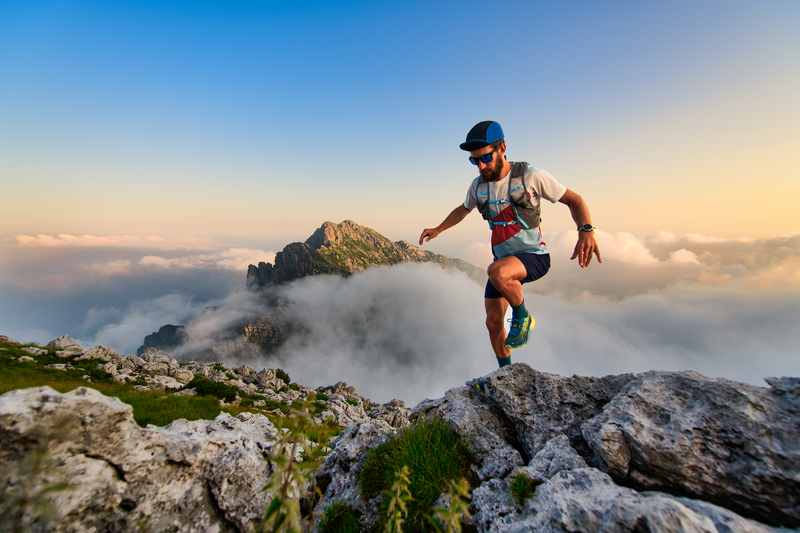
95% of researchers rate our articles as excellent or good
Learn more about the work of our research integrity team to safeguard the quality of each article we publish.
Find out more
REVIEW article
Front. Immunol. , 15 November 2022
Sec. Cancer Immunity and Immunotherapy
Volume 13 - 2022 | https://doi.org/10.3389/fimmu.2022.1050484
This article is part of the Research Topic The regulatory immune system as a target to improve adjuvants and novel vaccines View all 5 articles
Dendritic cells (DCs) are a heterogenous population of professional antigen presenting cells whose main role is diminished in a variety of malignancies, including cancer, leading to ineffective immune responses. Those mechanisms are inhibited due to the immunosuppressive conditions found in the tumor microenvironment (TME), where myeloid-derived suppressor cells (MDSCs), a heterogeneous population of immature myeloid cells known to play a key role in tumor immunoevasion by inhibiting T-cell responses, are extremely accumulated. In addition, it has been demonstrated that MDSCs not only suppress DC functions, but also their maturation and development within the myeloid linage. Considering that an increased number of DCs as well as the improvement in their functions boost antitumor immunity, DC-based vaccines were developed two decades ago, and promising results have been obtained throughout these years. Therefore, the remodeling of the TME promoted by DC vaccination has also been explored. Here, we aim to review the effectiveness of different DCs-based vaccines in murine models and cancer patients, either alone or synergistically combined with other treatments, being especially focused on their effect on the MDSC population.
The success of current cancer therapies depends on the knowledge of the tumor microenvironment (TME), which is a complex signaling network consisting of tumor, immune and stromal cells, as well as non-cellular components such as exosomes or the extracellular matrix (1). Immune cells such as regulatory T cells (Tregs), tumor-associated macrophages (TAMs) or myeloid-derived suppressor cells (MDSCs) expand systematically and play a key role by inhibiting effector T-cell responses and favoring tumor progression through the release of a variety of factors, such as arginase-1 (ARG-1), reactive oxygen species (ROS), interleukin (IL)-6, IL-10, transforming growth factor (TGF)-β, vascular endothelial growth factor (VEGF), or the expression of different proteins in their surface, including programmed cell death protein 1 (PD-1) or cytotoxic T-lymphocyte antigen 4 (CTLA-4), among many others (2–4).
Specifically, MDSCs are a heterogenous population of immature myeloid cells with a potent immunosuppressive capacity (as shown in Figure 1) that leads to tumor growth, development of pre-metastatic niches, resistance to immunotherapy, and poor outcomes (5–7). MDSCs are recruited into the TME via C-C motif chemokine ligand 2 (CCL2)/C-C motif chemokine receptor 2 (CCR2), CCL3/CCR5, CC15/CCR1, or CXC motif chemokine ligand 13 (CXCL13)/CXC motif receptor 5 (CXCR5) pathways (8), and other mediators such as granulocyte-macrophage colony-stimulating factor (GM-CSF), IL-6, or prostaglandin E2 (PGE2) participate to expand MDSCs. There are two main populations of MDSCs that share some phenotypic, morphological, and functional characteristics with inflammatory, immunosuppressive monocytes and neutrophils, called monocytic MDSCs (M-MDSCs) and granulocytic MDSCs (G-MDSCs) (9). Mouse and human MDSCs share some phenotypic expression, such a CD11b. However, mouse MDSCs are commonly defined as Gr1+CD11b+ cells characterized by the expression of Ly6ChighLy6G- (M-MDSCs) and Ly6ClowLy6G+ (G-MDSCs), whereas human MDSCs are CD11b+HLA-DRlow/- cells that also express CD14+CD15- (M-MDSCs) or CD14-CD15+ (G-MDSCs). Of note, G-MDSCs can also express high levels of CD66b (9).
Figure 1 Immunosuppressive role of myeloid-derived suppressor cells (MDSC). MDSC exert their function by promoting the development and expansion of suppressor cells such as M2-macrophages and regulatory T cells (Treg), and inhibiting cell populations that participate in immune responses against the tumor, including dendritic cells (DC), T cells, B cells, and natural killer (NK) cells. Arg, arginase; CCL2, C-C motif ligand 2; GM-CSF, granulocyte/monocyte-colony stimulating factor; HIF, hypoxia-inducible factor; IDO, indoleamine 2,3-dioxygenase; IFN, interferon; IL, interleukin; iNOS, inducible nitric oxide synthase; NO, nitric oxide; NOX, nicotinamide adenine dinucleotide phosphate (NADPH) oxidase; PGE2, prostaglandin E2; ROS, reactive oxygen species; TGF, transforming growth factor; VEGF, vascular endothelial growth factor.
MDSCs mainly inhibit antitumor T-cell responses through a variety of mechanisms (10). In this sense, MDSCs promote the loss of T-cell receptor (TCR) ζ-chain and cell cycle arrest in T cells by up-taking L-cysteine and L-arginine, two essential amino acids for proliferation and expansion of T cells (11, 12). MDSCs release ROS to provoke the loss of the TCR ζ-chain, and promote nitrosylation and nitration of components of the TCR complex (13). MDSCs are also known to downregulate the cytotoxicity of natural killer (NK) cells by releasing TGF-β or indoleamine 2,3-dyoxygenase (IDO) (14). The recruitment of other immunosuppressor cells by releasing IL-10 and TGF-β are also carried out by MDSCs, including M2 macrophages (15) and regulatory T cells (Tregs) (16).
In this context, conventional treatments such as chemotherapy activate multiple signaling pathways and promote the secretion of inflammatory mediators, but it may have dual roles and is not considered as an efficient option to eradicate tumors completely (17), and radiation therapy (RT) causes DNA damage in tumor cells to inhibit their proliferation, but it may also affect to adjacent healthy cells (18). Also, RT, at least under some settings, can promote favorable conditions for immunoevasion by stimulating the recruitment of immunosuppressive cell populations, including MDSCs (19). However, a different approach to treat cancer is based on immunotherapies, which target specific cellular or non-cellular components to boost the potential of the immune system to kill cancer cells. Different immunotherapies have been developed in last decades, including treatments to target stromal cells (20), cell surface proteins (21), or angiogenic factors (22), among others.
Particularly, targeting DC activation using DC-based vaccines can be therapeutically beneficial because DCs are the most potent type of antigen presenting cells and are able to activate their immunogenic machinery ex profeso to sample and present tumor-associated antigens (TAAs) to CD4+ T cells on major histocompatibility complex (MHC) class II molecules and CD8+ T cells on MHC class I molecules, in order to activate T cells to recognize similar TAAs within the TME (23), a process called “cancer-immunity cycle” (24). It has been described that immunogenic tumor cell death (ICD) improves T-cell immunity since it promotes the migration of tumor-infiltrating DCs to draining lymph nodes (25). In addition, ICD determinants have an effect on DCs. For example, proinflammatory mediators of tumor cells such as the high mobility group box 1 (HMGB1) protein or the 70 kilodalton heat shock protein (HSP70) facilitate TAA processing and presentation, whereas plasma membrane components such as calreticulin or phosphatidylserine residues promote phagocytosis or TAA recognition, respectively (26). In line with this, conventional DCs (cDCs) have demonstrated a preferential capacity to promote antigen presentation to T cells (27) rather than monocyte-derived DCs (moDCs) or plasmacytoid DCs (pDCs), which may have dual roles in anti-tumor immunity (28, 29).
Here, we explain the current knowledge regarding the impact of DC-based vaccines on MDSCs in both preclinical tumor models and oncological patients, either alone or combined with other treatments.
Immunotherapies have demonstrated to improve outcomes in cancer (30–33). DC infiltration into tumors has been positively correlated with prognosis and survival (34–36), leading to the design of DC-based immunotherapies. DC vaccines emerged as a promising alternative to further improve anti-tumor immunity (37–43). Specifically, cDC vaccines and pDC have shown better anti-tumor efficacy compared to moDC vaccines (44–46). Another interesting approach is the so-called in vivo vaccination to target DCs with DC receptor ligands, adjuvants, or other types of molecules that can accurately bind to DCs to exert better anti-tumor responses (47–49). Of note, DC-based immunotherapy could be inefficient due to the MDSC accumulation within the TME, so the combination of DC vaccines with other treatments may be a feasible approach to deplete MDSCs (50, 51), as shown in Figure 2.
Figure 2 Effects of dendritic cell (DC) vaccination (alone and combined with other treatments) on the tumor microenvironment (TME) in comparison with a non-treated TME. DC vaccines exert a beneficial (although limited) function, increasing the number of lymphoid cell populations within the TME, but with variable levels of myeloid-derived suppressor cells (MDSC), compared with non-treated subjects. However, the addition of some drugs may synergistically potentiate the effects of DC vaccines to further delay tumor growth, improve survival rates, and significantly decrease the number of suppressor cell populations, including MDSC, within the TME.
The use of DC vaccines has been widely investigated in murine models over the last decades. Although vaccination with DCs has demonstrated effectiveness in certain oncological settings in vivo (51–56), it has been extensively shown that the inhibition of immunosuppressive conditions has been slight. In this sense, combinatorial treatments have improved the efficacy of DC vaccines reducing tumor growth significantly, enhancing survival rates, and activating stronger tumor-specific T cell responses (57–62), thus overcoming immune tolerance. A growing preclinical literature illustrates the immunomodulatory capacity of DC vaccines combined with treatments such as IMiDs, inhibitors, or chemotherapeutic agents to reduce the proportion of MDSCs in cancer murine models (Table 1).
Table 1 Preclinical studies involving the use of dendritic cell-based vaccines (alone and combined with other treatments) and their effects on the myeloid-derived suppressor cell population.
Lenalidomide (LEN) and pomalidomide (POM) are IMiDs derived from thalidomide that increase cytotoxic responses driven by T cells and NK cells against tumors (74, 75). LEN has promoted the depletion of MDSCs in lymphoma patients with good response to the treatment (76) and in lymphoma-bearing mice, in which have been demonstrated an additive therapeutic antitumor effect when combined with a fusion DNA vaccine (77). In combination with TAA-loaded DC vaccine, LEN showed a remarkable tumor growth inhibition and significantly reduced MDSCs compared to LEN alone and DC vaccine alone in a colon mouse model (54).
Promising results were obtained with POM combined with dexamethasone in multiple myeloma (MM) (78, 79), although the combination with different inhibitors could further improve cell cycle arrest, deregulation of metabolic pathways, and tumor cell apoptosis in proliferative phases(80–82). When added to a DC vaccine, POM and dexamethasone synergistically improved antitumor immunity in MM mouse models due to the increased proportion of effector lymphoid cells and the depletion of not only splenic MDSCs, but also VEGF (71), which usually promotes angiogenesis and MDSC migration into the blood (83, 84).
The use of blockade agents has also improved the efficacy of different types of vaccines (85, 86). Specifically, immune checkpoint inhibitors have successfully targeted MDSCs in melanoma-bearing mice treated with the tyrosinase related protein (TRP)1/tyrosine DC vaccine (70). Similar results were obtained in myeloma murine models after combining a DC vaccine and anti-PD-1 with IMiDs (52, 53).
In addition, DC vaccines has improved the effects of other inhibitors such as tranilast (TRA) or dasatinib (DAS) in lung and melanoma mouse models, respectively, because the combinatorial treatment reduced the number of MDSCs (56, 68). TRA is an anti-fibrotic agent to inhibit not only cancer-associated fibroblasts (CAFs; which are one of the most abundant and critical components of the tumor mesenchyme to promote carcinogenesis), but also tumor cell interactions, and the modulation of immune factors (87, 88), including MDSC differentiation and recruitment (89, 90). In the same line, DAS allows the blockade of the SRC kinase family (91) and has shown to improve T-cell responses after decreasing MDSCs in head and neck squamous cell carcinoma (92). Of note, M-MDSCs have been suggested as a promising prognostic biomarker in patients with myeloid leukemia treated with DAS (93).
Analogs of biological compounds to inhibit metabolic routes also reduced the proportion of MDSCs when combined with DC-based vaccines. 5-fluorouracil (5-FU) is a fluoropyrimidine that inhibits essential biosynthetic processes and RNA and DNA functions by incorporating their metabolites into the nucleic acids and inhibiting the enzyme thymidylate synthase (94), which promotes the depletion of MDSCs (95). 5-FU in combination with a DC vaccine showed a greater MDSC reduction and improved survival rates in melanoma-bearing mice compared with DC vaccination alone (which maintained MDSC levels), and/or 5-FU alone (which significantly depleted MDSCs, although survival rates were lower compared to the combinatorial treatment) (50). Interestingly, these schedules were used in a melanoma-bearing mice to stablish an agent-based model to simulate the interactions between tumor and immune cells, as well as comparing different scenarios to determine the role of each component (including MDSCs) during tumor progression (69).
Also, rose bengal (RB), a staining agent and an inhibitor of ribonucleic acid chain elongation some decades ago (96), induced not only the regression of injected tumors in melanoma murine models, but also immunogenic cell death and the release of HMGB1, which improved DC infiltration into draining lymph nodes and, consequently, the activation of T cell responses (97). Combined with a DC vaccine, RB reduced MDSCs and enhanced the activation of effector cells and the release of TNF-α, leading to the inhibition of tumor growth in lung cancer-bearing mice (55).
DC vaccines have also been combined with chemotherapeutic agents such as gemcitabine (GEM). GEM is known to inhibit DNA synthesis of tumor cells (98) and has shown to inhibit MDSC expansion both in vitro and in vivo (99–101). In line with this notion, DC vaccination improved the effects of low-dose GEM due to not only DC maturation, T-cell activation, and the production of IFN-γ, but also the reduction of tumor cells and MDSCs (GEM alone also did), and tumor growth inhibition, thus enhancing survival rates (GEM alone did not) in lymphoma-bearing mice (51). GEM alone increased the apoptosis of splenic MDSCs significantly in pancreatic cancer-bearing mice, whereas the combination with a DC vaccine also enhanced the overall survival (73).
In a pancreatic cancer mouse model, overall survival was prolonged after using a tumor-exosome (TEX)-DC vaccine but both circulating and tumor-infiltrating MDSCs were only depleted in the group of mice treated with GEM, all-trans retinoic acid (ATRA), and/or sunitinib, or the combinatorial schedule with the vaccine. However, the latter group unexpectedly experienced tumor reappearance due to persisting drug application (72).
Chitosan is a biological polysaccharide that play a role in multiple medical functions, such as absorption enhancer or drug releaser. In cancer, chitosan is mainly used in chemotherapeutic delivery and as an immunoadjuvant in vaccines (102). Folate (FA)-modified chitosan has demonstrated to enhance tumor targeting and cytotoxic T-cell responses in some oncological settings (103–106). FA-modified chitosan nanoparticles carrying a plasmid of the mouse interferon-induced protein-10 (mIP-10) gene, a chemoattractant for cytotoxic T cells, reduced MDSCs from spleen, tumor, and bone marrow in combination with a DC/tumor cell fusion vaccine in hepatocellular carcinoma-bearing mice (67). In this context, neovascularization, metastasis, and survival of cancer cells can also be promoted by adenosine and its producing molecules, such as CD73, which has been proposed as one of the next-generation targets in cancer (107, 108). In line with this notion, CD73 expression is a biomarker of poor prognosis in breast cancer (109) and the use of chitosan-lactate nanoparticles to target CD73-specific small interfering RNA (siRNA) in combination with a DC vaccine have been successfully tested in breast cancer models after inhibiting tumor growth, and MDSCs (64). Interestingly, this combinatorial treatment also reduced the levels of matrix metalloproteinase (MMP)-2 and MMP-9, which are pro-angiogenic factors released by MDSCs to facilitate extravasation processes and angiogenesis (110).
In addition, vascular endothelial (VE)-cadherin is an adhesion molecule with a key role in the development of the blood vascular system (111). VE-cadherin promotes tumor development and progression by enhancing angiogenesis (112) via interaction with VEGF receptor-2 and stimulation of TGF-β signaling pathway (113, 114). For these reasons, a VE-cadherin gene modified DC-based vaccine was developed and successfully tested in kidney, breast, and colon cancer models, resulting in delayed tumor progression and enhancing survival rates by the production of a large amounts of immunoglobulins, and the increase of T effector cells and cytotoxicity against VE-cadherin, as well as the reduction of immunosuppressor cells, including MDSCs (63).
The protein Rv2299c has also demonstrated to induce DC activation and maturation, and enhance the expression of MHC molecules, CD80, and CD86 proteins in vitro to promote naïve-T-cell proliferation (115). Combined with a DC vaccine, Rv2299c reduced tumor growth in a colon cancer murine model and improved antitumor immunity by activating T-cell responses and reducing MDSCs (65).
Interestingly, a DC vaccine with irradiated freeze-thaw-necrotic cells has also been tested and it increased tumor rejection and reduced the number of immunosuppressive cells, including MDSCs (66).
Immunotherapy consisting in DC vaccination alone or combined with other treatments has also been tested in a variety of clinical trials (116–123). Most of them has been focused on drug effectiveness and efficacy rather than the analysis of immunosuppressive cell populations. Therefore, the impact of DC vaccination on human MDSCs (Table 2) has been little studied.
Table 2 Clinical studies involving the use of dendritic cell-based vaccines (alone and combined with other treatments) and their effects on the myeloid-derived suppressor cell population.
DC vaccines alone have shown conflicting results in terms of MDSC levels. MoDC vaccines loaded with TAAs demonstrated the stimulation of a preexisting immune response against TAAs in children, adolescents, and young adults with sarcoma tumors in a phase I/II clinical trial. Interestingly, one of those patients had low levels of MDSCs and Tregs prior to vaccination and experienced significant regression of metastatic lesions after a second disease relapse (133). A similar vaccine was tested in a clinical trial involving metastatic melanoma patients, but M-MDSCs increased after vaccination and were inversely associated with survival (129), demonstrating their immunosuppressive role.
Another anti-tumor target is the stromal tumor suppressor p53, since its loss showed to modify cytokine secretion to increase myeloid infiltration (134), including MDSCs (135). In this sense, the deletion of stromal p53 demonstrated to increase the proliferation of fibroblasts and epithelial cells in KrasG12D-bearing mammary glands together with DNA damage and replication stress, which finally reduced apoptosis of tumor cells and increased the frequency of MDSCs (136). The DC vaccine transduced with wild-type p53 was tested in small cell lung cancer patients without previous positive p53 responses and only 20% of them developed p53-specific responses, with no significant variations in the levels of granzyme B-positive CD8 T cells and MDSCs (128).
In addition, promising results have been shown after using a adenovirus (AdV)-loaded DC vaccine with the TAAs tyrosinase, melanoma-associated antigen recognized by T cells (MART-1), and melanoma-antigen gene (MAGE)-A6 (collectively known as TMM2) since it produced the depletion of blood HLA-DR−CD11b+CD33+ MDSCs (130). Even better, a DC-based vaccine loaded with the glioma stem cell line GBM6-AD promoted a significant decrease of both M-MDSCs and e-MDSCs in patients with stable disease, whereas their non-stable counterparts experienced an increase of G-MDSCs (124).
Conversely, DC-based vaccines combined with other treatments have notably improved clinical responses and were associated with a significant MDSC reduction compared to DC vaccination alone:
IFNα, which belongs to the IFN1 family, is downregulated in the MDSC gene expression profile (137), suggesting that IFN-α signaling may be a key pathway to restrict the suppressive activity of MDSCs (138). Also, nitric oxygen produced by MDSCs may reduce the IFN responsiveness in other immune cells in vivo (139). Therefore, IFN-α therapies began to be tested, demonstrating an increment of the antibody-dependent cellular cytotoxicity in tumor-bearing mice (140), but adverse effects were also reported and they should be taken into consideration (141). When combined with an AdVTMM2-transduced DC vaccine in melanoma patients, IFN-α slightly decreased both M-MDSCs and G-MDSCs compared to DC vaccination alone and did not improve clinical responses (130).
Contrarily, sunitinib, which is a multi-target tyrosine kinase inhibitor that blocks stem cell factor receptor c-KIT, platelet-derived growth factor receptors, CSF receptors, and VEGF receptors 1, 2 and 3, has been extensively described as a treatment for renal cell carcinoma (142–144). Sunitinib reduced the level of MDSCs (145, 146), mainly due to the inhibition of the signal transducer and activator of transcription (STAT)3 signaling pathway (147). TAA-loaded DC-based vaccine improved the effects of sunitinib due to the reduction in the percentage of MDSCs in patients with renal cell carcinoma (132).
Another compound, ATRA, which is a targeted therapy for peptidylprolyl cis/trans isomerase, NIMA-Interacting 1 (best known as Pin1) in breast cancer and acute promyelocytic leukemia (148), is an active metabolite of vitamin A and exerts important effects not only in cell growth, differentiation, and apoptosis (149), but also in enhancing the influx of DCs into the draining lymph nodes (150) and promoting the maturation of MDSCs into neutrophils and monocytes (151). Specifically, vaccination with p53-transduced DCs combined with ATRA in lung cancer patients resulted in a significant depletion of MDSCs in more than twofold, that was accompanied by more p53-specific responses compared to vaccination alone (128).
When combined with vaccines, chemotherapeutic agents may be promising strategies (152) because they improve the efficacy of DC vaccination synergistically in cancer patients (153, 154). Neoadjuvant chemotherapy (NAC) demonstrated to increase the levels of MDSCs in breast cancer (155, 156), whereas DC vaccination with conventional NAC was suggested to be more effective in cancer patients with lower MDSCs and Tregs prior to treatment because they may develop beneficial immunological responses (126). However, other studies have reported pathological complete responses after depleting MDSCs in breast cancer patients (157, 158), similarly to the combination with an autologous Mo-DC vaccine (125).
Also, the chemotherapeutic drug docetaxel, which has shown to boost immune responses after inducing M1 macrophages and antigen presentation in vitro (159) and inhibiting MDSCs in breast cancer in vivo (160), depleted MDSCs and promoted positive immune responses when combined with DC vaccines in esophageal and prostate cancers (127, 131).
DC vaccines emerged two decades ago as alternative strategies to overcome tumor resistance and improve survival rates in cancer. DC vaccination has demonstrated better (although still limited) results in terms of efficacy and overall survival compared with other treatments. Probably, cellular immune responses promoted by DC vaccines alone are not sufficiently strong to overcome immunosuppression. Despite this, DC vaccines are currently considered as promising therapeutic approaches to be still optimized. In this sense, the addition of different drugs may be needed to synergically improved the effects of this type of vaccination, what should be further tested in both murine models and clinical trials.
Tumor resistance is mainly led by cells with suppressive functions. Specifically, MDSCs are considered as the “queen-bee” cell population of the TME because they lead multiple mechanisms of resistance and finally protect tumor cells for their surveillance and proliferation (161). In this situation, a variety of therapeutic approaches are currently being used to target MDSCs in cancer, such as their direct elimination, preventing their recruitment into the TME, inhibiting their immunosuppressive role, or inducing their differentiation into mature myeloid cells (162).
The therapeutic benefit of DC vaccines as monotherapies may have been limited due to the resistance promoted by MDSCs, which is supported by the extensive literature that address the conflicting results regarding the levels of MDSCs after using DC vaccines. However, combinatorial approaches with other drugs such as IMiDs, antimetabolites, or chemotherapeutic agents have shown to reduce tumor growth and improve overall survivals in vivo compared to DC vaccines alone, which have been also associated with a significant depletion of MDSCs in clinical trials. Therefore, it seems clear that targeting MDSCs with combinatorial regimens based on DC vaccination plus different types of treatment may be therapeutically useful. However, we should take into consideration some aspects, including (a) the type of DCs used in the vaccine (e.g., cDCs can initiate effective immune responses, whereas moDCs seems to play a dual role in cancer) (b), the type of adjuvant (e.g., some vaccines have been loaded with TAAs, but others with dying tumor cells or RB) (c), the dosage used, that may depends on the status of the patients (e.g. levels of blood lymphocytes or MDSCs) and may be personalized, and (d) adverse effects (e.g., as it is with the use of IFN-α therapies). Altogether, some therapeutic approaches to overcome MDSC-mediated immunosuppression and improve survival rates may be focused on DC vaccination combined with MDSC inhibitors (e.g., receptor antagonists of cytokines or chemokines that recruit MDSC into the tumor), which may be promising, alternative strategies to be tested in vitro and in vivo in the future.
MLS-L, C-J-C, GC, MV, LC-M, and VS-M contributed to conceptualization, literature search, and reviewing of the draft. MLS-L, and CJ-C wrote the draft. All authors have read and agreed to publish this version of the manuscript.
CJ-C is supported by a Margarita Salas fellowship, granted by the University of Seville (Seville, Spain).
The authors declare that the research was conducted in the absence of any commercial or financial relationships that could be construed as a potential conflict of interest.
All claims expressed in this article are solely those of the authors and do not necessarily represent those of their affiliated organizations, or those of the publisher, the editors and the reviewers. Any product that may be evaluated in this article, or claim that may be made by its manufacturer, is not guaranteed or endorsed by the publisher.
1. Anderson NM, Simon MC. The tumor microenvironment. Curr Biol (2020) 30(16):R921–5. doi: 10.1016/j.cub.2020.06.081
2. Lindau D, Gielen P, Kroesen M, Wesseling P, Adema GJ. The immunosuppressive tumour network: myeloid-derived suppressor cells, regulatory T cells and natural killer T cells. Immunology (2013) 138(2):105–15. doi: 10.1111/imm.12036
3. Davidov V, Jensen G, Mai S, Chen SH, Pan PY. Analyzing one cell at a TIME: Analysis of myeloid cell contributions in the tumor immune microenvironment. Front Immunol (2020) 11:1842. doi: 10.3389/fimmu.2020.01842
4. Labani-Motlagh A, Ashja-Mahdavi M, Loskog A. The tumor microenvironment: A milieu hindering and obstructing antitumor immune responses. Front Immunol (2020) 11:940. doi: 10.3389/fimmu.2020.00940
5. Gao D, Joshi N, Choi H, Ryu S, Hahn M, Catena R, et al. Myeloid progenitor cells in the premetastatic lung promote metastases by inducing mesenchymal to epithelial transition. Cancer Res (2012) 72(6):1384–94. doi: 10.1158/0008-5472.CAN-11-2905
6. Sceneay J, Chow MT, Chen A, Halse HM, Wong CS, Andrews DM, et al. Primary tumor hypoxia recruits CD11b+/Ly6Cmed/Ly6G+ immune suppressor cells and compromises NK cell cytotoxicity in the premetastatic niche. Cancer Res (2012) 72(16):3906–11. doi: 10.1158/0008-5472.CAN-11-3873
7. Sica A, Bronte V. Altered macrophage differentiation and immune dysfunction in tumor development. J Clin Invest (2007) 117(5):1155–66. doi: 10.1172/JCI31422
8. Li BH, Garstka MA, Li ZF. Chemokines and their receptors promoting the recruitment of myeloid-derived suppressor cells into the tumor. Mol Immunol (2020) 117:201–15. doi: 10.1016/j.molimm.2019.11.014
9. Bronte V, Brandau S, Chen SH, Colombo MP, Frey AB, Greten TF, et al. Recommendations for myeloid-derived suppressor cell nomenclature and characterization standards. Nat Commun (2016) 7:12150. doi: 10.1038/ncomms12150
10. Gabrilovich DI, Ostrand-Rosenberg S, Bronte V. Coordinated regulation of myeloid cells by tumours. Nat Rev Immunol (2012) 12(4):253–68. doi: 10.1038/nri3175
11. Srivastava MK, Sinha P, Clements VK, Rodriguez P, Ostrand-Rosenberg S. Myeloid-derived suppressor cells inhibit T-cell activation by depleting cystine and cysteine. Cancer Res (2010) 70(1):68–77. doi: 10.1158/0008-5472.CAN-09-2587
12. Rodriguez PC, Ochoa AC. Arginine regulation by myeloid derived suppressor cells and tolerance in cancer: mechanisms and therapeutic perspectives. Immunol Rev (2008) 222:180–91. doi: 10.1111/j.1600-065X.2008.00608.x
13. Ohl K, Tenbrock K. Reactive oxygen species as regulators of MDSC-mediated immune suppression. Front Immunol (2018) 9:2499. doi: 10.3389/fimmu.2018.02499
14. Bruno A, Mortara L, Baci D, Noonan DM, Albini A. Myeloid derived suppressor cells interactions with natural killer cells and pro-angiogenic activities: Roles in tumor progression. Front Immunol (2019) 10:771. doi: 10.3389/fimmu.2019.00771
15. Beury DW, Parker KH, Nyandjo M, Sinha P, Carter KA, Ostrand-Rosenberg S. Cross-talk among myeloid-derived suppressor cells, macrophages, and tumor cells impacts the inflammatory milieu of solid tumors. J Leukoc Biol (2014) 96(6):1109–18. doi: 10.1189/jlb.3A0414-210R
16. Huang B, Pan PY, Li Q, Sato AI, Levy DE, Bromberg J, et al. Gr-1+CD115+ immature myeloid suppressor cells mediate the development of tumor-induced T regulatory cells and T-cell anergy in tumor-bearing host. Cancer Res (2006) 66(2):1123–31. doi: 10.1158/0008-5472.CAN-05-1299
17. Behranvand N, Nasri F, Zolfaghari Emameh R, Khani P, Hosseini A, Garssen J, et al. Chemotherapy: a double-edged sword in cancer treatment. Cancer Immunol Immunother (2022) 71(3):507–26. doi: 10.1007/s00262-021-03013-3
18. Baskar R, Lee KA, Yeo R, Yeoh KW. Cancer and radiation therapy: current advances and future directions. Int J Med Sci (2012) 9(3):193–9. doi: 10.7150/ijms.3635
19. Jimenez-Cortegana C, Galassi C, Klapp V, Gabrilovich DI, Galluzzi L. Myeloid-derived suppressor cells and radiotherapy. Cancer Immunol Res (2022) 10(5):545–57. doi: 10.1158/2326-6066.CIR-21-1105
20. Barrett RL, Pure E. Cancer-associated fibroblasts and their influence on tumor immunity and immunotherapy. Elife (2020) 9:e57243. doi: 10.7554/eLife.57243
21. Buchbinder EI, Desai A. CTLA-4 and PD-1 pathways: Similarities, differences, and implications of their inhibition. Am J Clin Oncol (2016) 39(1):98–106. doi: 10.1097/COC.0000000000000239
22. Lopes-Coelho F, Martins F, Pereira SA, Serpa J. Anti-angiogenic therapy: Current challenges and future perspectives. Int J Mol Sci (2021) 22(7):3765. doi: 10.3390/ijms22073765
23. Eisenbarth SC. Dendritic cell subsets in T cell programming: location dictates function. Nat Rev Immunol (2019) 19(2):89–103. doi: 10.1038/s41577-018-0088-1
24. Chen DS, Mellman I. Oncology meets immunology: the cancer-immunity cycle. Immunity (2013) 39(1):1–10. doi: 10.1016/j.immuni.2013.07.012
25. Moriya T, Kitagawa K, Hayakawa Y, Hemmi H, Kaisho T, Ueha S, et al. Immunogenic tumor cell death promotes dendritic cell migration and inhibits tumor growth via enhanced T cell immunity. iScience (2021) 24(5):102424. doi: 10.1016/j.isci.2021.102424
26. Tesniere A, Panaretakis T, Kepp O, Apetoh L, Ghiringhelli F, Zitvogel L, et al. Molecular characteristics of immunogenic cancer cell death. Cell Death Differ (2008) 15(1):3–12. doi: 10.1038/sj.cdd.4402269
27. Gardner A, Ruffell B. Dendritic cells and cancer immunity. Trends Immunol (2016) 37(12):855–65. doi: 10.1016/j.it.2016.09.006
28. Azeem W, Bakke RM, Appel S, Oyan AM, Kalland KH. Dual pro- and anti-inflammatory features of monocyte-derived dendritic cells. Front Immunol (2020) 11:438. doi: 10.3389/fimmu.2020.00438
29. Hernandez SS, Jakobsen MR, Bak RO. Plasmacytoid dendritic cells as a novel cell-based cancer immunotherapy. Int J Mol Sci (2022) 23(19):11397. doi: 10.3390/ijms231911397
30. Steven A, Fisher SA, Robinson BW. Immunotherapy for lung cancer. Respirology (2016) 21(5):821–33. doi: 10.1111/resp.12789
31. Bilusic M, Madan RA, Gulley JL. Immunotherapy of prostate cancer: Facts and hopes. Clin Cancer Res (2017) 23(22):6764–70. doi: 10.1158/1078-0432.CCR-17-0019
32. Basu A, Ramamoorthi G, Jia Y, Faughn J, Wiener D, Awshah S, et al. Immunotherapy in breast cancer: Current status and future directions. Adv Cancer Res (2019) 143:295–349. doi: 10.1016/bs.acr.2019.03.006
33. Johdi NA, Sukor NF. Colorectal cancer immunotherapy: Options and strategies. Front Immunol (2020) 11:1624. doi: 10.3389/fimmu.2020.01624
34. Miyagawa S, Soeda J, Takagi S, Miwa S, Ichikawa E, Noike T. Prognostic significance of mature dendritic cells and factors associated with their accumulation in metastatic liver tumors from colorectal cancer. Hum Pathol (2004) 35(11):1392–6. doi: 10.1016/j.humpath.2004.07.018
35. Melaiu O, Chierici M, Lucarini V, Jurman G, Conti LA, De Vito R, et al. Cellular and gene signatures of tumor-infiltrating dendritic cells and natural-killer cells predict prognosis of neuroblastoma. Nat Commun (2020) 11(1):5992. doi: 10.1038/s41467-020-19781-y
36. Szpor J, Streb J, Glajcar A, Fraczek P, Winiarska A, Tyrak KE, et al. Dendritic cells are associated with prognosis and survival in breast cancer. Diagnostics (Basel) (2021) 11(4):702. doi: 10.3390/diagnostics11040702
37. Gardner A, de Mingo Pulido A, Ruffell B. Dendritic cells and their role in immunotherapy. Front Immunol (2020) 11:924. doi: 10.3389/fimmu.2020.00924
38. Block MS, Dietz AB, Gustafson MP, Kalli KR, Erskine CL, Youssef B, et al. Th17-inducing autologous dendritic cell vaccination promotes antigen-specific cellular and humoral immunity in ovarian cancer patients. Nat Commun (2020) 11(1):5173. doi: 10.1038/s41467-020-18962-z
39. Carreno BM, Magrini V, Becker-Hapak M, Kaabinejadian S, Hundal J, Petti AA, et al. Cancer immunotherapy. a dendritic cell vaccine increases the breadth and diversity of melanoma neoantigen-specific T cells. Science (2015) 348(6236):803–8. doi: 10.1126/science.aaa3828
40. Liau LM, Ashkan K, Tran DD, Campian JL, Trusheim JE, Cobbs CS, et al. First results on survival from a large phase 3 clinical trial of an autologous dendritic cell vaccine in newly diagnosed glioblastoma. J Transl Med (2018) 16(1):142. doi: 10.1186/s12967-018-1507-6
41. Ribas A, Comin-Anduix B, Chmielowski B, Jalil J, de la Rocha P, McCannel TA, et al. Dendritic cell vaccination combined with CTLA4 blockade in patients with metastatic melanoma. Clin Cancer Res (2009) 15(19):6267–76. doi: 10.1158/1078-0432.CCR-09-1254
42. Esmaily M, Masjedi A, Hallaj S, Nabi Afjadi M, Malakotikhah F, Ghani S, et al. Blockade of CTLA-4 increases anti-tumor response inducing potential of dendritic cell vaccine. J Control Release (2020) 326:63–74. doi: 10.1016/j.jconrel.2020.06.017
43. Kodumudi KN, Ramamoorthi G, Snyder C, Basu A, Jia Y, Awshah S, et al. Sequential anti-PD1 therapy following dendritic cell vaccination improves survival in a HER2 mammary carcinoma model and identifies a critical role for CD4 T cells in mediating the response. Front Immunol (2019) 10:1939. doi: 10.3389/fimmu.2019.01939
44. Zhou Y, Slone N, Chrisikos TT, Kyrysyuk O, Babcock RL, Medik YB, et al. Vaccine efficacy against primary and metastatic cancer with in vitro-generated CD103(+) conventional dendritic cells. J Immunother Cancer (2020) 8(1):e000474. doi: 10.1136/jitc-2019-000474
45. Johnson P, Rosendahl N, Radford KJ. Conventional type 1 dendritic cells (cDC1) as cancer therapeutics: challenges and opportunities. Expert Opin Biol Ther (2022) 22(4):465–72. doi: 10.1080/14712598.2022.1994943
46. Bol KF, Schreibelt G, Rabold K, Wculek SK, Schwarze JK, Dzionek A, et al. The clinical application of cancer immunotherapy based on naturally circulating dendritic cells. J Immunother Cancer (2019) 7(1):109. doi: 10.1186/s40425-019-0580-6
47. Baldin AV, Savvateeva LV, Bazhin AV, Zamyatnin AA Jr. Dendritic cells in anticancer vaccination: Rationale for ex vivo loading or In vivo targeting. Cancers (Basel) (2020) 12(3):590. doi: 10.3390/cancers12030590
48. Chiang CL, Kandalaft LE. In vivo cancer vaccination: Which dendritic cells to target and how? Cancer Treat Rev (2018) 71:88–101. doi: 10.1016/j.ctrv.2018.10.012
49. Arab S, Kheshtchin N, Ajami M, Ashurpoor M, Safvati A, Namdar A, et al. Increased efficacy of a dendritic cell-based therapeutic cancer vaccine with adenosine receptor antagonist and CD73 inhibitor. Tumour Biol (2017) 39(3):1010428317695021. doi: 10.1177/1010428317695021
50. Khosravianfar N, Hadjati J, Namdar A, Boghozian R, Hafezi M, Ashourpour M, et al. Myeloid-derived suppressor cells elimination by 5-fluorouracil increased dendritic cell-based vaccine function and improved immunity in tumor mice. Iran J Allergy Asthma Immunol (2018) 17(1):47–55.
51. Zhu XJ, Yang ZF, Zhou JY, Liu L, Sun XM, Fan ZF, et al. Progression of Large lymphoma is significantly impeded with a combination of gemcitabine chemotherapy and dendritic cells intra-tumor vaccination. PloS One (2015) 10(7):e0132799. doi: 10.1371/journal.pone.0132799
52. Vo MC, Jung SH, Chu TH, Lee HJ, Lakshmi TJ, Park HS, et al. Lenalidomide and programmed death-1 blockade synergistically enhances the effects of dendritic cell vaccination in a model of murine myeloma. Front Immunol (2018) 9:1370. doi: 10.3389/fimmu.2018.01370
53. Chu TH, Vo MC, Park HS, Lakshmi TJ, Jung SH, Kim HJ, et al. Potent anti-myeloma efficacy of dendritic cell therapy in combination with pomalidomide and programmed death-ligand 1 blockade in a preclinical model of multiple myeloma. Cancer Immunol Immunother (2021) 70(1):31–45. doi: 10.1007/s00262-020-02654-0
54. Vo MC, Nguyen-Pham TN, Lee HJ, Jaya Lakshmi T, Yang S, Jung SH, et al. Combination therapy with dendritic cells and lenalidomide is an effective approach to enhance antitumor immunity in a mouse colon cancer model. Oncotarget (2017) 8(16):27252–62. doi: 10.18632/oncotarget.15917
55. Zhang L, Du J, Song Q, Zhang C, Wu X. A novel In situ dendritic cell vaccine triggered by rose Bengal enhances adaptive antitumour immunity. J Immunol Res (2022) 2022:1178874. doi: 10.1155/2022/1178874
56. Lowe DB, Bose A, Taylor JL, Tawbi H, Lin Y, Kirkwood JM, et al. Dasatinib promotes the expansion of a therapeutically superior T-cell repertoire in response to dendritic cell vaccination against melanoma. Oncoimmunology (2014) 3(1):e27589. doi: 10.4161/onci.27589
57. Nair SK, Snyder D, Rouse BT, Gilboa E. Regression of tumors in mice vaccinated with professional antigen-presenting cells pulsed with tumor extracts. Int J Cancer (1997) 70(6):706–15. doi: 10.1002/(SICI)1097-0215(19970317)70:6<706::AID-IJC13>3.0.CO;2-7
58. Casati A, Zimmermann VS, Benigni F, Bertilaccio MT, Bellone M, Mondino A. The immunogenicity of dendritic cell-based vaccines is not hampered by doxorubicin and melphalan administration. J Immunol (2005) 174(6):3317–25. doi: 10.4049/jimmunol.174.6.3317
59. Shih NY, Yang HY, Cheng HT, Hung YM, Yao YC, Zhu YH, et al. Conditioning vaccination site with irradiated MIP-3alpha-transfected tumor cells enhances efficacy of dendritic cell-based cancer vaccine. J Immunother (2009) 32(4):363–9. doi: 10.1097/CJI.0b013e31819d29d8
60. Wei SM, Fei JX, Tao F, Pan HL, Shen Q, Wang L, et al. Anti-CD27 antibody potentiates antitumor effect of dendritic cell-based vaccine in prostate cancer-bearing mice. Int Surg (2015) 100(1):155–63. doi: 10.9738/INTSURG-D-14-00147.1
61. Lapenta C, Donati S, Spadaro F, Lattanzi L, Urbani F, Macchia I, et al. Lenalidomide improves the therapeutic effect of an interferon-alpha-dendritic cell-based lymphoma vaccine. Cancer Immunol Immunother (2019) 68(11):1791–804. doi: 10.1007/s00262-019-02411-y
62. Ferris ST, Ohara RA, Ou F, Wu R, Huang X, Kim S, et al. cDC1 vaccines drive tumor rejection by direct presentation independently of host cDC1. Cancer Immunol Res (2022) 10(8):920–31. doi: 10.1158/2326-6066.CIR-21-0865
63. Zhou J, Xi Y, Mu X, Zhao R, Chen H, Zhang L, et al. Antitumor immunity induced by VE-cadherin modified DC vaccine. Oncotarget (2017) 8(40):67369–79. doi: 10.18632/oncotarget.18654
64. Jadidi-Niaragh F, Atyabi F, Rastegari A, Kheshtchin N, Arab S, Hassannia H, et al. CD73 specific siRNA loaded chitosan lactate nanoparticles potentiate the antitumor effect of a dendritic cell vaccine in 4T1 breast cancer bearing mice. J Control Release (2017) 246:46–59. doi: 10.1016/j.jconrel.2016.12.012
65. Vo MC, Lee HJ, Kim JS, Hoang MD, Choi NR, Rhee JH, et al. Dendritic cell vaccination with a toll-like receptor agonist derived from mycobacteria enhances anti-tumor immunity. Oncotarget (2015) 6(32):33781–90. doi: 10.18632/oncotarget.5281
66. Vandenberk L, Garg AD, Verschuere T, Koks C, Belmans J, Beullens M, et al. Irradiation of necrotic cancer cells, employed for pulsing dendritic cells (DCs), potentiates DC vaccine-induced antitumor immunity against high-grade glioma. Oncoimmunology (2016) 5(2):e1083669. doi: 10.1080/2162402X.2015.1083669
67. Hu Z, Chen J, Zhou S, Yang N, Duan S, Zhang Z, et al. Mouse IP-10 gene delivered by folate-modified chitosan nanoparticles and dendritic/tumor cells fusion vaccine effectively inhibit the growth of hepatocellular carcinoma in mice. Theranostics (2017) 7(7):1942–52. doi: 10.7150/thno.16236
68. Ohshio Y, Teramoto K, Hanaoka J, Tezuka N, Itoh Y, Asai T, et al. Cancer-associated fibroblast-targeted strategy enhances antitumor immune responses in dendritic cell-based vaccine. Cancer Sci (2015) 106(2):134–42. doi: 10.1111/cas.12584
69. Rahbar S, Shafiekhani S, Allahverdi A, Jamali A, Kheshtchin N, Ajami M, et al. Agent-based modeling of tumor and immune system interactions in combinational therapy with low-dose 5-fluorouracil and dendritic cell vaccine in melanoma B16F10. Iran J Allergy Asthma Immunol (2022) 21(2):151–66. doi: 10.18502/ijaai.v21i2.9223
70. Grees M, Sharbi-Yunger A, Evangelou C, Baumann D, Cafri G, Tzehoval E, et al. Optimized dendritic cell vaccination induces potent CD8 T cell responses and anti-tumor effects in transgenic mouse melanoma models. Oncoimmunology (2018) 7(7):e1445457. doi: 10.1080/2162402X.2018.1445457
71. Vo MC, Yang S, Jung SH, Chu TH, Lee HJ, Lakshmi TJ, et al. Synergistic antimyeloma activity of dendritic cells and pomalidomide in a murine myeloma model. Front Immunol (2018) 9:1798. doi: 10.3389/fimmu.2018.01798
72. Xiao L, Erb U, Zhao K, Hackert T, Zoller M. Efficacy of vaccination with tumor-exosome loaded dendritic cells combined with cytotoxic drug treatment in pancreatic cancer. Oncoimmunology (2017) 6(6):e1319044. doi: 10.1080/2162402X.2017.1319044
73. Ghansah T, Vohra N, Kinney K, Weber A, Kodumudi K, Springett G, et al. Dendritic cell immunotherapy combined with gemcitabine chemotherapy enhances survival in a murine model of pancreatic carcinoma. Cancer Immunol Immunother (2013) 62(6):1083–91. doi: 10.1007/s00262-013-1407-9
74. McDaniel JM, Pinilla-Ibarz J, Epling-Burnette PK. Molecular action of lenalidomide in lymphocytes and hematologic malignancies. Adv Hematol (2012) 2012:513702. doi: 10.1155/2012/513702
75. Chanan-Khan AA, Swaika A, Paulus A, Kumar SK, Mikhael JR, Rajkumar SV, et al. Pomalidomide: the new immunomodulatory agent for the treatment of multiple myeloma. Blood Cancer J (2013) 3:e143. doi: 10.1038/bcj.2013.38
76. Jimenez-Cortegana C, Palazon-Carrion N, Martin Garcia-Sancho A, Nogales-Fernandez E, Carnicero-Gonzalez F, Rios-Herranz E, et al. Circulating myeloid-derived suppressor cells and regulatory T cells as immunological biomarkers in refractory/relapsed diffuse large b-cell lymphoma: translational results from the R2-GDP-GOTEL trial. J Immunother Cancer (2021) 9(6):e002323. doi: 10.1136/jitc-2020-002323
77. Sakamaki I, Kwak LW, Cha SC, Yi Q, Lerman B, Chen J, et al. Lenalidomide enhances the protective effect of a therapeutic vaccine and reverses immune suppression in mice bearing established lymphomas. Leukemia (2014) 28(2):329–37. doi: 10.1038/leu.2013.177
78. Rychak E, Mendy D, Shi T, Ning Y, Leisten J, Lu L, et al. Pomalidomide in combination with dexamethasone results in synergistic anti-tumour responses in pre-clinical models of lenalidomide-resistant multiple myeloma. Br J Haematol (2016) 172(6):889–901. doi: 10.1111/bjh.13905
79. Ocio EM, Fernandez-Lazaro D, San-Segundo L, Lopez-Corral L, Corchete LA, Gutierrez NC, et al. In vivo murine model of acquired resistance in myeloma reveals differential mechanisms for lenalidomide and pomalidomide in combination with dexamethasone. Leukemia (2015) 29(3):705–14. doi: 10.1038/leu.2014.238
80. Paino T, Gonzalez-Mendez L, San-Segundo L, Corchete LA, Hernandez-Garcia S, Diaz-Tejedor A, et al. Protein translation inhibition is involved in the activity of the pan-PIM kinase inhibitor PIM447 in combination with pomalidomide-dexamethasone in multiple myeloma. Cancers (Basel) (2020) 12(10):2743. doi: 10.3390/cancers12102743
81. Hernandez-Garcia S, San-Segundo L, Gonzalez-Mendez L, Corchete LA, Misiewicz-Krzeminska I, Martin-Sanchez M, et al. The kinesin spindle protein inhibitor filanesib enhances the activity of pomalidomide and dexamethasone in multiple myeloma. Haematologica (2017) 102(12):2113–24. doi: 10.3324/haematol.2017.168666
82. Sanchez E, Li M, Wang CS, Tang G, Gillespie A, Chen H, et al. Anti-angiogenic and anti-multiple myeloma effects of oprozomib (OPZ) alone and in combination with pomalidomide (Pom) and/or dexamethasone (Dex). Leuk Res (2017) 57:45–54. doi: 10.1016/j.leukres.2017.03.002
83. Koinis F, Vetsika EK, Aggouraki D, Skalidaki E, Koutoulaki A, Gkioulmpasani M, et al. Effect of first-line treatment on myeloid-derived suppressor cells' subpopulations in the peripheral blood of patients with non-small cell lung cancer. J Thorac Oncol (2016) 11(8):1263–72. doi: 10.1016/j.jtho.2016.04.026
84. Horikawa N, Abiko K, Matsumura N, Hamanishi J, Baba T, Yamaguchi K, et al. Expression of vascular endothelial growth factor in ovarian cancer inhibits tumor immunity through the accumulation of myeloid-derived suppressor cells. Clin Cancer Res (2017) 23(2):587–99. doi: 10.1158/1078-0432.CCR-16-0387
85. Salewski I, Kuntoff S, Kuemmel A, Feldtmann R, Felix SB, Henze L, et al. Combined vaccine-immune-checkpoint inhibition constitutes a promising strategy for treatment of dMMR tumors. Cancer Immunol Immunother (2021) 70(12):3405–19. doi: 10.1007/s00262-021-02933-4
86. Du Y, Liu Y, Wang D, Bai H, Wang Z, He X, et al. Peptidic microarchitecture-trapped tumor vaccine combined with immune checkpoint inhibitor or PI3Kgamma inhibitor can enhance immunogenicity and eradicate tumors. J Immunother Cancer (2022) 10(2):e003564. doi: 10.1136/jitc-2021-003564
87. Liu T, Han C, Wang S, Fang P, Ma Z, Xu L, et al. Cancer-associated fibroblasts: an emerging target of anti-cancer immunotherapy. J Hematol Oncol (2019) 12(1):86. doi: 10.1186/s13045-019-0770-1
88. Sahai E, Astsaturov I, Cukierman E, DeNardo DG, Egeblad M, Evans RM, et al. A framework for advancing our understanding of cancer-associated fibroblasts. Nat Rev Cancer (2020) 20(3):174–86. doi: 10.1038/s41568-019-0238-1
89. Lin Y, Cai Q, Chen Y, Shi T, Liu W, Mao L, et al. CAFs shape myeloid-derived suppressor cells to promote stemness of intrahepatic cholangiocarcinoma through 5-lipoxygenase. Hepatology (2022) 75(1):28–42. doi: 10.1002/hep.32099
90. Pereira BA, Vennin C, Papanicolaou M, Chambers CR, Herrmann D, Morton JP, et al. CAF subpopulations: A new reservoir of stromal targets in pancreatic cancer. Trends Cancer (2019) 5(11):724–41. doi: 10.1016/j.trecan.2019.09.010
91. Appel CK, Gallego-Pedersen S, Andersen L, Blancheflor Kristensen S, Ding M, Falk S, et al. The src family kinase inhibitor dasatinib delays pain-related behaviour and conserves bone in a rat model of cancer-induced bone pain. Sci Rep (2017) 7(1):4792. doi: 10.1038/s41598-017-05029-1
92. Yu GT, Mao L, Wu L, Deng WW, Bu LL, Liu JF, et al. Inhibition of SRC family kinases facilitates anti-CTLA4 immunotherapy in head and neck squamous cell carcinoma. Cell Mol Life Sci (2018) 75(22):4223–34. doi: 10.1007/s00018-018-2863-3
93. Giallongo C, Parrinello NL, La Cava P, Camiolo G, Romano A, Scalia M, et al. Monocytic myeloid-derived suppressor cells as prognostic factor in chronic myeloid leukaemia patients treated with dasatinib. J Cell Mol Med (2018) 22(2):1070–80. doi: 10.1111/jcmm.13326
94. Longley DB, Harkin DP, Johnston PG. 5-fluorouracil: mechanisms of action and clinical strategies. Nat Rev Cancer (2003) 3(5):330–8. doi: 10.1038/nrc1074
95. Vincent J, Mignot G, Chalmin F, Ladoire S, Bruchard M, Chevriaux A, et al. 5-fluorouracil selectively kills tumor-associated myeloid-derived suppressor cells resulting in enhanced T cell-dependent antitumor immunity. Cancer Res (2010) 70(8):3052–61. doi: 10.1158/0008-5472.CAN-09-3690
96. Wu FY, Wu CW. Rose Bengal: an inhibitor of ribonucleic acid chain elongation. Biochemistry (1973) 12(22):4343–8. doi: 10.1021/bi00746a007
97. Liu H, Innamarato PP, Kodumudi K, Weber A, Nemoto S, Robinson JL, et al. Intralesional rose bengal in melanoma elicits tumor immunity via activation of dendritic cells by the release of high mobility group box 1. Oncotarget (2016) 7(25):37893–905. doi: 10.18632/oncotarget.9247
98. Kramer ED, Abrams SI. Granulocytic myeloid-derived suppressor cells as negative regulators of anticancer immunity. Front Immunol (2020) 11:1963. doi: 10.3389/fimmu.2020.01963
99. Le HK, Graham L, Cha E, Morales JK, Manjili MH, Bear HD. Gemcitabine directly inhibits myeloid derived suppressor cells in BALB/c mice bearing 4T1 mammary carcinoma and augments expansion of T cells from tumor-bearing mice. Int Immunopharmacol (2009) 9(7-8):900–9. doi: 10.1016/j.intimp.2009.03.015
100. Gargett T, Christo SN, Hercus TR, Abbas N, Singhal N, Lopez AF, et al. GM-CSF signalling blockade and chemotherapeutic agents act in concert to inhibit the function of myeloid-derived suppressor cells in vitro. Clin Transl Immunol (2016) 5(12):e119. doi: 10.1038/cti.2016.80
101. Wang Z, Liu Y, Zhang Y, Shang Y, Gao Q. MDSC-decreasing chemotherapy increases the efficacy of cytokine-induced killer cell immunotherapy in metastatic renal cell carcinoma and pancreatic cancer. Oncotarget (2016) 7(4):4760–9. doi: 10.18632/oncotarget.6734
102. Babu A, Ramesh R. Multifaceted applications of chitosan in cancer drug delivery and therapy. Mar Drugs (2017) 15(4):96. doi: 10.3390/md15040096
103. Yu B, Tang C, Yin C. Enhanced antitumor efficacy of folate modified amphiphilic nanoparticles through co-delivery of chemotherapeutic drugs and genes. Biomaterials (2014) 35(24):6369–78. doi: 10.1016/j.biomaterials.2014.04.095
104. Duan S, Song M, He J, Zhou N, Zhou S, Zhao J, et al. Folate-modified chitosan nanoparticles coated interferon-inducible protein-10 gene enhance cytotoxic T lymphocytes' responses to hepatocellular carcinoma. J BioMed Nanotechnol (2016) 12(4):700–9. doi: 10.1166/jbn.2016.2216
105. Zhu H, Cao J, Cui S, Qian Z, Gu Y. Enhanced tumor targeting and antitumor efficacy via hydroxycamptothecin-encapsulated folate-modified n-succinyl-N'-octyl chitosan micelles. J Pharm Sci (2013) 102(4):1318–32. doi: 10.1002/jps.23470
106. He J, Duan S, Yu X, Qian Z, Zhou S, Zhang Z, et al. Folate-modified chitosan nanoparticles containing the IP-10 gene enhance melanoma-specific cytotoxic CD8(+)CD28(+) T lymphocyte responses. Theranostics (2016) 6(5):752–61. doi: 10.7150/thno.14527
107. de Leve S, Wirsdorfer F, Jendrossek V. Targeting the immunomodulatory CD73/Adenosine system to improve the therapeutic gain of radiotherapy. Front Immunol (2019) 10:698. doi: 10.3389/fimmu.2019.00698
108. Allard D, Allard B, Gaudreau PO, Chrobak P, Stagg J. CD73-adenosine: a next-generation target in immuno-oncology. Immunotherapy (2016) 8(2):145–63. doi: 10.2217/imt.15.106
109. Loi S, Pommey S, Haibe-Kains B, Beavis PA, Darcy PK, Smyth MJ, et al. CD73 promotes anthracycline resistance and poor prognosis in triple negative breast cancer. Proc Natl Acad Sci USA (2013) 110(27):11091–6. doi: 10.1073/pnas.1222251110
110. Vetsika EK, Koukos A, Kotsakis A. Myeloid-derived suppressor cells: Major figures that shape the immunosuppressive and angiogenic network in cancer. Cells (2019) 8(12):1647. doi: 10.3390/cells8121647
111. Duong CN, Vestweber D. Mechanisms ensuring endothelial junction integrity beyond VE-cadherin. Front Physiol (2020) 11:519. doi: 10.3389/fphys.2020.00519
112. Wallez Y, Vilgrain I, Huber P. Angiogenesis: the VE-cadherin switch. Trends Cardiovasc Med (2006) 16(2):55–9. doi: 10.1016/j.tcm.2005.11.008
113. Labelle M, Schnittler HJ, Aust DE, Friedrich K, Baretton G, Vestweber D, et al. Vascular endothelial cadherin promotes breast cancer progression via transforming growth factor beta signaling. Cancer Res (2008) 68(5):1388–97. doi: 10.1158/0008-5472.CAN-07-2706
114. Yu W, Yang L, Li T, Zhang Y. Cadherin signaling in cancer: Its functions and role as a therapeutic target. Front Oncol (2019) 9:989. doi: 10.3389/fonc.2019.00989
115. Choi HG, Choi S, Back YW, Paik S, Park HS, Kim WS, et al. Rv2299c, a novel dendritic cell-activating antigen of mycobacterium tuberculosis, fused-ESAT-6 subunit vaccine confers improved and durable protection against the hypervirulent strain HN878 in mice. Oncotarget (2017) 8(12):19947–67. doi: 10.18632/oncotarget.15256
116. Fucikova J, Hensler M, Kasikova L, Lanickova T, Pasulka J, Rakova J, et al. An autologous dendritic cell vaccine promotes anticancer immunity in patients with ovarian cancer with low mutational burden and cold tumors. Clin Cancer Res (2022) 28(14):3053–65. doi: 10.1158/1078-0432.CCR-21-4413
117. Nickles E, Dharmadhikari B, Yating L, Walsh RJ, Koh LP, Poon M, et al. Dendritic cell therapy with CD137L-DC-EBV-VAX in locally recurrent or metastatic nasopharyngeal carcinoma is safe and confers clinical benefit. Cancer Immunol Immunother (2022) 71(6):1531–43. doi: 10.1007/s00262-021-03075-3
118. Vogelzang NJ, Beer TM, Gerritsen W, Oudard S, Wiechno P, Kukielka-Budny B, et al. Efficacy and safety of autologous dendritic cell-based immunotherapy, docetaxel, and prednisone vs placebo in patients with metastatic castration-resistant prostate cancer: The VIABLE phase 3 randomized clinical trial. JAMA Oncol (2022) 8(4):546–52. doi: 10.1001/jamaoncol.2021.7298
119. Hu JL, Omofoye OA, Rudnick JD, Kim S, Tighiouart M, Phuphanich S, et al. A phase I study of autologous dendritic cell vaccine pulsed with allogeneic stem-like cell line lysate in patients with newly diagnosed or recurrent glioblastoma. Clin Cancer Res (2022) 28(4):689–96. doi: 10.1158/1078-0432.CCR-21-2867
120. Tryggestad AMA, Axcrona K, Axcrona U, Bigalke I, Brennhovd B, Inderberg EM, et al. Long-term first-in-man phase I/II study of an adjuvant dendritic cell vaccine in patients with high-risk prostate cancer after radical prostatectomy. Prostate (2022) 82(2):245–53. doi: 10.1002/pros.24267
121. Ota S, Miyashita M, Yamagishi Y, Ogasawara M. Baseline immunity predicts prognosis of pancreatic cancer patients treated with WT1 and/or MUC1 peptide-loaded dendritic cell vaccination and a standard chemotherapy. Hum Vaccin Immunother (2021) 17(12):5563–72. doi: 10.1080/21645515.2021.2003645
122. Storkus WJ, Maurer D, Lin Y, Ding F, Bose A, Lowe D, et al. Dendritic cell vaccines targeting tumor blood vessel antigens in combination with dasatinib induce therapeutic immune responses in patients with checkpoint-refractory advanced melanoma. J Immunother Cancer (2021) 9(11):e003675. doi: 10.1136/jitc-2021-003675
123. Chevallier P, Saiagh S, Dehame V, Guillaume T, Peterlin P, Bercegeay S, et al. A phase I/II feasibility vaccine study by autologous leukemic apoptotic corpse-pulsed dendritic cells for elderly AML patients. Hum Vaccin Immunother (2021) 17(10):3511–4. doi: 10.1080/21645515.2021.1943991
124. Olin MR, Low W, McKenna DH, Haines SJ, Dahlheimer T, Nascene D, et al. Vaccination with dendritic cells loaded with allogeneic brain tumor cells for recurrent malignant brain tumors induces a CD4(+)IL17(+) response. J Immunother Cancer (2014) 2:4. doi: 10.1186/2051-1426-2-4
125. Santisteban M, Solans BP, Hato L, Urrizola A, Mejias LD, Salgado E, et al. Final results regarding the addition of dendritic cell vaccines to neoadjuvant chemotherapy in early HER2-negative breast cancer patients: Clinical and translational analysis. Ther Adv Med Oncol (2021) 13:17588359211064653. doi: 10.1177/17588359211064653
126. Zhang W, Lu X, Cui P, Piao C, Xiao M, Liu X, et al. Phase I/II clinical trial of a wilms' tumor 1-targeted dendritic cell vaccination-based immunotherapy in patients with advanced cancer. Cancer Immunol Immunother (2019) 68(1):121–30. doi: 10.1007/s00262-018-2257-2
127. Matsuda T, Takeuchi H, Sakurai T, Mayanagi S, Booka E, Fujita T, et al. Pilot study of WT1 peptide-pulsed dendritic cell vaccination with docetaxel in esophageal cancer. Oncol Lett (2018) 16(1):1348–56. doi: 10.3892/ol.2018.8734
128. Iclozan C, Antonia S, Chiappori A, Chen DT, Gabrilovich D. Therapeutic regulation of myeloid-derived suppressor cells and immune response to cancer vaccine in patients with extensive stage small cell lung cancer. Cancer Immunol Immunother (2013) 62(5):909–18. doi: 10.1007/s00262-013-1396-8
129. Van Wigcheren GF, De Haas N, Mulder TA, Horrevorts SK, Bloemendal M, Hins-Debree S, et al. Cisplatin inhibits frequency and suppressive activity of monocytic myeloid-derived suppressor cells in cancer patients. Oncoimmunology (2021) 10(1):1935557. doi: 10.1080/2162402X.2021.1935557
130. Butterfield LH, Vujanovic L, Santos PM, Maurer DM, Gambotto A, Lohr J, et al. Multiple antigen-engineered DC vaccines with or without IFNalpha to promote antitumor immunity in melanoma. J Immunother Cancer (2019) 7(1):113. doi: 10.1186/s40425-019-0552-x
131. Kongsted P, Borch TH, Ellebaek E, Iversen TZ, Andersen R, Met O, et al. Dendritic cell vaccination in combination with docetaxel for patients with metastatic castration-resistant prostate cancer: A randomized phase II study. Cytotherapy (2017) 19(4):500–13. doi: 10.1016/j.jcyt.2017.01.007
132. Matsushita H, Enomoto Y, Kume H, Nakagawa T, Fukuhara H, Suzuki M, et al. A pilot study of autologous tumor lysate-loaded dendritic cell vaccination combined with sunitinib for metastatic renal cell carcinoma. J Immunother Cancer (2014) 2:30. doi: 10.1186/s40425-014-0030-4
133. Fedorova L, Mudry P, Pilatova K, Selingerova I, Merhautova J, Rehak Z, et al. Assessment of immune response following dendritic cell-based immunotherapy in pediatric patients with relapsing sarcoma. Front Oncol (2019) 9:1169. doi: 10.3389/fonc.2019.01169
134. Blagih J, Zani F, Chakravarty P, Hennequart M, Pilley S, Hobor S, et al. Cancer-specific loss of p53 leads to a modulation of myeloid and T cell responses. Cell Rep (2020) 30(2):481–96.e6. doi: 10.1016/j.celrep.2019.12.028
135. Guo G, Marrero L, Rodriguez P, Del Valle L, Ochoa A, Cui Y. Trp53 inactivation in the tumor microenvironment promotes tumor progression by expanding the immunosuppressive lymphoid-like stromal network. Cancer Res (2013) 73(6):1668–75. doi: 10.1158/0008-5472.CAN-12-3810
136. Wu J, Liu X, Reeser JAW, Trimboli AJ, Pecot T, Sizemore GM, et al. Stromal p53 regulates breast cancer development, the immune landscape, and survival in an oncogene-specific manner. Mol Cancer Res (2022) 20(8):1233–46. doi: 10.1158/1541-7786.MCR-21-0960
137. Youn JI, Collazo M, Shalova IN, Biswas SK, Gabrilovich DI. Characterization of the nature of granulocytic myeloid-derived suppressor cells in tumor-bearing mice. J Leukoc Biol (2012) 91(1):167–81. doi: 10.1189/jlb.0311177
138. Alicea-Torres K, Sanseviero E, Gui J, Chen J, Veglia F, Yu Q, et al. Immune suppressive activity of myeloid-derived suppressor cells in cancer requires inactivation of the type I interferon pathway. Nat Commun (2021) 12(1):1717. doi: 10.1038/s41467-021-22033-2
139. Mundy-Bosse BL, Lesinski GB, Jaime-Ramirez AC, Benninger K, Khan M, Kuppusamy P, et al. Myeloid-derived suppressor cell inhibition of the IFN response in tumor-bearing mice. Cancer Res (2011) 71(15):5101–10. doi: 10.1158/0008-5472.CAN-10-2670
140. Eisenthal A, Cameron RB, Rosenberg SA. Induction of antibody-dependent cellular cytotoxicity in vivo by IFN-alpha and its antitumor efficacy against established B16 melanoma liver metastases when combined with specific anti-B16 monoclonal antibody. J Immunol (1990) 144(11):4463–71.
141. Sleijfer S, Bannink M, Van Gool AR, Kruit WH, Stoter G. Side effects of interferon-alpha therapy. Pharm World Sci (2005) 27(6):423–31. doi: 10.1007/s11096-005-1319-7
142. Motzer RJ, Rini BI, Bukowski RM, Curti BD, George DJ, Hudes GR, et al. Sunitinib in patients with metastatic renal cell carcinoma. JAMA (2006) 295(21):2516–24. doi: 10.1001/jama.295.21.2516
143. Rizzo M, Porta C. Sunitinib in the treatment of renal cell carcinoma: an update on recent evidence. Ther Adv Urol (2017) 9(8):195–207. doi: 10.1177/1756287217713902
144. Kollmannsberger C, Soulieres D, Wong R, Scalera A, Gaspo R, Bjarnason G. Sunitinib therapy for metastatic renal cell carcinoma: recommendations for management of side effects. Can Urol Assoc J (2007) 1(2 Suppl):S41–54. doi: 10.5489/cuaj.67
145. Ko JS, Zea AH, Rini BI, Ireland JL, Elson P, Cohen P, et al. Sunitinib mediates reversal of myeloid-derived suppressor cell accumulation in renal cell carcinoma patients. Clin Cancer Res (2009) 15(6):2148–57. doi: 10.1158/1078-0432.CCR-08-1332
146. Finke JH, Rini B, Ireland J, Rayman P, Richmond A, Golshayan A, et al. Sunitinib reverses type-1 immune suppression and decreases T-regulatory cells in renal cell carcinoma patients. Clin Cancer Res (2008) 14(20):6674–82. doi: 10.1158/1078-0432.CCR-07-5212
147. Xin H, Zhang C, Herrmann A, Du Y, Figlin R, Yu H. Sunitinib inhibition of Stat3 induces renal cell carcinoma tumor cell apoptosis and reduces immunosuppressive cells. Cancer Res (2009) 69(6):2506–13. doi: 10.1158/0008-5472.CAN-08-4323
148. Wei S, Kozono S, Kats L, Nechama M, Li W, Guarnerio J, et al. Active Pin1 is a key target of all-trans retinoic acid in acute promyelocytic leukemia and breast cancer. Nat Med (2015) 21(5):457–66. doi: 10.1038/nm.3839
149. Guruvayoorappan C, Berlin Grace VM. All trans retinoic acid and cancer. Immunopharmacol Immunotoxicol (2011) 33(2):241–9. doi: 10.3109/08923973.2010.521507
150. Darmanin S, Chen J, Zhao S, Cui H, Shirkoohi R, Kubo N, et al. All-trans retinoic acid enhances murine dendritic cell migration to draining lymph nodes via the balance of matrix metalloproteinases and their inhibitors. J Immunol (2007) 179(7):4616–25. doi: 10.4049/jimmunol.179.7.4616
151. Mirza N, Fishman M, Fricke I, Dunn M, Neuger AM, Frost TJ, et al. All-trans-retinoic acid improves differentiation of myeloid cells and immune response in cancer patients. Cancer Res (2006) 66(18):9299–307. doi: 10.1158/0008-5472.CAN-06-1690
152. Saxena M, van der Burg SH, Melief CJM, Bhardwaj N. Therapeutic cancer vaccines. Nat Rev Cancer (2021) 21(6):360–78. doi: 10.1038/s41568-021-00346-0
153. Bol KF, Schreibelt G, Gerritsen WR, de Vries IJ, Figdor CG. Dendritic cell-based immunotherapy: State of the art and beyond. Clin Cancer Res (2016) 22(8):1897–906. doi: 10.1158/1078-0432.CCR-15-1399
154. Truxova I, Hensler M, Skapa P, Halaska MJ, Laco J, Ryska A, et al. Rationale for the combination of dendritic cell-based vaccination approaches with chemotherapy agents. Int Rev Cell Mol Biol (2017) 330:115–56. doi: 10.1016/bs.ircmb.2016.09.003
155. Uruena C, Lasso P, Bernal-Estevez D, Rubio D, Salazar AJ, Olaya M, et al. The breast cancer immune microenvironment is modified by neoadjuvant chemotherapy. Sci Rep (2022) 12(1):7981. doi: 10.1038/s41598-022-12108-5
156. Wesolowski R, Duggan MC, Stiff A, Markowitz J, Trikha P, Levine KM, et al. Circulating myeloid-derived suppressor cells increase in patients undergoing neo-adjuvant chemotherapy for breast cancer. Cancer Immunol Immunother (2017) 66(11):1437–47. doi: 10.1007/s00262-017-2038-3
157. Li F, Zhao Y, Wei L, Li S, Liu J. Tumor-infiltrating treg, MDSC, and IDO expression associated with outcomes of neoadjuvant chemotherapy of breast cancer. Cancer Biol Ther (2018) 19(8):695–705. doi: 10.1080/15384047.2018.1450116
158. Fallah J, Diaz-Montero CM, Rayman P, Wei W, Finke JH, Kim JS, et al. Myeloid-derived suppressor cells in nonmetastatic urothelial carcinoma of bladder is associated with pathologic complete response and overall survival. Clin Genitourin Cancer (2020) 18(6):500–8. doi: 10.1016/j.clgc.2020.03.004
159. Millrud CR, Mehmeti M, Leandersson K. Docetaxel promotes the generation of anti-tumorigenic human macrophages. Exp Cell Res (2018) 362(2):525–31. doi: 10.1016/j.yexcr.2017.12.018
160. Kodumudi KN, Woan K, Gilvary DL, Sahakian E, Wei S, Djeu JY. A novel chemoimmunomodulating property of docetaxel: suppression of myeloid-derived suppressor cells in tumor bearers. Clin Cancer Res (2010) 16(18):4583–94. doi: 10.1158/1078-0432.CCR-10-0733
161. Tesi RJ. MDSC; the most important cell you have never heard of. Trends Pharmacol Sci (2019) 40(1):4–7. doi: 10.1016/j.tips.2018.10.008
Keywords: dendritic cells, myeloid-derived suppressor cells, vaccines, cancer, immunosuppression
Citation: Sánchez-León ML, Jiménez-Cortegana C, Cabrera G, Vermeulen EM, de la Cruz-Merino L and Sánchez-Margalet V (2022) The effects of dendritic cell-based vaccines in the tumor microenvironment: Impact on myeloid-derived suppressor cells. Front. Immunol. 13:1050484. doi: 10.3389/fimmu.2022.1050484
Received: 22 September 2022; Accepted: 27 October 2022;
Published: 15 November 2022.
Edited by:
Fernando Aranda, Instituto de Investigación Sanitaria de Navarra (IdiSNA), SpainReviewed by:
Oliver Kepp, INSERM U1138 Centre de Recherche des Cordeliers (CRC), FranceCopyright © 2022 Sánchez-León, Jiménez-Cortegana, Cabrera, Vermeulen, de la Cruz-Merino and Sánchez-Margalet. This is an open-access article distributed under the terms of the Creative Commons Attribution License (CC BY). The use, distribution or reproduction in other forums is permitted, provided the original author(s) and the copyright owner(s) are credited and that the original publication in this journal is cited, in accordance with accepted academic practice. No use, distribution or reproduction is permitted which does not comply with these terms.
*Correspondence: Carlos Jiménez-Cortegana, Y2pjb3J0ZWdhbmFAdXMuZXM=
†These authors have contributed equally to this work
Disclaimer: All claims expressed in this article are solely those of the authors and do not necessarily represent those of their affiliated organizations, or those of the publisher, the editors and the reviewers. Any product that may be evaluated in this article or claim that may be made by its manufacturer is not guaranteed or endorsed by the publisher.
Research integrity at Frontiers
Learn more about the work of our research integrity team to safeguard the quality of each article we publish.