- 1Department of Anesthesiology, the First Affiliated Hospital, College of Medicine, Zhejiang University, Hangzhou, Zhejiang, China
- 2Department of Anesthesiology, Shenzhen People’s Hospital, The Second Clinical Medical College, Jinan University, The First Affiliated Hospital, Southern University of Science and Technology, Shenzhen, Guangdong, China
Ischemic stroke is a major cause of death and disability around the world. However, ischemic stroke treatment is currently limited, with a narrow therapeutic window and unsatisfactory post-treatment outcomes. Therefore, it is critical to investigate the pathophysiological mechanisms following ischemic stroke brain injury. Changes in the immunometabolism and endocrine system after ischemic stroke are important in understanding the pathophysiological mechanisms of cerebral ischemic injury. Hormones are biologically active substances produced by endocrine glands or endocrine cells that play an important role in the organism’s growth, development, metabolism, reproduction, and aging. Hormone research in ischemic stroke has made very promising progress. Hormone levels fluctuate during an ischemic stroke. Hormones regulate neuronal plasticity, promote neurotrophic factor formation, reduce cell death, apoptosis, inflammation, excitotoxicity, oxidative and nitrative stress, and brain edema in ischemic stroke. In recent years, many studies have been done on the role of thyroid hormone, growth hormone, testosterone, prolactin, oxytocin, glucocorticoid, parathyroid hormone, and dopamine in ischemic stroke, but comprehensive reviews are scarce. This review focuses on the role of hormones in the pathophysiology of ischemic stroke and discusses the mechanisms involved, intending to provide a reference value for ischemic stroke treatment and prevention.
Introduction
Ischemic stroke is a neurological disorder caused by the disturbance of blood supply to the brain (1). Globally, stroke was the third leading cause of death after neonatal diseases and ischemic heart disease in 2019, accounting for more than half of new strokes (2). Low-income countries bear a greater disease burden than high-income countries (2). From 1990 to 2019, the incidence of ischemic stroke has increased significantly in China (3). Treatment options include tissue plasminogen activator (tPA) and mechanical thrombectomy (MT) (4). However, these are limited by the narrow treatment-time window (4). Endovascular thrombectomy and intravenous thrombolysis (IVT) combined with drug therapy have been popular treatment regimes in recent years (5–7). However, less than 5% of acute ischemic stroke patients receive IVT within the eligible treatment window, and fewer than 100,000 MTs were performed worldwide in 2016 (8). Nevertheless, complications such as cerebral hemorrhage, vessel re-occlusion, and cerebral edema arise after MT (9). Therefore, further research is required on the prevention and treatment of stroke.
Hypothalamus serves as an endocrine organ. It secretes regulatory factors, acts on the pituitary anterior lobe cell, and stimulates the secretion of hormones that control the endocrine glands (10). Hormones transmit information to intracellular by binding to specific receptors inside the cell or on the plasma membrane (11). In the 1970s, there were some reports about the role of dexamethasone in ischemic stroke (12, 13). However, these reports were primarily negative, probably because the concentration of dexamethasone at the site of action was too small to achieve a therapeutic effect (14). Success can only be expected if a sufficiently high dose of dexamethasone is administered immediately after an ischemic attack (14). Since the 1990s, the study of hormones in an ischemic stroke has become popular. Insulin (15), estrogen (16), progesterone (17), testosterone (18), arginine vasopressin (19), and thyroid hormone (20) have been reported successively in ischemic stroke, which gradually fills the gap of hormones in the field of ischemic stroke research. Specific hormonal changes are a risk factor for ischemic stroke (21). Moreover, ischemic stroke can cause hormonal changes (22). Brain damage after stroke results from a complex series of pathophysiological events like excitotoxicity, oxidative and nitrative stress, inflammation, and apoptosis (23). Our study describes the mechanism of the hormones involved in the pathophysiological process of ischemic stroke and gives ideas on the prevention and treatment of ischemic brain injury.
Thyroid hormone and ischemic stroke
Meta-analysis studies associate the thyroid hormone with the prognosis of ischemic stroke (24). Patients with low initial triiodothyronine (T3) are linked with worse acute ischemic stroke outcomes (25). At the same time, serum thyroid stimulating hormone levels are negatively correlation to the risk of post-stroke patient fatigue in the acute phase and follow-up assessment (26). Thyroid hormone improves neurological outcomes after experimental stroke through different pathways, such as being anti-edema (27), promoting the expression of neurotrophic factors (28), regulating neuronal plasticity (29), and increasing adenosine triphosphate (ATP) production (30). Simultaneously, reversing T3 (rT3) increases neuronal survival after ischemia-reperfusion injury in rat models since it reduces brain metabolism (31). Published review has demonstrated that thyroid hormone-regulated genes are associated with neuronal plasticity after ischemic stroke (32). Recent data suggest that astrocytes are sensitive to T3, and their response to T3 is related to their maturity, for a total of 117 genes are regulated by T3 transcription (33, 34). Astrocytes play a significant role in thyroid hormone deiodination (35), a process affected by ischemic stroke (36). Type 2 iodothyronine deiodinase (D2) is the primary source of plasma T3 in normal thyroid function (37). In the astrocytes, D2 deiodinases T4 to form T3, exerting thyroid hormone effects on other nerve cells in the brain (38). The D2 mRNA expression was upregulated in the ipsilateral striatum after 6h of rat middle cerebral artery occlusion and disappeared after 24h (36). In the ipsilateral cortex, the D2 mRNA was induced at 6h; increased at 24h and decreased at 72h (36). A similar situation was found in the rat traumatic brain injury, where the astrocytes’ D2 mRNA expression was upregulated (39).
Retrospective studies show that Low T3 predicts poor functional prognosis in patients with acute ischemic stroke and is more significant in the elderly (40, 41). Further, T3 infusion promoted D2 gene expression in risk areas in cardiac ischemia-reperfusion models (42). After T3 infusion, serum T3 levels in the tested risk area are the same as the basal level (42). We hypothesize that ischemic stroke promotes D2 expression in astrocytes, thereby promoting the deiodination of T4 to T3. T3 then promotes D2 expression, forming a positive feedback loop. This cascade contributed to the recovery of T3 levels, and the protective effect of T3 was exerted in ischemic stroke.
Thyroid hormone derivatives and ischemic stroke
3-Iodothyroamine (T1AM) is a derivative of endogenous thyroxine (43). T1AM is derived from the enzymatic digestion and decarboxylation of T4 (43). Studies indicate that T1AM biosynthesis depends on the sodium-iodine transporter and thyroid peroxidase (44). In the mice’s intestinal tissues, T4 forms T1AM by decarboxylation of ornithine decarboxylase and subsequent deiodination (45). In a mouse model, T1AM reduced the infarct size by inducing hypothermia (46). Meanwhile, T1AM was used as an antecedent treatment to induce neuroprotection from subsequent ischemia (46). Hypothermia is believed to be due to peripheral vascular dilation and subsequent heat loss (47). T1AM induced tail vessel dilation in male mice through the hypothalamus signaling pathway (47).
Hypothermia is a feasible treatment for stroke (48). Preclinical studies have recognized the protective role of hypothermia in ischemic stroke (49). Moderate hypothermia reduces the inflammatory response Interleukin 1 beta (IL-1β) and Tumor Necrosis Factors alpha (TNF-α), oxidative stress (50), and energy consumption (51) after an ischemic stroke. Recent progress has been observed in studies involving low temperature combined with other neuroprotective measures (anesthetics, psychotropic agents, antibiotics, oxidative stress scavengers) (52). Reducing the surface temperature to 35°C was possible in conscious patients with acute ischemic stroke, but cooling was associated with the risk of pneumonia (53). Combining intra-arterial recanalization with isotonic saline infusion (4°C) in the ischemic area using an angiographic catheter reduced the ischemic area temperature by at least 2°C; the body temperature decreased slightly (up to 0.3°C) (54). No intracerebral complications associated with hypothermia were observed (54). Intravascular hypothermia circumvented the core hypothermia and reduced the risk of pneumonia associated with systemic hypothermia. Preclinical studies indicate the protection offered by T1AM on ischemic stroke by inducing hypothermia. However, further studies are required to determine the clinical utility.
Growth hormone and ischemic stroke
Additional clinical investigations are required to conclude the effects of growth hormone (GH) on ischemic stroke. Patients with a stroke are at risk for growth hormone deficiency (55). Agonistic analogs of growth hormone-releasing hormone are beneficial in mouse ischemic stroke (56). Low GH is common after severe ischemic stroke patients, and GH may be related to the prognosis of ischemic stroke (57).
Growth hormone improves motor function after ischemic stroke
Growth hormone (GH) has a nutritional effect on the nerves (58). It functioned as an effective neurotrophic factor for the inner ear neurons and significantly increased neurite extension and neuronal branching of rat spiral ganglion cells (59). It also repaired nerves (60). In the chronic denervation injury model, GH showed robust nerve regeneration through axon density, axon diameter, and myelin sheath thickness (61). At the same time, GH improved muscle innervation and reduced muscle atrophy (61). Randomized controlled trials demonstrated that human growth hormone improves quadriceps atrophy and deficiency drop after Anterior Cruciate Ligament (ACL) reconstruction and increases quadriceps strength in patients (62). GH improved motor function after an experimental stroke, as demonstrated by the cylinder and grid walk tests (63). This is associated with GH promoting increased cell proliferation, neurogenesis, synaptic plasticity, and angiogenesis within the peri-infarct region (63). GH also increased insulin growth factor 1 (IGF-1). After GH treatment, a significant positive correlation existed between plasma IGF-1 levels and cylinder task performance (63). In an ischemic stroke rat model, IGF-1 plays multiple roles in increasing sensorimotor function, improving cognitive function, and reducing infarct size (64–66). Patients with higher serum IGF-1 were significantly associated with a lower risk of ischemic stroke (67). Compared with the same shuttle vector, female rats carrying the IGF-1 gene exhibited better sensorimotor function in the early and late acute stages of stroke (68). In conclusion, GH improves motor function after stroke through its neuromuscular nutrition and repair function. Additionally, it improves motor function by increasing IGF-1.
Growth hormone improves cognitive function after ischemic stroke
The prevalence of cognitive impairment in stroke survivors ranges from 20% to 80%, depending on country, ethnicity, and diagnostic basis (69). Stroke was associated with a sharp decline in cognitive performance that accelerated and continued over the next few years (70). At the same time, patients with cognitive impairment have a higher risk of future stroke than those with normal cognitive function (71). Post-stroke cognitive impairment as an independent predictor of ischemic stroke recurrence (72). Hippocampal atrophy was related to cognitive impairment in Alzheimer’s disease (73), Lewy’s dementia (74), small vascular disease (75), type 2 diabetes (76), and Parkinson’s dementia (77). The hippocampal atrophy rate was higher in the stroke participants than in the control group, and the hippocampal atrophy rate was higher in the early stage than in the late stage (78). Also, more severe atrophy was observed in the CA1 region of the hippocampus and caudal hippocampus in ischemic stroke patients (79). However, a study demonstrated that long-term cognitive impairment in ischemic stroke patients was associated with hippocampal deformation, not atrophy (80). Resting-state functional magnetic resonance imaging has shown that reduced hippocampal-subparietal lobule connectivity is associated with cognitive impairment in patients with ischemic stroke (81). In summary, cognitive impairment after ischemic stroke was closely related to the hippocampus.
GH therapy may play a role in improving cognitive function (82). In patients with an isolated growth hormone deficiency, white matter abnormalities in the corpus callosum and corticospinal tracts and reduced thalamic and globus pallidus volumes are associated with deficits in cognitive function and motor function performance (83). In older rats, age-related reductions in growth hormone lead to cognitive decline, partly through changes in short-term hippocampal plasticity (84). GH treatment enhanced the regulation of excitatory synaptic transmission and plasticity in the aged rat hippocampus by activating N-methyl-D-aspartate receptor (NMDAR)-dependent basal synaptic transmission and alpha-amino-3-hydroxy-5-methyl-4-isoxazolepropionate receptor (AMPA-R)-dependent basal synaptic transmission, which altered the course of cognitive decline (85). GH increases the density of dendritic spines in the hippocampus, thus strongly influencing hippocampal plasticity and memory (86, 87). Randomized controlled trials demonstrated the beneficial effects of recombinant human growth hormone on cognitive impairment after stroke (88). Mice treated with GH after a stroke had a more remarkable ability to complete paired associative learning tasks (89). This ability was associated with GH increasing the neurotrophic factors (IGF-1, Vascular endothelial growth factor (VEGF)) and promoting synapses, myelin, and brain vascular network formation (89). GH also increased hippocampal-dependent visual discrimination in male mice after experimental cortical stroke, which was associated with GH stimulation of neural progenitor cell proliferation, increased synaptic plasticity in the hippocampus, and increased plasma IGF-1 levels (90). Thus, GH improved cognitive function after ischemic stroke via the hippocampus.
Sex hormones and ischemic stroke
Testosterone and ischemic stroke
Serum testosterone was reduced after acute ischemic stroke in men, and total testosterone negatively correlated with infarct size (18). Low testosterone levels were associated with an increased risk of ischemic stroke in older men (91, 92) and possibly higher all-cause mortality after acute ischemic stroke (93). Also, anger tendencies and emotional incontinence after ischemic stroke were related to low testosterone levels (94). However, in the pediatric population, increased testosterone elevates the risk of stroke (95). The effect of testosterone on ischemic stroke was age-dependent. Testosterone exacerbated ischemic brain injury in young adult mice, while testosterone supplementation reduced cortical infarction in middle-aged mice (96). This protection was mediated by androgen receptors (AR) and unrelated to the brain aromatase (96). AR expression was reduced after cerebral ischemia, and overexpression of AR reduced the infarct size after ischemic stroke (97). Interestingly, exposure to testosterone during neonatal life in adult male rats increased their resistance to ischemic stroke (98). The upregulated testicular aromatase expression increased the serum estradiol levels, which exerts a protective effect by increasing X-linked apoptosis inhibitors (98). Also, supplementation of testosterone in middle age rats to the normal physiological levels of young male rats reduced infarcts (96).
However, testosterone can be detrimental to ischemic stroke. Dihydrotestosterone (DHT) suppresses peripheral immunity after ischemic stroke (99). DHT eliminates the presence of immature neurons in the ischemic region and reduces the repair of damaged tissue after ischemia (100). More research is required for applying testosterone replacement therapy (TRT) to ischemic stroke (101). In older men with low testosterone levels, TRT increases the risk of cardiovascular events, especially in the first two years of use (102). However, TRT reduced the risk of cardiovascular outcomes in androgen-deficient men during a median follow-up of 3.4 years (103). Further research on testosterone is warranted, including its therapeutic effects on different age groups, the mechanism of its protection, and its role as a prognostic predictor of ischemic stroke.
Estrogen and progestin with ischemic stroke
The Women’s Health Initiative (WHI) showed that estrogen (E) plus progestin (P) increased the risk of ischemic stroke in generally healthy post-menopausal women (104). However, altering the route of hormone administration and the type of hormone may remedy this drawback. Encouraging hormone therapy users to switch from oral to transdermal estrogen and from synthetic to micronized progesterone reduced the risk of ischemic stroke by ≤ 3000 per million hormone therapy users per year (105). Meanwhile, using E and P in combination has progressed in the preclinical study of ischemic stroke. Combined E and P treatment reduced cortical infarct size in rats suffering from ischemic stroke (106–108). Combined E and P treatment inhibited ischemia-induced neuronal apoptosis by suppressing Calpain-1 upregulation and caspase-3 activation in rat cortical infarct areas (109). E plus P also reduced the extracellular glutamate levels by inducing the glutamate transporter protein (glutamate transporter 1 (GLT-1) and amino-acid transporters (EAAT3)) expression in an ischemic stroke rat (110). The neuroprotective role of E and P in stroke may be due to reduced phosphorylation of the heat shock protein 27 (HSP27) in rat ischemic areas (111). 17β-estradiol plus P displayed anti-inflammatory effects by selectively reducing absent in melanoma 2 (AIM2) and NLR family CARD domain-containing protein 4 (NLRC4) inflammasomes in primary cortical astrocytes and microglia after ischemic stroke in rats (112).
After transient middle cerebral artery occlusion in rats, E plus P regulated chemokine-microglia/lymphocyte interactions, a mechanism associated with cytoprotection (113). E plus P attenuated the expression of ischemic stroke-induced proinflammatory chemokines chemokine ligand 2 (CCL2), chemokine ligand 5 (CCL5), and interleukin 6 (IL-6) (113). Moreover, the local expression of microglia/macrophage/lymphocyte markers (ionized calcium -binding adapter molecule 1(Iba-1), cluster of differentiation 8 (CD8), and cluster of differentiation 3 (CD3)) in the penumbra areas was significantly reduced after hormone treatment (113). In a rat model, E plus P indirectly regulated pro-apoptotic and inflammatory gene translation by selectively inhibiting miR-223 and miR-214 and further enhancing miR-375 (114).
Further, few studies report the relation between the estradiol/testosterone ratio and ischemic stroke, and they are less optimistic. Increased estradiol and decreased testosterone levels were associated with acute ischemic stroke in male patients (115). For post-menopausal women with a body mass index < 25 kg/m2, a higher estradiol/testosterone ratio was associated with a significantly higher risk of ischemic stroke among the patients currently treated with exogenous hormones (116).
Oxytocin and ischemic stroke
Clinical studies of oxytocin (OT) use in ischemic stroke are scarce, but experimental studies have robust progression (Figure 1). OT reduces brain damage after experimental stroke (117–119). Compared with the ischemia control group, OT significantly reduced the infarct volume in the cerebral cortex and striatum (117), thus, improving the spatial memory function (118). Meanwhile, OT pretreatment significantly reduced the number of hippocampal neuronal deaths after focal cerebral ischemia (119). The protective effect of OT on brain injury after ischemic stroke was correlated with the increased expression of VEGF, Aquaporin 4 (AQP4), and Brain-derived neurotrophic factor (BDNF) proteins, reduced leakage from the blood-brain barrier (BBB), decreased inflammatory mediators TNF-α and IL-1β, and reduced cell death and apoptosis (117, 118). In addition, OT ameliorated ischemic stroke by attenuating Calpain-1 (117). Calpain-1 and caspase-3 were positively correlated in ischemic stroke, suggesting that down-regulating calpain-1 inhibited apoptosis (109). Calpain-1-specific inhibitor PD151746 promoted phosphorylated signal transducer and activator of transcription 3 (p-STAT3) expression and was auxiliary to the proliferation and functional recovery of neural precursor cells in the subventricular zone after stroke (120).
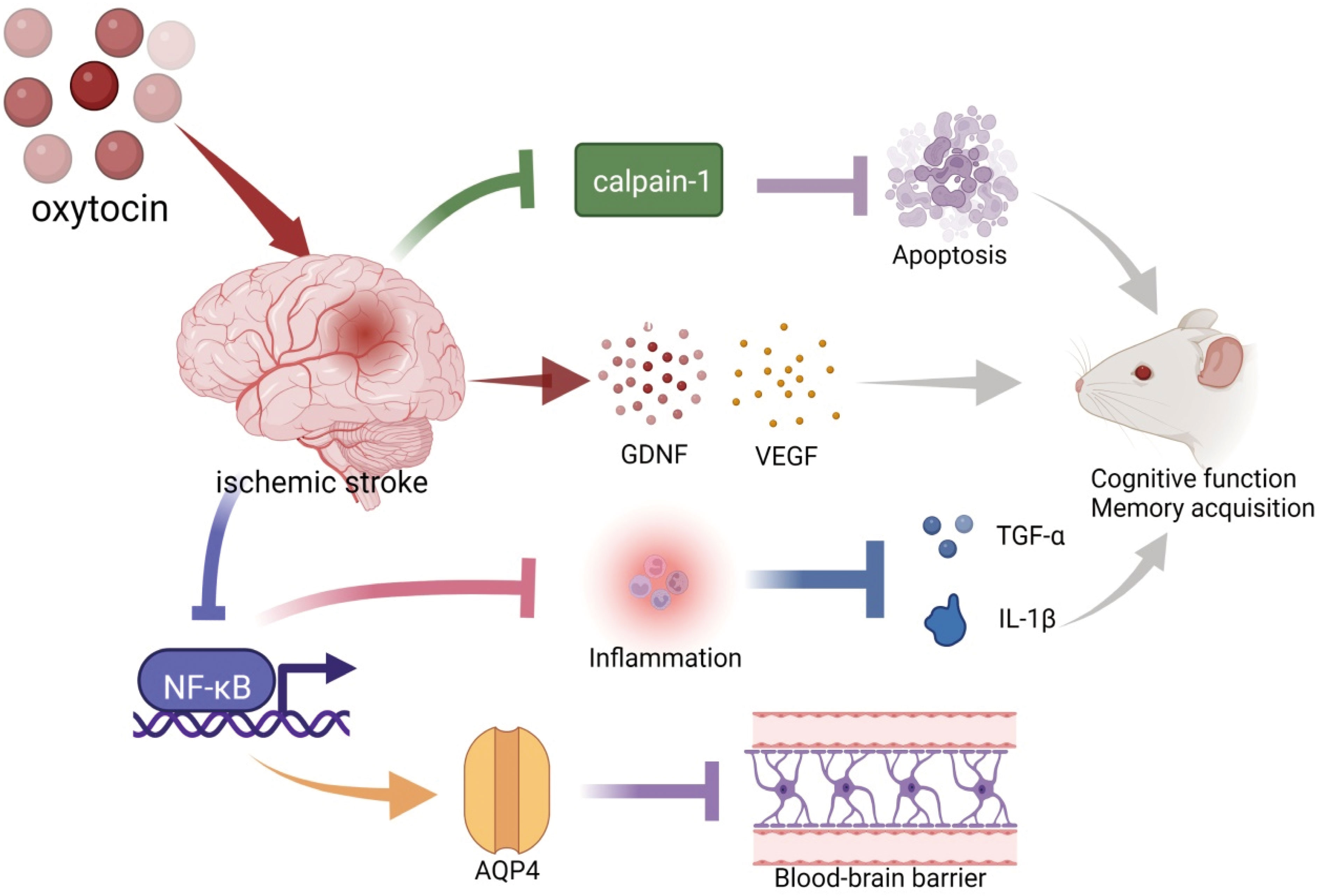
Figure 1 Schematic illustration of oxytocin and ischemic stroke. OT decreased ischemia-induced Caipain-1 overexpression to inhibit apoptosis. Pretreatment with OT before ischemic stroke promoted the expression of BDNF and VEGF. OT suppressed inflammation (TNF-α and IL-1β) by inhibiting the expression of NF-κB. These are beneficial for the recovery of cognitive function after an ischemic stroke. In addition, OT attenuated blood-brain barrier leakage and cerebral hematoma by promoting the expression of AQP4, which may be associated with the inhibition of NF-κB. BDNF, brain-derived neurotrophic factor; VEGF, vascular endothelial growth factor; TNF-α, tumor necrosis factor-α; IL-1β, Interleukin-1 beta; AQP4, Aquaporin 4. The illustration was supported by BioRender (https://biorender.com).
Prolactin and ischemic stroke
Studies on prolactin (PRL) and ischemic stroke are scarce, but reports on brain injury (121) and neuroprotection (122, 123) have seen some advances. PRL mainly exerts neuroprotective effects by inhibiting excitatory toxicity (124, 125) and neuroinflammation (126, 127). In the cerebral ischemia model, PRL reduced the cerebral infarction area and cerebral water content and restored the physiological status (128). Transient ischemic attack increased PRL concentrations and increased plasma PRL levels were significantly linked with platelet P-selectin (129, 130). Platelet surface P-selectin expression was associated with a worsening clinical course in acute ischemic stroke (131). These results suggested that patients with high prolactin levels after ischemic stroke may have a worse prognosis. More research is needed to investigate the prolactin role in ischemic stroke.
Glucocorticoid and ischemic stroke
Many patients have increased cortisol after acute ischemic stroke, which negatively impacts organ function (132). Ischemic injury to neurons in the rat brain was enhanced by exposure to high physiological titers of glucocorticoid (GC) (133). Pre-hospital GC use increased the 30-day mortality in patients with ischemic stroke (134). Also, the current use of GC increased the risk of myocardial infarction and venous thromboembolism in the first year of ischemic stroke (135). However, a clinical study also indicated an improved level of consciousness in patients with acute ischemic stroke associated with cerebral edema after giving dexamethasone (136). GC resistance was associated with poorer functional outcomes after an ischemic stroke (137).
GC and ischemic stroke
Many studies suggest that GC is involved in immune regulation in ischemic stroke (138, 139). Intranasal dexamethasone reduced mortality, neurological deficits, infarct size, blood-brain barrier permeability, inflammatory cell infiltration, and glial activation in mice after ischemic stroke (140). In experimental focal cerebral ischemia, dexamethasone was neuroprotective by inhibiting the inflammation-dependent NF-kB-p65 pathway, including the inhibition of inducible nitric oxide synthase (iNOS), Cyclooxygenase-2 (COX-2), TNF-α, and IL-1β expression (141). At the same time, inhibiting the expression of glucocorticoid receptors (GR) significantly increased the expression of proinflammatory cytokines (IL-6, IL-1β, and TNF-α) and decreased the brain-derived neurotrophic factor/pro-myosin receptor kinase B (BDNF/TrkB) signaling in the mice brain, which can increase the infarct size and worsen neurobehavioral deficits in ischemic stroke (142). However, elevated cortisol levels were negatively correlated with blood lymphocyte counts in 20 patients with acute stroke (143). In mice, stroke-induced glucocorticoid release significantly triggered defective B-lymphocyte production (143). Blocking GR prevented post-ischemic lymphocyte reduction (144). Plasma corticosterone levels were elevated in diabetic mice after ischemic stroke (145). Using glucocorticoid synthesis inhibitors reduced the infarct size and IL-6 expression (145). Glucocorticoids are anti-inflammatory and immunosuppressive. Hence, treating ischemic stroke with glucocorticoids is contradictory and complex. More research is needed to maximize the protection of glucocorticoids in ischemic stroke.
Parathyroid hormone and ischemic stroke
Parathyroid hormone (PTH) and 25-dihydroxyvitamin D levels together can make important contributions to determination of stroke risk (21). PTH levels were elevated in patients with acute ischemic cerebrovascular events (22). In peritoneal dialysis patients, lower serum PTH levels were significantly associated with an increased risk of stroke (146). PTH was beneficial in ischemic stroke. PTH promoted the expression of neuroangiogenesis factors and increased angiogenesis around the infarction after focal cerebral ischemia (147).
Additionally, PTH promoted the migration of bone marrow stem cells (148). Bone marrow-derived endothelial progenitor cells and endothelial stem cells increased in the peripheral blood of stroke mice after PTH treatment (147). These cells highly expressed the migratory chemokine stromal cell derived-factor 1 (SDF-1), which promoted the migration of neuroblasts from the subventricular region to the ischemic cortical region and increased the number of cortical neurons around infarction (147). Meanwhile, parathyroid hormone-related protein (PTHrp) reduced the cortical infarct area in ischemic stroke animals by vasodilating and increasing cerebral blood flow (149). More research is required on parathyroid hormone and ischemic stroke.
Catecholamines and ischemic stroke
Catecholamines have been linked to an increased risk of infection after stroke (150). Catecholamines increase levels of the pro-inflammatory cytokines IL-1β and Interferon-γ (INF-γ) and decrease levels of the anti-inflammatory cytokine Interleukin 10 (IL-10) after experimental stroke, an immunosuppressive state that lowers the threshold for infection and increases the risk of infection (151). Dopamine release occurs in the early stage of ischemia, and the amplitude of dopamine release correlates with the duration of ischemic injury (152). Reperfusion induces more striatal dopamine release (152). Levodopa is a dopamine precursor, and studies have shown that levodopa is expected to enhance motor recovery after stroke (153–159). Levodopa also enhanced post-stroke plasticity (160). The combination of dopamine precursors significantly reduced the infarct size, proinflammatory cytokine levels, oxidative stress levels, and neurological deficits in the striatum of rats with cerebral ischemia-reperfusion injury (161). Meanwhile, amantadine, a drug promoting dopamine release, improved cognitive and functional recovery after a stroke (162).
β-adrenergic receptors and ischemic stroke
Pharmacological inhibition of β-adrenergic receptors, but not steroid inhibition, effectively reduced infection and improved clinical outcomes in experimental stroke (163). In a retrospective series of studies, β-blocker use was associated with reduced risk of early death in patients with ischemic stroke (164). β-blocker was negatively associated with the incidence of nosocomial pneumonia before and during the stroke (165). β1 adrenergic receptor of neutrophils is associated with migration during increased inflammation, and β1 adrenergic receptor blocking improves brain damage by targeting neutrophils (166). The β-blocker carvedilol may protect the ischemic brain in the rat by inhibiting apoptosis and attenuating the expression of TNF-α and IL-1β (167). Interestingly, in stroke models, Augmented β2-adrenergic signaling has also been reported as neuroprotective. Unlike systemic administration, central administration of norepinephrine lowers blood pressure and exerting anti-inflammatory and neuroprotective effects (168). Increased β2-adrenergic signaling after an experimental stroke typically inhibits microglial/monocyte-derived macrophage response and reduces the upregulation of pro-inflammatory and anti-inflammatory cytokines (TNFα and IL-10) (169). In mice, increased β2-adrenergic signaling after stroke inhibited post-stroke pneumonia but increased post-stroke infarct size (170).
Dopamine receptors and ischemic stroke
Cerebral ischemia affects dopamine receptors in the striatum (171, 172) and hippocampus (173). Ischemic dopamine release in the striatum was associated with early transient changes in dopamine receptor-mediated dopamine neurotransmission (172). Cerebral ischemia reduced the number of dopamine D1 receptors (D1R) (171) and also their affinity for receptor ligands (172). Cerebral ischemia slightly affects D2 receptors (D2R) in the striatum for up to seven days (171). Subsequent studies have shown that D2R continued to bind ligands in the first week after cerebral ischemia, declining sharply from day 14 to day 28 (174). These results suggested the critical role of D1R and D2R in the recovery from ischemic stroke.
D1R and ischemic stroke
D1R activation inhibits the excitatory postsynaptic currents in post-ischemic striatal neurons because it activates Cyclic Adenosine Monophosphate (cAMP)-dependent protein A and adenosine A1 receptors (175). Systemic D1R agonists significantly reduced ischemia-induced striatum cell death after ischemia (175). D1R in astrocytes was also associated with GNDF expression. In the transient middle cerebral artery occlusion (tMCAO) model, adding selective D1R agonists increased GNDF expression, while D1R inhibitors significantly reduced GNDF expression (176). After 2h of ischemia stroke in rats, endogenous tissue fibrinogen activator (tPA) increased in the region of BBB injury, and intrastriatal D1R antagonists significantly reduced ischemia-induced endogenous tPA upregulation and BBB injury (177). Experimental stroke in the dorsolateral striatum induced alcohol preference, enhancing glutamatergic energy input to D1-neurons in the dorsomedial striatum (178). Inhibition of D1R mitigated the stroke-induced increment in the self-intake of alcohol (178).
D2R/D3R and ischemic stroke
Resident microglia do not express D2R in healthy brains, but this population expresses D2R after cerebral ischemia (179). Dopamine acts as a regulator of microglial function during neuroinflammation, and the D2R/D3R agonist pramipexole enhances nitrite secretion in response to proinflammatory stimuli (179). The D2R agonist bromocriptine prevented ischemia-induced neuron damage in the gerbil by preserving superoxide dismutase (SOD) (180). In the middle cerebral artery occlusion (MCAO) mouse model, Sino suppresses neuroinflammation after ischemic stroke by upregulating D2R/αB-crystallin (CRYAB) expression (181). Also, agonistic D2R induces neurological recovery in ischemia/reperfusion injury following rats via the mitochondrial pathway (182). Pramipexole inhibited the transfer of cytochrome C from mitochondria to cytosol, thereby inhibiting the mitochondrial permeability transition pore (182). In the tMCAO rat model, Sumanirole repaired mitochondrial dysfunction by reducing mitochondrial reactive oxygen species production, increasing mitochondrial membrane potential and the activity of protective mitochondrial complexes and histological changes, thereby alleviating ischemic injury (183). Meanwhile, Sumanirole reduced the infarct size, restored behavioral changes, and promoted neuronal survival (183). D2/D3 receptor activation was associated with ischemic preconditioning (IPC), and IPC was beneficial against ischemic reperfusion injury in mice (184). However, compared with D1R on astrocytes, agonistic D2R on astrocytes did not affect the GNDF levels (176).
Conclusion and future direction
Abnormal hormone levels are typical after an ischemic stroke. Growth hormone and testosterone levels decrease while prolactin, corticosterone, parathyroid hormone, and dopamine levels increase. Also, hormone changes have an effect on the prognosis of ischemic stroke (Table 1). Hormones are involved in various pathophysiological mechanisms of ischemic stroke, including cerebral edema formation, neuroplasticity regulation, neurotrophic factor formation, cell death reduction, apoptosis, inflammation, and oxidative stress (Tables 2 and 3). It is essential to understand the role of hormones in the pathophysiology of brain injury in ischemic stroke for preventing and treating ischemic stroke.
Hormones, hormone derivatives, hormone receptors, and hormone combinations should be the focus of future studies. Hormone research has significantly advanced in preclinical studies of ischemic stroke, and most results are beneficial. However, the use of hormones in the clinical management of ischemic stroke is scarce, and the available results present a contradictory picture because of the complexity of the brain injury process in ischemic stroke. Recently, pyroptosis have attracted more and more attention in the study of cerebral ischemia (185). However, there are few studies on the relationship between hormones and pyroptosis in ischemic stroke. Studies have shown that hormone enhances the therapeutic effect of plasma exosomes against cerebral Ischemia-Induced pyroptosis through the Toll-like receptors/nuclear factor kappa-B (TLR/NF-κB) Pathway (186). Other modes of cell death besides apoptosis, such as ferroptosis and necroptosis, may be a good area for further research. In conclusion, we need to explore the mechanisms of brain damage in ischemic stroke and provide methods for treating and preventing ischemic stroke.
Author contributions
XK and SZ contributed design of the study and manuscript editing. SH wrote the first draft of the manuscript. LL and XT helped prepare the manuscript and collected the data. All authors contributed to the article and approved the submitted version.
Funding
The National Science Foundation of China supported this study under Grant No.81971008.
Conflict of interest
The authors declare that the research was conducted in the absence of any commercial or financial relationships that could be construed as a potential conflict of interest.
Publisher’s note
All claims expressed in this article are solely those of the authors and do not necessarily represent those of their affiliated organizations, or those of the publisher, the editors and the reviewers. Any product that may be evaluated in this article, or claim that may be made by its manufacturer, is not guaranteed or endorsed by the publisher.
References
1. Kanyal N. The science of ischemic stroke: Pathophysiology & pharmacological treatment. Int. J. Pharma Res. Rev (2015) 4(10):65–84.
2. Feigin VL, Stark BA, Johnson CO, Roth GA, Bisignano C, Abady GG, et al. Global, regional, and national burden of stroke and its risk factors, 1990–2019: A systematic analysis for the global burden of disease study 2019. Lancet Neurol (2021) 20(10):795–820. doi: 10.1016/s1474-4422(21)00252-0
3. Sun T, Chen S, Wu K, Sun M, Zhang X, You C. Trends in incidence and mortality of stroke in China from 1990 to 2019. Front Neurol (2021) 12:759221. doi: 10.3389/fneur.2021.759221
4. Ghosh D, Sehgal K, Sodnar B, Bhosale N, Sarmah D, Datta A, et al. Drug repurposing for stroke intervention. Drug Discovery Today (2022) 27(7):1974–82. doi: 10.1016/j.drudis.2022.03.003
5. Suzuki K, Matsumaru Y, Takeuchi M, Morimoto M, Kanazawa R, Takayama Y, et al. Effect of mechanical thrombectomy without vs with intravenous thrombolysis on functional outcome among patients with acute ischemic stroke: The skip randomized clinical trial. JAMA (2021) 325(3):244–53. doi: 10.1001/jama.2020.23522
6. Zi W, Qiu Z, Li F, Sang H, Wu D, Luo W, et al. Effect of endovascular treatment alone vs intravenous alteplase plus endovascular treatment on functional independence in patients with acute ischemic stroke: The devt randomized clinical trial. JAMA (2021) 325(3):234–43. doi: 10.1001/jama.2020.23523
7. Johnston SC, Amarenco P, Aunes M, Denison H, Evans SR, Himmelmann A, et al. Ischemic benefit and hemorrhage risk of ticagrelor-aspirin versus aspirin in patients with acute ischemic stroke or transient ischemic attack. Stroke (2021) 52(11):3482–9. doi: 10.1161/STROKEAHA.121.035555
8. Saini V, Guada L, Yavagal DR. Global epidemiology of stroke and access to acute ischemic stroke interventions. Neurology (2021) 97(20 Suppl 2):S6–S16. doi: 10.1212/WNL.0000000000012781
9. Krishnan R, Mays W, Elijovich L. Complications of mechanical thrombectomy in acute ischemic stroke. Neurology (2021) 97(20 Suppl 2):S115–S25. doi: 10.1212/WNL.0000000000012803
10. Clarke IJ. Hypothalamus as an endocrine organ. Compr Physiol (2015) 5(1):217–53. doi: 10.1002/cphy.c140019
11. Tell GP, Haour F, Saez JM. Hormonal regulation of membrane receptors and cell responsiveness: A review. Metabolism (1978) 27(10):1566–92. doi: 10.1016/s0026-0495(78)80029-8
12. Donley RF, Sundt TM Jr. The effect of dexamethasone on the edema of focal cerebral ischemia. Stroke (1973) 4(2):148–55. doi: 10.1161/01.str.4.2.148
13. Lee MC, Mastri AR, Waltz AG, Loewenson RB. Ineffectiveness of dexamethasone for treatment of experimental cerebral infarction. Stroke (1974) 5(2):216–8. doi: 10.1161/01.str.5.2.216
14. Fenske A, Fischer M, Regli F, Hase U. The response of focal ischemic cerebral edema to dexamethasone. J Neurol (1979) 220(3):199–209. doi: 10.1007/BF00705537
15. Strong AJ, Fairfield JE, Monteiro E, Kirby M, Hogg AR, Snape M, et al. Insulin protects cognitive function in experimental stroke. J Neurol Neurosurg Psychiatry (1990) 53(10):847–53. doi: 10.1136/jnnp.53.10.847
16. Toung TJ, Traystman RJ, Hurn PD. Estrogen-mediated neuroprotection after experimental stroke in Male rats. Stroke (1998) 29(8):1666–70. doi: 10.1161/01.str.29.8.1666
17. Murphy SJ, Traystman RJ, Hurn PD, Duckles SP. Progesterone exacerbates striatal stroke injury in progesterone-deficient female animals. Stroke (2000) 31(5):1173–8. doi: 10.1161/01.str.31.5.1173
18. Jeppesen LL, Jorgensen HS, Nakayama H, Raaschou HO, Olsen TS, Winther K. Decreased serum testosterone in men with acute ischemic stroke. Arterioscler Thromb Vasc Biol (1996) 16(6):749–54. doi: 10.1161/01.atv.16.6.749
19. Qu F, He X, Lu W, Wang Y. Neurohypophysial avp concentration in stroke patients. Chin Med J (Engl) (1995) 108(4):259–61. doi: CNKI:SUN:ZHSS.0.1995-04-009
20. Junik R, Piechowski A, Sowinski J, Wender M. [the thyroid function markers and their prognostic significance patients with ischemic stroke]. Neurol Neurochir Pol (1995) 29(3):317–23.
21. Celik G, Dogan A, Dener S, Ozturk S, Kulaksizoglu S, Ekmekci H. Parathyroid hormone levels in the prediction of ischemic stroke risk. Dis Markers (2017) 2017:4343171. doi: 10.1155/2017/4343171
22. Altay H, Altin C, Coner A, Muderrisoglu H, Giray S. Parathyroid hormone and ischemic cerebrovascular event. Endocr Metab Immune Disord Drug Targets (2019) 19(8):1134–40. doi: 10.2174/1871530319666190215150410
23. Khoshnam SE, Winlow W, Farzaneh M, Farbood Y, Moghaddam HF. Pathogenic mechanisms following ischemic stroke. Neurol Sci (2017) 38(7):1167–86. doi: 10.1007/s10072-017-2938-1
24. Jiang X, Xing H, Wu J, Du R, Liu H, Chen J, et al. Prognostic value of thyroid hormones in acute ischemic stroke - a meta analysis. Sci Rep (2017) 7(1):16256. doi: 10.1038/s41598-017-16564-2
25. Dhital R, Poudel DR, Tachamo N, Gyawali B, Basnet S, Shrestha P, et al. Ischemic stroke and impact of thyroid profile at presentation: A systematic review and meta-analysis of observational studies. J Stroke Cerebrovasc Dis (2017) 26(12):2926–34. doi: 10.1016/j.jstrokecerebrovasdis.2017.07.015
26. Wang J, Li F, Xiao L, Peng F, Sun W, Li M, et al. Depressed tsh level as a predictor of poststroke fatigue in patients with acute ischemic stroke. Neurology (2018) 91(21):e1971–e8. doi: 10.1212/WNL.0000000000006534
27. Sadana P, Coughlin L, Burke J, Woods R, Mdzinarishvili A. Anti-edema action of thyroid hormone in mcao model of ischemic brain stroke: Possible association with Aqp4 modulation. J Neurol Sci (2015) 354(1-2):37–45. doi: 10.1016/j.jns.2015.04.042
28. Mokhtari T, Akbari M, Malek F, Kashani IR, Rastegar T, Noorbakhsh F, et al. Improvement of memory and learning by intracerebroventricular microinjection of T3 in rat model of ischemic brain stroke mediated by upregulation of bdnf and gdnf in Ca1 hippocampal region. Daru (2017) 25(1):4. doi: 10.1186/s40199-017-0169-x
29. Talhada D, Feiteiro J, Costa AR, Talhada T, Cairrao E, Wieloch T, et al. Triiodothyronine modulates neuronal plasticity mechanisms to enhance functional outcome after stroke. Acta Neuropathol Commun (2019) 7(1):216. doi: 10.1186/s40478-019-0866-4
30. Sayre NL, Sifuentes M, Holstein D, Cheng SY, Zhu X, Lechleiter JD. Stimulation of astrocyte fatty acid oxidation by thyroid hormone is protective against ischemic stroke-induced damage. J Cereb Blood Flow Metab (2017) 37(2):514–27. doi: 10.1177/0271678X16629153
31. Rastogi L, Godbole MM, Sinha RA, Pradhan S. Reverse triiodothyronine (Rt3) attenuates ischemia-reperfusion injury. Biochem Biophys Res Commun (2018) 506(3):597–603. doi: 10.1016/j.bbrc.2018.10.031
32. Talhada D, Santos CRA, Goncalves I, Ruscher K. Thyroid hormones in the brain and their impact in recovery mechanisms after stroke. Front Neurol (2019) 10:1103. doi: 10.3389/fneur.2019.01103
33. Liu Z, Chopp M. Astrocytes, therapeutic targets for neuroprotection and neurorestoration in ischemic stroke. Prog Neurobiol (2016) 144:103–20. doi: 10.1016/j.pneurobio.2015.09.008
34. Morte B, Gil-Ibanez P, Bernal J. Regulation of gene expression by thyroid hormone in primary astrocytes: Factors influencing the genomic response. Endocrinology (2018) 159(5):2083–92. doi: 10.1210/en.2017-03084
35. Guadano-Ferraz A, Obregon MJ, St Germain DL, Bernal J. The type 2 iodothyronine deiodinase is expressed primarily in glial cells in the neonatal rat brain. Proc Natl Acad Sci USA (1997) 94(19):10391–6. doi: 10.1073/pnas.94.19.10391
36. Margaill I, Royer J, Lerouet D, Ramauge M, Le Goascogne C, Li WW, et al. Induction of type 2 iodothyronine deiodinase in astrocytes after transient focal cerebral ischemia in the rat. J Cereb Blood Flow Metab (2005) 25(4):468–76. doi: 10.1038/sj.jcbfm.9600041
37. Maia AL, Kim BW, Huang SA, Harney JW, Larsen PR. Type 2 iodothyronine deiodinase is the major source of plasma T3 in euthyroid humans. J Clin Invest (2005) 115(9):2524–33. doi: 10.1172/JCI25083
38. Morte B, Bernal J. Thyroid hormone action: Astrocyte-neuron communication. Front Endocrinol (Lausanne) (2014) 5:82. doi: 10.3389/fendo.2014.00082
39. Zou L, Burmeister LA, Styren SD, Kochanek PM, DeKosky ST. Up-regulation of type 2 iodothyronine deiodinase mrna in reactive astrocytes following traumatic brain injury in the rat. J Neurochem (1998) 71(2):887–90. doi: 10.1046/j.1471-4159.1998.71020887.x
40. Li LQ, Xu XY, Li WY, Hu XY, Lv W. The prognostic value of total T3 after acute cerebral infarction is age-dependent: A retrospective study on 768 patients. BMC Neurol (2019) 19(1):54. doi: 10.1186/s12883-019-1264-z
41. Suda S, Muraga K, Kanamaru T, Okubo S, Abe A, Aoki J, et al. Low free triiodothyronine predicts poor functional outcome after acute ischemic stroke. J Neurol Sci (2016) 368:89–93. doi: 10.1016/j.jns.2016.06.063
42. Sabatino L, Kusmic C, Iervasi G. Modification of cardiac thyroid hormone deiodinases expression in an Ischemia/Reperfusion rat model after T3 infusion. Mol Cell Biochem (2020) 475(1-2):205–14. doi: 10.1007/s11010-020-03873-w
43. Scanlan TS, Suchland KL, Hart ME, Chiellini G, Huang Y, Kruzich PJ, et al. 3-iodothyronamine is an endogenous and rapid-acting derivative of thyroid hormone. Nat Med (2004) 10(6):638–42. doi: 10.1038/nm1051
44. Hackenmueller SA, Marchini M, Saba A, Zucchi R, Scanlan TS. Biosynthesis of 3-iodothyronamine (T1am) is dependent on the sodium-iodide symporter and thyroperoxidase but does not involve extrathyroidal metabolism of T4. Endocrinology (2012) 153(11):5659–67. doi: 10.1210/en.2012-1254
45. Hoefig CS, Wuensch T, Rijntjes E, Lehmphul I, Daniel H, Schweizer U, et al. Biosynthesis of 3-iodothyronamine from T4 in murine intestinal tissue. Endocrinology (2015) 156(11):4356–64. doi: 10.1210/en.2014-1499
46. Doyle KP, Suchland KL, Ciesielski TM, Lessov NS, Grandy DK, Scanlan TS, et al. Novel thyroxine derivatives, thyronamine and 3-iodothyronamine, induce transient hypothermia and marked neuroprotection against stroke injury. Stroke (2007) 38(9):2569–76. doi: 10.1161/STROKEAHA.106.480277
47. Gachkar S, Oelkrug R, Martinez-Sanchez N, Rial-Pensado E, Warner A, Hoefig CS, et al. 3-iodothyronamine induces tail vasodilation through central action in Male mice. Endocrinology (2017) 158(6):1977–84. doi: 10.1210/en.2016-1951
48. Krieger DW, Yenari MA. Therapeutic hypothermia for acute ischemic stroke: What do laboratory studies teach us? Stroke (2004) 35(6):1482–9. doi: 10.1161/01.STR.0000126118.44249.5c
49. Dumitrascu OM, Lamb J, Lyden PD. Still cooling after all these years: Meta-analysis of pre-clinical trials of therapeutic hypothermia for acute ischemic stroke. J Cereb Blood Flow Metab (2016) 36(7):1157–64. doi: 10.1177/0271678X16645112
50. Ceulemans AG, Zgavc T, Kooijman R, Hachimi-Idrissi S, Sarre S, Michotte Y. The dual role of the neuroinflammatory response after ischemic stroke: Modulatory effects of hypothermia. J Neuroinflamm (2010) 7:74. doi: 10.1186/1742-2094-7-74
51. Bardutzky J, Georgiadis D, Kollmar R, Schwab S. Energy expenditure in ischemic stroke patients treated with moderate hypothermia. Intensive Care Med (2004) 30(1):151–4. doi: 10.1007/s00134-003-1988-4
52. Zhang Z, Zhang L, Ding Y, Han Z, Ji X. Effects of therapeutic hypothermia combined with other neuroprotective strategies on ischemic stroke: Review of evidence. Aging Dis (2018) 9(3):507–22. doi: 10.14336/AD.2017.0628
53. Geurts M, Petersson J, Brizzi M, Olsson-Hau S, Luijckx GJ, Algra A, et al. Coolist (Cooling for ischemic stroke trial): A multicenter, open, randomized, phase ii, clinical trial. Stroke (2017) 48(1):219–21. doi: 10.1161/STROKEAHA.116.014757
54. Chen J, Liu L, Zhang H, Geng X, Jiao L, Li G, et al. Endovascular hypothermia in acute ischemic stroke: Pilot study of selective intra-arterial cold saline infusion. Stroke (2016) 47(7):1933–5. doi: 10.1161/STROKEAHA.116.012727
55. Kreber LA, Ashley MJ, Masel BE, Singh CK, Randle KD, Johnson C, et al. Prevalence of growth hormone deficiency in middle-age adults recovering from stroke. Brain Inj (2020) 34(2):276–80. doi: 10.1080/02699052.2019.1682195
56. Liu Y, Yang J, Che X, Huang J, Zhang X, Fu X, et al. Agonistic analog of growth hormone-releasing hormone promotes neurofunctional recovery and neural regeneration in ischemic stroke. Proc Natl Acad Sci USA (2021) 118(47):e2109600118. doi: 10.1073/pnas.2109600118
57. Lillicrap T, Garcia-Esperon C, Walker FR, Ong LK, Nilsson M, Spratt N, et al. Growth hormone deficiency is frequent after recent stroke. Front Neurol (2018) 9:713. doi: 10.3389/fneur.2018.00713
58. Bianchi VE, Locatelli V, Rizzi L. Neurotrophic and neuroregenerative effects of Gh/Igf1. Int J Mol Sci (2017) 18(11):2441. doi: 10.3390/ijms18112441
59. Gabrielpillai J, Geissler C, Stock B, Stover T, Diensthuber M. Growth hormone promotes neurite growth of spiral ganglion neurons. Neuroreport (2018) 29(8):637–42. doi: 10.1097/WNR.0000000000001011
60. Tuffaha SH, Singh P, Budihardjo JD, Means KR, Higgins JP, Shores JT, et al. Therapeutic augmentation of the growth hormone axis to improve outcomes following peripheral nerve injury. Expert Opin Ther Targets (2016) 20(10):1259–65. doi: 10.1080/14728222.2016.1188079
61. Lopez J, Quan A, Budihardjo J, Xiang S, Wang H, Kiron K, et al. Growth hormone improves nerve regeneration, muscle re-innervation, and functional outcomes after chronic denervation injury. Sci Rep (2019) 9(1):3117. doi: 10.1038/s41598-019-39738-6
62. Mendias CL, Enselman ERS, Olszewski AM, Gumucio JP, Edon DL, Konnaris MA, et al. The use of recombinant human growth hormone to protect against muscle weakness in patients undergoing anterior cruciate ligament reconstruction: A pilot, randomized placebo-controlled trial. Am J Sports Med (2020) 48(8):1916–28. doi: 10.1177/0363546520920591
63. Sanchez-Bezanilla S, Aberg ND, Crock P, Walker FR, Nilsson M, Isgaard J, et al. Growth hormone promotes motor function after experimental stroke and enhances recovery-promoting mechanisms within the peri-infarct area. Int J Mol Sci (2020) 21(2):606. doi: 10.3390/ijms21020606
64. Serhan A, Aerts JL, Boddeke E, Kooijman R. Neuroprotection by insulin-like growth factor-1 in rats with ischemic stroke is associated with microglial changes and a reduction in neuroinflammation. Neuroscience (2020) 426:101–14. doi: 10.1016/j.neuroscience.2019.11.035
65. Yang W, Li G, Cao K, Ma P, Guo Y, Tong W, et al. Exogenous insulin-like growth factor 1 attenuates acute ischemic stroke-induced spatial memory impairment via modulating inflammatory response and tau phosphorylation. Neuropeptides (2020) 83:102082. doi: 10.1016/j.npep.2020.102082
66. Serhan A, Boddeke E, Kooijman R. Insulin-like growth factor-1 is neuroprotective in aged rats with ischemic stroke. Front Aging Neurosci (2019) 11:349. doi: 10.3389/fnagi.2019.00349
67. Li Y, Yang W, Li J, Zhang Y, Zhang L, Chen S, et al. Relationship between serum insulin-like growth factor 1 levels and ischaemic stroke: A systematic review and meta-analysis. BMJ Open (2022) 12(6):e045776. doi: 10.1136/bmjopen-2020-045776
68. Okoreeh AK, Bake S, Sohrabji F. Astrocyte-specific insulin-like growth factor-1 gene transfer in aging female rats improves stroke outcomes. Glia (2017) 65(7):1043–58. doi: 10.1002/glia.23142
69. Sun JH, Tan L, Yu JT. Post-stroke cognitive impairment: Epidemiology, mechanisms and management. Ann Transl Med (2014) 2(8):80. doi: 10.3978/j.issn.2305-5839.2014.08.05
70. Levine DA, Galecki AT, Langa KM, Unverzagt FW, Kabeto MU, Giordani B, et al. Trajectory of cognitive decline after incident stroke. JAMA (2015) 314(1):41–51. doi: 10.1001/jama.2015.6968
71. Lee M, Saver JL, Hong KS, Wu YL, Liu HC, Rao NM, et al. Cognitive impairment and risk of future stroke: A systematic review and meta-analysis. CMAJ (2014) 186(14):E536–46. doi: 10.1503/cmaj.140147
72. Kwon HS, Lee D, Lee MH, Yu S, Lim JS, Yu KH, et al. Post-stroke cognitive impairment as an independent predictor of ischemic stroke recurrence: Picasso Sub-study. J Neurol (2020) 267(3):688–93. doi: 10.1007/s00415-019-09630-4
73. Schroder J, Pantel J. Neuroimaging of hippocampal atrophy in early recognition of alzheimer's disease–a critical appraisal after two decades of research. Psychiatry Res Neuroimaging (2016) 247:71–8. doi: 10.1016/j.pscychresns.2015.08.014
74. Elder GJ, Mactier K, Colloby SJ, Watson R, Blamire AM, O'Brien JT, et al. The influence of hippocampal atrophy on the cognitive phenotype of dementia with lewy bodies. Int J Geriatr Psychiatry (2017) 32(11):1182–9. doi: 10.1002/gps.4719
75. Wong FCC, Yatawara C, Low A, Foo H, Wong BYX, Lim L, et al. Cerebral small vessel disease influences hippocampal subfield atrophy in mild cognitive impairment. Transl Stroke Res (2021) 12(2):284–92. doi: 10.1007/s12975-020-00847-4
76. Milne NT, Bucks RS, Davis WA, Davis TME, Pierson R, Starkstein SE, et al. Hippocampal atrophy, asymmetry, and cognition in type 2 diabetes mellitus. Brain Behav (2018) 8(1):e00741. doi: 10.1002/brb3.741
77. Low A, Foo H, Yong TT, Tan LCS, Kandiah N. Hippocampal subfield atrophy of Ca1 and subicular structures predict progression to dementia in idiopathic parkinson's disease. J Neurol Neurosurg Psychiatry (2019) 90(6):681–7. doi: 10.1136/jnnp-2018-319592
78. Brodtmann A, Khlif MS, Egorova N, Veldsman M, Bird LJ, Werden E. Dynamic regional brain atrophy rates in the first year after ischemic stroke. Stroke (2020) 51(9):e183–e92. doi: 10.1161/STROKEAHA.120.030256
79. Khlif MS, Werden E, Bird LJ, Egorova-Brumley N, Brodtmann A. Atrophy of ipsilesional hippocampal subfields vary over first year after ischemic stroke. J Magn Reson Imaging (2022) 56(1):273–81. doi: 10.1002/jmri.28009
80. Delattre C, Bournonville C, Auger F, Lopes R, Delmaire C, Henon H, et al. Hippocampal deformations and entorhinal cortex atrophy as an anatomical signature of long-term cognitive impairment: From the mcao rat model to the stroke patient. Transl Stroke Res (2018) 9(3):294–305. doi: 10.1007/s12975-017-0576-9
81. Jung J, Laverick R, Nader K, Brown T, Morris H, Wilson M, et al. Altered hippocampal functional connectivity patterns in patients with cognitive impairments following ischaemic stroke: A resting-state fmri study. NeuroImage Clin (2021) 32:102742. doi: 10.1016/j.nicl.2021.102742
82. Nyberg F, Hallberg M. Growth hormone and cognitive function. Nat Rev Endocrinol (2013) 9(6):357–65. doi: 10.1038/nrendo.2013.78
83. Webb EA, O'Reilly MA, Clayden JD, Seunarine KK, Chong WK, Dale N, et al. Effect of growth hormone deficiency on brain structure, motor function and cognition. Brain (2012) 135(Pt 1):216–27. doi: 10.1093/brain/awr305
84. Ramsey MM, Weiner JL, Moore TP, Carter CS, Sonntag WE. Growth hormone treatment attenuates age-related changes in hippocampal short-term plasticity and spatial learning. Neuroscience (2004) 129(1):119–27. doi: 10.1016/j.neuroscience.2004.08.001
85. Molina DP, Ariwodola OJ, Linville C, Sonntag WE, Weiner JL, Brunso-Bechtold JK, et al. Growth hormone modulates hippocampal excitatory synaptic transmission and plasticity in old rats. Neurobiol Aging (2012) 33(9):1938–49. doi: 10.1016/j.neurobiolaging.2011.09.014
86. Haugland KG, Olberg A, Lande A, Kjelstrup KB, Brun VH. Hippocampal growth hormone modulates relational memory and the dendritic spine density in Ca1. Learn Mem (2020) 27(2):33–44. doi: 10.1101/lm.050229.119
87. Nylander E, Zelleroth S, Stam F, Nyberg F, Gronbladh A, Hallberg M. Growth hormone increases dendritic spine density in primary hippocampal cell cultures. Growth Horm IGF Res (2020) 50:42–7. doi: 10.1016/j.ghir.2019.12.003
88. Feng X, Li G, Wu W, Xu Y, Lin H, Fan J. Recombinant human growth hormone ameliorates cognitive impairment in stroke patients. J Comput Assist Tomogr (2020) 44(2):255–61. doi: 10.1097/RCT.0000000000000990
89. Ong LK, Chow WZ, TeBay C, Kluge M, Pietrogrande G, Zalewska K, et al. Growth hormone improves cognitive function after experimental stroke. Stroke (2018) 49(5):1257–66. doi: 10.1161/STROKEAHA.117.020557
90. Sanchez-Bezanilla S, Aberg ND, Crock P, Walker FR, Nilsson M, Isgaard J, et al. Growth hormone treatment promotes remote hippocampal plasticity after experimental cortical stroke. Int J Mol Sci (2020) 21(12):4653. doi: 10.3390/ijms21124563
91. Yeap BB, Hyde Z, Almeida OP, Norman PE, Chubb SA, Jamrozik K, et al. Lower testosterone levels predict incident stroke and transient ischemic attack in older men. J Clin Endocrinol Metab (2009) 94(7):2353–9. doi: 10.1210/jc.2008-2416
92. Zeller T, Schnabel RB, Appelbaum S, Ojeda F, Berisha F, Schulte-Steinberg B, et al. Low testosterone levels are predictive for incident atrial fibrillation and ischaemic stroke in men, but protective in women - results from the finrisk study. Eur J Prev Cardiol (2018) 25(11):1133–9. doi: 10.1177/2047487318778346
93. Ho CH, Wu CC, Lee MC, Huang PH, Chen JT, Liu SP, et al. The association of serum testosterone levels with recurrence and mortality after acute ischemic stroke in males. Am J Mens Health (2019) 13(3):563–8. doi: 10.1177/1557988319847097
94. Choi MH, Lim TS, Yoon BS, Son KS, Hong JM, Lee JS. Low testosterone level as a predictor of poststroke emotional disturbances: Anger proneness and emotional incontinence. J Stroke Cerebrovasc Dis (2018) 27(12):3549–54. doi: 10.1016/j.jstrokecerebrovasdis.2018.08.014
95. Normann S, de Veber G, Fobker M, Langer C, Kenet G, Bernard TJ, et al. Role of endogenous testosterone concentration in pediatric stroke. Ann Neurol (2009) 66(6):754–8. doi: 10.1002/ana.21840
96. Cheng J, Hu W, Toung TJ, Zhang Z, Parker SM, Roselli CE, et al. Age-dependent effects of testosterone in experimental stroke. J Cereb Blood Flow Metab (2009) 29(3):486–94. doi: 10.1038/jcbfm.2008.138
97. Ayala P, Uchida M, Akiyoshi K, Cheng J, Hashimoto J, Jia T, et al. Androgen receptor overexpression is neuroprotective in experimental stroke. Transl Stroke Res (2011) 2(3):346–57. doi: 10.1007/s12975-011-0079-z
98. Persky RW, Liu F, Xu Y, Weston G, Levy S, Roselli CE, et al. Neonatal testosterone exposure protects adult Male rats from stroke. Neuroendocrinology (2013) 97(3):271–82. doi: 10.1159/000343804
99. Dziennis S, Akiyoshi K, Subramanian S, Offner H, Hurn PD. Role of dihydrotestosterone in post-stroke peripheral immunosuppression after cerebral ischemia. Brain Behav Immun (2011) 25(4):685–95. doi: 10.1016/j.bbi.2011.01.009
100. Zhang W, Cheng J, Vagnerova K, Ivashkova Y, Young J, Cornea A, et al. Effects of androgens on early post-ischemic neurogenesis in mice. Transl Stroke Res (2014) 5(2):301–11. doi: 10.1007/s12975-013-0298-6
101. Loo SY, Chen BY, Yu OHY, Azoulay L, Renoux C. Testosterone replacement therapy and the risk of stroke in men: A systematic review. Maturitas (2017) 106:31–7. doi: 10.1016/j.maturitas.2017.08.013
102. Loo SY, Azoulay L, Nie R, Dell'Aniello S, Yu OHY, Renoux C. Cardiovascular and cerebrovascular safety of testosterone replacement therapy among aging men with low testosterone levels: A cohort study. Am J Med (2019) 132(9):1069–77.e4. doi: 10.1016/j.amjmed.2019.03.022
103. Cheetham TC, An J, Jacobsen SJ, Niu F, Sidney S, Quesenberry CP, et al. Association of testosterone replacement with cardiovascular outcomes among men with androgen deficiency. JAMA Intern Med (2017) 177(4):491–9. doi: 10.1001/jamainternmed.2016.9546
104. Wassertheil-Smoller S, Hendrix SL, Limacher M, Heiss G, Kooperberg C, Baird A, et al. Effect of estrogen plus progestin on stroke in postmenopausal women: The women's health initiative: A randomized trial. JAMA (2003) 289(20):2673–84. doi: 10.1001/jama.289.20.2673
105. Canonico M, Carcaillon L, Plu-Bureau G, Oger E, Singh-Manoux A, Tubert-Bitter P, et al. Postmenopausal hormone therapy and risk of stroke: Impact of the route of estrogen administration and type of progestogen. Stroke (2016) 47(7):1734–41. doi: 10.1161/STROKEAHA.116.013052
106. Toung TJ, Chen TY, Littleton-Kearney MT, Hurn PD, Murphy SJ. Effects of combined estrogen and progesterone on brain infarction in reproductively senescent female rats. J Cereb Blood Flow Metab (2004) 24(10):1160–6. doi: 10.1097/01.WCB.0000135594.13576.D2
107. Littleton-Kearney MT, Klaus JA, Hurn PD. Effects of combined oral conjugated estrogens and medroxyprogesterone acetate on brain infarction size after experimental stroke in rat. J Cereb Blood Flow Metab (2005) 25(4):421–6. doi: 10.1038/sj.jcbfm.9600052
108. Alkayed NJ, Murphy SJ, Traystman RJ, Hurn PD, Miller VM. Neuroprotective effects of female gonadal steroids in reproductively senescent female rats. Stroke (2000) 31(1):161–8. doi: 10.1161/01.str.31.1.161
109. Vahidinia Z, Alipour N, Atlasi MA, Naderian H, Beyer C, Azami Tameh A. Gonadal steroids block the calpain-1-Dependent intrinsic pathway of apoptosis in an experimental rat stroke model. Neurol Res (2017) 39(1):54–64. doi: 10.1080/01616412.2016.1250459
110. Nematipour S, Vahidinia Z, Nejati M, Naderian H, Beyer C, Azami Tameh A. Estrogen and progesterone attenuate glutamate neurotoxicity via regulation of Eaat3 and glt-1 in a rat model of ischemic stroke. Iran J Basic Med Sci (2020) 23(10):1346–52. doi: 10.22038/ijbms.2020.48090.11039
111. Vahidinia Z, Mahdavi E, Talaei SA, Naderian H, Tamtaji A, Haddad Kashani H, et al. The effect of female sex hormones on Hsp27 phosphorylation and histological changes in prefrontal cortex after tmcao. Pathol Res Pract (2021) 221:153415. doi: 10.1016/j.prp.2021.153415
112. Habib P, Harms J, Zendedel A, Beyer C, Slowik A. Gonadal hormones E2 and p mitigate cerebral ischemia-induced upregulation of the Aim2 and Nlrc4 inflammasomes in rats. Int J Mol Sci (2020) 21(13):4795. doi: 10.3390/ijms21134795
113. Dang J, Mitkari B, Kipp M, Beyer C. Gonadal steroids prevent cell damage and stimulate behavioral recovery after transient middle cerebral artery occlusion in Male and female rats. Brain Behav Immun (2011) 25(4):715–26. doi: 10.1016/j.bbi.2011.01.013
114. Herzog R, Zendedel A, Lammerding L, Beyer C, Slowik A. Impact of 17beta-estradiol and progesterone on inflammatory and apoptotic microrna expression after ischemia in a rat model. J Steroid Biochem Mol Biol (2017) 167:126–34. doi: 10.1016/j.jsbmb.2016.11.018
115. Choi JW, Ryoo IW, Hong JY, Lee KY, Nam HS, Kim WC, et al. Clinical impact of Estradiol/Testosterone ratio in patients with acute ischemic stroke. BMC Neurol (2021) 21(1):91. doi: 10.1186/s12883-021-02116-9
116. Hu J, Lin JH, Jimenez MC, Manson JE, Hankinson SE, Rexrode KM. Plasma estradiol and testosterone levels and ischemic stroke in postmenopausal women. Stroke (2020) 51(4):1297–300. doi: 10.1161/STROKEAHA.119.028588
117. Etehadi Moghadam S, Azami Tameh A, Vahidinia Z, Atlasi MA, Hassani Bafrani H, Naderian H. Neuroprotective effects of oxytocin hormone after an experimental stroke model and the possible role of calpain-1. J Stroke Cerebrovasc Dis (2018) 27(3):724–32. doi: 10.1016/j.jstrokecerebrovasdis.2017.10.020
118. Momenabadi S, Vafaei AA, Bandegi AR, Zahedi-Khorasani M, Mazaheri Z, Vakili A. Oxytocin reduces brain injury and maintains blood-brain barrier integrity after ischemic stroke in mice. Neuromolecular Med (2020) 22(4):557–71. doi: 10.1007/s12017-020-08613-3
119. Saffari M, Momenabadi S, Vafaei AA, Vakili A, Zahedi-Khorasani M. Prophylactic effect of intranasal oxytocin on brain damage and neurological disorders in global cerebral ischemia in mice. Iran J Basic Med Sci (2021) 24(1):79–84. doi: 10.22038/ijbms.2020.50265.11456
120. Wu X, Liu S, Hu Z, Zhu G, Zheng G, Wang G. Enriched housing promotes post-stroke neurogenesis through calpain 1-Stat3/Hif-1alpha/Vegf signaling. Brain Res Bull (2018) 139:133–43. doi: 10.1016/j.brainresbull.2018.02.018
121. Yousefvand S, Hadjzadeh MA, Vafaee F, Dolatshad H. The protective effects of prolactin on brain injury. Life Sci (2020) 263:118547. doi: 10.1016/j.lfs.2020.118547
122. Molina-Salinas G, Rivero-Segura NA, Cabrera-Reyes EA, Rodriguez-Chavez V, Langley E, Cerbon M. Decoding signaling pathways involved in prolactin-induced neuroprotection: A review. Front Neuroendocrinol (2021) 61:100913. doi: 10.1016/j.yfrne.2021.100913
123. Rodriguez-Chavez V, Moran J, Molina-Salinas G, Zepeda Ruiz WA, Rodriguez MC, Picazo O, et al. Participation of glutamatergic ionotropic receptors in excitotoxicity: The neuroprotective role of prolactin. Neuroscience (2021) 461:180–93. doi: 10.1016/j.neuroscience.2021.02.027
124. Anagnostou I, Munoz-Mayorga D, Morales T. Prolactin neuroprotective action against excitotoxic insult in the hippocampus of Male mice. Peptides (2021) 135:170425. doi: 10.1016/j.peptides.2020.170425
125. Rivero-Segura NA, Flores-Soto E, Garcia de la Cadena S, Coronado-Mares I, Gomez-Verjan JC, Ferreira DG, et al. Prolactin-induced neuroprotection against glutamate excitotoxicity is mediated by the reduction of [Ca2+]I overload and nf-kappab activation. PloS One (2017) 12(5):e0176910. doi: 10.1371/journal.pone.0176910
126. Ramos-Martinez E, Ramos-Martinez I, Molina-Salinas G, Zepeda-Ruiz WA, Cerbon M. The role of prolactin in central nervous system inflammation. Rev Neurosci (2021) 32(3):323–40. doi: 10.1515/revneuro-2020-0082
127. Jayakumar P, Martinez-Moreno CG, Lorenson MY, Walker AM, Morales T. Prolactin attenuates neuroinflammation in lps-activated sim-A9 microglial cells by inhibiting nf-kappab pathways via Erk1/2. Cell Mol Neurobiol (2021) 42(7):2171–86. doi: 10.1007/s10571-021-01087-2
128. Vermani B, Mukherjee S, Kumar G, Patnaik R. Prolactin attenuates global cerebral ischemic injury in rat model by conferring neuroprotection. Brain Inj (2020) 34(5):685–93. doi: 10.1080/02699052.2020.1726466
129. Wallaschofski H, Lohmann T, Hild E, Kobsar A, Siegemund A, Spilcke-Liss E, et al. Enhanced platelet activation by prolactin in patients with ischemic stroke. Thromb Haemost (2006) 96(1):38–44. doi: 10.1160/TH05-09-0634
130. Klimenko LL, Skalny AV, Turna AA, Tinkov AA, Budanova MN, Baskakov IS, et al. Serum trace element profiles, prolactin, and cortisol in transient ischemic attack patients. Biol Trace Elem Res (2016) 172(1):93–100. doi: 10.1007/s12011-015-0586-y
131. Cha JK, Jeong MH, Kim EK, Lim YJ, Ha BR, Kim SH, et al. Surface expression of p-selectin on platelets is related with clinical worsening in acute ischemic stroke. J Korean Med Sci (2002) 17(6):811–6. doi: 10.3346/jkms.2002.17.6.811
132. Olsson T, Astrom M, Eriksson S, Forssell A. Hypercortisolism revealed by the dexamethasone suppression test in patients [Corrected] with acute ischemic stroke. Stroke (1989) 20(12):1685–90. doi: 10.1161/01.str.20.12.1685
133. Sapolsky RM, Pulsinelli WA. Glucocorticoids potentiate ischemic injury to neurons: Therapeutic implications. Science (1985) 229(4720):1397–400. doi: 10.1126/science.4035356
134. Sundboll J, Horvath-Puho E, Schmidt M, Dekkers OM, Christiansen CF, Pedersen L, et al. Preadmission use of glucocorticoids and 30-day mortality after stroke. Stroke (2016) 47(3):829–35. doi: 10.1161/STROKEAHA.115.012231
135. Sundboll J, Darvalics B, Horvath-Puho E, Adelborg K, Laugesen K, Schmidt M, et al. Preadmission use of glucocorticoids and risk of cardiovascular events in patients with ischemic stroke. J Thromb Haemost (2018) 16(11):2175–83. doi: 10.1111/jth.14283
136. Shaikh AK, Mohammad QD, Ullah MA, Ahsan MM, Rahman A, Shakoor MA. Effect of dexamethasone on brain oedema following acute ischemic stroke. Mymensingh Med J (2011) 20(3):450–8. doi: 10.3329/mmj.v20i3.8329
137. Lopatkiewicz AM, Gradek-Kwinta E, Czyzycki M, Pera J, Slowik A, Dziedzic T. Glucocorticoid resistance is associated with poor functional outcome after stroke. Cell Mol Neurobiol (2020) 40(8):1321–6. doi: 10.1007/s10571-020-00818-1
138. Ghelani DP, Kim HA, Zhang SR, Drummond GR, Sobey CG, De Silva TM. Ischemic stroke and infection: A brief update on mechanisms and potential therapies. Biochem Pharmacol (2021) 193:114768. doi: 10.1016/j.bcp.2021.114768
139. Santos Samary C, Pelosi P, Leme Silva P, Rieken Macedo Rocco P. Immunomodulation after ischemic stroke: Potential mechanisms and implications for therapy. Crit Care (2016) 20(1):391. doi: 10.1186/s13054-016-1573-1
140. Espinosa A, Meneses G, Chavarria A, Mancilla R, Pedraza-Chaverri J, Fleury A, et al. Intranasal dexamethasone reduces mortality and brain damage in a mouse experimental ischemic stroke model. Neurotherapeutics (2020) 17(4):1907–18. doi: 10.1007/s13311-020-00884-9
141. Sun WH, He F, Zhang NN, Zhao ZA, Chen HS. Time dependent neuroprotection of dexamethasone in experimental focal cerebral ischemia: The involvement of nf-kappab pathways. Brain Res (2018) 1701:237–45. doi: 10.1016/j.brainres.2018.09.029
142. Li Y, Huang L, Ma Q, Concepcion KR, Song MA, Zhang P, et al. Repression of the glucocorticoid receptor aggravates acute ischemic brain injuries in adult mice. Int J Mol Sci (2018) 19(8):2428. doi: 10.3390/ijms19082428
143. Courties G, Frodermann V, Honold L, Zheng Y, Herisson F, Schloss MJ, et al. Glucocorticoids regulate bone marrow b lymphopoiesis after stroke. Circ Res (2019) 124(9):1372–85. doi: 10.1161/CIRCRESAHA.118.314518
144. Mracsko E, Liesz A, Karcher S, Zorn M, Bari F, Veltkamp R. Differential effects of sympathetic nervous system and hypothalamic-Pituitary-Adrenal axis on systemic immune cells after severe experimental stroke. Brain Behav Immun (2014) 41:200–9. doi: 10.1016/j.bbi.2014.05.015
145. Kim S, Park ES, Chen PR, Kim E. Dysregulated hypothalamic-Pituitary-Adrenal axis is associated with increased inflammation and worse outcomes after ischemic stroke in diabetic mice. Front Immunol (2022) 13:864858. doi: 10.3389/fimmu.2022.864858
146. You X, Zhou Y, Zhang J, Zhou Q, Shi Y, Su Z, et al. Effects of parathyroid hormone and vitamin d supplementation on stroke among patients receiving peritoneal dialysis. BMC Nephrol (2020) 21(1):183. doi: 10.1186/s12882-020-01817-6
147. Wang LL, Chen D, Lee J, Gu X, Alaaeddine G, Li J, et al. Mobilization of endogenous bone marrow derived endothelial progenitor cells and therapeutic potential of parathyroid hormone after ischemic stroke in mice. PloS One (2014) 9(2):e87284. doi: 10.1371/journal.pone.0087284
148. Huber BC, Grabmaier U, Brunner S. Impact of parathyroid hormone on bone marrow-derived stem cell mobilization and migration. World J Stem Cells (2014) 6(5):637–43. doi: 10.4252/wjsc.v6.i5.637
149. Funk JL, Migliati E, Chen G, Wei H, Wilson J, Downey KJ, et al. Parathyroid hormone-related protein induction in focal stroke: A neuroprotective vascular peptide. Am J Physiol Regul Integr Comp Physiol (2003) 284(4):R1021–30. doi: 10.1152/ajpregu.00436.2002
150. Chamorro A, Amaro S, Vargas M, Obach V, Cervera A, Gomez-Choco M, et al. Catecholamines, infection, and death in acute ischemic stroke. J Neurol Sci (2007) 252(1):29–35. doi: 10.1016/j.jns.2006.10.001
151. Yan FL, Zhang JH. Role of the sympathetic nervous system and spleen in experimental stroke-induced immunodepression. Med Sci Monit (2014) 20:2489–96. doi: 10.12659/MSM.890844
152. Ahn SS, Blaha CD, Alkire MT, Wood E, Gray-Allan P, Marrocco RT, et al. Biphasic striatal dopamine release during transient ischemia and reperfusion in gerbils. Stroke (1991) 22(5):674–9. doi: 10.1161/01.str.22.5.674
153. Viale L, Catoira NP, Di Girolamo G, Gonzalez CD. Pharmacotherapy and motor recovery after stroke. Expert Rev Neurother (2018) 18(1):65–82. doi: 10.1080/14737175.2018.1400910
154. Ruscher K, Kuric E, Wieloch T. Levodopa treatment improves functional recovery after experimental stroke. Stroke (2012) 43(2):507–13. doi: 10.1161/STROKEAHA.111.638767
155. Floel A, Hummel F, Breitenstein C, Knecht S, Cohen LG. Dopaminergic effects on encoding of a motor memory in chronic stroke. Neurology (2005) 65(3):472–4. doi: 10.1212/01.wnl.0000172340.56307.5e
156. Scheidtmann K, Fries W, Muller F, Koenig E. Effect of levodopa in combination with physiotherapy on functional motor recovery after stroke: A prospective, randomised, double-blind study. Lancet (2001) 358(9284):787–90. doi: 10.1016/S0140-6736(01)05966-9
157. Cramer SC. Drugs to enhance motor recovery after stroke. Stroke (2015) 46(10):2998–3005. doi: 10.1161/STROKEAHA.115.007433
158. Lokk J, Salman Roghani R, Delbari A. Effect of methylphenidate and/or levodopa coupled with physiotherapy on functional and motor recovery after stroke–a randomized, double-blind, placebo-controlled trial. Acta Neurol Scand (2011) 123(4):266–73. doi: 10.1111/j.1600-0404.2010.01395.x
159. Oczkowski W. Pharmacological therapies to enhance motor recovery and walking after stroke: Emerging strategies. Expert Rev Neurother (2013) 13(8):903–9. doi: 10.1586/14737175.2013.814940
160. Talhada D, Marklund N, Wieloch T, Kuric E, Ruscher K. Plasticity-enhancing effects of levodopa treatment after stroke. Int J Mol Sci (2021) 22(19):10226. doi: 10.3390/ijms221910226
161. Wang H, Du YS, Xu WS, Li CJ, Sun H, Hu KR, et al. Exogenous glutathione exerts a therapeutic effect in ischemic stroke rats by interacting with intrastriatal dopamine. Acta Pharmacol Sin (2022) 43(3):541–51. doi: 10.1038/s41401-021-00650-3
162. Gagnon DJ, Leclerc AM, Riker RR, Brown CS, May T, Nocella K, et al. Amantadine and modafinil as neurostimulants during post-stroke care: A systematic review. Neurocrit Care (2020) 33(1):283–97. doi: 10.1007/s12028-020-00977-5
163. Vogelgesang A, Becker KJ, Dressel A. Immunological consequences of ischemic stroke. Acta Neurol Scand (2014) 129(1):1–12. doi: 10.1111/ane.12165
164. Dziedzic T, Slowik A, Pera J, Szczudlik A. Beta-blockers reduce the risk of early death in ischemic stroke. J Neurol Sci (2007) 252(1):53–6. doi: 10.1016/j.jns.2006.10.007
165. Sykora M, Siarnik P, Diedler J, Collaborators VA. Beta-blockers, pneumonia, and outcome after ischemic stroke: Evidence from virtual international stroke trials archive. Stroke (2015) 46(5):1269–74. doi: 10.1161/STROKEAHA.114.008260
166. Clemente-Moragon A, Oliver E, Calle D, Cusso L, Tech MG, Pradillo JM, et al. Neutrophil Beta1 adrenergic receptor blockade blunts stroke-associated neuroinflammation. Br J Pharmacol (2022). doi: 10.1111/bph.15963
167. Savitz SI, Erhardt JA, Anthony JV, Gupta G, Li X, Barone FC, et al. The novel beta-blocker, carvedilol, provides neuroprotection in transient focal stroke. J Cereb Blood Flow Metab (2000) 20(8):1197–204. doi: 10.1097/00004647-200008000-00005
168. Sternberg Z, Schaller B. Central noradrenergic agonists in the treatment of ischemic stroke-an overview. Transl Stroke Res (2020) 11(2):165–84. doi: 10.1007/s12975-019-00718-7
169. Lechtenberg KJ, Meyer ST, Doyle JB, Peterson TC, Buckwalter MS. Augmented Beta2-adrenergic signaling dampens the neuroinflammatory response following ischemic stroke and increases stroke size. J Neuroinflamm (2019) 16(1):112. doi: 10.1186/s12974-019-1506-4
170. Xu Y, Ge Y, Zhou M, Zhang Z. Clenbuterol, a selective Beta2-adrenergic receptor agonist, inhibits or limits post-stroke pneumonia, but increases infarct volume in mcao mice. J Inflammation Res (2022) 15:295–309. doi: 10.2147/JIR.S344521
171. Araki T, Kato H, Shuto K, Fujiwara T, Kogure K, Itoyama Y. Effects of cerebral ischemia on dopamine receptors in the gerbil striatum. Eur J Pharmacol (1996) 306(1-3):73–9. doi: 10.1016/0014-2999(96)00227-0
172. Chang CJ, Ishii H, Yamamoto H, Yamamoto T, Spatz M. Effects of cerebral ischemia on regional dopamine release and D1 and D2 receptors. J Neurochem (1993) 60(4):1483–90. doi: 10.1111/j.1471-4159.1993.tb03311.x
173. Araki T, Kato H, Shuto K, Fujiwara T, Itoyama Y. Effect of cerebral ischemia on dopamine receptors and uptake sites in the gerbil hippocampus. Eur Neuropsychopharmacol (1997) 7(4):275–82. doi: 10.1016/s0924-977x(97)00033-3
174. Martin A, Gomez-Vallejo V, San Sebastian E, Padro D, Markuerkiaga I, Llarena I, et al. In vivo imaging of dopaminergic neurotransmission after transient focal ischemia in rats. J Cereb Blood Flow Metab (2013) 33(2):244–52. doi: 10.1038/jcbfm.2012.162
175. Zhang Y, Deng P, Ruan Y, Xu ZC. Dopamine D1-like receptors depress excitatory synaptic transmissions in striatal neurons after transient forebrain ischemia. Stroke (2008) 39(8):2370–6. doi: 10.1161/STROKEAHA.107.506824
176. Kuric E, Wieloch T, Ruscher K. Dopamine receptor activation increases glial cell line-derived neurotrophic factor in experimental stroke. Exp Neurol (2013) 247:202–8. doi: 10.1016/j.expneurol.2013.04.016
177. Wang Y, Wang X, Zhang X, Chen S, Sun Y, Liu W, et al. D1 receptor-mediated endogenous tpa upregulation contributes to blood-brain barrier injury after acute ischaemic stroke. J Cell Mol Med (2020) 24(16):9255–66. doi: 10.1111/jcmm.15570
178. Huang CCY, Ma T, Roltsch Hellard EA, Wang X, Selvamani A, Lu J, et al. Stroke triggers nigrostriatal plasticity and increases alcohol consumption in rats. Sci Rep (2017) 7(1):2501. doi: 10.1038/s41598-017-02714-z
179. Huck JH, Freyer D, Bottcher C, Mladinov M, Muselmann-Genschow C, Thielke M, et al. De novo expression of dopamine D2 receptors on microglia after stroke. J Cereb Blood Flow Metab (2015) 35(11):1804–11. doi: 10.1038/jcbfm.2015.128
180. Liu XH, Kato H, Chen T, Kato K, Itoyama Y. Bromocriptine protects against delayed neuronal death of hippocampal neurons following cerebral ischemia in the gerbil. J Neurol Sci (1995) 129(1):9–14. doi: 10.1016/0022-510x(94)00239-k
181. Qiu J, Yan Z, Tao K, Li Y, Li Y, Li J, et al. Sinomenine activates astrocytic dopamine D2 receptors and alleviates neuroinflammatory injury via the Cryab/Stat3 pathway after ischemic stroke in mice. J Neuroinflamm (2016) 13(1):263. doi: 10.1186/s12974-016-0739-8
182. Andrabi SS, Ali M, Tabassum H, Parveen S, Parvez S. Pramipexole prevents ischemic cell death via mitochondrial pathways in ischemic stroke. Dis Model Mech (2019) 12(8):dmm033860. doi: 10.1242/dmm.033860
183. Kaushik P, Ali M, Tabassum H, Parvez S. Post-ischemic administration of dopamine D2 receptor agonist reduces cell death by activating mitochondrial pathway following ischemic stroke. Life Sci (2020) 261:118349. doi: 10.1016/j.lfs.2020.118349
184. Sharma P, Kulkarni GT, Sharma B. Possible involvement of D2/D3 receptor activation in ischemic preconditioning mediated protection of the brain. Brain Res (2020) 1748:147116. doi: 10.1016/j.brainres.2020.147116
185. Gou X, Xu D, Li F, Hou K, Fang W, Li Y. Pyroptosis in stroke-new insights into disease mechanisms and therapeutic strategies. J Physiol Biochem (2021) 77(4):511–29. doi: 10.1007/s13105-021-00817-w
Keywords: ischemic stroke, hormones, immunomodulation, brain protection, mechanisms
Citation: Huang S, Liu L, Tang X, Xie S, Li X, Kang X and Zhu S (2022) Research progress on the role of hormones in ischemic stroke. Front. Immunol. 13:1062977. doi: 10.3389/fimmu.2022.1062977
Received: 06 October 2022; Accepted: 18 November 2022;
Published: 07 December 2022.
Edited by:
Hong-Fei Zhang, Southern Medical University, ChinaReviewed by:
Jiahe Tan, First Affiliated Hospital of Chongqing Medical University, ChinaAlexander Dressel, Hospital Carl-Thiem-Klinikum Cottbus, Germany
Copyright © 2022 Huang, Liu, Tang, Xie, Li, Kang and Zhu. This is an open-access article distributed under the terms of the Creative Commons Attribution License (CC BY). The use, distribution or reproduction in other forums is permitted, provided the original author(s) and the copyright owner(s) are credited and that the original publication in this journal is cited, in accordance with accepted academic practice. No use, distribution or reproduction is permitted which does not comply with these terms.
*Correspondence: Xianhui Kang, a3hodWk2NkB6anUuZWR1LmNu; Shengmei Zhu, c216aHUyMDA4OEB6anUuZWR1LmNu