- 1Department of Respiratory and Critical Care Medicine, The Second Affiliated Hospital of Nanjing Medical University, Nanjing, Jiangsu, China
- 2The Second Clinical Medical School of Nanjing Medical University, Nanjing, Jiangsu, China
- 3Department of Infectious Disease, The Second Affiliated Hospital of Nanjing Medical University, Nanjing, Jiangsu, China
Acinetobacter baumannii is a gram-negative bacterium and a crucial opportunistic pathogen in hospitals. A. baumannii infection has become a challenging problem in clinical practice due to the increasing number of multidrug-resistant strains and their prevalence worldwide. Vaccines are effective tools to prevent and control A. baumannii infection. Many researchers are studying subunit vaccines against A. baumannii. Subunit vaccines have the advantages of high purity, safety, and stability, ease of production, and highly targeted induced immune responses. To date, no A. baumannii subunit vaccine candidate has entered clinical trials. This may be related to the easy degradation of subunit vaccines in vivo and weak immunogenicity. Using adjuvants or delivery vehicles to prepare subunit vaccines can slow down degradation and improve immunogenicity. The common immunization routes include intramuscular injection, subcutaneous injection, intraperitoneal injection and mucosal vaccination. The appropriate immunization method can also enhance the immune effect of subunit vaccines. Therefore, selecting an appropriate adjuvant and immunization method is essential for subunit vaccine research. This review summarizes the past exploration of A. baumannii subunit vaccines, hoping to guide current and future research on these vaccines.
1. Introduction
Acinetobacter baumannii is a strictly aerobic gram-negative bacillus, that exists widely in nature (1). A. baumannii is an opportunistic pathogen that can cause various nosocomial infections, including pneumonia, sepsis, meningitis, posttraumatic infections and urinary tract infections (2–4). This ESKAPE pathogen poses a significant threat to global public health because of its widespread drug resistance (5–8). In 2019, A. baumannii was responsible for more than 250 000 deaths associated with antimicrobial resistance (9). Effective antibiotic treatment is thus complicated and alternative therapeutic strategies are urgently needed, and vaccine is an effective tool to prevent and control A. baumannii infection (10–12).
Many researchers are currently developing subunit vaccines for A. baumannii. The subunit vaccine is a type of vaccine that contains active fragments of the pathogen to stimulate a protective immune response. Subunit vaccines have the advantages of high purity, safety, and stability, ease of production and highly targeted induced immune responses (13). Most of the A. baumannii candidate subunit vaccines are proteins, such as outer membrane protein A (OmpA). Polysaccharides, and outer membrane vesicles (OMVs) can also be used as subunit vaccines for A. baumannii (14). However, the subunit antigen fragment is small and lacks the tertiary structure of the protein. This fragment is easy to degrade in vivo and has weak immunogenicity. The use of adjuvants or delivery vehicles during subunit preparation can protect the antigen from degradation and enhance its immune efficacy (15, 16).
The development of reverse vaccinology, pan-genomics, core genomics, proteomics, immunoinformatics, and biophysical analyses have brought broad prospects in the exploration of potential antigen candidates of the subunit vaccine against A. baumannii (17–19). Afreenish Hassan et al. analyzed genetic data for all strains of A. baumannii, known as the pangenome, using proteomics and reverse vaccinology, thirteen types of proteins with high antigenicity were found in the conserved core genome (17). Among these four outer membrane proteins were prioritized: TonB-dependent siderphore receptor, OmpA family protein, type IV pilus biogenesis stability protein, and OprD family outer membrane porin (18). What’s more, recent vaccination trials used in silico computational approaches a type of epitope mapping of antigens to identify the prominent B-cell and T-cell epitopes (19, 20). To improve the immunogenicity of the epitope vaccine, it is important to combine multiple epitopes in series to form multi-epitope vaccines. Biophysical analysis was used to build a 3D model to test the stability of the vaccine and its ability to bind with host MHC-I, MHC-II, and toll-like receptors 4 (TLR-4) molecules on the surface of immune cells in the body (17, 18). Till now, epitope vaccines for A. baumannii candidate antigens, such as outer membrane protein 22, DcaP porin, MacB protein, and PcTPRs1 have been studied (20–23).
A. baumannii subunit vaccines (Table 1) that provide partial or complete protection have been previously studied. The development of new vaccines generally takes decades or even centuries (55). To date, no A. baumannii vaccine candidate has entered clinical trials. With the increase in multidrug-resistant strains and their prevalence worldwide, the development of new vaccines to prevent A. baumannii infection is urgently needed (5, 6). The general process for creating a subunit vaccine for A. baumannii is shown in Figure 1. This review summarizes past studies of A. baumannii subunit vaccines (Figure 2), hoping to provide guidance for current and future research on A. baumannii subunit vaccines.
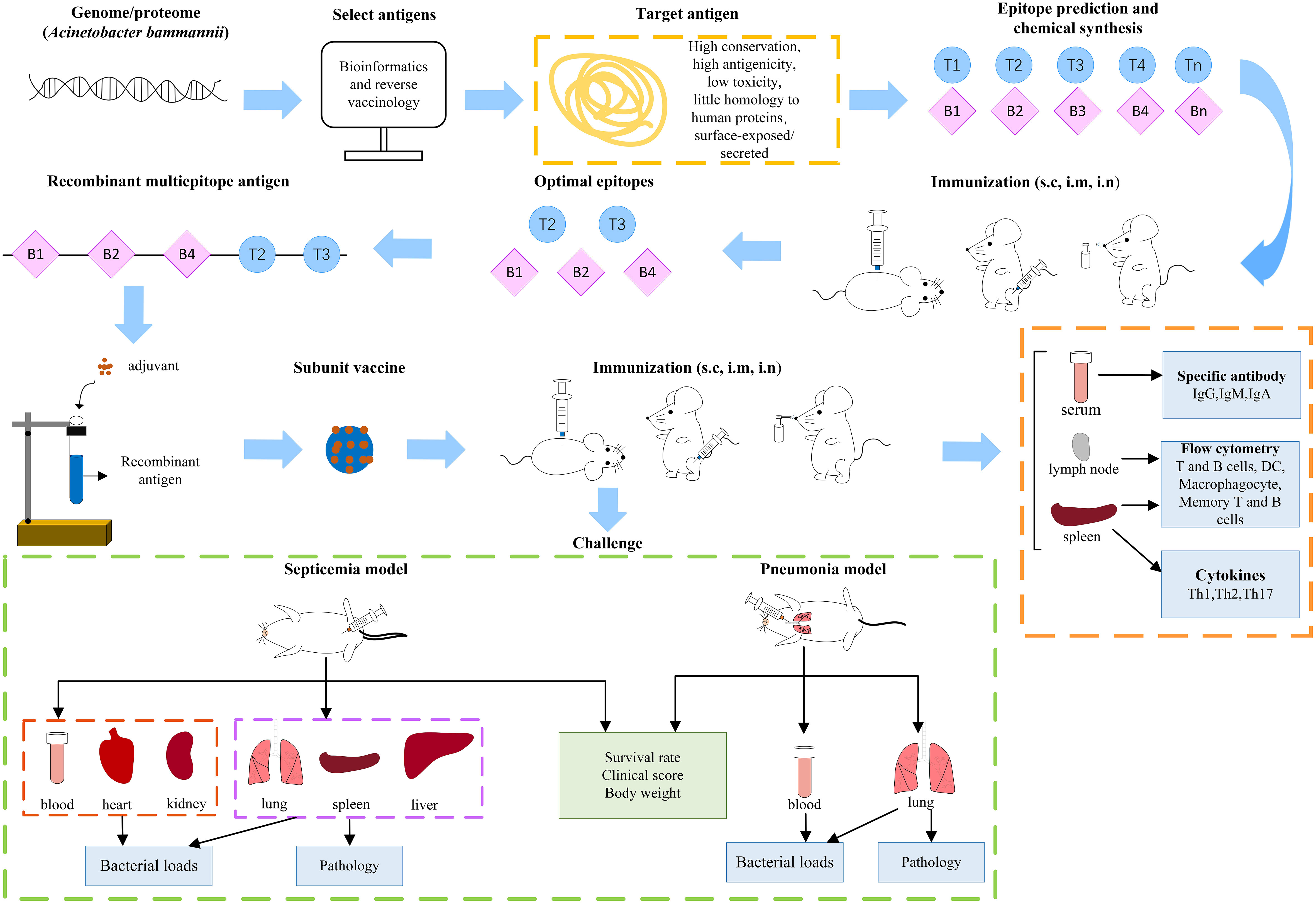
Figure 1 The general process of Acinetobacter baumannii subunit vaccine research. (s.c, subcutaneous; i.m, intramuscular; i.n, intranasal).
2. Candidate subunit vaccines for Acinetobacter baumannii
Many subunit vaccine antigens for A. baumannii have been explored with varying degree of success. Around twenty A. baumannii subunit vaccines have been evaluated in vivo for their immunogenicity and protective effects. Among them, the most studied antigens are outer membrane proteins (OmpA, Omp33-36, Omp22, OmpW, and Ata, et al.), fimbrial proteins (CsuA/B and FimA), and capsular polysaccharide (11, 14, 56). Outer membrane vesicles (OMVs) have been also studied in recent years. In addition, over ten novel antigens, such as Pfsr, LptE, OmpH, CarO and FimF, predicted by reverse vaccinology are worthy of further exploration (57, 58). The candidate antigens for A. baumannii subunit vaccines can be divided into three categories: proteins, polysaccharides, and outer membrane vesicles.
2.1. Proteins
2.1.1. Outer membrane proteins
2.1.1.1. OmpA
Outer membrane protein A (OmpA), also known as Omp38, with a molecular weight of 38 kDa, is one of the most abundant porins in the outer membrane of A. baumannii (59). OmpA plays a key role in regulating the adhesion, invasiveness, biofilm formation, apoptosis and the associated host immune response of A. baumannii (60–65). Additionally, OmpA regulates autophagy (66). In recent years, with improved understanding of the natural structure of OmpA, researchers have found that the amino acids of OmpA from various clinical isolates of A. baumannii are highly conserved (>99%), and they are of a different origin compared to the human proteome (24).
Some studies have shown that immunization with OmpA can protect against A. baumannii infection. Mice were passively immunized with a yolk antibody (IgY) specific to OmpA, which provided partial protection. IgY prolonged the survival of mice attacked by A. baumannii (67, 68). Mice were subcutaneously immunized with recombinant OmpA (rOmpA). After challenge with A. baumannii, the survival rate of actively immunized mice was more than 40%, and that of passively immunized mice was up to 90% (24). Increased doses of the rOmpA vaccine resulted in enhanced type 2 immune responses, increased IL-4-induced T-cell epitope spread and decreased levels of IFN-γ-induced epitopes (69). When mice were intranasally immunized with rOmpA, anti-OmpA IgG was produced in the serum, and anti-OmpA IgA was produced in saliva. After intraperitoneal immunization of mice with rOmpA and stimulation with different doses of A. baumannii, the 15-day survival rate of the mice was 40%-100% (25). The total IgG concentration in the serum of mice immunized with OmpA and the serine protease PKF was significantly increased. This protein can provide 85.71% protection against A. baumannii (26). The serum IgG antibody level was significantly increased in mice immunized with OmpA and BauA. After stimulation with A. baumannii, the bacterial loads in the lung, spleen and liver of immunized mice were significantly lower than those in the control group (70).
OmpA is an important protein in A. baumannii. This protein is highly conserved, exhibiting high immunogenicity and low endotoxin levels after treatment. OmpA can induce a robust protective effect against A. baumannii in mice. Therefore, OmpA is considered the most promising subunit vaccine candidate and the antigen with the most clinical translational value. However, OmpA has not yet entered clinical trials which may be related to its toxicity. Luo et al. used a detoxifying gel endotoxin removal column to greatly reduce OmpA endotoxin level, but 1-4 EU/3μg of endotoxin remained (24). The measurement of endotoxin level has been limited to animal studies. Immune cell membranes coated or fused with OmpA may neutralize OmpA toxicity and improve OmpA immunogenicity.
2.1.1.2. Omp33-36
Outer membrane protein 33-36, known as Omp33 or Omp34 in some Acinetobacter species, is another outer membrane virulence factor involved in host cell adhesion. Omp33-36, OmpA and TonB are collectively known as fibronectin-binding proteins (FBPs) (71). Omp33-36 is associated with the adhesion and invasion abilities, cytotoxicity and metabolic adaptability of A. baumannii (72). Omp33-36 can promote apoptosis and regulate autophagy in human cells (73, 74).
Omp33-36 is recognized explicitly by IgM, IgA, and IgG from patients infected with A. baumannii. Omp33-36 did not cross-react with sera from patients infected with pathogens other than A. baumannii (75). Omp33-36 sequences showed ≥98% identity among more than 1670 strains of A. baumannii (76). Passive immunization with a yolk antibody (IgY) specific to Omp33-36 successfully protected a mouse model from pneumonia caused by A. baumannii (67, 68, 77). The conserved exposure loop 3 of Omp33-36 combined with the loopless-C-lobe (LCL) of TbpB of Neisseria meningitidis was used to actively immunize mice. Mice were stimulated with lethal doses of A. baumannii. In the sepsis model, the 96-hour survival rate of mice was 42.85%-80%. In the pneumonia model, the survival rate of mice was 57.14%-100% (27). The immunogenic loops of Omp33-36 are nontoxic. Mice were immunized with a hybrid antigen composed of BauA and immunogenic loops of Omp33-36 and LCL. The 7-day survival rate of mice vaccinated with hybrid antigens (71.43%) was higher than that of mice immunized with Omp33-36 immunogenic loop alone (42.86%) (28). The survival rate of mice immunized with recombinant Omp33-36 (rOmp33-36) was 100% under stimulation with A. baumannii (29).
Omp33-36 is highly conserved, nontoxic, and has high immunogenicity in A. baumannii, providing partial or complete protection against A. baumannii infection. Omp33-36 has the potential for clinical transformation. This protein is under preclinical study and may be related to its immunogenicity. Omp33-36 does not provide complete protection in all kinds of challenge models. Novel adjuvants, such as nanomaterials and bacterial outer membrane vesicles, may improve the immunogenicity of Omp33-36.
2.1.1.3. Omp22
Outer membrane protein 22 (Omp22) is 217 amino acids in length and exhibits >95% conservation among 851 A. baumannii strains, showing little homology to human proteins (30). Omp22 is slightly toxic to mammalian cells, and high doses of Omp22 do not cause obvious pathological changes in mice. Mice were immunized subcutaneously with a high dose of recombinant Trx-Omp22 fusion protein. Survival reached 100% at 7 days after challenge with lethal doses of A. baumannii, whereas low doses showed protection in one-third of the mice (30). Recombinant Omp22 (rOmp22) was encapsulated with chitosan (CS) and polylactic-co-glycolic acid (PLGA) to form the CS-PLGA-rOmp22 nanovaccine. The 7-day survival rate of mice immunized subcutaneously with CS-PLGA-rOmp22 was 57.14%-83.3% in the pneumonia model (21). The fusion protein Omp22/OmpK was formed by using Omp22 and OmpK. Mice immunized subcutaneously with Omp22/OmpK and Freund’s adjuvant had a survival rate of 66.7% under A. baumannii attack (31). The combination of Omp22/OmpK and MF59 was injected into the trachea of mice, and the protective effect was found to be stronger than that of OmpK alone. The survival rate was 83.3% at 10 days after stimulation (32). Omp22 is highly conserved with low toxicity and some immunogenicity. Omp22 is not highly protective in mice, but its protective effect can be improved by changing the type of adjuvant. Omp22 is a valuable candidate for further study.
2.1.1.4. OmpW
Outer membrane protein W (OmpW) is an eight-stranded β-barrel pore protein that forms a channel through the outer membrane to absorb small hydrophobic molecules (78). Hypoxia leads to downregulation of OmpW, resulting in decreased adhesion and invasion of A. baumannii in human lung epithelial cells and reduced biofilm formation (79). OmpW is also involved in colistin binding and plays an important role in regulating bacterial iron homeostasis (33, 80). The homology of OmpW among 804 reported A. baumannii strains was > 91%. OmpW had a slight inhibitory effect on 293FT and A549 cells, indicating that OmpW may affect the growth of normal cells and tumor cells. In a sepsis model, both active and passive immunization with recombinant Trx-OmpW protein were remarkably effective against A. baumannii infection. Seven days after A. baumannii challenge, the survival rate of actively immunized mice was 100% and that of passively immunized mice was 83.3% (33). OmpW is conserved among A. baumannii strains and has high immunogenicity, with the ability to protect against A. baumannii infection. OmpW is one of the candidate subunit vaccines of A. baumannii. However, further research is needed to eliminate or attenuate the virulence of OmpW, for example, by selecting some reagents that can neutralize or remove the toxin. Additional research is needed before the use of OmpW can be translated to clinical trials.
2.1.1.5. Ata
Acinetobacter trimeric autotransporter adhesin (Ata) belongs to the superfamily of trimeric autotransporter adhesins, which are crucial virulence factors in A. baumannii. Ata mediates adhesion and invasion, induces apoptosis and contributes to pathogenicity in vivo (81, 82). Ata also participates in biofilm formation and binds to various extracellular matrix/basement membrane (ECM/BM) components (82).
Ata is a potential vaccine target. The antibodies against Ata have a strong opsonization effect on A. baumannii, with low to moderate killing activity against four A. baumannii strains (83). A conserved 263-amino-acid fragment from the C-terminus of Ata could elicit specific antibody responses and protect against challenges in mice. Mice were immunized with Ata fragments by abdominal, subcutaneous, and nasal mucosal immunization. When challenged with A. baumannii, the survival rate of mice immunized in the abdominal and subcutaneous areas was 66%, and that of mice immunized in the nasal mucosa was 100% (34). A short peptide containing only 39 amino acids located in the extracellular region of the C-terminal region of Ata was fused with the B subunit of cholera toxin (CTB), and experiments showed that this protein had no systemic toxicity. Subcutaneous and intraperitoneal immunization of mice with the fusion protein elicited T1 and T2 immune responses in vivo. When challenged with A. baumannii, the survival rate of mice immunized subcutaneously was 60% to 90% and that of mice immunized intraperitoneally was 100% (35).
Ata has high immunogenicity, no toxicity, and good safety and can prevent A. baumannii infection. Ata is one of the candidate subunit vaccines for A. baumannii and has value in clinical translation. Its effect on nasal mucosal immunity is promising. Mice immunized with Ata via nasal mucosa had a strong immune effect and strong protective effect on mice. The long-term protective effects of Ata should be examined in future studies. Research on the protective effect of nasally immunized mice against various types of A. baumannii strains will be of great significance.
2.1.1.6. NucAb
Outer membrane nuclease (NucAb) is a protein in the outer membrane of A. baumannii. As the name suggests, this protein has nuclease activity. Both gram-negative and gram-positive bacteria can produce outer membrane nucleases, which may be related to bacterial virulence and survival in harsh environments (84–89). NucAb has all the characteristics of a vaccine candidate, such as outer membrane localization, and lack of homology to human proteins. The NucAb gene was found to be highly conserved (100%) among 40 clinical isolates of A. baumannii and showed more than 98% conservation among sequenced Acinetobacter strains present in the NCBI database (36). The endotoxin level of recombinant NucAb was low (< 1 EU/ml). Mice immunized with recombinant NucAb showed significant inhibition of inflammation in lung histopathological examination. Lung and serum levels of proinflammatory cytokines (TNF-α and IL-6) were significantly reduced, and those of anti-inflammatory (IL-10) cytokines were increased. NucAb is a candidate subunit vaccine against A. baumannii. NucAb is highly conserved, with good safety and some immunogenicity. However, the survival rate was only 20% in the group of actively immunized mice, and the survival rate of passively immunized mice was approximately 40% after A. baumannii infection (36). Therefore, we think NucAb has a low probability of being clinically translatable.
2.1.1.7. BamA
BamA is an outer membrane β-barrel assembly protein responsible for organizing protein complex on the outer membrane of bacteria (37, 90, 91). The formation and assembly of outer membrane proteins on bacterial membranes are related to the action of the Bam protein complex (91). Among these proteins, BamA represents a potential target. BamA is anchored to the cell membrane and has a small extracellular portion that can generate immunogenic epitopes (92). The sequence identity of BamA in A. baumannii strains was 92.3% to 99.9%. Mice immunized with recombinant BamA produced IgG with high titers. In a mouse pneumonia model, the 7-day survival rate was 80% in actively immunized mice and 60% in passively immunized mice. Compared with unimmunized mice, immunized mice had a lower bacterial load in their lungs and significantly reduced the levels of the serum proinflammatory factors IL-6 and IL-1β (37). In silico analyses revealed that Oma87 is the same as BamA. Mice immunized with recombinant Oma87 (rOma87) showed a high specific IgG titer in serum. Immunized mice stimulated with twice the lethal dose of A. baumannii had a survival rate of 100%. Mice immunized with seven times the lethal dose of A. baumannii had a survival rate of 25% (38). As a candidate subunit vaccine, BamA has high conservation and immunogenicity and low toxicity. BamA can provide vital protection to immunized mice, with potential for clinical translation. The immunogenicity of BamA has room for improvement. The T-cell and B-cell epitopes of BamA were screened by bioinformatics, and the recombinant protein was constructed to immunize mice, which may improve the immunogenicity of BamA.
2.1.1.8. BauA
Baumannii acinetobactin utilization (BauA) is the most important member of the iron-regulated outer membrane proteins (IROMPs) family of A. baumannii. BauA plays a key role in the absorption of acinetobactin and iron complexes under iron-restricted conditions (93, 94). This protein is a monomer, composed of the cork and the β-barrel domains. The barrel is composed of 22 antiparallel transmembrane β-chains. The N-terminal domain, called the cork occludes the β-barrel (95). BauA is conserved among members of Acinetobacter genus (96). The antibody titers in the serum of mice immunized with recombinant BauA (rBauA) were significantly higher than those in the control group (97, 98). Exposure loop 7 of BauA was combined with LCL to form a hybrid antigen. Immunizing mice with hybrid antigens resulted in rather high antigen titers. BauA can provide complete protection against A. baumannii and is a promising candidate subunit vaccine (39).
2.1.1.9. DcaP
DcaP is a porin of A. baumannii that is involved in biofilm formation (99). This protein is the most abundant dispersal channel for A. baumannii during rodent infection. The X-ray crystal structure of DcaP showed a trimeric pore structure. DcaP may be involved in the uptake of clinically relevant negatively charged β-lactamase inhibitors, such as sulbactam and tazobactam (100). The DcaP sequence was more than 90% identical among the 1450 A. baumannii strains studied. There was no similarity between DcaP and human or mouse proteins. The survival rates of active and passive DcaP-immunized mice infected with two times the lethal dose of A. baumannii strains were 50% and 66.7%, respectively. Mice actively and passively immunized with the multiepitope vaccine of DcaP showed 33.3% and 50% survival after challenge with twice the lethal dose of A. baumannii (22). DcaP, a candidate subunit vaccine, is highly conserved and has a different origin from human proteins. This protein exhibits some immunogenicity and is not a toxin or an allergen. DcaP did not provide sufficient protection to immunized mice, and the survival rate of immunized mice stimulated by A. baumannii was not high. The prospects for clinical translation of DcaP are low.
2.1.1.10. FilF
FilF is a putative pilus assembly protein located in the outer membrane of A. baumannii. It is highly conserved among A. baumannii strains, but its function remains unclear (40). This protein contains 641 amino acids, 20 of which form signal peptides for localization to the outer membrane. The level of FilF endotoxin was less than 1 EU/ml. Immunization of mice with FilF by subcutaneous injection induced specific IgG production and significantly decreased the levels of the proinflammatory factors TNF-α, IL-6, IL-33, IFN-γ, and IL-1β. When treated with lethal doses of A. baumannii, the bacterial load in the lung tissue of mice was significantly reduced compared with that in the control group, and the survival rate of mice was up to 50%, indicating that FilF provided immunological protection against A. baumannii infection (40). The T-cell and B-cell epitopes of FilF and NucAb were screened by bioinformatics software to generate the recombinant multiepitope assembly peptide (rMEP). Immunizing mice with rMEP could induce high levels of IgG antibodies and provide effective protection (88.9%) against a lethal dose of A. baumannii (41). FilF is conserved, immunogenic, and weakly toxic. FilF has a weak protective effect on mice, and the survival rate of mice under A. baumannii attack is not very high. Therefore, the possibility of clinical translation is low.
2.1.1.11. PcTPRs1
PcTPRs1 is a protein containing a tetrapeptide repeat (TPR) that is associated with bacterial pathogenicity and virulence (20, 101, 102). PcTPRs1 is also involved in a variety of biological processes, such as gene regulation, cell cycle regulation, transfer of bacterial virulence factors to host cells, binding to host cells, and inhibition of phagolysosome maturation (103–105). PcTPRs1 is an outer membrane protein that is very highly conserved (identity>90%) in A. baumannii. Mice immunized with PcTPRs1 and its subunits had survival rates of 50% and 40%, respectively, after stimulation with twice the lethal dose of A. baumannii. Immunized mice produce specific IgG antibodies. Mice passively immunized with PcTPRs1 and its subunits had survival rates of 66.7% and 50%, respectively (20). PcTPRs1, a candidate subunit vaccine of A. baumannii, is very highly conserved and is immunogenic. PcTPRs1 did not have a strong protective effect on mice. Therefore, the probability of clinical translation is low.
2.1.2. Fimbrial proteins
2.1.2.1. ABAYE2132
ABAYE2132 is a fimbrial protein associated with the adhesion, invasion, biofilm formation and motility of A. baumannii. When ABAYE2132-resistant serum was cultured with A. baumannii and A549 cells, the adhesion of A. baumannii to A549 cells was reduced by 40% (42). ABAYE2132 is highly conserved, with an identity of more than 99% (42, 106). The full-length protein sequence consists of 209 amino acids. Immunized of mice with ABAYE2132 provided complete protection against A. baumannii stimulation, and the bacterial loads in the lungs, liver and spleen of the immunized mice were significantly lower than those in the control group (42).
2.1.2.2. CsuA/B and FimA
Chaperone-Usher (CU) pili is a virulence factor involved in bacterial adhesion; CsuA/B and FimA are important components of CU pili (107–110). CsuA/B and FimA are highly conserved among A. baumannii strains. The identity of CsuA/B in 2927 A. baumannii strains was ≥99.44%, and the query coverage (QC) was ≥98%. The QC of FimA among 2300 strains of A. baumannii was 100% (43). The two antigens, CsuA/B and FimA, are safe and do not cause toxicity to mammalian cells. Mice immunized with CsuA/B had a survival rate of only 37% when challenged with sublethal doses of A. baumannii (43). The survival rate of mice vaccinated with FimA was up to 50% when the mice were challenged with sublethal doses of A. baumannii (43). Mice immunized with recombinant of CsuA/B and FimA proteins had a survival rate of 62.5%, which was higher than that of mice immunized with CsuA/B or FimA alone (43). CsuA/B and FimA are candidate subunit vaccines against A. baumannii that are highly conserved and have immunogenicity without toxicity. CsuA/B and FimA did not had a strong protective effect on mice, and the probability of clinical translation is low.
2.1.3. Other types of proteins
2.1.3.1. Bap
Biofilm-associated protein (Bap) is found on the surface of bacteria, confers the ability to form biofilms, and plays a relevant role during bacterial infection (111). Bap is a specific bacterial surface protein directly involved in biofilm formation in A. baumannii and is involved in intercellular adhesion in mature biofilms (112, 113). Bap is one of the largest bacterial proteins described to date and contains 8621 amino acids. Its predicted isoelectric point (pI) is 2.9, making it one of the most acidic bacterial proteins (112). Bap is composed of seven tandem repeat modules, which are the main components of functional and conserved regions (114). The mice were passively immunized with IgY specifically targeting Bap to prevent A. baumannii infection (115). Mice immunized with the recombinant Bap subunit produced high titers of antibodies, which were able to prevent A. baumannii infection. The 3-day survival rate of the immunized mice was 60% to 100% (44). Intranasally immunizing mice with chitosan-loaded Bap increased the specific IgG and IgA levels in serum, lung and fecal samples (116). The combined OmpA and Bap vaccines were more potent, with a seven-day survival rate of more than 80% observed in mice when challenged with A. baumannii (45). Bap is a candidate subunit vaccine for A. baumannii. This protein has high immunogenicity and can protect against A. baumannii attack. There is a lack of research on the toxicity of Bap, which is critical for subunit vaccine research.
2.1.3.2. Blp1
The structure of the A. baumannii Blp1 protein is similar to that of the giant A. baumannii protein Bap. Blp1 is related to the adhesion, virulence and biofilm formation of A. baumannii (46, 117). The Blp1 proteins encoded in the genomes of the IC I and IC II strains share 71-74% identity. Purified Blp1 was mildly toxic to lung epithelial cells. Blp1 has a tripartite structure consisting of C-terminal and N-terminal domains with a repetitive region containing multiple motif combinations in the middle. Immunization with the C-terminal fragment of the Blp1 protein protected mice from lethal doses of A. baumannii. Immunized mice produced specific IgG antibodies. Mice were injected with a lethal dose of A. baumannii intraperitoneally. The seven-day survival rate of actively immunized mice was 60%. Passive immunization by intraperitoneal injection of serum from Blp1-immunized mice provided complete protection to mice (46). Blp1, one of the candidate subunits of A. baumannii, is less well conserved than other antigens. Blp1 has some immunogenicity and low toxicity. Blp1-immunized mice showed some protection against A. baumannii infection. The probability of clinical translation of Blp1 is not particularly high.
2.1.3.3. VgrG
The type VI secretory system (T6SS) is associated with the virulence and drug resistance of A. baumannii. Valine-glycine repeat protein G (VgrG), the core component of the T6SS, is a potent mediator of A. baumannii pathogenicity (118, 119). The selected regions of VgrG were conserved in 118 strains of A. baumannii (identity ≥97.63%) (47). VgrG is toxic to monocytes and A549 cells. The VgrG1263-1608 sequence was incorporated into the LCL of N. meningitidis for surface display and exposure to functional epitopes. The recombinant protein LCL-VgrG was expressed and purified. Mice immunized with LCL-VgrG produced specific IgG antibodies. After challenge with a lethal dose of A. baumannii, the survival rates of the mice were 33.3% and 66.6% in the actively and passively immunized groups, respectively (47). Under attack by A. baumannii, mice immunized with recombinant VgrG (rVgrG) had higher serum IgG levels, lower bacterial loads in the lungs and spleen, and a 3-day survival rate of 75% (48).
2.1.3.4. MacB
MacAB-tolC is an ABC-type efflux pump responsible for conferring resistance to several antibiotics in bacteria. MacB is an essential control hub in the network and plays a crucial role in the MacAB-tolC efflux pump (120, 121). The MacB protein contains 664 amino acid residues, and 99 homologous sequences were found in Acinetobacter, with query coverage and identity greater than 95%. Three epitopes of MacB were screened to form a recombinant epitope (RAE). Mice immunized with RAE produced specific IgG and exhibited increased serum IFN-γ levels. After intraperitoneal injection with A. baumannii, the longest survival time of the immunized mice was 14 days, which was significantly higher than that of the control group (23). MacB, a candidate subunit vaccine for A. baumannii, is highly conserved and exhibits some immunogenicity. MacB prolonged the survival of infected mice, but did not prevent death, exhibiting little clinical translational value.
2.1.3.5. TolB
TolB is associated with bacterial growth kinetics, motility, and virulence, and is involved in maintaining the integrity of the bacterial envelope (122, 123). TolB is an allosteric β-propeller protein that acts in the bacterial periplasmic space and may interact with other proteins (124–126). Song et al. used bioinformatics software to predict the structure of the TolB protein and indicated that TolB is a potential vaccine antigen. The gene segments of four strains of A. baumannii were sequenced, and the similarity of the TolB protein was 96.2%. The T-cell and B-cell epitopes on TolB were screened and reconstituted, and an excellent epitope was designed and verified by experiments (123).
2.1.3.6. CipA and PBP-7/8
CipA and PBP-7/8 are serum drug resistance factors that play a crucial role in the pathogenesis of A. baumannii (127, 128). CipA is a plasminogen binding protein. CipA binds to plasminogen and converts it to the active serine protease plasmin, which degrades fibrinogen and complements C3b. CipA directly inhibits the alternative pathway of complement in vitro (127). Penicillin-binding protein 7/8 (PGP-7/8) plays a role in cell wall remodeling. Moreover, PBP-7/8 directly or indirectly affects serum drug resistance (128). Seven-day survival rates of mice immunized with CipA, PBP-7/8, and CipA+PBP-7/8 were 60%, 60%, and 80%, respectively, in the A. baumannii sepsis model. Immunized mice had higher serum total IgG levels and lower bacterial loads in their spleens than control mice (49). CipA and PBP-7/8 are candidate subunit vaccines against A. baumannii that have high immunogenicity and can protect mice against infection. However, its toxicity and conservation remain to be explored.
2.1.4. Novel predicted subunit vaccine candidate proteins
Ahmad et al. used a virome-based reverse vaccinology method to screen two vaccine candidates, polysaccharide export outer membrane protein (EpsA) and chaperone-usher pathway protein B (CsuB). EpsA and CsuB are toxic, antigenic, nonallergenic, and highly conserved (129). Fereshteh et al. showed that Dcap-like proteins and HP2 could be used as vaccine candidates by a reverse vaccinology approach and B-cell epitope analysis. Dcap-like protein and HP-2 have highly conserved surface-exposed epitopes (130). Zadeh Hosseingholi et al. used bioinformatics analysis and found that four hypothetical proteins, HP4, HP6, HP8, and HP15, had the characteristics of vaccine candidates (131). Beiranvand et al. used bioinformatics methods, reverse vaccinology, and subtractive genomics to select five vaccine candidates, namely, Pfsr, LptE, OmpH, CarO and FimF, which have appropriate antigenicity, solubility, and immunogenicity (57, 58).
2.2. Polysaccharides
Capsular polysaccharide (CPS) produced by A. baumannii surrounds the outer membrane. Composed of repetitive oligosaccharide units (K Units), CPS is a major virulence factor that protects bacteria from environmental damage (132–134). CPS participates in host cell interactions and provides protection against phagocytosis and complement-mediated bactericidal effects (135, 136). CPS can help bacteria evade host immune responses. The pathogen-covering CPS is different from mammalian glycans and therefore unlikely to trigger autoimmunity or allergies in humans (133). Yang et al. passively immunized mice with rabbit polyclonal CPS antibody, which provided 50% protection against A. baumannii challenge (136). Three inert carrier proteins were coupled to the type K9 CPS fragment of A. baumannii. Immunizing mice with the conjugate induced high levels of IgG antibodies in serum and stimulated the production of IL-10, IL-17A, and TNF-α. Mice immunized with the conjugate together with Freund’s adjuvant and calcium phosphate adjuvant, had 100% and 70% survival after stimulation with A. baumannii, respectively (50). Li et al. created a vaccine the glycoprotein CTB4573C‐CPS (C‐CPS) against A. baumannii by introducing the O-linked glycosylation system into the host strain. Mice immunized with C-CPS showed a significant increase in the serum-specific IgG antibody level. The survival rate of immunized mice under attack by different A. baumannii strains was 70% to 100% (51). CPS has good immunogenicity and can provide protection against A. baumannii stimulation, so it is a promising candidate subunit vaccine. Further research is needed on the toxicity of CPS.
2.3. Outer membrane vesicles
Outer membrane vesicles (OMVs) are spherical vesicles produced by gram-negative bacteria (137). OMVs play a role in pathogenesis, intercellular communication, and stress responses, as well as in immune regulation and the establishment and balance of the gut microbiota (138). OMVs consist of various proteins (such as OmpA), lipopolysaccharides (LPS), phospholipids, DNA and RNA (139–141). They are naturally occurring candidate subunit vaccines containing multiple candidate vaccine components. Micheal et al. immunized mice with OMVs produced by A. baumannii ATCC19606 and produced specific IgG and IgM in serum. In the A. baumannii sepsis model, the survival rate of immunized mice was 87.5% to 100%. The bacterial loads in the lungs and proinflammatory factor levels in the serum of immunized mice were reduced compared with those in the control (52). Huang et al. immunized mice with OMVs derived from clinically multi-resistant A. baumannii strains. In the sepsis model, the survival rate of actively immunized mice was 73.3% and that of passively immunized mice was 100% (53). Marina et al. immunized mice with LPS-free OMVs. Under challenge with A. baumannii, 10 μg of OMVs provided 75% protection, and 100 μg of OMV provided complete protection (54).
The OMVs produced by A. baumannii are toxic. LPS-deficient OMVs have low toxicity, high safety, and high immunogenicity and can protect against A. baumannii attacks. LPS-deficient OMVs are promising subunit vaccine candidates with the potential for clinical translation. However, LPS-deficient OMVs are less immunogenic than those produced by wild-type A. baumannii. Improving the immunogenicity of LPS-deficient OMVs is a priority. Fusion of immune cell membranes, such as those of neutrophils, with LPS-deficient OMVs may eliminate their toxicity and improve their immunogenicity (142, 143).
3. Adjuvants
Adjuvants are a class of substances added to vaccine preparations that improve the immunogenicity of vaccine antigens (144). Reasonable addition of different adjuvants can improve the immune efficacy of vaccines and regulate the immune balance of the body. Adjuvants used in FDA-licensed human vaccines include aluminum salts, MF59, AS01B, AS03, AS04, and CpG ODN (145).
Aluminum adjuvants were the earliest adjuvants to be developed and are the most widely used adjuvants. These adjuvants mainly stimulate humoral immunity, produce a high titer of IgG, and activate Th2 cells (146, 147). Aluminum adjuvants are dispersed in colloidal form in liquids, which is unsuitable for membrane filtration; therefore, membrane filtration cannot guarantee sterility. Aluminum adjuvants also cannot be frozen or lyophilized. Freund’s adjuvant is a water-in-oil emulsion that can cause granulomas and have other adverse effects after injection, so it cannot be used in the manufacture of human vaccines (148). Freund’s adjuvant is still widely used in animal experiments because of its strong adjuvant effect and affordable cost (27, 50, 149). MF59 and AS03 are oil-in-water adjuvants that overcome the shortcomings of Freund’s adjuvant and are effective adjuvants in many vaccines. The fundamental mechanism of action of oil-in-water adjuvant is chemokine-driven cellular immune cell recruitment. MF59 can induce cellular and humoral immunity and produce functional antibodies with high titers. AS03 stimulates the immune system by activating NF-κB, producing proinflammatory cytokines and chemokines, recruiting immune cells (monocytes and macrophages), and inducing high antibody titers (148, 150). CpG ODN is an immune booster that enhances the antibody response and polarizes to the Th1 profile (151, 152). AS01 and AS04 are complex adjuvants, which are combinations of different adjuvants, that enhance the immune response (148). Heat-labile enterotoxin (LT) and cholera toxin (CT) are bacterial toxins extracted from bacteria and are the most promising mucosal adjuvants.
With further in-depth study of adjuvants, especially the development of materials science, the diversity of available adjuvants has greatly increased. Freund’s adjuvant and aluminum adjuvants were the first to be studied, followed composite adjuvants and cytokine adjuvants. Nanomaterials such as CS, PLGA, gold nanoparticles, silver nanoparticles and mesoporous silica have also been shown to have special adjuvant effects (153–158). Bacterial OMVs are natural functional nanomaterials that can be used as drug carriers and adjuvants (159, 160). The OMVs of plants are rarely studied and may also have adjuvant effects. The role of adjuvants has changed from simply inducing innate immunity at the beginning to regulating the level and type of immunity in a systematic manner. Our research team has also explored in A. baumannii nanovaccines. The CS-PLGA-rOmp22 nanovaccine was constructed by combining CS, PLGA, and rOmp22 of A. baumannii. Immunizing mice with CS-PLGA-rOmp22 greatly enhanced the immune effect (21). Nanomaterials have great application prospects in subunit vaccines and are worthy of further exploration.
4. Common immune routes
The common routes of vaccine immunization are intramuscular injection, subcutaneous injection, intraperitoneal injection and mucosal immunization. Muscle tissue is tight, body fluid levels are moderate, and blood vessels are abundant, and these features are conducive to antigen and adjuvant residence. Antigen-induced proinflammatory factors rapidly recruit leukocytes in the blood and lymphatic circulation to infiltrate the injection site (161). Intramuscular injection is easy to perform and widely applicable. Many vaccines are administered intramuscularly, such as the hepatitis B vaccine, and tetanus vaccine. The subcutaneous injection site is located in the connective tissue of the fat layer below the dermis, and the presence of fat can play a significant role in the storage of vaccines (162, 163). Dermal tissue is rich in dendritic cells, macrophages, and a large number of memory T and B cells. Immune cells can take up antigens in subcutaneous tissue through cellular osmosis and present them to the skin for induction of immunity (164, 165). Subcutaneous immunization is suitable for vaccines with a slightly higher dose and a slightly higher risk of adverse reactions. The common vaccines that confer immunity through subcutaneous administration include the inactivated plague vaccine and varicella attenuated live vaccine. The abdominal cavity can accommodate large-volume injections, which are more common for vaccines in animal immunity experiments.
The mucosal immune system is the largest component of the immune system and plays an extremely important role in the process of fighting infection (166). Both mucosal and systemic immune responses can be produced during induction of mucosal immunity. Not only can specific IgA be produced locally but also specific IgG and IgM can be produced in serum (25, 167). A quadrivalent live attenuated influenza vaccine (QLAIV), FluMist/Fluenz, was approved for use in the United States of America (USA) in 2012 and the European Union (EU) in 2013 (168, 169). This representative mucosal vaccine is a nasal spray vaccine. The protective effects of the COVID-19 vaccine and Pseudomonas aeruginosa vaccine after nasal mucosal immunization are strong (170–172). Mucosal immunity has the advantages of ease of operation, good safety, relatively few adverse reactions, low cost, and good compliance (173). Mucosal immunity is a promising immunization route, and has been widely studied.
5. Animal models
The most relevant experimental animal model should satisfy several key conditions. First, it should offer a heterogeneous immunogenic response. Second, its genetic background should be well defined. BALB/c mice are the most common animals used to evaluate the immunogenicity and protection effects of A. baumannii subunit vaccines, C57BL/6, and ICR mice are also used in some research (Table 1) (24–26, 30). Some studies have proved that BALB/c and C57BL/6 mice have different immune responses after vaccine administration, Th1 immune response and IFN-γ production dominated in C57BL/6 mice, while BALB/c easily triggered Th2 immune response (174). Floris Fransen et al. indicated that BALB/c, but not C57BL/6 mice had genetic predisposition to produce polyreactive IgAs, has a strong impact on the generation of antigen-specific IgAs (175). We speculate that BALB/c mice are more suitable for A. baumannii mucosal immunization vaccine development. It is better to select both BALB/c and C57BL/c mouse models for a more comprehensive assessment of the efficacy of the vaccine.
The specific animal experimental process is shown in Figure 1. After immunizing mice subcutaneously, intramuscularly or intranasally with A. baumannii subunit vaccine, serum and saliva were collected to detect specific antibodies, immune cells from spleen and lymph nodes were analyzed by flow cytometry, and various cytokines (IFN-γ, IL-4, IL-17) in the spleen supernatant were detected. To test the protective effect of the subunit vaccine, the immunized mice were challenged with A. baumannii strains. Common used A. baumannii infection models include pneumonia, bloodstream infection, and wound infection models. Clinically, lung infections are more common, such as those contracted during ventilator use, tracheal intubation, and mechanical ventilation. Therefore, the pneumonia model has better application prospects, and it is more meaningful for researchers to use the pneumonia model. After challenged with A. baumannii strains, survival rate, weight change, clinical score of the immunized and non-immunized mice, as well as bacterial load and pathology of each organ are observed as protective indicators of A. baumannii subunit vaccines.
Bacterial load and pathology injury are important indicators to evaluate the protective efficacy of vaccine. In the pneumonia model, bacterial load in blood and lung tissue and the degree of inflammation in the lung tissue are usually measured. In bloodstream infection model, which is a systemic infection, could affect the main organs, bacterial load in blood, lung, spleen, liver, kidney and heart, and the pathology of lung, spleen and liver are often detected (Figure 1). A. baumannii bloodstream infection probably causes bacterial endocarditis and produces bacterial valve growth. Although there was no study has examined the pathology of endocarditis, studies indicate that the heart bacterial load in the immunized group was significantly lower than that in the non-immunized group (143, 176). Therefore, selection of indicators based on different infection model is of great significance for the comprehensive evaluation of the protective efficacy of the vaccine.
6. Conclusion and prospects
A. baumannii, as an ESKAPE pathogen, has caused serious harm to global public health (5–8). Vaccines are effective tools to prevent and control A. baumannii infection (10). As shown in Figure 3, vaccine immunization could induce cellular immunity and humoral immunity, Th1 and Th2 cells secrete IL-4, IL-10, and so on, which activate B cells and induce them to secrete specific antibodies. Meanwhile, a large number of memory T and B cells are generated, which can induce a rapid immune response when exposed to A. baumannii again. Subunit vaccines have been widely studied because of their high purity, safety, and stability, ease of production and strong targeted immune response (13). Because of the high cost and time investment associated with the A. baumannii subunit vaccine, novel research results have been obtained. Several candidate subunit vaccines have been studied and found to provide partial or complete protection. OmpA, Omp33-36, Ata, LPS-deficient OMVs and BamA are safe, immunogenic and have strong protective effects against A. baumannii infection, exhibiting prospects for further development and research.
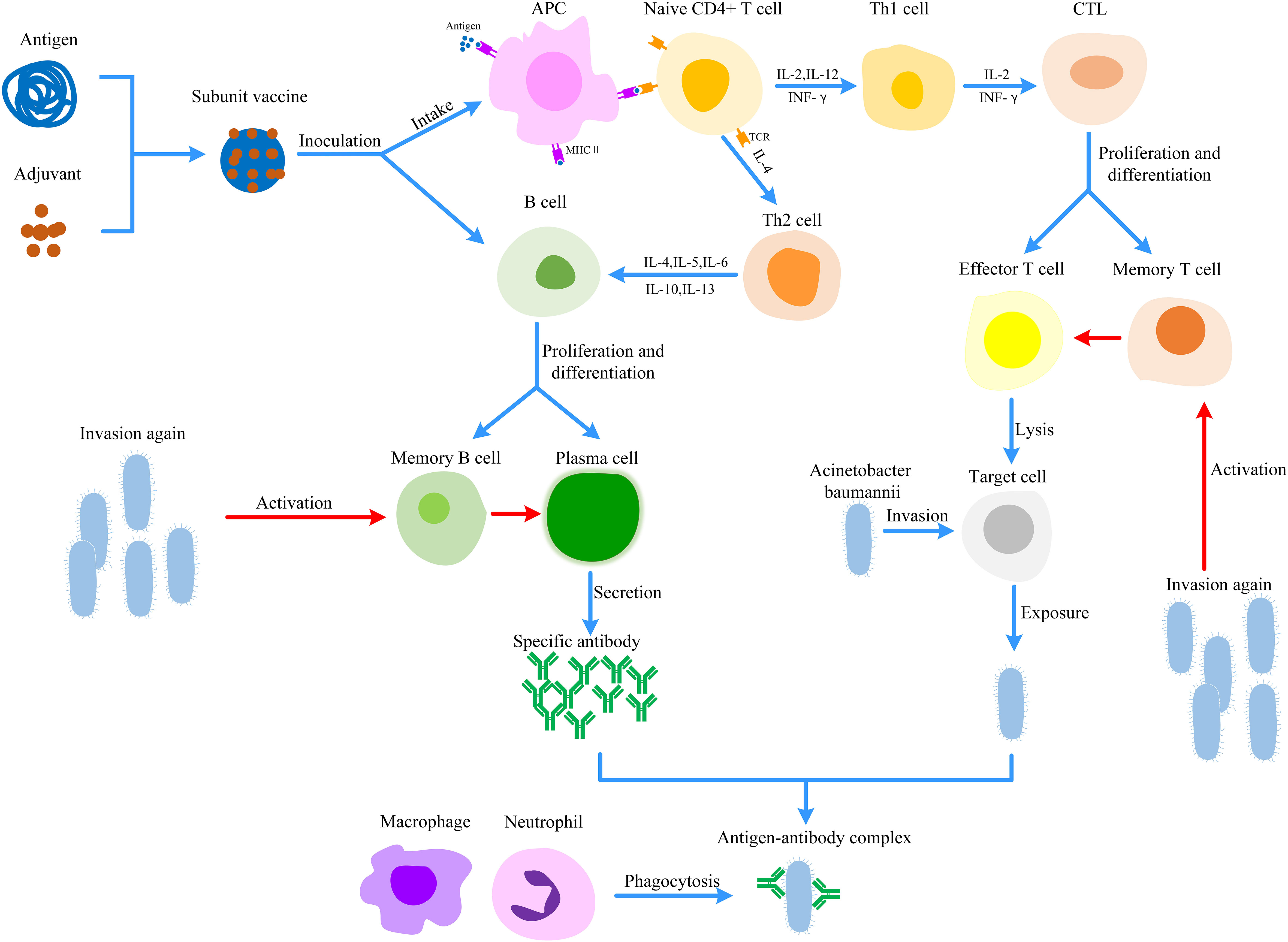
Figure 3 Protective mechanism of Acinetobacter baumannii subunit vaccine. Antigens can be taken up and digested into fragments by APC. These antigen fragments are recognized by TCR on CD4+ T cells, which activates cellular immunity to lysis target cells. In parallel, subunit vaccines or cytokines could activate B cells to induce humoral immunity. Once A. baumannii invades the body again, memory T cells and B cells could induce rapid and effective immune responses. (APC, Antigen-presenting cell; CTL, Cytotoxic T lymphocyte; MHC, Major histocompatibility complex; TCR, T cell receptor).
More and more antigen candidates for the A. baumannii subunit vaccine have been predicted and discovered by using proteomics, reverse vaccinology, pan-genomics, core genomics, immunoinformatics, and biophysical analyses (17, 18). Different candidate antigens, such as pure OMPs and detoxified LPS, combined to form a new antigen that can enhance the immune efficacy of vaccines, which is a new idea for vaccine design (19). For particular clinical strains, some unique proteins and metabolic systems involved in the immune escape, nutrient acquisition mechanism, and community interaction of A. baumannii may affect the survival of the bacteria. Using these proteins as candidate antigens also offers potential for the development of subunit vaccines (177, 178).
Adjuvants used in FDA-licensed human vaccines include aluminum salts, MF59, AS01B, AS03, AS04, and CpG ODN (145). Freund’s adjuvant is a water-in-oil emulsion that can cause granulomas and other adverse effects after injection, so Freund’s adjuvant cannot be used in the manufacture of human vaccines (148). Freund’s adjuvant is still widely used in animal experiments due to its strong adjuvant effect, immunological enhancement and affordable cost. LT and CT are the most commonly used and promising mucosal adjuvants. Nanomaterials, such as CS, PLGA, gold nanoparticles, silver nanoparticles, mesoporous silicon, and bacterial OMVs, have special adjuvant effects and have attracted the attention of many researchers. The application of nanomaterials as adjuvants in vaccine research is a new and promising direction.
The common methods of vaccine immunization are intramuscular injection, subcutaneous injection, intraperitoneal injection and mucosal immunization. Based on the characteristics of the vaccine, different immunization routes should be chosen. Mucosal immunity is an emerging research hotspot. Mucosal immunity can induce not only the mucosal immune response but also the humoral immune response (25, 167). Mucosal immunity has the advantages of ease of operation, good safety, relatively few adverse reactions, low cost, and good compliance (173).
BALB/c mice, C57BL/6 mice, and ICR mice are three experimental mice of the A. baumannii subunit vaccine. Common used animal infection models for A. baumannii include pneumonia, bloodstream infection, and wound infection models. Based on the actual situation, different researchers use different animals, infection models and adjuvants. This review summarized the adjuvants, infection models and immunization routes used by different vaccine candidates. By using the same animal, same adjuvant, and same infection model, the immune effects of different vaccines could be compared.
In conclusion, subunit vaccine is one of the effective methods to prevent and control A. baumannii infection. So far, no A. baumannii subunit vaccine candidate has entered clinical trials. Emerging approaches such as bioinformatics, proteomics, immunoinformatics, biophysical analyses, and reverse vaccinology have played an important role in vaccine candidate screening, protein epitope selection, vaccine spatial structure construction, and vaccine immunogenicity detection. In this review, we summarized the candidate antigens, adjuvants, immunization routes, and animal models for the research of A. baumannii subunit vaccines. We also provide opinions and suggestions on novel vaccine development, hoping to guide current and future research on A. baumannii subunit vaccines.
Author contributions
NY primarily wrote the manuscript and drew the figures aided by XD. CZ and FG searched the literatures. XJ and ZW collated the information in Table 1, and revised the draft. GF and XD conceived and edited the manuscript. All authors contributed to the article and approved the submitted version.
Funding
This work was partially funded by the National Natural Science Foundation of China (82100014, 82070017, 81870009), Natural Science Foundation of Jiangsu Province (BK20210981), Basic Science Foundation of Jiangsu Universities (21KJB320002), and Key Project of Innovation Training for College Students of Jiangsu Province (202110312008Z).
Conflict of interest
The authors declare that the research was conducted in the absence of any commercial or financial relationships that could be construed as a potential conflict of interest.
Publisher’s note
All claims expressed in this article are solely those of the authors and do not necessarily represent those of their affiliated organizations, or those of the publisher, the editors and the reviewers. Any product that may be evaluated in this article, or claim that may be made by its manufacturer, is not guaranteed or endorsed by the publisher.
References
1. Antunes LC, Visca P, Towner KJ. Acinetobacter baumannii: evolution of a global pathogen. Pathog Dis (2014) 71(3):292–301. doi: 10.1111/2049-632X.12125
2. Ayoub Moubareck C, Hammoudi Halat D. Insights into acinetobacter baumannii: A review of microbiological, virulence, and resistance traits in a threatening nosocomial pathogen. Antibiot (Basel) (2020) 9(3). doi: 10.3390/antibiotics9030119
3. Karageorgopoulos DE FM. Current control and treatment of multidrug-resistant acinetobacter baumannii infections. Lancet Infect Dis (2008) 8(12):751–62. doi: 10.1016/S1473-3099(08)70279-2
4. Howard A, O'Donoghue M, Feeney A, Sleator RD. Acinetobacter baumannii: an emerging opportunistic pathogen. Virulence (2012) 3(3):243–50. doi: 10.4161/viru.19700
5. Vazquez-Lopez R, Solano-Galvez SG, Juarez Vignon-Whaley JJ, Abello Vaamonde JA, Padro Alonzo LA, Rivera Resendiz A, et al. Acinetobacter baumannii resistance: A real challenge for clinicians. Antibiot (Basel) (2020) 9(4). doi: 10.3390/antibiotics9040205
6. Nasr P. Genetics, epidemiology, and clinical manifestations of multidrug-resistant acinetobacter baumannii. J Hosp Infect (2020) 104(1):4–11. doi: 10.1016/j.jhin.2019.09.021
7. Nang SC, Azad MAK, Velkov T, Zhou QT, Li J. Rescuing the last-line polymyxins: Achievements and challenges. Pharmacol Rev (2021) 73(2):679–728. doi: 10.1124/pharmrev.120.000020
8. Chokshi A, Sifri Z, Cennimo D, Horng H. Global contributors to antibiotic resistance. J Glob Infect Dis (2019) 11(1):36–42. doi: 10.4103/jgid.jgid_110_18
9. Murray CJL, Ikuta KS, Sharara F, Swetschinski L, Robles Aguilar G, Gray A, et al. Global burden of bacterial antimicrobial resistance in 2019: a systematic analysis. Lancet (2022) 399(10325):629–55. doi: 10.1016/S0140-6736(21)02724-0
10. Kennedy DA, Read AF. Why does drug resistance readily evolve but vaccine resistance does not? Proc Biol Sci (2017) 284(1851). doi: 10.1098/rspb.2016.2562
11. Garcia-Quintanilla M PM, McConnell MJ. First steps towards a vaccine against acinetobacter baumannii. Curr Pharm Biotechnol (2013) 14(10):897–902. doi: 10.3967/bes2018.019
12. Ahmad TA, Tawfik DM, Sheweita SA, Haroun M, El-Sayed LH. Development of immunization trials against acinetobacter baumannii. Trials Vaccinol (2016) 5:53–60. doi: 10.1016/j.trivac.2016.03.001
13. Hajissa K, Zakaria R, Suppian R, Mohamed Z. Epitope-based vaccine as a universal vaccination strategy against toxoplasma gondii infection: A mini-review. J Adv Vet Anim Res (2019) 6(2):174–82. doi: 10.5455/javar.2019.f329
14. Singh R, Capalash N, Sharma P. Vaccine development to control the rising scourge of antibiotic-resistant acinetobacter baumannii: A systematic review. 3 Biotech (2022) 12(3):85. doi: 10.1007/s13205-022-03148-9
15. Khalaj-Hedayati A, Chua CLL, Smooker P, Lee KW. Nanoparticles in influenza subunit vaccine development: Immunogenicity enhancement. Influenza Other Respir Viruses (2020) 14(1):92–101. doi: 10.1111/irv.12697
16. Azim KF, Hasan M, Hossain MN, Somana SR, Hoque SF, Bappy MNI, et al. Immunoinformatics approaches for designing a novel multi epitope peptide vaccine against human norovirus (Norwalk virus). Infect Genet Evol (2019) 74:103936. doi: 10.1016/j.meegid.2019.103936
17. Hassan A, Naz A, Obaid A, Paracha RZ, Naz K, Awan FM, et al. Pangenome and immuno-proteomics analysis of acinetobacter baumannii strains revealed the core peptide vaccine targets. BMC Genomics (2016) 17(1):732. doi: 10.1186/s12864-016-2951-4
18. Ud-Din M, Albutti A, Ullah A, Ismail S, Ahmad S, Naz A, et al. Vaccinomics to design a multi-epitopes vaccine for acinetobacter baumannii. Int J Environ Res Public Health (2022) 19(9). doi: 10.3390/ijerph19095568
19. Tawfik DM, Ahmad TA, Sheweita SA, Haroun M, El-Sayed LH. The detection of antigenic determinants of acinetobacter baumannii. Immunol Lett (2017) 186:59–67. doi: 10.1016/j.imlet.2017.04.004
20. Abdollahi S, Raoufi Z, Fakoor MH. Physicochemical and structural characterization, epitope mapping and vaccine potential investigation of a new protein containing tetratrico peptide repeats of acinetobacter baumannii: An in-silico and in-vivo approach. Mol Immunol (2021) 140:22–34. doi: 10.1016/j.molimm.2021.10.004
21. Du X, Xue J, Jiang M, Lin S, Huang Y, Deng K, et al. A multiepitope peptide, rOmp22, encapsulated in chitosan-PLGA nanoparticles as a candidate vaccine against acinetobacter baumannii infection. Int J Nanomed (2021) 16:1819–36. doi: 10.2147/IJN.S296527
22. Raoufi Z, Abdollahi S, Armand R. DcaP porin and its epitope-based subunit promise effective vaccines against acinetobacter baumannii; in-silico and in-vivo approaches. Microb Pathog (2022) 162:105346. doi: 10.1016/j.micpath.2021.105346
23. Song X, Zhao G, Ding M. Antigen epitope developed based on acinetobacter baumannii MacB protein can provide partial immune protection in mice. BioMed Res Int (2020) 2020:1975875. doi: 10.1155/2020/1975875
24. Luo G, Lin L, Ibrahim AS, Baquir B, Pantapalangkoor P, Bonomo RA, et al. Active and passive immunization protects against lethal, extreme drug resistant-acinetobacter baumannii infection. PloS One (2012) 7(1):e29446. doi: 10.1371/journal.pone.0029446
25. Zhang X, Yang T, Cao J, Sun J, Dai W, Zhang L. Mucosal immunization with purified OmpA elicited protective immunity against infections caused by multidrug-resistant acinetobacter baumannii. Microb Pathog (2016) 96:20–5. doi: 10.1016/j.micpath.2016.04.019
26. Bolourchi N, Shahcheraghi F, Shirazi AS, Janani A, Bahrami F, Badmasti F. Immunogenic reactivity of recombinant PKF and AbOmpA proteins as serum resistance factors against sepsis of acinetobacter baumannii. Microb Pathog (2019) 131:9–14. doi: 10.1016/j.micpath.2019.03.031
27. Golestani F, Malekan M, Rasooli I, Jahangiri A, Ramezanalizadeh F, Chaudhuri S, et al. Immunogenicity of loop 3 of Omp34 from a. baumannii in loopless c-lobe of TbpB of n. meningitidis. Int Immunopharmacol (2022) 110:109013. doi: 10.1016/j.intimp.2022.109013
28. Akbari Z, Rasooli I, Ghaini MH, Chaudhuri S, Farshchi Andisi V, Jahangiri A, et al. BauA and Omp34 surface loops trigger protective antibodies against acinetobacter baumannii in a murine sepsis model. Int Immunopharmacol (2022) 108:108731. doi: 10.1016/j.intimp.2022.108731
29. Naghipour Erami A, Rasooli I, Jahangiri A, Darvish Alipour Astaneh S. Anti-Omp34 antibodies protect against acinetobacter baumannii in a murine sepsis model. Microb Pathog (2021) 161(Pt B):105291. doi: 10.1016/j.micpath.2021.105291
30. Huang W, Yao Y, Wang S, Xia Y, Yang X, Long Q, et al. Immunization with a 22-kDa outer membrane protein elicits protective immunity to multidrug-resistant acinetobacter baumannii. Sci Rep (2016) 6:20724. doi: 10.1038/srep20724
31. Guo SJ, Ren S, Xie YE. Evaluation of the protective efficacy of a fused OmpK/Omp22 protein vaccine candidate against acinetobacter baumannii infection in mice. BioMed Environ Sci (2018) 31(2):155–8. doi: 10.3967/bes2018.019
32. Yang AQ, Yang HY, Guo SJ, Xie YE. MF59 adjuvant enhances the immunogenicity and protective immunity of the OmpK/Omp22 fusion protein from acineterbacter baumannii through intratracheal inoculation in mice. Scand J Immunol (2019) 90(1):e12769. doi: 10.1111/sji.12769
33. Huang W, Wang S, Yao Y, Xia Y, Yang X, Long Q, et al. OmpW is a potential target for eliciting protective immunity against acinetobacter baumannii infections. Vaccine (2015) 33(36):4479–85. doi: 10.1016/j.vaccine.2015.07.031
34. Hatefi Oskuei R, Darvish Alipour Astaneh S, Rasooli I. A conserved region of acinetobacter trimeric autotransporter adhesion, ata, provokes suppression of acinetobacter baumannii virulence. Arch Microbiol (2021) 203(6):3483–93. doi: 10.1007/s00203-021-02343-1
35. Sun P, Li X, Pan C, Liu Z, Wu J, Wang H, et al. A short peptide of autotransporter ata is a promising protective antigen for vaccination against acinetobacter baumannii. Front Immunol (2022) 13:884555. doi: 10.3389/fimmu.2022.884555
36. Garg N, Singh R, Shukla G, Capalash N, Sharma P. Immunoprotective potential of in silico predicted acinetobacter baumannii outer membrane nuclease, NucAb. Int J Med Microbiol (2016) 306(1):1–9. doi: 10.1016/j.ijmm.2015.10.005
37. Singh R, Capalash N, Sharma P. Immunoprotective potential of BamA, the outer membrane protein assembly factor, against MDR acinetobacter baumannii. Sci Rep (2017) 7(1):12411. doi: 10.1038/s41598-017-12789-3
38. Rasooli I, Abdolhamidi R, Jahangiri A, Darvish Alipour Astaneh S. Outer membrane protein, Oma87 prevents acinetobacter baumannii infection. Int J Pept Res Ther (2020) 26(4):2653–60. doi: 10.1007/s10989-020-10056-0
39. Chaudhuri S, Rasooli I, Oskouei RH, Pishgahi M, Jahangir A, Andisi VF, et al. Hybrid antigens expressing surface loops of BauA from acinetobacter baumannii are capable of inducing protection against infection. Front Immunol (2022) 13:933445. doi: 10.3389/fimmu.2022.933445
40. Singh R, Garg N, Shukla G, Capalash N, Sharma P. Immunoprotective efficacy of acinetobacter baumannii outer membrane protein, FilF, predicted in silico as a potential vaccine candidate. Front Microbiol (2016) 7:158. doi: 10.3389/fmicb.2016.00158
41. Ren S, Guan L, Dong Y, Wang C, Feng L, Xie Y. Design and evaluation of a multi-epitope assembly peptide vaccine against acinetobacter baumannii infection in mice. Swiss Med Wkly (2019) 149:w20052. doi: 10.4414/smw.2019.20052
42. Mahmoudi Z, Rasooli I, Jahangiri A, Darvish Alipour Astaneh S. Prevention of nosocomial acinetobacter baumannii infections with a conserved immunogenic fimbrial protein. APMIS (2020) 128(7):476–83. doi: 10.1111/apm.13061
43. Ramezanalizadeh F, Owlia P, Rasooli I. Type I pili, CsuA/B and FimA induce a protective immune response against acinetobacter baumannii. Vaccine (2020) 38(34):5436–46. doi: 10.1016/j.vaccine.2020.06.052
44. Fattahian Y, Rasooli I, Mousavi Gargari SL, Rahbar MR, Darvish Alipour Astaneh S, Amani J. Protection against acinetobacter baumannii infection via its functional deprivation of biofilm associated protein (Bap). Microb Pathog (2011) 51(6):402–6. doi: 10.1016/j.micpath.2011.09.004
45. Badmasti F, Ajdary S, Bouzari S, Fooladi AA, Shahcheraghi F, Siadat SD. Immunological evaluation of OMV(PagL)+Bap(1-487aa) and AbOmpA(8-346aa)+Bap(1-487aa) as vaccine candidates against acinetobacter baumannii sepsis infection. Mol Immunol (2015) 67(2 Pt B):552–8. doi: 10.1016/j.molimm.2015.07.031
46. Skerniskyte J, Karazijaite E, Deschamps J, Krasauskas R, Armalyte J, Briandet R, et al. Blp1 protein shows virulence-associated features and elicits protective immunity to acinetobacter baumannii infection. BMC Microbiol (2019) 19(1):259. doi: 10.1186/s12866-019-1615-3
47. Pazoki M, Darvish Alipour Astaneh S, Ramezanalizadeh F, Jahangiri A, Rasooli I. Immunoprotectivity of valine-glycine repeat protein G, a potent mediator of pathogenicity, against acinetobacter baumannii. Mol Immunol (2021) 135:276–84. doi: 10.1016/j.molimm.2021.04.026
48. Alipouri S, Rasooli I, Ghaini MH, Jahangiri A, Darvish Alipour Astaneh S, Ramezanalizadeh F. Immunity induced by valine-glycine repeat protein G imparts histoprotection of vital body organs against acinetobacter baumannii. J Genet Eng Biotechnol (2022) 20(1):42. doi: 10.1186/s43141-022-00325-4
49. Badmasti F, Habibi M, Firoozeh F, Fereshteh S, Bolourchi N, Goodarzi NN. The combination of CipA and PBP-7/8 proteins contribute to the survival of C57BL/6 mice from sepsis of acinetobacter baumannii. Microb Pathog (2021) 158:105063. doi: 10.1016/j.micpath.2021.105063
50. Rudenko N KA, Zamyatina A, Shepelyakovskaya A, Semushina S, Brovko F, Shpirt A, et al. Immune response to conjugates of fragments of the type K9 capsular polysaccharide of acinetobacter baumannii with carrier proteins. Microbiol Spectr (2022) 18:e0167422. doi: 10.1128/spectrum.01674-22
51. Li X, Pan C, Liu Z, Sun P, Hua X, Feng E, et al. Safety and immunogenicity of a new glycoengineered vaccine against acinetobacter baumannii in mice. Microb Biotechnol (2022) 15(2):703–16. doi: 10.1111/1751-7915.13770
52. McConnell MJ, Rumbo C, Bou G, Pachon J. Outer membrane vesicles as an acellular vaccine against acinetobacter baumannii. Vaccine (2011) 29(34):5705–10. doi: 10.1016/j.vaccine.2011.06.001
53. Huang W, Yao Y, Long Q, Yang X, Sun W, Liu C, et al. Immunization against multidrug-resistant acinetobacter baumannii effectively protects mice in both pneumonia and sepsis models. PloS One (2014) 9(6):e100727. doi: 10.1371/journal.pone.0100727
54. Pulido MR, Garcia-Quintanilla M, Pachon J, McConnell MJ. A lipopolysaccharide-free outer membrane vesicle vaccine protects against acinetobacter baumannii infection. Vaccine (2020) 38(4):719–24. doi: 10.1016/j.vaccine.2019.11.043
55. Kim YC, Dema B, Reyes-Sandoval A. COVID-19 vaccines: Breaking record times to first-in-human trials. NPJ Vaccines (2020) 5(1):34. doi: 10.1038/s41541-020-0188-3
56. Gellings PS, Wilkins AA, Morici LA. Recent advances in the pursuit of an effective acinetobacter baumannii vaccine. Pathogens (2020) 9(12). doi: 10.3390/pathogens9121066
57. Beiranvand S, Doosti A, Mirzaei SA. Putative novel b-cell vaccine candidates identified by reverse vaccinology and genomics approaches to control acinetobacter baumannii serotypes. Infect Genet Evol (2021) 96:105138. doi: 10.1016/j.meegid.2021.105138
58. Bazmara H, Rasooli I, Jahangiri A, Sefid F, Astaneh SDA, Payandeh Z. Antigenic properties of iron regulated proteins in acinetobacter baumannii: An in silico approach. Int J Pept Res Ther (2017) 25(1):205–13. doi: 10.1007/s10989-017-9665-6
59. Kim SW, Choi CH, Moon DC, Jin JS, Lee JH, Shin JH, et al. Serum resistance of acinetobacter baumannii through the binding of factor h to outer membrane proteins. FEMS Microbiol Lett (2009) 301(2):224–31. doi: 10.1111/j.1574-6968.2009.01820.x
60. Choi CH, Lee JS, Lee YC, Park TI, Lee JC. Acinetobacter baumannii invades epithelial cells and outer membrane protein a mediates interactions with epithelial cells. BMC Microbiol (2008) 8:216. doi: 10.1186/1471-2180-8-216
61. Choi CH, Hyun SH, Lee JY, Lee JS, Lee YS, Kim SA, et al. Acinetobacter baumannii outer membrane protein a targets the nucleus and induces cytotoxicity. Cell Microbiol (2008) 10(2):309–19. doi: 10.1111/j.1462-5822.2007.01041.x
62. Choi CH, Lee EY, Lee YC, Park TI, Kim HJ, Hyun SH, et al. Outer membrane protein 38 of acinetobacter baumannii localizes to the mitochondria and induces apoptosis of epithelial cells. Cell Microbiol (2005) 7(8):1127–38. doi: 10.1111/j.1462-5822.2005.00538.x
63. Gaddy JA, Tomaras AP, Actis LA. The acinetobacter baumannii 19606 OmpA protein plays a role in biofilm formation on abiotic surfaces and in the interaction of this pathogen with eukaryotic cells. Infect Immun (2009) 77(8):3150–60. doi: 10.1128/IAI.00096-09
64. Lee JS, Lee JC, Lee C-M, Jung ID, Jeong Y-I, Seong E-Y, et al. Outer membrane protein a of acinetobacter baumannii induces differentiation of CD4+ T cells toward a Th1 polarizing phenotype through the activation of dendritic cells. Biochem Pharmacol (2007) 74(1):86–97. doi: 10.1016/j.bcp.2007.02.012
65. Confer AW, Ayalew S. The OmpA family of proteins: roles in bacterial pathogenesis and immunity. Vet Microbiol (2013) 163(3-4):207–22. doi: 10.1016/j.vetmic.2012.08.019
66. An Z, Huang X, Zheng C, Ding W. Acinetobacter baumannii outer membrane protein a induces HeLa cell autophagy via MAPK/JNK signaling pathway. Int J Med Microbiol (2019) 309(2):97–107. doi: 10.1016/j.ijmm.2018.12.004
67. Jahangiri A, Owlia P, Rasooli I, Salimian J, Derakhshanifar E, Naghipour Erami A, et al. Specific egg yolk antibodies (IgY) confer protection against acinetobacter baumannii in a murine pneumonia model. J Appl Microbiol (2019) 126(2):624–32. doi: 10.1111/jam.14135
68. Jahangiri A, Owlia P, Rasooli I, Salimian J, Derakhshanifar E, Aghajani Z, et al. Specific egg yolk immunoglobulin as a promising non-antibiotic biotherapeutic product against acinetobacter baumannii pneumonia infection. Sci Rep (2021) 11(1):1914. doi: 10.1038/s41598-021-81356-8
69. Lin L, Tan B, Pantapalangkoor P, Ho T, Hujer AM, Taracila MA, et al. Acinetobacter baumannii rOmpA vaccine dose alters immune polarization and immunodominant epitopes. Vaccine (2013) 31(2):313–8. doi: 10.1016/j.vaccine.2012.11.008
70. Tamehri M, Rasooli I, Pishgahi M, Jahangiri A, Ramezanalizadeh F, Banisaeed Langroodi SR. Combination of BauA and OmpA elicit immunoprotection against acinetobacter baumannii in a murine sepsis model. Microb Pathogen (2022) 173. doi: 10.1016/j.micpath.2022.105874
71. Smani Y, McConnell MJ, Pachon J. Role of fibronectin in the adhesion of acinetobacter baumannii to host cells. PloS One (2012) 7(4):e33073. doi: 10.1371/journal.pone.0033073
72. Smani Y, Dominguez-Herrera J, Pachon J. Association of the outer membrane protein Omp33 with fitness and virulence of acinetobacter baumannii. J Infect Dis (2013) 208(10):1561–70. doi: 10.1093/infdis/jit386
73. Rumbo C, Tomas M, Fernandez Moreira E, Soares NC, Carvajal M, Santillana E, et al. The acinetobacter baumannii Omp33-36 porin is a virulence factor that induces apoptosis and modulates autophagy in human cells. Infect Immun (2014) 82(11):4666–80. doi: 10.1128/IAI.02034-14
74. An Z, Su J. Acinetobacter baumannii outer membrane protein 34 elicits NLRP3 inflammasome activation via mitochondria-derived reactive oxygen species in RAW264.7 macrophages. Microbes Infect (2019) 21(3-4):143–53. doi: 10.1016/j.micinf.2018.10.005
75. Islam AH, Singh KK, Ismail A. Demonstration of an outer membrane protein that is antigenically specific for acinetobacter baumannii. Diagn Microbiol Infect Dis (2011) 69(1):38–44. doi: 10.1016/j.diagmicrobio.2010.09.008
76. Jahangiri A, Rasooli I, Owlia P, Imani Fooladi AA, Salimian J. Highly conserved exposed immunogenic peptides of Omp34 against acinetobacter baumannii: An innovative approach. J Microbiol Methods (2018) 144:79–85. doi: 10.1016/j.mimet.2017.11.008
77. Mesbahi Moghaddam M, Rasooli I, Ghaini MH, Jahangiri A, Ramezanalizadeh F, Ghasemkhah Tootkleh R. Immunoprotective characterization of egg yolk immunoglobulin raised to loop 3 of outer membrane protein 34 (Omp34) in a murine model against acinetobacter baumannii. Mol Immunol (2022) 149:87–93. doi: 10.1016/j.molimm.2022.06.010
78. Siroy A, Cosette P, Seyer D, Lemaître-Guillier C, Vallenet D, Van Dorsselaer A, et al. Global comparison of the membrane subproteomes between a multidrug-resistant acinetobacter baumannii strain and a reference strain. J Proteome Res (2006) 5(12):3385–98. doi: 10.1021/pr060372s
79. Gil-Marqués ML, Pachón J YS. iTRAQ-based quantitative proteomic analysis of acinetobacter baumannii under hypoxia and normoxia reveals the role of OmpW as a virulence factor. Microbiol Spectr (2022) 10(2):e0232821. doi: 10.1128/spectrum.02328-21
80. Catel-Ferreira M, Marti S, Guillon L, Jara L, Coadou G, Molle V, et al. The outer membrane porin OmpW of acinetobacter baumannii is involved in iron uptake and colistin binding. FEBS Lett (2016) 590(2):224–31. doi: 10.1002/1873-3468.12050
81. Weidensdorfer M, Ishikawa M, Hori K, Linke D, Djahanschiri B, Iruegas R, et al. The acinetobacter trimeric autotransporter adhesin ata controls key virulence traits of acinetobacter baumannii. Virulence (2019) 10(1):68–81. doi: 10.1080/21505594.2018.1558693
82. Bentancor LV, Camacho-Peiro A, Bozkurt-Guzel C, Pier GB, Maira-Litran T. Identification of ata, a multifunctional trimeric autotransporter of acinetobacter baumannii. J Bacteriol (2012) 194(15):3950–60. doi: 10.1128/JB.06769-11
83. Bentancor LV, Routray A, Bozkurt-Guzel C, Camacho-Peiro A, Pier GB, Maira-Litran T. Evaluation of the trimeric autotransporter ata as a vaccine candidate against acinetobacter baumannii infections. Infect Immun (2012) 80(10):3381–8. doi: 10.1128/IAI.06096-11
84. Heun M, Binnenkade L, Kreienbaum M, Thormann KM. Functional specificity of extracellular nucleases of shewanella oneidensis MR-1. Appl Environ Microbiol (2012) 78(12):4400–11. doi: 10.1128/AEM.07895-11
85. Liechti GW, Goldberg JB. Helicobacter pylori salvages purines from extracellular host cell DNA utilizing the outer membrane-associated nuclease NucT. J Bacteriol (2013) 195(19):4387–98. doi: 10.1128/JB.00388-13
86. Uchiyama S, Andreoni F, Schuepbach RA, Nizet V, Zinkernagel AS. DNase Sda1 allows invasive M1T1 group a streptococcus to prevent TLR9-dependent recognition. PloS Pathog (2012) 8(6):e1002736. doi: 10.1371/journal.ppat.1002736
87. Juneau RA, Stevens JS, Apicella MA, Criss AK. A thermonuclease of neisseria gonorrhoeae enhances bacterial escape from killing by neutrophil extracellular traps. J Infect Dis (2015) 212(2):316–24. doi: 10.1093/infdis/jiv031
88. Hasegawa T, Minami M, Okamoto A, Tatsuno I, Isaka M, Ohta M. Characterization of a virulence-associated and cell-wall-located DNase of streptococcus pyogenes. Microbiol (Reading) (2010) 156(Pt 1):184–90. doi: 10.1099/mic.0.031955-0
89. Berends ET, Horswill AR, Haste NM, Monestier M, Nizet V, von Kockritz-Blickwede M. Nuclease expression by staphylococcus aureus facilitates escape from neutrophil extracellular traps. J Innate Immun (2010) 2(6):576–86. doi: 10.1159/000319909
90. Vieira de Araujo AE, Conde LV, da Silva Junior HC, de Almeida Machado L, Lara FA, Chapeaurouge A, et al. Cross-reactivity and immunotherapeutic potential of BamA recombinant protein from acinetobacter baumannii. Microbes Infect (2021) 23(4-5):104801. doi: 10.1016/j.micinf.2021.104801
91. Albrecht R, Schutz M, Oberhettinger P, Faulstich M, Bermejo I, Rudel T, et al. Structure of BamA, an essential factor in outer membrane protein biogenesis. Acta Crystallogr D Biol Crystallogr (2014) 70(Pt 6):1779–89. doi: 10.1107/S1399004714007482
92. Jiang JH, Tong J, Tan KS, Gabriel K. From evolution to pathogenesis: the link between beta-barrel assembly machineries in the outer membrane of mitochondria and gram-negative bacteria. Int J Mol Sci (2012) 13(7):8038–50. doi: 10.3390/ijms13078038
93. Dorsey CW, Tomaras AP, Connerly PL, Tolmasky ME, Crosa JH, Actis LA. The siderophore-mediated iron acquisition systems of acinetobacter baumannii ATCC 19606 and vibrio anguillarum 775 are structurally and functionally related. Microbiol (Reading) (2004) 150(Pt 11):3657–67. doi: 10.1099/mic.0.27371-0
94. Gaddy JA, Arivett BA, McConnell MJ, Lopez-Rojas R, Pachon J, Actis LA. Role of acinetobactin-mediated iron acquisition functions in the interaction of acinetobacter baumannii strain ATCC 19606T with human lung epithelial cells, galleria mellonella caterpillars, and mice. Infect Immun (2012) 80(3):1015–24. doi: 10.1128/IAI.06279-11
95. Sefid F, Rasooli I, Jahangiri A. In silico determination and validation of baumannii acinetobactin utilization a structure and ligand binding site. BioMed Res Int (2013) 2013:172784. doi: 10.1155/2013/172784
96. Sefid F, Rasooli I, Jahangiri A, Bazmara H. Functional exposed amino acids of BauA as potential immunogen against acinetobacter baumannii. Acta Biotheor (2015) 63(2):129–49. doi: 10.1007/s10441-015-9251-2
97. Esmaeilkhani H, Rasooli I, Nazarian S, Sefid F. In vivo validation of the immunogenicity of recombinant baumannii acinetobactin utilization a protein (rBauA). Microb Pathog (2016) 98:77–81. doi: 10.1016/j.micpath.2016.06.032
98. Sangroodi YH, Rasooli I, Nazarian S, Ebrahimizadeh W, Sefid F. Immunogenicity of conserved cork and beta-barrel domains of baumannii acinetobactin utilization protein in an animal model. Turk J Med Sci (2015) 45(6):1396–402. doi: 10.3906/sag-1407-45
99. Cabral MP, Soares NC, Aranda J, Parreira JR, Rumbo C, Poza M, et al. Proteomic and functional analyses reveal a unique lifestyle for acinetobacter baumannii biofilms and a key role for histidine metabolism. J Proteome Res (2011) 10(8):3399–417. doi: 10.1021/pr101299j
100. Bhamidimarri SP, Zahn M, Prajapati JD, Schleberger C, Soderholm S, Hoover J, et al. A multidisciplinary approach toward identification of antibiotic scaffolds for acinetobacter baumannii. Structure (2019) 27(2):268–80.e6. doi: 10.1016/j.str.2018.10.021
101. Shin JH, Lee HW, Kim SM, Kim J. Proteomic analysis of acinetobacter baumannii in biofilm and planktonic growth mode. J Microbiol (2009) 47(6):728–35. doi: 10.1007/s12275-009-0158-y
102. Cerveny L, Straskova A, Dankova V, Hartlova A, Ceckova M, Staud F, et al. Tetratricopeptide repeat motifs in the world of bacterial pathogens: role in virulence mechanisms. Infect Immun (2013) 81(3):629–35. doi: 10.1128/IAI.01035-12
103. Goebl M YM. The TPR snap helix: A novel protein repeat motif from mitosis to transcription. Trends Biochem Sci (1991) 16(5):173–7. doi: 10.1016/0968-0004(91)90070-C
104. Blatch GL LM. The tetratricopeptide repeat: A structural motif mediating protein-protein interactions. Bioessays (1999) 21(11):932–9. doi: 10.1002/(SICI)1521-1878(199911)21:11<932::AID-BIES5>3.0.CO;2-N
105. D'Andrea LD, Regan L. TPR proteins: The versatile helix. Trends Biochem Sci (2003) 28(12):655–62. doi: 10.1016/j.tibs.2003.10.007
106. Moriel DG, Beatson SA, Wurpel DJ, Lipman J, Nimmo GR, Paterson DL, et al. Identification of novel vaccine candidates against multidrug-resistant acinetobacter baumannii. PloS One (2013) 8(10):e77631. doi: 10.1371/journal.pone.0077631
107. Hospenthal MK, Costa TRD, Waksman G. A comprehensive guide to pilus biogenesis in gram-negative bacteria. Nat Rev Microbiol (2017) 15(6):365–79. doi: 10.1038/nrmicro.2017.40
108. Werneburg GT, Thanassi DG. Pili assembled by the Chaperone/Usher pathway in escherichia coli and salmonella. EcoSal Plus. (2018) 8(1). doi: 10.1128/ecosalplus.ESP-0007-2017
109. Spaulding CN, HLt S, Zheng W, KW D, JE H, MS C, et al. Functional role of the type 1 pilus rod structure in mediating host-pathogen interactions. Elife (2018) 7. doi: 10.7554/eLife.31662
110. Pakharukova N, Garnett JA, Tuittila M, Paavilainen S, Diallo M, Xu Y, et al. Structural insight into archaic and alternative chaperone-usher pathways reveals a novel mechanism of pilus biogenesis. PloS Pathog (2015) 11(11):e1005269. doi: 10.1371/journal.ppat.1005269
111. Lasa I, Penades JR. Bap: A family of surface proteins involved in biofilm formation. Res Microbiol (2006) 157(2):99–107. doi: 10.1016/j.resmic.2005.11.003
112. Loehfelm TW, Luke NR, Campagnari AA. Identification and characterization of an acinetobacter baumannii biofilm-associated protein. J Bacteriol (2008) 190(3):1036–44. doi: 10.1128/JB.01416-07
113. Goh HM, Beatson SA, Totsika M, Moriel DG, Phan MD, Szubert J, et al. Molecular analysis of the acinetobacter baumannii biofilm-associated protein. Appl Environ Microbiol (2013) 79(21):6535–43. doi: 10.1128/AEM.01402-13
114. Rahbar MR, Rasooli I, Mousavi Gargari SL, Amani J, Fattahian Y. In silico analysis of antibody triggering biofilm associated protein in acinetobacter baumannii. J Theor Biol (2010) 266(2):275–90. doi: 10.1016/j.jtbi.2010.06.014
115. Ranjbar A, Rasooli I, Jahangiri A, Ramezanalizadeh F. Specific egg yolk antibody raised to biofilm associated protein (Bap) is protective against murine pneumonia caused by acinetobacter baumannii. Sci Rep (2022) 12(1):12576. doi: 10.1038/s41598-022-16894-w
116. Darzi Eslam E, Darvish Alipour Astaneh S, Rasooli I, Nazarian S, Jahangiri A. Passive immunization with chitosan-loaded biofilm-associated protein against acinetobacter baumannii murine infection model. Gene Rep (2020) 20. doi: 10.1016/j.genrep.2020.100708
117. De Gregorio E, Del Franco M, Martinucci M, Roscetto E, Zarrilli R, Di Nocera PP. Biofilm-associated proteins: News from acinetobacter. BMC Genomics (2015) 16:933. doi: 10.1186/s12864-015-2136-6
118. Wang J, Zhou Z, He F, Ruan Z, Jiang Y, Hua X, et al. The role of the type VI secretion system vgrG gene in the virulence and antimicrobial resistance of acinetobacter baumannii ATCC 19606. PloS One (2018) 13(2):e0192288. doi: 10.1371/journal.pone.0192288
119. Repizo GD, Espariz M, Seravalle JL, Salcedo SP. Bioinformatic analysis of the type VI secretion system and its potential toxins in the acinetobacter genus. Front Microbiol (2019) 10:2519. doi: 10.3389/fmicb.2019.02519
120. Debroy R, Miryala SK, Naha A, Anbarasu A, Ramaiah S. Gene interaction network studies to decipher the multi-drug resistance mechanism in salmonella enterica serovar typhi CT18 reveal potential drug targets. Microb Pathog (2020) 142:104096. doi: 10.1016/j.micpath.2020.104096
121. Okada U, Yamashita E, Neuberger A, Morimoto M, van Veen HW, Murakami S. Crystal structure of tripartite-type ABC transporter MacB from acinetobacter baumannii. Nat Commun (2017) 8(1):1336. doi: 10.1038/s41467-017-01399-2
122. Turner L, Praszkier J, Hutton ML, Steer D, Ramm G, Kaparakis-Liaskos M, et al. Increased outer membrane vesicle formation in a helicobacter pylori tolB mutant. Helicobacter (2015) 20(4):269–83. doi: 10.1111/hel.12196
123. Song X, Zhang H, Zhang D, Xie W, Zhao G. Bioinformatics analysis and epitope screening of a potential vaccine antigen TolB from acinetobacter baumannii outer membrane protein. Infect Genet Evol (2018) 62:73–9. doi: 10.1016/j.meegid.2018.04.019
124. Clavel T, Germon P, Vianney A, Portalier R, Lazzaroni JC. TolB protein of escherichia coli K-12 interacts with the outer membrane peptidoglycan-associated proteins pal, lpp and OmpA. Mol Microbiol (1998) 29(1):359–67. doi: 10.1046/j.1365-2958.1998.00945.x
125. Bouveret E, Derouiche R, Rigal A, Lloubès R, Lazdunski C, Benedeti H. Peptidoglycan-associated lipoprotein-TolB interaction. a possible key to explaining the formation of contact sites between the inner and outer membranes of escherichia coli. J Biol Chem (1995) 270(19):11071–7. doi: 10.1074/jbc.270.19.11071
126. Ray MC, Germon P, Vianney A, Portalier R, Lazzaroni JC. Identification by genetic suppression of escherichia coli TolB residues important for TolB-pal interaction. J Bacteriol (2000) 182(3):821–4. doi: 10.1128/JB.182.3.821-824.2000
127. Koenigs A SJ, Averhoff B, Göttig S, Wichelhaus TA, Wallich R, Zipfel PF, et al. CipA of acinetobacter baumannii is a novel plasminogen binding and complement inhibitory protein. J Infect Dis (2016) 213(9):1388–99. doi: 10.1093/infdis/jiv601
128. Russo TA, MacDonald U, Beanan JM, Olson R, MacDonald IJ, Sauberan SL, et al. Penicillin-binding protein 7/8 contributes to the survival of acinetobacter baumannii in vitro and in vivo. J Infect Dis (2009) 199(4):513–21. doi: 10.1086/596317
129. Ahmad S, Azam SS. A novel approach of virulome based reverse vaccinology for exploring and validating peptide-based vaccine candidates against the most troublesome nosocomial pathogen: Acinetobacter baumannii. J Mol Graph Model (2018) 83:1–11. doi: 10.1016/j.jmgm.2018.04.020
130. Fereshteh S, Abdoli S, Shahcheraghi F, Ajdary S, Nazari M, Badmasti F. New putative vaccine candidates against acinetobacter baumannii using the reverse vaccinology method. Microb Pathog (2020) 143:104114. doi: 10.1016/j.micpath.2020.104114
131. Zadeh Hosseingholi E, Zarrini G, Pashazadeh M, Gheibi Hayat SM, Molavi G. In silico identification of probable drug and vaccine candidates against antibiotic-resistant acinetobacter baumannii. Microb Drug Resist (2020) 26(5):456–67. doi: 10.1089/mdr.2019.0236
132. Talyansky Y, Nielsen TB, Yan J, Carlino-Macdonald U, Di Venanzio G, Chakravorty S, et al. Capsule carbohydrate structure determines virulence in acinetobacter baumannii. PloS Pathog (2021) 17(2):e1009291. doi: 10.1371/journal.ppat.1009291
133. Russo TA, Luke NR, Beanan JM, Olson R, Sauberan SL, MacDonald U, et al. The K1 capsular polysaccharide of acinetobacter baumannii strain 307-0294 is a major virulence factor. Infect Immun (2010) 78(9):3993–4000. doi: 10.1128/IAI.00366-10
134. Shashkov AS KJ, Senchenkova SN, Shneider MM, Popova AV, Arbatsky NP, Miroshnikov KA, et al. Acinetobacter baumannii K27 and K44 capsular polysaccharides have the same K unit but different structures due to the presence of distinct wzy genes in otherwise closely related K gene clusters. Glycobiology (2016) 26(5):501–8. doi: 10.1093/glycob/cwv168
135. Russo TA, Beanan JM, Olson R, MacDonald U, Cox AD, St Michael F, et al. The K1 capsular polysaccharide from acinetobacter baumannii is a potential therapeutic target via passive immunization. Infect Immun (2013) 81(3):915–22. doi: 10.1128/IAI.01184-12
136. Yang FL, Lou TC, Kuo SC, Wu WL, Chern J, Lee YT, et al. A medically relevant capsular polysaccharide in acinetobacter baumannii is a potential vaccine candidate. Vaccine (2017) 35(10):1440–7. doi: 10.1016/j.vaccine.2017.01.060
137. McBroom AJ, Johnson AP, Vemulapalli S, Kuehn MJ. Outer membrane vesicle production by escherichia coli is independent of membrane instability. J Bacteriol (2006) 188(15):5385–92. doi: 10.1128/JB.00498-06
138. Sartorio MG, Pardue EJ, Feldman MF, Haurat MF. Bacterial outer membrane vesicles: From discovery to applications. Annu Rev Microbiol (2021) 75:609–30. doi: 10.1146/annurev-micro-052821-031444
139. Kesavan D, Vasudevan A, Wu L, Chen J, Su Z, Wang S, et al. Integrative analysis of outer membrane vesicles proteomics and whole-cell transcriptome analysis of eravacycline induced acinetobacter baumannii strains. BMC Microbiol (2020) 20(1):31. doi: 10.1186/s12866-020-1722-1
140. Kwon SO, Gho YS, Lee JC, Kim SI. Proteome analysis of outer membrane vesicles from a clinical acinetobacter baumannii isolate. FEMS Microbiol Lett (2009) 297(2):150–6. doi: 10.1111/j.1574-6968.2009.01669.x
141. Lee EY, Choi DS, Kim KP, Gho YS. Proteomics in gram-negative bacterial outer membrane vesicles. Mass Spectrom Rev (2008) 27(6):535–55. doi: 10.1002/mas.20175
142. Angsantikul P, Fang RH, Zhang L. Toxoid vaccination against bacterial infection using cell membrane-coated nanoparticles. Bioconjug Chem (2018) 29(3):604–12. doi: 10.1021/acs.bioconjchem.7b00692
143. Zhou J, Ventura CJ, Yu Y, Gao W, Fang RH, Zhang L. Biomimetic neutrophil nanotoxoids elicit potent immunity against acinetobacter baumannii in multiple models of infection. Nano Lett (2022) 22(17):7057–65. doi: 10.1021/acs.nanolett.2c01948
144. Reed SG, Orr MT, Fox CB. Key roles of adjuvants in modern vaccines. Nat Med (2013) 19(12):1597–608. doi: 10.1038/nm.3409
145. Shi S, Zhu H, Xia X, Liang Z, Ma X, Sun B. Vaccine adjuvants: Understanding the structure and mechanism of adjuvanticity. Vaccine (2019) 37(24):3167–78. doi: 10.1016/j.vaccine.2019.04.055
146. He P, Zou Y, Hu Z. Advances in aluminum hydroxide-based adjuvant research and its mechanism. Hum Vaccin Immunother (2015) 11(2):477–88. doi: 10.1080/21645515.2014.1004026
147. Gherardi RK, Aouizerate J, Cadusseau J, Yara S, Authier FJ. Aluminum adjuvants of vaccines injected into the muscle: Normal fate, pathology and associated disease. Morphologie (2016) 100(329):85–94. doi: 10.1016/j.morpho.2016.01.002
148. Apostolico Jde S, Lunardelli VA, Coirada FC, Boscardin SB, Rosa DS. Adjuvants: Classification, modus operandi, and licensing. J Immunol Res (2016) 2016:1459394. doi: 10.1155/2016/1459394
149. Stills HF Jr. Adjuvants and antibody production: Dispelling the myths associated with freund's complete and other adjuvants. ILAR J (2005) 46(3):280–93. doi: 10.1093/ilar.46.3.280
150. Garçon N VD, Didierlaurent AM. Development and evaluation of AS03, an adjuvant system containing α-tocopherol and squalene in an oil-in-water emulsion. Expert Rev Vaccines (2012) 11(3):349–66. doi: 10.1586/erv.11.192
151. Hartmann G, Battiany J, Poeck H, Wagner M, Kerkmann M, Lubenow N, et al. Rational design of new CpG oligonucleotides that combine b cell activation with high IFN-alpha induction in plasmacytoid dendritic cells. Eur J Immunol (2003) 33(6):1633–41. doi: 10.1002/eji.200323813
152. Marshall JD, Hessel EM, Gregorio J, Abbate C, Yee P, Chu M, et al. Novel chimeric immunomodulatory compounds containing short CpG oligodeoxyribonucleotides have differential activities in human cells. Nucleic Acids Res (2003) 31(17):5122–33. doi: 10.1093/nar/gkg700
153. Carroll EC, Jin L, Mori A, Munoz-Wolf N, Oleszycka E, Moran HBT, et al. The vaccine adjuvant chitosan promotes cellular immunity via DNA sensor cGAS-STING-Dependent induction of type I interferons. Immunity (2016) 44(3):597–608. doi: 10.1016/j.immuni.2016.02.004
154. Vasiliev YM. Chitosan-based vaccine adjuvants: Incomplete characterization complicates preclinical and clinical evaluation. Expert Rev Vaccines (2015) 14(1):37–53. doi: 10.1586/14760584.2015.956729
155. Macho Fernandez E, Chang J, Fontaine J, Bialecki E, Rodriguez F, Werkmeister E, et al. Activation of invariant natural killer T lymphocytes in response to the alpha-galactosylceramide analogue KRN7000 encapsulated in PLGA-based nanoparticles and microparticles. Int J Pharm (2012) 423(1):45–54. doi: 10.1016/j.ijpharm.2011.04.068
156. Dykman LA. Gold nanoparticles for preparation of antibodies and vaccines against infectious diseases. Expert Rev Vaccines (2020) 19(5):465–77. doi: 10.1080/14760584.2020.1758070
157. Xu L, Wang YY, Huang J, Chen CY, Wang ZX, Xie H. Silver nanoparticles: Synthesis, medical applications and biosafety. Theranostics (2020) 10(20):8996–9031. doi: 10.7150/thno.45413
158. Li Q, Liu Q, Li H, Dong L, Zhou Y, Zhu J, et al. Modified hollow mesoporous silica nanoparticles as immune adjuvant-nanocarriers for photodynamically enhanced cancer immunotherapy. Front Bioeng Biotechnol (2022) 10:1039154. doi: 10.3389/fbioe.2022.1039154
159. Prior JT, Davitt C, Kurtz J, Gellings P, McLachlan JB, Morici LA. Bacterial-derived outer membrane vesicles are potent adjuvants that drive humoral and cellular immune responses. Pharmaceutics (2021) 13(2). doi: 10.3390/pharmaceutics13020131
160. Banstola A, Jeong JH, Yook S. Immunoadjuvants for cancer immunotherapy: A review of recent developments. Acta Biomater (2020) 114:16–30. doi: 10.1016/j.actbio.2020.07.063
161. Kuklin N DM, Karem K, Manickan E, Rouse BT. Induction of mucosal immunity against herpes simplex virus by plasmid DNA immunization. J Virol (1997) 71(4):3138–45. doi: 10.1128/jvi.71.4.3138-3145.1997
162. Lambert PH, Laurent PE. Intradermal vaccine delivery: will new delivery systems transform vaccine administration? Vaccine (2008) 26(26):3197–208. doi: 10.1016/j.vaccine.2008.03.095
163. Nicolas JF, Guy B. Intradermal, epidermal and transcutaneous vaccination: from immunology to clinical practice. Expert Rev Vaccines (2008) 7(8):1201–14. doi: 10.1586/14760584.7.8.1201
164. Kupper TS, Fuhlbrigge RC. Immune surveillance in the skin: mechanisms and clinical consequences. Nat Rev Immunol (2004) 4(3):211–22. doi: 10.1038/nri1310
165. Ochoa MT, Loncaric A, Krutzik SR, Becker TC, Modlin RL. "Dermal dendritic cells" comprise two distinct populations: CD1+ dendritic cells and CD209+ macrophages. J Invest Dermatol (2008) 128(9):2225–31. doi: 10.1038/jid.2008.56
166. Kim SH, Jang YS. Recent insights into cellular crosstalk in respiratory and gastrointestinal mucosal immune systems. Immune Netw (2020) 20(6):e44. doi: 10.4110/in.2020.20.e4
167. Russell MW, Moldoveanu Z, Ogra PL, Mestecky J. Mucosal immunity in COVID-19: A neglected but critical aspect of SARS-CoV-2 infection. Front Immunol (2020) 11:611337. doi: 10.3389/fimmu.2020.611337
168. Dempsey R, Tamburrino G, Schewe KE, Crowe J, Nuccitelli A, Dibben O. Haemagglutinin substitutions N125D, D127E, D222G and R223Q improve replicative fitness and vaccine effectiveness of an A/H1N1pdm09 live attenuated influenza vaccine virus by enhancing alpha-2,6 receptor binding. PloS Pathog (2022) 18(5):e1010585. doi: 10.1371/journal.ppat.1010585
169. Carter NJ, Curran MP. Live attenuated influenza vaccine (FluMist®; fluenz™) a review of its use in the prevention of seasonal influenza in children and adults. Drugs (2011) 71(12):1591–622. doi: 10.2165/11206860-000000000-00000
170. Tiboni M, Casettari L, Illum L. Nasal vaccination against SARS-CoV-2: Synergistic or alternative to intramuscular vaccines? Int J Pharm (2021) 603:120686. doi: 10.1016/j.ijpharm.2021.120686
171. Cabral MP, Correia A, Vilanova M, Gartner F, Moscoso M, Garcia P, et al. A live auxotrophic vaccine confers mucosal immunity and protection against lethal pneumonia caused by pseudomonas aeruginosa. PloS Pathog (2020) 16(2):e1008311. doi: 10.1371/journal.ppat.1008311
172. Blackwood CB, Sen-Kilic E, Boehm DT, Hall JM, Varney ME, Wong TY, et al. Innate and adaptive immune responses against bordetella pertussis and pseudomonas aeruginosa in a murine model of mucosal vaccination against respiratory infection. Vaccines (Basel) (2020) 8(4). doi: 10.3390/vaccines8040647
173. Xu H, Cai L, Hufnagel S, Cui Z. Intranasal vaccine: Factors to consider in research and development. Int J Pharm (2021) 609:121180. doi: 10.1016/j.ijpharm.2021.121180
174. Mills CD, Kincaid K, Alt JM, Heilman MJ, Hill AM. M-1/M-2 macrophages and the Th1/Th2 paradigm. J Immunol (2000) 164(12):6166–73. doi: 10.4049/jimmunol.164.12.6166
175. Fransen F, Zagato E, Mazzini E, Fosso B, Manzari C, El Aidy S, et al. BALB/c and C57BL/6 mice differ in polyreactive IgA abundance, which impacts the generation of antigen-specific IgA and microbiota diversity. Immunity (2015) 43(3):527–40. doi: 10.1016/j.immuni.2015.08.011
176. Zhou J KN, Guo Z, Ventura CJ, Holay M, Zhang Q, Wei X, et al. Nanotoxoid vaccination protects against opportunistic bacterial infections arising from immunodeficiency. Sci Adv (2022) 8(35):eabq5492. doi: 10.1126/sciadv.abq5492
177. Morris FC, Dexter C, Kostoulias X, Uddin MI, Peleg AY. The mechanisms of disease caused by acinetobacter baumannii. Front Microbiol (2019) 10:1601. doi: 10.3389/fmicb.2019.01601
Keywords: Acinetobacter baumannii, subunit vaccine, immunity, adjuvant, immunization route
Citation: Yang N, Jin X, Zhu C, Gao F, Weng Z, Du X and Feng G (2023) Subunit vaccines for Acinetobacter baumannii. Front. Immunol. 13:1088130. doi: 10.3389/fimmu.2022.1088130
Received: 03 November 2022; Accepted: 22 December 2022;
Published: 12 January 2023.
Edited by:
Susu M. Zughaier, Qatar University, QatarReviewed by:
Weiwei Huang, Chinese Academy of Medical Sciences and Peking Union Medical College, ChinaAbolfazl Jahangiri, Baqiyatallah University of Medical Sciences, Iran
Tarek A. Ahmad, Bibliotheca Alexandrina, Egypt
Iraj Rasooli, Shahed University, Iran
Copyright © 2023 Yang, Jin, Zhu, Gao, Weng, Du and Feng. This is an open-access article distributed under the terms of the Creative Commons Attribution License (CC BY). The use, distribution or reproduction in other forums is permitted, provided the original author(s) and the copyright owner(s) are credited and that the original publication in this journal is cited, in accordance with accepted academic practice. No use, distribution or reproduction is permitted which does not comply with these terms.
*Correspondence: Xingran Du, eGluZ3JhbmR1QG5qbXUuZWR1LmNu; Ganzhu Feng, Zmd6NjI2OTFAMTYzLmNvbQ==