoline therapy for latent malaria. Clin Microbiol Rev (2019) 32:e00011–19. doi: 10.1128/CMR.00011-19
265. Hounkpatin AB, Kreidenweiss A, Held J. Clinical utility of tafenoquine in the prevention of relapse of plasmodium vivax malaria: a review on the mode of action and emerging trial data. Infection Drug Resistance (2019) 12:553. doi: 10.2147/IDR.S151031
266. Leitao R, Rodriguez A. Inhibition of plasmodium sporozoites infection by targeting the host cell. Exp parasitol (2010) 126:273–7. doi: 10.1016/j.exppara.2010.05.012
267. Lopes da Silva M, Thieleke-Matos C, Cabrita-Santos L, Ramalho JS, Wavre-Shapton ST, Futter CE, et al. The host endocytic pathway is essential for plasmodium berghei late liver stage development. Traffic. (2012) 13:1351–63. doi: 10.1111/j.1600-0854.2012.01398.x
268. Raphemot R, Toro-Moreno M, Lu KY, Posfai D, Derbyshire ER. Discovery of druggable host factors critical to plasmodium liver-stage infection. Cell Chem Biol (2019) 26:1253–62. doi: 10.1016/j.chembiol.2019.05.011
269. Posfai D, Sylvester K, Reddy A, Ganley JG, Wirth J, Cullen QE, et al. Plasmodium parasite exploits host aquaporin-3 during liver stage malaria infection. PLos pathogens (2018) 14:e1007057. doi: 10.1371/journal.ppat.1007057
270. Posfai D, Maher SP, Roesch C, Vantaux A, Sylvester K, Péneau J, et al. Plasmodium vivax liver and blood stages recruit the druggable host membrane channel aquaporin-3. Cell Chem Biol (2020) 27:719–27. doi: 10.1016/j.chembiol.2020.03.009
271. Kain HS, Glennon EK, Vijayan K, Arang N, Douglass AN, Fortin CL, et al. Liver stage malaria infection is controlled by host regulators of lipid peroxidation. Cell Death Differentiation (2020) 27:44–54. doi: 10.1038/s41418-019-0338-1
272. Kaushansky A, Metzger PG, Douglass AN, Mikolajczak SA, Lakshmanan V, Kain HS, et al. Malaria parasite liver stages render host hepatocytes susceptible to mitochondria-initiated apoptosis. Cell Death disease. (2013) 4:e762. doi: 10.1038/cddis.2013.286
273. Boulet C, Siddiqui G, Gaynor TL, Doerig C, Creek DJ, Carvalho TG. Red blood cell BCL-xL is required for plasmodium falciparum survival: Insights into host-directed malaria therapies. Microorganisms. (2022) 10:824. doi: 10.3390/microorganisms10040824
274. Ebert G, Lopaticki S, O’Neill MT, Steel RW, Doerflinger M, Rajasekaran P, et al. Targeting the extrinsic pathway of hepatocyte apoptosis promotes clearance of plasmodium liver infection. Cell Rep (2020) 30:4343–54. doi: 10.1016/j.celrep.2020.03.032
275. Crosnier C, Bustamante LY, Bartholdson SJ, Bei AK, Theron M, Uchikawa M, et al. Basigin is a receptor essential for erythrocyte invasion by plasmodium falciparum. Nature. (2011) 480:534–7. doi: 10.1038/nature10606
276. Egan ES, Jiang RH, Moechtar MA, Barteneva NS, Weekes MP, Nobre LV, et al. A forward genetic screen identifies erythrocyte CD55 as essential for plasmodium falciparum invasion. Science. (2015) 348:711–4. doi: 10.1126/science.aaa3526
277. Bhalla K, Chugh M, Mehrotra S, Rathore S, Tousif S, Prakash Dwivedi V, et al. Host ICAMs play a role in cell invasion by mycobacterium tuberculosis and plasmodium falciparum. Nat Commun (2015) 6:1–3. doi: 10.1038/ncomms7049
278. Doerig C, Abdi A, Bland N, Eschenlauer S, Dorin-Semblat D, Fennell C, et al. Malaria: targeting parasite and host cell kinomes. Biochim Biophys Acta (BBA)-Proteins Proteomics (2010) 1804:604–12. doi: 10.1016/j.bbapap.2009.10.009
279. Sicard A, Semblat JP, Doerig C, Hamelin R, Moniatte M, Dorin-Semblat D, et al. Activation of a PAK-MEK signalling pathway in malaria parasite-infected erythrocytes. Cell Microbiol (2011) 13:836–45. doi: 10.1111/j.1462-5822.2011.01582.x
280. Smith CM, Jerkovic A, Puy H, Winship I, Deybach JC, Gouya L, et al. Red cells from ferrochelatase-deficient erythropoietic protoporphyria patients are resistant to growth of malarial parasites. Blood J Am Soc Hematol (2015) 125:534–41. doi: 10.1182/blood-2014-04-567149
281. Brizuela M, Huang HM, Smith C, Burgio G, Foote SJ, McMorran BJ. Treatment of erythrocytes with the 2-cys peroxiredoxin inhibitor, conoidin a, prevents the growth of plasmodium falciparum and enhances parasite sensitivity to chloroquine. PLos One (2014) 9:e92411. doi: 10.1371/journal.pone.0092411
282. Jezewski AJ, Lin YH, Reisz JA, Culp-Hill R, Barekatain Y, Yan VC, et al. Targeting host glycolysis as a strategy for antimalarial development. Front Cell infection Microbiol (2021) 11:730413. doi: 10.3389/fcimb.2021.730413
283. Moreno M, Gonzalez V M. Advances on aptamers targeting plasmodium and trypanosomatids. Curr medicinal Chem (2011) 18:5003–10. doi: 10.2174/092986711797535218
284. Achtman AH, Pilat S, Law CW, Lynn DJ, Janot L, Mayer ML, et al. Effective adjunctive therapy by an innate defense regulatory peptide in a preclinical model of severe malaria. Sci Trans Med (2012) 4:135ra64. doi: 10.1126/scitranslmed.3003515
285. Hawkes M, Opoka RO, Namasopo S, Miller C, Thorpe KE, Lavery JV, et al. Inhaled nitric oxide for the adjunctive therapy of severe malaria: protocol for a randomized controlled trial. Trials (2011) 12:1–3. doi: 10.1186/1745-6215-12-176
286. Lu Z, Serghides L, Patel SN, Degousee N, Rubin BB, Krishnegowda G, et al. Disruption of JNK2 decreases the cytokine response to plasmodium falciparum glycosylphosphatidylinositol in vitro and confers protection in a cerebral malaria model. J Immunol (2006) 177:6344–52. doi: 10.4049/jimmunol.177.9.6344
287. Liu M, Solomon W, Cespedes JC, Wilson NO, Ford B, Stiles JK. Neuregulin-1 attenuates experimental cerebral malaria (ECM) pathogenesis by regulating ErbB4/AKT/STAT3 signaling. J neuroinflamm (2018) 15:1–5. doi: 10.1186/s12974-018-1147-z
288. Brooks HM, Hawkes MT. Repurposing pharmaceuticals as neuroprotective agents for cerebral malaria. Curr Clin Pharmacol (2017) 12:62–72. doi: 10.2174/1574884712666170704144042
289. Joice R, Nilsson SK, Montgomery J, Dankwa S, Egan E, Morahan B, et al. Plasmodium falciparum transmission stages accumulate in the human bone marrow. Sci Trans Med (2014) 6:244re5. doi: 10.1126/scitranslmed.3008882
290. Brancucci NM, Gerdt JP, Wang C, De Niz M, Philip N, Adapa SR, et al. Lysophosphatidylcholine regulates sexual stage differentiation in the human malaria parasite plasmodium falciparum. Cell. (2017) 171:1532–44. doi: 10.1016/j.cell.2017.10.020
291. Foote SJ. Can nature's defence against malaria be mimicked by the development of host-directed therapies. Pharmacogenomics. (2004) 4:141–2. doi: 10.1038/sj.tpj.6500241
292. Goh YS, McGuire D, Rénia L. Vaccination with sporozoites: Models and correlates of protection. Front Immunol (2019) 10:1227. doi: 10.3389/fimmu.2019.01227
Keywords: Plasmodium, immune evasion, immunotherapeutics, vaccine candidates, antibody therapy, host-directed therapy
Citation: Chandley P, Ranjan R, Kumar S and Rohatgi S (2023) Host-parasite interactions during Plasmodium infection: Implications for immunotherapies. Front. Immunol. 13:1091961. doi: 10.3389/fimmu.2022.1091961
Received: 07 November 2022; Accepted: 12 December 2022;
Published: 04 January 2023.
Edited by:
Rahul Shivahare, The Ohio State University, United StatesReviewed by:
Manish Goyal, Boston University, United StatesRajni Kant Shukla, The Ohio State University, United States
Copyright © 2023 Chandley, Ranjan, Kumar and Rohatgi. This is an open-access article distributed under the terms of the Creative Commons Attribution License (CC BY). The use, distribution or reproduction in other forums is permitted, provided the original author(s) and the copyright owner(s) are credited and that the original publication in this journal is cited, in accordance with accepted academic practice. No use, distribution or reproduction is permitted which does not comply with these terms.
*Correspondence: Soma Rohatgi, c29tYS5yb2hhdGdpQGJ0LmlpdHIuYWMuaW4=