- Department of Orthopedics, National Cancer Center/National Clinical Research Center for Cancer/Cancer Hospital, Chinese Academy of Medical Sciences and Peking Union Medical College, Beijing, China
Three-dimensional cancer organoids derived from self-organizing cancer stems are ex vivo miniatures of tumors that faithfully recapitulate their structure, distinctive cancer features, and genetic signatures. As novel tools, current cancer organoids have been well established and rapidly applied in drug testing, genome editing, and transplantation, with the ultimate aim of entering clinical practice for guiding personalized therapy. However, given that the lack of a tumor microenvironment, including immune cells and fibrous cells, is a major limitation of this emerging methodology, co-culture models inspire high hope for further application of this technology in cancer research. Co-culture of cancer organoids and immune cells or fibroblasts is available to investigate the tumor microenvironment, molecular interactions, and chimeric antigen receptor-engineered lymphocytes in cancer treatment. In light of the recent progress in cancer organoid co-culture models, it is only possible to recognize the advantages and drawbacks of this novel model to exploit its full potential. In this review, we summarize the recent advances in the application of cancer organoids and co-culture models and how they could be improved in the future to benefit cancer research, especially precision medicine.
1 Introduction
Cancer precision medicine has been deeply and widely explored through experimental studies to clinical evaluations to achieve the goal of individualized treatment and improve patient prognosis. However, genotype-based cancer precision medicine has some limitations or deficiencies. Genomic instability during sustained cancer proliferation within the tumor microenvironment (TME) gives rise to intra- and inter-tumoral heterogeneity, contributing to drug resistance, treatment failure, and progression. Cancer development is an evolving, highly regulated, dynamic biochemical process associated with TME. Genetic mutations accumulated in the copying process during DNA replication of cancer cells drive the generation of intratumoral heterogeneity within a tumor or inter-tumoral heterogeneity between tumors, with interactions among cancer cells, immune cells, and fibroblasts leading to TME alterations. Moreover, tumor heterogeneity increases due to TME alterations. Thus, the provoked interaction network within TME hinders the clinical application of precision cancer therapy. Further developing preclinical models to investigate the TME and guide clinical precision therapy is required.
Organoids are three-dimensional (3D) cell clusters in vitro that contain key characteristics of an organ in vivo, including a self-organizing stem cell population that can differentiate into organ-specific cells (1). Organoids recapitulate the structure, function, and genetic signature of the original organ, thus providing a solid foundation for future research on stem cells, regenerative biology, organogenesis, precision medicine, and human pathologies (2). Organoids can be derived from embryonic stem cells (ESCs), induced pluripotent stem cells (iPSCs), and adult stem cells (ASCs) through a process similar to the acquisition of their distinctive organization (1). Self-assembly and differentiation through organoid formation depend on implicated cell signaling pathways mediated by intrinsic components and the extracellular environment, including the extracellular matrix (ECM) and media. Due to a better understanding of ECM biology and technologies for cell culture (3, 4), organoid culture has been progressively implemented. Moreover, organoids have shown exciting potential and have been a popular research focus in tissue engineering and biological research in the past decade.
Intra-tumoral heterogeneity is one of the characteristics of cancers that promotes tumor evolution and makes cancer treatment challenging (5). Given that heterogeneous cancer cells and cancer-associated cells within a tumor contribute unequally to progression with complex intercellular interactions, cancer research findings using 2D cancer cell lines have been challenged. Cancer organoids retain the 3D structure of the TME, providing a physical context for molecular interactions. Recently, a cancer organoid co-culture model was developed and utilized to elucidate cell-to-cell interactions, mechanisms of cancer immune responses, and the mechanism of tumor metastasis. We summarize the history of cancer organoids that show great potential and promise in modeling human diseases. We also summarized the applications of cancer organoids in cancer research on multiple systems in the human body. Various excellent reviews have discussed the applications of cancer organoid model systems in genomic analysis and drug screening. Here, we discuss a novel cancer organoid co-culture model system with the advancement of its applications for investigating cell-cell interactions, immune response within cancers, and underlying mechanisms of cancer evolution and personalized precision medicine. We also provide further insights into novel applications and development directions of the cancer organoid co-culture model system. We hope that this review provides a better perspective to help researchers apply this practical method to cancer research and precision medicine.
2 Organoid overview
An organoid is a stem cell-derived 3D cell culture that obtains the structure, collection of multiple cell types, and functionality of the corresponding tissue. Organoids are promising candidates for biomedical applications with tremendous potential and appealing prospects (6, 7). For the past few decades, numerous studies of stem cells have led to a better understanding of their behavior, with emerging methodologies of controlling stem cells’ self-organization and differentiation, which provides a scientific basis for the further establishment of organoids (7). There are two types of stem cells. One is ESCs and the other is ASCs. ESCs possess developmental totipotency and can differentiate into all cell types. ASCs are undifferentiated stem cells with the capacity to differentiate, maintain homeostasis, and regenerate in a specific organ (8). Since 1981, substantial scientific progress in stem cell research has paved the way for organoid development (Figure 1). Moreover, more restricted ASCs have exhibited the capacity to form organoids in vitro once grown in the appropriate extracellular matrix and provided with specific molecular factors (9). The critical point for organoid formation and growth is whether the culture conditions duplicate the in vivo niche signaling pathways for stem cells, which contributes to sustaining stem cell functions and inducing differentiation (10).
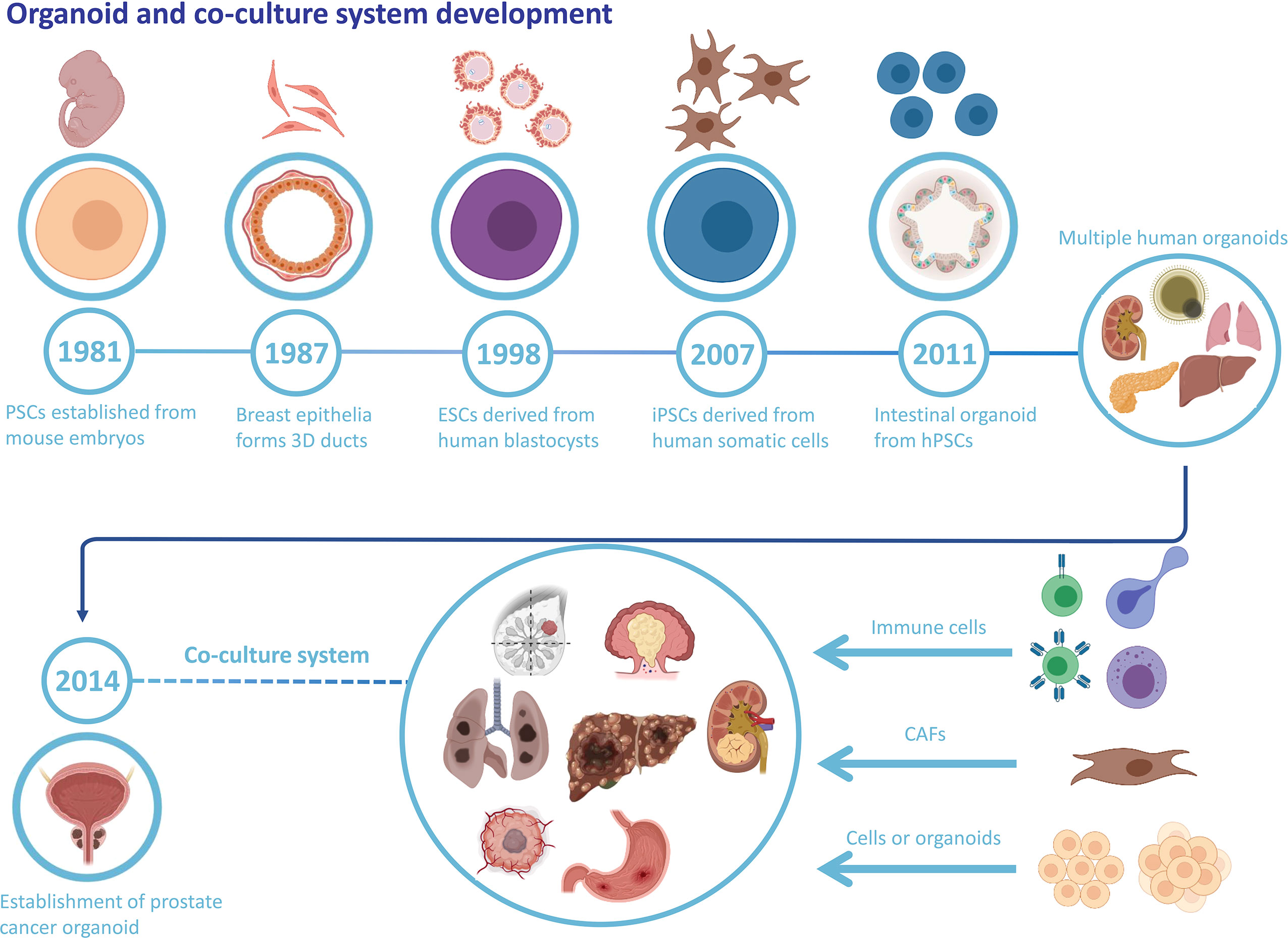
Figure 1 Organoid and co-culture system development. The history of organoid and cancer organoids and the well-established co-culture system of various cancer organoids and specific cell types.
As a novel tool and methodology, organoids have been sufficiently developed, widely studied, and adopted in various biomedical research fields (11). Organoids preserve the principal features of organ biology with greater experimental accessibility than that of animal models. Moreover, studies focusing on human embryonic and fetal tissues can be easier to conduct without restricting ethical concerns, such as prenatal development and tissue maintenance. Organoids also provide a platform to simulate the pathological environment for experiments at the organ level that cannot be performed at molecular, cellular, or animal levels. Based on this, precision medicine can be carried out using an organoid-based high-throughput screening and profiling strategy, facilitating preclinical evaluation and treatment guidance.
3 Cancer organoid
Organoids have been widely used to investigate neoplastic diseases, aside from being used to establish a normal developmental model. Patient-derived prostate cancer organoids were established for nearly a decade by Chen et al. (12). Recently, various cancer organoids, including colorectal cancer (CRC) (13), breast cancer (14), hepatocellular cancer (15), and non-small cell lung cancer (16), have been developed for drug screening, radiotherapy screening, genome editing, transplantation, and oncogene identification (Table 1). Most cancer organoids were obtained from patient-derived cancer samples and generated under ASCs-organoid conditions, but CRISPR-Cas9 nuclease genome editing system established the minority. Yilmaz et al. established CRC organoids via CRISPR-Cas9-based APC editing with lentivirus transfection using colon organoids derived from transgenic mice (33). Few studies have focused on developing organoids using other biological samples. Gao et al. successfully established prostate cancer organoids by culturing collected circulating tumor cells (12). Moreover, cancer organoids from cells in the urine and bronchoalveolar lavage fluid have already been established, providing a novel approach for cancer organoid establishment (34, 35).
Likewise, cancer organoids contain the cancerous cell composition of the original tumors and possess the corresponding features and genetics. The generation of various cancer organoids requires distinct methods. There are no standardized culture media or procedures for experiments. Optimal tissues are commonly obtained from tumor margins with a minimal necrosis rate. Generally, the entire process is initiated by mechanical and enzymatic digestion of tumor samples into ~1 mm diameter pieces, subsequently seeding the tissue suspension onto Matrigel as a biomimetic scaffold. Matrigel, which mainly contains laminin, entactin, proteoglycans, and collagen IV, primarily contributes to the cellular architecture of organoids (36). Unlike culturing healthy organoids, media with reduced growth factors is preferred for cancer organoid culture to minimize clonal selection and avoid confounding drug treatment effects (37). Growth factors for cancer organoid culture include Wnt3A, R-spondin-1, TGF-β receptor inhibitor, epidermal growth factor, and Noggin, but the combination and concentration of these factors added to the media depend on the specific cancer type. Compared to the initiated culture process, organoid passage is a more simplified but essential process during the culture period. The issue we must be concerned with is the passage number of cancer organoids that can be used for investigations. Upon passage, the genetic material of cancer cells within organoids undergoes mutations or alterations (18, 20, 23). Consequently, the authentication of cancer organoids is particularly important for their authenticity and credibility. Although cancer organoids are preferred and widely used in cancer research, their limitations cannot be neglected. Exogenous growth factors and small molecules added to organoid growth may result in clonal selection, and the components of the culture media may interact with the tested drug, complicating the conclusion. In addition, inappropriate sample collection may significantly impact the successful culturing rate because of less active proliferating cells and increased necrosis.
4 Cancer organoid application
As advancements have been made in cancer organoid development, cancer organoids have become a widely accepted practical model in cancer research (Figure 2). Cancer organoids are primarily used in drug screening for personalized medicine approaches. The drug response of patient-derived organoids (PDOs) mainly simulates patients’ initial responses to the same agents (28, 38–40). These studies have shown that the genetic changes that drive oncogenic pathways correspond to this therapy. Therefore, drug screening is the primary part of cancer organoid applications in cancer research to screen the most effective drugs and predict their therapeutic effects. A combination of other conditions, including radiation, was added to determine the most effective therapeutic strategy. For example, the FOXM1 inhibitor thiostrepton combined with radiotherapy (4 Gy) remarkably suppresses the proliferation of meningioma organoid models (26). Such a cancer organoid model may be applied in immunoradiotherapy investigations. In addition, the transplantation model is an excellent platform for mimicking human disease.
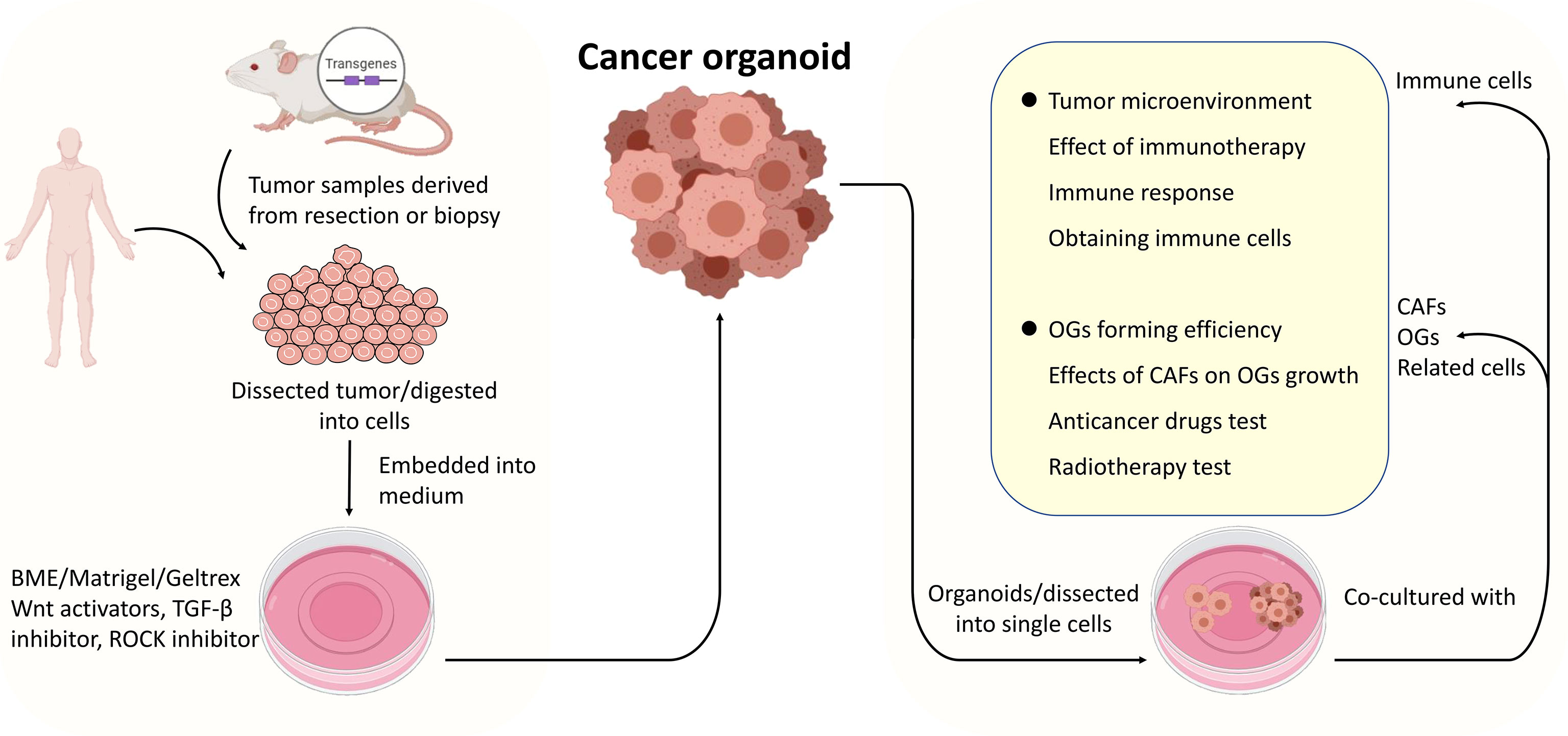
Figure 2 The procedures of cancer organoids establishment and applications of the co-culture system. Tumor tissues derived from surgically resected tumors or biopsies were dissected into small pieces or digested into cells, mixed with Matrigel, and cultured in media supplemented with specific growth factors. Cancer organoids (dissected into cells or not) were co-cultured with organoids, cancer-associated fibroblasts or related cells primarily immune cells to model the interactions between specific cells within tumors or TME.
The orthotopic transplantation of cancer organoids has been established in preclinical models. Sequencing analysis is commonly used to identify type-specific differentially expressed genes. RNA sequencing analysis and whole-exome sequencing were performed to identify cancer-related oncogenes. Moreover, as time passes, these techniques are the primary authentication methods for cancer organoids, and targeted sequencing is used to identify target mutations. CRISPR-Cas9 technology is revolutionary in genome editing and has been applied in organoid research. However, the precise integration of exogenous DNA sequences into human organoids is deficient in knock-in approaches. To address this, CRISPR-Cas9-mediated homology-independent organoid transgenesis was established to enable the efficient generation of knock-in human organoids representing different tissues (41), concluding that this technique can be used to achieve fast and efficient gene knock-in in human wild-type organoids.
Cancer organoids are now versatile tools in cancer research with a wide range of potential applications, showing the prospects of this advanced methodology in cancer research. However, there are some shortcomings. Organoids only comprise the epithelial layer without the native microenvironment of the surrounding mesenchyme, immune cells, nervous system, or muscular layer (42). Developing a novel co-culture model system of cancer organoids with other cells or organoids may recapitulate cell-cell interactions. In addition, the culture media should be improved to promote organoid growth and long-term expansion while minimizing the impact of growth factors in the media on the behavior of organoids (43). Cancer organoids have been established based on various human cancers; however, some cancer types, such as sarcomas, are still not involved. Consequently, refining culture approaches for rare heterogeneous cancer organoids and applying this tool for precision medicine should be addressed in future studies.
5 Co-culture model in cancer organoids
Co-culture is a method for culturing multiple distinct cell types, directly or indirectly, within the same culture environment (44). The cancer organoid co-culture model can efficiently simulate the environment for interactions between cancer organoids and cells within a tumor. Given that there are no immune cells, the nervous system, or mature TME in cancer organoids, a co-culture model was developed to solve this problem. The cancer organoid co-culture model was established for three main purposes. The first and most common application is to drive organoid formation via direct or indirect interactions between specific cell types within tumors. The second is the generation of specific tumor-targeting cytotoxic immune cells for cancer therapy using cancer organoids. The third is to detect the immune crosstalk between cancer organoids and specific cells, which is commonly implemented as a suspension of one cell population, usually cancer-associated fibroblasts, with the secretion of signaling factors and cytokines to condition the medium for the organoid. Marked advances in cancer organoids have been made by employing co-cultures of cancer organoids with specific cell types (Table 2). Moreover, the cancer organoid co-culture system with a specific type of cell can be used for different research purposes, and the co-culture of cancer organoids with multiple types of cells may accurately mimic tumor conditions.
5.1 Cancer-associated fibroblasts (CAFs) co-culture
CAFs play a major role in tumor-stromal crosstalk, which can be mediated by cell-cell contact, soluble factors, extracellular vesicles, and metabolites (69). CAFs account for most TME, particularly in CRC, and play critical roles in cancer progression, from the regulation of cancer cell proliferation and stem cell maintenance to drug resistance (70). CRC organoids and CAFs co-culture were established by Farin et al. to obtain an in vitro model for fibroblast plasticity in CRC, revealing that co-culture increased the contractility of CAFs, which was modulated by Wnt and IWP-2. Moreover, CRC PDO-CAF models were developed for drug testing and elucidation of CRC-CAF crosstalk, demonstrating that CAFs maintained the proliferation of CRC organoids in the hydrogels without adding growth factors and regained distinct signaling pathways that were absent in the CRC organoid culture alone but existed in tumors (59). This indicated that the CRC-CAF co-culture model was appropriate for evaluating drugs and helped bring us closer to the goal of personalized cancer medicine. Similarly, CAFs promoted the growth of in vitro HCC tumor organoids and transplantation xenograft models, conferring drug resistance to sorafenib, regorafenib, and 5-fluorouracil (61). This study was also conducted recently to determine the effect of CAFs on drug resistance in pancreatic ductal adenocarcinoma (PDAC) (63). CAFs are essential for tumor progression, and co-culture of CAFs and cancer organoids can recapitulate the TME within the origin of the tumor. Thus, elucidating the interactions between tumor organoids and CAFs may help improve culture media for better growth of organoids and simulation of the tumor.
Cancer organoid co-culture systems have been established for a wide range of applications. Fallopian tube epithelial cells co-cultured with fallopian stromal cells and endothelial cells form a miniature 3D structure with high efficiency, significantly suppressing Wnt inhibitors (66). Bone marrow stromal cells (BMSCs) enhance the anticancer effect of radiotherapy on CRC cells by secreting cytokines that inhibit proliferation and induce apoptosis of CRC cells. Such a mechanism would presumably be based on suppression of the PI3K/AKT signaling pathway, which may contribute to the attenuation of cell proliferation and death under irradiation with co-cultured BMSCs (65). A cancer organoid co-culture system was also developed to establish metastasis models to investigate the metastatic features of cancer. Breast cancer cells were co-cultured with lung epithelial cells to test organoid-forming efficiency (67). The cancer organoid co-culture model is an ideal tool to test the effect of various stromal cells on cancer development and progression, metastasis, and drug efficacy.
5.2 Immune cells co-culture
The major application of cancer organoid co-culture models is the co-culture of cancer organoids with immune cells, including cytotoxic T lymphocytes and dendritic cells (45), NK cells (55), macrophages (49, 54), and lymphocytes (46, 47) (Table 2). James et al. co-cultured PDAC organoids with CAFs and CD3+ T lymphocytes to develop a specific TME for PDAC (46). To obtain tumor-reactive T cells, peripheral blood lymphocytes were added and co-cultured with non-small cell lung cancer organoids, providing a clinically feasible strategy for generating patient-specific T cells for adoptive T cell transfer (47). Similarly, to investigate how CRC driver mutations dysregulate epithelial signaling from stromal and immune cells, CRC organoids were cultured either alone or with colonic fibroblasts and macrophages to directly compare mutation- and microenvironment-driven cell-type-specific signaling networks in CRC organoid mono- and co-cultures (49). More recently, a novel co-culture approach was developed to predict the efficacy of precision medicine to achieve a better prognosis for gastric cancer patients, using tumor antigens to stimulate antigen-presenting dendritic cells (DCs), followed by co-culture with CD8+ T cells to promote cytolysis and proliferation of these T cells before co-culture with patient-derived gastric cancer organoids (51). Such an approach may be considered more relevant within the TME instead of being induced by an artificial approach to T cell activation (32).
The era of checkpoint blockade immunotherapy is in full swing, exhibiting outstanding efficacy by unblocking negatively controlled T cells and triggering anticancer T cell responses. Co-culturing PDO with immune cells combined with checkpoint blockade inhibitors has been applied in a series of studies on cancer precision medicine (51–53, 56), providing important insights into predicting precision therapy efficacy with PDO. This process takes nearly ten days and includes cancer organoid establishment after surgical resection, preparation of immune cells, co-culture, drug testing, and determination of efficacy. This novel platform may guide clinical treatment at the microscopic level and benefit patients without needing long waiting periods based on advances in organoid culture techniques. Moreover, several studies have focused on generating tumor-specific lymphocytes for tumor-targeting therapy through co-culture (45–48, 51, 52). This method, with its excellent prospects, may achieve more accurate cell therapy with better clinical efficacy.
5.3. CAR-T cells co-culture
Currently, immunotherapy approaches, primarily immune checkpoint blockades, only select specific patient populations. The co-culture of cancer organoids and TME cells may provide an environment for immunotherapy research, making it a highly appealing and efficient option (47, 71). CAR-T cells are autologous and allogeneic T cells engineered to target specific antigens and markers on cancer cells, explicitly recognizing and eliminating cancer cells through direct T-cell cytotoxicity (72). The co-culture of cancer organoids and CAR-T cells provides a platform for predicting CAR-T cell efficacy and toxicity assessment (Table 3). Recently, Chen et al. established a successful preclinical testing ex vivo technological platform for a co-culture model system to evaluate CAR-T cell-mediated cytotoxicity against bladder cancer organoids targeting MUC1 (74). Such co-culture model systems for autologous HBVs+ HCC organoids and CD39+ HBV-CAR-T cells or CD39+ personalized tumor-reactive CD8+ T cells were also modeled to assess their anticancer efficiency (75). In addition, a co-culture of GBM organoids expressing EGFRvIII with 2173BBz CAR-T cells was performed, demonstrating the utility of rapid testing of antigen-specific CAR-T cell treatment responses (25). In addition, Farin et al. co-cultured CRC organoids with EGFRvIII-CAR NK-92 cells to model a platform for identifying and selecting suitable target antigens and assessing the anticancer activity of CAR-NK-92 cells (73). Notably, only one clinical trial has been carried out to evaluate the anticancer effects of CAR-engineered lymphocytes on cancer organoids, focusing on investigating the anticancer effects of CAR-macrophages in organoids derived from patients with breast cancer (NCT05007379). Because cancers exhibit diverse heterogeneity and genetic instability, cancer therapeutic approaches should be personalized. Cancer organoids preserve the histological features, cellular diversity, genetic heterogeneity, and mutational diversity of the tumor origin. Accordingly, co-culture of CAR-derived cells and cancer organoids can fully capture the molecular and cellular processes of immunotherapy, showing enormous potential in predicting therapeutic efficacy and cytotoxicity.
6 Discussion
As a major technological breakthrough, organoids are now well-established and vigorously developed as a vital methodology in biomedical studies. Organoids have been applied in tissue engineering, regenerative medicine, disease modeling, drug screening, and toxicological studies, restoring the 3D structure and primary cell types, but also in translational applications such as the prediction of chemotherapy, radiotherapy resistance before treatment, and gene editing, enabling mutation rectification (76). Although organoids have a wide range of applications in cancer research and clinical practice, the current version is a rough model, and culture procedures for specific cancer types must be constantly standardized and improved. Organoids are found in various organs that have been created, such as the brain, retina, gastrointestinal tract, tongue, thyroid, liver, pancreas, skin, lung, kidney, and heart (7). However, obstacles impede the generation of organoids in some organs, such as bone and soft tissue engineering. Human‐periosteum‐derived cells allow the scalable generation of semiautonomous callus organoids that induce the formation of bone micro-organs upon implantation (77). For most cancers, 3D organoid models have already been established for cancer investigations with barely any technological restrictions. However, no relevant studies have investigated organoids in rare malignant tumors that are equally promising in research on these rare cancers, such as bone and soft tissue sarcomas and neuroendocrine tumors. Bone and soft tissue sarcomas are commonly characterized by chromosomal translocations with a low mutational burden. Because of the advantage of gene editing of cancer organoids, mechanism-based sarcoma organoid models may provide theoretical support for investigations focusing on elucidating sarcomagenesis and other extensive applications.
Tumor cell lines in mice and patient-derived xenografts have long been used as cancer research models and have made significant contributions. However, various shortcomings hinder using these experimental models in clinical applications. Cell lines generally contain only one type of cell without co-cultured immune cells, stromal cells, TME, or organ-specific capability, losing the genetic heterogeneity of the origin tumor after multiple passages and clonal selection. In addition, there is a lack of immune response between the original tumor and the immune environment in immunodeficient mice. Human-derived cancer cells have evolved, potentially impacting chemotherapy by reshaping the genomic landscape. Such xenograft models are highly time- and resource-intensive. Cancer organoids may also overcome the aforementioned restrictions. Genetic modification of the insertions of oncogenic mutations in stem cells leads to the generation of genetically modified organoids. Unlike patient-derived xenografts, cancer organoids are easier to obtain and establish biobanks for restoration and can be used for high-throughput drug screening. Cancer organoids based on specific cancers and even on a specific individual used in high-throughput screening are expected to become powerful tools for precision therapy. Furthermore, screening can be performed using biobanks to identify new drugs and explore new indications.
Cancer organoids have a major shortcoming: fewer immune cells and specific types of cancer-associated stromal cell organoids. A cancer organoid co-culture model system appears to address this issue (Figure 3). This approach may be considered closer to what occurs within the TME (48). With regard to the co-culture of cancer-associated cells, CAFs account for most cancer organoid co-culture studies. CAFs, as essential complements of TME, have been shown to facilitate cancer progression and promote treatment resistance. CAFs contribute to treatment resistance, mainly through impaired drug delivery and biochemical signaling (61).
Given the existence of direct or indirect biochemical crosstalk between cancer cells and CAFs, the roles of CAFs in the immune response, drug resistance, and cancer proliferation must be demonstrated in specific cancer types. Although other cell types, such as BMSCs, were observed to be radiation protective through their well-known regenerative functions after ionizing radiation (65), such co-culture models with additional conditions are encouraged in cancer research for comprehensive treatment of cancer owing to the characteristics of the subtype. Co-culture of cancer organoids and immune cells can not only establish a model system to interrogate cancer sensitivity to immunotherapy for individuals at any period during treatment but also provide a clinically practical method for the creation of patient-specific T cell products for adoptive T cell transfer through expanding circulating tumor-reactive T cells by co-culture (47). NK cells, T cells, and dendritic cells were co-cultured with cancer organoids to investigate immune responses in cancer. This novel model was considered an efficient method for rapidly evaluating the effect of immune checkpoint inhibitors on activating cytotoxic lymphocytes and increasing infiltration in the context of T-cell infiltrates (46). Single-cell T-cell receptor sequencing (scTCR-seq) is another novel single-cell approach that can identify paired α- and β-TCR subunits that determine the specificity of infiltrating T cells. Association analysis of scTCR-seq with T-cell phenotypes (activation, memory, and exhaustion) and antigen specificity determination may provide more in-depth insights into cancer immunotherapy based on this novel tool.
7 Conclusions
The advanced technology of co-culturing CAR-engineered lymphocytes and cancer organoids is superior in personalized medicine owing to the maintenance of heterogeneity and the TME. CAR-engineered lymphocytes combined with organoid applications in drug tests, genome editing, and high-throughput screening will be our future research direction. However, because the therapeutic effect of CAR-T cell immunotherapy in solid tumors has not been as effective as in blood cancers owing to poor trafficking, limited persistence, limited infiltration, and T cell inhibitory activity in the TME, there has been little research on this area to date (78–80). Consequently, it has been proposed that checkpoint blockade inhibitors combined with CAR-engineered cells are a promising treatment approach for solid tumors (81–85), with a platform of organoids providing recapitulation of the environment. Recently, CAR-engineered cell therapy has been expanded to novel cell types, and the expression of CARs in NK cells has been considered a more successful variant. Cancer organoid-based co-culture systems may provide a platform for evaluating the clinical therapeutic effects of adoptive cell therapies. Moreover, genetic mutations in altered surface antigens in specific cancer cells must be identified to provide primary evidence for this methodology to achieve higher targeting and therapeutic efficiency. To broaden its applications, further research is needed to establish various co-culture model systems for organoid cancer research.
Author contributions
JY: Conceptualization, Investigation, Writing- Original draft preparation, SY, XL: Conceptualization, Supervision, Writing- Review and Editing. All authors contributed to the article and approved the submitted version.
Funding
This research was supported by the National Natural Science Foundation of China (No.82272964; No.82002848; No.82003397), the CAMS Innovation Fund for Medical Sciences (CIFMS) (2021- I2M-C&T-B-054, 2021-I2M-C&T-B-053), the Capital Characterized Clinical Application Research Fund of Beijing Municipal Science and Technology Commission of China (No. Z171100001017210), Fundamental Research Funds for the Central Universities (No. 3332021097), Beijing Hope Run Special Fund of Cancer Foundation of China (No. LC2021A14).
Conflict of interest
The authors declare that the research was conducted in the absence of any commercial or financial relationships that could be construed as a potential conflict of interest.
Publisher’s note
All claims expressed in this article are solely those of the authors and do not necessarily represent those of their affiliated organizations, or those of the publisher, the editors and the reviewers. Any product that may be evaluated in this article, or claim that may be made by its manufacturer, is not guaranteed or endorsed by the publisher.
Abbreviations
3D, three-dimensional; ASCs, adult stem cells; CAFs, cancer-associated fibroblasts; CRC, colorectal cancer; ECM, extracellular matrix; ESCs, embryonic stem cells; iPSCs, pluripotent stem cells; BMSCs, bone marrow stroma cell; PDAC, pancreatic ductal adenocarcinoma; PDOs, patient-derived organoids; TME, tumor microenvironment.
References
1. Corro C, Novellasdemunt L, Li VSW. A brief history of organoids. Am J Physiol Cell Physiol (2020) 319(1):C151–C65. doi: 10.1152/ajpcell.00120.2020
2. Dutta D, Heo I, Clevers H. Disease modeling in stem cell-derived 3D organoid systems. Trends Mol Med (2017) 23(5):393–410. doi: 10.1016/j.molmed.2017.02.007
3. Orkin RW, Gehron P, McGoodwin EB, Martin GR, Valentine T, Swarm R. A murine tumor producing a matrix of basement membrane. J Exp Med (1977) 145(1):204–20. doi: 10.1084/jem.145.1.204
4. Michalopoulos G, Pitot HC. Primary culture of parenchymal liver cells on collagen membranes. morphological and biochemical observations. Exp Cell Res (1975) 94(1):70–8. doi: 10.1016/0014-4827(75)90532-7
5. McGranahan N, Swanton C. Clonal heterogeneity and tumor evolution: Past, present, and the future. Cell (2017) 168(4):613–28. doi: 10.1016/j.cell.2017.01.018
6. Clevers H. Modeling development and disease with organoids. Cell (2016) 165(7):1586–97. doi: 10.1016/j.cell.2016.05.082
7. Rossi G, Manfrin A, Lutolf MP. Progress and potential in organoid research. Nat Rev Genet (2018) 19(11):671–87. doi: 10.1038/s41576-018-0051-9
8. Artegiani B, Clevers H. Use and application of 3D-organoid technology. Hum Mol Genet (2018) 27(R2):R99–R107. doi: 10.1093/hmg/ddy187
9. Rauth S, Karmakar S, Batra SK, Ponnusamy MP. Recent advances in organoid development and applications in disease modeling. Biochim Biophys Acta Rev Cancer (2021) 1875(2):188527. doi: 10.1016/j.bbcan.2021.188527
10. McCauley HA, Wells JM. Pluripotent stem cell-derived organoids: using principles of developmental biology to grow human tissues in a dish. Development (2017) 144(6):958–62. doi: 10.1242/dev.140731
11. Tuveson D, Clevers H. Cancer modeling meets human organoid technology. Science (2019) 364(6444):952–5. doi: 10.1126/science.aaw6985
12. Gao D, Vela I, Sboner A, Iaquinta PJ, Karthaus WR, Gopalan A, et al. Organoid cultures derived from patients with advanced prostate cancer. Cell (2014) 159(1):176–87. doi: 10.1016/j.cell.2014.08.016
13. Ooft SN, Weeber F, Dijkstra KK, McLean CM, Kaing S, van Werkhoven E, et al. Patient-derived organoids can predict response to chemotherapy in metastatic colorectal cancer patients. Sci Transl Med (2019) 11(513):eaay2574. doi: 10.1126/scitranslmed.aay2574
14. Dekkers JF, Whittle JR, Vaillant F, Chen H-R, Dawson C, Liu K, et al. Modeling breast cancer using CRISPR-Cas9–mediated engineering of human breast organoids. JNCI: J Natl Cancer Inst (2019) 112(5):540–4. doi: 10.1093/jnci/djz196
15. Wang S, Wang Y, Xun X, Zhang C, Xiang X, Cheng Q, et al. Hedgehog signaling promotes sorafenib resistance in hepatocellular carcinoma patient-derived organoids. J Exp Clin Cancer Res (2020) 39(1):22. doi: 10.1186/s13046-020-1523-2
16. Shi R, Radulovich N, Ng C, Liu N, Notsuda H, Cabanero M, et al. Organoid cultures as preclinical models of non-small cell lung cancer. Clin Cancer Res (2020) 26(5):1162–74. doi: 10.1158/1078-0432.CCR-19-1376
17. Ukai S, Honma R, Sakamoto N, Yamamoto Y, Pham QT, Harada K, et al. Molecular biological analysis of 5-FU-resistant gastric cancer organoids; KHDRBS3 contributes to the attainment of features of cancer stem cell. Oncogene (2020) 39:7265–78. doi: 10.1038/s41388-020-01492-9
18. Steele NG, Chakrabarti J, Wang J, Biesiada J, Holokai L, Chang J, et al. An organoid-based preclinical model of human gastric cancer. Cell Mol Gastroenterol Hepatol (2019) 7(1):161–84. doi: 10.1016/j.jcmgh.2018.09.008
19. Biffi G, Oni TE, Spielman B, Hao Y, Elyada E, Park Y, et al. IL1-induced JAK/STAT signaling is antagonized by TGFβ to shape CAF heterogeneity in pancreatic ductal adenocarcinoma. Cancer Discovery (2019) 9:282–301. doi: 10.1158/2159-8290.CD-18-0710
20. Kijima T, Nakagawa H, Shimonosono M, Chandramouleeswaran PM, Hara T, Sahu V, et al. Three-dimensional organoids reveal therapy resistance of esophageal and oropharyngeal squamous cell carcinoma cells. Cell Mol Gastroenterol Hepatol (2019) 7(1):73–91. doi: 10.1016/j.jcmgh.2018.09.003
21. Li Z, Qian Y, Li W, Liu L, Yu L, Liu X, et al. Human lung adenocarcinoma-derived organoid models for drug screening. iScience (2020) 23:101411. doi: 10.1016/j.isci.2020.101411
22. Zhang S, Iyer S, Ran H, Dolgalev I, Gu S, Wei W, et al. Genetically defined, syngeneic organoid platform for developing combination therapies for ovarian cancer. Cancer Discovery (2021) 11:362–83. doi: 10.1158/2159-8290.CD-20-0455
23. Boretto M, Maenhoudt N, Luo X, Hennes A, Boeckx B, Bui B, et al. Patient-derived organoids from endometrial disease capture clinical heterogeneity and are amenable to drug screening. Nat Cell Biol (2019) 21(8):1041–51. doi: 10.1038/s41556-019-0360-z
24. Maru Y, Tanaka N, Ebisawa K, Odaka A, Sugiyama T, Itami M, et al. Establishment and characterization of patient-derived organoids from a young patient with cervical clear cell carcinoma. Cancer Sci (2019) 110:2992–3005. doi: 10.1111/cas.14119
25. Jacob F, Salinas RD, Zhang DY, Nguyen PTT, Schnoll JG, Wong SZH, et al. A patient-derived glioblastoma organoid model and biobank recapitulates inter- and intra-tumoral heterogeneity. Cell (2020) 180(1):188–204.e22. doi: 10.1016/j.cell.2019.11.036
26. Yamazaki S, Ohka F, Hirano M, Shiraki Y, Motomura K, Tanahashi K, et al. Newly established patient-derived organoid model of intracranial meningioma. Neuro Oncol (2021) 23. doi: 10.1093/neuonc/noab155
27. Ballabio C, Anderle M, Gianesello M, Lago C, Miele E, Cardano M, et al. Modeling medulloblastoma in vivo and with human cerebellar organoids. Nat Commun (2020) 11:583. doi: 10.1038/s41467-019-13989-3
28. Lee SH, Hu W, Matulay JT, Silva MV, Owczarek TB, Kim K, et al. Tumor evolution and drug response in patient-derived organoid models of bladder cancer. Cell (2018) 173(2):515–28.e17. doi: 10.1016/j.cell.2018.03.017
29. Puca L, Bareja R, Prandi D, Shaw R, Benelli M, Karthaus WR, et al. Patient derived organoids to model rare prostate cancer phenotypes. Nat Commun (2018) 9:2404. doi: 10.1038/s41467-018-04495-z
30. Sondorp LHJ, Ogundipe VML, Groen AH, Kelder W, Kemper A, Links TP, et al. Patient-derived papillary thyroid cancer organoids for radioactive iodine refractory screening. Cancers (Basel) (2020) 12:3212. doi: 10.3390/cancers12113212
31. Goldhammer N, Kim J, Timmermans-Wielenga V, Petersen OW. Characterization of organoid cultured human breast cancer. Breast Cancer Res (2019) 21:141. doi: 10.1186/s13058-019-1233-x
32. Neal JT, Li X, Zhu J, Giangarra V, Grzeskowiak CL, Ju J, et al. Organoid modeling of the tumor immune microenvironment. Cell (2018) 175(7):1972–88.e16. doi: 10.1016/j.cell.2018.11.021
33. Roper J, Tammela T, Cetinbas NM, Akkad A, Roghanian A, Rickelt S, et al. In vivo genome editing and organoid transplantation models of colorectal cancer and metastasis. Nat Biotechnol (2017) 35(6):569–76. doi: 10.1038/nbt.3836
34. Schutgens F, Rookmaaker MB, Margaritis T, Rios A, Ammerlaan C, Jansen J, et al. Tubuloids derived from human adult kidney and urine for personalized disease modeling. Nat Biotechnol (2019) 37(3):303–13. doi: 10.1038/s41587-019-0048-8
35. Sachs N, Papaspyropoulos A, Zomer-van Ommen DD, Heo I, Böttinger L, Klay D, et al. Long-term expanding human airway organoids for disease modeling. EMBO J (2019) 38(4):e100300. doi: 10.15252/embj.2018100300
36. Aboulkheyr Es H, Montazeri L, Aref AR, Vosough M, Baharvand H. Personalized cancer medicine: An organoid approach. Trends Biotechnol (2018) 36(4):358–71. doi: 10.1016/j.tibtech.2017.12.005
37. Jacob F, Ming GL, Song H. Generation and biobanking of patient-derived glioblastoma organoids and their application in CAR T cell testing. Nat Protoc (2020) 15(12):4000–33. doi: 10.1038/s41596-020-0402-9
38. Hill SJ, Decker B, Roberts EA, Horowitz NS, Muto MG, Worley MJ Jr., et al. Prediction of DNA repair inhibitor response in short-term patient-derived ovarian cancer organoids. Cancer Discovery (2018) 8(11):1404–21. doi: 10.1158/2159-8290.CD-18-0474
39. Tiriac H, Belleau P, Engle DD, Plenker D, Deschênes A, Somerville TDD, et al. Organoid profiling identifies common responders to chemotherapy in pancreatic cancer. Cancer Discovery (2018) 8(9):1112–29. doi: 10.1158/2159-8290.CD-18-0349
40. Vlachogiannis G, Hedayat S, Vatsiou A, Jamin Y, Fernández-Mateos J, Khan K, et al. Patient-derived organoids model treatment response of metastatic gastrointestinal cancers. Science (2018) 359(6378):920–6. doi: 10.1126/science.aao2774
41. Artegiani B, Hendriks D, Beumer J, Kok R, Zheng X, Joore I, et al. Fast and efficient generation of knock-in human organoids using homology-independent CRISPR-Cas9 precision genome editing. Nat Cell Biol (2020) 22(3):321–31. doi: 10.1038/s41556-020-0472-5
42. Jabs J, Zickgraf FM, Park J, Wagner S, Jiang X, Jechow K, et al. Screening drug effects in patient-derived cancer cells links organoid responses to genome alterations. Mol Syst Biol (2017) 13(11):955. doi: 10.15252/msb.20177697
43. Xu H, Lyu X, Yi M, Zhao W, Song Y, Wu K. Organoid technology and applications in cancer research. J Hematol Oncol (2018) 11(1):116. doi: 10.1186/s13045-018-0662-9
44. Paschos NK, Brown WE, Eswaramoorthy R, Hu JC, Athanasiou KA. Advances in tissue engineering through stem cell-based co-culture. J Tissue Eng Regener Med (2015) 9(5):488–503. doi: 10.1002/term.1870
45. Chakrabarti J, Holokai L, Syu L, Steele N, Chang J, Dlugosz A, et al. Mouse-derived gastric organoid and immune cell Co-culture for the study of the tumor microenvironment. Methods Mol Biol (2018) 1817:157–68. doi: 10.1007/978-1-4939-8600-2_16
46. Tsai S, McOlash L, Palen K, Johnson B, Duris C, Yang Q, et al. Development of primary human pancreatic cancer organoids, matched stromal and immune cells and 3D tumor microenvironment models. BMC Cancer (2018) 18(1):335. doi: 10.1186/s12885-018-4238-4
47. Dijkstra KK, Cattaneo CM, Weeber F, Chalabi M, van de Haar J, Fanchi LF, et al. Generation of tumor-reactive T cells by Co-culture of peripheral blood lymphocytes and tumor organoids. Cell (2018) 174(6):1586–98.e12. doi: 10.1016/j.cell.2018.07.009
48. Holokai L, Chakrabarti J, Lundy J, Croagh D, Adhikary P, Richards SS, et al. Murine- and human-derived autologous Organoid/Immune cell Co-cultures as preclinical models of pancreatic ductal adenocarcinoma. Cancers (Basel) (2020) 12(12):3816. doi: 10.3390/cancers12123816
49. Qin X, Sufi J, Vlckova P, Kyriakidou P, Acton SE, Li VSW, et al. Cell-type-specific signaling networks in heterocellular organoids. Nat Methods (2020) 17(3):335–42. doi: 10.1038/s41592-020-0737-8
50. Marcon F, Zuo J, Pearce H, Nicol S, Margielewska-Davies S, Farhat M, et al. NK cells in pancreatic cancer demonstrate impaired cytotoxicity and a regulatory IL-10 phenotype. Oncoimmunology (2020) 9:1845424. doi: 10.1080/2162402X.2020.1845424
51. Chakrabarti J, Koh V, So JBY, Yong WP, Zavros Y. A preclinical human-derived autologous gastric cancer Organoid/Immune cell Co-culture model to predict the efficacy of targeted therapies. JoVE 2021(173):e61443. doi: 10.3791/61443
52. Meng Q, Xie S, Gray GK, Dezfulian MH, Li W, Huang L, et al. Empirical identification and validation of tumor-targeting T cell receptors from circulation using autologous pancreatic tumor organoids. J Immunother Cancer (2021) 9(11):e003213. doi: 10.1136/jitc-2021-003213
53. Chakrabarti J, Koh V, Steele N, Hawkins J, Ito Y, Merchant JL, et al. Disruption of Her2-induced PD-L1 inhibits tumor cell immune evasion in patient-derived gastric cancer organoids. Cancers (Basel) (2021) 13(24):6158. doi: 10.3390/cancers13246158
54. Fang H, Huang Y, Luo Y, Tang J, Yu M, Zhang Y, et al. SIRT1 induces the accumulation of TAMs at colorectal cancer tumor sites via the CXCR4/CXCL12 axis. Cell Immunol (2022) 371:104458. doi: 10.1016/j.cellimm.2021.104458
55. Chan IS, Ewald AJ. Organoid Co-culture methods to capture cancer cell–natural killer cell interactions. In: Shimasaki N, editor. Natural killer (NK) cells: Methods and protocols. New York, NY: Springer US (2022). p. 235–50.
56. Zhou G, Lieshout R, van Tienderen GS, de Ruiter V, van Royen ME, Boor PPC, et al. Modelling immune cytotoxicity for cholangiocarcinoma with tumour-derived organoids and effector T cells. Br J Cancer (2022) 127. doi: 10.1038/s41416-022-01839-x
57. Chen S, Giannakou A, Wyman S, Gruzas J, Golas J, Zhong W, et al. Cancer-associated fibroblasts suppress SOX2-induced dysplasia in a lung squamous cancer coculture. Proc Natl Acad Sci (2018) 115:E11671–E80. doi: 10.1073/pnas.1803718115
58. Mosa MH, Michels BE, Menche C, Nicolas AM, Darvishi T, Greten FR, et al. A wnt-induced phenotypic switch in cancer-associated fibroblasts inhibits EMT in colorectal cancer. Cancer Res (2020) 80:5569–82. doi: 10.1158/0008-5472.CAN-20-0263
59. Luo X, Fong ELS, Zhu C, Lin QXX, Xiong M, Li A, et al. Hydrogel-based colorectal cancer organoid co-culture models. Acta Biomater (2021) 132:461–72. doi: 10.1016/j.actbio.2020.12.037
60. Zhao H, Jiang E, Shang Z. 3D Co-culture of cancer-associated fibroblast with oral cancer organoids. J Dent Res (2021) 100:201–8. doi: 10.1177/0022034520956614
61. Liu J, Li P, Wang L, Li M, Ge Z, Noordam L, et al. Cancer-associated fibroblasts provide a stromal niche for liver cancer organoids that confers trophic effects and therapy resistance. Cell Mol Gastroenterol Hepatol (2021) 11(2):407–31. doi: 10.1016/j.jcmgh.2020.09.003
62. Chen X, Li R, Zhao H, Wang X, Shao Z, Shang Z. Phenotype transition of fibroblasts incorporated into patient-derived oral carcinoma organoids. Oral Dis (2021). doi: 10.1111/odi.14071
63. Xiao W, Pahlavanneshan M, Eun CY, Zhang X, DeKalb C, Mahgoub B, et al. Matrix stiffness mediates pancreatic cancer chemoresistance through induction of exosome hypersecretion in a cancer associated fibroblasts-tumor organoid biomimetic model. Matrix Biol Plus (2022) 14:100111. doi: 10.1016/j.mbplus.2022.100111
64. Lau AN, Li Z, Danai LV, Westermark AM, Darnell AM, Ferreira R, et al. Dissecting cell-type-specific metabolism in pancreatic ductal adenocarcinoma. Elife (2020) 9:e56782. doi: 10.7554/eLife.56782
65. Feng H, Zhao JK, Schiergens TS, Wang PX, Ou BC, Al-Sayegh R, et al. Bone marrow-derived mesenchymal stromal cells promote colorectal cancer cell death under low-dose irradiation. Br J Cancer (2018) 118(3):353–65. doi: 10.1038/bjc.2017.415
66. Chang YH, Chu TY, Ding DC. Human fallopian tube epithelial cells exhibit stemness features, self-renewal capacity, and wnt-related organoid formation. J BioMed Sci (2020) 27(1):32. doi: 10.1186/s12929-019-0602-1
67. Ombrato L, Nolan E, Kurelac I, Mavousian A, Bridgeman VL, Heinze I, et al. Metastatic-niche labelling reveals parenchymal cells with stem features. Nature (2019) 572(7771):603–8. doi: 10.1038/s41586-019-1487-6
68. da Silva B, Mathew RK, Polson ES, Williams J, Wurdak H. Spontaneous glioblastoma spheroid infiltration of early-stage cerebral organoids models brain tumor invasion. SLAS Discovery (2018) 23:862–8. doi: 10.1177/2472555218764623
69. Gandellini P, Andriani F, Merlino G, D'Aiuto F, Roz L, Callari M. Complexity in the tumour microenvironment: Cancer associated fibroblast gene expression patterns identify both common and unique features of tumour-stroma crosstalk across cancer types. Semin Cancer Biol (2015) 35:96–106. doi: 10.1016/j.semcancer.2015.08.008
70. Peng S, Chen D, Cai J, Yuan Z, Huang B, Li Y, et al. Enhancing cancer-associated fibroblast fatty acid catabolism within a metabolically challenging tumor microenvironment drives colon cancer peritoneal metastasis. Mol Oncol (2021) 15(5):1391–411. doi: 10.1002/1878-0261.12917
71. Bar-Ephraim YE, Kretzschmar K, Clevers H. Organoids in immunological research. Nat Rev Immunol (2020) 20(5):279–93. doi: 10.1038/s41577-019-0248-y
72. Kumari R, Ouyang X, Wang J, Xu X, Zheng M, An X, et al. Preclinical pharmacology modeling of chimeric antigen receptor T therapies. Curr Opin Pharmacol (2021) 61:49–61. doi: 10.1016/j.coph.2021.08.008
73. Schnalzger TE, de Groot MH, Zhang C, Mosa MH, Michels BE, Röder J, et al. 3D model for CAR-mediated cytotoxicity using patient-derived colorectal cancer organoids. EMBO J (2019) 38(12):e100928. doi: 10.15252/embj.2018100928
74. Yu L, Li Z, Mei H, Li W, Chen D, Liu L, et al. Patient-derived organoids of bladder cancer recapitulate antigen expression profiles and serve as a personal evaluation model for CAR-T cells in vitro. Clin Transl Immunol (2021) 10(2):e1248. doi: 10.1002/cti2.1248
75. Zou F, Tan J, Liu T, Liu B, Tang Y, Zhang H, et al. The CD39(+) HBV surface protein-targeted CAR-T and personalized tumor-reactive CD8(+) T cells exhibit potent anti-HCC activity. Mol Ther (2021) 29(5):1794–807. doi: 10.1016/j.ymthe.2021.01.021
76. Semertzidou A, Brosens JJ, McNeish I, Kyrgiou M. Organoid models in gynaecological oncology research. Cancer Treat Rev (2020) 90:102103. doi: 10.1016/j.ctrv.2020.102103
77. Nilsson Hall G, Mendes LF, Gklava C, Geris L, Luyten FP, Papantoniou I. Developmentally engineered callus organoid bioassemblies exhibit predictive In vivo long bone healing. Adv Sci (Weinh). (2020) 7(2):1902295. doi: 10.1002/advs.201902295
78. Newick K, Moon E, Albelda SM. Chimeric antigen receptor T-cell therapy for solid tumors. Mol Ther Oncol (2016) 3:16006. doi: 10.1038/mto.2016.6
79. Yeku OO, Purdon TJ, Koneru M, Spriggs D, Brentjens RJ. Armored CAR T cells enhance antitumor efficacy and overcome the tumor microenvironment. Sci Rep (2017) 7(1):10541. doi: 10.1038/s41598-017-10940-8
80. Martinez M, Moon EK. CAR T cells for solid tumors: New strategies for finding, infiltrating, and surviving in the tumor microenvironment. Front Immunol (2019) 10:128. doi: 10.3389/fimmu.2019.00128
81. Johnson CB, Win SY. Combination therapy with PD-1/PD-L1 blockade: An overview of ongoing clinical trials. Oncoimmunology (2018) 7(4):e1408744. doi: 10.1080/2162402X.2017.1408744
82. John LB, Devaud C, Duong CP, Yong CS, Beavis PA, Haynes NM, et al. Anti-PD-1 antibody therapy potently enhances the eradication of established tumors by gene-modified T cells. Clin Cancer Res (2013) 19(20):5636–46. doi: 10.1158/1078-0432.CCR-13-0458
83. Hu B, Zou Y, Zhang L, Tang J, Niedermann G, Firat E, et al. Nucleofection with plasmid DNA for CRISPR/Cas9-mediated inactivation of programmed cell death protein 1 in CD133-specific CAR T cells. Hum Gene Ther (2019) 30(4):446–58. doi: 10.1089/hum.2017.234
84. Rupp LJ, Schumann K, Roybal KT, Gate RE, Ye CJ, Lim WA, et al. CRISPR/Cas9-mediated PD-1 disruption enhances anti-tumor efficacy of human chimeric antigen receptor T cells. Sci Rep (2017) 7(1):737. doi: 10.1038/s41598-017-00462-8
Keywords: cancer organoids, co-culture models, tumor microenvironment, cancer-associated fibroblasts, CAR-T cells
Citation: Yuan J, Li X and Yu S (2023) Cancer organoid co-culture model system: Novel approach to guide precision medicine. Front. Immunol. 13:1061388. doi: 10.3389/fimmu.2022.1061388
Received: 04 October 2022; Accepted: 22 December 2022;
Published: 12 January 2023.
Edited by:
Boris Chichkov, Leibniz University Hannover, GermanyReviewed by:
Valeria Lucarini, Bambino Gesù Children’s Hospital (IRCCS), ItalyMichael R. Hughes, Biomedical Research Centre, University of British Columbia, Canada
Copyright © 2023 Yuan, Li and Yu. This is an open-access article distributed under the terms of the Creative Commons Attribution License (CC BY). The use, distribution or reproduction in other forums is permitted, provided the original author(s) and the copyright owner(s) are credited and that the original publication in this journal is cited, in accordance with accepted academic practice. No use, distribution or reproduction is permitted which does not comply with these terms.
*Correspondence: Shengji Yu, emx5eWdrQDE2My5jb20=