- 1Systems Biology of Reproduction Research Group, Institute of Enzymology, Research Centre for Natural Sciences, Budapest, Hungary
- 2Doctoral School of Biology, Institute of Biology, ELTE Eötvös Loránd University, Budapest, Hungary
- 3Perinatology Research Branch, Eunice Kennedy Shriver National Institute of Child Health and Human Development, National Institutes of Health, United States Department of Health and Human Services, Detroit, MI, United States
- 4Department of Obstetrics and Gynecology, University of Michigan, Ann Arbor, MI, United States
- 5Department of Epidemiology and Biostatistics, Michigan State University, East Lansing, MI, United States
- 6Center for Molecular Medicine and Genetics, Wayne State University, Detroit, MI, United States
- 7Detroit Medical Center, Detroit, MI, United States
- 8Károly Rácz Doctoral School of Clinical Medicine, Semmelweis University, Budapest, Hungary
- 9Department of Obstetrics and Gynecology, Wayne State University, Detroit, MI, United States
- 10Department of Obstetrics and Gynecology, Universidad Del Valle, Cali, Colombia
- 11Genesis Theranostix Group, Budapest, Hungary
- 12Department of Obstetrics and Gynecology, Soroka University Medical Center, Beer Sheva, Israel
- 13Department of Obstetrics and Gynecology, Semmelweis University, Budapest, Hungary
- 14Maternity Private Clinic of Obstetrics and Gynecology, Budapest, Hungary
Introduction: Galectins are master regulators of maternal immune responses and placentation in pregnancy. Galectin-13 (gal-13) and galectin-14 (gal-14) are expressed solely by the placenta and contribute to maternal-fetal immune tolerance by inducing the apoptosis of activated T lymphocytes and the polarization of neutrophils toward an immune-regulatory phenotype.Furthermore, their decreased placental expression is associated with pregnancy complications, such as preeclampsia and miscarriage. Yet, our knowledge of the immunoregulatory role of placental galectins is incomplete.
Methods: This study aimed to investigate the effects of recombinant gal-13 and gal-14 on cell viability, apoptosis, and cytokine production of peripheral blood mononuclear cells (PBMCs) and the signaling pathways involved.
Results: Herein, we show that gal-13 and gal-14 bind to the surface of non-activated PBMCs (monocytes, natural killer cells, B cells, and T cells) and increase their viability while decreasing the rate of their apoptosis without promoting cell proliferation. We also demonstrate that gal-13 and gal-14 induce the production of interleukin (IL)-8, IL-10, and interferon-gamma cytokines in a concentration-dependent manner in PBMCs. The parallel activation of Erk1/2, p38, and NF-ĸB signaling evidenced by kinase phosphorylation in PBMCs suggests the involvement of these pathways in the regulation of the galectin-affected immune cell functions.
Discussion: These findings provide further evidence on how placenta-specific galectins assist in the establishment and maintenance of a proper immune environment during a healthy pregnancy.
Introduction
The interactions of maternal, fetal, and placental immune responses during pregnancy as well as the mechanisms that maintain maternal immune tolerance to the semi-allogeneic fetus, while guarding against microbial infection, are of great interest in reproductive medicine, even though these topics have been only partially explored (1–12). Investigators have described that time- and site-specific modifications are characteristic of these immune interactions, such as the pro-inflammatory milieu at the time of implantation, the anti-inflammatory state during the second trimester, and local and systemic inflammation in the third trimester, preceding delivery (13–17). The mediators of this complex network of maternal-fetal-placental immune interactions involve a large array of cellular, vesicular, and soluble components (8, 18–29). In the past decade, an increasing body of evidence has been collected on the key contribution of galectins to immune interactions in pregnancy (30–43).
Galectins are glycan-binding proteins belonging to the lectin subfamily, with the common property of specifically binding to glycoconjugates containing β-galactoside carbohydrates (9, 20, 44). Of the 19 galectins found in mammals, 13 are expressed in human tissues (20, 44–46). These human galectins affect a variety of cellular processes when they contact immune cells, e.g., apoptosis, cell proliferation, cell adhesion, chemotaxis, cytokine production, or degranulation (31, 39, 41, 47–52). In pregnancy, several galectins are expressed at the maternal-fetal interface and assist in regulating placental and fetal development, local and systemic inflammation as well as the establishment and maintenance of maternal immune tolerance toward the fetus (9, 38, 46, 48, 49, 53–58).
In humans, three galectins (gal-13, gal-14, and gal-16) are expressed solely by the placenta (20, 46, 57, 59). The genes encoding these galectins are located within a cluster of galectin genes and pseudogenes on chromosome 19, which arose in anthropoid primates through birth-and-death evolution (20, 46, 59, 60). This evolutionary gene duplication process led to neofunctionalization, the acquisition of a new function(s) in the duplicated genes, as measured by the differing carbohydrate-binding capacity of these placental galectins (59). At the RNA and protein levels, these are primarily expressed in the multinucleated syncytiotrophoblast layer of the placenta, formed by the fusion of mononucleated cytotrophoblast cells, but not in the underlying progenitor cytotrophoblasts (59, 61). In comparison, expression of these galectins is less abundant in the extravillous trophoblasts, amniotic epithelium, and fetal endothelium (53, 59, 60, 62).
During a healthy pregnancy, maternal blood gal-13 concentrations increase with advancing gestation and then diminish after delivery (61, 63). Notably, gal-13 is secreted into the maternal circulation from the syncytiotrophoblast (62–65), and low gal-13 concentrations were found in the maternal circulation during the first trimester in women who later developed preterm preeclampsia (66–70), a severe obstetrical syndrome with a strong systemic immune dysregulation (19, 71–76). In line with these findings, we have also demonstrated that the placental expression of gal-13 and gal-14 is down-regulated in preterm preeclampsia (60, 62, 64, 77) and in miscarriage (78).
Although several galectins have been studied extensively regarding their effects on maternal immune cells (79–85), placenta-specific galectins have been less studied in this context. We and others have explored that gal-13 and gal-14 treatment induces apoptosis of activated T cells (59, 78) and alters the cell surface expression of T-cell activation markers (78). On the other hand, gal-13 induces the production of interleukin (IL)-1ɑ and IL-6 in peripheral blood mononuclear cells (PBMC) (86) and the production of IL-8 in non-activated T cells (78), but decreases tumor necrosis factor-alpha expression in neutrophils (87). Overall, gal-13 may have a critical role in the maintenance of the physiological immune balance at the maternal-fetal interface and its lower expression may contribute to immune dysregulation in pregnancy complications such as preeclampsia, intrauterine growth restriction, and miscarriage. This phenomenon was also suggested for other galectins expressed at the maternal-fetal interface such as gal-1 and gal-3, highlighting their importance in proper placental functions (20, 36, 43, 55, 86, 88–91).
To further characterize the role of placenta-specific galectins in the regulation of maternal-fetal immune interactions, we aimed to investigate the effects of recombinant gal-13 and gal-14 on the viability, apoptosis, cytokine production, and cell signaling of PBMCs.
Materials and methods
Expression and purification of recombinant gal-13 and gal-14
Recombinant gal-13 and gal-14 were expressed in ClearColi BL21(DE3) (Lucigen, Middleton, WI, USA) bacterial strains with slight modifications, as previously described by Balogh et al. (78). All purification steps were carried out in the presence of 1mM dithiothreitol (DTT). Gal-13 and gal-14 were aliquoted in phosphate-buffered saline (PBS; Thermo Fisher Scientific, Waltham, MA, USA) supplemented with 1 mM DTT and stored at −80°C until use.
Isolation of peripheral blood mononuclear cells
Buffy coats, obtained from healthy, non-pregnant human females (n=3-5, depending on the experiment), were purchased from the Hungarian National Blood Transfusion Service (Budapest, Hungary). PBMCs were isolated by Ficoll-Paque PLUS (GE Healthcare Life Sciences, Chicago, IL, USA) density gradient centrifugation. Isolated cells were washed with PBS before the experiments. PBMCs were placed into RPMI-1640 medium (Thermo Fisher Scientific) supplemented with 10% fetal bovine serum (FBS; Thermo Fisher Scientific) and gentamicin (Thermo Fisher Scientific). Cell counts were determined with trypan blue stain and a TC20 automated cell counter (Bio-Rad, Hercules, CA, USA). Isolated PBMCs were cultured for 24–72 hours depending on the experiment. To obtain experimental replicates, all assays were repeated with multiple donors.
Binding of recombinant gal-13 and gal-14 to peripheral blood mononuclear cells
Recombinant gal-13 or gal-14, conjugated with CF488 (Mix-n-Stain CF488 Kit, Biotium, Fremont, CA, USA), according to the manufacturer’s instructions, was added to 5×105 PBMCs in PBS containing 0.5% bovine serum albumin (BSA, Sigma-Aldrich, St. Louis, MO, USA) and 0.05% sodium azide (flow cytometry wash buffer), and incubated on ice for 45 minutes. Samples were washed with wash buffer at 500g for 5 minutes at 4°C. To reduce nonspecific antibody binding, Fc receptors were blocked with human FcR blocking reagent (Miltenyi Biotec, Bergisch Gladbach, Germany) for 5 minutes on ice. Population marker antibodies (Supplementary Table 1) were added to the cells, then incubated for 20 minutes on ice in the dark. Cells were then washed twice and resuspended in wash buffer. Flow cytofluorimetric measurements were carried out on a CytoFLEX device and with CytExpert software (Beckman Coulter, Brea, CA, USA) for data acquisition. Data were collected from 50,000 cells/sample and analyzed with FlowJo v10 software (FlowJo LLC, Ashland, OR, USA).
Cell viability assay
PBMCs (2×105 cells/well) were treated with 0.04 µM, 0.4 µM, or 4 µM recombinant gal-13, gal-14, or vehicle (PBS supplemented with 1 mM DTT in the same dilution) and incubated on 96-well plates in RPMI-1640 medium supplemented with 10% FBS and gentamicin for 68 hours (at 37°C, 5% CO2). CCK-8 solution (Sigma-Aldrich) was added to the wells, and the plates were incubated for 4 hours at 37°C. Absorbance at 450 nm was measured by using an EnSpire microplate reader (Perkin Elmer, Waltham, MA, USA).
Apoptosis assay
PBMCs (4×105 cells/well) were treated with 4 µM of recombinant gal-13 or gal-14. Galectin-treated and vehicle-treated cells were incubated for 24 hours on 24-well tissue culture plates (Eppendorf, Hamburg, Germany) in RPMI-1640 medium supplemented with 10% FBS and gentamicin. PBMCs were transferred to flow cytometry tubes and washed with wash buffer at 350g for 10 minutes at 4°C. Then, cells were stained with population marker antibodies (Supplementary Table 1) to discriminate between PBMC populations. After incubation on ice for 20 minutes in the dark, cells were washed twice in wash buffer. To differentiate between early-stage and late-stage apoptotic cells, phycoerythrin-conjugated annexin V (annexin V-PE) and 7-aminoactinomycin D (7-AAD) were applied in the presence of annexin binding buffer (Annexin-V/7-AAD Apoptosis Detection Kit, BioLegend, San Diego, CA, USA). After incubation for 15 minutes in the dark at room temperature, annexin binding buffer was added, and samples were measured immediately on a CytoFLEX flow cytometer. Data were collected from 30,000 cells/sample and analyzed by using FlowJo v10 software (FlowJo, LLC).
Measurement of cell proliferation by flow cytometry
PBMCs (4×106 cells/ml) were incubated with carboxyfluorescein succinimidyl ester (CFSE) cell staining dye (BioLegend) for 20 minutes at room temperature in the dark. Next, cells were incubated for 5 minutes at room temperature in RPMI-1640 medium supplemented with 10% FBS and gentamicin. Cells were then centrifuged twice with medium at 300g for 10 minutes at 20°C. Cell concentration was determined by trypan blue staining (Bio-Rad). Overall, 5×105 cells were plated in 48-well cell culture plates (Eppendorf) in completed RPMI-1640 medium and treated with 4 μM of gal-13, gal-14, or vehicle for 72 hours (37°C, 5% CO2). Then, cells were centrifuged with PBS (350 g, 4°C, 8 minutes) and incubated for 20 minutes with Zombie Violet vitality dye (BioLegend) at room temperature. After centrifugation in wash buffer, PBMC populations were labeled with cell-specific antibodies (Supplementary Table 1). Cells were incubated for 20 minutes in the dark on ice, followed by the addition of wash buffer and centrifugation twice. Subsequently, cells were resuspended in wash buffer and measured with a CytoFLEX cytofluorimeter. Data were collected from 100,000 cells/sample, and the results were analyzed with FlowJo v10 software (FlowJo, LLC).
Measurement of cytokine production by enzyme-linked immunosorbent assay
Commercially available Human ELISA Kits for IL-8, IL-10, and interferon-gamma (IFN-γ) (Biolegend) were used to estimate the concentration of cytokines in the culture medium (RPMI-1640 supplemented with 10% FBS and gentamicin) of PBMCs treated with 0.04 µM, 0.4 µM, or 4 µM of recombinant gal-13, gal-14, or vehicle for 24 hours. The procedure was carried out by following the manufacturer’s protocol. The reaction was stopped with 4N sulfuric acid and then the absorbance was measured at 450 nm (reference filter: 620 nm) with an EnSpire microplate reader (Perkin Elmer).
Western blot for phospho-protein detection
PBMCs (6×105 cells/sample) were incubated with recombinant gal-13 (4 µM), gal-14 (4 µM), vehicle, or cell culture medium (absolute control). Samples were incubated for 10, 20, or 30 minutes at 37° C in a thermoblock (Grant Instruments Ltd., Shepreth, Cambridgeshire, United Kingdom) at 300 rpm. At the end of the treatment, samples were centrifuged at 20,000g for 30 seconds, supernatants were discarded, and cell pellets were frozen in liquid nitrogen. Then, a mixture of PBS, reducing 2× Laemmli sample buffer (Bio-Rad), protease, and phosphatase inhibitors (Thermo Fisher Scientific), was prepared and added to the frozen samples that were then heat-denatured at 95°C for 5 minutes. The electrophoretic separation was performed on 4-20% SDS-polyacrylamide gel (Thermo Fisher Scientific) in Tris-glycine buffer. Following SDS-PAGE, samples were blotted onto nitrocellulose membranes (Bio-Rad), which were blocked for 1 hour in Tris-buffered saline supplemented with 0.05% of Tween-20 (Sigma-Aldrich) (TBST) and 5% of BSA. Next, membranes were incubated overnight with rabbit anti-human primary antibodies specific to phosphorylated proteins, such as extracellular signal-regulated kinase 1/2 (Erk1/2), mitogen-activated protein kinase (MAPK) p38, or nuclear factor kappa B (NF-κB) (Cell Signaling Technology, Danvers, MA, USA) at 4°C in TBST containing 0.5% of BSA (Supplementary Table 2). The next day, membranes were incubated with horseradish peroxidase (HRP)-conjugated goat anti-rabbit IgG secondary antibody for 1 hour in TBST containing 0.5% of BSA (Sigma-Aldrich). β-actin-specific antibody was used as a loading control. Membranes were placed in an iBind Western blot device (Thermo Fisher Scientific) and incubated for 2 hours and 30 minutes with mouse anti-human β-actin (Thermo Fisher Scientific) primary antibody and HRP-conjugated rabbit anti-mouse secondary antibody (Life Technologies, Waltham, MA, USA), according to the manufacturer’s protocol (Supplementary Table 2). SuperSignal Western Pico PLUS Chemiluminescent Substrate (Thermo Fisher Scientific) was used to visualize the protein bands, and the ChemiDoc imaging device (Bio-Rad) was utilized for band detection. The relative signal intensities of phospho-proteins and β-actin were analyzed with ImageLab software (Bio-Rad).
Statistical analysis
The one-way analysis of variance test with Tukey’s post-hoc test was used to compare cell viability/proliferation, apoptosis, and cytokine production of various galectin- and vehicle-treated groups. The one-sample t-test was used to analyze Western blot experiments. All analyses were performed with GraphPad Prism 5 software (GraphPad Software, San Diego, CA, USA). To ensure the concentration-dependent effect of recombinant galectins, we performed ordered factor analysis in R environment, using linear models, in case of cell viability and cytokine production experiments. Results were considered statistically significant at p<0.05.
Results
Gal-13 and gal-14 bind differentially to T and B lymphocytes, natural killer cells, and monocytes
The binding of gal-13 and gal-14 to helper and cytotoxic T cells as well as to neutrophil granulocytes has already been demonstrated by flow cytometry (78, 87). Herein, we extended these observations by examining the binding of fluorescent gal-13 and gal-14 to other PBMC populations as well, including B cells, natural killer (NK) cells, and monocytes (Figures 1A–Q). Recombinant gal-13 and gal-14 bound to the investigated PBMC populations to a different extent. The strongest binding of both galectins to monocytes (Figures 1F–H, R, S) was observed (47-95%), followed by NK cells (27-83%) and B cells (11-51%) (Figures 1I–K, L–N, R, S, respectively). Gal-13 and gal-14 bound the least to T cells (2-12%) (Figures 1O–Q, R, S).
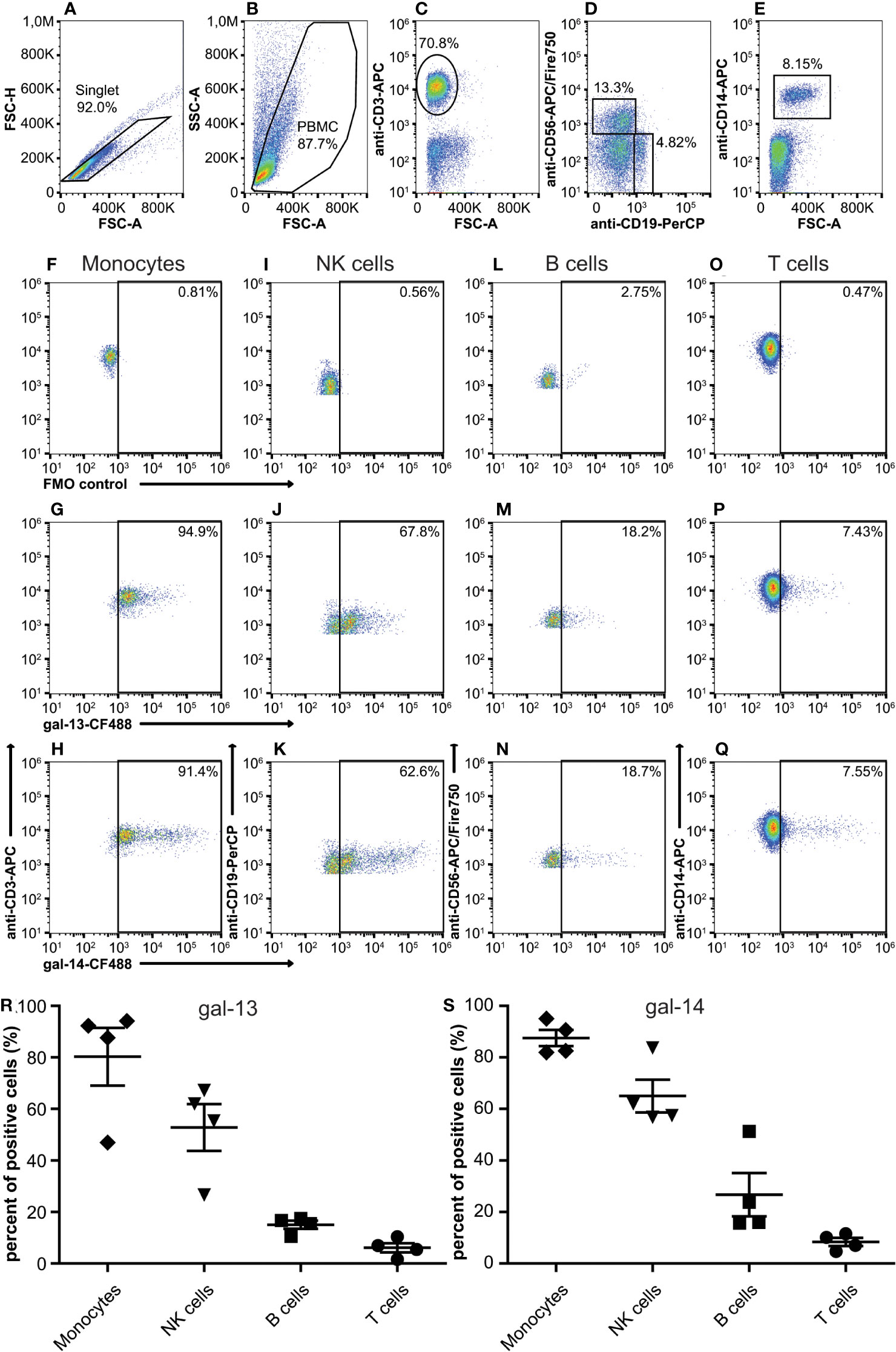
Figure 1 Binding of gal-13 and gal-14 to the peripheral blood mononuclear cells. Detection of gal-13 or gal-14 binding to human PBMCs was achieved by flow cytometry. Cells were incubated with recombinant gal-13-CF488 or gal-14-CF488. PBMCs were also stained with anti-CD3-APC, or anti-CD19-PerCP, anti-CD14-APC, and anti-CD56-APC/Fire750. Gating strategy (A–Q). Graphs show the percentage of cells to which gal-13 (R) or gal-14 (S) bound (mean ± SEM, n = 4). FMO, Fluorescence minus one; PBMC, peripheral blood mononuclear cells; SEM, standard error of the mean.
Gal-13 and gal-14 increase cell viability of immune cells through decreased apoptosis
Next, we examined the effect of recombinant gal-13 and gal-14 on the viability of PBMCs after 24 and 72 hours of treatment. Interestingly, gal-13 and gal-14 increased PBMC viability after 24 hours of treatment by 62–82% (gal-13) and 58–83% (gal-14), depending on the applied galectin concentration (Figure 2A). Ordered factor analysis revealed the significant concentration-dependent effect of both galectins (gal-13: p<0.01; gal-14: p<0.05).
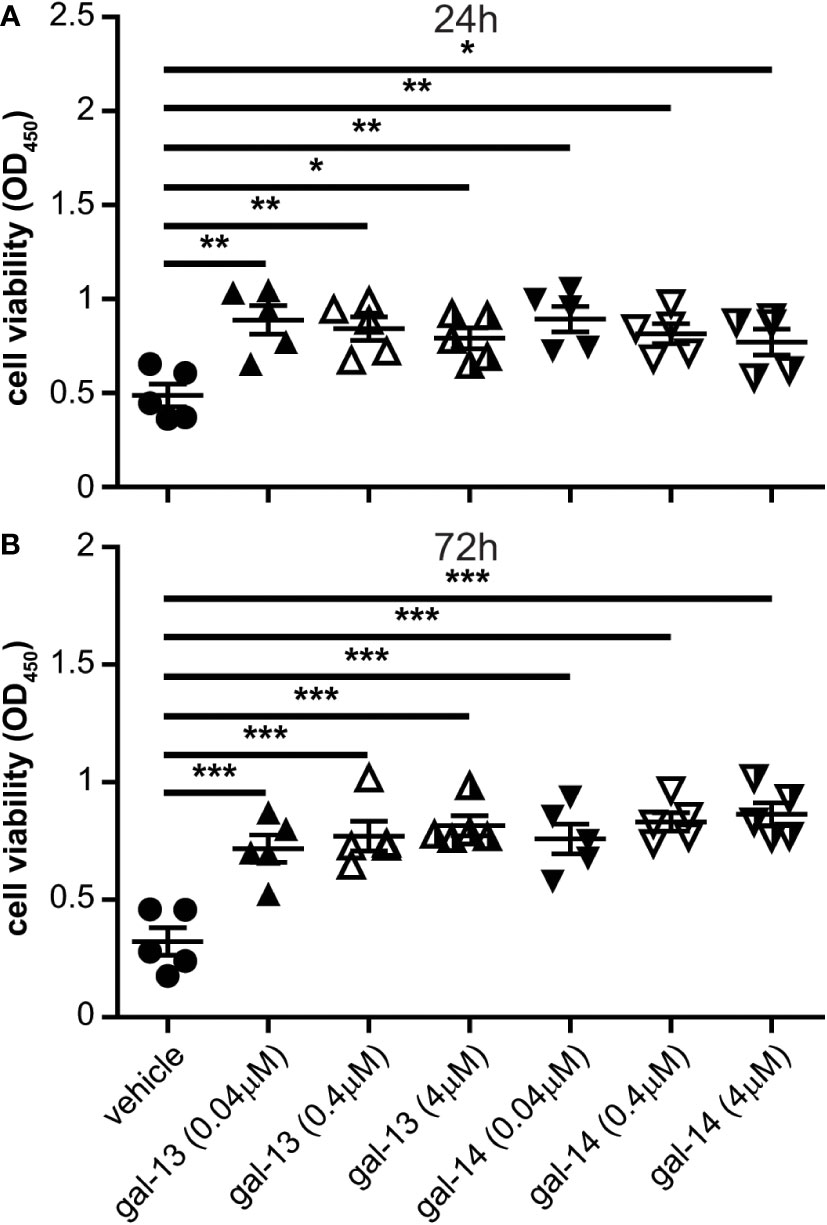
Figure 2 Gal-13 or gal-14 treatment increases the viability of immune cells. PBMCs were treated with gal-13, gal-14, or the vehicle for 24 and 72 hours. CCK-8 cell counting kit was used to examine cell viability. Graphs show the mean ± SEM of the optical density (450 nm) of viable cells after 24 hours (A) or 72 hours (B) of treatment (n=5). One-way ANOVA and Tukey post-hoc tests were used. Statistical significance was set at *p<0.05, **p<0.01, or ***p<0.001. PBMC: peripheral blood mononuclear cell, SEM: standard error of the mean.
After 72 hours of gal-13 and gal-14 administration, the viability of PBMCs showed even more of an increase than that of cells treated with the vehicle (gal-13: 123–153% increase; gal-14: 136–168% increase, depending on the galectin concentration) (Figure 2B). Herein, we also observed the significant concentration-dependent effect of both galectins (gal-13: p<10-4; gal-14: p<10-5).
To examine whether the increased cell viability could be attributed to altered apoptosis or to proliferation, the impact of gal-13 and gal-14 on the apoptosis of immune cells was then explored (Supplementary Figure 1 and Figure 3). In non-activated conditions, gal-13 and gal-14 reduced the proportion of early apoptotic T cells (gal-13: 19.6%; gal-14: 16.9%), B cells (gal-13: 13.6%; gal-14: 12.3%), NK cells (gal-13: 22.5%; gal-14: 21.7%), and monocytes (gal-13: 6.2%; gal-14: 5.9%) compared to vehicle-treated cells (Figures 3A–D). The proportion of late apoptotic B cells (Figure 3B) and monocytes (Figure 3D) was also reduced after gal-13 (by 4.2% and 12.1%, respectively) or gal-14 treatment (by 3.3% and 12.4%, respectively); however, the proportion of late apoptotic T cells and NK cells did not change upon treatment with placental galectins.
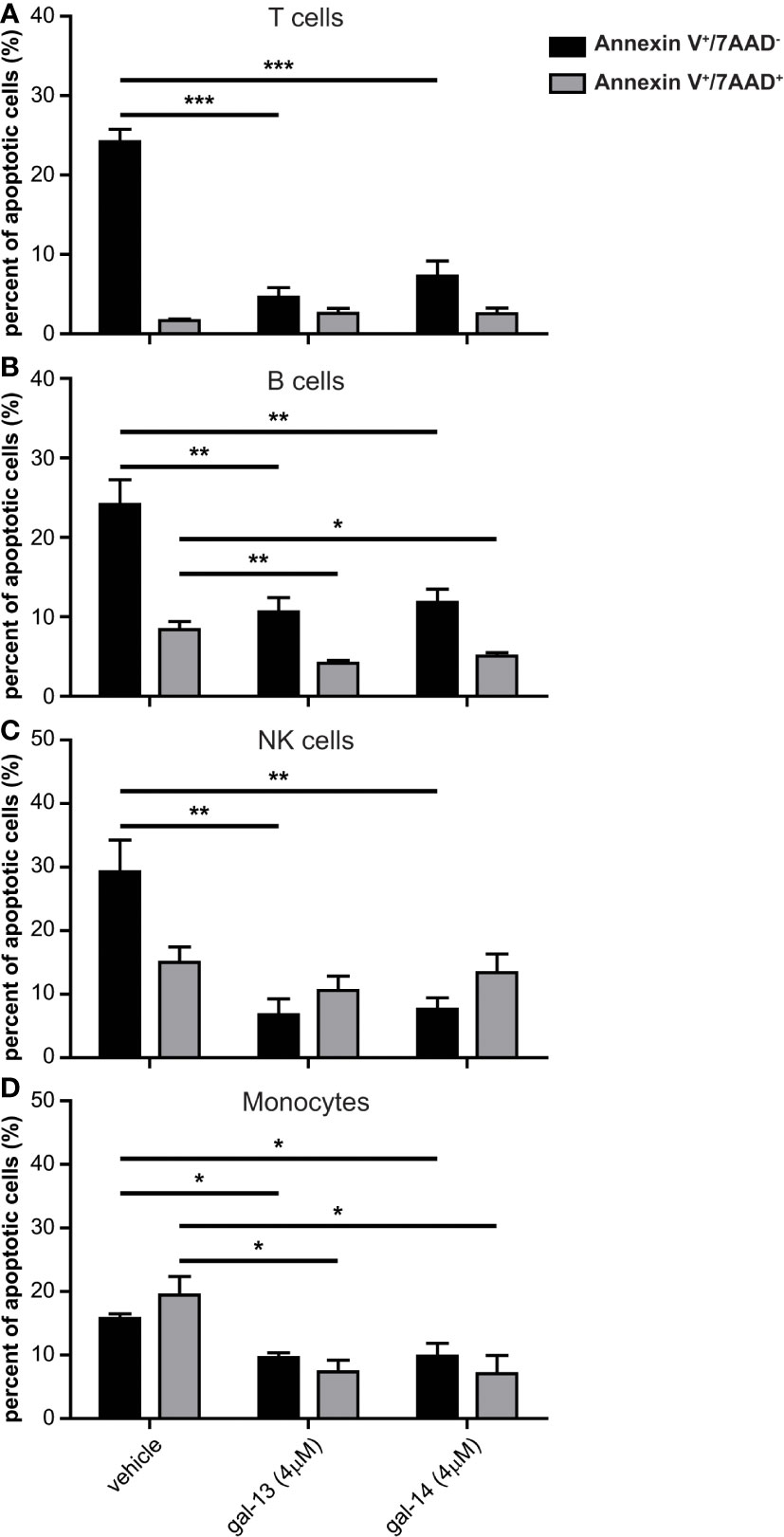
Figure 3 Gal-13 or gal-14 treatment reduces the apoptosis of PBMCs. PBMCs were incubated for 24 hours with gal-13, gal-14, or the vehicle. Graphs show the mean ± SEM of the percent of single positive (Annexin V +/7-AAD-, black bars) and double positive (Annexin V +/7-AAD +, grey bars) T lymphocytes, (A) B lymphocytes (B), NK cells (C), and monocytes (D) (n=4). One-way ANOVA and Tukey post-hoc tests were used. Statistical significance was set at *p<0.05, **p<0.01, or *** p<0.001. PBMC, peripheral blood mononuclear cell, SEM, standard error of the mean.
We also examined how gal-13 and gal-14 affect the proliferation of immune cell populations after 72 hours of treatment. Our results demonstrated that gal-13 and gal-14 had no impact on the proliferation of the PBMC populations under investigation (Supplementary Figure 2).
Gal-13 and gal-14 treatment induce IL-8, IL-10, and IFN-γ production by immune cells
Based on our previous results concerning IL-8 production of T cells (78), we were interested in how placental galectins may regulate the production of an anti-inflammatory cytokine (IL-10) and a mainly pro-inflammatory cytokine (IFN-γ), both of which are important at the maternal-fetal interface (92–94). Therefore, PBMCs were treated with three different concentrations of gal-13 or gal-14 for 24 hours or for 72 hours. In comparison to the vehicle (mean: 0.137 ng/ml), IL-8 production increased after 24 hours of gal-13 or gal-14 treatment in a dose-dependent manner, ranging from 33.89 to 150.3 ng/ml (Figures 4A, B). Furthermore, a concentration-dependent effect of galectin treatment was also detected for IL-10 secretion into cell culture supernatants, ranging from 2.361 to 5.173 ng/ml (vehicle mean: 0.003 ng/ml) (Figures 4C, D). Twenty-four hours of gal-13 or gal-14 treatment increased IFN-γ secretion by immune cells as well (vehicle mean: 3.62 pg/ml, gal-13/gal-14 mean: from 112.1 to 392.5 pg/ml), although not as robustly as seen in IL-8 and IL-10 (Figures 4E, F). Ordered factor analysis revealed the significant concentration-dependent effect of both galectins in all comparisons (gal-13: IL-8: p<10-5; IL-10: p<10-5; IFN-γ: p<10-5; gal-14: IL-8: p<10-5; IL-10: p<10-5; IFN-γ: p<0.01). Similar outcomes, but with fewer significant values, were observed after the 72-hour galectin treatment (Supplementary Figure 3). Again, we observed significant concentration-dependent effect of both galectins in all comparisons (gal-13: IL-8: p<10-5; IL-10: p<10-5; IFN-γ: p<0.01; gal-14: IL-8: p<10-5; IL-10: p<10-5; IFN-γ: p<0.01). Overall, these results showed that treatment with either gal-13 or gal-14 increased the production of certain cytokines in immune cells to various extents—IL-8 production the most and IFN-γ production the least.
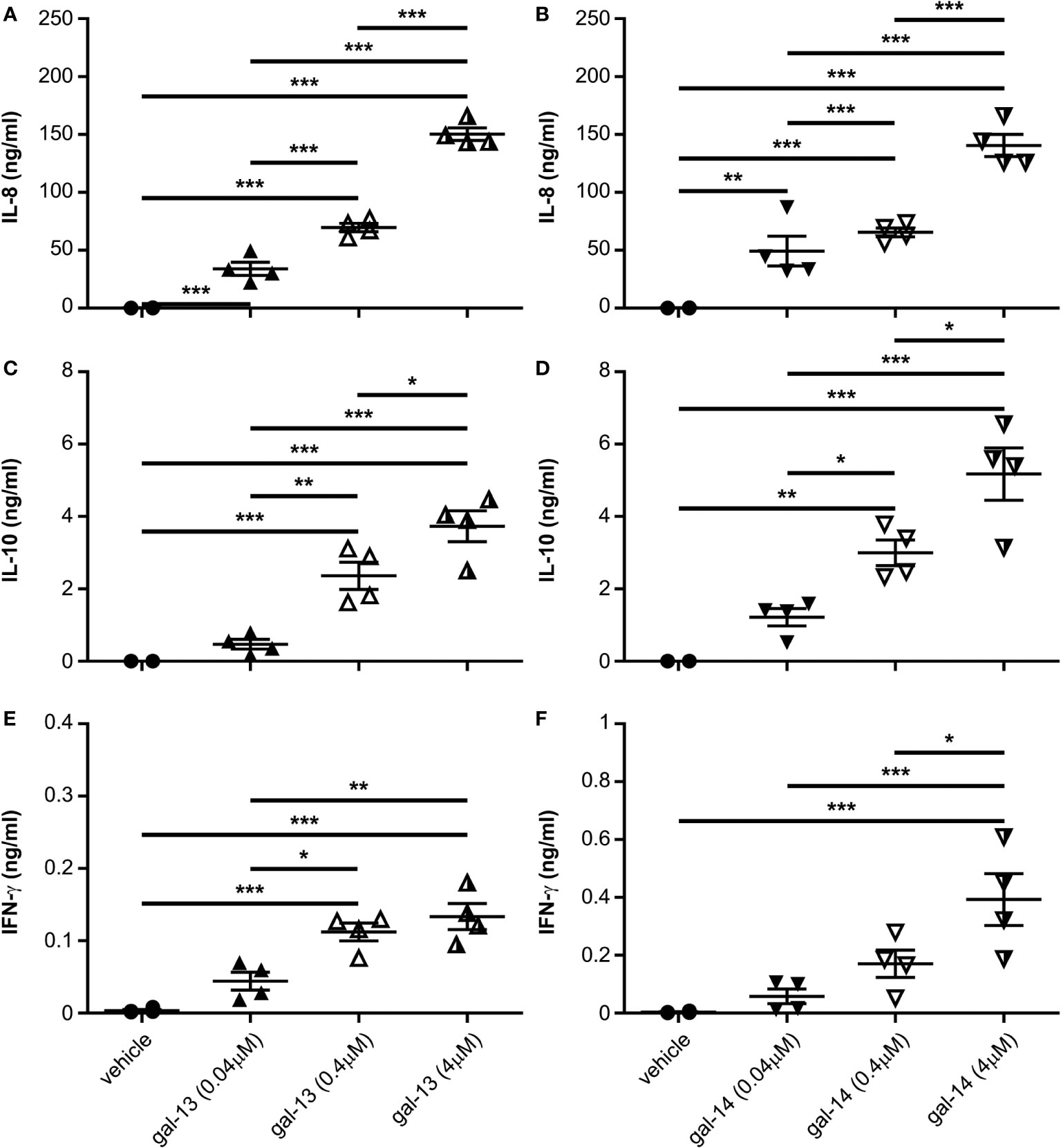
Figure 4 Gal-13 and gal-14 enhance the secretion of cytokines (IL-8, IL-10, IFN-γ) by immune cells. Cells were treated for 24 hours with different concentrations of gal-13 or gal-14. Cytokine production was measured by ELISA. Graphs show the mean ± SEM of IL-8 (A, B), IL-10 (C, D), and IFN-γ (E, F) cytokine concentrations (n=4). One-way ANOVA and Tukey post-hoc tests were used. Statistical significance was set at *p<0.05, **p<0.01, or ***p<0.001. IFN, interferon; IL, interleukin; SEM, standard error of the mean.
Gal-13 and gal-14 cause phosphorylation of Erk1/2 and p38 MAPKs and of NF-ĸB in immune cells
To identify signaling pathways upon gal-13 or gal-14 stimuli in PBMCs, the phosphorylation of two MAPKs (Erk1/2 and p38) and a transcription factor (NF-κB), all of which are crucial in immune cell functions, was examined. We found that 10–30 minutes of gal-13 or gal-14 treatment significantly phosphorylated Erk1/2 (gal-13: 4.40–13.85-fold; gal-14: 25.79–31.07-fold) (Supplementary Figures 4A, B, and Figures 5A, B) and p38 (gal-13: 3.05–3.77-fold; gal-14: 5.53–6.45-fold) (Supplementary Figures 4C, D, and Figures 5C, D). Furthermore, the phosphorylation of NF-κB was also increased after stimuli with recombinant galectins at all time points (gal-13: 2.47–2.69-fold; gal-14: 2.50–3.39-fold) (Supplementary Figures 4E, F, and Figures 5E, F).
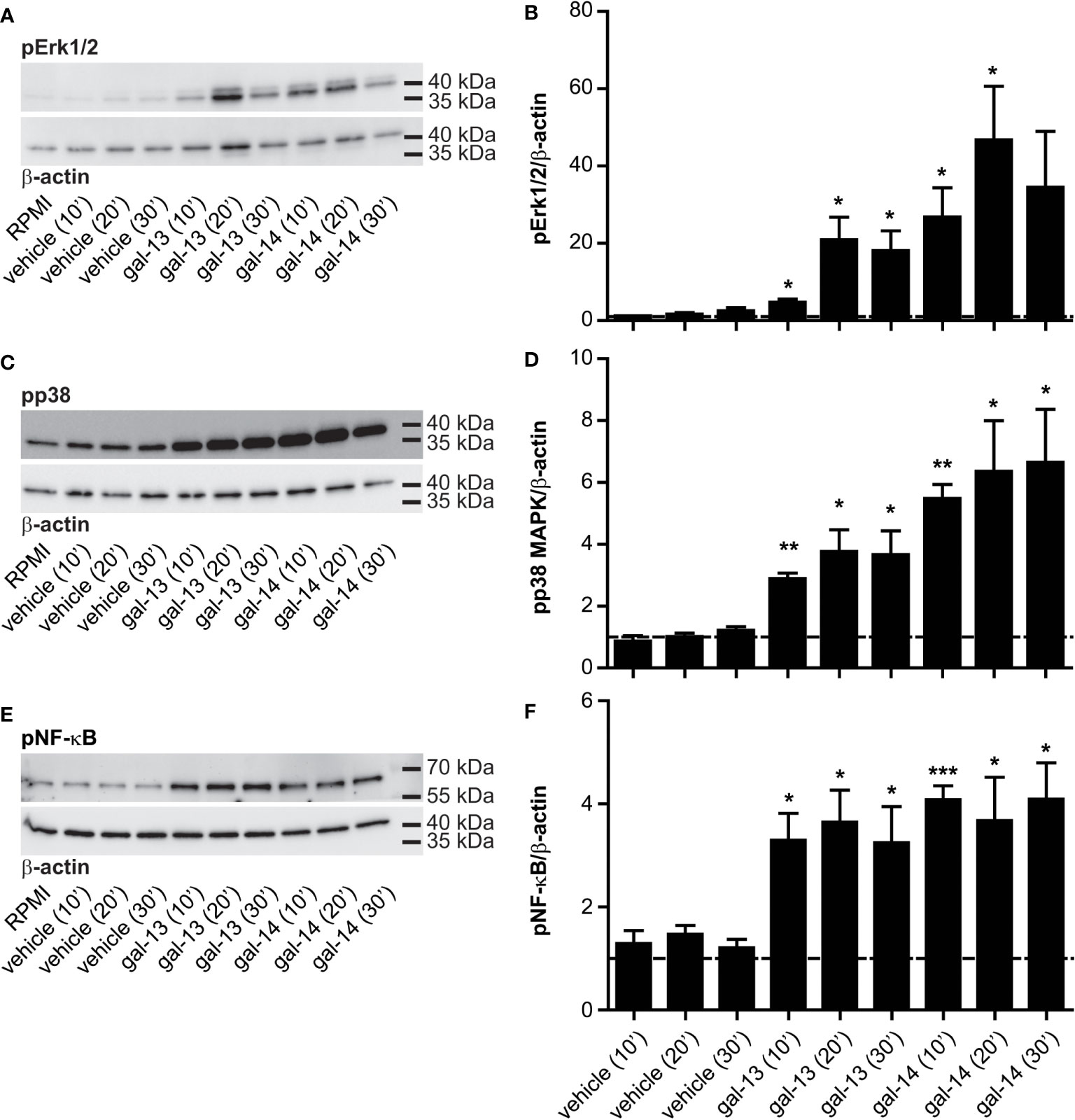
Figure 5 Galectin-13 and galectin-14 induce phosphorylation of Erk1/2, p38, and NF-κB in immune cells. The phosphorylation level of the mitogen-activated protein kinases Erk1/2 and p38, as well as transcription factor NF-κB was examined by Western blot. Freshly isolated PBMCs were incubated with the vehicle, gal-13, or gal-14 for 10, 20, and 30 minutes. Serum-free medium (RPMI-1640) was used as absolute control. Representative membrane strips for Erk1/2 (A), p38 (C), and NF-κB (E), as well as for β-actin as a loading control. Graphs show the mean value ± SEM of densitometry results for Erk1/2 (B), p38 (D), and NF-κB (F) (n=4). One-sample t-test was used. Statistical significance was set at *p<0.05, **p<0.01, or ***p<0.001. Erk1/2, extracellular signal-regulated kinase 1/2; NF-κB, nuclear factor kappa B; SEM, standard error of the mean.
Discussion
Principal findings of the study
The current study provides the following evidence: 1) Gal-13 and gal-14 bind to all investigated PBMC populations: the greatest extent to monocytes, followed by NK cells and B cells, and the least extent to T cells; 2) Gal-13 and gal-14 enhance the viability of PBMCs; 3) Gal-13 and gal-14 decrease the apoptosis of T cells, B cells, NK cells, and monocytes but have no effect on the proliferation of lymphocytic populations under the experimental conditions; 4) Gal-13 and gal-14 increase the production of cytokines in PBMCs, to the greatest extent in the pro-angiogenic and granulocyte chemoattractant IL-8, to a lesser extent in the main anti-inflammatory cytokine IL-10, and to the least extent in the pro-inflammatory and Th17 differentiation inhibiting IFN-γ production; and 5) Gal-13 and gal-14 induce Erk1/2 and p38 MAPKs and persistent NF-κB phosphorylation, which may contribute to the aforementioned effects by modulating the production of anti-apoptotic proteins and cytokines. These findings provide insights into the causal relationship between placenta-specific galectins and maternal-fetal immune regulation, which is further discussed below.
Gal-13 and gal-14 increase cell viability via decreasing the apoptosis of immune cells
Several galectins affect immune responses by regulating cell viability, apoptosis, and proliferation, resulting in a shift in innate and adaptive immune responses at the maternal-fetal interfaces (30, 49, 50, 52). For instance, gal-9 is highly expressed by fetal cells (95) and suppresses cytotoxic T-cell functions by the induction of their apoptosis (96). In this study, we found that placental galectins increase the viability of the examined PBMC populations. Since viability assay alone could not determine whether reduced cell death or increased proliferation resulted in increased viability, we examined how gal-13 and gal-14 affected the apoptosis and proliferation of PBMCs. Both galectins reduced the early apoptosis of T and B lymphocytes, NK cells, and monocytes, and the late apoptosis of B cells and monocytes, in line with the results of the viability test. This is in accord with our recent study showing that gal-13 reduces the apoptosis of purified neutrophil granulocytes, thus promoting the polarization toward a placenta-growth-permissive phenotype, which contributes to trophoblast invasion and normal placentation during pregnancy (87). Our previous study found that gal-13 or gal-14 treatment increased the apoptosis of T cells (57, 60, 78). It is likely that the varying experimental conditions contributed to these differences since earlier studies (57, 60, 78) examined the effect of these galectins on the apoptosis of activated T lymphocytes or lymphocytes already kept in culture for several days, whereas, herein non-activated cells were used for the experiments after a short incubation period. These differences might have affected cell surface glycosylation events, which are known to significantly influence the overall effect of galectins on cell survival (97–99). Additionally, the effect of the microenvironment and surrounding immune cell populations in the mixed cell culture may have also impacted the overall anti-apoptotic activity of gal-13 and gal-14.
An important difference might be that apoptosis-inducing or -inhibiting effects of galectins are highly influenced by the cell-surface glycosylation status of target cells (98). This is tightly regulated by their activation status, e.g., resting, activated, and various differentiation states, determined by the local microenvironment and the set of stimuli reaching the cell. For example, activated Th1 and Th17 cells express a repertoire of glycans required for gal-1 binding and are susceptible to gal-1-induced apoptosis. For example, it was demonstrated that activated T cells and Th1 cells express a repertoire of glycans required for gal-1 binding, which makes them susceptible to gal-1-induced apoptosis, whereas Th2 cells are less responsive to gal-1 binding and gal-1-induced apoptosis due to their 2,6-sialylation on the cell-surface glycoproteins (99). Activated T cells, Th1 cells, and Th2 cells, however, displayed comparable levels of gal-3-induced apoptosis, proving that Th2 cells’ resistance to gal-1-induced death is specific and does not signify a generally higher resistance to apoptosis (99). Another study by Kopcow et al. found that peripheral and decidual T cells have different glycophenotypes, thus a distinct gal-1-binding capacity compatible with higher sensitivity to gal-1 of decidual T cells (40). This finding is supported by the substantial proportion of apoptotic T cells in the decidua due to T-cell death mediated by local gal-1 (48). As neither gal-13 nor gal-14 had an effect on the proliferation of T and B lymphocytes as well as NK cells, we concluded that the anti-apoptotic activity of these placental galectins is responsible for the observed increase in PBMC viability.
Gal-13 and gal-14 induce the production of IL-8, IL-10, and IFN-γ cytokines in immune cells
Earlier, gal-13 was described to increase the production of IL-1ɑ and IL-6 in PBMCs (86) as well as gal-13 and gal-14 to enhance IL-8 production in T cells (78). Herein, we were interested in determining whether gal-13 and gal-14 may influence the production of other cytokines in PBMCs as well. Our experimental conditions differed from the study applied by Kliman et al. (86), also by using DTT and higher galectin concentrations, which might have led to the observation of galectin-induced production of IL-8, IL-10, and IFN-γ in PBMCs in our study. IL-8 can contribute to the development of blood supply at the maternal-fetal interface through its pro-angiogenic effect on endothelial cells (100, 101). IL-8 is also a chemoattractant for neutrophils, so by attracting these cells to the maternal-fetal interface and promoting their phenotypic change, gal-13 and gal-14 may contribute to placentation and the formation of a proper immune environment (87). The increased production of IL-10, which can be produced by several immune cells, may contribute to the shift toward a tolerogenic immune environment in pregnancy (94). Previous publications described that gal-9 increased the production of IL-4, IL-6, and IL-10 by PBMCs (102) and that gal-1 also increased the production of IL-10 but decreased the production of IFN-γ by T lymphocytes (103). Since IFN-γ is produced by NK cells, inhibits Th17 differentiation (104), and promotes decidual NK cell differentiation and placental development (93, 105), a modest IFN-γ production upon gal-13 and gal-14 treatment may also show profound effects at the maternal-fetal interface. Decidual T cells express a high level of IFN-γ besides IL-4 (106), which may also relate to local effects of progesterone (107). Therefore, in the future, it would be interesting to study whether placental galectins can contribute to the phenotypic changes of these immune cells in the decidua.
Gal-13 and gal-14 regulate immune cells by the activation of Erk1/2, p38, and NF-κB signaling pathways
Galectins were found to influence immune cell functions via key signaling pathways, e.g., the MAPK and NF-κB pathways (108–113). However, the interaction partners and receptors of gal-13 and gal-14 expressed on immune cells, and the effects of these galectins on signal transduction, have not yet been revealed. Herein, we showed that gal-13 and gal-14 treatment induced the phosphorylation of Erk1/2 and p38 MAPKs and the persistent phosphorylation of the p65 subunit of the NF-κB transcription factor. These signaling molecules are essential for the generation of immune responses, such as inflammation, pathogen recognition, T- and B-cell responses, and lymphocyte longevity (15, 114–117), and several galectins, e.g., gal-1, gal-8, gal-9, have been shown to induce or inhibit their activation (108, 110, 118, 119). For example, it was found that gal-8 induced apoptosis in Jurkat T cells by triggering an Erk1/2-dependent death pathway (108). In another study, human monocytes upregulated FcγRI and downregulated MHC-II surface expression in an Erk1/2-dependent manner upon gal-1 treatment (110). It was also demonstrated that gal-9 increased the phosphorylation of Akt, c-Jun N-terminal kinase, and Erk1/2—the key kinases involved in NK cell function—in peripheral TIM-3+ NK cells, which led to their suppressed activation (118). Furthermore, gal-14 was found to regulate cell function through the interaction with the transcription factor c-Rel, a subunit of NF-ĸB, which has many functions in pathways related to inflammation, apoptosis, cell growth, and cell differentiation, among others (119). However, in the current study, gal-14 was overexpressed in a HeLa (cervical cancer) cell line, in which gal-14 is normally not expressed, so the results may not be physiologically relevant (119). In light of these findings, it will be valuable to investigate additional signaling pathways that may be regulated by gal-13 and gal-14.
Of significance, while intracellular galectins can bind to proteins, extracellular galectins bind to glycan sidechains of glycoproteins at cell surfaces. Therefore, various glycoproteins with the same carbohydrate moieties can be considered as receptors for placental galectins, similar to other galectins. Indeed, we have shown previously that gal-13 differentially bound to red blood cells due to its varying affinity for blood-group AB0 glycans. For example, gal-13 binds blood-group AB erythrocytes with the strongest while blood-group B erythrocytes with the weakest affinity (120). The affinity of gal-13 and gal-14 to certain mono- or disaccharide ligands was also revealed (53, 59, 62). It is an interesting question whether placental galectins can bind to the glycan chains on the surface of extracellular vesicles secreted or deported from the placenta, which would add another layer of signaling events between the fetus and the mother. Earlier studies showed that gal-13 is indeed localized in/on extracellular vesicles, but the ways of this binding require further examination (62, 65). Future studies also need to investigate the cell surface glycoprotein receptors of these galectins on white blood cells and how the glycosylation pattern of these cells may determine the strength of galectin binding and galectin-induced signaling events.
Strengths and limitations of the study
Strengths: 1) A large set of functional assays was applied to examine the effects of gal-13 and gal-14 on immune cells obtained from non-pregnant female donors; 2) we used a mixed PBMC population instead of purified cell populations to better mimic the physiological conditions in human circulation. However, future investigations of isolated immune cell populations shall reveal more insights into the effects of gal-13 and gal-14 on specific immune cell types; and 3) recombinant galectins were expressed in a modified bacterial vector to avoid lipopolysaccharide contamination and biased immune cell activation. Limitations: 1) The applied quantities of gal-13 and gal-14 were supraphysiologic relative to that found in human circulation. However, these concentrations were similar to those previously used by us and others (10 to 100 µg/mL) to study the functional effects of a large set of galectins (121, 122). Furthermore, galectin blood concentrations do not represent their effective tissue or cell surface concentrations, where galectin-glycan lattices present a high local galectin concentration (123–126). Another technical rationale for using larger galectin concentrations was to prevent subunit dissociation in the DTT-containing solvent, which could lead to functional changes (127); 2) due to the use of mixed PBMC populations, we cannot exclude the effects of immune cell populations on each other; and 3) immune cells were isolated from non-pregnant female donors, which do not represent a perfect match for those cells that could be isolated from pregnant women. However, this method was the only option to avoid pre-exposure of immune cells to gal-13/gal-14 binding, which could have biased our studies. Nevertheless, future studies shall also characterize the effects of gal-13 and gal-14 on peripheral blood and decidual leukocytes isolated from pregnant women, since pregnancy hormones may strongly impact the glycosylation pattern and the galectin-binding capacity of these cells.
Conclusions
We demonstrate herein that gal-13 and gal-14 bind to non-activated PBMC populations, increase their viability by reducing their apoptosis, and induce the production of IL-8, IL-10, and IFN-γ, probably by the involvement of Erk1/2, p38, and NF-ĸB signaling pathways (Figure 6). Our findings suggest that these placental galectins regulate immune cells and their decreased expression in obstetrical syndromes may play a role in the severe immunopathology of these syndromes.
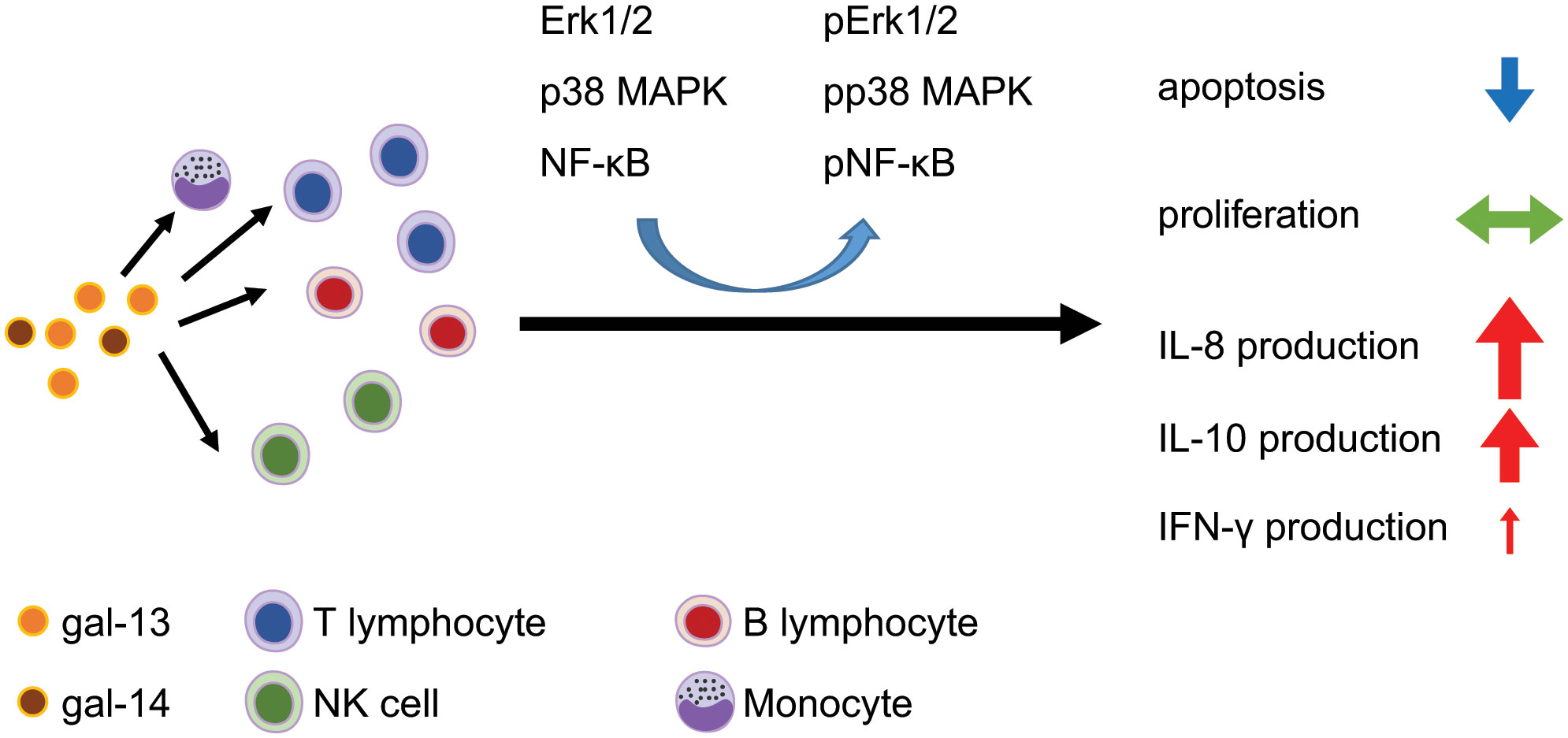
Figure 6 The effects of placental galectins on leukocyte functions. Gal-13 and gal-14 bind to the surface of immune cell populations where they activate Erk1/2, p38 MAPK, and NF-κB signaling pathways. These signaling effects of placenta-specific galectins increase the viability of leukocytes, decrease the apoptosis of immune cells without influencing the proliferation of cells, and induce the production of IL-8, IL-10, and IFN-γ in a different manner. Erk1/2, extracellular signal-regulated kinase 1/2; IFN, interferon; IL, interleukin; MAPK, mitogen-activated protein kinase; NF-κB, nuclear factor kappa B.
Data availability statement
The raw data supporting the conclusions of this article willbe made available by the authors, without undue reservation.
Ethics statement
Ethical review and approval were not required for the study on human participants in accordance with the local legislation and institutional requirements. Written informed consent for participation was not required for this study in accordance with the national legislation and the institutional requirements.
Author contributions
OO, JM, NGT, and AB conceptualized the study and designed research. OO, ET, JK, and AB performed research. RR, NGT, and AB contributed new reagents/analytic tools/clinical specimens. OO, RR, JK, MP, DG, SR, AT, OE, ZP, JM, NGT, and AB analyzed and interpreted data. All authors contributed to the article and approved the submitted version.
Funding
This research was funded, in part, by the Perinatology Research Branch, Division of Obstetrics and Maternal-Fetal Medicine, Division of Intramural Research, Eunice Kennedy Shriver National Institute of Child Health and Human Development, National Institutes of Health, USA Department of Health and Human Services (NICHD/NIH/DHHS); in part, with Federal funds from NICHD/NIH/DHHS under Contract No. HHSN275201300006C (RR); by the Hungarian Academy of Sciences Momentum Grant No. LP2014-7/2014 (NGT) and Premium Postdoc_2019-436 Grant (AB); and by the Ministry of Innovation and Technology of Hungary from the National Research, Development Innovation Fund financed under the OTKA K124862 (NGT) and FIEK_16-1-2016- 0005 (NGT) funding schemes.
Acknowledgments
We thank Judit Baunoch (Research Centre for Natural Sciences) for her technical assistance and Maureen McGerty (Wayne State University) for her critical reading of the manuscript.
Conflict of interest
The authors declare that the research was conducted in the absence of any commercial or financial relationships that could be construed as a potential conflict of interest.
Publisher’s note
All claims expressed in this article are solely those of the authors and do not necessarily represent those of their affiliated organizations, or those of the publisher, the editors and the reviewers. Any product that may be evaluated in this article, or claim that may be made by its manufacturer, is not guaranteed or endorsed by the publisher.
Supplementary material
The Supplementary Material for this article can be found online at: https://www.frontiersin.org/articles/10.3389/fimmu.2022.1088024/full#supplementary-material
References
1. Szekeres-Bartho J, Wegmann TG. A progesterone-dependent immunomodulatory protein alters the Th1/Th2 balance. J Reprod Immunol (1996) 31(1-2):81–95. doi: 10.1016/0165-0378(96)00964-3
2. Abrahams VM, Kim YM, Straszewski SL, Romero R, Mor G. Macrophages and apoptotic cell clearance during pregnancy. Am J Reprod Immunol (2004) 51(4):275–82. doi: 10.1111/j.1600-0897.2004.00156.x
3. Mor G, Romero R, Aldo PB, Abrahams VM. Is the trophoblast an immune regulator? the role of toll-like receptors during pregnancy. Crit Rev Immunol (2005) 25(5):375–88. doi: 10.1615/CritRevImmunol.v25.i5.30
4. Richani K, Soto E, Romero R, Espinoza J, Chaiworapongsa T, Nien JK, et al. Normal pregnancy is characterized by systemic activation of the complement system. J Matern Fetal Neonatal Med (2005) 17(4):239–45. doi: 10.1080/14767050500072722
5. Blois SM, Kammerer U, Alba Soto C, Tometten MC, Shaikly V, Barrientos G, et al. Dendritic cells: key to fetal tolerance? Biol Reprod (2007) 77(4):590–8. doi: 10.1095/biolreprod.107.060632
6. Kalkunte S, Chichester CO, Gotsch F, Sentman CL, Romero R, Sharma S. Evolution of non-cytotoxic uterine natural killer cells. Am J Reprod Immunol (2008) 59(5):425–32. doi: 10.1111/j.1600-0897.2008.00595.x
7. Mor G. Inflammation and pregnancy: the role of toll-like receptors in trophoblast-immune interaction. Ann N Y Acad Sci (2008) 1127:121–8. doi: 10.1196/annals.1434.006
8. Gomez-Lopez N, Vega-Sanchez R, Castillo-Castrejon M, Romero R, Cubeiro-Arreola K, Vadillo-Ortega F. Evidence for a role for the adaptive immune response in human term parturition. Am J Reprod Immunol (2013) 69(3):212–30. doi: 10.1111/aji.12074
9. El-Azzamy H, Balogh A, Romero R, Xu Y, LaJeunesse C, Plazyo O, et al. Characteristic changes in decidual gene expression signature in spontaneous term parturition. J Pathol Transl Med (2017) 51(3):264–83. doi: 10.4132/jptm.2016.12.20
10. Szekeres-Bartho J, Sucurovic S, Mulac-Jericevic B. The role of extracellular vesicles and PIBF in embryo-maternal immune-interactions. Front Immunol (2018) 9:2890. doi: 10.3389/fimmu.2018.02890
11. Vacca P, Vitale C, Munari E, Cassatella MA, Mingari MC, Moretta L. Human innate lymphoid cells: Their functional and cellular interactions in decidua. Front Immunol (2018) 9:1897. doi: 10.3389/fimmu.2018.01897
12. Ander SE, Diamond MS, Coyne CB. Immune responses at the maternal-fetal interface. Sci Immunol (2019) 4(31):eaat6114. doi: 10.1126/sciimmunol.aat6114
13. Mor G, Cardenas I, Abrahams V, Guller S. Inflammation and pregnancy: the role of the immune system at the implantation site. Ann N Y Acad Sci (2011) 1221:80–7. doi: 10.1111/j.1749-6632.2010.05938.x
14. Racicot K, Kwon JY, Aldo P, Silasi M, Mor G. Understanding the complexity of the immune system during pregnancy. Am J Reprod Immunol (2014) 72(2):107–16. doi: 10.1111/aji.12289
15. Aghaeepour N, Ganio EA, McIlwain D, Tsai AS, Tingle M, Van Gassen S, et al. An immune clock of human pregnancy. Sci Immunol (2017) 2(15):eaan2946. doi: 10.1126/sciimmunol.aan2946
16. Peterson LS, Stelzer IA, Tsai AS, Ghaemi MS, Han X, Ando K, et al. Multiomic immune clockworks of pregnancy. Semin Immunopathol (2020) 42(4):397–412. doi: 10.1007/s00281-019-00772-1
17. Piccinni MP, Robertson SA, Saito S. Editorial: Adaptive immunity in pregnancy. Front Immunol (2021) 12:770242. doi: 10.3389/fimmu.2021.770242
18. Dam TK, Brewer CF. Lectins as pattern recognition molecules: the effects of epitope density in innate immunity. Glycobiology (2010) 20(3):270–9. doi: 10.1093/glycob/cwp186
19. Miller D, Motomura K, Galaz J, Gershater M, Lee ED, Romero R, et al. Cellular immune responses in the pathophysiology of preeclampsia. J Leukoc Biol (2022) 111(1):237–60. doi: 10.1002/JLB.5RU1120-787RR
20. Than NG, Romero R, Balogh A, Karpati E, Mastrolia SA, Staretz-Chacham O, et al. Galectins: Double-edged swords in the cross-roads of pregnancy complications and female reproductive tract inflammation and neoplasia. J Pathol Transl Med (2015) 49(3):181–208. doi: 10.4132/jptm.2015.02.25
21. Vasta GR, Ahmed H, Nita-Lazar M, Banerjee A, Pasek M, Shridhar S, et al. Galectins as self/non-self recognition receptors in innate and adaptive immunity: an unresolved paradox. Front Immunol (2012) 3:199. doi: 10.3389/fimmu.2012.00199
22. Toldi G, Svec P, Vasarhelyi B, Meszaros G, Rigo J, Tulassay T, et al. Decreased number of FoxP3+ regulatory T cells in preeclampsia. Acta Obstet Gynecol Scand (2008) 87(11):1229–33. doi: 10.1080/00016340802389470
23. Hahn S, Hasler P, Vokalova L, van Breda SV, Lapaire O, Than NG, et al. The role of neutrophil activation in determining the outcome of pregnancy and modulation by hormones and/or cytokines. Clin Exp Immunol (2019) 198(1):24–36. doi: 10.1111/cei.13278
24. Murrieta-Coxca JM, Fuentes-Zacarias P, Ospina-Prieto S, Markert UR, Morales-Prieto DM. Synergies of extracellular vesicles and microchimerism in promoting immunotolerance during pregnancy. Front Immunol (2022) 13:837281. doi: 10.3389/fimmu.2022.837281
25. Depierreux DM, Kieckbusch J, Shreeve N, Hawkes DA, Marsh B, Blelloch R, et al. Beyond maternal tolerance: Education of uterine natural killer cells by maternal MHC drives fetal growth. Front Immunol (2022) 13:808227. doi: 10.3389/fimmu.2022.808227
26. Zhuang B, Shang J, Yao Y. HLA-G: An important mediator of maternal-fetal immune-tolerance. Front Immunol (2021) 12:744324. doi: 10.3389/fimmu.2021.744324
27. Ding J, Zhang Y, Cai X, Diao L, Yang C, Yang J. Crosstalk between trophoblast and macrophage at the maternal-fetal interface: Current status and future perspectives. Front Immunol (2021) 12:758281. doi: 10.3389/fimmu.2021.758281
28. Xu L, Li Y, Sang Y, Li DJ, Du M. Crosstalk between trophoblasts and decidual immune cells: The cornerstone of maternal-fetal immunotolerance. Front Immunol (2021) 12:642392. doi: 10.3389/fimmu.2021.642392
29. Sharma S, Banerjee S, Krueger PM, Blois SM. Immunobiology of gestational diabetes mellitus in post-medawar era. Front Immunol (2021) 12:758267. doi: 10.3389/fimmu.2021.758267
30. Molvarec A, Blois SM, Stenczer B, Toldi G, Tirado-Gonzalez I, Ito M, et al. Peripheral blood galectin-1-expressing T and natural killer cells in normal pregnancy and preeclampsia. Clin Immunol (2011) 139(1):48–56. doi: 10.1016/j.clim.2010.12.018
31. Meggyes M, Miko E, Polgar B, Bogar B, Farkas B, Illes Z, et al. Peripheral blood TIM-3 positive NK and CD8+ T cells throughout pregnancy: TIM-3/galectin-9 interaction and its possible role during pregnancy. PloS One (2014) 9(3):e92371. doi: 10.1371/journal.pone.0092371
32. Miko E, Meggyes M, Bogar B, Schmitz N, Barakonyi A, Varnagy A, et al. Involvement of galectin-9/TIM-3 pathway in the systemic inflammatory response in early-onset preeclampsia. PloS One (2013) 8(8):e71811. doi: 10.1371/journal.pone.0071811
33. Blois SM, Barrientos G. Galectin signature in normal pregnancy and preeclampsia. J Reprod Immunol (2014) 101-102:127–34. doi: 10.1016/j.jri.2013.05.005
34. Barrientos G, Freitag N, Tirado-Gonzalez I, Unverdorben L, Jeschke U, Thijssen VL, et al. Involvement of galectin-1 in reproduction: past, present and future. Hum Reprod Update (2014) 20(2):175–93. doi: 10.1093/humupd/dmt040
35. Blois SM, Verlohren S, Wu G, Clark G, Dell A, Haslam SM, et al. Role of galectin-glycan circuits in reproduction: from healthy pregnancy to preterm birth (PTB). Semin Immunopathol (2020) 42(4):469–86. doi: 10.1007/s00281-020-00801-4
36. Blois SM, Dveksler G, Vasta GR, Freitag N, Blanchard V, Barrientos G. Pregnancy galectinology: Insights into a complex network of glycan binding proteins. Front Immunol (2019) 10:1166. doi: 10.3389/fimmu.2019.01166
37. Tirado-Gonzalez I, Freitag N, Barrientos G, Shaikly V, Nagaeva O, Strand M, et al. Galectin-1 influences trophoblast immune evasion and emerges as a predictive factor for the outcome of pregnancy. Mol Hum Reprod (2013) 19(1):43–53. doi: 10.1093/molehr/gas043
38. Blois SM, Ilarregui JM, Tometten M, Garcia M, Orsal AS, Cordo-Russo R, et al. A pivotal role for galectin-1 in fetomaternal tolerance. Nat Med (2007) 13(12):1450–7. doi: 10.1038/nm1680
39. Meister S, Hahn L, Beyer S, Mannewitz M, Perleberg C, Schnell K, et al. Regulatory T cell apoptosis during preeclampsia may be prevented by gal-2. Int J Mol Sci (2022) 23(3):1880. doi: 10.3390/ijms23031880
40. Blidner AG, Rabinovich GA. 'Sweetening' pregnancy: galectins at the fetomaternal interface. Am J Reprod Immunol (2013) 69(4):369–82. doi: 10.1111/aji.12090
41. Ramhorst RE, Giribaldi L, Fraccaroli L, Toscano MA, Stupirski JC, Romero MD, et al. Galectin-1 confers immune privilege to human trophoblast: implications in recurrent fetal loss. Glycobiology (2012) 22(10):1374–86. doi: 10.1093/glycob/cws104
42. Menkhorst E, Than NG, Jeschke U, Barrientos G, Szereday L, Dveksler G, et al. Medawar's PostEra: Galectins emerged as key players during fetal-maternal glycoimmune adaptation. Front Immunol (2021) 12:784473. doi: 10.3389/fimmu.2021.784473
43. Freitag N, Tirado-Gonzalez I, Barrientos G, Herse F, Thijssen VL, Weedon-Fekjaer SM, et al. Interfering with gal-1-mediated angiogenesis contributes to the pathogenesis of preeclampsia. Proc Natl Acad Sci U S A (2013) 110(28):11451–6. doi: 10.1073/pnas.1303707110
44. Cooper DN. Galectinomics: finding themes in complexity. Biochim Biophys Acta (2002) 1572(2-3):209–31. doi: 10.1016/S0304-4165(02)00310-0
45. Gitt MA, Barondes SH. Evidence that a human soluble beta-galactoside-binding lectin is encoded by a family of genes. Proc Natl Acad Sci U S A (1986) 83(20):7603–7. doi: 10.1073/pnas.83.20.7603
46. Than NG, Romero R, Kim CJ, McGowen MR, Papp Z, Wildman DE. Galectins: guardians of eutherian pregnancy at the maternal-fetal interface. Trends Endocrinol Metab (2012) 23(1):23–31. doi: 10.1016/j.tem.2011.09.003
47. Miko E, Meggyes M, Doba K, Barakonyi A, Szereday L. Immune checkpoint molecules in reproductive immunology. Front Immunol (2019) 10:846. doi: 10.3389/fimmu.2019.00846
48. Kopcow HD, Rosetti F, Leung Y, Allan DS, Kutok JL, Strominger JL. T Cell apoptosis at the maternal-fetal interface in early human pregnancy, involvement of galectin-1. Proc Natl Acad Sci U S A (2008) 105(47):18472–7. doi: 10.1073/pnas.0809233105
49. Than NG, Romero R, Erez O, Weckle A, Tarca AL, Hotra J, et al. Emergence of hormonal and redox regulation of galectin-1 in placental mammals: implication in maternal-fetal immune tolerance. Proc Natl Acad Sci U S A (2008) 105(41):15819–24. doi: 10.1073/pnas.0807606105
50. Terness P, Kallikourdis M, Betz AG, Rabinovich GA, Saito S, Clark DA. Tolerance signaling molecules and pregnancy: IDO, galectins, and the renaissance of regulatory T cells. Am J Reprod Immunol (2007) 58(3):238–54. doi: 10.1111/j.1600-0897.2007.00510.x
51. Sun J, Yang M, Ban Y, Gao W, Song B, Wang Y, et al. Tim-3 is upregulated in NK cells during early pregnancy and inhibits NK cytotoxicity toward trophoblast in galectin-9 dependent pathway. PloS One (2016) 11(1):e0147186. doi: 10.1371/journal.pone.0147186
52. Hu XH, Tang MX, Mor G, Liao AH. Tim-3: Expression on immune cells and roles at the maternal-fetal interface. J Reprod Immunol (2016) 118:92–9. doi: 10.1016/j.jri.2016.10.113
53. Than NG, Pick E, Bellyei S, Szigeti A, Burger O, Berente Z, et al. Functional analyses of placental protein 13/galectin-13. Eur J Biochem (2004) 271(6):1065–78. doi: 10.1111/j.1432-1033.2004.04004.x
54. Vicovac L, Jankovic M, Cuperlovic M. Galectin-1 and -3 in cells of the first trimester placental bed. Hum Reprod (1998) 13(3):730–5. doi: 10.1093/humrep/13.3.730
55. Jeschke U, Mayr D, Schiessl B, Mylonas I, Schulze S, Kuhn C, et al. Expression of galectin-1, -3 (gal-1, gal-3) and the thomsen-friedenreich (TF) antigen in normal, IUGR, preeclamptic and HELLP placentas. Placenta (2007) 28(11-12):1165–73. doi: 10.1016/j.placenta.2007.06.006
56. Van den Brule FA, Fernandez PL, Buicu C, Liu FT, Jackers P, Lambotte R, et al. Differential expression of galectin-1 and galectin-3 during first trimester human embryogenesis. Dev Dyn (1997) 209(4):399–405. doi: 10.1002/(SICI)1097-0177(199708)209:4<399::AID-AJA7>3.0.CO;2-D
57. Than NG, Balogh A, Romero R, Karpati E, Erez O, Szilagyi A, et al. Placental protein 13 (PP13) - a placental immunoregulatory galectin protecting pregnancy. Front Immunol (2014) 5:348. doi: 10.3389/fimmu.2014.00348
58. Maquoi E, van den Brule FA, Castronovo V, Foidart JM. Changes in the distribution pattern of galectin-1 and galectin-3 in human placenta correlates with the differentiation pathways of trophoblasts. Placenta (1997) 18(5-6):433–9. doi: 10.1016/S0143-4004(97)80044-6
59. Than NG, Romero R, Goodman M, Weckle A, Xing J, Dong Z, et al. A primate subfamily of galectins expressed at the maternal-fetal interface that promote immune cell death. Proc Natl Acad Sci U S A (2009) 106(24):9731–6. doi: 10.1073/pnas.0903568106
60. Than NG, Romero R, Xu Y, Erez O, Xu Z, Bhatti G, et al. Evolutionary origins of the placental expression of chromosome 19 cluster galectins and their complex dysregulation in preeclampsia. Placenta (2014) 35(11):855–65. doi: 10.1016/j.placenta.2014.07.015
61. Than NG, Sumegi B, Than GN, Berente Z, Bohn H. Isolation and sequence analysis of a cDNA encoding human placental tissue protein 13 (PP13), a new lysophospholipase, homologue of human eosinophil charcot-Leyden crystal protein. Placenta (1999) 20(8):703–10. doi: 10.1053/plac.1999.0436
62. Than NG, Abdul Rahman O, Magenheim R, Nagy B, Fule T, Hargitai B, et al. Placental protein 13 (galectin-13) has decreased placental expression but increased shedding and maternal serum concentrations in patients presenting with preterm pre-eclampsia and HELLP syndrome. Virchows Arch (2008) 453(4):387–400. doi: 10.1007/s00428-008-0658-x
63. Huppertz B, Sammar M, Chefetz I, Neumaier-Wagner P, Bartz C, Meiri H. Longitudinal determination of serum placental protein 13 during development of preeclampsia. Fetal Diagn Ther (2008) 24(3):230–6. doi: 10.1159/000151344
64. Balogh A, Pozsgay J, Matko J, Dong Z, Kim CJ, Varkonyi T, et al. Placental protein 13 (PP13/galectin-13) undergoes lipid raft-associated subcellular redistribution in the syncytiotrophoblast in preterm preeclampsia and HELLP syndrome. Am J Obstet Gynecol (2011) 205(2):156 e1–14. doi: 10.1016/j.ajog.2011.03.023
65. Sammar M, Dragovic R, Meiri H, Vatish M, Sharabi-Nov A, Sargent I, et al. Reduced placental protein 13 (PP13) in placental derived syncytiotrophoblast extracellular vesicles in preeclampsia - a novel tool to study the impaired cargo transmission of the placenta to the maternal organs. Placenta (2018) 66:17–25. doi: 10.1016/j.placenta.2018.04.013
66. Madar-Shapiro L, Karady I, Trahtenherts A, Syngelaki A, Akolekar R, Poon L, et al. Predicting the risk to develop preeclampsia in the first trimester combining promoter variant -98A/C of LGALS13 (Placental protein 13), black ethnicity, previous preeclampsia, obesity, and maternal age. Fetal Diagn Ther (2018) 43(4):250–65. doi: 10.1159/000477933
67. Romero R, Kusanovic JP, Than NG, Erez O, Gotsch F, Espinoza J, et al. First-trimester maternal serum PP13 in the risk assessment for preeclampsia. Am J Obstet Gynecol (2008) 199(2):122 e1– e11. doi: 10.1016/j.ajog.2008.01.013
68. Chafetz I, Kuhnreich I, Sammar M, Tal Y, Gibor Y, Meiri H, et al. First-trimester placental protein 13 screening for preeclampsia and intrauterine growth restriction. Am J Obstet Gynecol (2007) 197(1):35 e1–7. doi: 10.1016/j.ajog.2007.02.025
69. Meiri H, Sammar M, Herzog A, Grimpel YI, Fihaman G, Cohen A, et al. Prediction of preeclampsia by placental protein 13 and background risk factors and its prevention by aspirin. J Perinat Med (2014) 42(5):591–601. doi: 10.1515/jpm-2013-0298
70. Wortelboer EJ, Koster MP, Cuckle HS, Stoutenbeek PH, Schielen PC, Visser GH. First-trimester placental protein 13 and placental growth factor: markers for identification of women destined to develop early-onset pre-eclampsia. BJOG (2010) 117(11):1384–9. doi: 10.1111/j.1471-0528.2010.02690.x
71. Saito S, Shiozaki A, Nakashima A, Sakai M, Sasaki Y. The role of the immune system in preeclampsia. Mol Aspects Med (2007) 28(2):192–209. doi: 10.1016/j.mam.2007.02.006
72. Perez-Sepulveda A, Torres MJ, Khoury M, Illanes SE. Innate immune system and preeclampsia. Front Immunol (2014) 5:244. doi: 10.3389/fimmu.2014.00244
73. Geldenhuys J, Rossouw TM, Lombaard HA, Ehlers MM, Kock MM. Disruption in the regulation of immune responses in the placental subtype of preeclampsia. Front Immunol (2018) 9:1659. doi: 10.3389/fimmu.2018.01659
74. Laresgoiti-Servitje E. A leading role for the immune system in the pathophysiology of preeclampsia. J Leukoc Biol (2013) 94(2):247–57. doi: 10.1189/jlb.1112603
75. Than NG, Posta M, Gyorffy D, Orosz L, Orosz G, Rossi SW, et al. Early pathways, biomarkers, and four distinct molecular subclasses of preeclampsia: The intersection of clinical, pathological, and high-dimensional biology studies. Placenta (2022) 125:10–9. doi: 10.1016/j.placenta.2022.03.009
76. Eikmans M, Morales-Prieto DM, van der Hoorn ML, Markert UR. Editorial: Immunological challenges around pregnancy complications associated with failures of maternal tolerance to the fetus. Front Immunol (2022) 13:983739. doi: 10.3389/fimmu.2022.983739
77. Than NG, Romero R, Tarca AL, Kekesi KA, Xu Y, Xu Z, et al. Integrated systems biology approach identifies novel maternal and placental pathways of preeclampsia. Front Immunol (2018) 9:1661. doi: 10.3389/fimmu.2018.01661
78. Balogh A, Toth E, Romero R, Parej K, Csala D, Szenasi NL, et al. Placental galectins are key players in regulating the maternal adaptive immune response. Front Immunol (2019) 10:1240. doi: 10.3389/fimmu.2019.01240
79. Wu C, Thalhamer T, Franca RF, Xiao S, Wang C, Hotta C, et al. Galectin-9-CD44 interaction enhances stability and function of adaptive regulatory T cells. Immunity (2014) 41(2):270–82. doi: 10.1016/j.immuni.2014.06.011
80. Sano H, Hsu DK, Apgar JR, Yu L, Sharma BB, Kuwabara I, et al. Critical role of galectin-3 in phagocytosis by macrophages. J Clin Invest (2003) 112(3):389–97. doi: 10.1172/JCI200317592
81. Sanchez-Fueyo A, Tian J, Picarella D, Domenig C, Zheng XX, Sabatos CA, et al. Tim-3 inhibits T helper type 1-mediated auto- and alloimmune responses and promotes immunological tolerance. Nat Immunol (2003) 4(11):1093–101. doi: 10.1038/ni987
82. Chen HY, Liu FT, Yang RY. Roles of galectin-3 in immune responses. Arch Immunol Ther Exp (Warsz) (2005) 53(6):497–504.
83. Garin MI, Chu CC, Golshayan D, Cernuda-Morollon E, Wait R, Lechler RI. Galectin-1: a key effector of regulation mediated by CD4+CD25+ T cells. Blood (2007) 109(5):2058–65. doi: 10.1182/blood-2006-04-016451
84. Oomizu S, Arikawa T, Niki T, Kadowaki T, Ueno M, Nishi N, et al. Cell surface galectin-9 expressing Th cells regulate Th17 and Foxp3+ treg development by galectin-9 secretion. PloS One (2012) 7(11):e48574. doi: 10.1371/journal.pone.0048574
85. Oomizu S, Arikawa T, Niki T, Kadowaki T, Ueno M, Nishi N, et al. Galectin-9 suppresses Th17 cell development in an IL-2-dependent but Tim-3-independent manner. Clin Immunol (2012) 143(1):51–8. doi: 10.1016/j.clim.2012.01.004
86. Kliman HJ, Sammar M, Grimpel YI, Lynch SK, Milano KM, Pick E, et al. Placental protein 13 and decidual zones of necrosis: an immunologic diversion that may be linked to preeclampsia. Reprod Sci (2012) 19(1):16–30. doi: 10.1177/1933719111424445
87. Vokalova L, Balogh A, Toth E, Van Breda SV, Schafer G, Hoesli I, et al. Placental protein 13 (Galectin-13) polarizes neutrophils toward an immune regulatory phenotype. Front Immunol (2020) 11:145. doi: 10.3389/fimmu.2020.00145
88. Than NG, Erez O, Wildman DE, Tarca AL, Edwin SS, Abbas A, et al. Severe preeclampsia is characterized by increased placental expression of galectin-1. J Matern Fetal Neonatal Med (2008) 21(7):429–42. doi: 10.1080/14767050802041961
89. Hirashima C, Ohkuchi A, Nagayama S, Suzuki H, Takahashi K, Ogoyama M, et al. Galectin-1 as a novel risk factor for both gestational hypertension and preeclampsia, specifially its expression at a low level in the second trimester and a high level after onset. Hypertens Res (2018) 41(1):45–52. doi: 10.1038/hr.2017.85
90. Pankiewicz K, Szczerba E, Fijalkowska A, Szamotulska K, Szewczyk G, Issat T, et al. The association between serum galectin-3 level and its placental production in patients with preeclampsia. J Physiol Pharmacol (2020) 71(6):845–56. doi: 10.26402/jpp.2020.6.08
91. Freitag N, Tirado-Gonzalez I, Barrientos G, Powell KL, Boehm-Sturm P, Koch SP, et al. Galectin-3 deficiency in pregnancy increases the risk of fetal growth restriction (FGR) via placental insufficiency. Cell Death Dis (2020) 11(7):560. doi: 10.1038/s41419-020-02791-5
92. Yang F, Zheng Q, Jin L. Dynamic function and composition changes of immune cells during normal and pathological pregnancy at the maternal-fetal interface. Front Immunol (2019) 10:2317. doi: 10.3389/fimmu.2019.02317
93. Murphy SP, Tayade C, Ashkar AA, Hatta K, Zhang J, Croy BA. Interferon gamma in successful pregnancies. Biol Reprod (2009) 80(5):848–59. doi: 10.1095/biolreprod.108.073353
94. Cheng SB, Sharma S. Interleukin-10: a pleiotropic regulator in pregnancy. Am J Reprod Immunol (2015) 73(6):487–500. doi: 10.1111/aji.12329
95. Selno ATH, Schlichtner S, Yasinska IM, Sakhnevych SS, Fiedler W, Wellbrock J, et al. Transforming growth factor beta type 1 (TGF-beta) and hypoxia-inducible factor 1 (HIF-1) transcription complex as master regulators of the immunosuppressive protein galectin-9 expression in human cancer and embryonic cells. Aging (Albany NY) (2020) 12(23):23478–96. doi: 10.18632/aging.202343
96. Schlichtner S, Meyer NH, Yasinska IM, Aliu N, Berger SM, Gibbs BF, et al. Functional role of galectin-9 in directing human innate immune reactions to gram-negative bacteria and T cell apoptosis. Int Immunopharmacol (2021) 100:108155. doi: 10.1016/j.intimp.2021.108155
97. Pereira MS, Alves I, Vicente M, Campar A, Silva MC, Padrao NA, et al. Glycans as key checkpoints of T cell activity and function. Front Immunol (2018) 9:2754. doi: 10.3389/fimmu.2018.02754
98. Daniels MA, Hogquist KA, Jameson SC. Sweet 'n' sour: the impact of differential glycosylation on T cell responses. Nat Immunol (2002) 3(10):903–10. doi: 10.1038/ni1002-903
99. Toscano MA, Bianco GA, Ilarregui JM, Croci DO, Correale J, Hernandez JD, et al. Differential glycosylation of TH1, TH2 and TH-17 effector cells selectively regulates susceptibility to cell death. Nat Immunol (2007) 8(8):825–34. doi: 10.1038/ni1482
100. Li A, Dubey S, Varney ML, Dave BJ, Singh RK. IL-8 directly enhanced endothelial cell survival, proliferation, and matrix metalloproteinases production and regulated angiogenesis. J Immunol (2003) 170(6):3369–76. doi: 10.4049/jimmunol.170.6.3369
101. Lash GE, Ernerudh J. Decidual cytokines and pregnancy complications: focus on spontaneous miscarriage. J Reprod Immunol (2015) 108:83–9. doi: 10.1016/j.jri.2015.02.003
102. Enninga EA, Nevala WK, Holtan SG, Leontovich AA, Markovic SN. Galectin-9 modulates immunity by promoting Th2/M2 differentiation and impacts survival in patients with metastatic melanoma. Melanoma Res (2016) 26(5):429–41. doi: 10.1097/CMR.0000000000000281
103. van der Leij J, van den Berg A, Blokzijl T, Harms G, van Goor H, Zwiers P, et al. Dimeric galectin-1 induces IL-10 production in T-lymphocytes: an important tool in the regulation of the immune response. J Pathol (2004) 204(5):511–8. doi: 10.1002/path.1671
104. Chong WP, van Panhuys N, Chen J, Silver PB, Jittayasothorn Y, Mattapallil MJ, et al. NK-DC crosstalk controls the autopathogenic Th17 response through an innate IFN-gamma-IL-27 axis. J Exp Med (2015) 212(10):1739–52. doi: 10.1084/jem.20141678
105. Zhou JZ, Way SS, Chen K. Immunology of the uterine and vaginal mucosae. Trends Immunol (2018) 39(4):302–14. doi: 10.1016/j.it.2018.01.007
106. Powell RM, Lissauer D, Tamblyn J, Beggs A, Cox P, Moss P, et al. Decidual T cells exhibit a highly differentiated phenotype and demonstrate potential fetal specificity and a strong transcriptional response to IFN. J Immunol (2017) 199(10):3406–17. doi: 10.4049/jimmunol.1700114
107. Lissauer D, Eldershaw SA, Inman CF, Coomarasamy A, Moss PA, Kilby MD. Progesterone promotes maternal-fetal tolerance by reducing human maternal T-cell polyfunctionality and inducing a specific cytokine profile. Eur J Immunol (2015) 45(10):2858–72. doi: 10.1002/eji.201445404
108. Norambuena A, Metz C, Vicuna L, Silva A, Pardo E, Oyanadel C, et al. Galectin-8 induces apoptosis in jurkat T cells by phosphatidic acid-mediated ERK1/2 activation supported by protein kinase a down-regulation. J Biol Chem (2009) 284(19):12670–9. doi: 10.1074/jbc.M808949200
109. Hsu DK, Chen HY, Liu FT. Galectin-3 regulates T-cell functions. Immunol Rev (2009) 230(1):114–27. doi: 10.1111/j.1600-065X.2009.00798.x
110. Barrionuevo P, Beigier-Bompadre M, Ilarregui JM, Toscano MA, Bianco GA, Isturiz MA, et al. A novel function for galectin-1 at the crossroad of innate and adaptive immunity: galectin-1 regulates monocyte/macrophage physiology through a nonapoptotic ERK-dependent pathway. J Immunol (2007) 178(1):436–45. doi: 10.4049/jimmunol.178.1.436
111. Dai SY, Nakagawa R, Itoh A, Murakami H, Kashio Y, Abe H, et al. Galectin-9 induces maturation of human monocyte-derived dendritic cells. J Immunol (2005) 175(5):2974–81. doi: 10.4049/jimmunol.175.5.2974
112. Hong SH, Shin JS, Chung H, Park CG. Galectin-4 interaction with CD14 triggers the differentiation of monocytes into macrophage-like cells via the MAPK signaling pathway. Immune Netw (2019) 19(3):e17. doi: 10.4110/in.2019.19.e17
113. Walzel H, Blach M, Hirabayashi J, Arata Y, Kasai K, Brock J. Galectin-induced activation of the transcription factors NFAT and AP-1 in human jurkat T-lymphocytes. Cell Signal (2002) 14(10):861–8. doi: 10.1016/S0898-6568(02)00035-9
114. Hayden MS, Ghosh S. NF-kappaB in immunobiology. Cell Res (2011) 21(2):223–44. doi: 10.1038/cr.2011.13
115. Gomez-Chavez F, Correa D, Navarrete-Meneses P, Cancino-Diaz JC, Cancino-Diaz ME, Rodriguez-Martinez S. NF-kappaB and its regulators during pregnancy. Front Immunol (2021) 12:679106. doi: 10.3389/fimmu.2021.679106
116. Ariyakumar G, Morris JM, McKelvey KJ, Ashton AW, McCracken SA. NF-kappaB regulation in maternal immunity during normal and IUGR pregnancies. Sci Rep (2021) 11(1):20971. doi: 10.1038/s41598-021-00430-3
117. Fragiadakis GK, Baca QJ, Gherardini PF, Ganio EA, Gaudilliere DK, Tingle M, et al. Mapping the fetomaternal peripheral immune system at term pregnancy. J Immunol (2016) 197(11):4482–92. doi: 10.4049/jimmunol.1601195
118. Li Y, Zhang J, Zhang D, Hong X, Tao Y, Wang S, et al. Tim-3 signaling in peripheral NK cells promotes maternal-fetal immune tolerance and alleviates pregnancy loss. Sci Signal (2017) 10(498):eaah4323. doi: 10.1126/scisignal.aah4323
119. Si Y, Li Y, Yang T, Li X, Ayala GJ, Mayo KH, et al. Structure-function studies of galectin-14, an important effector molecule in embryology. FEBS J (2021) 288(3):1041–55. doi: 10.1111/febs.15441
120. Than NG, Romero R, Meiri H, Erez O, Xu Y, Tarquini F, et al. PP13, maternal ABO blood groups and the risk assessment of pregnancy complications. PloS One (2011) 6(7):e21564. doi: 10.1371/journal.pone.0021564
121. Yildirim C, Vogel DY, Hollander MR, Baggen JM, Fontijn RD, Nieuwenhuis S, et al. Galectin-2 induces a proinflammatory, anti-arteriogenic phenotype in monocytes and macrophages. PloS One (2015) 10(4):e0124347. doi: 10.1371/journal.pone.0124347
122. Stowell SR, Karmakar S, Stowell CJ, Dias-Baruffi M, McEver RP, Cummings RD. Human galectin-1, -2, and -4 induce surface exposure of phosphatidylserine in activated human neutrophils but not in activated T cells. Blood (2007) 109(1):219–27. doi: 10.1182/blood-2006-03-007153
123. Crocker PR. Mammalian carbohydrate recognition systems. Results and Problems in Cell Differentiation [Internet] (2001). doi: 10.1007/978-3-540-46410-5
124. Nabi IR, Shankar J, Dennis JW. The galectin lattice at a glance. J Cell Sci (2015) 128(13):2213–9. doi: 10.1242/jcs.151159
125. Bonzi J, Bornet O, Betzi S, Kasper BT, Mahal LK, Mancini SJ, et al. Pre-b cell receptor binding to galectin-1 modifies galectin-1/carbohydrate affinity to modulate specific galectin-1/glycan lattice interactions. Nat Commun (2015) 6:6194. doi: 10.1038/ncomms7194
126. Rabinovich GA, Toscano MA. Turning 'sweet' on immunity: galectin-glycan interactions in immune tolerance and inflammation. Nat Rev Immunol (2009) 9(5):338–52. doi: 10.1038/nri2536
Keywords: danger signal, evolution, glycomics, leukocyte, obstetrical syndrome, PP13, primate, trophoblast
Citation: Oravecz O, Romero R, Tóth E, Kapitány J, Posta M, Gallo DM, Rossi SW, Tarca AL, Erez O, Papp Z, Matkó J, Than NG and Balogh A (2022) Placental galectins regulate innate and adaptive immune responses in pregnancy. Front. Immunol. 13:1088024. doi: 10.3389/fimmu.2022.1088024
Received: 02 November 2022; Accepted: 05 December 2022;
Published: 28 December 2022.
Edited by:
Kirsty Pringle, The University of Newcastle, AustraliaReviewed by:
Laszlo Szereday, University of Pécs, HungaryVadim V. Sumbayev, University of Kent, United Kingdom
Hamutal Meiri, ASPRE-T consortium and TeleMarpe LTD, Israel
Copyright © 2022 Oravecz, Romero, Tóth, Kapitány, Posta, Gallo, Rossi, Tarca, Erez, Papp, Matkó, Than and Balogh. This is an open-access article distributed under the terms of the Creative Commons Attribution License (CC BY). The use, distribution or reproduction in other forums is permitted, provided the original author(s) and the copyright owner(s) are credited and that the original publication in this journal is cited, in accordance with accepted academic practice. No use, distribution or reproduction is permitted which does not comply with these terms.
*Correspondence: Nándor Gábor Than, dGhhbi5nYWJvckB0dGsuaHU=