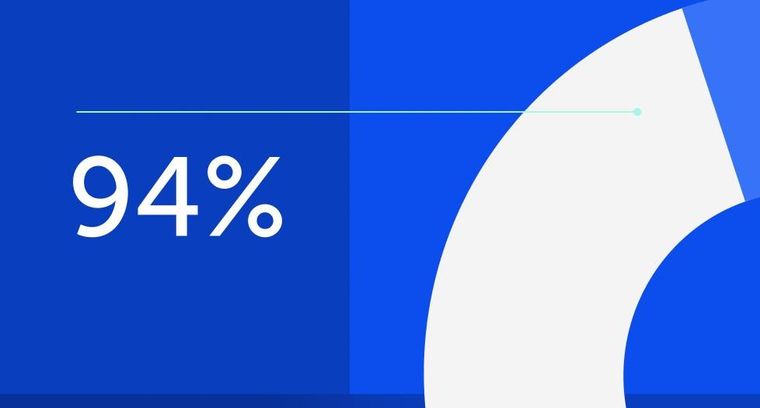
94% of researchers rate our articles as excellent or good
Learn more about the work of our research integrity team to safeguard the quality of each article we publish.
Find out more
MINI REVIEW article
Front. Immunol., 19 January 2023
Sec. Inflammation
Volume 13 - 2022 | https://doi.org/10.3389/fimmu.2022.1084460
This article is part of the Research TopicNew Insights of Immune Cells in Cardiovascular and Metabolic DisordersView all 36 articles
Myocardial infarction (MI) is a cardiovascular disease (CVD) with high morbidity and mortality worldwide, often leading to adverse cardiac remodeling and heart failure, which is a serious threat to human life and health. The immune system makes an important contribution to the maintenance of normal cardiac function. In the disease process of MI, necrotic cardiomyocytes release signals that activate nonspecific immunity and trigger the action of specific immunity. Complex immune cells play an important role in all stages of MI progression by removing necrotic cardiomyocytes and tissue and promoting the healing of damaged tissue cells. With the development of biomaterials, cardiac patches have become an emerging method of repairing MI, and the development of engineered cardiac patches through the construction of multiple animal models of MI can help treat MI. This review introduces immune cells involved in the development of MI, summarizes the commonly used animal models of MI and the newly developed cardiac patch, so as to provide scientific reference for the accurate diagnosis and effective treatment of MI.
Myocardial infarction (MI) is a major cardiovascular disease that may lead to death (1). MI is permanent damage to the myocardium caused by prolonged ischemia, which mainly occurs in the left ventricle, and will lead to diffuse discomfort from the chest to all parts of the body (2, 3). MI mainly includes spontaneous MI caused by primary coronary events, secondary MI caused by decreased oxygen supply or increased oxygen demand to the myocardium, and unexpected sudden cardiac death such as cardiac arrest (3). MI can be detected in several ways, the electrocardiogram shows the appearance of Q waves and dynamic changes in the ST-T segment waves, and damaged cardiomyocytes release substances such as myoglobin, lactate dehydrogenase, and creatine kinase (2).
The immune system is an inherent health defense system of animals, an interactive network of lymphoid organs, immune cells, and immunoreactive substances (4). The entire progression of the heart is regulated by the body’s immune system, which plays an important role in healing and remodeling after MI (5). Immune cells are the essential elements that support the immune system in its immune role. Immunity is divided into nonspecific and specific immunity (6). Nonspecific immunity is a natural immune defense developed during the long-term evolution of organisms, providing immediate and conservative host defense that may damage normal tissues because of its nonspecific nature (6). Specific immunity is a kind of acquired and precise immunity that mediates cellular and humoral immunity through T and B cells respectively (7).
After MI occurs, myocardial cells die and necrosis occurs in the tissue of the infarcted region, activating an inflammatory response. Immune cells can both promote cardiomyocyte death and inflammation, and facilitate the regeneration of damaged heart muscle (8). Animal model is an important part of disease research, and the construction of animal models that match the phenotype of human MI is essential for the in-depth study of the mechanism of immune cells and therapeutic approaches (9). The development of biological materials provides a new platform for the treatment of MI and is one of the important methods to achieve repair of the damaged heart (10). Engineered heart patches have been the focus of research in recent years for the development of materials that enable the damaged heart to self-renew (11). This review will be useful in elucidating the immune cells, animal models, and materials associated with MI.
Heart healing after MI goes through three successive stages of development: inflammation, hyperplasia, and maturation (12) (Supplementary Figure 1). Different immune cells infiltrate at different stages of development in response to MI (Figure 1). In the early stages of MI, necrotic myocytes release damage-associated molecular patterns, cytokines, and autoantigens (13). After the onset of MI, an inflammatory outbreak first activates the immune response of nonspecific immune cells, causing rapid entry of monocytes, neutrophils, and dendritic cells from the peripheral vasculature into the infarcted area (14, 15). Subsequently, the infarcted myocardium continues to recruit macrophages that polarize into anti-inflammatory macrophages, which activate the repair of MI by secreting anti-inflammatory cytokines, promoting the formation of granulation tissue, and eliminating dead myocardial cells (16). After the inflammatory phase, a specific immune response initiates the repair of myocardial injury, the extracellular matrix begins to reconstitute, and T and B lymphocytes infiltrate the infarcted area in large numbers (17, 18). T cells are activated by dendritic cells, and regulatory T cells produce cytokines to induce macrophages to polarize and promote myocardial healing (13). Insight into immune cells in MI contributes to the identification of effective therapeutic targets.
Figure 1 Immune cells in MI. The part with pink background on the left is immune cells differentiated to a pro-inflammatory phenotype after MI, and the part with green background on the right is immune cells differentiated to an anti-inflammatory phenotype after MI.
The mononuclear phagocytic system is generated by bone marrow progenitor cells and includes monocytes, macrophages, and dendritic cells (DCs) (19). Monocytes and macrophages are emerging therapeutic targets in cardiovascular disease and are involved in immune response and inflammatory injury after MI (20, 21). Monocytes/macrophages dominate cellular infiltration during the first 2 weeks after MI and are involved in infarct wound healing (22). In the early stages of MI, injury to the heart causes monocytes to infiltrate the area of infarction and then differentiate into macrophages (23). Macrophages are heterogeneous in their differentiation and function, with M1 macrophages secreting proinflammatory factors and M2 macrophages secreting anti-inflammatory factors (24). Studies have shown that healing after MI involves inflammatory Ly-6Chigh and reparative Ly-6Clow biphasic accumulation of monocytes/macrophages (20, 25). Ly-6Chigh monocytes are most abundant on day three of MI and decline on day seven, engulfing necrotic and apoptotic cardiomyocytes and reducing the size of the infarct (25, 26). Ly-6Clow macrophages promote scar formation, increase interleukin (IL) -21 receptor expression and prevent early infarct expansion (27). Studies have shown that treatment of myocardial infarcted mice with the CXC-motif receptor 4 (CXCR4) blocker AMD3100 significantly reduces the content of neutrophils and Ly-6Chigh monocytes, which contributes to the acceleration of the inflammatory phase and promotes the healing of myocardial infarcts (28). At 4-7 days of MI, Ly-6Clow monocytes are recruited to the infarct region via C-X3-C Motif Chemokine Receptor 1(CX3CR1) and promote the repair process (20). C-C Motif Chemokine Receptor 2(CCR2)- and CCR2+ macrophages coordinate monocyte recruitment after myocardial injury (29, 30). Mesenchymal stromal cells (MSCs) have anti-inflammatory effects and cardioprotective functions. In a mouse model of MI, MSCs mediated the transformation from a proinflammatory phenotype to an anti-inflammatory phenotype of macrophages in the infarcted region via IL-10, reducing apoptosis of cardiomyocytes and improving cardiac function (31). Treatment of a mouse model of MI with CCR2 antagonists resulted in increased survival of MSCs in the infarcted region and reduced cardiomyocyte death (32). Targeting the recruitment and differentiation of monocytes and macrophages at different stages of MI is an effective strategy for the treatment of MI. DCs can control monocyte/macrophage homeostasis during post-infarction healing, with DCs levels peaking on day 7 of MI. Studies have shown that mice exhibit left ventricular function deterioration and remodeling 7 days after DCs ablation, and MI disrupted by DCs enhances monocyte/macrophage recruitment (33). Decreased DCs numbers and increased macrophage infiltration play a protective role in post-infarction inflammation and subsequent healing, improving cardiac function and preventing adverse cardiac remodeling (34). Interleukin-37 (IL-37), an inhibitor of innate and adaptive immunity, enabled DCs to acquire the characteristics of tolerogenic DCs (tDCs). IL-37 also can induced regulatory T cells, attenuated inflammatory cell infiltration in the infarcted heart, reduced myocardial fibrosis and improved cardiac function, suggesting that modulation of DCs could be a therapeutic strategy for MI (35, 36).
Granulocytes are mainly divided into neutrophils, eosinophils, and basophils (37). Neutrophils include the proinflammatory N1 subgroup and the anti-inflammatory N2 subgroup. The initial MI contained more N1 neutrophils, and N2 expression was elevated during subsequent anti-inflammatory repair (38). Studies have shown that neutrophil deficiency leads to a decrease in Ly-6Chigh monocytes in mice with macrophage polarization to the M2 phenotype, promoting cardiac repair in MI (39). Reducing neutrophils during inflammation is an effective therapeutic strategy in mice with MI. Blockade of the proinflammatory factor S100A9 secreted by neutrophils reduces the number of neutrophils and monocytes/macrophages, providing an anti-inflammatory environment in the infarcted region and significantly improving cardiac function (40). Studies showed that immature CD10neg neutrophils promote the immune response to inflammation in MI by enhancing Interferon-gamma (IFN-γ) production in CD4 T cells (41). Additional studies have shown that gasdermin D (GSDMD) deficiency in the infarcted heart reduces neutrophil and monocyte content, decreases the extent of MI, and improves cardiac function (42). It is suggested that upstream gene regulation of neutrophils and monocytes is an effective way to treat MI. Eosinophils (EOS) are toxic effector cells that are significantly increased in the blood and heart of myocardial infarcted mice compared to normal mice in the infarcted region. Besides, EOS reduces cardiomyocyte death by secreting substances such as Th2 cytokines, reduces the accumulation of pro-inflammatory cells, enhances neutrophil adhesion, and has a cardioprotective function (43). Additionally, studies have shown that in ST-segment elevation MI in patients and mice, activated eosinophils are recruited to the infarct zone, resulting in a decrease in the number of eosinophils in the blood, attenuating the polarization of anti-inflammatory macrophages and promoting the inflammatory phenotype of MI (44). Crucially, interleukin (IL)-5 secreted by macrophages and CD127+ cells mediate eosinophil development in peripheral blood and infarcted myocardium, promoting recovery from cardiac dysfunction after MI (45). Basophil levels are highest between 3 and 7 days after MI (46). Basophils promote healing and proper scar formation and regulate cardiac remodeling in the late phase of MI by enhancing levels of reparative macrophages and basophil-derived cardiac IL-4 and IL-13 in the infarcted heart (46, 47).
Specific immune responses are critical for wound healing after MI, and after the onset of MI, a large number of T and B cells are recruited in the area of cardiac injury (17). T cells exert immune functions through lymphatic and blood circulation and are mainly divided into CD4+ T cells, CD4+ T cells are mainly divided into Helper T cells (Th), Regulatory T cells (Tregs), and CD8+ T cells (Tc) (48, 49). The cells have multiple phenotypes and play an immune role by activating other immune cells involved in the regulation of MI (50–53). Tregs cells have an immunosuppressive capacity and are enriched in myocardial infarcted mice, inhibiting the proliferation of CD4 and CD8 T cells and their IFN-γ production, promoting infarct repair (54–56). After MI, T cell activation is driven by recognition of the heart’s antigens, and CD4+ T cells promote the healing of myocardial infarct wounds (57, 58). Studies have shown that CD4+ T cell-specific ablation promotes macrophage polarization and contributes to reducing cardiac fibrosis and increasing cardiomyocyte proliferation in young mice (59). After acute MI in mice, CD8 T lymphocytes are recruited and activated in ischemic heart tissue and release granzyme B, leading to apoptosis, adverse ventricular remodeling, and deterioration of myocardial function (60). Infarcted hearts with infiltration of CD4(+)Foxp3(+)CD73(+) regulatory T cell help prevent adverse ventricular remodeling and improve cardiac function after MI by inhibiting inflammation and directly protecting cardiomyocytes (54, 61). In addition, studies have shown that Treg cells reduce the recruitment of IL-17+γδT cell and increase survival in mice with MI (62). CXCR4 antagonist POL5551 attenuated inflammatory gene expression in monocytes and macrophages by enhancing the action of Treg cells and attenuated left ventricular remodeling and systolic dysfunction, suggesting that enhancing Treg cell expression is important for restoring myocardial function (63, 64).
B cells can influence inflammation and remodeling after MI, and the recruitment of pro-inflammatory monocytes into the heart by mature B lymphocytes leads to increased infarct size and worsening cardiac function and can be a promising target for MI therapy (50). Studies have shown that depletion of mature B lymphocytes in mice with MI effectively inhibits C-C Motif Chemokine Ligand 7(CCL7)production and Ly6Chigh monocyte recruitment, improving cardiac function and treating myocardial injury (65). Rituximab is a monoclonal anti-CD20 antibody targeting human B cells (66). MI patients with peak depletion of B cells by rituximab injection on the sixth day after infarction significantly improved myocardial injury and promoted recovery of cardiac function (67). Regulatory B cells (Bregs) have therapeutic potential in a mouse MI model by reducing CCR2-mediated Ly-6Chigh monocyte infiltration, inhibiting cardiac recruitment of proinflammatory monocytes, and improving cardiac function (68). Bone marrow B-cell proliferation ceases within 24 hours of MI, and increasing the level of B cells from bone marrow significantly improves cardiac function and reduces infarct size after MI (69). The complexity of the role of lymphocytes in MI brings difficulties to the treatment. It is necessary to accurately detect the time point of MI in order to determine the role of lymphocytes in targeted and effective therapy.
In cardiovascular disease research, animal models are widely used in the exploration of pathogenesis and drug development. The establishment of experimental animal models of MI is important for the in-depth study of the pathology and treatment-related mechanisms of MI (9). The study of animal models that are highly consistent with the phenotype of human MI can provide a more comprehensive understanding of the progression of immune responses and the function of immune cells in MI and contribute to the therapeutic research of MI (70). Currently, the animals commonly used to make models of MI are mice, rats, rabbits, pigs, and monkeys (9, 71–73). Coronary artery ligation is the most commonly used method for modeling MI (Figure 2A) (9, 71, 73). The coronary artery stenosis or occlusion caused by ligation leads to ischemia and necrosis of the coronary artery feeding myocardium, which leads to MI in animal models, and produces the same pathological process and immune response as human MI. Real-time monitoring and evaluation of the modeling process through an electrocardiogram, pathology, and serum enzymology can achieve better clinical application (9, 71, 73).
Figure 2 Overview of modeling and uses of animal models of MI. (A) The modeling method of the MI model was characterized using mice as an example. First, the mouse was anesthetized and fixed, exposed the heart, and ligated the anterior descending branch of the cardiac coronary artery using sutures to obtain the MI mouse model. Myocardial infarct mice had necrosis of cardiomyocytes and blocked blood vessels. (B) Mice, rats and rabbits have small hearts and cannot be used for human heart transplantation. They can be used to prepare models of MI for research such as drug development. The hearts of monkeys and pigs are highly similar to humans, and gene editing techniques can be used to reduce or avoid immune rejection in human organ transplants.
Most studies have used mice for coronary artery ligation to construct animal models of MI (73). Studies have generally used 8-12-week-old female BALB/c mice (74), male C57BL/6 mice (75), and C57BL/6J mice to induce MI (65). Other studies have used 9-13-week-old adult male C57BL/6J mice (76) or female 10-12-week-old NOD-SCID IL2Rgamma (null) mice (31) to construct the MI models. It was shown that unlike male C57BL/6 mice in the same experiment, female MI mice did not exhibit the corresponding EOS deficient phenotype (43). Therefore, it is necessary to distinguish between male and female animals when selecting animals. For rat MI models, studies have generally used 7-10-week-old female Wistar-Kyoto (WKY) rats (70), adult female Wistar rats (72), 5-7-week-old female Sprague-Dawley (SD) rats (77), male SD rats (78), and male SD rats (79). Moreover, most models of MI in rabbits have been performed on New Zealand White rabbits using coronary artery ligation (80). Studies have also been performed in New Zealand White rabbits using thrombogenic coils placed in circumflex arteries to induce closed thoracic MI, describing post-infarction remodeling in small animal models for the first time in which the pericardium remains intact after coronary artery occlusion, providing a more physiologically and clinically consistent in a vivo detection system for left ventricular dysfunction after MI (71).
Rodent models have physiological features similar to human cardiac anatomy but are smaller in size (81). The cardiac anatomy and physiology of pigs are more similar to humans, especially the structure, size, and distribution of the coronary arteries, and can accurately mimic the phenotype of human MI (82, 83). In some studies, female and male crossbreed Landrace X Large White pigs were used to induce MI by double ligation of the first marginal branch of the left circumrotation artery 1.5 cm distal to the atrioventricular sulcus after left thoracotomy (84). In addition, studies on adult female Yucatan mini-pigs (70) or Mangalica pigs (85) in which MI was induced by balloon catheter occlusion of the coronary arteries for 90 minutes. There are also studies in which MI models were constructed in female Yorkshire pigs by open-heart surgery and LAD ligation of the distal second diagonal branch (77). Rhesus monkeys are genetically and physiologically similar to humans (86). The distribution of the heart and coronary arteries in rhesus monkeys is highly similar to humans, making them one of the best choices for preparing models of MI (87, 88). Studies generally construct MI models by ligating the left anterior descending coronary artery in 2-3 year old rhesus monkeys (88, 89). One study has used the gene-edited pig heart and xenotransplant it into a baboon, managing the severe immune rejection that occurs with xenotransplants and keeping the baboon alive for more than two years (90). Rodent models of MI can be used for research on biopharmaceuticals, monkeys can be used for research in translational medicine, and pigs have the potential to be used for heart xenotransplantation. Furthermore, the application of gene editing technology to MI mapping and allogeneic organ transplantation in heart can effectively control the occurrence of immune rejection (Figure 2B). In the studies of the mechanisms and pathways of MI, the selection of appropriate animals for modeling according to different research purposes and practical situations can help to explore the research methods of MI.
Cardiac tissue engineering and related biomaterials are emerging tools in the treatment of myocardial infarction (Supplementary Table 1) (91, 92). Basic cardiac tissue engineering involves the inoculation of cardiomyocytes or stem cells onto synthetic or natural biocompatible materials in vitro and transplantation into infarcted areas of the heart to promote repair of myocardial damage, with the scaffold degrading as the cells integrate with the organism’s tissue (91, 93). As an emerging strategy in tissue engineering, cardiac patches are a focus of research in myocardial tissue repair engineering (94). Cardiac patches are artificial materials that deliver regenerable cells or bioactive molecules to the site of MI for cardiac repair such as myocardial regeneration and can be used as a novel delivery system for cellular therapies and MI repair factors, with almost no immune rejection when implanted in vivo (95). Studies have embedded therapeutic synthetic cardiac stromal cells (synCSC) into the decellularized myocardial extracellular matrix (myoECM) to generate an artificial cardiac patch (artCP) with therapeutic characteristics of stem cells. The artCPs significantly reduced fibrosis and infarct size, increased surviving myocardial tissue, and improved cardiac function in rat and pig models of MI, overcoming the limitations of using live stem cells and representing a very promising therapeutic strategy (77). Electrical conduction abnormalities in infarcted myocardium induce adverse myocardial remodeling, causes almost no immune rejection, and limit the action of cardiac regenerative drugs (96). A study has developed a combination of electrospinning of gelatin methacryloyl (GelMA) electrospinning and choline-based bio-ionic liquid (Bio-IL) to construct the cardiac patch, GelMA/Bio-IL, which has a mechanical and electrical conductivity similar to that of native myocardium (97). GelMA/Bio-IL is tightly coupled to mouse myocardium to provide stable mechanical properties to the damaged myocardium and restore electromechanical coupling at the site of MI, reducing cardiac remodeling and maintaining normal function (97). Besides, another study developed an electroactive engineered cardiac patch, silk fibroin, and polypyrrole engineered cardiac patch (SP50 ECP), which significantly expressed cardiac marker proteins with good contractility and electrocoupling properties. SP50 ECP can effectively improve left ventricular remodeling in MI, restore ejection function (EF) and other cardiac functions, promote synchronous contraction of CM in the normal scar area of the myocardium, and effectively reduce the susceptibility to the arrhythmia in the rats with MI (98). During MI episodes, ischemia and hypoxia lead to myocardial cell damage and necrosis, inducing an increase in reactive oxygen species (ROS) that exacerbate tissue damage and cardiac remodeling (99). ROS-responsive biomaterials are considered promising antioxidant candidates for MI therapy, and attenuating oxidative stress in MI is beneficial for reducing inflammation and protecting cardiac tissue. Some studies have designed PFTU/gelatin (PFTU/Gt) fibrous patches with excellent antioxidant activity and ROS-responsive degradability to effectively attenuate oxidative stress in the unfavorable tissue microenvironment in vivo after MI in rats, and PFTU/Gt also can inhibit apoptosis, reduce the expression of proinflammatory-related genes, improve cardiac function and angiogenesis, and attenuate poor left ventricular remodeling (100). Currently, microneedle patch is a novel MI treatment that attenuates left ventricle remodeling by mechanical support and is compatible with minimally invasive implantation. Some studies have developed microneedle patches inspired by honeybee venom stings with unidirectional posterior barbs that firmly self-lock on the heart to provide mechanical support to the myocardium of infarcted rats and pigs, significantly reducing wall stress and strain in the infarcted region and maintaining cardiac function and left ventricular morphology (101). Studies of cardiac patches have avoided the harm to the organism from immune rejection, broadened the boundaries of MI therapy, opened up the new direction of subsequent research.
MI has a complex inflammatory response and damage to cardiomyocytes, and immune cells play a very crucial role in the infarcted area. After the onset of MI, monocytes, macrophages, and other cells accumulate in the infarcted region activate and polarize at different times, produce pro- or anti-inflammatory factors, regulate cardiomyocyte proliferation and apoptosis, and influence cardiac remodeling and healing (102). T lymphocytes and B lymphocytes are recruited to the infarcted region after the onset of the nonspecific immune response and participate in the clearance and repair of damaged cells and tissues (59, 65). The anatomical structure of the rodent cardiac model is significantly different from that of the human hearts in size, but the structure of pigs and rhesus monkeys’ hearts is basically the same as that of the human heart. The MI model constructed from pigs and rhesus monkeys can be effectively used for the development of clinical treatment (81–83, 88). With the progress of gene editing technology, studies have already been done to genetically edit pigs to make them resistant to human antibodies and immune cell killing, almost completely avoiding immune rejection from allogeneic organ transplants (90, 103). The pigs breeding cost is far lower than the monkeys, therefore, pig hearts hold the potential for use in allogeneic organ transplants in humans suffering from severe MI. In the development of biomaterials, artificially prepared engineered cardiac patches offer promising new approaches for the treatment and prognosis of MI (101). By optimizing cardiac patch materials loaded with self-proliferating cells or biologic factors with therapeutic benefits, immune rejection is minimized, repair of the infarct site is improved, and integration of the patch with host heart survival and function is promoted, providing damaged regeneration of the heart with mechanical support (95). Targeted modulation of immune cells in MI is a promising strategy, and based on the dual role of many immune cells, there is a need to explore the mechanism of action of immune cells in MI using suitable animal models, and combine bioengineering tools such as cardiac patches to investigate in depth the treatment and repair of human MI.
In this review, we summarize the recruitment and differentiation of major immune cells in nonspecific and specific immunity in MI and elucidate that immune cells of different typologies have different functions in various periods of MI. Additionally, this review also summarizes the current technologically mature animal models of MI and the potential clinical applications of the emerging cardiac patch in the treatment of MI. The in-depth exploration of immune cells provides effective approaches for the treatment of MI.
QF, QL, HZ, GG, and DW wrote the manuscript. QF, QL, HZ, LS, CL, YJ, GG, and DW collected the references and prepared figures. All authors contributed to the article and approved the submitted version.
This work was supported by the Scientific Research Project of Jilin University Key Laboratory ([2019]004), Jilin Science and Technology Development Program 20220505033ZP and 20210203080SF, Jilin Provincial Development and Reform Commission Project 2023C028-6.
The authors declare that the research was conducted in the absence of any commercial or financial relationships that could be construed as a potential conflict of interest.
All claims expressed in this article are solely those of the authors and do not necessarily represent those of their affiliated organizations, or those of the publisher, the editors and the reviewers. Any product that may be evaluated in this article, or claim that may be made by its manufacturer, is not guaranteed or endorsed by the publisher.
The Supplementary Material for this article can be found online at: https://www.frontiersin.org/articles/10.3389/fimmu.2022.1084460/full#supplementary-material
Supplementary Figure 1 | Immune cells that play a major role in the three phases of myocardial infarction.
1. Sahoo S, Losordo DW. Exosomes and cardiac repair after myocardial infarction. Circ Res (2014) 114(2):333–44. doi: 10.1161/circresaha.114.300639
2. Alpert JS, Thygesen K, Antman E, Bassand JP. Myocardial infarction redefined–a consensus document of the joint European society of Cardiology/American college of cardiology committee for the redefinition of myocardial infarction. J Am Coll Cardiol (2000) 36(3):959–69. doi: 10.1016/s0735-1097(00)00804-4
3. Thygesen K, Alpert JS, White HD. Universal definition of myocardial infarction. J Am Coll Cardiol (2007) 50(22):2173–95. doi: 10.1016/j.jacc.2007.09.011
4. Parkin J, Cohen B. An overview of the immune system. Lancet (2001) 357(9270):1777–89. doi: 10.1016/s0140-6736(00)04904-7
5. Swirski FK, Nahrendorf M. Cardioimmunology: The immune system in cardiac homeostasis and disease. Nat Rev Immunol (2018) 18(12):733–44. doi: 10.1038/s41577-018-0065-8
6. Delves PJ, Roitt IM. The immune system. first of two parts. N Engl J Med (2000) 343(1):37–49. doi: 10.1056/nejm200007063430107
7. Bonilla FA, Oettgen HC. Adaptive immunity. J Allergy Clin Immunol (2010) 125(2 Suppl 2):S33–40. doi: 10.1016/j.jaci.2009.09.017
8. Prabhu SD, Frangogiannis NG. The biological basis for cardiac repair after myocardial infarction: From inflammation to fibrosis. Circ Res (2016) 119(1):91–112. doi: 10.1161/circresaha.116.303577
9. Heusch G, Skyschally A, Schulz R. The in-situ pig heart with regional Ischemia/Reperfusion - ready for translation. J Mol Cell Cardiol (2011) 50(6):951–63. doi: 10.1016/j.yjmcc.2011.02.016
10. Li Y, Wei L, Lan L, Gao Y, Zhang Q, Dawit H, et al. Conductive biomaterials for cardiac repair: A review. Acta Biomater (2022) 139:157–78. doi: 10.1016/j.actbio.2021.04.018
11. Tariq U, Gupta M, Pathak S, Patil R, Dohare A, Misra SK. Role of biomaterials in cardiac repair and regeneration: Therapeutic intervention for myocardial infarction. ACS Biomater Sci Eng (2022) 8(8):3271–98. doi: 10.1021/acsbiomaterials.2c00454
12. Frangogiannis NG. The mechanistic basis of infarct healing. Antioxid Redox Signal (2006) 8(11-12):1907–39. doi: 10.1089/ars.2006.8.1907
13. Zhang Y, Wen W, Liu H. The role of immune cells in cardiac remodeling after myocardial infarction. J Cardiovasc Pharmacol (2020) 76(4):407–13. doi: 10.1097/fjc.0000000000000876
14. Ong SB, Hernández-Reséndiz S, Crespo-Avilan GE, Mukhametshina RT, Kwek XY, Cabrera-Fuentes HA, et al. Inflammation following acute myocardial infarction: Multiple players, dynamic roles, and novel therapeutic opportunities. Pharmacol Ther (2018) 186:73–87. doi: 10.1016/j.pharmthera.2018.01.001
15. Andreadou I, Cabrera-Fuentes HA, Devaux Y, Frangogiannis NG, Frantz S, Guzik T, et al. Immune cells as targets for cardioprotection: New players and novel therapeutic opportunities. Cardiovasc Res (2019) 115(7):1117–30. doi: 10.1093/cvr/cvz050
16. Gombozhapova A, Rogovskaya Y, Shurupov V, Rebenkova M, Kzhyshkowska J, Popov SV, et al. Macrophage activation and polarization in post-infarction cardiac remodeling. J BioMed Sci (2017) 24(1):13. doi: 10.1186/s12929-017-0322-3
17. Yan X, Anzai A, Katsumata Y, Matsuhashi T, Ito K, Endo J, et al. Temporal dynamics of cardiac immune cell accumulation following acute myocardial infarction. J Mol Cell Cardiol (2013) 62:24–35. doi: 10.1016/j.yjmcc.2013.04.023
18. Frangogiannis NG. Regulation of the inflammatory response in cardiac repair. Circ Res (2012) 110(1):159–73. doi: 10.1161/circresaha.111.243162
19. Guilliams M, Ginhoux F, Jakubzick C, Naik SH, Onai N, Schraml BU, et al. Dendritic cells, monocytes and macrophages: A unified nomenclature based on ontogeny. Nat Rev Immunol (2014) 14(8):571–8. doi: 10.1038/nri3712
20. Nahrendorf M, Swirski FK, Aikawa E, Stangenberg L, Wurdinger T, Figueiredo JL, et al. The healing myocardium sequentially mobilizes two monocyte subsets with divergent and complementary functions. J Exp Med (2007) 204(12):3037–47. doi: 10.1084/jem.20070885
21. Frantz S, Hofmann U, Fraccarollo D, Schäfer A, Kranepuhl S, Hagedorn I, et al. Monocytes/Macrophages prevent healing defects and left ventricular thrombus formation after myocardial infarction. FASEB J (2013) 27(3):871–81. doi: 10.1096/fj.12-214049
22. Nahrendorf M, Pittet MJ, Swirski FK. Monocytes: Protagonists of infarct inflammation and repair after myocardial infarction. Circulation (2010) 121(22):2437–45. doi: 10.1161/circulationaha.109.916346
23. Heidt T, Courties G, Dutta P, Sager HB, Sebas M, Iwamoto Y, et al. Differential contribution of monocytes to heart macrophages in steady-state and after myocardial infarction. Circ Res (2014) 115(2):284–95. doi: 10.1161/circresaha.115.303567
24. Yunna C, Mengru H, Lei W, Weidong C. Macrophage M1/M2 polarization. Eur J Pharmacol (2020) 877:173090. doi: 10.1016/j.ejphar.2020.173090
25. Hilgendorf I, Gerhardt LM, Tan TC, Winter C, Holderried TA, Chousterman BG, et al. Ly-6chigh monocytes depend on Nr4a1 to balance both inflammatory and reparative phases in the infarcted myocardium. Circ Res (2014) 114(10):1611–22. doi: 10.1161/circresaha.114.303204
26. DeBerge M, Yu S, Dehn S, Ifergan I, Yeap XY, Filipp M, et al. Monocytes prime autoreactive T cells after myocardial infarction. Am J Physiol Heart Circ Physiol (2020) 318(1):H116–h23. doi: 10.1152/ajpheart.00595.2019
27. Kubota A, Suto A, Suga K, Iwata A, Tanaka S, Suzuki K, et al. Inhibition of interleukin-21 prolongs the survival through the promotion of wound healing after myocardial infarction. J Mol Cell Cardiol (2021) 159:48–61. doi: 10.1016/j.yjmcc.2021.06.006
28. Hess A, Derlin T, Koenig T, Diekmann J, Wittneben A, Wang Y, et al. Molecular imaging-guided repair after acute myocardial infarction by targeting the chemokine receptor Cxcr4. Eur Heart J (2020) 41(37):3564–75. doi: 10.1093/eurheartj/ehaa598
29. Chen B, Brickshawana A, Frangogiannis NG. The functional heterogeneity of resident cardiac macrophages in myocardial Injury(Ccr2(+) cells promote inflammation, whereas Ccr2(-) cells protect). Circ Res (2019) 124(2):183–5. doi: 10.1161/circresaha.118.314357
30. Bajpai G, Bredemeyer A, Li W, Zaitsev K, Koenig AL, Lokshina I, et al. Tissue resident Ccr2- and Ccr2+ cardiac macrophages differentially orchestrate monocyte recruitment and fate specification following myocardial injury. Circ Res (2019) 124(2):263–78. doi: 10.1161/circresaha.118.314028
31. Dayan V, Yannarelli G, Billia F, Filomeno P, Wang XH, Davies JE, et al. Mesenchymal stromal cells mediate a switch to alternatively activated Monocytes/Macrophages after acute myocardial infarction. Basic Res Cardiol (2011) 106(6):1299–310. doi: 10.1007/s00395-011-0221-9
32. Lu W, Tang Y, Zhang Z, Zhang X, Yao Y, Fu C, et al. Inhibiting the mobilization of Ly6c(High) monocytes after acute myocardial infarction enhances the efficiency of mesenchymal stromal cell transplantation and curbs myocardial remodeling. Am J Transl Res (2015) 7(3):587–97.
33. Anzai A, Anzai T, Nagai S, Maekawa Y, Naito K, Kaneko H, et al. Regulatory role of dendritic cells in postinfarction healing and left ventricular remodeling. Circulation (2012) 125(10):1234–45. doi: 10.1161/circulationaha.111.052126
34. Nagai T, Honda S, Sugano Y, Matsuyama TA, Ohta-Ogo K, Asaumi Y, et al. Decreased myocardial dendritic cells is associated with impaired reparative fibrosis and development of cardiac rupture after myocardial infarction in humans. J Am Heart Assoc (2014) 3(3):e000839. doi: 10.1161/jaha.114.000839
35. Choo EH, Lee JH, Park EH, Park HE, Jung NC, Kim TH, et al. Infarcted myocardium-primed dendritic cells improve remodeling and cardiac function after myocardial infarction by modulating the regulatory T cell and macrophage polarization. Circulation (2017) 135(15):1444–57. doi: 10.1161/circulationaha.116.023106
36. Zhu R, Sun H, Yu K, Zhong Y, Shi H, Wei Y, et al. Interleukin-37 and dendritic cells treated with interleukin-37 plus troponin I ameliorate cardiac remodeling after myocardial infarction. J Am Heart Assoc (2016) 5(12). doi: 10.1161/jaha.116.004406
37. Stejskal S, Koutná I, Ručka Z. Isolation of granulocytes: Which transcriptome do we analyse - neutrophils or eosinophils? Folia Biol (Praha) (2010) 56(6):252–5.
38. Ma Y, Yabluchanskiy A, Iyer RP, Cannon PL, Flynn ER, Jung M, et al. Temporal neutrophil polarization following myocardial infarction. Cardiovasc Res (2016) 110(1):51–61. doi: 10.1093/cvr/cvw024
39. Horckmans M, Ring L, Duchene J, Santovito D, Schloss MJ, Drechsler M, et al. Neutrophils orchestrate post-myocardial infarction healing by polarizing macrophages towards a reparative phenotype. Eur Heart J (2017) 38(3):187–97. doi: 10.1093/eurheartj/ehw002
40. Marinković G, Grauen Larsen H, Yndigegn T, Szabo IA, Mares RG, de Camp L, et al. Inhibition of pro-inflammatory myeloid cell responses by short-term S100a9 blockade improves cardiac function after myocardial infarction. Eur Heart J (2019) 40(32):2713–23. doi: 10.1093/eurheartj/ehz461
41. Fraccarollo D, Neuser J, Möller J, Riehle C, Galuppo P, Bauersachs J. Expansion of Cd10(Neg) neutrophils and Cd14(+)Hla-Dr(Neg/Low) monocytes driving proinflammatory responses in patients with acute myocardial infarction. Elife (2021) 10. doi: 10.7554/eLife.66808
42. Jiang K, Tu Z, Chen K, Xu Y, Chen F, Xu S, et al. Gasdermin d inhibition confers antineutrophil-mediated cardioprotection in acute myocardial infarction. J Clin Invest (2022) 132(1). doi: 10.1172/jci151268
43. Liu J, Yang C, Liu T, Deng Z, Fang W, Zhang X, et al. Eosinophils improve cardiac function after myocardial infarction. Nat Commun (2020) 11(1):6396. doi: 10.1038/s41467-020-19297-5
44. Toor IS, Rückerl D, Mair I, Ainsworth R, Meloni M, Spiroski AM, et al. Eosinophil deficiency promotes aberrant repair and adverse remodeling following acute myocardial infarction. JACC Basic Transl Sci (2020) 5(7):665–81. doi: 10.1016/j.jacbts.2020.05.005
45. Xu JY, Xiong YY, Tang RJ, Jiang WY, Ning Y, Gong ZT, et al. Interleukin-5-Induced eosinophil population improves cardiac function after myocardial infarction. Cardiovasc Res (2022) 118(9):2165–78. doi: 10.1093/cvr/cvab237
46. Sicklinger F, Meyer IS, Li X, Radtke D, Dicks S, Kornadt MP, et al. Basophils balance healing after myocardial infarction Via il-4/Il-13. J Clin Invest (2021), 131(13). doi: 10.1172/jci136778
47. Prabhu SD. Healing and repair after myocardial infarction: The forgotten but resurgent basophil. J Clin Invest (2021) 131(13). doi: 10.1172/jci150555
48. Savage PA, Klawon DEJ, Miller CH. Regulatory T cell development. Annu Rev Immunol (2020) 38:421–53. doi: 10.1146/annurev-immunol-100219-020937
49. Kaech SM, Wherry EJ, Ahmed R. Effector and memory T-cell differentiation: Implications for vaccine development. Nat Rev Immunol (2002) 2(4):251–62. doi: 10.1038/nri778
50. Hofmann U, Frantz S. Role of lymphocytes in myocardial injury, healing, and remodeling after myocardial infarction. Circ Res (2015) 116(2):354–67. doi: 10.1161/circresaha.116.304072
51. Lin YZ, Wu BW, Lu ZD, Huang Y, Shi Y, Liu H, et al. Circulating Th22 and Th9 levels in patients with acute coronary syndrome. Mediators Inflammation (2013) 2013:635672. doi: 10.1155/2013/635672
52. Mao X, Zhu R, Zhang F, Zhong Y, Yu K, Wei Y, et al. Il-37 plays a beneficial role in patients with acute coronary syndrome. Mediators Inflammation (2019) 2019:9515346. doi: 10.1155/2019/9515346
53. Ávalos AM, Apablaza FA, Quiroz M, Toledo V, Peña JP, Michea L, et al. Il-17a levels increase in the infarcted region of the left ventricle in a rat model of myocardial infarction. Biol Res (2012) 45(2):193–200. doi: 10.4067/s0716-97602012000200012
54. Zhuang R, Meng Q, Ma X, Shi S, Gong S, Liu J, et al. Cd4(+)Foxp3(+)Cd73(+) regulatory T cell promotes cardiac healing post-myocardial infarction. Theranostics (2022) 12(6):2707–21. doi: 10.7150/thno.68437
55. Xia N, Lu Y, Gu M, Li N, Liu M, Jiao J, et al. A unique population of regulatory T cells in heart potentiates cardiac protection from myocardial infarction. Circulation (2020) 142(20):1956–73. doi: 10.1161/circulationaha.120.046789
56. Wang YP, Xie Y, Ma H, Su SA, Wang YD, Wang JA, et al. Regulatory T lymphocytes in myocardial infarction: A promising new therapeutic target. Int J Cardiol (2016) 203:923–8. doi: 10.1016/j.ijcard.2015.11.078
57. Hofmann U, Frantz S. Role of T-cells in myocardial infarction. Eur Heart J (2016) 37(11):873–9. doi: 10.1093/eurheartj/ehv639
58. Hofmann U, Beyersdorf N, Weirather J, Podolskaya A, Bauersachs J, Ertl G, et al. Activation of Cd4+ T lymphocytes improves wound healing and survival after experimental myocardial infarction in mice. Circulation (2012) 125(13):1652–63. doi: 10.1161/circulationaha.111.044164
59. Li J, Liang C, Yang KY, Huang X, Han MY, Li X, et al. Specific ablation of Cd4(+) T-cells promotes heart regeneration in juvenile mice. Theranostics (2020) 10(18):8018–35. doi: 10.7150/thno.42943
60. Santos-Zas I, Lemarié J, Zlatanova I, Cachanado M, Seghezzi JC, Benamer H, et al. Cytotoxic Cd8(+) T cells promote granzyme b-dependent adverse post-ischemic cardiac remodeling. Nat Commun (2021) 12(1):1483. doi: 10.1038/s41467-021-21737-9
61. Tang TT, Yuan J, Zhu ZF, Zhang WC, Xiao H, Xia N, et al. Regulatory T cells ameliorate cardiac remodeling after myocardial infarction. Basic Res Cardiol (2012) 107(1):232. doi: 10.1007/s00395-011-0232-6
62. Blanco-Domínguez R, de la Fuente H, Rodríguez C, Martín-Aguado L, Sánchez-Díaz R, Jiménez-Alejandre R, et al. Cd69 expression on regulatory T cells protects from immune damage after myocardial infarction. J Clin Invest (2022) 132. doi: 10.1172/jci152418
63. Wang Y, Dembowsky K, Chevalier E, Stüve P, Korf-Klingebiel M, Lochner M, et al. C-X-C motif chemokine receptor 4 blockade promotes tissue repair after myocardial infarction by enhancing regulatory T cell mobilization and immune-regulatory function. Circulation (2019) 139(15):1798–812. doi: 10.1161/circulationaha.118.036053
64. Li J, Yang KY, Tam RCY, Chan VW, Lan HY, Hori S, et al. Regulatory T-cells regulate neonatal heart regeneration by potentiating cardiomyocyte proliferation in a paracrine manner. Theranostics (2019) 9(15):4324–41. doi: 10.7150/thno.32734
65. Zouggari Y, Ait-Oufella H, Bonnin P, Simon T, Sage AP, Guérin C, et al. B lymphocytes trigger monocyte mobilization and impair heart function after acute myocardial infarction. Nat Med (2013) 19(10):1273–80. doi: 10.1038/nm.3284
66. Salles G, Barrett M, Foà R, Maurer J, O'Brien S, Valente N, et al. Rituximab in b-cell hematologic malignancies: A review of 20 years of clinical experience. Adv Ther (2017) 34(10):2232–73. doi: 10.1007/s12325-017-0612-x
67. Zhao TX, Aetesam-Ur-Rahman M, Sage AP, Victor S, Kurian R, Fielding S, et al. Rituximab in patients with acute St-elevation myocardial infarction: An experimental medicine safety study. Cardiovasc Res (2022) 118(3):872–82. doi: 10.1093/cvr/cvab113
68. Jiao J, He S, Wang Y, Lu Y, Gu M, Li D, et al. Regulatory b cells improve ventricular remodeling after myocardial infarction by modulating monocyte migration. Basic Res Cardiol (2021) 116(1):46. doi: 10.1007/s00395-021-00886-4
69. Xu Y, Jiang K, Chen F, Qian J, Wang D, Wu Y, et al. Bone marrow-derived naïve b lymphocytes improve heart function after myocardial infarction: A novel cardioprotective mechanism for empagliflozin. Basic Res Cardiol (2022) 117(1):47. doi: 10.1007/s00395-022-00956-1
70. de Couto G, Gallet R, Cambier L, Jaghatspanyan E, Makkar N, Dawkins JF, et al. Exosomal microrna transfer into macrophages mediates cellular postconditioning. Circulation (2017) 136(2):200–14. doi: 10.1161/circulationaha.116.024590
71. Edwards R, Yousef Z, Rakhit R, Wright M, Rosenthal E, Redwood S, et al. A model of closed chest regional myocardial infarction in the rabbit: A clinically relevant in vivo assay system of post-infarction remodelling. Basic Res Cardiol (2002) 97(5):374–83. doi: 10.1007/s003950200046
72. Chi NH, Yang MC, Chung TW, Chen JY, Chou NK, Wang SS. Cardiac repair achieved by bone marrow mesenchymal stem Cells/Silk Fibroin/Hyaluronic acid patches in a rat of myocardial infarction model. Biomaterials (2012) 33(22):5541–51. doi: 10.1016/j.biomaterials.2012.04.030
73. Gottumukkala RV, Lv H, Cornivelli L, Wagers AJ, Kwong RY, Bronson R, et al. Myocardial infarction triggers chronic cardiac autoimmunity in type 1 diabetes. Sci Transl Med (2012) 4(138):138ra80. doi: 10.1126/scitranslmed.3003551
74. Van der Borght K, Scott CL, Nindl V, Bouché A, Martens L, Sichien D, et al. Myocardial infarction primes autoreactive T cells through activation of dendritic cells. Cell Rep (2017) 18(12):3005–17. doi: 10.1016/j.celrep.2017.02.079
75. Zhu Y, Yang W, Wang H, Tang F, Zhu Y, Zhu Q, et al. Hypoxia-primed Monocytes/Macrophages enhance postinfarction myocardial repair. Theranostics (2022) 12(1):307–23. doi: 10.7150/thno.63642
76. Tokutome M, Matoba T, Nakano Y, Okahara A, Fujiwara M, Koga JI, et al. Peroxisome proliferator-activated receptor-gamma targeting nanomedicine promotes cardiac healing after acute myocardial infarction by skewing Monocyte/Macrophage polarization in preclinical animal models. Cardiovasc Res (2019) 115(2):419–31. doi: 10.1093/cvr/cvy200
77. Huang K, Ozpinar EW, Su T, Tang J, Shen D, Qiao L, et al. An off-the-Shelf artificial cardiac patch improves cardiac repair after myocardial infarction in rats and pigs. Sci Transl Med (2020) 12(538). doi: 10.1126/scitranslmed.aat9683
78. Li H, Hu D, Chen G, Zheng D, Li S, Lin Y, et al. Adropin-based dual treatment enhances the therapeutic potential of mesenchymal stem cells in rat myocardial infarction. Cell Death Dis (2021) 12(6):505. doi: 10.1038/s41419-021-03610-1
79. Kameli SM, Khorramirouz R, Eftekharzadeh S, Fendereski K, Daryabari SS, Tavangar SM, et al. Application of tissue-engineered pericardial patch in rat models of myocardial infarction. J BioMed Mater Res A (2018) 106(10):2670–8. doi: 10.1002/jbm.a.36464
80. Feng Y, Chen F, Ma Z, Dekeyzer F, Yu J, Xie Y, et al. Towards stratifying ischemic components by cardiac mri and multifunctional stainings in a rabbit model of myocardial infarction. Theranostics (2013) 4(1):24–35. doi: 10.7150/thno.7188
81. Wessels A, Sedmera D. Developmental anatomy of the heart: A tale of mice and man. Physiol Genomics (2003) 15(3):165–76. doi: 10.1152/physiolgenomics.00033.2003
82. McCall FC, Telukuntla KS, Karantalis V, Suncion VY, Heldman AW, Mushtaq M, et al. Myocardial infarction and intramyocardial injection models in swine. Nat Protoc (2012) 7(8):1479–96. doi: 10.1038/nprot.2012.075
83. Dondelinger RF, Ghysels MP, Brisbois D, Donkers E, Snaps FR, Saunders J, et al. Relevant radiological anatomy of the pig as a training model in interventional radiology. Eur Radiol (1998) 8(7):1254–73. doi: 10.1007/s003300050545
84. Monguió-Tortajada M, Prat-Vidal C, Martínez-Falguera D, Teis A, Soler-Botija C, Courageux Y, et al. Acellular cardiac scaffolds enriched with msc-derived extracellular vesicles limit ventricular remodelling and exert local and systemic immunomodulation in a myocardial infarction porcine model. Theranostics (2022) 12(10):4656–70. doi: 10.7150/thno.72289
85. Batkai S, Genschel C, Viereck J, Rump S, Bär C, Borchert T, et al. Cdr132l improves systolic and diastolic function in a Large animal model of chronic heart failure. Eur Heart J (2021) 42(2):192–201. doi: 10.1093/eurheartj/ehaa791
86. Gibbs RA, Rogers J, Katze MG, Bumgarner R, Weinstock GM, Mardis ER, et al. Evolutionary and biomedical insights from the rhesus macaque genome. Science (2007) 316(5822):222–34. doi: 10.1126/science.1139247
87. Clarkson TB, Prichard RW, Morgan TM, Petrick GS, Klein KP. Remodeling of coronary arteries in human and nonhuman primates. Jama (1994) 271(4):289–94. doi: 10.1001/jama.1994.03510280051032
88. Yang P, Han P, Hou J, Zhang L, Song H, Xie Y, et al. Electrocardiographic characterization of rhesus monkey model of ischemic myocardial infarction induced by left anterior descending artery ligation. Cardiovasc Toxicol (2011) 11(4):365–72. doi: 10.1007/s12012-011-9129-8
89. Wang K, Han P, Huang L, Xiao Y, Hou J, Yang P, et al. An improved monkey model of myocardial ischemic infarction for cardiovascular drug development. Cardiovasc Toxicol (2022) 22(9):787–801. doi: 10.1007/s12012-022-09754-6
90. Mohiuddin MM, Singh AK, Corcoran PC, Thomas Iii ML, Clark T, Lewis BG, et al. Chimeric 2c10r4 anti-Cd40 antibody therapy is critical for long-term survival of Gtko.Hcd46.Htbm pig-to-Primate cardiac xenograft. Nat Commun (2016) 7:11138. doi: 10.1038/ncomms11138
91. Jawad H, Lyon AR, Harding SE, Ali NN, Boccaccini AR. Myocardial tissue engineering. Br Med Bull (2008) 87:31–47. doi: 10.1093/bmb/ldn026
92. Giraud MN, Armbruster C, Carrel T, Tevaearai HT. Current state of the art in myocardial tissue engineering. Tissue Eng (2007) 13(8):1825–36. doi: 10.1089/ten.2006.0110
93. Shin H, Jo S, Mikos AG. Biomimetic materials for tissue engineering. Biomaterials (2003) 24(24):4353–64. doi: 10.1016/s0142-9612(03)00339-9
94. Jackman CP, Ganapathi AM, Asfour H, Qian Y, Allen BW, Li Y, et al. Engineered cardiac tissue patch maintains structural and electrical properties after epicardial implantation. Biomaterials (2018) 159:48–58. doi: 10.1016/j.biomaterials.2018.01.002
95. He L, Chen X. Cardiomyocyte induction and regeneration for myocardial infarction treatment: Cell sources and administration strategies. Adv Healthc Mater (2020) 9(22):e2001175. doi: 10.1002/adhm.202001175
96. Zhao G, Feng Y, Xue L, Cui M, Zhang Q, Xu F, et al. Anisotropic conductive reduced graphene Oxide/Silk matrices promote post-infarction myocardial function by restoring electrical integrity. Acta Biomater (2022) 139:190–203. doi: 10.1016/j.actbio.2021.03.073
97. Walker BW, Lara RP, Yu CH, Sani ES, Kimball W, Joyce S, et al. Engineering a naturally-derived adhesive and conductive cardiopatch. Biomaterials (2019) 207:89–101. doi: 10.1016/j.biomaterials.2019.03.015
98. Yin Q, Zhu P, Liu W, Gao Z, Zhao L, Wang C, et al. A conductive bioengineered cardiac patch for myocardial infarction treatment by improving tissue electrical integrity. Adv Healthc Mater (2022):e2201856. doi: 10.1002/adhm.202201856
99. Zhu Y, Matsumura Y, Velayutham M, Foley LM, Hitchens TK, Wagner WR. Reactive oxygen species scavenging with a biodegradable, thermally responsive hydrogel compatible with soft tissue injection. Biomaterials (2018) 177:98–112. doi: 10.1016/j.biomaterials.2018.05.044
100. Xie J, Yao Y, Wang S, Fan L, Ding J, Gao Y, et al. Alleviating oxidative injury of myocardial infarction by a fibrous polyurethane patch with condensed ros-scavenging backbone units. Adv Healthc Mater (2022) 11(4):e2101855. doi: 10.1002/adhm.202101855
101. Lu Y, Ren T, Zhang H, Jin Q, Shen L, Shan M, et al. A honeybee stinger-inspired self-interlocking microneedle patch and its application in myocardial infarction treatment. Acta Biomater (2022) 153:386–98. doi: 10.1016/j.actbio.2022.09.015
102. Hasan AS, Luo L, Yan C, Zhang TX, Urata Y, Goto S, et al. Cardiosphere-derived cells facilitate heart repair by modulating M1/M2 macrophage polarization and neutrophil recruitment. PloS One (2016) 11(10):e0165255. doi: 10.1371/journal.pone.0165255
Keywords: myocardial infarction, immune cells, non-specific immunity, specific immunity, animal model, heart patch
Citation: Feng Q, Li Q, Zhou H, Sun L, Lin C, Jin Y, Wang D and Guo G (2023) The role of major immune cells in myocardial infarction. Front. Immunol. 13:1084460. doi: 10.3389/fimmu.2022.1084460
Received: 30 October 2022; Accepted: 19 December 2022;
Published: 19 January 2023.
Edited by:
Yingmei Feng, Beijing Youan Hospital, Capital Medical University, ChinaReviewed by:
Ioanna Galani, Biomedical Research Foundation of the Academy of Athens (BRFAA), GreeceCopyright © 2023 Feng, Li, Zhou, Sun, Lin, Jin, Wang and Guo. This is an open-access article distributed under the terms of the Creative Commons Attribution License (CC BY). The use, distribution or reproduction in other forums is permitted, provided the original author(s) and the copyright owner(s) are credited and that the original publication in this journal is cited, in accordance with accepted academic practice. No use, distribution or reproduction is permitted which does not comply with these terms.
*Correspondence: Gongliang Guo, ZG9jZ0BqbHUuZWR1LmNu
†These authors have contributed equally to this work
Disclaimer: All claims expressed in this article are solely those of the authors and do not necessarily represent those of their affiliated organizations, or those of the publisher, the editors and the reviewers. Any product that may be evaluated in this article or claim that may be made by its manufacturer is not guaranteed or endorsed by the publisher.
Research integrity at Frontiers
Learn more about the work of our research integrity team to safeguard the quality of each article we publish.