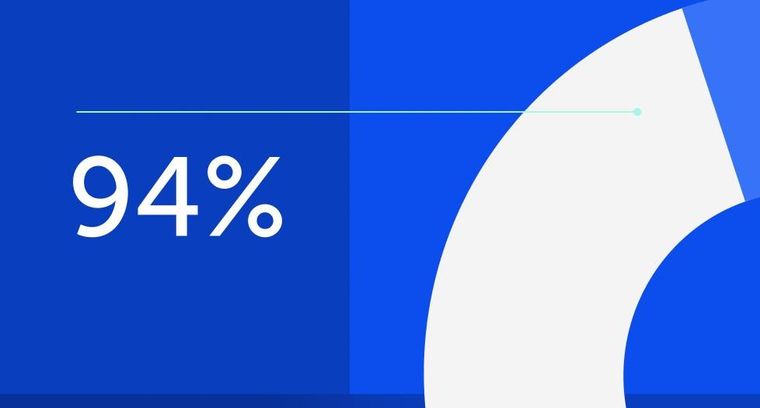
94% of researchers rate our articles as excellent or good
Learn more about the work of our research integrity team to safeguard the quality of each article we publish.
Find out more
REVIEW article
Front. Immunol., 13 December 2022
Sec. Viral Immunology
Volume 13 - 2022 | https://doi.org/10.3389/fimmu.2022.1070994
This article is part of the Research TopicImmunological Etiologies and Therapies of the Various Forms of Long-COVIDView all 16 articles
Background: Recovery from coronavirus disease 2019 (COVID-19) can be impaired by the persistence of symptoms or new-onset health complications, commonly referred to as Long COVID. In a subset of patients, Long COVID is associated with immune system perturbations of unknown etiology, which could be related to compromised immunoregulatory mechanisms.
Objective: The objective of this scoping review was to summarize the existing literature regarding the frequency and functionality of Tregs in convalescent COVID-19 patients and to explore indications for their potential involvement in the development of Long COVID
Design: A systematic search of studies investigating Tregs during COVID-19 convalescence was conducted on MEDLINE (via Pubmed) and Web of Science.
Results: The literature search yielded 17 relevant studies, of which three included a distinct cohort of patients with Long COVID. The reviewed studies suggest that the Treg population of COVID-19 patients can reconstitute quantitatively and functionally during recovery. However, the comparison between recovered and seronegative controls revealed that an infection-induced dysregulation of the Treg compartment can be sustained for at least several months. The small number of studies investigating Tregs in Long COVID allowed no firm conclusions to be drawn about their involvement in the syndrome’s etiology. Yet, even almost one year post-infection Long COVID patients exhibit significantly altered proportions of Tregs within the CD4+ T cell population.
Conclusions: Persistent alterations in cell frequency in Long COVID patients indicate that Treg dysregulation might be linked to immune system-associated sequelae. Future studies should aim to address the association of Treg adaptations with different symptom clusters and blood parameters beyond the sole quantification of cell frequencies while adhering to consensualized phenotyping strategies.
Infections with the severe acute respiratory syndrome coronavirus 2 (SARS-CoV-2), the pathogen responsible for the ongoing coronavirus disease 2019 (COVID-19) pandemic, manifest with varying severity. The clinical spectrum ranges from asymptomatic/mild disease to severe pneumonia and respiratory distress syndrome that can ultimately lead to death (1, 2). Although the majority of patients experience moderate symptoms such as fever, cough, dyspnea, loss of smell and taste or sore throat, it has been highlighted that COVID-19 can include multi-system complications, such as thrombotic events, vasculitis and myocarditis (3–5). In addition to that, the clinical spectrum of COVID-19 encompasses acute immunopathology. Specifically, dysfunctional cellular and humoral immune responses characterized by exaggerated cytokine release, lymphopenia and new onset or aggravated autoimmunity are associated with negative disease outcomes (1, 6–9).
It is estimated that approximately one in eight patients who contracted COVID-19 experience symptoms beyond the acute symptomatic phase, as reported by a recent observational cohort study (10). The World Health Organization (WHO) defines these sequelae, by common usage now also referred to as “Long COVID”, as a post-COVID-19 condition that usually occurs three months after a confirmed or probable SARS-CoV-2 infection with a set of new-onset, persistent or fluctuating symptoms that last for at least two months (11). The most common symptoms in this context are fatigue, exercise intolerance, post-exertional malaise, difficulty breathing, headache, muscle pain, tachycardia, concentration deficits and diminished quality of life (12–15). In this context, it bears noting that the overall prevalence and types of symptoms can vary depending on the COVID-19 disease severity. Specifically, the proportion of patients that experience residual symptoms appears to be higher among previously hospitalized patients (16–18). In addition, the results of a clustering analysis conducted as a part of the REACT-2 study suggest that patients with severe initial symptoms report post-acute respiratory symptoms more often than those with mild or asymptomatic COVID-19 whose symptomatology is rather fatigue-dominant (19). Likewise, recent evidence suggests that those who had one or multiple reinfections exhibit an increased risk of experiencing post-acute sequelae compared to those who were infected once (20).
The pathophysiological mechanisms involved in the devolvement of Long COVID remain to be elucidated, with a range of theories currently under discussion. Merad et al. (21), for instance, postulated that immunopathological processes, such as chronic inflammation with viral persistence, post-viral autoimmunity, microbiome dysbiosis and unrepaired tissue damage might contribute to the pathophysiology of the Long COVID phenotype. In line with that, it has been documented that affected patients exhibit a significant elevation of multiple inflammatory markers compared to recovered subjects, indicating a dysregulated and overactive immune system (22, 23). Correspondingly, the triad of pro-inflammatory cytokines IL-1β, IL-6 and TNF-α showed a significant correlation with persistent symptoms of COVID-19 at an eight-months follow-up (24). In addition, the discovery of functional autoantibodies specific to G-protein coupled receptors (25) has been linked to an autonomic dysregulation and assumed to be a cause of residual symptoms (26).
Despite this, components of the immune system that regulate and fine-tune (auto)immune responses, favoring immune homeostasis, such as regulatory T cells (Tregs), have only been investigated sparsely in COVID-19 convalescence and Long COVID. Tregs are a dynamic CD4+ T cell subpopulation that is characterized by CD25 (IL-2 receptor α-chain) upregulation, CD127 (IL-7 receptor α-chain) downregulation and expression of the transcription factor forkhead box P3 (FoxP3) that is considered the master regulator of Treg development (27–30). It is estimated that 80% of the peripheral cell repertoire is represented by functionally mature natural Tregs that develop in the thymus as antigen-primed cells (31, 32), while peripherally-derived Tregs are induced from naïve T cells upon antigen encounter (32–34) (Figure 1). During self-directed and pathogen-directed immune responses, activated Tregs exert suppressive effects on effector cells and antigen-presenting cells, for example, through the secretion of the inhibitory cytokines IL-10, IL-35 and TGF-β, by limiting the amount of IL-2 available for conventional T cells or by stimulating the formation of indoleamine 2, 3-dioxygenase (28, 35) (Figure 1). In this way, Tregs promote self-tolerance and prevent excessive inflammation, which makes their proper functioning indispensable for a balanced immune response. Correspondingly, diseases such as type 1 diabetes, multiple sclerosis, lupus erythematosus and rheumatoid arthritis are associated with functional deficiencies in the Treg compartment and altered cell frequencies (36, 37).
Figure 1 Schematic illustration of Treg development, modes of activation and physiological functions. According to an affinity-based model of T cell development, a fraction of self-reactive T cells with intermediate affinity for self-peptide-MHC complexes differentiates into natural Tregs by upregulation of FoxP3 and CD25 in response to T cell receptor (TCR) signaling (thymus-derived Tregs; ~80% of peripheral Treg repertoire) (31). Also, antigenic stimulation of peripheral naïve T cells in the presence of TGF-β, IL-2 and retinoic acid induces their differentiation into FoxP3+ Tregs (peripherally-derived Tregs) (28). Of note, phenotypical transition of Tregs upon activation is not associated with de novo expression of surface proteins but rather with quantitative shifts in the expression levels of molecules already expressed in a resting state. Specifically, upon TCR activation, Tregs downregulate CD45RA and upregulate CD45RO, CD25, CTLA-4 and FoxP3 (32, 33). In contrast to other T cell subsets, the transition of Tregs into a stable memory pool after antigen elimination is still debated and so far, only a few marker candidates such as CD62L have been proposed for identification of memory Tregs (34). Functionally, Tregs are involved in suppressing immune responses towards self and non-self-antigens via inhibitory cytokine secretion, granzyme-dependent and cytokine-deprivation-mediated effector cell inhibition as well as cell-contact-dependent alteration of dendritic cell function and maturation (potential consequences of deficient Treg development or function are indicated by red lightnings) (35) (created with BioRender.com). CM, central memory; CTLA-4, cytotoxic T lymphocyte antigen 4; DC, dendritic cell; EM, effector memory; IDO, indoleamine 2,3-dioxygenase; IPEX, Immunodysregulation, polyendocrinopathy, enteropathy, X-linked syndrome; RA, retinoic acid.
Studying COVID-19, previous reviews have already made an effort to characterize Tregs during the acute phase of a SARS-CoV-2-infection (38–41). Although evidence exists that alterations in the Treg compartment might contribute to the immunopathology of COVID-19, the literature concerning their functionality and frequency during the acute illness is controversial. Nevertheless, a trend toward functional impairments and decreased levels of circulating Tregs in severe cases of COVID-19 has been reported previously (38–40). It was shown that patients dying from COVID-19 exhibited significantly lower Treg counts and higher Th17/Treg ratio compared to recovered and healthy controls (42–44) and that low Treg counts at hospital admission were associated with clinical worsening and longer duration to discharge (45, 46). Correspondingly, several studies demonstrated a downregulation of FoxP3 in severe cases of COVID-19 (44, 47–49).
Given the aforementioned evidence suggesting that an ongoing inflammatory profile, autoantibody formation, inflammatory tissue damage, SARS-CoV-2 persistence and viral reactivation (herpes virus family) likely contribute to the persistence of symptoms, it is conceivable that Tregs are involved in the pathophysiology of Long COVID (50). Yet, there exist uncertainties concerning the longitudinal dynamics of the Treg population during recovery from COVID-19, and it remains unclear if Treg dysfunction is involved in the immunopathology of Long COVID. Therefore, we aimed to summarize the existing literature on the frequency (e.g., absolute counts, relative frequency) and functionality (e.g., cytokine secretion, ex vivo suppressive capacity) of Tregs in convalescent COVID-19 patients with and without persisting symptoms and to explore indications for their potential involvement in the development of Long COVID.
A scoping review of the literature was conducted following the Preferred Reporting Items for Systematic Reviews and Meta-Analysis (PRISMA) extension for scoping reviews (51).
The literature search was conducted up to July 23, 2022, using Pubmed and Web of Science with no restriction on publication date. The search string included controlled vocabulary, Medical Subject Headings (MeSH) and common synonyms for the domains COVID-19 and regulatory T cells while excluding review articles (see Supplementary Material 1). Furthermore, reference lists of included studies were examined in order avoid missing any relevant studies.
The titles and abstracts of the records that were identified through the literature search were independently screened for their eligibility by two investigators (SH and CP). Subsequently, full-text articles were retrieved to verify the inclusion decision that was made based on title and abstract screening (Figure 2).
Eligible studies were selected using the following PICOS (participants, intervention, comparison, outcomes, and study design) criteria.
This review targets convalescent COVID-19 patients with and without persistent symptoms at least four weeks after confirmed or probable detection of SARS-CoV-2 infection. No inclusion or exclusion criteria were set for participants’ age ranges, sex, or severity of infection.
Mass or flow cytometric analysis of Treg outcomes.
Acute COVID-19 cases sampled at least 14 days after confirmed COVID-19 infection and seronegative controls, who were asymptomatic for last four weeks and tested negative for SARS-CoV-2 by antibody testing or polymerase chain reaction at time of sampling were accepted as control groups.
Treg counts (absolute cell numbers), proportions (relative cell numbers) or regulatory function (cytokine secretion, ex vivo suppressive capacity), and the FoxP3 gene expression were considered as study outcomes.
Case‐control studies, cross‐sectional studies, cohort studies, clinical trials, or case reports published in English or German were included in this review. Book chapters, congress proceedings, meeting abstracts, reviews, animal studies, and studies published in languages other than English or German were deemed ineligible.
The following data items were extracted from the included studies: sample size, sex, gender, time elapsed since disease onset and severity of acute disease, markers used for Treg phenotyping, source of the cell sample, Treg-related outcome measures and outcome-related findings. Extracted data items are presented in a tabulated form (Table 1).
The literature search yielded a total of 405 potentially relevant records. After removing duplicates, 268 studies were screened for their eligibility, of which 17 were ultimately included in this review. Three studies (69–71) were deemed potentially relevant but could not be included due to insufficient characterization of the convalescent cohort in terms of the time point of blood sampling (Figure 2).
All studies investigated a cohort of patients with a confirmed or presumed COVID-19 infection at least four weeks and latest 50 weeks after index infection. The total number of participants investigated during COVID-19 convalescence was 1367 (range: 14-214). In addition, 13 studies included a cohort of seronegative controls (371 total participants), and seven included one or more cohorts of patients sampled during acute COVID-19 illness (375 total participants of which 368 had been admitted to the hospital). The number of studies characterizing Tregs in a cohort of patients with Long COVID, in accordance with the previously mentioned definition by the WHO, was limited to three, with a total number of 160 participants (Table 1).
Eleven of the included studies did not recruit an exclusive cohort of patients specifically diagnosed with Long COVID, thus we must assume that the convalescent patients investigated in these studies were all recovered from COVID-19 and did not exhibit any residual symptoms. Wiech et al. (65) assessed Long COVID symptoms of investigated subjects retrospectively at a one-year follow-up and reported that 72% of the participants experienced persistent symptoms (e.g., cognitive dysfunction, fatigue, dyspnea) for at least three months. Likewise, Ryan et al. (62) retrospectively assessed that 30% of the participants were referred to a Long COVID clinic and Shuwa et al. (63) reported that approximately half of the patients experienced dyspnea at follow-up sampling. However, since those subjects with persisting symptoms were analyzed together with recovered subjects, the results of these studies provide only limited evidence concerning Treg outcomes in Long COVID.
All studies measured the relative cell frequency as the central Treg-related outcome, whereas only one study reported absolute cell counts (58). In addition to that, the expression level of the FoxP3 transcription factor was investigated in two studies (52, 55) and domains of Treg functioning like suppressive function and cytokine production in one (55) and two (55, 65) studies, respectively. Studies analyzed whole blood or peripheral blood mononuclear cells using flow cytometry and mass cytometry. The markers used for Treg phenotyping differed markedly between the studies and are detailed in Table 1 together with the study and subject characteristics.
Although no study compared blood samples obtained from the same cohort of patients both during acute illness and convalescence, six studies compared Treg parameters between recovered and acute cases of different severity (Figure 3). A significant difference in peripheral Treg frequency in relation to recovering patients could only be demonstrated for acutely hospitalized patients, whereas non-hospitalized patients exhibited no different Treg proportions. Garcia-Gasalla et al. (53) investigated 74 patients between eight and 12 weeks after hospital discharge and compared their immune profiles to patients that were sampled within the first 48 hours after hospital admission with different disease severity. Accordingly, the recovered patients exhibited greater relative Treg frequencies compared to severe/critical (p<0.001) and mild/moderate (not significant) cases that were hospitalized for COVID-19 (53). This is in line with other studies that demonstrated an expansion of the Treg population and also a decreased Th17/Treg ratio compared to severely ill patients, albeit the investigated convalescent patients mostly recovered from mild or asymptomatic diseases (55, 57). On the other hand, only one study documented a decrease in the Treg frequency in convalescent compared to hospitalized COVID-19 patients at a median follow-up of 42 days after mild infection (p=0.01) (52). This change could however not be observed in comparison to outpatients (52). Likewise, another study found no change between patients recovering from severe COVID-19 and acutely ill patients, regardless of their disease severity (63).
Figure 3 Total Treg frequency in convalescent COVID-19 patients compared to other cohorts (references in brackets). The upwards arrow (↑) indicates a significant increase at p<0.05, the downwards arrow (↓) a significant decrease at p<0.05 and the left-right arrow (↔) not significantly different relative Treg proportions in convalescent patients (created with BioRender.com).
Adding to this body of literature, several studies assessed parameters of Treg functionality in relation to acute cases. Specifically, Galván-Peña et al. (52) reported that Tregs from severe acute cases showed overlaps with tumor-infiltrating Tregs. Specifically, the analysis of the transcriptional signature revealed for example an acute enrichment of hypoxia-induced transcripts that was not present in healthy donors and only scarcely in recovered patients. Furthermore, the expression of FoxP3, IL-10 production and the suppressive function on effector T cell proliferation has been reported to be higher in recovered compared to severely ill patients (55, 67). However, it bears noting that all patients that were investigated for Treg functioning during convalescence recovered from mild disease.
The comparison between recovered patients and seronegative controls produced ambiguous results. While five studies found no significant difference in frequencies of total Tregs between patients post-COVID-19 and patients without history of COVID-19 (52, 54, 55, 62, 63), two studies reported a lower (56, 58) and four reported a greater peripheral Treg frequency in recovered patients (57, 59, 60, 65) (Figure 3). However, this discrepancy did not seem to be attributable to differences in initial disease severity or the time elapsed since diagnosis. A study of 49 individuals recovering from COVID-19 in two different countries, of which approximately one-third were hospitalized, showed that the COVID-19 group exhibited significantly higher Treg frequencies than healthy controls at a mean follow-up of 41 or 112 days (p<0.05) (59). In line with that, Petrara et al. (60) demonstrated greater Treg proportions in patients several months after index infection compared to uninfected controls (p<0.0001), with that difference being even greater in the pediatric (<15 years) population. By contrast, Kratzer et al. (58) reported lower total Treg cell frequencies in individuals recovering from predominantly mild infections (p=0.004), which was further accompanied by lower proportions of several Treg subsets compared to controls (p<0.006).
A more detailed analysis of Treg subsets in a cohort of convalescent patients that did not exhibit significantly different total Treg proportions compared to controls showed that subsets in different maturation statuses differed significantly between investigated groups. Accordingly, naïve Tregs (CD4+ CD25high CD127low CD45RA+ CD62L+) and effector memory Tregs expressing CD45RA (TEMRA; CD4+ CD25high CD127low CD45RA+ CD62L-) were more frequent, whereas central memory (CD4+ CD25high CD127low CD45RA- CD62L+) and effector memory (CD4+ CD25high CD127low CD45RA- CD62L-) Tregs were lower in patients 12 weeks post-infection (62). Consistent with this, Wiech et al. (65) documented increased proportions of naïve cells among Tregs compared to healthy donors.
One study assessing the cytokine release of Tregs in seronegative controls reported that there was no significant difference in IL-10, IL-17 production and suppressive function compared to previously asymptomatic patients (55).
Five studies compared Treg outcomes among recovered patients with different initial disease severities, reaching equivocal results (54, 57, 62, 64, 65). A study that followed-up subjects that were hospitalized for mild and severe courses of COVID-19 found out that six months post-discharge there were patients with severe illness that presented with persistently low CD8+ T cell counts (<250/ml) (64). Those subjects exhibited a higher Treg frequency than severely ill who were categorized as having high CD8+ T cell counts (>250/ml) and patients with mild initial disease (64). Two other studies that investigated total Treg frequencies documented both higher and lower proportions in convalescent outpatients compared to those who were hospitalized (54, 62) (Figure 3). Similarly as heterogeneous were the results of the studies that investigated Treg subsets. While Ryan et al. (62) found no considerable difference between patients recovering from mild and severe initial disease for naïve, central memory, effector memory or TEMRA subsets, another study reported lower effector Treg populations and lower FoxP3 expression levels in previously hospitalized patients (54). At the same time, single-cell RNA sequencing analysis revealed that relative to all other severity groups, patients recovering from an asymptomatic infection exhibited an expansion of the Treg population with high expression of class II HLA molecules that are suggested to exert high contact-mediated suppression of T helper cell responses (54).
Six studies obtained blood samples at multiple time points during COVID-19 convalescence (54, 56, 60–62, 65). The longitudinal analysis conducted by Kostopoulos et al. (56) showed that the relative frequency of total Tregs that was decreased two months post-infection continued to decrease at the eight-month follow-up (p<0.001). Similarly, Wiech et al. (65) reported that naïve Tregs further increased from first (14-90 days post-infection) to second (91-180 days post-infection) follow-up regardless of the patients’ initial disease severity, whereas central memory Tregs decreased. Additionally, the in vitro production of IL-2 decreased significantly over time, while the TGF-β production did not change (65). Another three studies demonstrated that Treg frequency and parameters of Treg functioning that were up- or downregulated earlier in the recovery period, returned to an equilibrium point (54, 60, 62). Accordingly, Ryan et al. (62)documented that naïve and TEMRA subsets that were greater as well as effector memory and central memory subsets that were lower in recovered subjects 12 weeks post-infection, decreased or increased towards the values exhibited by seronegative controls at the 16- and 24-weeks follow-ups.
Three studies investigated the proportion of Tregs among CD4+ cells in patients that exhibit residual symptoms of COVID-19 and compared them to seronegative controls and individuals that recovered from COVID-19 (66–68) (Figure 3). The symptoms experienced by the patients in the Long COVID group covered a broad spectrum, including concentration and memory impairments, headaches, palpitations, insomnia, myalgia, fatigue and shortness of breath. The two studies that sampled patients with lingering symptoms almost one year post-infection reported contradictory results. While Galán et al. (66) documented a 2.5-fold greater Treg frequency in Long COVID patients compared to subjects that recovered completely (p=0.0007), Utrero-Rico et al. (68) found a change in the opposite direction (p<0.001). Likewise, 121 Long COVID patients exhibited significantly lower proportions of FoxP3-expressing CD4+ cells compared to seronegative controls (67). None of the studies investigated parameters of Treg functioning such as cytokine secretion or suppressive capacity in Long COVID.
The objective of this scoping review was to summarize the existing literature regarding the frequency and functionality of Tregs in convalescent COVID-19 patients and to explore indications for their potential involvement in the development of Long COVID. Owing to the fact that none of the studies investigating a cohort of convalescent COVID-19 patients obtained baseline blood samples during the acute phase of the disease, the exact longitudinal dynamics of Tregs after infection with SARS-CoV-2 remain elusive. However, the comparisons of separate cohorts of patients sampled during severe acute disease and recovery showed increased systemic frequency of Tregs and greater suppressive function in recovering subjects, which points towards a possible restoration of Treg frequency and function (53, 55, 57).
Earlier studies investigating patients during acute illness revealed that COVID-19 can induce alterations in lymphocyte frequency and functioning that are associated with disease severity and prognosis (2, 45, 72–74). In severe cases, a loss of CD4+ lymphocytes, including Tregs is a prominent clinical feature that, together with an enhanced myelopoiesis, contributes to an expansion of neutrophils, dendritic cells and macrophages, favoring immunopathology, excessive tissue infiltration and acute respiratory distress syndrome (45, 46, 75–78). Of note, the decrease of Tregs in severe COVID-19 patients is not only a reflection of diminished absolute cell counts in the context of lymphopenia but is also linked to altered relative cell frequencies, as a result of Th17 cell expansion (79).
The trend towards a quantitative reconstitution of Tregs was supported by five studies investigating patients recovering from diseases of different severity (52, 54, 55, 62, 63). The fact that the studies were not able to find significant differences in Treg frequency between recovering subjects and seronegative controls suggests that the peripheral Treg population was either unaffected by the initial disease or returned to normal values within the first six weeks following mild disease (52, 54, 55) or four months following severe disease (54, 62). Further illustrating this, three studies that obtained multiple blood samples during recovery from COVID-19 showed that the Treg frequency exhibited by subjects at the second and third follow-ups was closer to the frequency of seronegative controls than during the first follow-up (60, 62, 65). Beyond that, the expression of the Treg-defining transcription factor FoxP3 has been described to be significantly higher in convalescent patients (55). Yet, the significance of this finding is unclear, as the recruited subjects recovered from asymptomatic infections, which might induce less pronounced Treg perturbations in the first place, as it has been indicated by previous reviews (38, 40). Correspondingly, in comparison to acute non-hospitalized patients, recovered patients did not show significantly different Treg levels (52, 53, 63).
Nevertheless, it also became apparent that infection-induced adaptations in the Treg compartment are heterogeneous and that Treg dysregulation can persist for at least several months post-infection. Correspondingly, several of the reviewed studies found that compared to seronegative controls, recovering subjects exhibited significantly different Treg frequencies (56–60). Kostopoulos et al. (56), for example, reported that even eight months after disease onset the proportion of Tregs had not returned to normal. Beyond that, Shuwa et al.’s (63) findings showed that the range of the Treg frequency, as well as the deviation from the mean within the recovering group was considerably greater than what would be expected based on the data from seronegative controls, which indicates that SARS-CoV-2 might induce Treg adaptations that are highly individual and dependent on factors like age, gender, co-morbidities, training status or immunological homeostasis and epigenetic landscape (80–82).
Still, the studies reviewed here did not allow to draw any firm conclusions on how alterations in Treg frequency or function are associated with initial disease severity and time since disease onset. It is therefore yet unsolved what the mechanisms behind persistent dysregulations of the Treg compartment are and what factors determine if the immunological homeostasis is reinstated. Several mechanisms have been proposed to which the acute reduction of Tregs, especially in severely ill patients, might be attributable. The surge of IL-6 caused by an exaggerated innate immune response for example might selectively antagonize the generation of Tregs and FoxP3 induction while promoting a pro-inflammatory Th17 cell profile (83, 84). Supporting this point, a positive correlation between IL-6 and Th17, a greater Th17/Treg ratio, as well as an inverse correlation between neutrophil and lymphocyte numbers have been documented in severe cases of COVID-19 (85). Additionally, other factors that are important for Treg development, such as dehydroepiandrosterone sulfate, which inhibits IL-6 and activates Tregs as well as retinoic acid, which is essential for Treg differentiation are depleted in patients with COVID-19 (86, 87). Finally, Treg extravasation and compartmentalization into inflamed tissues and an increased apoptosis rate could be responsible for low peripheral Treg levels in a subset of patients (40).
The scarcity of studies investigating Tregs in this specific cohort allows no firm conclusions to be drawn about the nature of Treg adaptations in Long COVID. Still, the reviewed studies indicated that Long COVID patients exhibit Treg dysregulations long after their initial infection with SARS-CoV-2. Two studies that were comparable in terms of the time elapsed from disease onset to follow-up sampling, markers used for cell phenotyping and the subjects investigated, found both a significantly greater and lower proportion of Tregs among CD4+ cells in patients with residual symptoms compared to recovered subjects (66, 68). A decreased Treg frequency compared to seronegative controls was reported in a study of 121 subjects with post-acute sequelae of COVID-19 (67).
When interpreting these results, it must be kept in mind that Long COVID constitutes a heterogenous and multifaceted syndrome, presenting diverse clinical manifestations (88, 89). Previous research has proposed that several phenotypes and distinct sub-diagnoses might exist under the umbrella of Long COVID, some of which with little immunological contribution (19, 90, 91). Since the post-acute sequelae of COVID-19 can most certainly not be attributed to a single pathophysiological mechanism (18), it is conceivable that a Treg dysregulation contributes to a Long COVID-associated immunopathology in multiple ways (Figure 4), as it has also been suggested in other viral infections. For instance, the investigation of patients infected with hepatitis C virus (HCV) showed that those with chronic infections and HCV persistence exhibited higher Treg levels than recovered subjects (92, 93). By contrast, chronically infected patients that developed autoimmune complications exhibited significant reductions in Treg levels compared to those who did not and to healthy controls (93, 94).
Figure 4 Commonly suggested theories for the non-exclusive pathophysiological mechanisms involved in Long COVID (1–4) and how they might be related to Treg dysregulation (created with BioRender.com). GPCR, G-protein coupled receptor.
Post-viral autoimmunity is also one of the main hypotheses discussed with regard to the development of Long COVID (Figure 4). Functional autoantibodies against a wide array of cell components and messenger molecules have been identified in patients with persistent symptoms (25, 95–97). Clinically, the formation of vasoactive autoantibodies directed against G-protein-coupled receptors (GPCR), for example, might consequently impede vascular tone and autonomic functions. Correspondingly, the treatment with a DNA aptamer drug neutralizing autoantibodies targeting GPCR improved retinal capillary microcirculation and led to the disappearance of Long COVID symptoms (98). If Long COVID patients with latent autoimmunity also exhibit low levels or dysfunctional Tregs warrants further investigation.
On the other hand, an increase in the number and function Tregs can result in a suppression of effector immune responses, ultimately favoring deficient viral clearance and persistent infections that cause cyclical symptoms flare-ups (93) (Figure 4). The persistence of SARS-CoV-2 RNA and infected cells in certain tissue reservoirs, such as the olfactory mucosa or the gastrointestinal tract is a common observation that seems to be facilitated by immunosuppression (99–102). Persistent detection of viral RNA in plasma, stool and cerebrospinal fluid has further been reported in patients with Long COVID (103–105). Whether viral persistence in these patients is a reflection of perturbed Treg dynamics or rather the virus’ own capability to evade immune responses and to shield its RNA from degradation remains to be delineated. A study that examined molecular features of 50 patients with long and short duration of viral shedding found that Treg frequency was significantly higher in those with long duration shedding, accompanied by a general immunosuppressive status (106). Likewise, other studies demonstrated that the total Treg frequency was associated with prolonged SARS-CoV-2 positivity and RNA shedding (107, 108), collectively suggesting that high Treg levels constitute one factor that might favor viral persistence. Furthermore, Taeschler et al. (64) provided evidence that persistently low levels of CD8+ T cells six months post-discharge were associated with a higher Treg frequency.
However, it bears noting that an increased Treg frequency is not necessarily a reflection of a greater regulatory capacity within the cell population (30). The studies reviewed here did not specifically investigate aspects of Treg function in Long COVID, but some reports raise the possibility that pathophysiological mechanisms that are associated with Long COVID could also adversely affect Treg functions. It has, for example, been shown that the integrity of specific intracellular metabolic processes is essential for the maintenance of Treg suppressive function and that the reliance on mitochondrial metabolism is greater relative to other cells of the CD4 lineage (109, 110). Evidence indicating a mitochondrial dysfunction in Long COVID that compromises this metabolic integrity comes from studies that reported a loss of mitochondrial membrane potential (111), greater glycolysis rates (112, 113) or oxidative stress (114) in Long COVID. Additionally, persistently high systemic and tissue-level concentrations of inflammatory cytokines like IL-6 and TNF could subvert the Tregs’ regulatory capacity (115). For future studies, it would therefore be desirable to get further in-depth insights into Treg functional aspects in Long COVID and COVID-19 convalescence in general. In this context, it might also be important to consider the migratory capacity of Tregs, since the selective expression of adhesion molecules and chemokine receptors is a major determinant of their regulatory function (116). As Tregs play a decisive role in resolving tissue injuries (117, 118), it might also be worthwhile to investigate them in tissue samples from sites in which persistent inflammation and damage to the organ infrastructure have been documented throughout the post-acute phase, such as the lungs, myocardium or intestines (119–122). Studies investigating fatal cases of COVID-19 found a profound dysfunction of Tregs in the lungs and pulmonary draining lymph nodes of autopsied patients, as indicated by lower FoxP3 expression, limited IL-10 production, and a paucity of FoxP3+ Tregs compared to surviving and non-COVID-19 controls (123, 124). Beyond that, a case study of four intubated patients showed that the T cell profile in the tissue and blood compartment can be profoundly different, as the Treg proportion for instance was higher in the bronchoalveolar lavage fluid than in blood (125). Still, publications investigating tissue resident Tregs are biased towards severe or critical cases of COVID-19 with a lack of studies recruiting outpatients and convalescents.
Owing to the relative novelty of the research area, the studies reviewed in this scoping review had several limitations that need to be considered when interpreting their results, which could be harnessed to improve future research efforts in this area. A general issue that can limit the comparability of study results is the identification and isolation of Tregs. An extensive list of potential Treg marker candidates has been proposed (126). However, the fact that Tregs represent a highly dynamic cell population and that none of the markers is exclusively expressed by Tregs makes their characterization a complicated task (35). To facilitate standardized and reliable isolation procedures across studies, Santegoets et al. (127) published a consensus on essential markers for the detection and functional analysis of Tegs. They concluded that basal phenotyping should minimally include staining for CD4, CD25, CD127 and FoxP3, a recommendation that only three studies (58, 60, 61) sufficiently adhered to. Moreover, the studies included here used five different marker sets to identify total Treg frequencies, which might account for the broad range of Treg proportions across studies that was also present among seronegative controls.
None of the studies reviewed here explicitly stated which SARS-CoV-2 variant the included subjects contracted. Twelve studies conducted participant recruitment before the alpha variant was designated a variant of concern in December 2020. Therefore, it can be assumed that participants in these studies contracted the wild type. Four studies did not specify the date of sampling (52, 54, 55, 67)and Wiech et al. (65) reported that during sampling in early 2021 the alpha variant became dominant in Poland. Given that participant recruitment was carried out relatively early after the global emergence of SARS-CoV-2, it is also likely that most subjects have only been infected once. Furthermore, as the widespread rollout of vaccination campaigns in Europe and the United States began in early 2021, subjects investigated in the included studies were most certainly unvaccinated. While this homogeneity between studies in terms of the stage of viral evolution and lack of population immunity at the time of infection might enhance the comparability of results, it also leads to the circumstance that the presented data provide a temporary snapshot that is not necessarily representative of the current clinical situation. Over the last two years, several new variants have emerged that can effectively evade immunity acquired through vaccination or natural infection (128). The latest omicron variant encodes 37 amino acid substitutions in the spike protein, 15 of which are in the receptor binding domain, resulting in an antigenic variation that subverts innate and adaptive immunity and enhances cell entry (129, 130). Besides that, previous research has also indicated that many pathogens have developed mechanisms to use immune regulatory networks for their advantage, for example by upregulating the expression of IL-10 or TGF-β and by dampening effector cell responses (93). Preliminary evidence showed that in vitro stimulation with omicron variant peptide induced significantly greater FoxP3 expression in draining lymph node cells than with the wild-type, which was interpreted as an indication that the variant peptide might induce a switch in T cell function from an effector to a regulatory program (131). In this context, it is also important to mention that none of the included studies investigated Tregs with regard to their antigen specificity. Taking these considerations into account, it should be further investigated if Treg adaptations are affected by the variant contracted and if they are altered in individuals that already had some level of SARS-CoV-2 specific immunity. Furthermore, the investigation of SARS-CoV-2-specific Tregs would provide deeper insights into the virally induced landscape.
Beyond that, it becomes more and more apparent that Long COVID cannot be regarded as a single diagnosis but that it encompasses several diagnoses with distinct pathophysiological mechanisms. It can therefore be assumed that given the range of symptoms exhibited by the included patients, the three studies investigating Long COVID portrayed a mix of different pathologies. For future studies, it would be intriguing to investigate if Treg frequencies and Th17/Treg ratio correlate with specific symptom clusters like they were previously proposed or with laboratory parameters such as serological status, cytokine levels or (auto)antibody titers.
COVID-19 is associated with perturbations in the Treg homeostasis, albeit reports about exact changes in cell number remain controversial. The studies reviewed here indicated that dysregulation in the Treg compartment can persist for at least several months post-infection. Also, the results of the three studies included in this review, accompanied by previously postulated hypotheses regarding the development of Long COVID suggest that Treg dysregulation might be involved in the post-acute persistence of symptoms. However, the methodological heterogeneity of the included studies with respect to the subjects recruited, the phenotyping strategies and the sampling at different stages of the recovery makes it difficult to delineate what factors determine the reinstatement of immunological homeostasis. Collectively, it became apparent that despite plausible connections to the pathophysiology of COVID-19 and its associated sequelae, to this day, Tregs have received little scientific attention that goes beyond the quantification of cell numbers. Correspondingly, several aspects, such as alterations in Treg functionality need further investigation. Others like the influence of new variants, vaccination status and previous infections remained completely unaddressed in the included studies and should be considered for future research efforts.
SH and CP drafted the manuscript and created the figures. WB, FJ, KK, SB, SD, CL, PR, AS, MM, CZ, MP and HG critically reviewed and edited the manuscript. All authors contributed to the manuscript, read, and approved the submitted version.
BMBF Verbundprojekt SARS-CoV-2Dx to SD. Leibniz Center for Photonics in Infection Research (LPI-BT6, CZ), Germany’s Excellence Strategy (Balance of the Microverse, CZ)
The authors declare that the research was conducted in the absence of any commercial or financial relationships that could be construed as a potential conflict of interest.
All claims expressed in this article are solely those of the authors and do not necessarily represent those of their affiliated organizations, or those of the publisher, the editors and the reviewers. Any product that may be evaluated in this article, or claim that may be made by its manufacturer, is not guaranteed or endorsed by the publisher.
The Supplementary Material for this article can be found online at: https://www.frontiersin.org/articles/10.3389/fimmu.2022.1070994/full#supplementary-material
1. Guan W-J, Ni Z-Y, Hu Y, Liang W-H, Ou C-Q, He J-X, et al. Clinical characteristics of coronavirus disease 2019 in China. New Engl J Med (2020) 382:NEJMoa2002032. doi: 10.1056/nejmoa2002032
2. Huang C, Wang Y, Li X, Ren L, Zhao J, Hu Y, et al. Clinical features of patients infected with 2019 novel coronavirus in wuhan, China. Lancet Lond Engl (2020) 395:497–506. doi: 10.1016/s0140-6736(20)30183-5
3. Gupta A, Madhavan MV, Sehgal K, Nair N, Mahajan S, Sehrawat TS, et al. Extrapulmonary manifestations of COVID-19. Nat Med (2020) 26:1017–32. doi: 10.1038/s41591-020-0968-3
4. White-Dzuro G, Gibson LE, Zazzeron L, White-Dzuro C, Sullivan Z, Diiorio DA, et al. Multisystem effects of COVID-19: A concise review for practitioners. Postgrad Med (2020) 133:1–8. doi: 10.1080/00325481.2020.1823094
5. Ackermann M, Verleden SE, Kuehnel M, Haverich A, Welte T, Laenger F, et al. Pulmonary vascular endothelialitis, thrombosis, and angiogenesis in covid-19. New Engl J Med (2020) 383:120–8. doi: 10.1056/nejmoa2015432
6. Henderson LA, Canna SW, Schulert GS, Volpi S, Lee PY, Kernan KF, et al. On the alert for cytokine storm: Immunopathology in COVID-19. Arthritis Rheumatol Hoboken N J (2020) 72:1059–63. doi: 10.1002/art.41285
7. Diao B, Wang C, Tan Y, Chen X, Liu Y, Ning L, et al. Reduction and functional exhaustion of T cells in patients with coronavirus disease 2019 (COVID-19). Front Immunol (2020) 11:827. doi: 10.3389/fimmu.2020.00827
8. Liu R, Wang Y, Li J, Han H, Xia Z, Liu F, et al. Decreased T cell populations contribute to the increased severity of COVID-19. Clin Chimica Acta Int J Clin Chem (2020) 508:110–4. doi: 10.1016/j.cca.2020.05.019
9. Zhou Y, Han T, Chen J, Hou C, Hua L, He S, et al. Clinical and autoimmune characteristics of severe and critical cases of COVID-19. Clin Transl Sci (2020) 13:1077–86. doi: 10.1111/cts.12805
10. Ballering AV, van Zon SKR, olde Hartman TC, Rosmalen JGM, Initiative LCR. Persistence of somatic symptoms after COVID-19 in the Netherlands: an observational cohort study. Lancet (2022) 400:452–61. doi: 10.1016/s0140-6736(22)01214-4
11. Soriano JB, Murthy S, Marshall JC, Relan P, Diaz JV, Condition WCCDWG on p-C-19. A clinical case definition of post-COVID-19 condition by a delphi consensus. Lancet Infect Dis (2021) 22:E102-E107. doi: 10.1016/s1473-3099(21)00703-9
12. Iqbal FM, Lam K, Sounderajah V, Clarke JM, Ashrafian H, Darzi A. Characteristics and predictors of acute and chronic post-COVID syndrome: A systematic review and meta-analysis. Eclinicalmedicine (2021) 36:100899. doi: 10.1016/j.eclinm.2021.100899
13. Davis HE, Assaf GS, McCorkell L, Wei H, Low RJ, Re’em Y, et al. Characterizing long COVID in an international cohort: 7 months of symptoms and their impact. Eclinicalmedicine (2021) 38:101019. doi: 10.1016/j.eclinm.2021.101019
14. Lopez-Leon S, Wegman-Ostrosky T, Perelman C, Sepulveda R, Rebolledo PA, Cuapio A, et al. More than 50 long-term effects of COVID-19: A systematic review and meta-analysis. Sci Rep-uk (2021) 11:16144. doi: 10.1038/s41598-021-95565-8
15. Giszas B, Trommer S, Schüßler N, Rodewald A, Besteher B, Bleidorn J, et al. Post-COVID-19 condition is not only a question of persistent symptoms: structured screening including health-related quality of life reveals two separate clusters of post-COVID. Infection (2022), 1–13. doi: 10.1007/s15010-022-01886-9
16. Bellan M, Baricich A, Patrucco F, Zeppegno P, Gramaglia C, Balbo PE, et al. Long-term sequelae are highly prevalent one year after hospitalization for severe COVID-19. Sci Rep-uk (2021) 11:22666. doi: 10.1038/s41598-021-01215-4
17. Huang L, Yao Q, Gu X, Wang Q, Ren L, Wang Y, et al. 1-year outcomes in hospital survivors with COVID-19: A longitudinal cohort study. Lancet (2021) 398:747–58. doi: 10.1016/s0140-6736(21)01755-4
18. Haunhorst S, Bloch W, Wagner H, Ellert C, Krüger K, Vilser DC, et al. Long COVID: a narrative review of the clinical aftermaths of COVID-19 with a focus on the putative pathophysiology and aspects of physical activity. Oxf Open Immunol (2022) 3:iqac006. doi: 10.1093/oxfimm/iqac006
19. Whitaker M, Elliott J, Chadeau-Hyam M, Riley S, Darzi A, Cooke G, et al. Persistent COVID-19 symptoms in a community study of 606,434 people in England. Nat Commun (2022) 13:1957. doi: 10.1038/s41467-022-29521-z
20. Bowe B, Xie Y, Al-Aly Z. Acute and postacute sequelae associated with SARS-CoV-2 reinfection. Nat Med (2022) 28:1–8. doi: 10.1038/s41591-022-02051-3
21. Merad M, Blish CA, Sallusto F, Iwasaki A. He immunology and immunopathology of COVID-19. Sci New York N Y (2022) 375:1122–7. doi: 10.1126/science.abm8108
22. Sun B, Tang N, Peluso MJ, Iyer NS, Torres L, Donatelli JL, et al. Characterization and biomarker analyses of post-COVID-19 complications and neurological manifestations. Cells (2021) 10:386. doi: 10.3390/cells10020386
23. Peluso MJ, Lu S, Tang AF, Durstenfeld MS, Ho H, Goldberg SA, et al. Markers of immune activation and inflammation in individuals with postacute sequelae of severe acute respiratory syndrome coronavirus 2 infection. J Infect Dis (2021) 11:1839–48. doi: 10.1093/infdis/jiab490
24. Schultheiß C, Willscher E, Paschold L, Gottschick C, Klee B, Henkes S-S, et al. The IL-1β, IL-6, and TNF cytokine triad is associated with post-acute sequelae of COVID-19. Cell Rep Med (2022) 3:100663. doi: 10.1016/j.xcrm.2022.100663
25. Wallukat G, Hohberger B, Wenzel K, Fürst J, Schulze-Rothe S, Wallukat A, et al. Functional autoantibodies against g-protein coupled receptors in patients with persistent long-COVID-19 symptoms. J Transl Autoimmun (2021) 4:100100. doi: 10.1016/j.jtauto.2021.100100
26. Brodin P, Casari G, Townsend L, O’Farrelly C, Tancevski I, Löffler-Ragg J, et al. Studying severe long COVID to understand post-infectious disorders beyond COVID-19. Nat Med (2022) 28:879–82. doi: 10.1038/s41591-022-01766-7
27. Fontenot JD, Gavin MA, Rudensky AY. Foxp3 programs the development and function of CD4+CD25+ regulatory T cells. Nat Immunol (2003) 4:330–6. doi: 10.1038/ni904
28. Sakaguchi S, Yamaguchi T, Nomura T, Ono M. Regulatory T cells and immune tolerance. Cell (2008) 133:775–87. doi: 10.1016/j.cell.2008.05.009
29. Belkaid Y. Regulatory T cells and infection: a dangerous necessity. Nat Rev Immunol (2007) 7:875–88. doi: 10.1038/nri2189
30. Proschinger S, Winker M, Joisten N, Bloch W, Palmowski J, Zimmer P. The effect of exercise on regulatory T cells: A systematic review of human and animal studies with future perspectives and methodological recommendations. Exerc Immunol Rev (2021) 27:142–66.
31. Klein L, Robey EA, Hsieh C-S. Central CD4+ T cell tolerance: deletion versus regulatory T cell differentiation. Nat Rev Immunol (2019) 19:7–18. doi: 10.1038/s41577-018-0083-6
32. Sakaguchi S, Mikami N, Wing JB, Tanaka A, Ichiyama K, Ohkura N. Regulatory T cells and human disease. Annu Rev Immunol (2020) 38:1–26. doi: 10.1146/annurev-immunol-042718-041717
33. Sakaguchi S, Miyara M, Costantino CM, Hafler DA. FOXP3+ regulatory T cells in the human immune system. Nat Rev Immunol (2010) 10:490–500. doi: 10.1038/nri2785
34. Rosenblum MD, Way SS, Abbas AK. Regulatory T cell memory. Nat Rev Immunol (2016) 16:90–101. doi: 10.1038/nri.2015.1
35. Vignali DAA, Collison LW, Workman CJ. How regulatory T cells work. Nat Rev Immunol (2008) 8:523–32. doi: 10.1038/nri2343
36. Dominguez-Villar M, Hafler DA. Regulatory T cells in autoimmune disease. Nat Immunol (2018) 19:665–73. doi: 10.1038/s41590-018-0120-4
37. Miyara M, Gorochov G, Ehrenstein M, Musset L, Sakaguchi S, Amoura Z. Human FoxP3+ regulatory T cells in systemic autoimmune diseases. Autoimmun Rev (2011) 10:744–55. doi: 10.1016/j.autrev.2011.05.004
38. Wang H, Wang Z, Cao W, Wu Q, Yuan Y, Zhang X. Regulatory T cells in COVID-19. Aging Dis (2021) 12:1545–53. doi: 10.14336/ad.2021.0709
39. Wang Y, Zheng J, Islam MS, Yang Y, Hu Y, Chen X. The role of CD4+FoxP3+ regulatory T cells in the immunopathogenesis of COVID-19: implications for treatment. Int J Biol Sci (2021) 17:1507–20. doi: 10.7150/ijbs.59534
40. Rahimzadeh M, Naderi N. Toward an understanding of regulatory T cells in COVID-19: A systematic review. J Med Virol (2021) 93:4167–81. doi: 10.1002/jmv.26891
41. Alahyari S, Rajaeinejad M, Jalaeikhoo H, Amani D. Regulatory T cells in immunopathogenesis and severity of COVID-19: A systematic review. Arch Iran Med (2021) 25:127–32. doi: 10.34172/aim.2022.22
42. Madonna G, Sale S, Capone M, Falco CD, Santocchio V, Matola TD, et al. Clinical outcome prediction in COVID-19 patients by lymphocyte subsets analysis and monocytes’ iTNF-α expression. Biology (2021) 10:735. doi: 10.3390/biology10080735
43. Sami R, Fathi F, Eskandari N, Ahmadi M, ArefNezhad R, Motedayyen H. Characterizing the immune responses of those who survived or succumbed to COVID-19: Can immunological signatures predict outcome? Cytokine (2021) 140:155439. doi: 10.1016/j.cyto.2021.155439
44. Sadeghi A, Tahmasebi S, Mahmood A, Kuznetsova M, Valizadeh H, Taghizadieh A, et al. Th17 and treg cells function in SARS-CoV2 patients compared with healthy controls. J Cell Physiol (2021) 236:2829–39. doi: 10.1002/jcp.30047
45. Caldrer S, Mazzi C, Bernardi M, Prato M, Ronzoni N, Rodari P, et al. Regulatory T cells as predictors of clinical course in hospitalised COVID-19 patients. Front Immunol (2021) 12:789735. doi: 10.3389/fimmu.2021.789735
46. Kaya H, Usuda D. Duration from onset to discharge is longer in COVID-19 patients with low blood regulatory T-cell counts. J Med Virol (2022) 94:840–1. doi: 10.1002/jmv.27401
47. Kalfaoglu B, Almeida-Santos J, Tye CA, Satou Y, Ono M. T-Cell hyperactivation and paralysis in severe COVID-19 infection revealed by single-cell analysis. Front Immunol (2020) 11:589380. doi: 10.3389/fimmu.2020.589380
48. Abdelhafiz AS, Fouad MA, Sayed-Ahmed MM, Kamel MM, Ali A, Fouda M, et al. Upregulation of FOXP3 is associated with severity of hypoxia and poor outcomes in COVID-19 patients. Virology (2021) 563:74–81. doi: 10.1016/j.virol.2021.08.012
49. Mohebbi SR, Baghaei K, Rostami-Nejad M, Mojarad EN, Mirjalali H, Yadegar A, et al. Significant changes of CD4, FOXP3, CD25, and IL6 expression level in Iranian COVID-19 patients. Gastroenterol Hepatol Bed Bench (2020) 13:388–92. doi: 10.22037/ghfbb.v13i4.2157
50. Choutka J, Jansari V, Hornig M, Iwasaki A. Unexplained post-acute infection syndromes. Nat Med (2022) 28:911–23. doi: 10.1038/s41591-022-01810-6
51. Tricco AC, Lillie E, Zarin W, O’Brien KK, Colquhoun H, Levac D, et al. PRISMA extension for scoping reviews (PRISMA-ScR): Checklist and explanation. Ann Intern Med (2018) 169:467–73. doi: 10.7326/m18-0850
52. Galván-Peña S, Leon J, Chowdhary K, Michelson DA, Vijaykumar B, Yang L, et al. Profound treg perturbations correlate with COVID-19 severity. Proc Natl Acad Sci (2021) 118:e2111315118. doi: 10.1073/pnas.2111315118
53. Garcia-Gasalla M, Berman-Riu M, Pons J, Rodríguez A, Iglesias A, Martínez-Pomar N, et al. Hyperinflammatory state and low T1 adaptive immune response in severe and critical acute COVID-19 patients. Front Med (2022) 9:828678. doi: 10.3389/fmed.2022.828678
54. Hoffmann AD, Weinberg SE, Swaminathan S, Chaudhuri S, Mubarak HF, Schipma MJ, et al. Unique molecular signatures sustained in circulating monocytes and regulatory T cells in convalescent COVID-19 patients. Biorxiv (2022). doi: 10.1101/2022.03.26.485922
55. Khesht AMS, Karpisheh V, Saeed BQ, Zekiy AO, Yapanto LM, Afjadi MN, et al. Different T cell related immunological profiles in COVID-19 patients compared to healthy controls. Int Immunopharmacol (2021) 97:107828. doi: 10.1016/j.intimp.2021.107828
56. Kostopoulos IV, Orologas-Stavrou N, Rousakis P, Panteli C, Ntanasis-Stathopoulos I, Charitaki I, et al. Recovery of innate immune cells and persisting alterations in adaptive immunity in the peripheral blood of convalescent plasma donors at eight months post SARS-CoV-2 infection. Microorg (2021) 9:546. doi: 10.3390/microorganisms9030546
57. Orologas-Stavrou N, Politou M, Rousakis P, Kostopoulos IV, Ntanasis-Stathopoulos I, Jahaj E, et al. Peripheral blood immune profiling of convalescent plasma donors reveals alterations in specific immune subpopulations even at 2 months post SARS-CoV-2 infection. Viruses (2020) 13:26. doi: 10.3390/v13010026
58. Kratzer B, Trapin D, Ettel P, Körmöczi U, Rottal A, Tuppy F, et al. Immunological imprint of COVID-19 on human peripheral blood leukocyte populations. Allergy (2021) 76:751–65. doi: 10.1111/all.14647
59. Liu J, Yang X, Wang H, Li Z, Deng H, Liu J, et al. Analysis of the long-term impact on cellular immunity in COVID-19-Recovered individuals reveals a profound NKT cell impairment. Mbio (2021) 12:e00085-21. doi: 10.1128/mbio.00085-21
60. Petrara MR, Bonfante F, Costenaro P, Cantarutti A, Carmona F, Ruffoni E, et al. Asymptomatic and mild SARS-CoV-2 infections elicit lower immune activation and higher specific neutralizing antibodies in children than in adults. Front Immunol (2021) 12:741796. doi: 10.3389/fimmu.2021.741796
61. Rajamanickam A, Kumar NP, Pandiaraj AN, Selvaraj N, Munisankar S, Renji RM, et al. Characterization of memory T cell subsets and common γ–chain cytokines in convalescent COVID-19 individuals. J Leukocyte Biol (2022) 112:201–12. doi: 10.1002/jlb.5cova0721-392rr
62. Ryan FJ, Hope CM, Masavuli MG, Lynn MA, Mekonnen ZA, Yeow AEL, et al. Long-term perturbation of the peripheral immune system months after SARS-CoV-2 infection. BMC Med (2022) 20:26. doi: 10.1186/s12916-021-02228-6
63. Shuwa HA, Shaw TN, Knight SB, Wemyss K, McClure FA, Pearmain L, et al. Alterations in T and b cell function persist in convalescent COVID-19 patients. Med (2021) 2:720–735.e4. doi: 10.1016/j.medj.2021.03.013
64. Taeschler P, Adamo S, Deng Y, Cervia C, Zurbuchen Y, Chevrier S, et al. T-Cell recovery and evidence of persistent immune activation 12 months after severe COVID-19. Allergy (2022) 77:10. doi: 10.1111/all.15372
65. Wiech M, Chroscicki P, Swatler J, Stepnik D, Biasi SD, Hampel M, et al. Remodeling of T cell dynamics during long COVID is dependent on severity of SARS-CoV-2 infection. Front Immunol (2022) 13:886431. doi: 10.3389/fimmu.2022.886431
66. Galán M, Vigón L, Fuertes D, Murciano-Antón MA, Casado-Fernández G, Domínguez-Mateos S, et al. Persistent overactive cytotoxic immune response in a Spanish cohort of individuals with long-COVID: Identification of diagnostic biomarkers. Front Immunol (2022) 13:848886. doi: 10.3389/fimmu.2022.848886
67. Patterson BK, Guevara-Coto J, Yogendra R, Francisco EB, Long E, Pise A, et al. Immune-based prediction of COVID-19 severity and chronicity decoded using machine learning. Front Immunol (2021) 12:700782. doi: 10.3389/fimmu.2021.700782
68. Utrero-Rico A, Ruiz-Ruigómez M, Laguna-Goya R, Arrieta-Ortubay E, Chivite-Lacaba M, González-Cuadrado C, et al. A short corticosteroid course reduces symptoms and immunological alterations underlying long-COVID. Biomed (2021) 9:1540. doi: 10.3390/biomedicines9111540
69. Shi W, Liu X, Cao Q, Ma P, Le W, Xie L, et al. High-dimensional single-cell analysis reveals the immune characteristics of COVID-19. Am J Physiol-lung C (2021) 320:L84–98. doi: 10.1152/ajplung.00355.2020
70. Burnett CE, Okholm TLH, Tenvooren I, Marquez DM, Tamaki S, Sandoval PM, et al. Mass cytometry reveals a conserved immune trajectory of recovery in hospitalized COVID-19 patients. Immunity (2022) 55:1284–1298.e3. doi: 10.1016/j.immuni.2022.06.004
71. Yeo JG, Leong JY, Tay SH, Nadua KD, Anderson DE, Lim AJM, et al. A virus-specific immune rheostat in the immunome of patients recovering from mild COVID-19. Front Immunol (2021) 12:674279. doi: 10.3389/fimmu.2021.674279
72. Chen G, Wu D, Guo W, Cao Y, Huang D, Wang H, et al. Clinical and immunological features of severe and moderate coronavirus disease 2019. J Clin Invest (2020) 130:2620–9. doi: 10.1172/jci137244
73. Liu Z, Long W, Tu M, Chen S, Huang Y, Wang S, et al. Lymphocyte subset (CD4+, CD8+) counts reflect the severity of infection and predict the clinical outcomes in patients with COVID-19. J Infection (2020) 81:318–56. doi: 10.1016/j.jinf.2020.03.054
74. Huang W, Berube J, McNamara M, Saksena S, Hartman M, Arshad T, et al. Lymphocyte subset counts in COVID-19 patients: A meta-analysis. Cytom Part A (2020) 97:772–6. doi: 10.1002/cyto.a.24172
75. Song J-W, Zhang C, Fan X, Meng F-P, Xu Z, Xia P, et al. Immunological and inflammatory profiles in mild and severe cases of COVID-19. Nat Commun (2020) 11:3410. doi: 10.1038/s41467-020-17240-2
76. Wang F, Hou H, Luo Y, Tang G, Wu S, Huang M, et al. The laboratory tests and host immunity of COVID-19 patients with different severity of illness. JCI Insight (2020) 5:e137799. doi: 10.1172/jci.insight.137799
77. Qin C, Zhou L, Hu Z, Zhang S, Yang S, Tao Y, et al. Dysregulation of immune response in patients with coronavirus 2019 (COVID-19) in wuhan, China. Clin Infect Dis Off Publ Infect Dis Soc Am (2020) 71:762–8. doi: 10.1093/cid/ciaa248
78. Segundo DS, de las RFA, Lamadrid-Perojo P, Comins-Boo A, González-Rico C, Alonso-Peña M, et al. Innate and adaptive immune assessment at admission to predict clinical outcome in COVID-19 patients. Biomed (2021) 9:917. doi: 10.3390/biomedicines9080917
79. Adamo S, Chevrier S, Cervia C, Zurbuchen Y, Raeber ME, Yang L, et al. Profound dysregulation of T cell homeostasis and function in patients with severe COVID-19. Allergy (2021) 76:2866–81. doi: 10.1111/all.14866
80. Weinhold M, Shimabukuro-Vornhagen A, Franke A, Theurich S, Wahl P, Hallek M, et al. Physical exercise modulates the homeostasis of human regulatory T cells. J Allergy Clin Immunol (2016) 137:1607–1610.e8. doi: 10.1016/j.jaci.2015.10.035
81. Ohkura N, Yasumizu Y, Kitagawa Y, Tanaka A, Nakamura Y, Motooka D, et al. Regulatory T cell-specific epigenomic region variants are a key determinant of susceptibility to common autoimmune diseases. Immunity (2020) 52:1119–1132.e4. doi: 10.1016/j.immuni.2020.04.006
82. Ohkura N, Sakaguchi S. Transcriptional and epigenetic basis of treg cell development and function: its genetic anomalies or variations in autoimmune diseases. Cell Res (2020) 30:465–74. doi: 10.1038/s41422-020-0324-7
83. Kappelmann N, Dantzer R, Khandaker GM. Interleukin-6 as potential mediator of long-term neuropsychiatric symptoms of COVID-19. Psychoneuroendocrino (2021) 131:105295. doi: 10.1016/j.psyneuen.2021.105295
84. Bettelli E, Carrier Y, Gao W, Korn T, Strom TB, Oukka M, et al. Reciprocal developmental pathways for the generation of pathogenic effector TH17 and regulatory T cells. Nature (2006) 441:235–8. doi: 10.1038/nature04753
85. Gutiérrez-Bautista JF, Rodriguez-Nicolas A, Rosales-Castillo A, Jiménez P, Garrido F, Anderson P, et al. Negative clinical evolution in COVID-19 patients is frequently accompanied with an increased proportion of undifferentiated Th cells and a strong underrepresentation of the Th1 subset. Front Immunol (2020) 11:596553. doi: 10.3389/fimmu.2020.596553
86. Tomo S, Banerjee M, Karli S, Purohit P, Mitra P, Sharma P, et al. Assessment of DHEAS, cortisol, and DHEAS/cortisol ratio in patients with COVID-19: a pilot study. Hormones Athens Greece (2022) 21:515–8. doi: 10.1007/s42000-022-00382-x
87. Sarohan AR, Kızıl M, İnkaya AÇ, Mahmud S, Akram M, Cen O. A novel hypothesis for COVID-19 pathogenesis: Retinol depletion and retinoid signaling disorder. Cell Signal (2021) 87:110121. doi: 10.1016/j.cellsig.2021.110121
88. Suvvari TK, Kutikuppala LVS, Tsagkaris C, Corriero AC, Kandi V. Post-COVID-19 complications: Multisystemic approach. J Med Virol (2021) 93:6451–5. doi: 10.1002/jmv.27222
89. Zarei M, Bose D, Nouri-Vaskeh M, Tajiknia V, Zand R, Ghasemi M. Long-term side effects and lingering symptoms post COVID-19 recovery. Rev Med Virol (2021) 23:e2289. doi: 10.1002/rmv.2289
90. Ståhlberg M, Reistam U, Fedorowski A, Villacorta H, Horiuchi Y, Bax J, et al. Post-COVID-19 tachycardia syndrome: A distinct phenotype of post-acute COVID-19 syndrome. Am J Med (2021) 134:1451–6. doi: 10.1016/j.amjmed.2021.07.004
91. Afrin LB, Weinstock LB, Molderings GJ. Covid-19 hyperinflammation and post-Covid-19 illness may be rooted in mast cell activation syndrome. Int J Infect Dis (2020) 100:327–32. doi: 10.1016/j.ijid.2020.09.016
92. Sugimoto K, Ikeda F, Stadanlick J, Nunes FA, Alter HJ, Chang K. Suppression of HCV-specific T cells without differential hierarchy demonstrated ex vivo in persistent HCV infection. Hepatology (2003) 38:1437–48. doi: 10.1016/j.hep.2003.09.026
93. Belkaid Y, Rouse BT. Natural regulatory T cells in infectious disease. Nat Immunol (2005) 6:353–60. doi: 10.1038/ni1181
94. Boyer O, Saadoun D, Abriol J, Dodille M, Piette J-C, Cacoub P, et al. CD4+CD25+ regulatory T-cell deficiency in patients with hepatitis c-mixed cryoglobulinemia vasculitis. Blood (2004) 103:3428–30. doi: 10.1182/blood-2003-07-2598
95. Acosta-Ampudia Y, Monsalve DM, Rojas M, Rodríguez Y, Zapata E, Ramírez-Santana C, et al. Persistent autoimmune activation and proinflammatory state in post-coronavirus disease 2019 syndrome. J Infect Dis (2022) 225:2155–62. doi: 10.1093/infdis/jiac017
96. Seeßle J, Waterboer T, Hippchen T, Simon J, Kirchner M, Lim A, et al. Persistent symptoms in adult patients 1 year after coronavirus disease 2019 (COVID-19): A prospective cohort study. Clin Infect Dis Off Publ Infect Dis Soc Am (2021) 74:1191–8. doi: 10.1093/cid/ciab611
97. Rojas M, Rodríguez Y, Acosta-Ampudia Y, Monsalve DM, Zhu C, Li Q-Z, et al. Autoimmunity is a hallmark of post-COVID syndrome. J Transl Med (2022) 20:129. doi: 10.1186/s12967-022-03328-4
98. Hohberger B, Harrer T, Mardin C, Kruse F, Hoffmanns J, Rogge L, et al. Case report: Neutralization of autoantibodies targeting G-Protein-Coupled receptors improves capillary impairment and fatigue symptoms after COVID-19 infection. Front Med (2021) 8:754667. doi: 10.3389/fmed.2021.754667
99. Reuken PA, Stallmach A, Pletz MW, Brandt C, Andreas N, Hahnfeld S, et al. Severe clinical relapse in an immunocompromised host with persistent SARS-CoV-2 infection. Leukemia (2021) 35:920–3. doi: 10.1038/s41375-021-01175-8
100. Choi B, Choudhary MC, Regan J, Sparks JA, Padera RF, Qiu X, et al. Persistence and evolution of SARS-CoV-2 in an immunocompromised host. New Engl J Med (2020) 383:NEJMc2031364. doi: 10.1056/nejmc2031364
101. Arostegui D, Castro K, Schwarz S, Vaidy K, Rabinowitz S, Wallach T. Persistent SARS-CoV-2 nucleocapsid protein presence in the intestinal epithelium of a pediatric patient 3 months after acute infection. Jpgn Rep (2021) 3:e152. doi: 10.1097/pg9.0000000000000152
102. Ceulemans LJ, Khan M, Yoo S-J, Zapiec B, Gerven LV, Slambrouck JV, et al. Persistence of SARS-CoV-2 RNA in lung tissue after mild COVID-19. Lancet Respir Med (2021) 9:e78–9. doi: 10.1016/s2213-2600(21)00240-x
103. Swank Z, Senussi Y, Alter G, Walt DR. Persistent circulating SARS-CoV-2 spike is associated with post-acute COVID-19 sequelae. Medrxiv (2022) 2022:6. doi: 10.1101/2022.06.14.22276401
104. Tejerina F, Catalan P, Rodriguez-Grande C, Adan J, Rodriguez-Gonzalez C, Muñoz P, et al. Post-COVID-19 syndrome. SARS-CoV-2 RNA detection in plasma, stool, and urine in patients with persistent symptoms after COVID-19. BMC Infect Dis (2022) 22:211. doi: 10.1186/s12879-022-07153-4
105. Viszlayová D, Sojka M, Dobrodenková S, Szabó S, Bilec O, Turzová M, et al. SARS-CoV-2 RNA in the cerebrospinal fluid of a patient with long COVID. Ther Adv Infect Dis (2021) 8:20499361211048572. doi: 10.1177/20499361211048572
106. Yang B, Fan J, Huang J, Guo E, Fu Y, Liu S, et al. Clinical and molecular characteristics of COVID-19 patients with persistent SARS-CoV-2 infection. Nat Commun (2021) 12:3501. doi: 10.1038/s41467-021-23621-y
107. Yang J, Zhong M, Hong K, Yang Q, Zhang E, Zhou D, et al. Characteristics of T-cell responses in COVID-19 patients with prolonged SARS-CoV-2 positivity – a cohort study. Clin Transl Immunol (2021) 10:e1259. doi: 10.1002/cti2.1259
108. Tang X, Sun R, Ge W, Mao T, Qian L, Huang C, et al. Enhanced inflammation and suppressed adaptive immunity in COVID-19 with prolonged RNA shedding. Cell Discovery (2022) 8:70. doi: 10.1038/s41421-022-00441-y
109. Weinberg SE, Singer BD, Steinert EM, Martinez CA, Mehta MM, Martínez-Reyes I, et al. Mitochondrial complex III is essential for suppressive function of regulatory T cells. Nature (2019) 565:495–9. doi: 10.1038/s41586-018-0846-z
110. Galgani M, Rosa VD, Cava AL, Matarese G. Role of metabolism in the immunobiology of regulatory T cells. J Immunol (2016) 197:2567–75. doi: 10.4049/jimmunol.1600242
111. Díaz-Resendiz KJG, Benitez-Trinidad AB, Covantes-Rosales CE, Toledo-Ibarra GA, Ortiz-Lazareno PC, Girón-Pérez DA, et al. Loss of mitochondrial membrane potential (ΔΨ m) in leucocytes as post-COVID-19 sequelae. J Leukocyte Biol (2022) 112:23–9. doi: 10.1002/jlb.3ma0322-279rrr
112. de Boer E, Petrache I, Goldstein NM, Olin JT, Keith RC, Modena B, et al. Decreased fatty acid oxidation and altered lactate production during exercise in patients with post-acute COVID-19 syndrome. Am J Resp Crit Care (2021) 205:126–9. doi: 10.1164/rccm.202108-1903le
113. Burtscher J, Cappellano G, Omori A, Koshiba T, Millet GP. Mitochondria: In the cross fire of SARS-CoV-2 and immunity. Iscience (2020) 23:101631. doi: 10.1016/j.isci.2020.101631
114. Paul BD, Lemle MD, Komaroff AL, Snyder SH. Redox imbalance links COVID-19 and myalgic encephalomyelitis/chronic fatigue syndrome. Proc Natl Acad Sci (2021) 118:e2024358118. doi: 10.1073/pnas.2024358118
115. Korn T, Reddy J, Gao W, Bettelli E, Awasthi A, Petersen TR, et al. Myelin-specific regulatory T cells accumulate in the CNS but fail to control autoimmune inflammation. Nat Med (2007) 13:423–31. doi: 10.1038/nm1564
116. Chow Z, Banerjee A, Hickey MJ. Controlling the fire — tissue-specific mechanisms of effector regulatory T-cell homing. Immunol Cell Biol (2015) 93:355–63. doi: 10.1038/icb.2014.117
117. Wang L, Zhao L, Lv J, Yin Q, Liang X, Chu Y, et al. BLT1-dependent alveolar recruitment of CD4+CD25+ Foxp3+ regulatory T cells is important for resolution of acute lung injury. Am J Resp Crit Care (2012) 186:989–98. doi: 10.1164/rccm.201202-0261oc
118. Burzyn D, Kuswanto W, Kolodin D, Shadrach JL, Cerletti M, Jang Y, et al. A special population of regulatory T cells potentiates muscle repair. Cell (2013) 155:1282–95. doi: 10.1016/j.cell.2013.10.054
119. Raman B, Bluemke DA, Lüscher TF, Neubauer S. Long COVID: post-acute sequelae of COVID-19 with a cardiovascular focus. Eur Heart J (2022) 43:1157–72. doi: 10.1093/eurheartj/ehac031
120. Puntmann VO, Carerj ML, Wieters I, Fahim M, Arendt C, Hoffmann J, et al. Outcomes of cardiovascular magnetic resonance imaging in patients recently recovered from coronavirus disease 2019 (COVID-19). JAMA Cardiol (2020) 5:1265–73. doi: 10.1001/jamacardio.2020.3557
121. Fu Z, Tang N, Chen Y, Ma L, Wei Y, Lu Y, et al. CT features of COVID-19 patients with two consecutive negative RT-PCR tests after treatment. Sci Rep-uk (2020) 10:11548. doi: 10.1038/s41598-020-68509-x
122. Eiros R, Barreiro-Perez M, Martin-Garcia A, Almeida J, Villacorta E, Perez-Pons A, et al. Pericarditis and myocarditis long after SARS-CoV-2 infection: a cross-sectional descriptive study in health-care workers. Medrxiv (2020) 2020. doi: 10.1101/2020.07.12.20151316
123. Haslbauer JD, Zinner C, Stalder AK, Schneeberger J, Menter T, Bassetti S, et al. Vascular damage, thromboinflammation, plasmablast activation, T-cell dysregulation and pathological histiocytic response in pulmonary draining lymph nodes of COVID-19. Front Immunol (2021) 12:763098. doi: 10.3389/fimmu.2021.763098
124. Gonçalves-Pereira MH, Santiago L, Ravetti CG, Vassallo PF, Andrade MVM, Vieira MS, et al. Dysfunctional phenotype of systemic and pulmonary regulatory T cells associates with lethal COVID-19 cases. Immunology (2022). doi: 10.1111/imm.13603
125. Ronit A, Berg RMG, Bay JT, Haugaard AK, Ahlström MG, Burgdorf KS, et al. Compartmental immunophenotyping in COVID-19 ARDS: A case series. J Allergy Clin Immunol (2021) 147:81–91. doi: 10.1016/j.jaci.2020.09.009
126. Liechti T, Roederer M. OMIP-060: 30-parameter flow cytometry panel to assess T cell effector functions and regulatory T cells. Cytom Part A (2019) 95:1129–34. doi: 10.1002/cyto.a.23853
127. Santegoets SJAM, Dijkgraaf EM, Battaglia A, Beckhove P, Britten CM, Gallimore A, et al. Monitoring regulatory T cells in clinical samples: consensus on an essential marker set and gating strategy for regulatory T cell analysis by flow cytometry. Cancer Immunol Immunother (2015) 64:1271–86. doi: 10.1007/s00262-015-1729-x
128. Andeweg SP, Vennema H, Veldhuijzen I, Smorenburg N, Schmitz D, Zwagemaker F, et al. Elevated risk of infection with SARS-CoV-2 beta, gamma, and delta variant compared to alpha variant in vaccinated individuals. Sci Transl Med (2022):eabn4338. doi: 10.1126/scitranslmed.abn4338
129. McCallum M, Czudnochowski N, Rosen LE, Zepeda SK, Bowen JE, Walls AC, et al. Structural basis of SARS-CoV-2 omicron immune evasion and receptor engagement. Science (2022) 375:864–8. doi: 10.1126/science.abn8652
130. Cameroni E, Bowen JE, Rosen LE, Saliba C, Zepeda SK, Culap K, et al. Broadly neutralizing antibodies overcome SARS-CoV-2 omicron antigenic shift. Nature (2022) 602:664–70. doi: 10.1038/s41586-021-04386-2
Keywords: regulatory T cells (T reg), COVID-19, SARS-CoV-2, Long Covid, immune system, adaptive immunity
Citation: Haunhorst S, Bloch W, Javelle F, Krüger K, Baumgart S, Drube S, Lemhöfer C, Reuken P, Stallmach A, Müller M, Zielinski CE, Pletz MW, Gabriel HHW and Puta C (2022) A scoping review of regulatory T cell dynamics in convalescent COVID-19 patients – indications for their potential involvement in the development of Long COVID? Front. Immunol. 13:1070994. doi: 10.3389/fimmu.2022.1070994
Received: 15 October 2022; Accepted: 28 November 2022;
Published: 13 December 2022.
Edited by:
Rubeshan Perumal, Centre for the AIDS Programme of Research in South Africa, South AfricaReviewed by:
Waleed Mahallawi, Taibah University, Saudi ArabiaCopyright © 2022 Haunhorst, Bloch, Javelle, Krüger, Baumgart, Drube, Lemhöfer, Reuken, Stallmach, Müller, Zielinski, Pletz, Gabriel and Puta. This is an open-access article distributed under the terms of the Creative Commons Attribution License (CC BY). The use, distribution or reproduction in other forums is permitted, provided the original author(s) and the copyright owner(s) are credited and that the original publication in this journal is cited, in accordance with accepted academic practice. No use, distribution or reproduction is permitted which does not comply with these terms.
*Correspondence: Christian Puta, Y2hyaXN0aWFuLnB1dGFAdW5pLWplbmEuZGU=
Disclaimer: All claims expressed in this article are solely those of the authors and do not necessarily represent those of their affiliated organizations, or those of the publisher, the editors and the reviewers. Any product that may be evaluated in this article or claim that may be made by its manufacturer is not guaranteed or endorsed by the publisher.
Research integrity at Frontiers
Learn more about the work of our research integrity team to safeguard the quality of each article we publish.