- 1Diretoria de Ensino e Pesquisa, Fundação Hospitalar de Hematologia e Hemoterapia do Amazonas (HEMOAM), Manaus, Brazil
- 2Programa de Pós-Graduação em Ciências da Saúde, Instituto René Rachou - Fundação Oswaldo Cruz (FIOCRUZ) Minas, Belo Horizonte, Brazil
- 3Grupo Integrado de Pesquisas em Biomarcadores de Diagnóstico e Monitoração, Instituto René Rachou – FIOCRUZ Minas, Belo Horizonte, Brazil
- 4Programa de Pós-Graduação em Imunologia Básica e Aplicada, Instituto de Ciências Biológicas, Universidade Federal do Amazonas (UFAM), Manaus, Brazil
- 5Programa de Pós-Graduação em Ciências Aplicadas à Hematologia, Universidade do Estado do Amazonas (UEA), Manaus, Brazil
- 6Escola de Enfermagem de Manaus, UFAM, Manaus, Brazil
The leukemic microenvironment has a high diversity of immune cells that are phenotypically and functionally distinct. However, our understanding of the biology, immunology, and clinical implications underlying these cells remains poorly investigated. Among the resident immune cells that can infiltrate the leukemic microenvironment are myeloid cells, which correspond to a heterogeneous cell group of the innate immune system. They encompass populations of neutrophils, macrophages, and myeloid-derived suppressor cells (MDSCs). These cells can be abundant in different tissues and, in the leukemic microenvironment, are associated with the clinical outcome of the patient, acting dichotomously to contribute to leukemic progression or stimulate antitumor immune responses. In this review, we detail the current evidence and the many mechanisms that indicate that the activation of different myeloid cell populations may contribute to immunosuppression, survival, or metastatic dissemination, as well as in immunosurveillance and stimulation of specific cytotoxic responses. Furthermore, we broadly discuss the interactions of tumor-associated neutrophils and macrophages (TANs and TAMs, respectively) and MDSCs in the leukemic microenvironment. Finally, we provide new perspectives on the potential of myeloid cell subpopulations as predictive biomarkers of therapeutical response, as well as potential targets in the chemoimmunotherapy of leukemias due to their dual Yin-Yang roles in leukemia.
Introduction
Leukemias correspond to a heterogeneous group of hematological malignancies that are characterized by a blockage in maturation and/or proliferation of hematopoietic cells of the myeloid or lymphoid lineage, which can be divided into acute or chronic forms (1). As a hallmark, acute leukemias have a deep blockage in hematopoietic differentiation, which results in overproduction of leukemic blasts, while chronic leukemias are characterized by the excess production of partially mature differentiated cells (2, 3). Collectively, leukemia affects individuals of all ages; however, in adults, the most common types of leukemia are acute myeloid (AML), chronic myeloid (CML), and chronic lymphocytic leukemia (CLL); while acute lymphoblastic leukemia (ALL) affects mainly children and represents the most frequent pediatric cancer in the world (4–7).
As in other types of cancer, one of the most important mechanisms by which leukemic cells (LCs) promote their development is the generation of an immune microenvironment that inhibits and impairs antitumor responses (8–10). The leukemic microenvironment represents a highly complex cellular compartment that comprises diverse cell populations, which include non-hematopoietic stromal cells, vascular endothelial cells, and innate and adaptive immune cells (11–13). During the process of leukemogenesis, stromal cells within the medullary compartment keep premalignant cells under control; however, as LCs develop, they initiate intense crosstalk with the full range of adjacent cells (11, 14). This dynamic crosstalk between LCs and components of the medullary compartment, in addition to contributing to the increase in the availability of growth and survival factors, also promotes the recruitment and polarization of immune cells into the leukemic niche (10, 11).
Among the immune cells that can infiltrate the leukemic microenvironment are myeloid cell populations, which correspond to a heterogeneous cell group of the innate immune system, including tumor-associated neutrophils (TANs), tumor-associated macrophages (TAMs), and myeloid-derived suppressor cells (MDSCs) (15). These cell populations have received great attention over the last decade because they are recruited to tumor niches on a large scale, and can act as critical components for the suppression of innate and adaptive immune responses, and also in the reduction of efficacy of immunotherapeutic approaches (16, 17). At the same time, due to their high plasticity, the subpopulations of tumor-associated neutrophils and macrophages have been shown to represent important antitumor effector cells, which can eliminate malignant cells and activating other cytotoxic effector cells. This highlights their ability to be reprogrammed into an antitumor phenotype in order to maximize tumoricidal defenses (18–22).
In this context, the advent of a “Yin-Yang” role for these innate immune cell populations in the field of leukemias remains poorly understood, despite their multiple functions and effects in solid tumors being well understood. Thus, in this review, we describe the main aspects related to myeloid cell populations, including their biological characteristics, immunological mechanisms, involvement in tumor-targeted responses, clinical implications, as well as their impact on the leukemic microenvironment. Finally, we provide a new perspective on the importance of investigating the wide diversity of these cell types in hematological malignancies and highlight their potential as prognostic biomarkers and relevant therapeutical targets, which can complement the treatment of patients with leukemia.
Tumor-associated neutrophils (TANs)
Neutrophils are the most abundant leukocytes in the blood and are considered the first line of defense during inflammatory and infectious processes, in which they release a range of inflammatory mediators (23–25). Besides their classical roles in antimicrobial responses, neutrophils can be found in tumor infiltrates, and are named tumor-associated neutrophils (TANs). They also exhibit great plasticity that allows them to perform diverse and often opposite functions (26–29). TANs can display favorable or harmful responses to the host depending on their polarization, which, in a simplified manner, can be classified into two distinct phenotypes, N1 (tumor suppressor) and N2 (tumor promoter) (30, 31).
N1 TANs are usually found in the early stages of the tumorigenic process, and act in the recruitment and activation of cytotoxic T cells and T helper (Th) cells (32–35). They emerge mainly from IFN-β–mediated stimuli, and are characterized by the high expression of immuno-activating cytokines and chemokines, such as TNF, IL-12, CCL-3, CXCL-9, and CXCL-10, in addition to the expression of ICAM-1, FAS and low levels of Arginase-1 (26, 36, 37). As for N2 TANs, they appear in later stages, inhibit effector responses and preferentially recruit regulatory T cells (32–35). They differ mainly after stimulation mediated by TGF-β and IL-35 and, unlike N1 TANs, they are characterized by the overexpression of Arginase-1, the presence of protease-enriched granules, such as neutrophil elastase (NE), cathepsin G (CG) and matrix metalloproteinases (MMPs), in addition to the production of a wide range of chemokines that include CCL-2, CCL-5, and CXCL-8 (Figure 1) (26, 34, 36, 38). Notably, TANs are usually distinguished based on their anti- or pro-tumor functions, as mentioned earlier (33). In terms of expression of intracellular and extracellular markers, the literature generally mentions CD11b, HLA-DRlo/int, CD15hi, CD16hi, and CD66, with varying expression of CD33 and Arginase-1 (16, 36, 39–41). In addition, recent studies have characterized anti- or pro-tumor TAN populations based on the presence and expression of CD54+, HLA-DR+, CD86+ and CD15hi; and CD170hi and PD-L1+ (42).
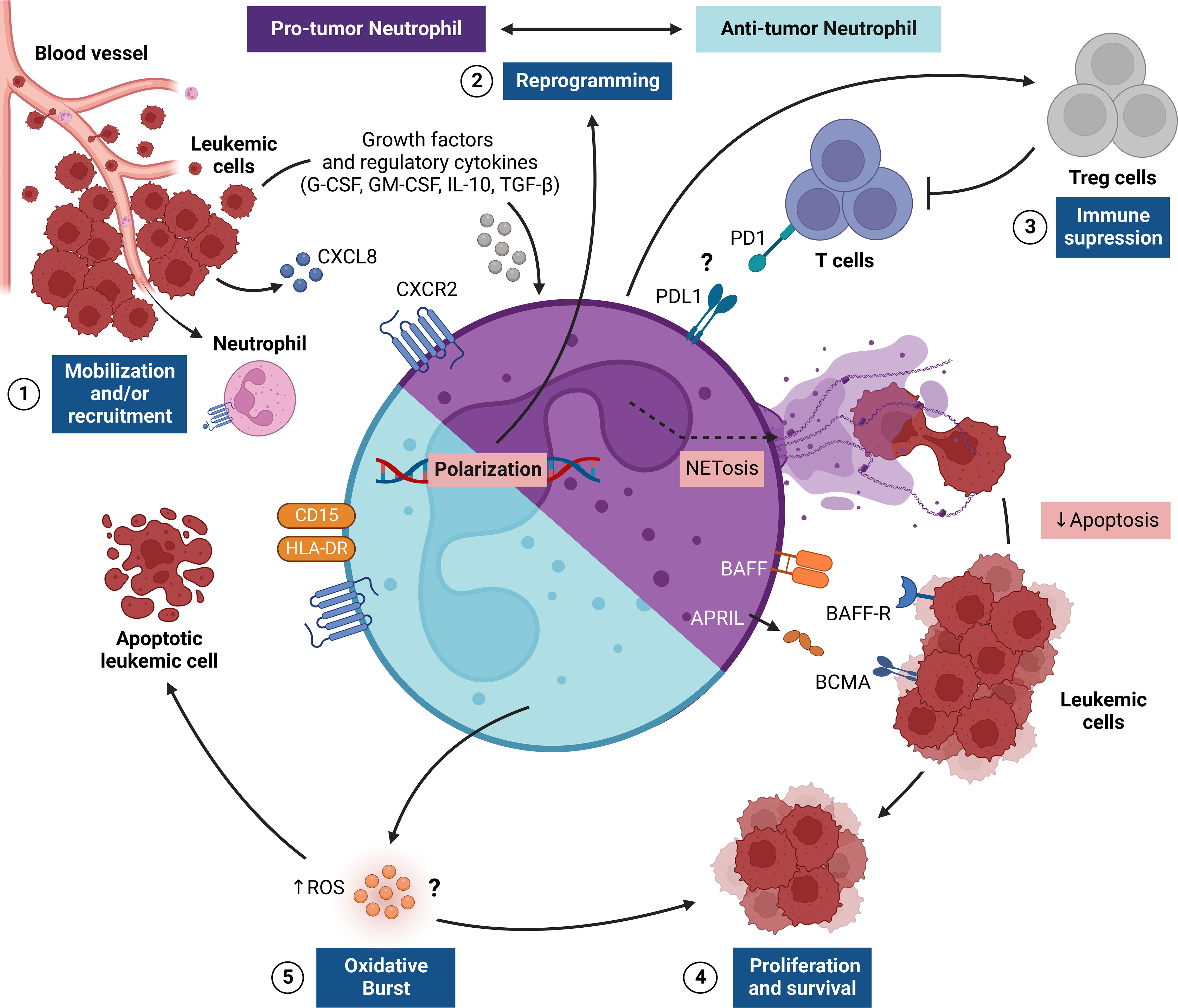
Figure 1 Regulation of TAN responses in the leukemic microenvironment. The figure shows the pro-tumor and antitumor activities of tumor-associated neutrophils (TANs), which provide critical signals for leukemic progression. This network of interactions between TANs, leukemic cells (LCs) and other immune cells [e.g., regulatory T cells (Treg)] results in four main events: [1] recruitment and/or mobilization of neutrophils via CXCL8/CXCR2 to the leukemic microenvironment, where LCs promote the survival of TANs through the release of G-CSF and GM-CSF; [2] reprogramming of TANs to become pro-tumor (N2) or antitumor (N1) cells through stimulation of the leukemic microenvironment through the cytokines IL-10 and TGF-β; [3] immunosuppression promoted by N2 TANs that can preferentially recruit Treg cells and can suppress T cell effector responses, apparently via a PD1-PDL1-mediated pathway; [4] LC proliferation and survival in the leukemic microenvironment regulated by a range of surface molecules (e.g., BAFF and APRIL) expressed by N2 TANs, which also release NETs, which, in turn, delay LC apoptosis; [5] In another scenario, the oxidative burst triggered by N1 TANs can promote the release of reactive oxygen species (ROS) that control proliferation or mediate the killing of LCs. APRIL, A proliferation-inducing ligand; BAFF, B-cell activating factor; G-CSF, granulocyte colony-stimulating-factor; GM-CSF, Human granulocyte-macrophage colony-stimulating factor; NETs, Neutrophil extracellular traps; PD1, Programmed cell death protein 1; PDL1, Programmed cell death-ligand 1; TGF-β, Transforming growth factor-β.
Traditionally, neutrophils act as key elements in the immune system, and play a fundamental role in the immune response through a range of mechanisms, which include: phagocytosis and generation of reactive oxygen species (ROS); antibody-dependent cell-mediated cytotoxicity (ADCC); degranulation and release of proteases; immune cell modulation; and release of extracellular neutrophil traps (NETs), which consists of modified chromatin decorated with bactericidal proteins from granules and cytoplasm (37, 43–46). However, in the tumor context, the effector function of these cells is usually modified to contribute to cancer cell progression through processes such as extracellular matrix remodeling, angiogenesis and lymphangiogenesis, immunosuppression, and promotion of cancer cell invasion and metastasis (47–52). Given these characteristics, besides the fact that they represent up to 70% of circulating leukocytes and are present in large numbers in the tumor microenvironment (TME), TANs are being increasingly investigated in many types of cancer (53–57).
In the context of hematological malignancies, the role of neutrophils in the TME is poorly studied. In the field of leukemias, the role of TANs has been investigated mainly in CLL, in which it was observed that in vitro stimulated neutrophils were more prone to release of NETs, which in turn, have been shown to delay apoptosis and increase the expression of activation markers on LCs (58). This increased susceptibility is driven by the CXCL-8 chemokine, which exhibited significantly elevated levels in the plasma of CLL patients and correlated with the ability to release NETs after neutrophil activation. Of note, plasma from CLL patients has been shown to increase CXCR2 expression in neutrophils, thus allowing CXCL-8-mediated activation; however, the plasma factors responsible for these upregulatory effects remain unknown (58).
Initial observations suggested the possibility that LCs could be driving the maintenance and regulation of TANs to promote leukemic progression. Nonetheless, via further investigations, it was observed that LCs promoted TANs survival through release of G-CSF/GM-CSF and induced their reprogramming through IL-10 and TGF-β cytokines in CD16high CD62Ldim subsets, which are capable of significantly suppressing the effector functions of T cells, such as proliferation and IFN-γ production (59). Moreover, it has been shown that patients with CLL exhibit increased amounts of circulating immunosuppressive neutrophils (CD16high CD62Ldim), and that, depending on the disease stage in the intermediate or advanced state, there is a strong trend toward an increase in the frequency of this TANs subset (59). Such observations reinforce the ability of LCs to induce neutrophil reprogramming to the N2 phenotype and suggest their association with a poor prognosis in CLL patients.
CLL is usually accompanied by immunosuppression and susceptibility to infections (60). Studies carried out seeking to compare the characteristics of neutrophils from patients with CLL who had infections with non-infected patients observed a decrease in chemotaxis and oxidative burst in neutrophils in infected patients (60, 61). Similar results were seen in pediatric patients with ALL, in which oxidative burst was shown to be significantly suppressed in these patients at the time of diagnosis and after remission chemotherapy (62). In addition, studies have also shown that neutrophils from CLL patients have impaired bactericidal activity (60, 63). These data are indicative that neutrophils derived from patients with CLL and ALL tend to lose their effector functions while acquiring pro-tumorigenic capabilities, thus polarizing to the N2 phenotype.
Significant differences in phenotypic characteristics of neutrophils were found in another study that evaluated the expression of receptors and adhesion molecules associated with inflammation. An increased frequency of CD54+ and CD64+ (FcγRI) neutrophils was observed, along with an increase in ROS-generating activity (64). It has been suggested that the activation phenotype of circulating neutrophils could be the result of a systemic inflammatory environment, and is reinforced by high levels of TNF, which would contribute to the survival and proliferation of LCs, while the increase in oxidative potential could be related to chronic activation of the immune system. Interestingly, despite the activation status, neutrophils from CLL patients failed to mount a standard inflammatory response, thus highlighting an unusual activation phenotype with suppressed functional features that possibly comes from stimuli from the leukemic microenvironment (64).
Indeed, stromal cells from the leukemic microenvironment have been shown to interact substantially with neutrophils (65, 66). A study realized in an Eμ-TCL1 CLL murine model indicated that stromal cells from red pulp and the marginal zone of spleens presented LCs infiltrate, overexpressed genes related to neutrophil chemotaxis, including the chemotactic factors S100A8 and S100A9 (MRP8 and MRP14, respectively), the chemokine receptor CXCR2, the pro-inflammatory cytokine IL-1β, and integrin CD11b (Itgam) (65, 66). In addition, neutrophils infiltrated in the leukemic niche were observed to secrete survival cytokines, including APRIL and BAFF, which are important for B cell proliferation. Finally, the neutrophil depletion in Eμ-TCL1 mice, with already developed CLL, demonstrated a reduction in the leukemic burden in spleens, clearly showing that neutrophils support leukemia progression in Eμ-TCL1 mice (65, 66).
Despite all the data presented, little is known about the influence of neutrophils on the development of the antileukemic immune response, especially if we consider the other types of leukemia, which practically remain unexplored. This reinforces the need for investigations into the mechanisms by which neutrophils contribute to disease pathogenesis, besides the impact of their dysfunction on effective immune defense against pathogens and their potential association with the frequent occurrence of severe infections during the period during chemotherapy treatment.
Tumor-associated macrophages (TAMs)
Macrophages are innate effector cells with high phagocytic power and exhibit high functional plasticity that is context-dependent. In other words, they appear in response to different stimuli and assume different functional, phenotypic, and morphological identities (67, 68). Overall, macrophages play a crucial role in activating innate and adaptive responses, since they are important components in defense against infections, tissue repair, antigen presentation, and subsequent initiation of T and NK cell responses in different microenvironments (68).. When detecting tissue alterations, non-activated macrophages (Mφ) assume various activation states within a broad phenotypic and functional spectrum, thus characterizing the M1-M2 macrophage polarization system, which indicates whether these innate immune cells are more pro-inflammatory or anti-inflammatory (69).
M1 or ‘classically activated’ macrophages arise after stimuli mediated by IFN-γ, TNF, GM-CSF, and bacterial LPS. They are capable of producing substantial levels of pro-inflammatory cytokines, such as IL-1β, IL-6, IL-12, IL-23, and TNF (70), and express different intracellular and extracellular markers that allow their distinction, and present cells that are positive for CD68, CD11c, CD14, CD80, CD86, HLA-DR, iNOS and signal transducer and activator of transcription (STAT)-1 (71). On the other hand, M2 or ‘alternatively activated’ macrophages appear after stimuli mediated by cytokines such as IL-4 and IL-13, and are able to produce anti-inflammatory molecules such as IL-10 and TGF-β (Figure 2) (70). Common intracellular and extracellular markers for M2 macrophages include CD68, CD14, CD163, CD204, CD206, STAT3, STAT6, Arginase-1, VEGF and cMAF (72). It is important to note that the designation M2 covers other macrophage populations (M2a, M2b, M2c, and M2d), whose phenotypic and functional diversity remains poorly understood (72).
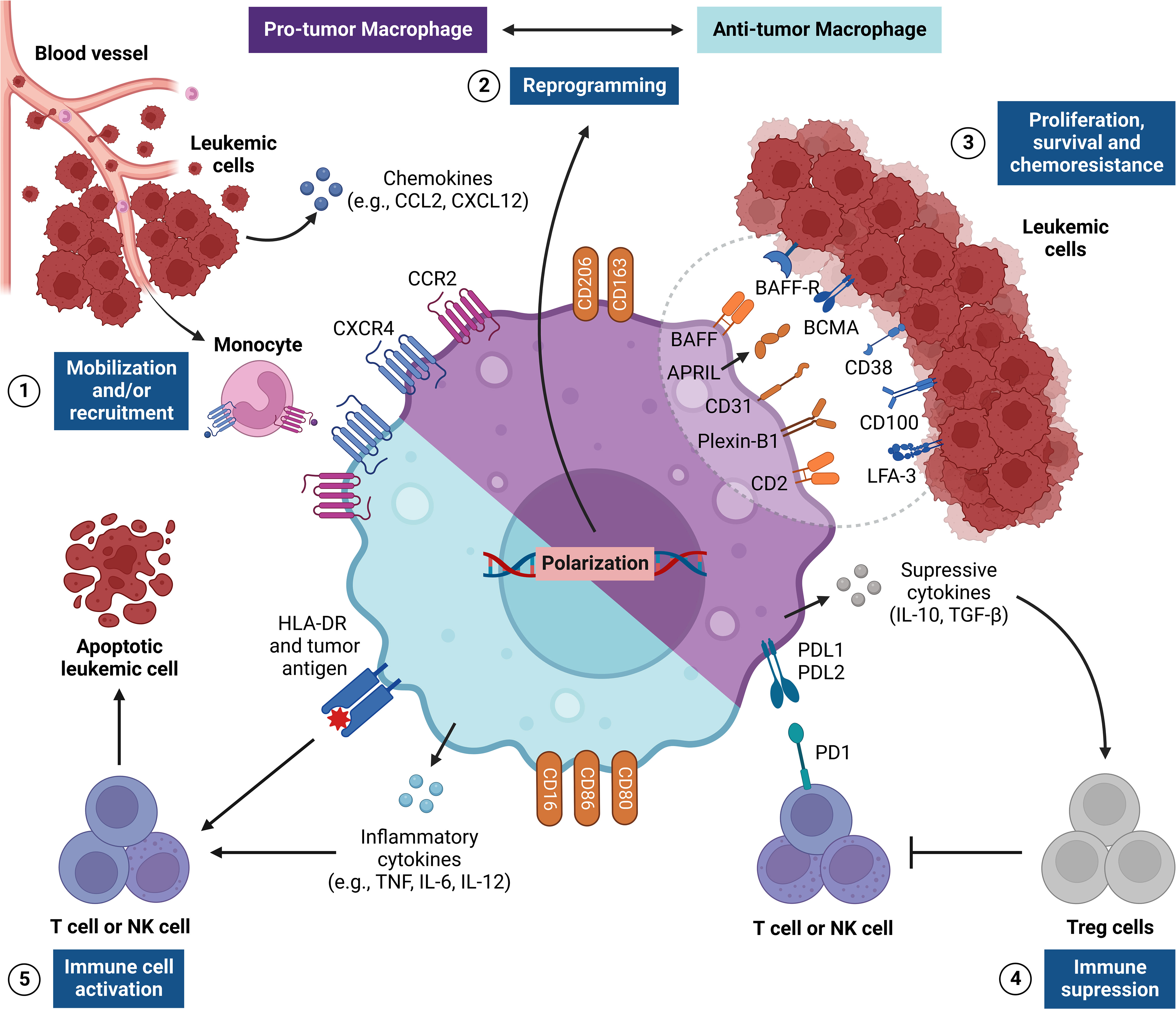
Figure 2 Regulation of TAM responses in the leukemic microenvironment. The figure shows the pro-tumor and antitumor activities of tumor-associated macrophages (TAMs), which are critical signs for leukemic progression. This network of interactions between TAMs, LCs and other immune cells [e.g., NK cells and Treg cells] results in five main events: [1] recruitment and/or mobilization of monocytes via CXCL8/CXCR2 and CCL2/CCR2 to the leukemic microenvironment, which differentiate into TAMs; [2] reprogramming TAMs to become pro-tumor (M2) or antitumor (M1) cells through many stimuli from the leukemic microenvironment, such as cytokines and cell-cell crosstalk (i.e., context dependent); [3] immune interactions with LCs within the leukemic microenvironment through a wide range of surface molecules that send many signals to stimulate the proliferation, survival and chemoresistance in LCs (e.g., BAFF, APRIL, CD31, Plexin-B1 and CD2); [4] establishment of a strongly tolerogenic environment, such as M2 TAMs, which produce many suppressive cytokines (e.g., IL-10 and TGF-β) and express immune checkpoints (PDL1 and/or PDL2), and which regulate the responses of T NK cells, and stimulate Treg cell activities; [5] establishment of an inflammatory environment, such as M1 TAMs, thus stimulating the cytotoxic activity of T and NK cells by the production of inflammatory cytokines (e.g., TNF and IL-12) and the expression of co-stimulatory molecules (e.g., CD80, CD86 and HLA-DR), which can promote antitumor responses and the death of the tumor. APRIL, A proliferation-inducing ligand; BAFF, B-cell activating factor; BAFF-R, BAFF receptor; BCMA, B-cell maturation antigen; CCR2, C-C motif chemokine receptor 2; CXCR4, C-X-C chemokine receptor 4; HLA-DR, Human leukocyte antigen DR; LFA-3, Lymphocyte function-associated antigen 3; NK, Natural killer; PD1, Programmed cell death protein 1.
The different subsets of macrophages are critically involved in the progression or regression of several diseases, including cancer (73). As the tumors progress, they are accompanied by an extensively dysregulated hematopoiesis, reflecting in a continuous expansion and renewal of myeloid cells in the bone marrow (BM) and in peripheral sites, which travel between different tissues and contribute to immunosuppression or immunosurveillance (74, 75). As highly heterogeneous cells, macrophages are in continuous communication with the periphery beyond the TME. Therefore, the presence of tumor-associated macrophages (TAMs) is now seen as an important signal of the regulation of tumor immunity, since (i) TAMs infiltrate the TME to regulate tumor growth and (ii) the TME shapes the activity and functional use of TAMs to promote cancer cell metastasis and survival (76).
Many signals that shape the M1/M2 polarization vary according to the type, stage, location of the tumor, and result in different highly dynamic TAM phenotypes (77). Each macrophage is not always functionally restricted to a specific role. For example, it is important to note that IL-6 and IL-1β-producing TAMs, possibly M1-like, promote tumor growth through the production of these cytokines, which shows that M1 TAMs are not always able to precisely exert their antitumor functions during cancer progression (78–80). Further strong evidence of this is that, in some tumors, TAMs that exhibit an M2 phenotype (CD163+ CD206+) perform an activity that is equivalent to M1-like TAMs, and initiate antitumor responses (81–83). Therefore, the M1/M2 classification is too simplistic for this highly heterogeneous cell type.
Generally, TAMs have a recognized role in solid tumors, but their involvement in hematologic malignancies, such as leukemia, remains unclear. There is evidence that crosstalk between TAMs and LCs occurs at different sites during leukemia progression (60, 84–90). The fact that these cells are widely distributed in tissues suggests that TAMs travel to leukemic niches and support the proliferation of LCs, which influences the clinical outcome of the disease (90–94). These early insights are supported by several clinical and preclinical findings that have demonstrated that tumor progression affects the biodistribution of TAMs in the BM and at extramedullary sites, in which M1/M2 balance is extensively dysregulated following recruitment and reprogramming of TAMs to a pro-tumor phenotype (M2) (88, 90, 95–99).
Mobilization of TAMs to leukemic niches is supported by chemokines such as CCL2, CCL3, CCL4, and CXCL12, which regulate their biodistribution (100, 101). Among these molecules, CXCL12 promotes the mobilization of CXCR4+ TAMs to the BM microenvironment and supports M2 polarization (87, 102–104). It is important to note that CCL2 also regulates the infiltration of TAMs into the TME, which, in turn, also produces CCL2 and amplifies the mobilization of more CCR2+ macrophages to the tumor niche (104–106). Leukemia patients exhibit elevated levels of CCL2 and CXCL12 in the BM and blood, which can critically influence the activation and recruitment of TAMs (104, 107–111).
Another important point is that extramedullary sites, such as the lymph nodes, the spleen and the liver, also support the growth and metastatic spread of LCs, and macrophages act as essential stromal components in these compartments (112). Therefore, the idea that local macrophage-mediated signaling pathways are decisive elements for tumor progression is well established in the context of leukemia (91, 113, 114). In line with this, several reports have shown that LCs mobilize CCR1+ or CCR4+ TAMs in lymph nodes via CCL3 and CCL4 (100, 115), which in turn preserve the survival of LCs through cell-cell interactions (116). Similar events also occur in the spleen and liver of mice, in which a high accumulation of M2 TAMs is regulated by the CCR2-CCL2 axis (95, 117). On the other hand, in some cases, M1 TAMs exhibit a curious predominance in the BM and liver of these mice (118). This is evidenced when spleen-derived macrophages are cultured with LCs and promote greater tumor growth in vitro when compared to cultured BM-derived macrophages (99, 119), thus suggesting that different subsets of TAMs may play pleiotropic roles at different sites, since liver TAMs exhibited greater production of inflammatory factors such as CCL5, TNF, and IL-12 than BM and spleen TAMs (120, 121).
By infiltrating different leukemic niches, M2 TAMs produce soluble mediators that support LC expansion and survival (84). When monocytes from healthy donors are cultured with LCs, they differentiate into M2 and increase the resistance of LCs to apoptosis via CXCL12 secretion (113). Additionally, some cytokines and chemokines may also participate in this process, since M2 TAMs also secrete IL-8, IL-10, TGF-β, CCL2, CCL4, CXCL13, and other soluble mediators that are capable of inducing tumor growth in vitro and in vivo (98, 117, 121–125). In turn, LCs can produce substantial levels of IL-4, IL-10, IL-13, NAMPT, Arginase-2, and BMP-4, which regulate the pro-tumor functions of TAMs (84, 122, 123, 126).
An emerging mechanism of cell-cell communication during tumor progression is the release of exosomes, endosomal-derived vesicles, which carry different molecular components (e.g., nucleic acids, proteins, or metabolites) and are present in several biological fluids, being secreted by many cell populations, including immune and cancer cells (127, 128). These extracellular vesicles play an important role in the crosstalk between LCs and TAMs and reprogram these leukocytes into leukemia-supportive effector cells. Previous studies have reported that LC-derived exosomes are able to polarize macrophages to an immunosuppressive phenotype with enriched expression of PD-L1 on the cell surface and overproduction of IL-10 (129, 130). The high expression of PD-L1 by TAMs allows it to suppress the activity of PD1+ T cells and NK cells (11, 117).
Other receptors act to send survival signals to LCs. Studies have shown that CD163 binds to the unknown ligand on LCs, and its expression correlates with an increased leukemia burden (90, 98). In this sense, it is worth noting that CD163 induces a strong production of IL-10 by macrophages (131), in which this cytokine is a potent stimulator of malignant B cells (132, 133). In addition, M2 TAMs upregulate the expression of BAFF and APRIL, which bind to BAFF-R and BCMA expressed in LCs, respectively (134, 135). These ligands may be related to the resistance of LCs to chemotherapy and apoptosis, as previously reported (134, 136, 137). Finally, the expression of CD31 and plexin-B1 by these cells leads to an increased potential for LC survival (101, 138). This is because LCs express CD38 and CD100, which are receptors for CD31 and plexin-B1, respectively (138, 139). The CD2–LFA-3 axis was also shown to be critical in the crosstalk between M2 TAMs and LCs, as it also acts on the sending of stimulatory signals to LCs (116).
Although the functions of TAMs are often related to leukemic progression, it is still difficult to establish their precise prognostic value in leukemia. While several reports highlight their strong pro-tumor interactions through the production of soluble mediators and surface receptors, some evidence suggests that M1 TAMs can be mobilized to the BM or extramedullary sites (99, 118–121). Whether they are playing a protective role is still an open question, but strong evidence of this is that when M2 TAMs are treated with IFN-γ, they polarize to an M1 profile, inhibit their regulatory activity, and increase HLA-DR expression, CD86, and CD64, and exhibit a high antileukemic response in vitro (140). However, the antitumor activity of TAMs in leukemia remains poorly investigated; although, it is evident that M1 TAMs produce inflammatory factors in the TME (120, 121). The fact is that the presence of M2 TAMs is generally associated with an increased leukemic burden and, subsequently, an unfavorable prognosis (90, 118, 141). Therefore, future investigations should focus on the different macrophage subsets and their functional and prognostic relevance in different leukemia subtypes.
Myeloid-derived suppressor cells (MDSCs)
Myeloid-derived suppressor cells (MDSCs) correspond to a phenotypically heterogeneous cell population that is derived from immature myeloid precursors of the granulocytic or monocytic lineage (16). The ontogeny process of MDSCs involves blocking differentiation in normal hematopoiesis, thus preventing their terminal differentiation, and promoting their expansion. This feature highlights their distinction from terminally differentiated, mature myeloid cells; although their distinction from neutrophils is usually a controversial topic (16, 142). However, studies have shown that these cells can also differentiate from a monocyte-like precursor of granulocytes (143). In humans, there is still a recently discovered subpopulation known as “early MDSCs”, which represent less than 5% of the MDSC population; however, little is known about their role (144). In general, as evidenced by their name, pathologically activated MDSCs exhibit strong immunosuppressive capabilities, and act as crucial drivers of an immunosuppressive microenvironment (16).
MDSCs are subdivided into two groups: polymorphonuclear MDSCs (PMN-MDSCs), which are morphologically similar to neutrophils; and monocytic MDSCs (M-MDSCs), which are morphologically similar to monocytes (142, 145). In humans, they are identified from specific cell markers; however, these are far from uniform. PMN-MDSCs are defined as CD11b+ CD14-CD15+/CD66+ cells (146–148). In turn, M-MDSCs are defined as CD14+ CD15-/CD66- HLA-DRlo/- (144). CD14 is a characteristic surface marker of monocytes, while HLA-DRlo/- helps distinguish M-MDSCs from mature monocytes and CD15- distinguishes M-MDSCs from PMN-MDSCs (149). As PMN-MDSCs are morphologically and phenotypically like classical neutrophils, the main way to differentiate them is functionally, i.e., based on their ability to suppress other immune cells, since normal neutrophils are not immunosuppressive cells (150–152). On the other hand, there is still an intense debate on the distinction between N2 TANs and PMN-MDSCs, due to shared origin, phenotypic and functional characteristics. However, despite the high similarity between these cell populations, it has recently been shown that lectin-like oxidized LDL receptor-1 (LOX-1), which is highly expressed in human PMN-MDSCs, may represent a specific marker to distinguish these cells from mature neutrophils in peripheral blood and tumor tissues (153).
PMN-MDSCs and M-MDSCs are activated by prolonged stimulation that is mediated by growth factors and pro-inflammatory cytokines (GM-CSF, CSF1, IL-6, and 1L-1β), as seen in conditions such as cancer, chronic infections, and autoimmune diseases (144). The stimuli that lead to their activation occur in two stages, and these are referred to as Phase 1, which is characterized by the expansion of MDSCs, and Phase 2, which is characterized by differentiation into a granulocytic or monocytic lineage (154). The expansion and activation of these cells are both dependent on transcription factors such as STAT1, STAT3, STAT6, and NF-κB, which result in the upregulation of the cytokines IL-10, TGF-β, and, in some conditions, IFN-γ; of immunosuppressive factors such as arginase 1 (ARG1), inducible nitric oxide synthase (iNOS) and reactive oxygen species (ROS); in addition to immunological checkpoint inhibitors such as Programmed death-ligand 1 (PDL1) and V-domain Ig suppressor of T cell activation (VISTA) (Figure 3). Together, these mediators promote anergy of cytotoxic T cells and tumor-specific T helper (Th); expansion of regulatory T cells (Treg); reprogramming of TANs and TAMs in N2 and M2, respectively; and decrease in L-arginine and L-cysteine, which are amino acids necessary for the activation and proliferation of T cells, thus collaborating in the remodeling of an immunosuppressive microenvironment that is susceptible to neoplastic progression (155–162).
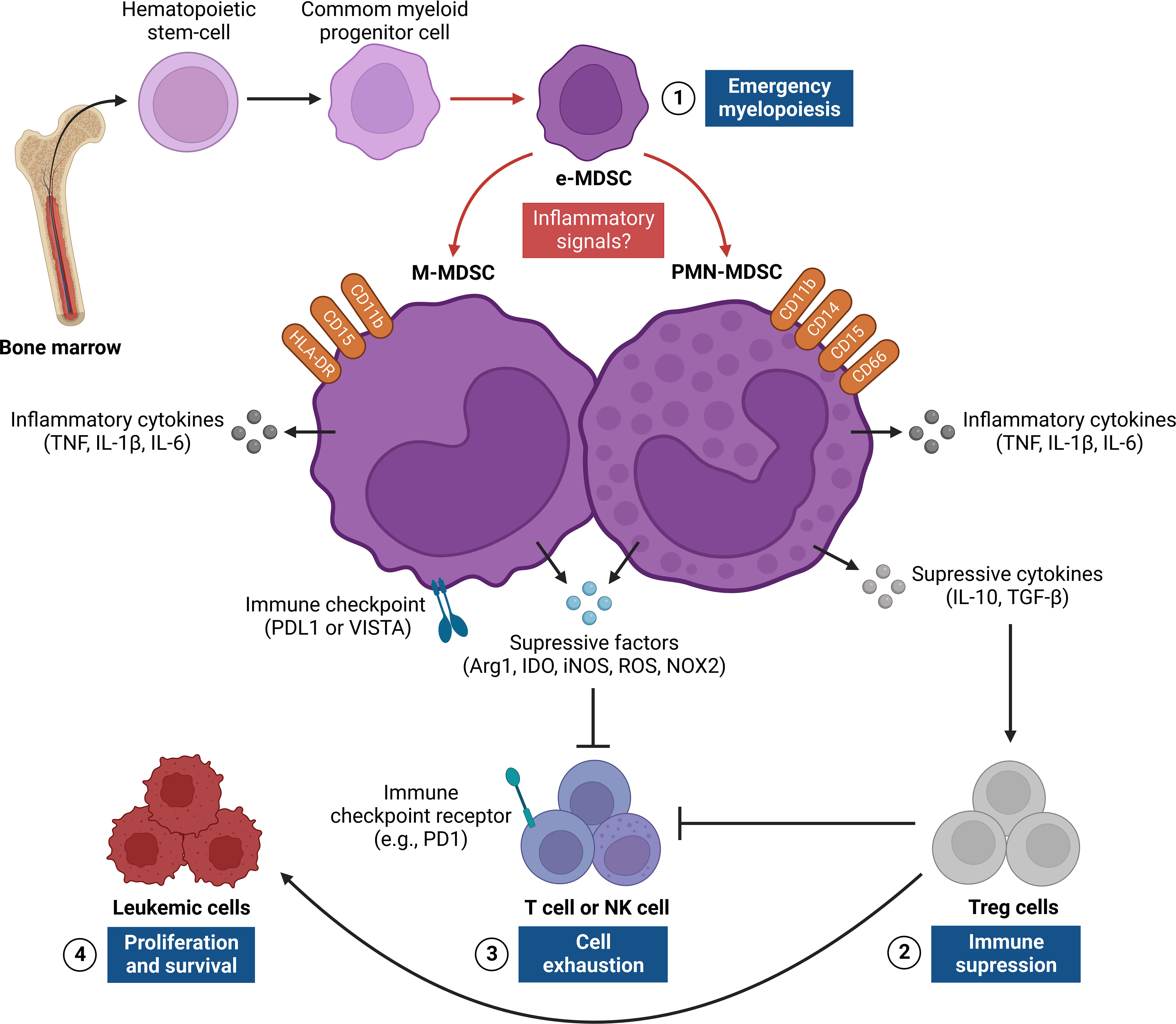
Figure 3 Regulation of MDSC responses in the leukemic microenvironment. The figure shows the origin and pro-tumor activities of myeloid-derived suppressor cells (MDSCs), which provide critical signals for leukemic progression. This network of interactions between MDSCs, LCs, and other immune cells [e.g., T cells, NK cells, and Treg cells] results in four main events: [1] emergence of MDSCs in bone marrow from common myeloid progenitor cells that differentiate into PMN-MDSC and M-MDSC after persistent inflammatory signals, thus characterizing a process known as “emergency myelopoiesis”; [2] activation and recruitment of Treg cells through the release of regulatory cytokines (e.g., IL-10, TGF-β), which contribute to immunosuppression of the leukemic microenvironment;[3] exhaustion of T and NK cells from different inflammatory (e.g., IL-6, TNF and IL-1β) and/or anti-inflammatory (e.g., IL-10, TGF-β, Arg1, IDO and iNOS) mediators and surface molecules (PDL1 or VISTA) expressed by MDSCs, which regulate antitumor responses;[4] LC proliferation and survival, which are a consequence of suppression of T cell and NK cell effector responses and activation of Treg cells in the leukemic microenvironment. Arg1, Arginase 1; IDO, Indoleamine; iNOS, nitric oxide synthase; M-MDSC, monocytic-MDSC; NK, Natural killer; PDL1, Programmed death-ligand 1; PMN-MDSC, polymorphic mononuclear-MDSC; TGF-β, Transforming growth factor beta; TNF, Necrosis factor tumoral; Treg, Regulatory T cells; VISTA, V-domain Ig suppressor of T cell activation.
In addition, MDSCs may harbor tumor-promoting functions that are independent of immune suppression, such as promoting metastasis and angiogenesis through the production of vascular endothelial growth factor (VEGF), fibroblast growth factor β (FGF-β) and matrix metalloproteinase-9 (MMP9). Furthermore, studies have described that these cells can be mobilized from the BM through G-CSF, GM-CSF, or hypoxia to metastatic environments, in which pro-inflammatory mediators, such as IL-6, TNF-α, and prostaglandin E2 (PGE2), can increase their immunosuppressive functions (16, 75, 163, 164). Thus, once activated, MDSCs become crucial factors in TME and have a significant role in the development of several solid and hematological neoplasms, in addition to being frequently associated with different stages of cancer (165–169).
In the field of acute leukemias, studies performed in pediatric patients with B-cell ALL showed a significant increase in the frequency of PMN-MDSCs, along with T reg cells in the circulation and BM compartment, which exhibited a positive association with the presence of measurable residual disease (MRD) (170). In addition, it was observed that the number of PMN-MDSCs decreased markedly in patients who went into remission, and was comparable to the control group (171). Similarly, in adult AML patients, MDSCs (CD33+ CD11b+ HLA-DRlow/neg) were significantly increased in BM and were associated with extramedullary infiltration and increased serum D-dimer concentration in plasma; however, after induction chemotherapy, there was a decrease in the frequency of these cells (172). In addition, the frequency of M-MDSCs also showed a significant increase, both in circulation and in the percentage of peripheral blood mononuclear cells (PBMCs), which was associated with a low rate of remission, high rate of relapse, and low long-term survival (173).
Regarding chronic leukemias, it was reported that patients with high-risk CML showed an increase in the frequency of PMN-MDSCs, as well as in the expression of Arg-1, which is known to inhibit T cells. In addition, PMN-MDSCs exhibited a positive upregulation for PD-L1, in conjunction with the PD-1 receptor on T cells (174). Studies also observed that the frequency of PMN-MDSCs was elevated at the time of diagnosis, and decreased to normal percentages after imatinib therapy (175). Finally, studies performed on patients with CML and CLL also observed a significant increase in the frequency of M-MDSCs at diagnosis, together with increased expression of IL-10 and TGF-β, which in vitro have been shown to induce T cell suppression and activation of Treg cells (174, 176, 177). In general, high frequencies of PMN-MDSCs and M-MDSCs can directly influence the clinical course of acute and chronic leukemias, thus highlighting their role as potential prognostic biomarkers and therapeutic targets in these patients (178–182).
Concluding remarks and future perspectives
The ‘Hallmarks of Cancer’ were proposed as a set of functional capabilities acquired by human cells as they progress from normality to neoplastic growth states (183). In the most recent elaboration of this concept, after little more than a decade of incessant research into the immunobiology of cancer, the role of immune cells in the neoplastic progression is well recognized. Today, inflammation and immune evasion are considered hallmarks of cancer progression, highlighting the direct involvement of immune cells, including myeloid lineage cell populations (184). Supporting this fact, TANs, TAMs, and MDSCs represent one of the main immune infiltrates in tumor niches, and are usually associated with suppressive mechanisms that attenuate immune surveillance, cytotoxic response, and, in many cases, the success of T cell-based immunotherapies (16).
Similarly, eosinophils and basophils also infiltrate multiple tumors and are activated to regulate tumor progression, either by directly interacting with cancer cells or indirectly by modulating the TME (185–188). Platelets, small anucleate structures derived from BM megakaryocytes, have also been shown to play a broad role in tumor progression, and favor proliferation from the release of growth factors and drug resistance (189–191). In addition, they act in the formation of secondary niches through a mechanism called “cloaking”, in which platelets produce physical protection in cancer cells, thus assisting in vascular migration and extravasation to the tissues to form metastases (192–194). It is noteworthy that, although promising, these myeloid cell populations and products still present themselves as poorly recognized targets and therefore require further investigation.
In this review, we seek to elucidate the role, mechanisms, and clinical implications of TANs, TAMs, and MDSCs in the leukemic microenvironment (Figure 4), as well as in the prognosis of patients with leukemia (Table 1). Based on the body of evidence, it is possible to suggest that the high frequency of tumor-associated neutrophils and macrophages, leaning towards an anti-inflammatory phenotype (N2 and M2, respectively), along with PMN-MDSCs and M-MDSCs could contribute to the identification of patients that are characterized by high-risk of disease at diagnosis and during treatment. In addition, several studies have highlighted the role of these cell populations as critical determinants of resistance to chemoimmunotherapy and targeted therapy, acting as a “Yin” role (5–10).
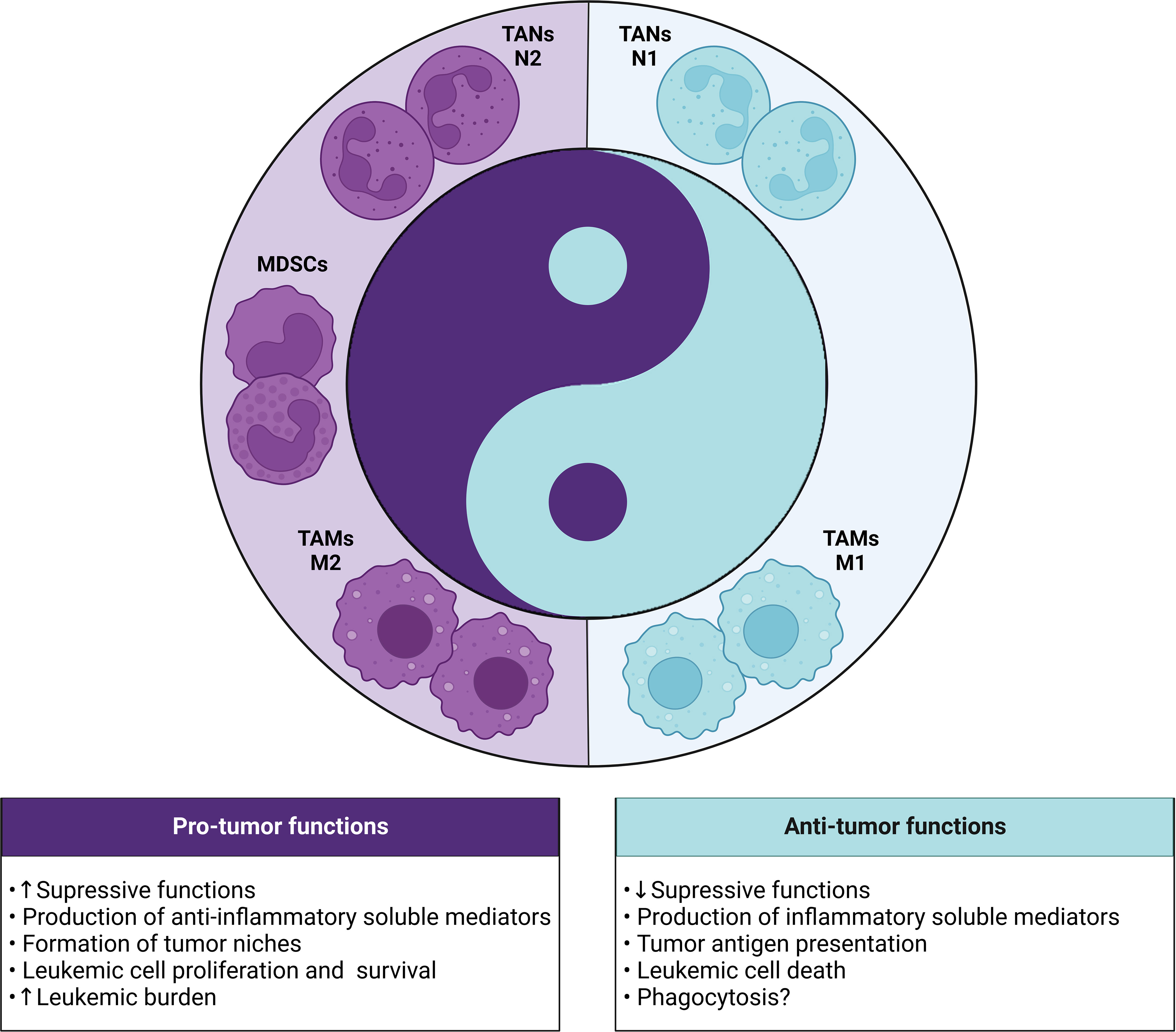
Figure 4 Yin-Yang with the dual role (pro and antitumor) of myeloid cells in the leukemic microenvironment. In leukemia, the different subsets of myeloid cells (TANs, TAMs and MDSCs) can exert dichotomous functions dictated by the polarization status of each cell. The pro-tumor phenotype is highlighted by the presence of N2 TANs, M2 TAMs and MDSCs, which show strong immunosuppressive activity through the production of anti-inflammatory mediators and formation of leukemic niches that contribute to the increase in tumor burden. In contrast, the antitumor phenotype involves the presence of N1 TANs and M1 TAMs that can exert protective functions through the release of cytotoxic mediators, tumor antigen presentation, death or phagocytosis of leukemic cells.
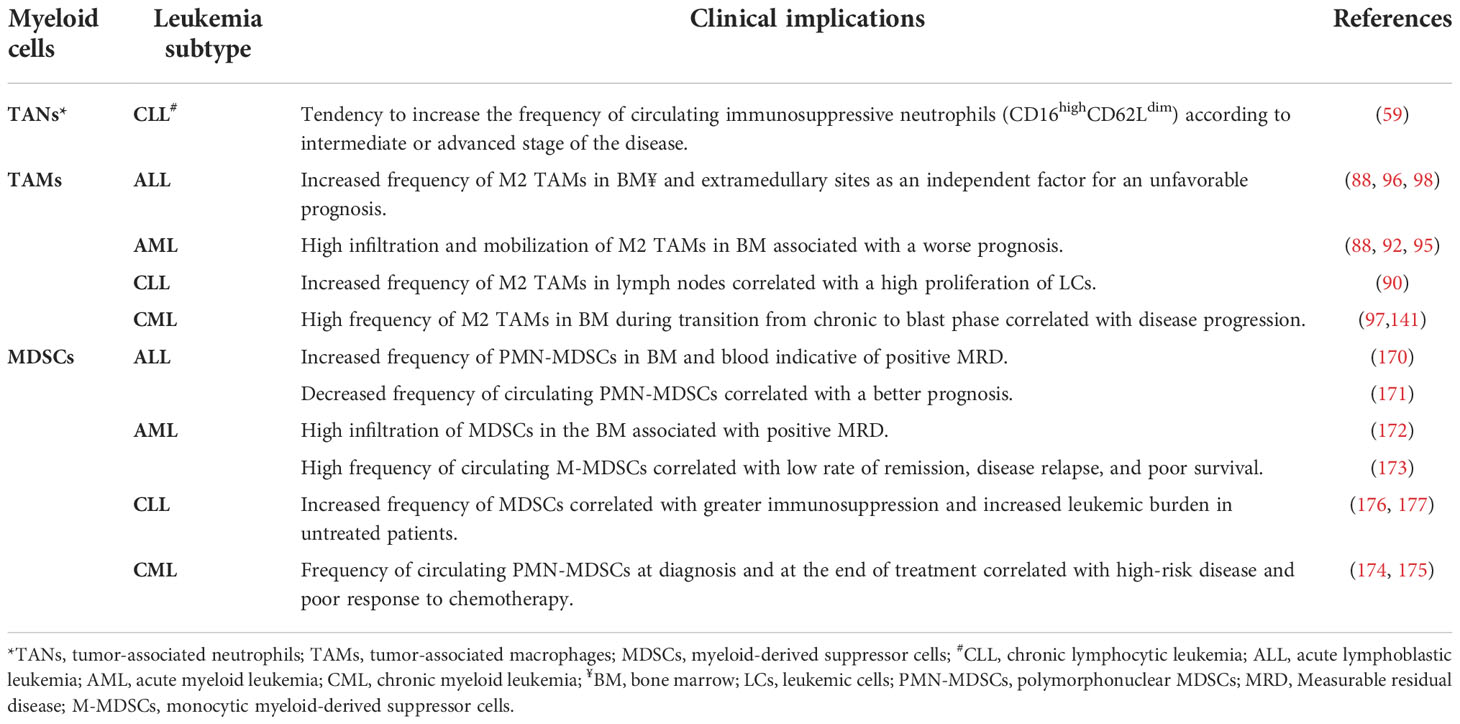
Table 1 Association of frequency of myeloid cell populations with the clinical implications of leukemia patients.
On the other hand, we also cannot rule out the potential “Yang” role of myeloid cells in stimulating the immune response. Recent technological advances have helped the generation of genetically modified myeloid cells to enhance their antitumor properties. In summary, through genetic engineering, these cells increase the expression of cell surface receptors and antigens, as well as cytokines capable of modulating TME contributing to a pro-inflammatory environment, thus increasing the activation of cytotoxic immune cells (195). In in vivo studies, genetically modified myeloid cells have been shown to be able to migrate to primary tumor niches, which increases antigen presentation and IFN-γ production, promotes T cell activation, and reduces tumor burden (196, 197).
Collectively, these data indicate that the reprogramming or repolarization of myeloid cells presents itself as a promising and effective strategy, which should be explored in the context of immunotherapeutic approaches aimed at leukemias, especially considering the large cellular repertoire of the leukemic microenvironment, in addition to the intense and dynamic crosstalk between LCs and surrounding cells. Finally, it is important to highlight the scarcity of data on the dual role (Yin-Yang) of myeloid cell populations in different types of leukemia, since the characterization of the immune microenvironment of the medullary compartment can indicate relevant therapeutic targets and follow-up biomarkers of patients, in addition to providing promising immunotherapies, which would aid in controlling the disease in the long term and improving quality of life for patients.
Author contributions
FM-G, FA-H, NA, and AC were responsible for the initial conception, project, and writing of this manuscript. FM-G, FA-H, MB, FS, CC, JM, and IF collected, analyzed, and reviewed the data. MB, FS, and FM-G designed the illustrations. FM-G, NA, FA-H, AT, AM, AT-C, and AC supervised the project development, interpreted the data, and reviewed this manuscript. All authors read, discussed the general outline of the article together and approved the final manuscript.
Funding
This work was funded by Fundação de Amparo à Pesquisa do Estado do Amazonas (FAPEAM) (Pró-Estado Program [#002/2008, #007/2018 and #005/2019], and POSGRAD Program [#008/2021 and #005/2022]), Conselho Nacional de Desenvolvimento Científico e Tecnológico (CNPq), Coordenação de Aperfeiçoamento de Pessoal de Nível Superior (CAPES) (PROCAD-Amazônia 2018 Program-#88881.200581/2018-01). FM-G, NA, FA-H, MB, and FS have fellowships from FAPEAM, CAPES and CNPq (PhD and MSc students). JM and IF have fellowships from FAPEAM (Scientific Initiation students). AT-C and AM are level 1 and 2 research fellows from CNPq, respectively. AT-C and AC is research fellow from FAPEAM (PECTI-AM program #004/2020 and PRODOC Program #003/2022). The funders had no role in study design and decision to publish, or preparation of the manuscript.
Acknowledgments
We would like to thank our collaborators at the HEMOAM Foundation, especially the Amazon InterScience and Leukemia Immunology Research Group; the researchers of the Post-graduate Program in Basic and Applied Immunology (UFAM) and of the Post-graduate Program in Hematology Sciences (UEA); as well as our external collaborators from the Integrated Research Group on Biomarkers (FIOCRUZ-Minas) for their critical discussions and insightful and encouraging ideas.
Conflict of interest
The authors declare that the research was conducted in the absence of any commercial or financial relationships that could be construed as a potential conflict of interest.
Publisher’s note
All claims expressed in this article are solely those of the authors and do not necessarily represent those of their affiliated organizations, or those of the publisher, the editors and the reviewers. Any product that may be evaluated in this article, or claim that may be made by its manufacturer, is not guaranteed or endorsed by the publisher.
References
1. Ehsanpour A, Saki N, Bagheri M, Behzad MM, Abroun S. The expression of microvesicles in leukemia: Prognostic approaches. Cell J (2018) 21:115–23. doi: 10.22074/cellj.2019.5847
2. Van Etten RA. Mechanisms of transformation by the BCR-ABL oncogene: New perspectives in the post-imatinib era. Leuk Res (2004) 28:21–8. doi: 10.1016/j.leukres.2003.10.005
3. Van Etten RA. Aberrant cytokine signaling in leukemia. Oncogene (2007) 26:6738–49. doi: 10.1038/sj.onc.1210758
4. Khwaja A, Bjorkholm M, Gale RE, Levine RL, Jordan CT, Ehninger G, et al. Acute myeloid leukaemia. Nat Rev Dis Prim (2016) 2:16010. doi: 10.1038/nrdp.2016.10
5. Houshmand M, Simonetti G, Circosta P, Gaidano V, Cignetti A, Martinelli G, et al. Chronic myeloid leukemia stem cells. Leukemia (2019) 33:1543–56. doi: 10.1038/s41375-019-0490-0
6. Bosch F, Dalla-Favera R. Chronic lymphocytic leukaemia: from genetics to treatment. Nat Rev Clin Oncol (2019) 16:684–701. doi: 10.1038/s41571-019-0239-8
7. Terwilliger T, Abdul-Hay M. Acute lymphoblastic leukemia: A comprehensive review and 2017 update. Blood Cancer J (2017) 7:e577–7. doi: 10.1038/bcj.2017.53
8. Konopleva MY, Jordan CT. Leukemia stem cells and microenvironment: Biology and therapeutic targeting. J Clin Oncol (2011) 29:591–9. doi: 10.1200/JCO.2010.31.0904
9. Tabe Y, Konopleva M. Advances in understanding the leukaemia microenvironment. Br J Haematol (2014) 164:767–78. doi: 10.1111/bjh.12725
10. Witkowski MT, Kousteni S, Aifantis I. Mapping and targeting of the leukemic microenvironment. J Exp Med (2020) 217:e20190589. doi: 10.1084/jem.20190589
11. Höpken UE, Rehm A. Targeting the tumor microenvironment of leukemia and lymphoma. Trends Cancer (2019) 5:351–64. doi: 10.1016/j.trecan.2019.05.001
12. Burger JA, Ghia P, Rosenwald A, Caligaris-Cappio F. The microenvironment in mature b-cell malignancies: A target for new treatment strategies. Blood (2009) 114:3367–75. doi: 10.1182/blood-2009-06-225326
13. Morrison SJ, Spradling AC. Stem cells and niches: Mechanisms that promote stem cell maintenance throughout life. Cell (2008) 132:598–611. doi: 10.1016/j.cell.2008.01.038
14. Awad RM, De Vlaeminck Y, Maebe J, Goyvaerts C, Breckpot K. Turn back the TIMe: Targeting tumor infiltrating myeloid cells to revert cancer progression. Front Immunol (2018) 9:1977. doi: 10.3389/fimmu.2018.01977
15. Engblom C, Pfirschke C, Pittet MJ. The role of myeloid cells in cancer therapies. Nat Rev Cancer (2016) 16:447–62. doi: 10.1038/nrc.2016.54
16. Haas L, Obenauf AC. Allies or enemies–the multifaceted role of myeloid cells in the tumor microenvironment. Front Immunol (2019) 10:2746. doi: 10.3389/fimmu.2019.02746
17. Hinshaw DC, Shevde LA. The tumor microenvironment innately modulates cancer progression. Cancer Res (2019) 79:4557–66. doi: 10.1158/0008-5472.CAN-18-3962
18. Brandau S, Dumitru CA, Lang S. Protumor and antitumor functions of neutrophil granulocytes. Semin Immunopathol (2013) 35:163–76. doi: 10.1007/s00281-012-0344-6
19. Tamura R, Tanaka T, Yamamoto Y, Akasaki Y, Sasaki H. Dual role of macrophage in tumor immunity. Immunotherapy (2018) 10:899–909. doi: 10.2217/imt-2018-0006
20. Jackaman C, Tomay F, Duong L, Abdol Razak NB, Pixley FJ, Metharom P, et al. Aging and cancer: The role of macrophages and neutrophils. Ageing Res Rev (2017) 36:105–16. doi: 10.1016/j.arr.2017.03.008
21. Zhang Y, Lee C, Geng S, Li L. Enhanced tumor immune surveillance through neutrophil reprogramming due to tollip deficiency. JCI Insight (2019) 4:e122939. doi: 10.1172/jci.insight.122939
22. Kashfi K, Kannikal J, Nath N. Macrophage reprogramming and cancer therapeutics: Role of iNOS-derived NO. Cells (2021) 10:3194. doi: 10.3390/cells10113194
23. Borregaard N. Neutrophils, from marrow to microbes. Immunity (2010) 33:657–70. doi: 10.1016/j.immuni.2010.11.011
24. Kolaczkowska E, Kubes P. Neutrophil recruitment and function in health and inflammation. Nat Rev Immunol (2013) 13:159–75. doi: 10.1038/nri3399
25. Nauseef WM, Borregaard N. Neutrophils at work. Nat Immunol (2014) 15:602–11. doi: 10.1038/ni.2921
26. Fridlender ZG, Albelda SM. Tumor-associated neutrophils: Friend or foe? Carcinogenesis (2012) 33:949–55. doi: 10.1093/carcin/bgs123
27. Mantovani A, Allavena P, Sica A, Balkwill F. Cancer-related inflammation. Nature (2008) 454:436–44. doi: 10.1038/nature07205
28. Shaul ME, Fridlender ZG. Cancer-related circulating and tumor-associated neutrophils – subtypes, sources and function. FEBS J (2018) 285:4316–42. doi: 10.1111/febs.14524
29. Shaul ME, Fridlender ZG. The dual role of neutrophils in cancer. Semin Immunol (2022), 57:101582. doi: 10.1016/j.smim.2021.101582
30. Coffelt SB, Wellenstein MD, De Visser KE. Neutrophils in cancer: Neutral no more. Nat Rev Cancer (2016) 16:431–46. doi: 10.1038/nrc.2016.52
31. Rosales C. Neutrophil: A cell with many roles in inflammation or several cell types? Front Physiol (2018) 9:113. doi: 10.3389/fphys.2018.00113
32. Eruslanov EB, Bhojnagarwala PS, Quatromoni JG, Stephen TL, Ranganathan A, Deshpande C, et al. Tumor-associated neutrophils stimulate T cell responses in early-stage human lung cancer. J Clin Invest (2014) 124:5466–80. doi: 10.1172/JCI77053
33. Fridlender ZG, Sun J, Kim S, Kapoor V, Cheng G, Ling L, et al. Polarization of tumor-associated neutrophil phenotype by TGF-β: “N1” versus “N2” TAN. Cancer Cell (2009) 16:183–94. doi: 10.1016/j.ccr.2009.06.017
34. Mishalian I, Bayuh R, Levy L, Zolotarov L, Michaeli J, Fridlender ZG. Tumor-associated neutrophils (TAN) develop pro-tumorigenic properties during tumor progression. Cancer Immunol Immunother (2013) 62:1745–56. doi: 10.1007/s00262-013-1476-9
35. Qian X, Chen H, Wu X, Hu L, Huang Q, Jin Y. Interleukin-17 acts as double-edged sword in anti-tumor immunity and tumorigenesis. Cytokine (2017) 89:34–44. doi: 10.1016/j.cyto.2015.09.011
36. Elliott LA, Doherty GA, Sheahan K, Ryan EJ. Human tumor-infiltrating myeloid cells: Phenotypic and functional diversity. Front Immunol (2017) 8:86/BIBTEX. doi: 10.3389/FIMMU.2017.00086/BIBTEX
37. Wu S, Awaji S. Tumor-associated neutrophils in cancer: Going pro. Cancers (Basel) (2019) 11:564. doi: 10.3390/cancers11040564
38. Zou J-M, Qin J, Li Y-C, Wang Y, Li D, Shu Y, et al. IL-35 induces N2 phenotype of neutrophils to promote tumor growth. Oncotarget (2017) 8:33501–14. doi: 10.18632/oncotarget.16819
39. Liu CY, Wang YM, Wang CL, Feng PH, Ko HW, Liu YH, et al. Population alterations of l-arginase- and inducible nitric oxide synthase-expressed CD11b+/CD14-/CD15+/CD33 + myeloid-derived suppressor cells and CD8+ T lymphocytes in patients with advanced-stage non-small cell lung cancer. J Cancer Res Clin Oncol (2010) 136:35–45. doi: 10.1007/s00432-009-0634-0
40. Mishalian I, Bayuh R, Eruslanov E, Michaeli J, Levy L, Zolotarov L, et al. Neutrophils recruit regulatory T-cells into tumors via secretion of CCL17 - a new mechanism of impaired antitumor immunity. Int J Cancer (2014) 135:1178–86. doi: 10.1002/ijc.28770
41. Sconocchia G, Zlobec I, Lugli A, Calabrese D, Iezzi G, Karamitopoulou E, et al. Tumor infiltration by FcIγRIII (CD16)+ myeloid cells is associated with improved survival in patients with colorectal carcinoma. Int J Cancer (2011) 128:2663–72. doi: 10.1002/ijc.25609
42. Jaillon S, Ponzetta A, Di Mitri D, Santoni A, Bonecchi R, Mantovani A. Neutrophil diversity and plasticity in tumour progression and therapy. Nat Rev Cancer (2020) 20:485–503. doi: 10.1038/s41568-020-0281-y
43. Galdiero MR, Varricchi G, Loffredo S, Mantovani A, Marone G. Roles of neutrophils in cancer growth and progression. J Leukoc Biol (2018) 103:457–64. doi: 10.1002/JLB.3MR0717-292R
44. Sionov RV, Fridlender ZG, Granot Z. The multifaceted roles neutrophils play in the tumor microenvironment. Cancer Microenviron (2015) 8:125–58. doi: 10.1007/s12307-014-0147-5
45. Thiam HR, Wong SL, Wagner DD, Waterman CM. Cellular mechanisms of NETosis. Annu Rev Cell Dev Biol (2020) 36:191–218. doi: 10.1146/annurev-cellbio-020520-111016
46. Vorobjeva NV, Chernyak BV. NETosis: Molecular mechanisms, role in physiology and pathology. Biochem (2020) 85:1178–90. doi: 10.1134/S0006297920100065
47. Hanahan D, Folkman J. Patterns and emerging mechanisms of the angiogenic switch during tumorigenesis. Cell (1996) 86:353–64. doi: 10.1016/S0092-8674(00)80108-7
48. Lonardi S, Missale F, Calza S, Bugatti M, Vescovi R, Debora B, et al. Tumor-associated neutrophils (TANs) in human carcinoma-draining lymph nodes: A novel TAN compartment. Clin Transl Immunol (2021) 10:1–20. doi: 10.1002/cti2.1252
49. López-Otín C, Bond JS. Proteases: Multifunctional enzymes in life and disease. J Biol Chem (2008) 283:30433–7. doi: 10.1074/jbc.R800035200
50. Mohamed MM, Sloane BF. Cysteine cathepsins: Multifunctional enzymes in cancer. Nat Rev Cancer (2006) 6:764–75. doi: 10.1038/nrc1949
51. Andzinski L, Wu CF, Lienenklaus S, Kröger A, Weiss S, Jablonska J. Delayed apoptosis of tumor associated neutrophils in the absence of endogenous IFN-β. Int J Cancer (2015) 136:572–83. doi: 10.1002/ijc.28957
52. Zhang J, Qiao X, Shi H, Han X, Liu W, Tian X, et al. Circulating tumor-associated neutrophils (cTAN) contribute to circulating tumor cell survival by suppressing peripheral leukocyte activation. Tumor Biol (2016) 37:5397–404. doi: 10.1007/s13277-015-4349-3
53. Governa V, Trella E, Mele V, Tornillo L, Amicarella F, Cremonesi E, et al. The interplay between neutrophils and CD8+ T cells improves survival in human colorectal cancer. Clin Cancer Res (2017) 23:3847–58. doi: 10.1158/1078-0432.CCR-16-2047
54. Hao Y, Hu P, Zhang J. Genomic analysis of the prognostic effect of tumor-associated neutrophil-related genes across 15 solid cancer types: an immune perspective. Ann Transl Med (2020) 8:1507–7. doi: 10.21037/atm-20-6629
55. Jensen HK, Donskov F, Marcussen N, Nordsmark M, Lundbeck F, Von Der Maase H. Presence of intratumoral neutrophils is an independent prognostic factor in localized renal cell carcinoma. J Clin Oncol (2009) 27:4709–17. doi: 10.1200/JCO.2008.18.9498
56. Lecot P, Sarabi M, Pereira Abrantes M, Mussard J, Koenderman L, Caux C, et al. Neutrophil heterogeneity in cancer: From biology to therapies. Front Immunol (2019) 10:2155. doi: 10.3389/fimmu.2019.02155
57. Reid MD, Basturk O, Thirabanjasak D, Hruban RH, Klimstra DS, Bagci P, et al. Tumor-infiltrating neutrophils in pancreatic neoplasia. Mod Pathol (2011) 24:1612–9. doi: 10.1038/modpathol.2011.113
58. Podaza E, Sabbione F, Risnik D, Borge M, Almejún MB, Colado A, et al. Neutrophils from chronic lymphocytic leukemia patients exhibit an increased capacity to release extracellular traps (NETs). Cancer Immunol Immunother (2017) 66:77–89. doi: 10.1007/s00262-016-1921-7
59. Podaza E, Risnik D, Colado A, Elías E, Belén M, Grecco HF, et al. As células de leucemia linfocítica crônica aumentam a sobrevivência dos neutrófilos e promovem sua diferenciação no subconjunto imunossupressor CD16 CD62L. Int J Cancer (2018) 144(5):1128–34. doi: 10.1002/ijc.31762
60. Hanna BS, Öztürk S, Seiffert M. Beyond bystanders: Myeloid cells in chronic lymphocytic leukemia. Mol Immunol (2019) 110:77–87. doi: 10.1016/j.molimm.2017.11.014
61. Itala M, Vainio ORK. Functional abnorma lities in granulocytes predict susceptibility to b acterial in chronic lymphocy tic le ukaernia. Eur J Haematol (1996) 57:46–53. doi: 10.1111/j.1600-0609.1996.tb00489.x
62. Tanaka F, Goto H, Yokosuka T, Yanagimachi M, Kajiwara R, Naruto T, et al. Suppressed neutrophil function in children with acute lymphoblastic leukemia. Int J Hematol (2009) 90:311–7. doi: 10.1007/s12185-009-0412-4
63. Kontoyiannis DP, Georgiadou SP, Wierda WG, Wright S, Albert ND, Ferrajoli A, et al. Impaired bactericidal but not fungicidal activity of polymorphonuclear neutrophils in patients with chronic lymphocytic leukemia. Leuk Lymphoma (2013) 54:1730–3. doi: 10.3109/10428194.2012.750723
64. Manukyan G, Papajik T, Gajdos P, Mikulkova Z, Urbanova R, Gabcova G, et al. Neutrophils in chronic lymphocytic leukemia are permanently activated and have functional defects. Oncotarget (2017) 8:84889–901. doi: 10.18632/oncotarget.20031
65. Gätjen M, Brand F, Grau M, Gerlach K, Kettritz R, Westermann J, et al. Splenic marginal zone granulocytes acquire an accentuated neutrophil b cellhelper phenotype in chronic lymphocytic leukemia. Cancer Res (2016) 76:5253–65. doi: 10.1158/0008-5472.CAN-15-3486
66. Wachowska M, Wojciechowska A, Muchowicz A. The role of neutrophils in the pathogenesis of chronic lymphocytic leukemia. (2022) 23(1):365. doi: 10.3390/ijms23010365
67. Lavin Y, Mortha A, Rahman A, Merad M. Regulation of macrophage development and function in peripheral tissues. Nat Rev Immunol (2015) 15:731–44. doi: 10.1038/nri3920
68. Hirayama D, Iida T, Nakase H. The phagocytic function of macrophage-enforcing innate immunity and tissue homeostasis. Int J Mol Sci (2018) 1998. doi: 10.3390/ijms19010092
69. Mosser DM, Edwards JP. Exploring the full spectrum of macrophage activation. Nat Rev Immunol (2008) 8:958–69. doi: 10.1038/nri2448
70. Duque GA, Descoteaux A. Macrophage cytokines: Involvement in immunity and infectious diseases. Front Immunol (2014) 5:491. doi: 10.3389/fimmu.2014.00491
71. Jayasingam SD, Citartan M, Thang TH, Mat Zin AA, Ang KC, Ch’ng ES. Evaluating the polarization of tumor-associated macrophages into M1 and M2 phenotypes in human cancer tissue: Technicalities and challenges in routine clinical practice. Front Oncol (2020) 9:1512. doi: 10.3389/fonc.2019.01512
72. Roszer T. Understanding the mysterious M2 macrophage through activation markers and effector mechanisms. Mediators Inflammation (2015) 2015:816460. doi: 10.1155/2015/816460
73. Gentles AJ, Newman AM, Liu CL, Bratman SV, Feng W, Kim D, et al. The prognostic landscape of genes and infiltrating immune cells across human cancers. Nat Med (2015) 21:938–45. doi: 10.1038/nm.3909
74. Hiam-Galvez KJ, Allen BM, Spitzer MH. Systemic immunity in cancer. Nat Rev Cancer (2021), 21:1–15. doi: 10.1038/s41568-021-00347-z
75. Gabrilovich DI, Ostrand-Rosenberg S, Bronte V. Coordinated regulation of myeloid cells by tumours. Nat Rev Immunol (2012) 12:253–68. doi: 10.1038/nri3175
76. DeNardo DG, Ruffell B. Macrophages as regulators of tumour immunity and immunotherapy. Nat Rev Immunol (2019) 19:369–82. doi: 10.1038/s41577-019-0127-6
77. Mantovani A, Allavena P, Marchesi F, Garlanda C. Macrophages as tools and targets in cancer therapy. Nat Rev Drug Discovery (2022) 21:799–820. doi: 10.1038/s41573-022-00520-5
78. Bardi GT, Smith MA, Hood JL. Melanoma exosomes promote mixed M1 and M2 macrophage polarization. Cytokine (2018) 105:63–72. doi: 10.1016/j.cyto.2018.02.002
79. Wan S, Zhao E, Kryczek I, Vatan L, Sadovskaya A, Ludema G, et al. Tumor-associated macrophages produce interleukin 6 and signal via STAT3 to promote expansion of human hepatocellular carcinoma stem cells. Gastroenterology (2014) 147:1393–404. doi: 10.1053/j.gastro.2014.08.039
80. Radharani NNV, Yadav AS, Nimma R, Kumar TVS, Bulbule A, Chanukuppa V, et al. Tumor-associated macrophage derived IL-6 enriches cancer stem cell population and promotes breast tumor progression via stat-3 pathway. Cancer Cell Int (2022) 22:122. doi: 10.1186/s12935-022-02527-9
81. Wu K, Lin K, Li X, Yuan X, Xu P, Ni P, et al. Redefining tumor-associated macrophage subpopulations and functions in the tumor microenvironment. Front Immunol (2020) 11:1731. doi: 10.3389/fimmu.2020.01731
82. Chong BF, Tseng L, Hosler GA, Teske NM, Zhang S, Karp DR, et al. Mohan c. a subset of CD163+ macrophages displays mixed polarizations in discoid lupus skin. Arthritis Res Ther (2015) 17:324. doi: 10.1186/s13075-015-0839-3
83. Yang M, McKay D, Pollard JW, Lewis CE. Diverse functions of macrophages in different tumor microenvironments. Cancer Res (2018) 78:5492–503. doi: 10.1158/0008-5472.CAN-18-1367
84. Wang L, Zheng G. Macrophages in leukemia microenvironment. Blood Sci (2019) 1:29–33. doi: 10.1097/BS9.0000000000000014
85. Petty AJ, Yang Y. Tumor-associated macrophages in hematologic malignancies: New insights and targeted therapies. Cells (2019) 8:1526. doi: 10.3390/cells8121526
86. Li Y, You MJ, Yang Y, Hu D, Tian C. The role of tumor-associated macrophages in leukemia. Acta Haematol (2020) 143:112–7. doi: 10.1159/000500315
87. Cencini E, Fabbri A, Sicuranza A, Gozzetti A, Bocchia M. The role of tumor-associated macrophages in hematologic malignancies. Cancers (Basel) (2021) 13:3597. doi: 10.3390/cancers13143597
88. Song JX, Wen Y, Li RW, Dong T, Tang YF, Zhang JJ, et al. Phenotypic characterization of macrophages in the BMB sample of human acute leukemia. Ann Hematol (2020) 99:539–47. doi: 10.1007/s00277-020-03912-y
89. Fiorcari S, Maffei R, Atene CG, Potenza L, Luppi M, Marasca R. Nurse-like cells and chronic lymphocytic leukemia b cells: A mutualistic crosstalk inside tissue microenvironments. Cells (2021) 10:1–12. doi: 10.3390/cells10020217
90. Boissard F, Laurent C, Ramsay AG, Quillet-Mary A, Fournié JJ, Poupot M, et al. Nurse-like cells impact on disease progression in chronic lymphocytic leukemia. Blood Cancer J (2016) 6:e381. doi: 10.1038/bcj.2015.108
91. Miari KE, Guzman ML, Wheadon H, Williams MTS. Macrophages in acute myeloid leukaemia: Significant players in therapy resistance and patient outcomes. Front Cell Dev Biol (2021) 9:692800. doi: 10.3389/fcell.2021.692800
92. Xu ZJ, Gu Y, Wang CZ, Jin Y, Wen XM, Ma JC, et al. The M2 macrophage marker CD206: A novel prognostic indicator for acute myeloid leukemia. Oncoimmunology (2020) 9:e1683347. doi: 10.1080/2162402X.2019.1683347
93. Guo R, Lü M, Cao F, Wu G, Gao F, Pang H, et al. Single-cell map of diverse immune phenotypes in the acute myeloid leukemia microenvironment. biomark Res (2021) 9:1–16. doi: 10.1186/s40364-021-00265-0
94. Zhou X, Xu N, Sun X, Lin T, Fan Z, Cao R, et al. Tumor-associated macrophages maybe associated with acute myeloid leukemia survival and prognosis. Blood (2017) 130:5090–0. doi: 10.1182/blood.V130.Suppl_1.5090.5090
95. Al-Matary YS, Botezatu L, Opalka B, Hönes JM, Lams RF, Thivakaran A, et al. Acute myeloid leukemia cells polarize macrophages towards a leukemia supporting state in a growth factor independence 1 dependent manner. Haematologica (2016) 101:1216–27. doi: 10.3324/haematol.2016.143180
96. Hohtari H, Brück O, Blom S, Turkki R, Sinisalo M, Kovanen PE, et al. Immune cell constitution in bone marrow microenvironment predicts outcome in adult ALL. Leukemia (2019) 33:1570–82. doi: 10.1038/s41375-018-0360-1
97. Song JX, Dian ZJ, Wen Y, Mei F, Li RW, Sa YL. Assessment of the number and phenotype of macrophages in the human BMB samples of CML. BioMed Res Int (2016) 2016:8086398. doi: 10.1155/2016/8086398
98. Komohara Y, Niino D, Saito Y, Ohnishi K, Horlad H, Ohshima K, et al. Clinical significance of CD163+ tumor-associated macrophages in patients with adult T-cell leukemia/lymphoma. Cancer Sci (2013) 104:945–51. doi: 10.1111/cas.12167
99. Chen S-Y, Yang X, Feng W-L, Liao J-F, Wang L-N, Feng L, et al. Organ-specific microenvironment modifies diverse functional and phenotypic characteristics of leukemia-associated macrophages in mouse T cell acute lymphoblastic leukemia. J Immunol (2015) 194:2919–29. doi: 10.4049/jimmunol.1400451
100. Burger JA, Quiroga MP, Hartmann E, Bürkle A, Wierda WG, Keating MJ, et al. High-level expression of the T-cell chemokines CCL3 and CCL4 by chronic lymphocytic leukemia b cells in nurselike cell cocultures and after BCR stimulation. Blood (2009) 113:3050–8. doi: 10.1182/blood-2008-07-170415
101. Zucchetto A, Benedetti D, Tripodo C, Bomben R, Bo MD, Marconi D, et al. CD38/CD31, the CCL3 and CCL4 chemokines, and cd49d/vascular cell adhesion molecule-1 are interchained bysequential events sustaining chronic lymphocytic leukemia cell survival. Cancer Res (2009) 69:4001–9. doi: 10.1158/0008-5472.CAN-08-4173
102. Beider K, Bitner H, Leiba M, Gutwein O, Koren-Michowitz M, Ostrovsky O, et al. Multiple myeloma cells recruit tumor-supportive macrophages through the CXCR4/CXCL12 axis and promote their polarization toward the M2 phenotype. Oncotarget (2014) 5:11283–96. doi: 10.18632/oncotarget.2207
103. Tian Y, Matsui S, Touma M, Wu Q, Sugimoto K. MicroRNA-342 inhibits tumor growth via targeting chemokine CXCL12 involved in macrophages recruitment/activation. Genes to Cells (2018) 23:1009–22. doi: 10.1111/gtc.12650
104. Dander E, Fallati A, Gulić T, Pagni F, Gaspari S, Silvestri D, et al. Monocyte–macrophage polarization and recruitment pathways in the tumour microenvironment of b-cell acute lymphoblastic leukaemia. Br J Haematol (2021) 193:1157–71. doi: 10.1111/bjh.17330
105. Gandhi V, Balakrishnan K. CCL2 in chronic lymphocytic leukemia: A macro in microenvironment? Leuk Lymphoma (2012) 53:1849–50. doi: 10.3109/10428194.2012.688966
106. Schulz A, Toedt G, Zenz T, Stilgenbauer S, Lichter P, Seiffert M. Inflammatory cytokines and signaling pathways are associated with survival of primary chronic lymphocytic leukemia cells in vitro: A dominant role of CCL2. Haematologica (2011) 96:408–16. doi: 10.3324/haematol.2010.031377
107. Magalhães-Gama F, Kerr MWA, de Araújo ND, Ibiapina HNS, Neves JCF, Hanna FSA, et al. Imbalance of chemokines and cytokines in the bone marrow microenvironment of children with b-cell acute lymphoblastic leukemia. J Oncol (2021) 2021:1–9. doi: 10.1155/2021/5530650
108. De Vasconcellos JF, Laranjeira ABA, Zanchin NIT, Otubo R, Vaz TH, Cardoso AA, et al. Increased CCL2 and IL-8 in the bone marrow microenvironment in acute lymphoblastic leukemia. Pediatr Blood Cancer (2011) 56:568–77. doi: 10.1002/PBC.22941
109. Rigo A, Gottardi M, Zamò A, Mauri P, Bonifacio M, Krampera M, et al. Macrophages may promote cancer growth via a GM-CSF/HB-EGF paracrine loop that is enhanced by CXCL12. Mol Cancer (2010) 9:1–13. doi: 10.1186/1476-4598-9-273
110. Sánchez-Martín L, Estecha A, Samaniego R, Sánchez-Ramón S, Vega MÁ, Sánchez-Mateos P. The chemokine CXCL12 regulates monocyte-macrophage differentiation and RUNX3 expression. Blood (2011) 117:88–97. doi: 10.1182/blood-2009-12-258186
111. Kerr MWA, Magalhães-Gama F, Ibiapina HNS, Hanna FSA, Xabregas LA, Alves EB, et al. Bone marrow soluble immunological mediators as clinical prognosis biomarkers in b-cell acute lymphoblastic leukemia patients undergoing induction therapy. Front Oncol (2021) 11:696032. doi: 10.3389/fonc.2021.696032
112. Whiteley AE, Price TT, Cantelli G, Sipkins DA. Leukaemia: A model metastatic disease. Nat Rev Cancer (2021) 21:461–75. doi: 10.1038/s41568-021-00355-z
113. Burger JA, Tsukada N, Burger M, Zvaifler NJ, Dell’Aquila M, Kipps TJ. Blood-derived nurse-like cells protect chronic lymphocytic leukemia b cells from spontaneous apoptosis through stromal cell-derived factor-1. Blood (2000) 96:2655–63. doi: 10.1182/blood.v96.8.2655
114. Tsukada N, Burger JA, Zvaifler NJ, Kipps TJ. Distinctive features of “nurselike” cells that differentiate in the context of chronic lymphocytic leukemia. Blood (2002) 99:1030–7. doi: 10.1182/blood.V99.3.1030
115. Zucchetto A, Tripodo C, Benedetti D, Deaglio S, Gaidano G, Del Poeta G, et al. Monocytes/macrophages but not T lymphocytes are the major targets of the CCL3/CCL4 chemokines produced by CD38+CD49d+ chronic lymphocytic leukaemia cells: Correspondence. Br J Haematol (2010) 150:111–2. doi: 10.1111/j.1365-2141.2010.08152.x
116. Boissard F, Tosolini M, Ligat L, Quillet-Mary A, Lopez F, Fournié J-J, et al. Nurse-like cells promote CLL survival through LFA-3/CD2 interactions. Oncotarget (2017) 8:52225–36. doi: 10.18632/oncotarget.13660
117. Hanna BS, McClanahan F, Yazdanparast H, Zaborsky N, Kalter V, Rößner PM, et al. Depletion of CLL-associated patrolling monocytes and macrophages controls disease development and repairs immune dysfunction in vivo. Leukemia (2016) 30:570–9. doi: 10.1038/leu.2015.305
118. Yang X, Feng W, Wang R, Yang F, Wang L, Chen S, et al. Repolarizing heterogeneous leukemia-associated macrophages with more M1 characteristics eliminates their pro-leukemic effects. Oncoimmunology (2018) 7:e1412910. doi: 10.1080/2162402X.2017.1412910
119. Amrein MA, Bührer ED, Forster S, Isringhausen S, Schürch CM, Bhate SS, et al. Splenic CD24low red pulp macrophages provide an alternate niche for chronic myeloid leukemia stem cells. Blood (2019) 134:1634–4. doi: 10.1182/blood-2019-126593
120. Yang X, Feng W, Wang R, Yang F, Wang L, Chen S, et al. Hepatic leukemia-associated macrophages exhibit a pro-inflammatory phenotype in Notch1-induced acute T cell leukemia. Immunobiology (2018) 223:73–80. doi: 10.1016/j.imbio.2017.10.009
121. Yang F, Feng W, Wang H, Wang L, Liu X, Wang R, et al. Monocyte-derived leukemia-associated macrophages facilitate extramedullary distribution of t-cell acute lymphoblastic leukemia cells. Cancer Res (2020) 80:3677–91. doi: 10.1158/0008-5472.CAN-20-0034
122. Audrito V, Serra S, Brusa D, Mazzola F, Arruga F, Vaisitti T, et al. Extracellular nicotinamide phosphoribosyltransferase (NAMPT) promotes M2 macrophage polarization in chronic lymphocytic leukemia. Blood (2015) 125:111–23. doi: 10.1182/blood-2014-07-589069
123. Valencia J M, Fernández-Sevilla L, Fraile-Ramos A, Sacedón R, Jiménez E, Vicente A, et al. Acute lymphoblastic leukaemia cells impair dendritic cell and macrophage differentiation: Role of BMP4. Cells (2019) 8:722. doi: 10.3390/cells8070722
124. Chen S, Yang X, Feng W, Yang F, Wang R, Chen C, et al. Characterization of peritoneal leukemia-associated macrophages in Notch1-induced mouse T cell acute lymphoblastic leukemia. Mol Immunol (2017) 81:35–41. doi: 10.1016/j.molimm.2016.11.014
125. Bürkle A, Niedermeier M, Schmitt-Gräff A, Wierda WG, Keating MJ, Burger JA. Overexpression of the CXCR5 chemokine receptor, and its ligand, CXCL13 in b-cell chronic lymphocytic leukemia. Blood (2007) 110:3316–25. doi: 10.1182/blood-2007-05-089409
126. Dilillo DJ, Weinberg JB, Yoshizaki A, Horikawa M, Bryant JM, Iwata Y, et al. Chronic lymphocytic leukemia and regulatory b cells share IL-10 competence and immunosuppressive function. Leukemia (2013) 27:170–82. doi: 10.1038/leu.2012.165
127. Dai J, Su Y, Zhong S, Cong L, Liu B, Yang J, et al. Exosomes: Key players in cancer and potential therapeutic strategy. Signal Transduct Target Ther (2020) 5:145. doi: 10.1038/s41392-020-00261-0
128. van Niel G, D’Angelo G, Raposo G. Shedding light on the cell biology of extracellular vesicles. Nat Rev Mol Cell Biol (2018) 19:213–28. doi: 10.1038/nrm.2017.125
129. Jafarzadeh N, Safari Z, Pornour M, Amirizadeh N, Forouzandeh MM, Sadeghizadeh M. Alteration of cellular and immune-related properties of bone marrow mesenchymal stem cells and macrophages by K562 chronic myeloid leukemia cell derived exosomes. J Cell Physiol (2019) 234:3697–710. doi: 10.1002/jcp.27142
130. Haderk F, Schulz R, Iskar M, Cid LL, Worst T, Willmund KV, et al. Tumor-derived exosomes modulate PD-L1 expression in monocytes. Sci Immunol (2017) 2:eaah5509. doi: 10.1126/sciimmunol.aah5509
131. Philippidis P, Mason JC, Evans BJ, Nadra I, Taylor KM, Haskard DO, et al. Hemoglobin scavenger receptor CD163 mediates interleukin-10 release and heme oxygenase-1 synthesis: Antiinflammatory monocyte-macrophage responses In vitro, in resolving skin blisters In vivo, and after cardiopulmonary bypass surgery. Circ Res (2004) 94:119–26. doi: 10.1161/01.RES.0000109414.78907.F9
132. Alhakeem SS, McKenna MK, Gachuki BW, Rangnekar VR, Byrd JC, Muthusamy N, et al. The role of IL-10 in b-cell chronic lymphocytic leukemia cell survival. J Immunol (2016) 196:211. doi: 10.4049/jimmunol.196.Supp.211.17
133. Fitch B, Hermiston ML, Wiemels JL, Kogan SC. Mechanism of IL-10 protective effect in development of childhood b cell acute lymphoblastic leukemia. Blood (2016) 128:4075–5. doi: 10.1182/blood.v128.22.4075.4075
134. Nishio M, Endo T, Tsukada N, Ohata J, Kitada S, Reed JC, et al. Nurselike cells express BAFF and APRIL, which can promote survival of chronic lymphocytic leukemia cells via a paracrine pathway distinct from that of SDF-1α. Blood (2005) 106:1012–20. doi: 10.1182/blood-2004-03-0889
135. Craxton A, Magaletti D, Ryan EJ, Clark EA. Macrophage- and dendritic cell-dependent regulation of human b-cell proliferation requires the TNF family ligand BAFF. Blood (2003) 101:4464–71. doi: 10.1182/blood-2002-10-3123
136. McWilliams EM, Lucas CR, Chen T, Harrington BK, Wasmuth R, Campbell A, et al. Anti–BAFF-R antibody VAY-736 demonstrates promising preclinical activity in CLL and enhances effectiveness of ibrutinib. Blood Adv (2019) 3:447–60. doi: 10.1182/bloodadvances.2018025684
137. Bolkun L, Grubczak K, Schneider G, Zembko P, Radzikowska U, Singh P, et al. Involvement of BAFF and APRIL in resistance to apoptosis of acute myeloid leukemia. J Cancer (2016) 7:1979–83. doi: 10.7150/jca.15966
138. Deaglio S, Vaisitti T, Bergui L, Bonello L, Horenstein AL, Tamagnone L, et al. CD38 and CD100 lead a network of surface receptors relaying positive signals for b-CLL growth and survival. Blood (2005) 105:3042–50. doi: 10.1182/blood-2004-10-3873
139. Deaglio S, Aydin S, Grand MM, Vaisitti T, Bergui L, D’Arena G, et al. CD38/CD31 interactions activate genetic pathways leading to proliferation and migration in chronic lymphocytic leukemia cells. Mol Med (2010) 16:87–91. doi: 10.2119/molmed.2009.00146
140. Gautam S, Fatehchand K, Elavazhagan S, Reader BF, Ren L, Mo X, et al. Reprogramming nurse-like cells with interferon γ to interrupt chronic lymphocytic leukemia cell survival. J Biol Chem (2016) 291:14356–62. doi: 10.1074/jbc.M116.723551
141. Brück O, Blom S, Dufva O, Turkki R, Chheda H, Ribeiro A, et al. Immune cell contexture in the bone marrow tumor microenvironment impacts therapy response in CML. Leukemia (2018) 32:1643–56. doi: 10.1038/s41375-018-0175-0
142. Movahedi K, Guilliams M, Van den Bossche J, Van den Bergh R, Gysemans C, Beschin A, et al. Identification of discrete tumor-induced myeloid-derived suppressor cell subpopulations with distinct T cell–suppressive activity. Blood (2008) 111:4233–44. doi: 10.1182/blood-2007-07-099226
143. Mastio J, Condamine T, Dominguez G, Kossenkov AV, Donthireddy L, Veglia F, et al. Identification of monocyte-like precursors of granulocytes in cancer as a mechanism for accumulation of PMN-MDSCs. J Exp Med (2019) 216:2150–69. doi: 10.1084/jem.20181952
144. Veglia F, Sanseviero E, Gabrilovich DI. Myeloid-derived suppressor cells in the era of increasing myeloid cell diversity. Nat Rev Immunol (2021) 21:485–98. doi: 10.1038/s41577-020-00490-y
145. Bronte V, Brandau S, Chen S-H, Colombo MP, Frey AB, Greten TF, et al. Recommendations for myeloid-derived suppressor cell nomenclature and characterization standards. Nat Commun (2016) 7:12150. doi: 10.1038/ncomms12150
146. Kim HR, Park SM, Seo SU, Jung I, Yoon HI, Gabrilovich DI, et al. The ratio of peripheral regulatory T cells to lox-1 1 polymorphonuclear myeloid-derived suppressor cells predicts the early response to anti–PD-1 therapy in patients with non–small cell lung cancer. Am J Respir Crit Care Med (2019) 199:243–6. doi: 10.1164/rccm.201808-1502LE
147. Kumar V, Donthireddy L, Marvel D, Condamine T, Wang F, Lavilla-Alonso S, et al. Cancer-associated fibroblasts neutralize the anti-tumor effect of CSF1 receptor blockade by inducing PMN-MDSC infiltration of tumors. Cancer Cell (2017) 32:654–668.e5. doi: 10.1016/j.ccell.2017.10.005
148. Si Y, Merz SF, Jansen P, Wang B, Bruderek K, Altenhoff P, et al. Multidimensional imaging provides evidence for down-regulation of T cell effector function by MDSC in human cancer tissue. Sci Immunol (2019) 4:eaaw9159. doi: 10.1126/SCIIMMUNOL.AAW9159
149. Mengos AE, Gastineau DA, Gustafson MP. The CD14+HLA-DrlO/NEG monocyte: An immunosuppressive phenotype that restrains responses to cancer immunotherapy. Front Immunol (2019) 10:1147. doi: 10.3389/fimmu.2019.01147
150. Lv M, Wang K, Huang XJ. Myeloid-derived suppressor cells in hematological malignancies: Friends or foes. J Hematol Oncol (2019) 12:1–12. doi: 10.1186/s13045-019-0797-3
151. De Lerma Barbaro A, Palano MT, Cucchiara M, Gallazzi M, Mortara L, Bruno A. Metabolic rewiring in the tumor microenvironment to support immunotherapy: A focus on neutrophils, polymorphonuclear myeloid-derived suppressor cells and natural killer cells. Vaccines (2021) 9:1178. doi: 10.3390/vaccines9101178
152. Zhou J, Nefedova Y, Lei A, Gabrilovich D. Neutrophils and PMN-MDSC: Their biological role and interaction with stromal cells. Semin Immunol (2018) 35:19–28. doi: 10.1016/j.smim.2017.12.004
153. Condamine T, Gabrilovich DI, Dominguez GA, Youn J-I, Kossenkov AV, Mony S, et al. Lectin-type oxidized LDL receptor-1 distinguishes population of human polymorphonuclear myeloid-derived suppressor cells in cancer patients. Science Immunology (2016), 1:1–32. doi: 10.1126/sciimmunol.aaf8943.Lectin-type
154. Condamine T, Mastio J, Gabrilovich DI. Transcriptional regulation of myeloid-derived suppressor cells. J Leukoc Biol (2015) 98:913–22. doi: 10.1189/jlb.4ri0515-204r
155. Ostrand-Rosenberg S, Beury DW, Parker KH, Horn LA. Survival of the fittest: how myeloid-derived suppressor cells survive in the inhospitable tumor microenvironment. Cancer Immunol Immunother (2020) 69:215–21. doi: 10.1007/s00262-019-02388-8
156. Wang W, Xia X, Mao L, Wang S. The CCAAT/Enhancer-binding protein family: Its roles in MDSC expansion and function. Front Immunol (2019) 10:1804. doi: 10.3389/fimmu.2019.01804
157. Huang B, Pan P-Y, Li Q, Sato AI, Levy DE, Bromberg J, et al. Gr-1 + CD115 + immature myeloid suppressor cells mediate the development of tumor-induced T regulatory cells and T-cell anergy in tumor-bearing host. Cancer Res (2006) 66:1123–31. doi: 10.1158/0008-5472.CAN-05-1299
158. Ostrand-Rosenberg S, Sinha P, Beury DW, Clements VK. Cross-talk between myeloid-derived suppressor cells (MDSC), macrophages, and dendritic cells enhances tumor-induced immune suppression. Semin Cancer Biol (2012) 22:275–81. doi: 10.1016/j.semcancer.2012.01.011
159. Sinha P, Clements VK, Bunt SK, Albelda SM, Ostrand-Rosenberg S. Cross-talk between myeloid-derived suppressor cells and macrophages subverts tumor immunity toward a type 2 response. J Immunol (2007) 179:977–83. doi: 10.4049/jimmunol.179.2.977
160. Filipazzi P, Huber V, Rivoltini L. Phenotype, function and clinical implications of myeloid-derived suppressor cells in cancer patients. Cancer Immunol Immunother (2012) 61:255–63. doi: 10.1007/s00262-011-1161-9
161. Wang J-C, Sun L. PD-1/PD-L1, MDSC pathways, and checkpoint inhibitor therapy in ph(-) myeloproliferative neoplasm: A review. Int J Mol Sci (2022) 23:5837. doi: 10.3390/ijms23105837
162. Wang L, Jia B, Claxton DF, Ehmann WC, Rybka WB, Mineishi S, et al. VISTA is highly expressed on MDSCs and mediates an inhibition of T cell response in patients with AML. Oncoimmunology (2018) 7:e1469594. doi: 10.1080/2162402X.2018.1469594
163. Hanahan D, Coussens LM. Accessories to the crime: Functions of cells recruited to the tumor microenvironment. Cancer Cell (2012) 21:309–22. doi: 10.1016/j.ccr.2012.02.022
164. Kiss M, Van Gassen S, Movahedi K, Saeys Y, Laoui D. Myeloid cell heterogeneity in cancer: not a single cell alike. Cell Immunol (2018) 330:188–201. doi: 10.1016/j.cellimm.2018.02.008
165. Umansky V, Sevko A, Gebhardt C, Utikal J. Myeloid-derived suppressor cells in malignant melanoma. JDDG - J Ger Soc Dermatol (2014) 12:1021–7. doi: 10.1111/ddg.12411
166. Li W, Wu K, Zhao E, Shi L, Li R, Zhang P, et al. HMGB1 recruits myeloid derived suppressor cells to promote peritoneal dissemination of colon cancer after resection. Biochem Biophys Res Commun (2013) 436:156–61. doi: 10.1016/j.bbrc.2013.04.109
167. Youn JI, Gabrilovich DI. The biology of myeloid-derived suppressor cells: The blessing and the curse of morphological and functional heterogeneity. Eur J Immunol (2010) 40:2969–75. doi: 10.1002/eji.201040895
168. Ma P, Beatty PL, McKolanis J, Brand R, Schoen RE, Finn OJ. Circulating myeloid derived suppressor cells (MDSC) that accumulate in premalignancy share phenotypic and functional characteristics with MDSC in cancer. Front Immunol (2019) 10:1401. doi: 10.3389/fimmu.2019.01401
169. Bizymi N, Bjelica S, Kittang AO, Mojsilovic S, Velegraki M, Pontikoglou C, et al. Myeloid-derived suppressor cells in hematologic diseases: Promising biomarkers and treatment targets. HemaSphere (2019) 3:e168. doi: 10.1097/HS9.0000000000000168
170. Salem ML, El-Shanshory MR, Abdou SH, Attia MS, Sobhy SM, Zidan MF, et al. Chemotherapy alters the increased numbers of myeloid-derived suppressor and regulatory T cells in children with acute lymphoblastic leukemia. Immunopharmacol Immunotoxicol (2018) 40:158–67. doi: 10.1080/08923973.2018.1424897
171. Liu Y, Chen Y, He Y, Wang J, Yang J, Zhong S, et al. Expansion and activation of granulocytic, myeloid-derived suppressor cells in childhood precursor b cell acute lymphoblastic leukemia. J Leukoc Biol (2017) 102:449–58. doi: 10.1189/jlb.5MA1116-453RR
172. Sun H, Li Y, Zhang ZF, Ju Y, Li L, Zhang BC, et al. Increase in myeloid-derived suppressor cells (MDSCs) associated with minimal residual disease (MRD) detection in adult acute myeloid leukemia. Int J Hematol (2015) 102:579–86. doi: 10.1007/s12185-015-1865-2
173. Wang H, Tao Q, Wang Z, Zhang Q, Xiao H, Zhou M, et al. Circulating monocytic myeloid-derived suppressor cells are elevated and associated with poor prognosis in acute myeloid leukemia. J Immunol Res (2020) 2020:7363084. doi: 10.1155/2020/7363084
174. Christiansson L, Söderlund S, Svensson E, Mustjoki S, Bengtsson M, Simonsson B, et al. Increased level of myeloid-derived suppressor cells, programmed death receptor ligand 1/Programmed death receptor 1, and soluble CD25 in sokal high risk chronic myeloid leukemia. PloS One (2013) 8:1–12. doi: 10.1371/journal.pone.0055818
175. Giallongo C, Parrinello N, Tibullo D, La Cava P, Romano A, Chiarenza A, et al. Myeloid derived suppressor cells (MDSCs) are increased and exert immunosuppressive activity together with polymorphonuclear leukocytes (PMNs) in chronic myeloid leukemia patients. PloS One (2014) 9:e101848. doi: 10.1371/journal.pone.0101848
176. Jitschin R, Braun M, Büttner M, Dettmer-Wilde K, Bricks J, Berger J, et al. CLL-cells induce IDOhi CD14+HLA-DRlo myeloid-derived suppressor cells that inhibit T-cell responses and promote TRegs. Blood (2014) 124:750–60. doi: 10.1182/blood-2013-12-546416
177. Kowalska W, Agnieszka B-J. Monocytic MDSC as a source of immunosuppressive cytokines in chronic lymphocytic leukemia (CLL) microenvironment. Folia Histochem Cytobiol (2020) 58:25–36. doi: 10.5603/FHC.a2020.0006
178. Xu H, Liu J, Shen N, Zhao Z, Cui J, Zhou S, et al. The interaction of tumor cells and myeloid-derived suppressor cells in chronic myelogenous leukemia. Leuk Lymphoma (2020) 61:128–37. doi: 10.1080/10428194.2019.1658098
179. Ahn A, Park CJ, Kim MS, Cho YU, Jang S, Bae MH, et al. Granulocytic and monocytic myeloid-derived suppressor cells are functionally and prognostically different in patients with chronic myeloid leukemia. Ann Lab Med (2021) 41:479–84. doi: 10.3343/ALM.2021.41.5.479
180. Zahran AM, Moeen SM, Thabet AF, Rayan A, Abdel-Rahim MH, Mohamed WMY, et al. Monocytic myeloid-derived suppressor cells in chronic lymphocytic leukemia patients: A single center experience. Leuk Lymphoma (2020) 61:1645–52. doi: 10.1080/10428194.2020.1728747
181. Zarobkiewicz M, Kowalska W, Chocholska S, Tomczak W, Szymańska A, Morawska I, et al. High m-MDSC percentage as a negative prognostic factor in chronic lymphocytic leukaemia. Cancers (Basel) (2020) 12:1–20. doi: 10.3390/cancers12092614
182. Ferrer G, Jung B, Chiu PY, Aslam R, Palacios F, Mazzarello AN, et al. Myeloid-derived suppressor cell subtypes differentially influence T-cell function, T-helper subset differentiation, and clinical course in CLL. Leukemia (2021) 35:3163–75. doi: 10.1038/s41375-021-01249-7
183. Hanahan D. Hallmarks of cancer: New dimensions. Cancer Discovery (2022) 12:31–46. doi: 10.1158/2159-8290.CD-21-1059
184. Hanahan D, Weinberg RA. Hallmarks of cancer: The next generation. Cell (2011) 144:646–74. doi: 10.1016/j.cell.2011.02.013
185. Grisaru-Tal S, Itan M, Klion AD, Munitz A. A new dawn for eosinophils in the tumour microenvironment. Nat Rev Cancer (2020) 20:594–607. doi: 10.1038/s41568-020-0283-9
186. Grisaru-Tal S, Rothenberg ME, Munitz A. Eosinophil–lymphocyte interactions in the tumor microenvironment and cancer immunotherapy. Nat Immunol (2022) 23:1309–16. doi: 10.1038/s41590-022-01291-2
187. Zhang J, Yin H, Chen Q, Zhao G, Lou W, Wu W, et al. Basophils as a potential therapeutic target in cancer. J Zhejiang Univ B (2021) 22:971–84. doi: 10.1631/jzus.B2100110
188. Khan ANH, Emmons TR, Wong JT, Alqassim E, Singel KL, Mark J, et al. Quantification of early-stage myeloid-derived suppressor cells in cancer requires excluding basophils. Cancer Immunol Res (2020) 8:819–28. doi: 10.1158/2326-6066.CIR-19-0556
189. Goubran HA, Stakiw J, Radosevic M, Burnouf T. Platelets effects on tumor growth. Semin Oncol (2014) 41:359–69. doi: 10.1053/j.seminoncol.2014.04.006
190. Yan M, Jurasz P. The role of platelets in the tumor microenvironment: From solid tumors to leukemia. Biochim Biophys Acta - Mol Cell Res (2016) 1863:392–400. doi: 10.1016/j.bbamcr.2015.07.008
191. Zhang L, Liu J, Qin X, Liu W. Platelet–acute leukemia interactions. Clin Chim Acta (2022) 536:29–38. doi: 10.1016/j.cca.2022.09.015
192. Schmied L, Höglund P, Meinke S. Platelet-mediated protection of cancer cells from immune surveillance – possible implications for cancer immunotherapy. Front Immunol (2021) 12:640578. doi: 10.3389/fimmu.2021.640578
193. Nieswandt B, Hafner M, Echtenacher B, Männel DN. Lysis of tumor cells by natural killer cells in mice is impeded by platelets. Cancer Res (1999) 59:1295–300.
194. Betz SA, Foucar K, Head DR, Chen IM, Willman CL. False-positive flow cytometric platelet glycoprotein IIb/IIIa expression in myeloid leukemias secondary to platelet adherence to blasts. Blood (1992) 79:2399–403. doi: 10.1182/blood.V79.9.2399.2399
195. Goswami S, Anandhan S, Raychaudhuri D, Sharma P. Myeloid cell-targeted therapies for solid tumours. Nat Rev Immunol (2022) 11. doi: 10.1038/s41577-022-00737-w
196. Kaczanowska S, Beury DW, Gopalan V, Tycko AK, Qin H, Clements ME, et al. Genetically engineered myeloid cells rebalance the core immune suppression program in metastasis. Cell (2021) 184:2033–2052.e21. doi: 10.1016/j.cell.2021.02.048
Keywords: leukemia, neutrophils, macrophages, myeloid-derived suppressor cells, immune response, tumor microenvironment, immunotherapy
Citation: Magalhães-Gama F, Alves-Hanna FS, Araújo ND, Barros MS, Silva FS, Catão CLS, Moraes JS, Freitas IC, Tarragô AM, Malheiro A, Teixeira-Carvalho A and Costa AG (2022) The Yin-Yang of myeloid cells in the leukemic microenvironment: Immunological role and clinical implications. Front. Immunol. 13:1071188. doi: 10.3389/fimmu.2022.1071188
Received: 15 October 2022; Accepted: 14 November 2022;
Published: 01 December 2022.
Edited by:
Shenghui Zhang, First Affiliated Hospital of Wenzhou Medical University, ChinaReviewed by:
Heiko Bruns, University Hospital Erlangen, GermanyANM Nazmul Khan, University at Buffalo, United States
Copyright © 2022 Magalhães-Gama, Alves-Hanna, Araújo, Barros, Silva, Catão, Moraes, Freitas, Tarragô, Malheiro, Teixeira-Carvalho and Costa. This is an open-access article distributed under the terms of the Creative Commons Attribution License (CC BY). The use, distribution or reproduction in other forums is permitted, provided the original author(s) and the copyright owner(s) are credited and that the original publication in this journal is cited, in accordance with accepted academic practice. No use, distribution or reproduction is permitted which does not comply with these terms.
*Correspondence: Allyson Guimarães Costa, YWxseXNvbi5ndWkuY29zdGFAZ21haWwuY29t
†These authors have contributed equally to this work