- 1Department of Emergency Medicine, Xinqiao Hospital, Army Medical University, Chongqing, China
- 2Department of Microbiology, College of Basic Medical Sciences, Army Medical University, Chongqing, China
Burkholderia pseudomallei is the causal agent of melioidosis, a deadly tropical infectious disease that lacks a vaccine. On the basis of the attenuated Staphylococcus aureus RN4220-Δagr (RN), we engineered the RN4220-Δagr/pdhB-hcp1 strain (RN-Hcp1) to generate B. pseudomallei hemolysin-coregulated protein 1 (Hcp1)-loaded membrane vesicles (hcp1MVs). The immunization of BALB/c mice with hcp1MVs mixed with adjuvant by a three-dose regimen increased the serum specific IgG production. The serum levels of inflammatory factors, including TNF-α and IL-6, in hcp1MV-vaccinated mice were comparable with those in PBS-challenged mice. The partial adjuvant effect of staphylococcal MVs was observed with the elevation of specific antibody titer in hcp1MV-vaccinated mice relative to those that received the recombinant Hcp1 protein (rHcp1) or MVs derived from RN strain (ΔagrMVs). The hcp1MVs/adjuvant vaccine protected 70% of mice from lethal B. pseudomallei challenge. Immunization with hcp1MVs only protected 60% of mice, whereas vaccination with rHcp1 or ΔagrMVs conferred no protection. Moreover, mice that received hcp1MVs/adjuvant and hcp1MVs immunization had low serum TNF-α and IL-6 levels and no inflammatory infiltration in comparison with other groups. In addition, all surviving mice in hcp1MVs/adjuvant and hcp1MVs groups exhibited no culturable bacteria in their lungs, livers, and spleens five days postinfection. Overall, our data highlighted a new strategy for developing B. pseudomallei vaccine and showed that Hcp1-incorporated staphylococcal MV is a promising candidate for the prevention of acute melioidosis.
Introduction
Burkholderia pseudomallei is a dangerous pathogen that causes melioidosis, a tropical infectious disease with clinical manifestation varying from local abscess to systemic sepsis (1). Human melioidosis was first described in 1911 by Dr. Alfred Whitmore in Yangon, Myanmar, and this disease has been neglected until 1985 when the Infectious Disease Association of Thailand has enabled people to recognize melioidosis as a remarkable public health problem (2, 3). The worldwide distribution of B. pseudomallei especially in tropical areas such as South America and the Caribbean, Southeast Asia, Northern Australia, and the Indian subcontinent, results in an estimate of 165,000 human melioidosis cases per year and a high mortality of 53.9% (2–5). B. pseudomallei can colonize and invade multiple organs of the body, including brain, lung, liver, kidney, and skin, and the extensive tissue tropism of B. pseudomallei contributes to the development of melioidosis (6, 7) and complicates the diagnosis due to the variable clinical presentations of the disease (2). Moreover, B. pseudomallei is intrinsically resistant to a variety of antibiotics (2, 8), and insufficient antibacterial treatment can lead to a fatality rate of more than 70% (9, 10). In addition, B. pseudomallei has been recognized as a category B biological agent by the US Centers for Disease Control and Prevention (11). The high prevalence and potential biological threat of B. pseudomallei calls for effective vaccines. However, commercial vaccines against melioidosis are still unavailable.
Substantial effort has been given to the development of melioidosis vaccines in recent years. Preclinical studies showed that inactivated whole-cell (such as paraformaldehyde-killed B. pseudomallei A2 (12)) and live attenuated (like the auxotrophic mutant ΔilvI (13)) vaccines can protect 20%–80% of vaccinated mice from lethal bacterial challenge. However, sterilizing immunity is not achieved (8). By contrast, subunit vaccines with one or several antigens are considered safe and protective. Therefore, the selection and assessment of potential antigen candidates from B. pseudomallei have attracted the attention of the research community. Many antigens, such as the lipoprotein export system component LolC (14), outer membrane protein OmpW (15), heat shock protein GroEL (16), trimeric autotransporter adhesin PSL2063 (17), type III secreted protein BopA (18), and the type VI secretion system (T6SS)-associated hemolysin-coregulated proteins (Hcp; including Hcp1, Hcp2, Hcp3, and Hcp6) (19), have been identified as good vaccine candidates for further investigation. However, these subunit vaccine candidates have not advanced to clinical trials due to lack of suitable delivery systems.
Bacterial membrane vesicles (MVs) are nanoscale structures naturally secreted by Gram-positive bacteria and Gram-negative ones (also termed outer membrane vesicles, OMVs) during their growth (20). MVs can incorporate bacterial proteins, which may effectively stimulate specific humoral and cellular immune responses, and grant remarkable protection against subsequent bacterial infections (21). The innate characteristics of bacterial MVs support vaccine candidates and provide excellent vaccine delivery systems (22, 23). Examples of the successful bacterial MV vaccines are two licensed meningococcal serogroup B (MenB) MV vaccines designated as MenB-4C and MenB-FHbp, which can protect against infections caused by all 14 pathogenic meningococcal strains tested (24). A safe staphylococcal platform has been constructed previously by deleting the whole agr locus in the genome of Staphylococcus aureus strain RN4220, and at least four dominant components in the RN4220-Δagr mutant are capable of delivering the antigens of dengue virus to MVs, which can induce specific antibodies against all four serotypes of the dengue virus (25).
As one of the subunit vaccine candidates against melioidosis, Hcp1 is a structural protein forming the secretion tube of T6SS and an important effector involving in B. pseudomallei pathogenesis (26). Anti-Hcp1 specific antibodies, including IgM and IgG, exist in the sera of patients with melioidosis, indicating the development of humoral response against Hcp1 (26, 27). In the present study, the hcp1 gene is genetically in-frame fused to the gene encoding pyruvate dehydrogenase E1 component subunit beta (PdhB), one of the aforementioned major vesicular components in S. aureus RN4220-Δagr. The immunization of BALB/c mice with Hcp1-loaded MVs derived from RN4220-Δagr/pdhB-hcp1 (hcp1MVs) induces a high titer of specific antibodies and protects about 70% of vaccinated mice from acute intraperitoneal challenge with lethal B. pseudomallei, highlighting a technical advancement in developing melioidosis vaccines.
Materials and methods
Plasmids, bacterial strains, and their growth conditions
The plasmids and bacterial strains used in this study were listed in Supplementary Table 1. S. aureus strains were cultured from a freezer stock and streaked on tryptic soy broth (TSB; Oxoid, UK) agar for 16 h at 37°C. The colonies of bacteria were inoculated into 2 mL of TSB and incubated for 16 h at 37°C with agitation. Escherichia coli strains were grown in Luria Broth (LB) medium or plated on LB agar for 16 h at 37°C. B. pseudomallei strains were cultured from a freezer stock and spread on LB agar supplemented with 200 μM FeSO4·7H2O for 48 h at 37°C. Bacterial colonies were then inoculated into 2 mL of LB broth and cultured at 37°C for 16 h with agitation. When required, cultures were supplemented with 100 μg/mL of kanamycin (Kan) or 50 μg/mL of ampicillin (Amp) for E. coli and 10 μg/mL of chloramphenicol (Cm) for S. aureus harboring recombinant plasmids.
The plasmid pBT2 and S. aureus RN4220 were kindly provided by Prof. Baolin Sun (University of Science and Technology of China). B. pseudomallei strain BPC006 was isolated from the clinical specimen of a patient hospitalized in Hainan People’s Hospital (28), and kindly provided by Prof. Xu-hu Mao (Army Medical University).
Construction of S. aureus strain producing Hcp1-loaded MVs
To construct S. aureus RN4220-Δagr/pdhB-hcp1, the hcp1 gene was amplified from B. pseudomallei BPC006 genomic DNA using primer pair hcp1-F/R (Supplementary Table 2). For homologous recombination, the left region (Up-pdhB) and right region (Down-pdhB) across the stop codon of the pdhB gene of S. aureus RN4220-Δagr were amplified from the genomic DNA using primers uppdhB-F/R and downpdhB-F/R (Supplementary Table 2), respectively. A fusion fragment was generated by overlap PCR using the hcp1 gene fragment, Up-pdhB, and Down-pdhB as templates. The fusion DNA was digested with BamH I/Sac I restriction enzymes and ligated into the temperature-sensitive shuttle vector pBT2 via Gibson assembly master mix (NEB, USA). The resultant vector pBT2-hcp1 was firstly transformed into E. coli DH5α and subsequently electroporated into S. aureus strain RN4220-Δagr. The seamless hcp1 insertion mutant designated as RN4220-Δagr/pdhB-hcp1 (RN-Hcp1) was screened as previously described (29), and confirmed through PCR amplification and DNA sequencing.
Preparation of bacterial MVs
Bacterial MVs were prepared from S. aureus culture supernatants as described previously (25). Briefly, overnight culture of S. aureus strains of interest was inoculated into 1 L TSB (1:100), and incubated for 20 h at 37°C with shaking (200 rpm). Culture supernatants were collected by centrifugation at 5,000 × g at 4°C for 30 min to remove bacterial cells, and then filtered through 0.45 μm Millex syringe filters (Beyotime) to remove any remaining cell debris. The filtrate was concentrated about 60 times with an ultrafiltration system with an interception molecular weight of 100 kDa (Shenchen, China). Culture supernatants were then centrifuged at 200,000 × g for 3 h at 4°C, and the MV pellets were resuspended in phosphate-buffered saline (PBS, pH 7.2) after two washes with PBS. The protein concentration of MV samples was determined using an enhanced BCA Protein Assay Kit according to the instruction provided by the Company (Beyotime). MVs were stored as aliquots at -80°C.
Transmission electron microscope (TEM) observation
The purified Hcp1-loaded MVs were added to 230-mesh formvar/carbon-coated copper grids (Zhongjingkeji Tech., China), and negatively stained with 2% (m/v) uranylacetate for 30 sec. For immunogold labeling, Hcp1-loaded MVs was 1:20 diluted in NTE buffer (10 mM Tris-Cl, pH 7.0, 100 mM NaCl) and adsorbed onto 230-mesh formvar/carbon-coated nickel grids. After 5 min wash with TBS buffer (50 mM Tris-HCl, 150 mM NaCl, pH 7.5), the specimen was blocked with 3% (m/v) bovine serum albumin (BSA) in TBS for 45 min. Mouse-anti-Hcp1 antibody was 1:500 diluted in 1% (m/v) BSA/TBS and applied to the nickel grids for 1.0 h at room temperature. After washed three times with TBS, gold-conjugated goat-anti-mouse IgG (Sigma, USA) was added and incubated for 1.0 h at room temperature. Next, the grids were washed twice with TBS, once with water and followed by negatively stained with 2% (m/v) uranylacetate for 15 sec. Electron micrographs were recorded with a JEM1011 microscope (JEOL, Japan) at 100 kV acceleration voltage.
Expression and purification of recombinant Hcp1
Recombinant Hcp1 (rHcp1) was expressed and purified as described previously with minor modifications (25). Briefly, the hcp1 gene was amplified from genomic DNA of B. pseudomallei BPC006 using primer pair pET28a-F/R (Supplementary Table 2), and cloned into the expression vector pET28a to generate pET28a-hcp1. E. coli strain BL21(DE3) harbouring the resulting plasmid pET28a-hcp1 was grown in 1.0 L fresh LB broth at 37°C to an OD600 of 0.5 and induced with 0.5 mM IPTG at 37°C for 6 h. For protein purification, bacterial cells were harvested by centrifugation at 5,000 × g at 4°C for 30 min and lysed by sonication in buffer A (50 mM Tris-HCl and 200 mM NaCl, pH 8.0). After centrifugation at 16,000 × g at 4°C for 30 min to remove cell debris, the supernatant was collected and centrifuged again at 10,000 × g for 30 min, filtered through a 0.22 mm filter unit (Beyotime), and applied to an Ni-NTA resin column (GE, USA) pre-equilibrated with buffer A. The column was washed with buffer A, followed by buffer A mixed with a linear gradient of 5%–100% (v/v) buffer B (buffer A containing 500 mM imidazole) to remove the nonspecifically bound proteins and elute the target proteins. Protein purity was analyzed by sodium dodecyl sulfate polyacrylamide gel electrophoresis (SDS-PAGE), and the major fractions containing target proteins were collected and dialyzed five times against buffer A to remove imidazole. The concentration of rHcp1 proteins was determined using a BCA protein assay kit (Pierce). Aliquots of the purified rHcp1 proteins were stored at -80°C.
SDS-PAGE and Western blot
Protein samples were solubilized in 5 × SDS-PAGE sample buffer and heated to 100°C for 10 min. After centrifugation, the sample supernatants were loaded and proteins were separated on 12% SDS-PAGE. Proteins were visualized via staining with Coomassie blue R-250. For Western blot, proteins on the PAGE gel were electrotransferred onto a PVDF membrane (Beyotime). The membrane was blocked with 5% skim milk in high-salt Tris-buffered saline (HS-TBS; 20 mM Tris, 500 mM NaCl, pH 7.5) for 60 min at room temperature. Then, the membrane was incubated overnight at 4°C with a 1:10,000 dilution of the B. pseudomallei Hcp1-specific polyclonal antibody. After washed five times with PBS-T (PBS containing 0.05% Tween-20), the membrane was incubated for 1.0 h at 37°C with 1:5,000 of goat-anti-mouse IgG-horseradish peroxidase conjugate (Solarbio). Blot was visualized using the Pierce ECL Western blotting substrate (Thermo Scientific) and a ChemiDoc XRS imaging system (Bio-Rad).
Median lethal dose (LD50) determination
Female BALB/c mice (aged 6–8 weeks) were purchased from the Animal Center of Army Medical University (Third Military Medical University) and acclimated in an ABLS-2+ laboratory for five days before experiments. All mice were fed an autoclaved pellet diet and sterile water under the following housing conditions: 23°, 50% humidity, half-day light/dark periods, and quiet surroundings with minimal disturbance. The experimental procedures for animals were performed according to the Regulations for Administration of Affairs Concerning Experimental Animals approved by Chinese State Council. Cervical dislocation was applied as euthanasia way of the experimental animals.
For LD50 determination, 50 female mice were challenged through intraperitoneal route with different concentrations of B. pseudomallei strain BPC006 (3.0 × 105, 6.0 × 105, 1.0 × 106, 3.0 × 106, and 6.0 × 106 CFU, n = 10 per group). Infected mice were monitored for survival over 21 days, and the LD50 was calculated using Bliss method by SPSS software.
Mouse vaccination and infection
Anesthetized BALB/c mice were grouped and administered in multisite routes (i.e., subcutaneous, intramuscular, and intraperitoneal) by three-dose vaccination regimens with (i) 100 µL PBS, (ii) 50 µg ΔagrMVs (in 100 µL PBS) prepared from S. aureus RN4220-Δagr (RN), (iii) 50 µg hcp1MVs derived from S. aureus RN-Hcp1, (iv) 50 µg hcp1MVs emulsified with 50% Freund’s adjuvant (Sigma, USA), and (v) 0.2 µg rHcp1 proteins (equal to the amount of Hcp1 in 50 µg hcp1MVs) on days -30, -20 and -10 prechallenge. Ten days after the third boost (day 0), the vaccinated mice were infected via intraperitoneal with lethal dose (5 × LD50) of B. pseudomallei BPC006. Challenged mice were monitored for behavior changes and survival time over 21 days.
Detection of B. pseudomallei specific antibodies
During the process of mouse vaccination, sera from immunized mice (n = 3) seven days after the last vaccination (day -3 prechallenge) were collected, and antibodies against B. pseudomallei Hcp1 were determined by enzyme-linked immunosorbent assay (ELISA) as described (30). Briefly, 96-well Maxisorp plates (Nunc) were coated overnight at 4°C with rHcp1 (50 ng/mL) solubilized in carbonate buffer (pH 9.6). The plates were blocked at room temperature for 1.0 h with StartingBlock T20 blocking buffer (Pierce). After blocking, plates were washed three times with TBS-T (Tris-buffered saline supplemented 10% StartingBlock T20 and 0.05% Tween 20, pH 7.5). Then, twofold dilutions of serum samples were made with TBS-T, added in triplicate to wells, and incubated for 2.0 h at 37°C. After washed three times with TBS-T, the plates were incubated for 1.0 h at 37°C with goat anti-mouse IgG-horseradish peroxidase conjugate (1:5,000 dilution, Solarbio). After incubation, the plates were washed three times with TBS-T and developed by the EL-ABTS Chromogenic Reagent kit (Sangon Biotech) and read at 405 nm by using a FLUOstar Omega microplate reader (BMG Labtech). The reciprocals of the highest dilutions exhibiting optical densities (OD) that were 2.1 times relative to the normal mouse serum levels were used to determine the endpoint titers of antibodies for individual vaccinated mice.
Detection of inflammatory factors
Serum samples were collected from individual vaccinated mice at 6 h after prime vaccination (day -30), days -20, -10, and -3 prechallenge, and days 0.5, 1, 2, and 5 postchallenge (n = 3 per time point). Then, the levels of inflammatory factors TNF-α and IL-6 in each serum samples were determined with an ELISA kit according to the manufacturer’s instructions (R&D Systems, USA). Results were examined with an ELISA reader Multiskan Mk3 (Thermo Fisher Scientific) at 450 nm, and inflammatory factor levels were determined according to the standard curve.
Bacterial burden in organs of infected mice
The organs, including lungs, livers, and spleens, were collected from vaccinated mice on day 0.5 and 5 postchallenge, and subjected to colony forming unit (CFU) enumeration with a plate dilution method. Briefly, vaccinated mice (n = 50 for PBS group, n = 25 per other groups) were infected via intraperitoneal with lethal dose (5 × LD50) of B. pseudomallei BPC006 on day 0. Twelve infected mice on day 0.5 were sacrificed for organ bacterial counting (n = 10) and histological evaluation (n = 2). The remained mice were sacrificed on day 5 postchallenge. For bacterial counting, mouse organs were harvested, weighed, and homogenized in PBS using Covidien Precision tissue grinders (Fisher Scientific). Tissue homogenates were tenfold diluted in PBS, plated on LB agar, and incubated for 48 h at 37°C. Colonies were counted.
Histological evaluation of mouse organs
The lungs, livers, and spleens collected from vaccinated mice (n = 2) on day 0.5 and 5 postchallenge were used for histological evaluation. Individual organ was fixed with 10% normal buffered formalin. The fixed tissues were embedded in paraffin and sectioned prior to staining with hematoxylin-eosin (HE). Tissue sections were observed under a microscope (Olympus BX53, Japan) and photographed.
Statistical analysis
All experiments were conducted at least three times. Statistical analysis was performed using GraphPad Prism 8.0 software. The one-way analysis of variance (ANOVA), or two-way ANOVA method was used to compare the means from multiple groups and assess the statistical significance. All values were expressed as the mean ± standard derivations (SD) or standard error of the means (SEM). Significant difference was expressed as *P < 0.05, **P < 0.01, or ***P < 0.001.
Results
Construction of S. aureus strain producing Hcp1-loaded membrane vesicles
The hcp1 gene of B. pseudomallei strain BPC006 was amplified with PCR and genetically fused to the 3′-terminus of the pdhB gene in S. aureus mutant RN4220-Δagr (RN) to load Hcp1 into staphylococcal MVs. The in-frame fusion of target genes was verified by PCR amplification and DNA sequencing (Supplementary Figure 1), and the resultant strain was designated as RN4220-Δagr/pdhB-hcp1 (RN-Hcp1).
The MVs produced by engineered S. aureus RN-Hcp1 (hcp1MVs) were prepared, and Western blot revealed the existence of a successful fusion protein with molecular weight of approximately 57 kDa in the total cell lysates of RN-Hcp1 and hcp1MVs by using PdhB polyclonal antibodies as probe. S. aureus mutant RN and its derived ΔagrMVs presented wild-type PdhB with a low molecular weight (37 kDa, Figure 1A and Supplementary Figure 2). When polyclonal antibodies against Hcp1 were used, the protein of interest was only detected in the total cell lysates of RN-Hcp1 and hcp1MVs but not in the RN mutant and ΔagrMVs (Figure 1B). TEM revealed the varied sizes of the purified MVs (Supplementary Figure 3A), and immuno-electron microscopy showed the membrane location of Hcp1 proteins (Supplementary Figures 3B, C). These data indicated that the engineered S. aureus RN-Hcp1 could successfully produce Hcp1-loaded MVs.
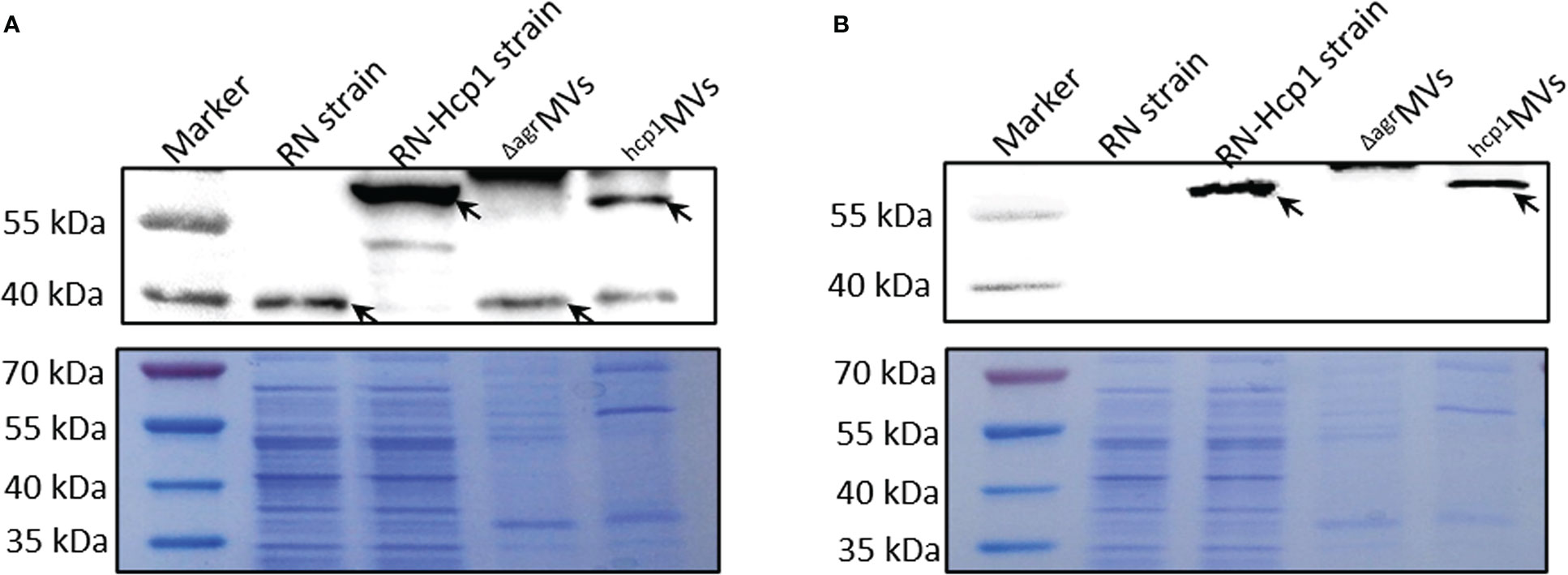
Figure 1 Characterization of PdhB-Hcp1 fusion proteins in S. aureus RN-Hcp1 strain and its MVs. Western blot with anti-PdhB (A) and anti-Hcp1 (B) antibodies, respectively. The top panels showed the blots of target proteins (indicated by black arrows) in bacterial cell lysates of S. aureus RN and RN-Hcp1 strains and their MVs, including ΔagrMVs and hcp1MVs. The proteins in samples of interest were separated by 12% SDS-PAGE, stained with Coomassie blue R-250 and served as loading controls (bottom panels). The sizes of the protein Marker were indicated on the left.
General manifestation and humoral immune response in mice after Hcp1-loaded MV immunization
BALB/c mice were immunized with PBS, ΔagrMVs, hcp1MVs, hcp1MVs/adjuvant, and recombinant Hcp1 (rHcp1, n = 10 per group) by a three-dose regimen at 10-day intervals via multiple routes (i.e., subcutaneous, intramuscular, and intraperitoneal) to assess the effects of Hcp1-loaded MVs on tested animals (Figure 2A). The body weights of MVs- or rHcp1-challenged mice showed no difference during the immunization process compared with those of the PBS-challenged mice (Figure 2B). All vaccinated mice exhibited good mental state, smooth fur, normal food and water intake, normal posture and gait, and comparable nutrition. Sera from vaccinated mice (n = 3 per group) on day -3 prechallenge were used to determine antibody responses to rHcp1 by using indirect ELISA. Results revealed that the titer of the total serum IgG antibodies from hcp1MVs/adjuvant-immunized mice was highest among all groups (Figure 2C). The sera from mice that received a prime and two boosts of hcp1MVs vaccination demonstrated higher IgG titers than those from rHcp1- and ΔagrMVs-vaccinated mice (Figure 2C), suggesting a partial adjuvant effect of staphylococcal MVs.
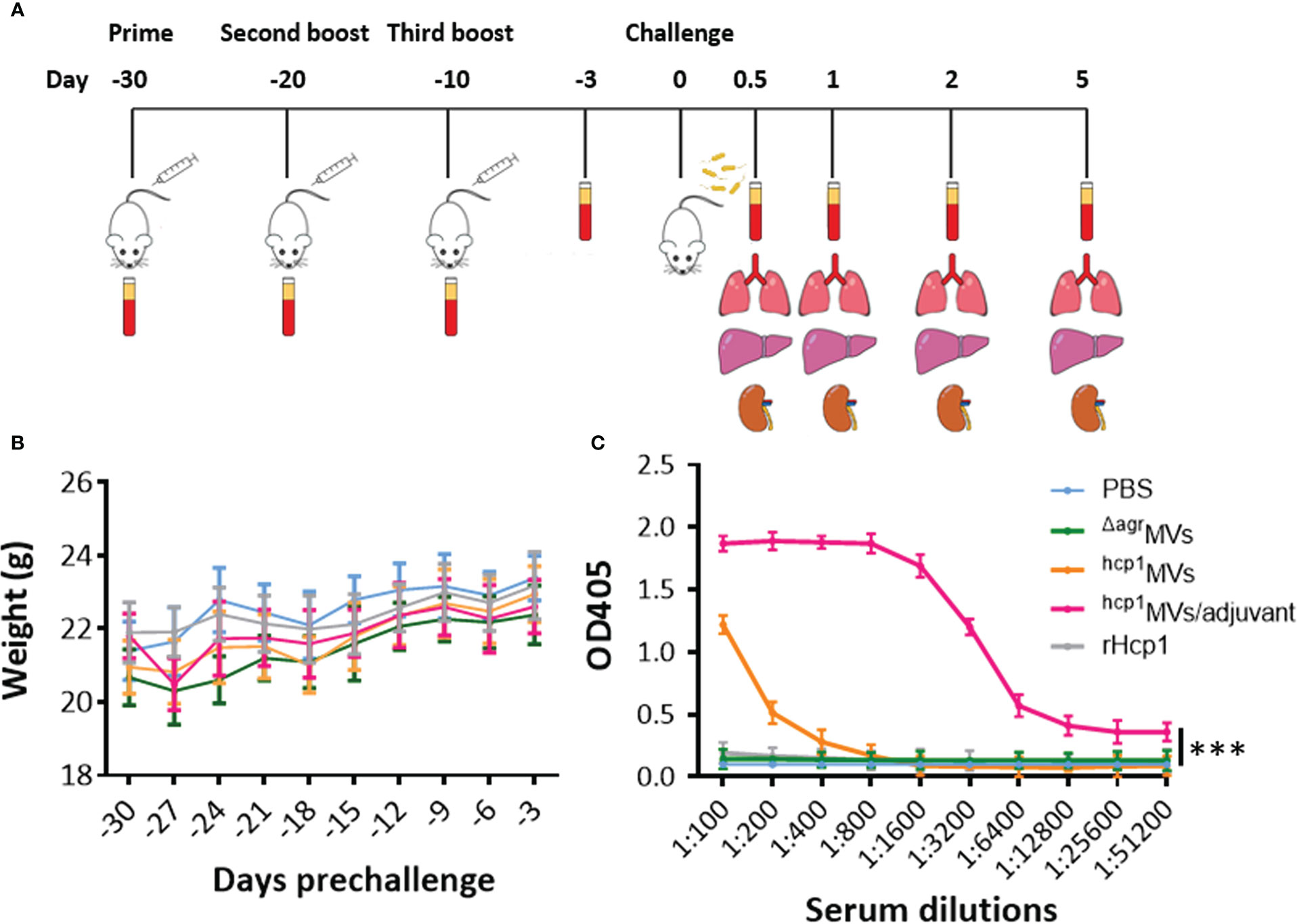
Figure 2 Body weight dynamics and serum antibody response of the vaccinated mice. (A) Experimental design and timeline of the regimen of PBS, ΔagrMVs, hcp1MVs, hcp1MVs/adjuvant, and rHcp1 vaccination, bacterial challenge, and sample collection. (B) Body weight dynamics of the vaccinated mice. Immunized BALB/c mice (n = 10 per group) were weighed every three days, and the weight of all groups increased gradually during vaccination. (C) Quantitation of serum antibody titers raised against rHcp1. The serum IgG antibody titers of immunized mice (n = 3 per group) on day -3 prechallenge were determined by ELISA and defined as the highest serum dilution that exhibited an optical density (OD) value of 2.1 times relative to the negative control (PBS group serum). Statistical significance was calculated by two-way ANOVA, ***P < 0.001.
Inflammatory factor production following Hcp1-loaded MV immunization
The levels of inflammatory factor production present the systemic inflammation of body caused by bacteria or toxins (31). However, certain antigens or adjuvants can activate immune cells to secrete cytokines, such as TNF-α, IL-6, and IL-12, that are involved in the promotion of Th1 immune responses (32). Sera from BALB/c mice vaccinated with PBS, ΔagrMVs, hcp1MVs, hcp1MVs/adjuvant, and rHcp1 (n = 3 per time point) were collected at 6 h after prime vaccination (day -30) and days -20, -10, and -3 preinfection, and subjected to quantification of IL-6 and TNF-α levels by using ELISA. As shown in Figure 3A, the IL-6 levels in mice 6 h after prime vaccination (day -30) with ΔagrMVs, hcp1MVs, and hcp1MVs/adjuvant were significantly increased compared with those in PBS- and rHcp1-vaccinated mice (P < 0.001). The TNF-α level in immunized mice 6 h after prime vaccination of hcp1MVs/adjuvant and ΔagrMVs remarkably increased relative to that in PBS-challenged mice, whereas comparable TNF-α level was observed in mice 6 h after prime vaccination of hcp1MVs and rHcp1. Furthermore, the IL-6 and TNF-α levels of ΔagrMVs-, hcp1MVs-, hcp1MVs/adjuvant-, and rHcp1-injected group on days -20, -10, and -3 were low and comparable with those of PBS-injected group (Figure 3B). These data indicated that the Hcp1-loaded MV vaccine was safe and only elicited increased inflammatory factor production during early stage of prime immunization.
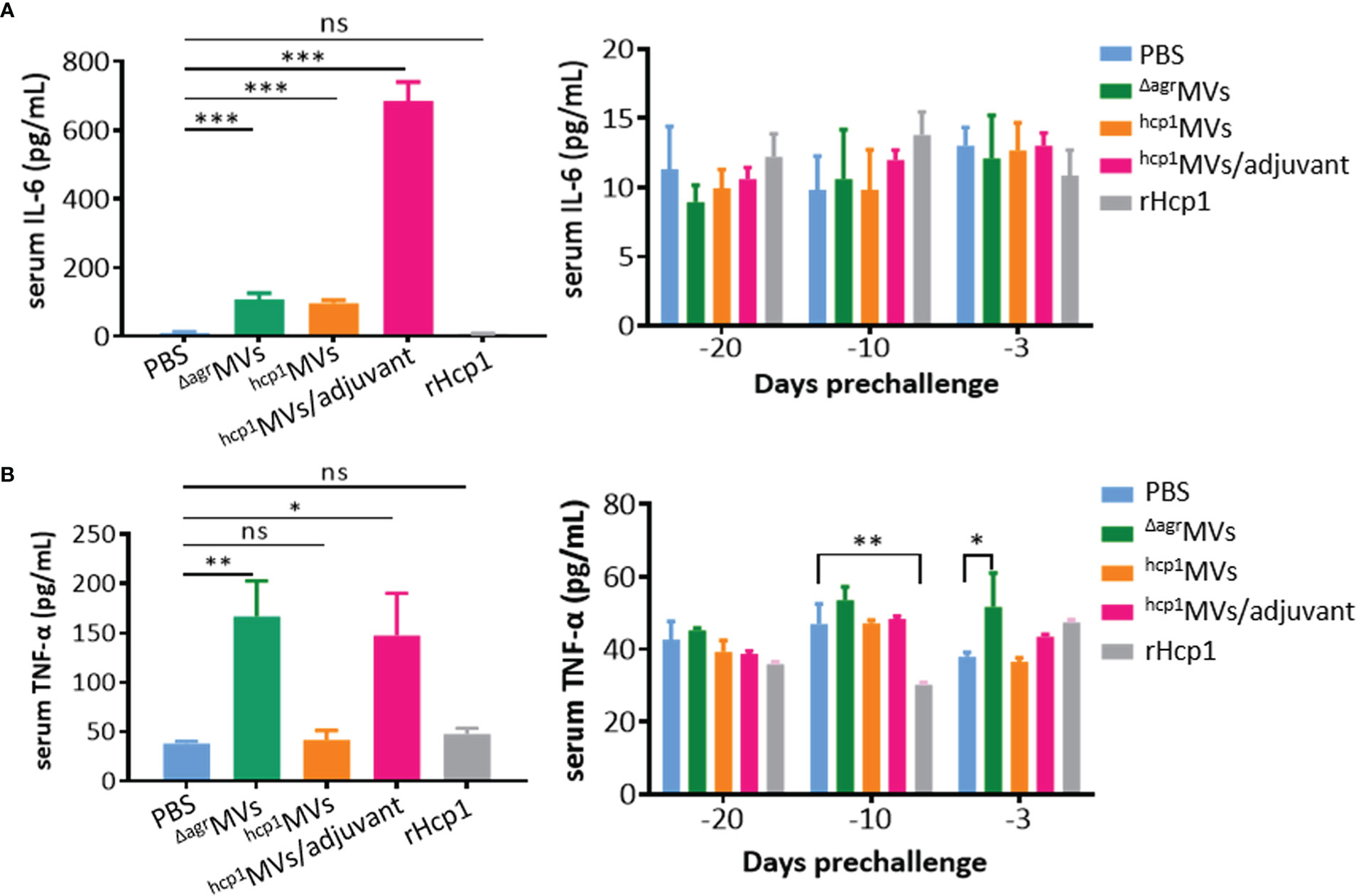
Figure 3 Inflammatory factor production determined by ELISA. BALB/c mice (n = 3 per group) were each vaccinated with PBS, ΔagrMVs, hcp1MVs, hcp1MVs/adjuvant, or rHcp1 on days -30, -20, and -10. Serum samples were collected at 6 h after prime vaccination (day -30, left panels) and days -20, -10 and -3 preinfection (right panels). ELISA was performed to quantitate serum IL-6 (A) and TNF-ɑ (B) levels with commercial kits (R&D Systems). The assay was repeated in triplicate. Statistical significances were calculated by one-way ANOVA; ns indicates no statistical significance, *P < 0.05, **P < 0.01, and ***P < 0.001.
Protective capacity of Hcp1-loaded MV vaccine against B. pseudomallei infection
The median lethal dose (LD50) was determined to evaluate the protective repertoire of Hcp1-loaded MV against B. pseudomallei challenge. Female BALB/c mice were challenged intraperitoneally with various dosages of B. pseudomallei BPC006 (3.0 × 105, 6.0 × 105, 1.0 × 106, 3.0 × 106, and 6.0 × 106 CFU). Mouse survival was monitored for 21 days postinfection, and the Bliss method of SPSS software showed that the LD50 was 5.75 × 105 CFU (Supplementary Figure 4). BALB/c mice were vaccinated with PBS, ΔagrMVs, hcp1MVs, hcp1MVs/adjuvant, or rHcp1 (n = 10 per group) by a three-dose regimen at 10-day intervals and then infected with lethal dose of B. pseudomallei BPC006 (5 × LD50) 10 days after the final boost to evaluate the protective capacity of the MV vaccine. All infected mice in the PBS-treated group succumbed rapidly to the lethal challenge of B. pseudomallei BPC006 (< 7 days). ΔagrMVs- or rHcp1-vaccinated mice survived less than 20 days after B. pseudomallei infection. By contrast, immunization with hcp1MVs and hcp1MVs/adjuvant protected 60% and 70% of mice, respectively, from lethal bacterial challenge with surviving time exceeding 21 days (Figure 4).
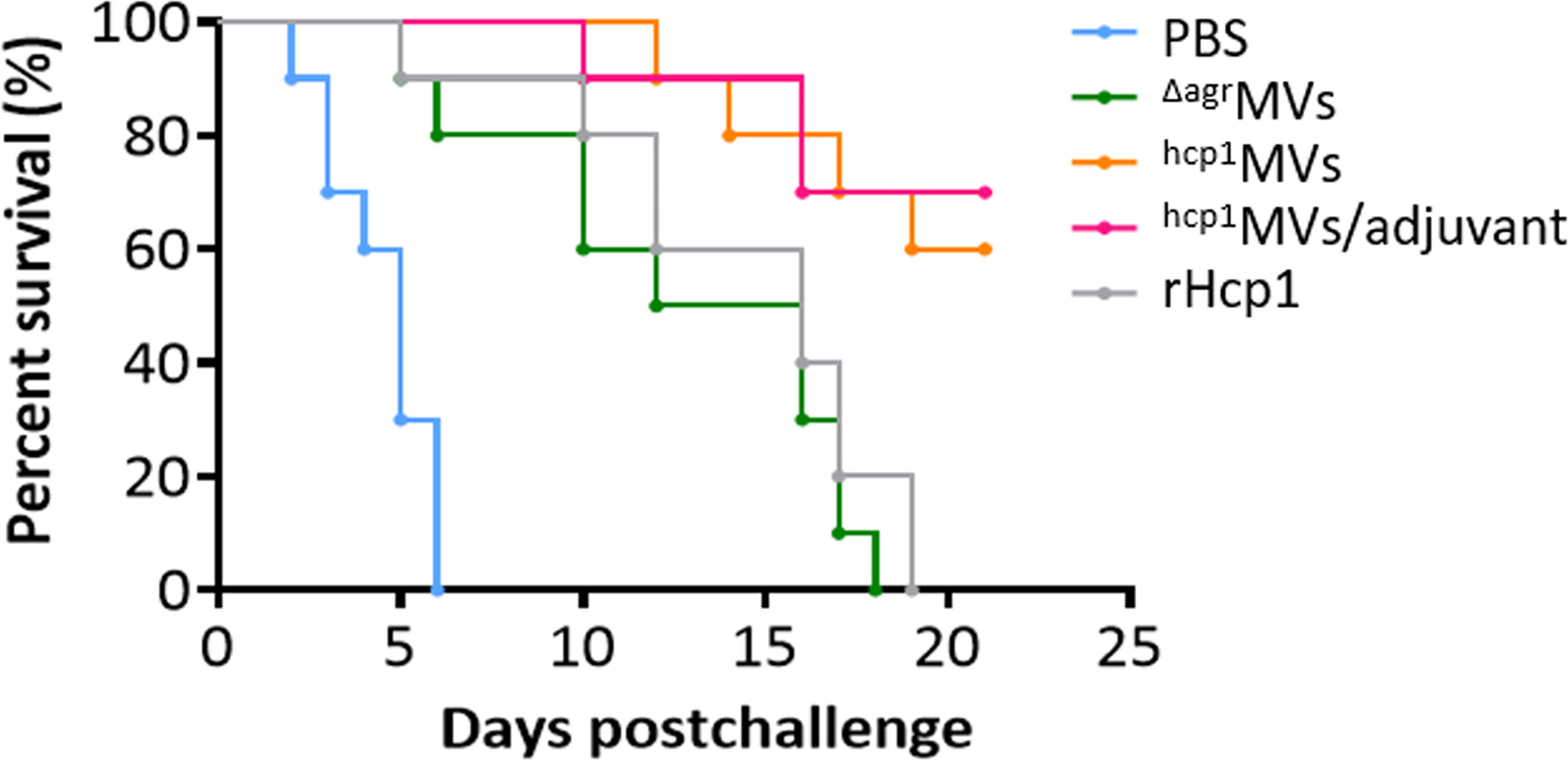
Figure 4 Hcp1-loaded MV vaccination protects mice from acute melioidosis. BALB/c mice (n = 10 per group) were vaccinated with PBS, ΔagrMVs, hcp1MVs, hcp1MVs/adjuvant, or rHcp1 by a three-dose regimen at 10-day intervals. Then, mice were infected with 5 × LD50 of B. pseudomallei BPC006 (lethal dose) 10 days after the final boost. Mouse mortality was evaluated daily for 21 days and the percent survival (%) was indicated.
Inflammatory cytokine production in vaccinated mice after B. pseudomallei infection
Serum samples of vaccinated mice on days 0.5, 1, 2, and 5 postinfection of B. pseudomallei BPC006 were collected and subjected to the determination of inflammatory cytokine levels. As shown in Figure 5, the levels of TNF-α and IL-6 in PBS-treated mice five days after lethal bacterial exposure were significantly elevated than those in other groups and increased over time. By contrast, the TNF-α and IL-6 levels in hcp1MVs- and hcp1MVs/adjuvant-immunized mice five days postchallenge decreased continuously after day 0.5 of infection (except TNF-α). Notably, inflammatory cytokine levels in the hcp1MVs/adjuvant-vaccinated mice increased remarkably after day 0.5 of exposure, decreased rapidly, and then maintained at a low level. The increased inflammatory cytokine levels during early period after B. pseudomallei exposure may contribute to bacterial clearance (32).
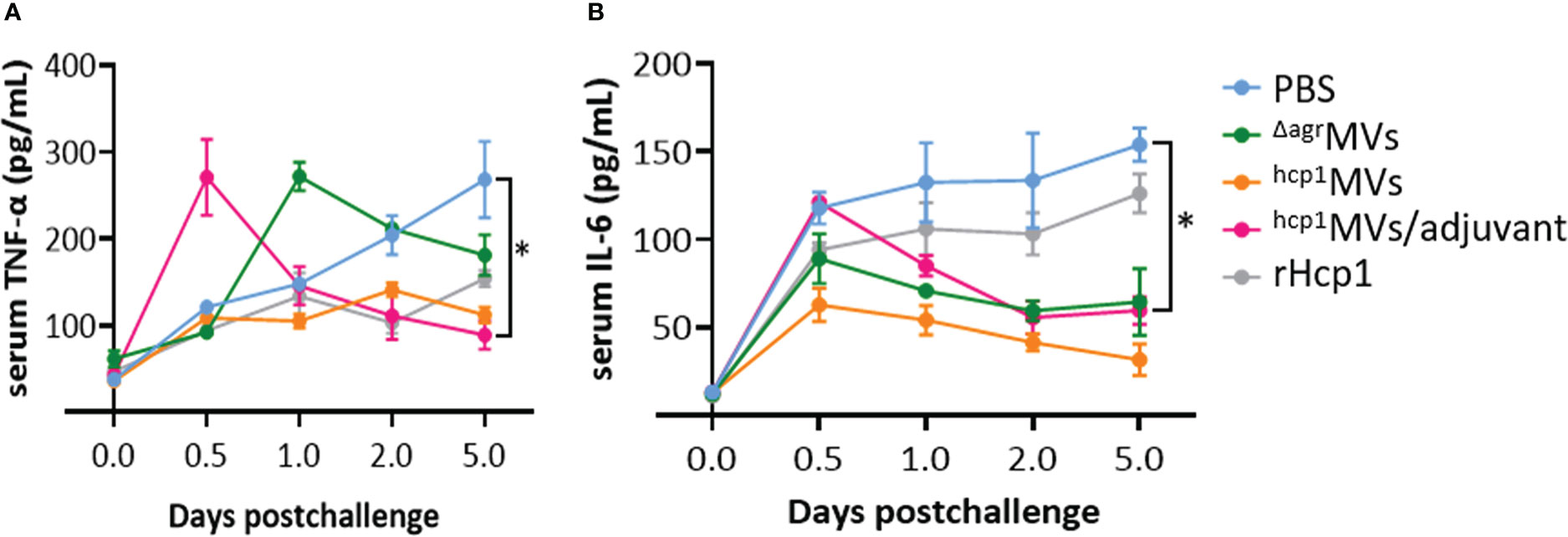
Figure 5 The inflammatory cytokine levels in sera of vaccinated mice after B. pseudomallei challenge. Mice (n = 3 per group) were vaccinated with PBS, ΔagrMVs, hcp1MVs, hcp1MVs/adjuvant, or rHcp1 by three inoculations at 10-day intervals. Ten days after the final boost, mice were infected with lethal dose of B. pseudomallei BPC006 (5 × LD50) via intraperitoneal injection. Serum samples were harvested on days 0.5, 1, 2, and 5 postinfection and the levels of inflammatory cytokines, including TNF-α (A) and IL-6 (B), were determined by ELISA. Data are presented as mean ± SD. Statistical significance was calculated by two-way ANOVA, *P < 0.05.
Bacterial burden in vaccinated mice after bacterial challenge
Powerful vaccines resulted in bacterial clearance in vivo. BALB/c mice were vaccinated with PBS, ΔagrMVs, hcp1MVs, hcp1MVs/adjuvant, or rHcp1 (n = 9 per group), and tissue samples of lungs, livers, and spleens were obtained from immunized mice on day 0.5 and 5 postchallenge with lethal B. pseudomallei. Bacterial enumeration revealed that the viable bacteria in collected organs of the hcp1MVs- and hcp1MVs/adjuvant-immunized mice on day 0.5 postchallenge were decreased than those of other groups (Figure 6A). Moreover, hcp1MVs- and hcp1MVs/adjuvant- immunized mice demonstrated undetectable viable bacteria in the three tissues on day 5 postinfection (Figure 6B). Results also showed that all mice immunized with hcp1MVs or hcp1MVs/adjuvant exhibited no detectable bacteria in their tissues on day 5 postinfection (Figure 6B). These data indicated that Hcp1-loaded MV vaccine is a promising candidate for B. pseudomallei clearance and protects against acute melioidosis.
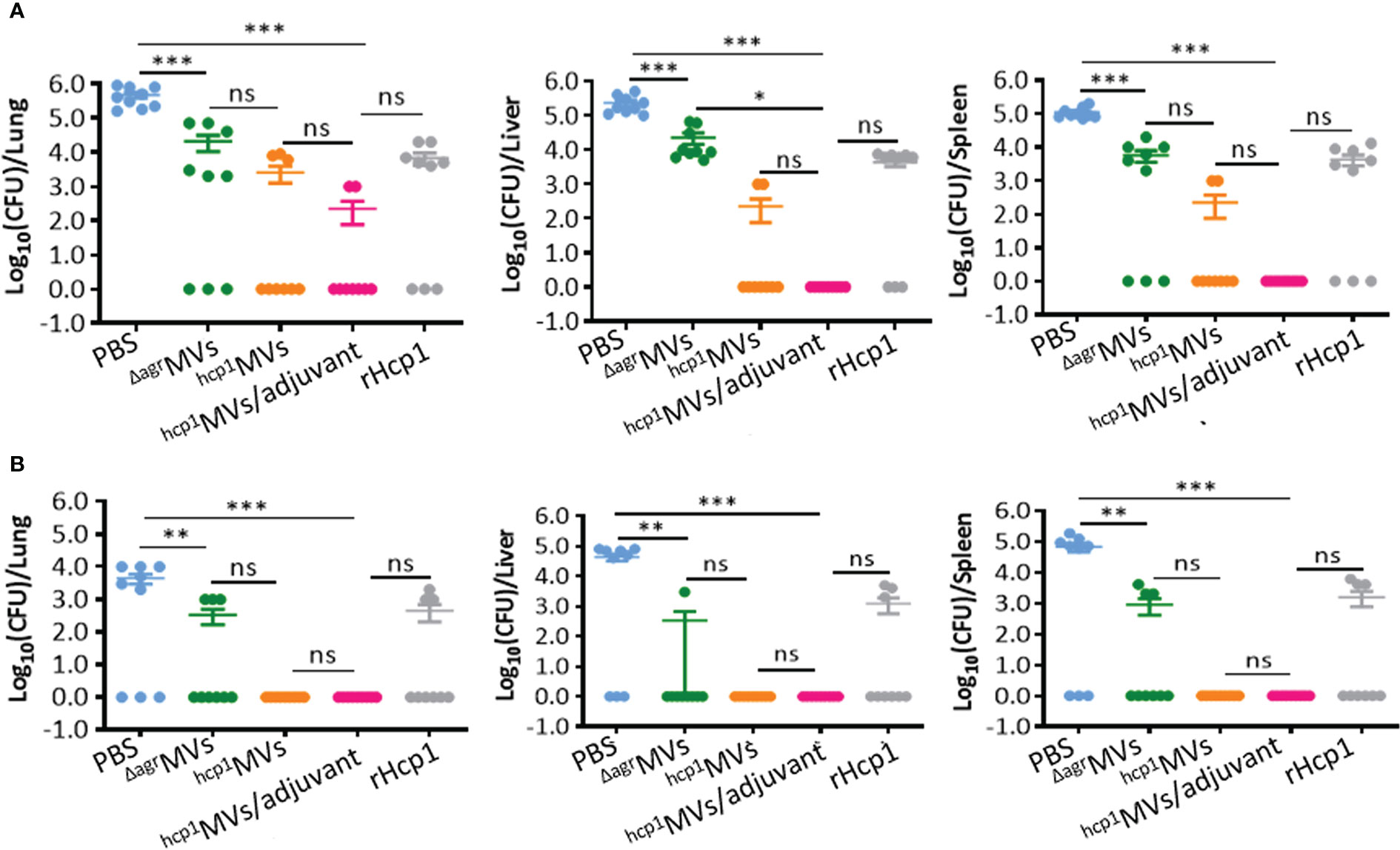
Figure 6 Bacterial enumeration in vaccinated mice challenged with B. pseudomallei. Viable bacteria in lungs, livers, and spleens of immunized mice (n = 9 per group) on day 0.5 (A) and 5 (B) postinfection. Tissues were weighed and homogenized in PBS. Viable bacteria were counted using the plate dilution method and calculated as CFU. Data are presented as mean ± standard error of the mean (SEM). Significant differences were analyzed by one-way ANOVA, ns indicates no difference, *P < 0.05, **P < 0.01, and ***P < 0.001.
Histopathological analysis of infected mouse tissues
The lungs, livers, and spleens of mice immunized with PBS, ΔagrMVs, hcp1MVs, hcp1MVs/adjuvant, or rHcp1 (n = 2 per group) were collected on day 0.5, 1, 2, and 5 postchallenge with B. pseudomallei BPC006 and subjected to histopathological analysis. In the gross view, the normal morphologies of livers, spleens, and lungs were maintained in hcp1MVs/adjuvant-vaccinated mice after five days of infection. However, small white abscesses formed in the livers, spleens, and lungs of PBS-immunized mice five days postinfection and enlarged spleens and lungs with mucinous exudate on the surface were also observed (Supplementary Figure 5).
Tissue sections, including lungs, livers, and spleens, were prepared, stained with hematoxylin and eosin (HE), and examined in a blind manner by a pathologist. Histopathological analysis revealed that the lungs, livers, and spleens from hcp1MVs/adjuvant-immunized mice on day 5 postinfection exhibited normal tissue architecture (Figure 7). By contrast, the lungs, livers, and spleens of animals vaccinated with hcp1MVs were almost normal except for some areas of mild interstitial inflammation. The bronchovascular inflammatory infiltrations were observed in lungs of ΔagrMVs- or rHcp1-vaccinated mice on the indicated days postchallenge (Supplementary Figure 6). Bronchovascular infiltration was a prevalent characteristic in the lungs of mice vaccinated with PBS. In addition, frequent interstitial inflammation and multinucleated giant cell infiltration were also observed in the spleens of PBS-vaccinated mice five days after bacterial challenge (Figure 7). Overall, these data demonstrated the vaccinogenic potential of Hcp1-loaded staphylococcal MVs.
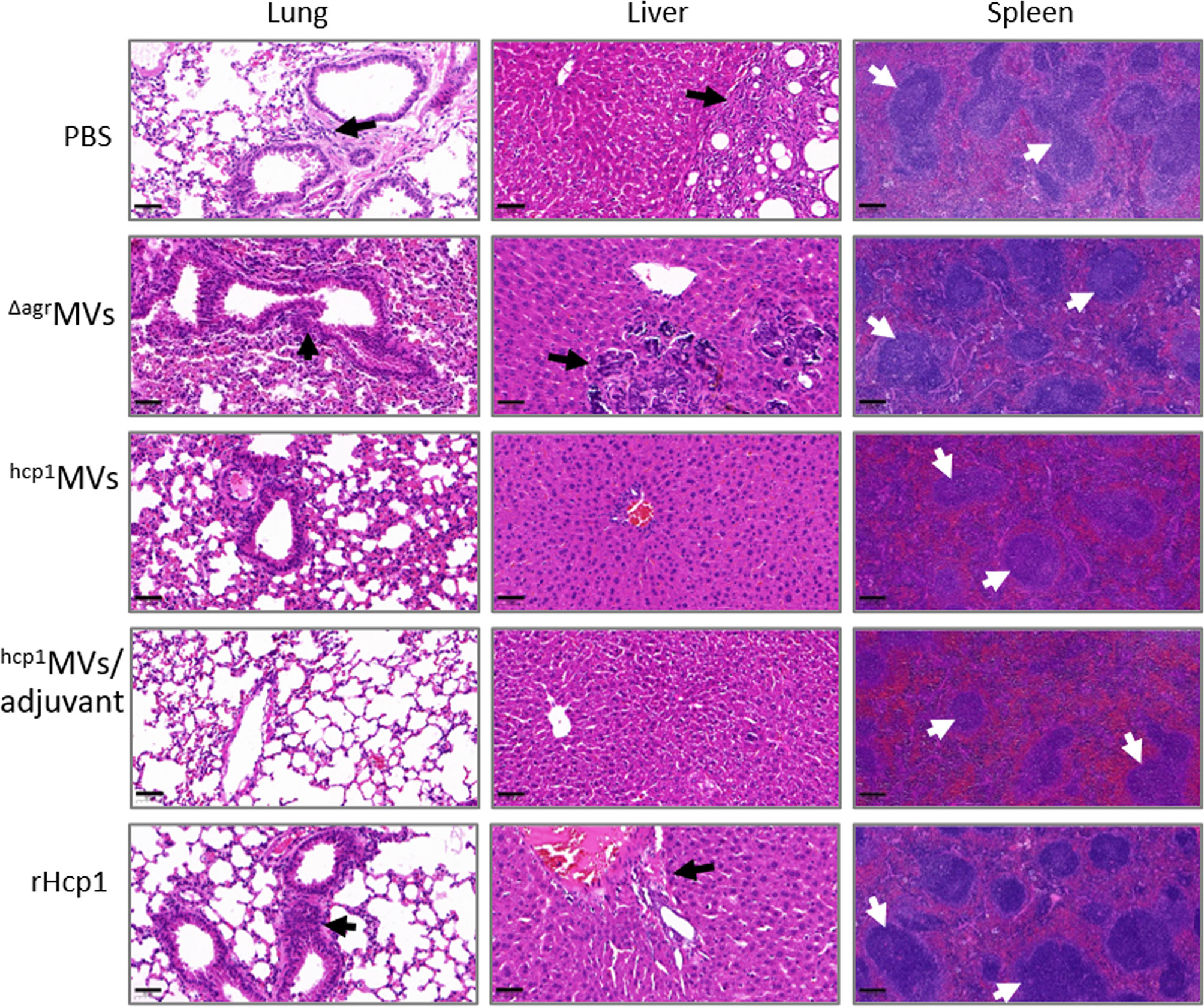
Figure 7 Reduced organ inflammations in vaccinated mice after exposure to B. pseudomallei. The lungs, livers, and spleens (n = 2 per group) of vaccinated mice on day 5 postchallenge were collected and fixed in 10% formalin. Then, tissues were paraffin-embedded, sectioned, stained with hematoxylin and eosin, and subjected to pathological examination. The representative field for certain tissues was observed at 20× (bar = 50 µm, lung and liver) and 10× (bar = 200 µm, spleen) magnifications under a microscope. White arrows indicated multinucleated giant cells, whereas black arrows showed inflammatory infiltrations.
Discussion
Despite the fact that licensed vaccines are unavailable, many vaccine candidates against melioidosis, including inactivated whole-cell (32, 33), live attenuated (13, 34), subunit (14, 15), glycoconjugate (35, 36), DNA (37, 38), and viral vector-based (39) vaccines, have been studied in recent years. However, these vaccines only have partial protection probably because B. pseudomallei is an intracellular organism that survives in macrophages, neutrophils, and monocytes, thus avoiding the induction of protective immune responses (40–43). Nieves et al. prepared MVs naturally derived from B. pseudomallei strain 1026b (44) and showed that the vaccination of BALB/c mice with MVs provides considerable protection against septic (100%) and pneumonic (60%) melioidosis (44, 45). However, natural MVs are commonly enriched with virulence factors, such as lipopolysaccharide (21, 25), and the application of MVs produced by wild-type B. pseudomallei strains raises safety concerns. In a previous study, a whole gene locus deletion of agr system is used to generate an S. aureus strain RN4220-Δagr (25). MVs prepared from RN4220-Δagr (ΔagrMVs) are remarkably attenuated, and PdhB is the most abundant component among ΔagrMVs that is suitable for the delivery of heterogeneous antigens from bacteria to the secreted MVs. On the basis of this safe protein delivery platform, the gene of B. pseudomallei protective antigen Hcp1 is genetically fused with pdhB in S. aureus RN4220-Δagr, and the protective immune responses generated by hcp1MVs vaccination against acute melioidosis are evaluated in the present study.
Recent studies showed that antibody responses play a critical role in the protection of live attenuated vaccine-vaccinated mice from B. pseudomallei infection and that bacterium-specific cellular responses make a minor contribution (34, 46). In this study, vaccination with rHcp1 proteins without adjuvants in a three-dose regimen has produced indistinguishable level of antibodies compared with the PBS control (Figure 2C), suggesting the requirement of immune adjuvants, such as Freund’s adjuvant (47), Sigma adjuvant system (19), and CpG DNA (37), in subunit vaccine immunization. By contrast, the vaccination of mice with Hcp1-loaded MVs (hcp1MVs) in the absence of adjuvants produces considerable humoral responses (serum IgG titer > 1:400) compared with the vaccination of mice with ΔagrMVs that do not encapsulate Hcp1 (Figure 2C), indicating an adjuvant effect of staphylococcal ΔagrMVs for Hcp1. However, the adjuvant effect of ΔagrMVs remains partial because the immunization of mice with a formulation of hcp1MVs/adjuvant induces high specific antibodies (IgG titer > 1:51,200, Figure 2C). The self-adjuvant activity of MVs is probably due to the presence of vesicle-related pathogen-associated molecular patterns (PAMPs), which can bind pathogen recognition receptors on innate immune cells to enhance antigen-presenting functions (48). The MV adjuvant activity is remarkably correlated with the type and amount of PAMPs incorporated in vesicles (48, 49). As heterogeneous MVs for B. pseudomallei Hcp1, PAMPs and their functions in staphylococcal ΔagrMVs deserve further investigation.
As a subunit vaccine, rHcp1 is previously prepared and mixed with Sigma adjuvant, and BALB/c mice are vaccinated by three inoculations of 10 µg rHcp1 proteins at 2-week intervals and infected with B. pseudomallei K96243 strain three weeks after the final boost. Results showed that the rHcp1/adjuvant can protect 50% of mice from lethal dose challenge but fails to prevent chronic colonization (19). In this study, the inability to prevent acute melioidosis is observed after vaccination of BALB/c mice with ΔagrMVs or rHcp1 protein only (Figure 4). Notably, a similar post-challenge survival pattern was observed between rHcp1- and ΔagrMVs- immunized mice. Vaccination of rHcp1 without adjuvant formulation can enhance the post-challenge survival of BALB/c mice against B. pseudomallei. S. aureus derived ΔagrMVs do not have specific antigens of B. pseudomallei, however, ΔagrMVs can exhibit intrinsic adjuvant activity (25). The PAMPs involved in ΔagrMVs may activate innate immune cells, such as dendritic cells and monocytes/macrophages, to provide certain roles in pathogen inactivation (48). By contrast, engineered hcp1MVs protects 60% of BALB/c mice against lethal challenge with BPC006, and the formulation of hcp1MVs/adjuvant protects 70% of mice against acute melioidosis. Compared with the rHcp1 protein/adjuvant formulation (19), the elevated protective ability of hcp1MVs may be ascribed to the intrinsic adjuvanticity of staphylococcal MVs. PAMPs encapsulated in bacterial MVs can also activate immune cells to secrete cytokines and promote a Th1 immune response (32). Consistently, the serum levels of TNF-α and IL-6 in mice 6 h after prime vaccination (day -30) with hcp1MVs/adjuvant are significantly increased compared with those in the PBS control group. The serum TNF-α and IL-6 levels in mice vaccinated with ΔagrMVs are also remarkably increased and then gradually decreased to normal levels on days -20, -10, and -3 prechallenge (Figure 3). This result is consistent with the finding in the previous study that ΔagrMVs inoculation induces lower level of inflammatory factor production than MVs derived from the wild-type S. aureus strain (25). However, the inflammatory cytokine levels, especially TNF-α, in the hcp1MVs/adjuvant-vaccinated mice increased remarkably on day 0.5 after B. pseudomallei exposure (Figure 5). The increased inflammatory cytokine levels during early period after infection may contribute to bacterial clearance (32), which is partially confirmed by the bacterial counting from infected mouse organs (Figure 6A). By contrast, hcp1MVs-vaccinated animals have low serum TNF-α and IL-6 levels throughout the experimental period. The reason behind this phenomenon is unclear, and the association between hcp1MV-stimulated inflammatory factor level and its protective potential is of interest and worthy of further study.
A major challenge in developing vaccines against diseases caused by facultative intracellular pathogens is the ability of the host to achieve sterilizing immunity (50). Importantly, vaccination with hcp1MVs or hcp1MVs/adjuvant can achieve sterilizing immunity. Culturable bacteria are not determined in lungs, livers, and spleens of hcp1MVs- or hcp1MVs/adjuvant-vaccinated mice on day 5 postinfection of B. pseudomallei BPC006, whereas most PBS-inoculated mice (66.7%) have bacterial burden in their organs (Figure 6B). In addition, the organ tissue histopathology of vaccinated mice after challenge with B. pseudomallei was assessed, and data showed decreased histopathological changes, reduced interstitial inflammation foci, and declined multinucleated giant cell numbers in hcp1MVs- and hcp1MVs/adjuvant- vaccinated mice compared with those in PBS-injected mice (Figure 7). These discrete organ bacterial burdens and histopathological observations indicate that the vaccination of Hcp1-loaded MVs contributes to the induction of specific immune responses that control the infection and prevent disseminated melioidosis disease in various organs. Our observations correlate well with the previous finding that pathogen clearance is observed in B. mallei live attenuated vaccine-vaccinated mice after challenge with bacteria (88% clearance) (34).
In conclusion, our data demonstrate that Hcp1, a tube component and secreted effector of T6SS in B. pseudomallei, is an effective vaccine candidate and that engineered S. aureus RN4220-Δagr/pdhB-hcp1 can successfully produce hcp1MVs that have protection potential against B. pseudomallei acute infections. We have reached an interesting partial adjuvant activity of staphylococcal MVs. The addition of an adjuvant to hcp1MVs will further improve the protection ability and stimulate an enhanced sterilizing immunity for complete bacterial clearance. Overall, our results present a new way to develop melioidosis vaccines and provide valuable insights into the development of an antigenically defined, safe, and effective MV vaccine.
Data availability statement
The original contributions presented in the study are included in the article/Supplementary Material. Further inquiries can be directed to the corresponding authors.
Ethics statement
The animal study was reviewed and approved by Third Military Medical University.
Author contributions
XR, RZ, and ML designed experiments, and revised manuscript. KZ, GL, MZ, XP, and JL performed experiments and interpreted data. KZ, GL, and YR investigated data and drew figures. XR and YR edited manuscript. All authors reviewed drafts and approved the submitted version.
Funding
This work was supported by the National Natural Science Foundation of China (82071857 and 81971565).
Acknowledgments
We thank Xu-hu Mao and Cheng-long Rao (Department of Clinical Microbiology and Immunology, College of Medical Laboratory, Army Medical University) for performance of animal experiment.
Conflict of interest
The authors declare that the research was conducted in the absence of any commercial or financial relationships that could be construed as a potential conflict of interest.
Publisher’s note
All claims expressed in this article are solely those of the authors and do not necessarily represent those of their affiliated organizations, or those of the publisher, the editors and the reviewers. Any product that may be evaluated in this article, or claim that may be made by its manufacturer, is not guaranteed or endorsed by the publisher.
Supplementary material
The Supplementary Material for this article can be found online at: https://www.frontiersin.org/articles/10.3389/fimmu.2022.1089225/full#supplementary-material
References
1. Birnie E, Virk HS, Savelkoel J, Spijker R, Bertherat E, Dance DAB, et al. Global burden of melioidosis in 2015: a systematic review and data synthesis. Lancet Infect Dis (2019) 19(8):892902. doi: 10.1016/S1473-3099(19)30157-4
2. Limmathurotsakul D, Golding N, Dance DA, Messina JP, Pigott DM, Moyes CL, et al. Predicted global distribution of Burkholderia pseudomallei and burden of melioidosis. Nat Microbiol (2016) 1:15008. doi: 10.1038/nmicrobiol.2015.8
3. Dance DA, Limmathurotsakul D. Global burden and challenges of melioidosis. Trop Med Infect Dis (2018) 3(1):13. doi: 10.3390/tropicalmed3010013
4. Suputtamongkol Y, Chaowagul W, Chetchotisakd P, Lertpatanasuwun N, Intaranongpai S, Ruchutrakool T, et al. Risk factors for melioidosis and bacteremic melioidosis. Clin Infect Dis (1999) 29(2):408413. doi: 10.1086/520223
5. Trinh TT, Nguyen LDN, Nguyen TV, Tran CX, Le AV, Nguyen HV, et al. Melioidosis in Vietnam: Recently improved recognition but still an uncertain disease burden after almost a century of reporting. Trop Med Infect Dis (2018) 3(2):39. doi: 10.3390/tropicalmed3020039
6. Wiersinga WJ, Virk HS, Torres AG, Currie BJ, Peacock SJ, Dance DAB, et al. Melioidosis. Nat Rev Dis Primers (2018) 4:17107. doi: 10.1038/nrdp.2017.107
7. Aziz A, Currie BJ, Mayo M, Sarovich DS, Price EP. Comparative genomics confirms a rare melioidosis human-to-human transmission event and reveals incorrect phylogenomic reconstruction due to polyclonality. Microb Genom (2020) 6(2):e000326. doi: 10.1099/mgen.0.000326
8. Wang G, Zarodkiewicz P, Valvano MA. Current advances in Burkholderia vaccines development. Cells (2020) 9(12):2671. doi: 10.3390/cells9122671
9. Hinjoy S, Hantrakun V, Kongyu S, Kaewrakmuk J, Wangrangsimakul T, Jitsuronk S, et al. Melioidosis in Thailand: Present and future. Trop Med Infect Dis (2018) 3(2):38. doi: 10.3390/tropicalmed3020038
10. Hatcher CL, Muruato LA, Torres AG. Recent advances in Burkholderia mallei and b. pseudomallei Res Curr Trop Med Rep (2015) 2(2):629. doi: 10.1007/s40475-015-0042-2
11. Limmathurotsakul D, Funnell SG, Torres AG, Morici LA, Brett PJ, Dunachie S, et al. Consensus on the development of vaccines against naturally acquired melioidosis. Emerg Infect Dis (2015) 21(6):e141480. doi: 10.3201/eid2106.141480
12. Breitbach K, Köhler J, Steinmetz I. Induction of protective immunity against Burkholderia pseudomallei using attenuated mutants with defects in the intracellular life cycle. Trans R Soc Trop Med Hyg (2008) 102(Suppl 1):S8994. doi: 10.1016/S0035-9203(08)70022-1
13. Atkins T, Prior RG, Mack K, Russell P, Nelson M, Oyston PC, et al. A mutant of burkholderia pseudomallei, auxotrophic in the branched chain amino acid biosynthetic pathway, is attenuated and protective in a murine model of melioidosis. Infect Immun (2002) 70(9):5290–4. doi: 10.1128/IAI.70.9.5290-5294.2002
14. Harland DN, Chu K, Haque A, Nelson M, Walker NJ, Sarkar-Tyson M, et al. Identification of a LolC homologue in Burkholderia pseudomallei, a novel protective antigen for melioidosis. Infect Immun (2007) 75(8):4173–80. doi: 10.1128/IAI.00404-07
15. Casey WT, Spink N, Cia F, Collins C, Romano M, Berisio R, et al. Identification of an OmpW homologue in Burkholderia pseudomallei, a protective vaccine antigen against melioidosis. Vaccine (2016) 34(23):2616–21. doi: 10.1016/j.vaccine.2016.03.088
16. Yi J, Simpanya MF, Settles EW, Shannon AB, Hernandez K, Pristo L, et al. Caprine humoral response to Burkholderia pseudomallei antigens during acute melioidosis from aerosol exposure. PloS Negl Trop Dis (2019) 13(2):e0006851. doi: 10.1371/journal.pntd.0006851
17. Gourlay LJ, Peano C, Deantonio C, Perletti L, Pietrelli A, Villa R, et al. Selecting soluble/foldable protein domains through single-gene or genomic ORF filtering: Structure of the head domain of burkholderia pseudomallei antigen BPSL2063. Acta Crystallogr Sect D Biol Crystallogr (2015) 71(Pt 11):2227–35. doi: 10.1107/S1399004715015680
18. Whitlock GC, Deeraksa A, Qazi O, Judy BM, Taylor K, Propst KL, et al. Protective response to subunit vaccination against intranasal Burkholderia mallei and b. pseudomallei challenge Proc Vaccinol (2010) 2(1):73–7. doi: 10.1016/j.provac.2010.03.013
19. Burtnick MN, Brett PJ, Harding SV, Ngugi SA, Ribot WJ, Chantratita N, et al. The cluster 1 type VI secretion system is a major virulence determinant in. Burkholderia pseudomallei Infect Immun (2011) 79(4):1512–25. doi: 10.1128/IAI.01218-10
20. Nagakubo T, Nomura N, Toyofuku M. Cracking open bacterial membrane vesicles. Front Microbiol (2019) 10:3026. doi: 10.3389/fmicb.2019.03026
21. Qiao L, Rao Y, Zhu K, Rao X, Zhou R. Engineered remolding and application of bacterial membrane vesicles. Front Microbiol (2021) 12:729369. doi: 10.3389/fmicb.2021.729369
22. Zhao X, Zhao R, Nie G. Nanocarriers based on bacterial membrane materials for cancer vaccine delivery. Nat Protoc (2022) 17(10):2240–74. doi: 10.1038/s41596-022-00713-7
23. Xie J, Li Q, Haesebrouck F, Van Hoecke L, Vandenbroucke RE. The tremendous biomedical potential of bacterial extracellular vesicles. Trends Biotechnol (2022) 40(10):1173–94. doi: 10.1016/j.tibtech.2022.03.005
24. Findlow J, Bayliss CD, Beernink PT, Borrow R, Liberator P, Balmer P. Broad vaccine protection against Neisseria meningitidis using factor h binding protein. Vaccine (2020) 38(49):7716–27. doi: 10.1016/j.vaccine.2020.08.031
25. Yuan J, Yang J, Hu Z, Yang Y, Shang W, Hu Q. Safe staphylococcal platform for the development of multivalent nanoscale vesicles against viral infections. Nano Lett (2018) 18(2):725–33. doi: 10.1021/acs.nanolett.7b03893
26. Lim YT, Jobichen C, Wong J, Limmathurotsakul D, Li S, Chen Y, et al. Extended loop region of Hcp1 is critical for the assembly and function of type VI secretion system in. Burkholderia pseudomallei Sci Rep (2015) 5:8235. doi: 10.1038/srep08235
27. Chieng S, Mohamed R, Nathan S. Transcriptome analysis of Burkholderia pseudomallei T6SS identifies Hcp1 as a potential serodiagnostic marker. Microb Pathog (2015) 79:47–56. doi: 10.1016/j.micpath.2015.01.006
28. Fang Y, Huang Y, Li Q, Chen H, Yao Z, Pan J, et al. First genome sequence of a Burkholderia pseudomallei isolate in China, strain BPC006, obtained from a melioidosis patient in hainan. J Bacteriol (2012) 194(23):6604–5. doi: 10.1128/JB.01577-12
29. Rao Y, Peng H, Shang W, Hu Z, Yang Y, Tan L, et al. A vancomycin resistance-associated WalK(S221P) mutation attenuates the virulence of vancomycin-intermediate. Staphylococcus aureus J Adv Res (2022) 40:167–78. doi: 10.1016/j.jare.2021.11.015
30. Burtnick MN, Heiss C, Schuler AM, Azadi P, Brett PJ. Development of novel O-polysaccharide based glycoconjugates for immunization against glanders. Front Cell Infect Microbiol (2012) 2:148. doi: 10.3389/fcimb.2012.00148
31. Kim OY, Choi SJ, Jang SC, Park KS, Kim SR, Choi JP, et al. Bacterial protoplast-derived nanovesicles as vaccine delivery system against bacterial infection. Nano Lett (2015) 15(1):26674. doi: 10.1021/nl503508h
32. Puangpetch A, Anderson R, Huang YY, Saengsot R, Sermswan RW, Wongratanacheewin S. Comparison of the protective effects of killed Burkholderia pseudomallei and CpG oligodeoxynucleotide against live challenge. Vaccine (2014) 32(45):5983–8. doi: 10.1016/j.vaccine.2014.08.035
33. Sarkar-Tyson M, Smither SJ, Harding SV, Atkins TP, Titball RW. Protective efficacy of heat-inactivated b. thailandensis, B. mallei or B. pseudomallei against experimental melioidosis and glanders. Vaccine (2009) 27(33):4447–51. doi: 10.1016/j.vaccine.2009.05.040
34. Khakhum N, Bharaj P, Myers JN, Tapia D, Walker DH, Endsley JJ, et al. Evaluation of Burkholderia mallei ΔtonB Δhcp1 (CLH001) as a live attenuated vaccine in murine models of glanders and melioidosis. PloS Negl Trop Dis (2019) 13:e0007578. doi: 10.1371/journal.pntd.0007578
35. Burtnick MN, Shaffer TL, Ross BN, Muruato LA, Sbrana E, DeShazer D, et al. Development of subunit vaccines that provide high-level protection and sterilizing immunity against acute inhalational melioidosis. Infect Immun (2017) 86(1):e00724–17. doi: 10.1128/IAI.00724-17
36. Garcia-Quintanilla F, Iwashkiw JA, Price NL, Stratilo C, Feldman MF. Production of a recombinant vaccine candidate against Burkholderia pseudomallei exploiting the bacterial n-glycosylation machinery. Front Microbiol (2014) 5:381. doi: 10.3389/fmicb.2014.00381
37. Chen YS, Hsiao YS, Lin HH, Liu Y, Chen YL. CpG-modified plasmid DNA encoding flagellin improves immunogenicity and provides protection against Burkholderia pseudomallei infection in BALB/c mice. Infect Immun (2006) 74(3):1699–705. doi: 10.1128/IAI.74.3.1699-1705.2006
38. Lankelma JM, Wagemakers A, Birnie E, Haak BW, Trentelman JJA, Weehuizen TAF, et al. Rapid DNA vaccination against Burkholderia pseudomallei flagellin by tattoo or intranasal application. Virulence (2017) 8(8):1683–94. doi: 10.1080/21505594.2017.1307485
39. Lafontaine ER, Chen Z, Huertas-Diaz MC, Dyke JS, Jelesijevic TP, Michel F, et al. The autotransporter protein BatA is a protective antigen against lethal aerosol infection with. Burkholderia mallei Burkholderia pseudomallei Vaccine X (2018) 1:100002. doi: 10.1016/j.jvacx.2018.100002
40. Willcocks SJ, Denman CC, Atkins HS, Wren BW. Intracellular replication of the well-armed pathogen. Burkholderia pseudomallei Curr Opin Microbiol (2016) 29:94–103. doi: 10.1016/j.mib.2015.11.007
41. Khakhum N, Bharaj P, Walker DH, Torres AG, Endsley JJ. Antigen-specific antibody and polyfunctional T cells generated by respiratory immunization with protective Burkholderia ΔtonB Δhcp1 live attenuated vaccines. NPJ Vaccines (2021) 6(1):72. doi: 10.1038/s41541-021-00333-4
42. Klimko CP, Shoe JL, Rill NO, Hunter M, Dankmeyer JL, Talyansky Y, et al. Layered and integrated medical countermeasures against Burkholderia pseudomallei infections in C57BL/6 mice. Front Microbiol (2022) 13:965572. doi: 10.3389/fmicb.2022.965572
43. Morici L, Torres AG, Titball RW. Novel multi-component vaccine approaches for. Burkholderia pseudomallei Clin Exp Immunol (2019) 196(2):178–88. doi: 10.1111/cei.13286
44. Nieves W, Asakrah S, Qazi O, Brown KA, Kurtz J, Aucoin DP, et al. A naturally derived outermembrane vesicle vaccine protects against lethal pulmonary Burkholderia pseudomallei infection. Vaccine (2011) 29(46):8381–9. doi: 10.1016/j.vaccine.2011.08.058
45. Nieves W, Petersen H, Judy BM, Blumentritt CA, Russell-Lodrigue K, Roy CJ, et al. A Burkholderia pseudomallei outer membrane vesicle vaccine provides protection against lethal sepsis. Clin Vaccine Immunol (2014) 21(5):747–54. doi: 10.1128/CVI.00119-14
46. Khakhum N, Bharaj P, Myers JN, Tapia D, Kilgore PB, Ross BN, et al. Burkholderia pseudomallei ΔtonB Δhcp1 live attenuated vaccine strain elicits full protective immunity against aerosolized melioidosis infection. mSphere (2019) 4(1):e00570–18. doi: 10.1128/mSphere.00570-18
47. Su YC, Wan KL, Mohamed R, Nathan S. Immunization with the recombinant Burkholderia pseudomallei outer membrane protein Omp85 induces protective immunity in mice. Vaccine (2010) 28(31):5005–11. doi: 10.1016/j.vaccine.2010.05.022
48. Sanders H, Feavers IM. Adjuvant properties of meningococcal outer membrane vesicles and the use of adjuvants in Neisseria meningitidis protein vaccines. Expert Rev Vaccines (2011) 10(3):323–34. doi: 10.1586/erv.11.10
49. Park KS, Svennerholm K, Crescitelli R, Lässer C, Gribonika I, Lötvall J. Synthetic bacterial vesicles combined with tumour extracellular vesicles as cancer immunotherapy. J Extracell Vesicles (2021) 10(9):e12120. doi: 10.1002/jev2.12120
Keywords: Burkholderia pseudomallei, vaccine, membrane vesicles, Hcp1, melioidosis, protection
Citation: Zhu K, Li G, Li J, Zheng M, Peng X, Rao Y, Li M, Zhou R and Rao X (2022) Hcp1-loaded staphylococcal membrane vesicle vaccine protects against acute melioidosis. Front. Immunol. 13:1089225. doi: 10.3389/fimmu.2022.1089225
Received: 04 November 2022; Accepted: 13 December 2022;
Published: 23 December 2022.
Edited by:
Nargis Khan, University of Calgary, CanadaReviewed by:
Ming-Hsien Chiang, National Defense Medical Center, TaiwanAndrés Corral Lugo, Carlos III Health Institute (ISCIII), Spain
Copyright © 2022 Zhu, Li, Li, Zheng, Peng, Rao, Li, Zhou and Rao. This is an open-access article distributed under the terms of the Creative Commons Attribution License (CC BY). The use, distribution or reproduction in other forums is permitted, provided the original author(s) and the copyright owner(s) are credited and that the original publication in this journal is cited, in accordance with accepted academic practice. No use, distribution or reproduction is permitted which does not comply with these terms.
*Correspondence: Ming Li, c290eDcwODBAMTYzLmNvbQ==; Renjie Zhou, emhvdV9yakBhbGl5dW4uY29t; Xiancai Rao, cmFveGlhbmNhaUAxMjYuY29t
†These authors have contributed equally to this work and share first authorship
‡These authors have contributed equally to this work and share last authorship