- 1Food Safety and Enteric Pathogens Research Unit, National Animal Disease Center, Agricultural Research Service, United States Department of Agriculture, Ames, IA, United States
- 2Immunobiology Graduate Program, Iowa State University, Ames, IA, United States
- 3Department of Veterinary Microbiology and Preventative Medicine, College of Veterinary Medicine, Iowa State University, Ames, IA, United States
Intraepithelial lymphocytes (IELs) include T cells and innate lymphoid cells that are important mediators of intestinal immunity and barrier defense, yet most knowledge of IELs is derived from the study of humans and rodent models. Pigs are an important global food source and promising biomedical model, yet relatively little is known about IELs in the porcine intestine, especially during formative ages of intestinal development. Due to the biological significance of IELs, global importance of pig health, and potential of early life events to influence IELs, we collate current knowledge of porcine IEL functional and phenotypic maturation in the context of the developing intestinal tract and outline areas where further research is needed. Based on available findings, we formulate probable implications of IELs on intestinal and overall health outcomes and highlight key findings in relation to human IELs to emphasize potential applicability of pigs as a biomedical model for intestinal IEL research. Review of current literature suggests the study of porcine intestinal IELs as an exciting research frontier with dual application for betterment of animal and human health.
Introduction
Intestinal intraepithelial lymphocytes (IELs) are “lymphoid cells that reside between the intestinal epithelial cells (IECs) that form the intestinal mucosal barrier” and include subsets of both intraepithelial T lymphocytes (T-IELs) and intraepithelial innate lymphoid cells [ILC-IELs (1);]. Close physical proximity to the intestinal lumen lends IELs the unique task of serving as first line immune mediators, requiring balance between immune defense versus tolerance towards enteric microbes and dietary substances. IELs are most well-studied in humans and rodent models, where roles in wound healing (2, 3), tissue homeostasis (3–5), epithelial surveillance (6, 7), epithelial integrity (7, 8), epithelial cell shedding (9), nutrient sensing (10), immune regulation (3, 11), pathogen defense (7, 12, 13), intestinal inflammation (14–16), and immunopathology (17–20) have been demonstrated and reviewed extensively (1, 21–29). IELs are also identified in veterinary species, including in porcine (referenced throughout), bovine (30–32), ovine (33), caprine (34), camelid (35), equine (36, 37), and avian (38–40) intestine. However, the biological significance of IELs across different species is not fully delineated, as veterinary research pertaining to IELs trails far behind that performed in humans and rodents.
Pork is the most highly consumed animal protein in the world (41), and keeping pigs healthy is crucial for global food security. Unlike humans, pigs are born without maternally-acquired passive immunity due to placental differences (42). Instead, piglets must ingest colostrum to acquire most maternally-derived immune factors. Piglet rearing environment can also be an especially formative influence for immune education and immune system development in early life (43–45) and is very different from humans. Conventional production pigs in developed countries are weaned at a relatively early age while intestinal immune development is still ongoing (46), predisposing pigs to adverse early-life events that may be detrimental to gut health and might shape the long-term intestinal immune landscape (47, 48). Hence, understanding how intestinal immune components, including IELs, are shaped by early life events is crucial for identifying processes promoting intestinal health over dysregulation. In addition, delineating trajectories of how intestinal development and cellular maturation might be altered through targeted intervention strategies is a cutting-edge approach for promoting a healthy gut (discussed in subsequent sections). In particular, immunomodulation of IELs at the intestine-lumen interface is a potential targeted strategy to maximize intestinal health without the use of antibiotics. All in all, the biological significance of IELs, global importance of pig health, and potential of early life events to influence how IELs impact intestinal and overall pig health point to a necessity for better biological understandings of IELs in the developing porcine intestinal tract.
This review reiterates current knowledge of porcine IELs, pertaining to dynamics of functional and phenotypic maturation in the context of the developing pig gut. Based on available findings, we formulate probable implications of IELs on intestinal and overall health outcomes as the intestinal immune system develops in the growing pig. We also highlight key findings in relation to human IELs, emphasizing the advantages and potential drawbacks of pigs as biomedical models for intestinal IEL research. In total, findings present porcine intestinal IELs as an exciting research frontier with application to both pig and human health.
IEL identification in pig intestinal tissues
While the root definition of IELs is based on location within the epithelium (situated between the epithelial basement membrane and the luminal surface), IEL distribution throughout the intestinal tract and/or within different regions of the epithelium can provide further insight into cellular function and biological importance. Thus, it’s integral we are able to identify IELs within their native tissue structures through histological examination of IELs with various in situ staining techniques.
Hematoxylin and eosin (H&E) staining identifies IELs
Lymphocytes subjected to H&E staining are customarily small cells (~7-um diameter) mainly composed of large, dark, rounded nuclei (stained with hematoxylin to identify acidic components in purple) and minimal cytoplasm [stained with eosin to identify basic components in pink (49)]. Therefore, IELs can be distinguished from IECs based on nuclear and cytoplasmic H&E staining properties [Figure 1 (50)]. However, a major drawback of IEL identification via H&E staining is the inability to further differentiate IEL subsets (e.g. T-IELs, ILC-IELs). As demonstrated in humans, IEL quantification via immunohistochemistry (IHC) has increased sensitivity compared to H&E quantification, even when staining for protein markers that only recognize subsets of IELs (51, 52). Thus, H&E identification of IELs in pigs could also potentially have decreased sensitivity compared to additional in situ staining methods discussed below.
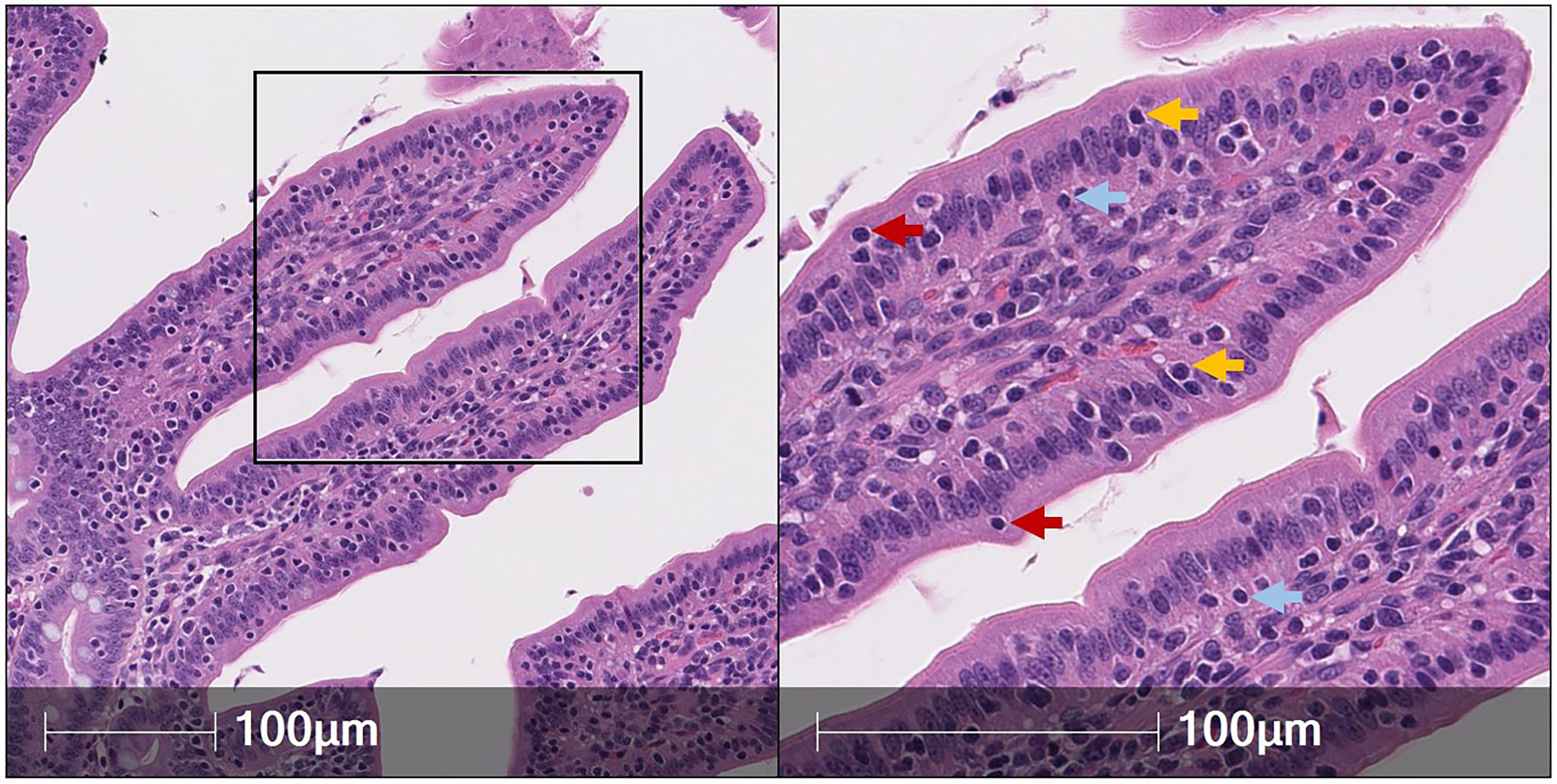
Figure 1 Identification of IELs from H&E staining in pig intestine. H&E-stained tissue section of jejunum from a ~9-week-old pig. IELs are identified as dark purple, round nuclei within the epithelial layer. Two each of IELs in apical epithelium (red arrows), nuclear-level epithelium (gold arrows), and basement membrane (light blue arrows) are indicated in the panel on the right. H&E (hematoxylin and eosin); IEL (intraepithelial lymphocyte).
Identifying IELs via immunohistochemical detection of CD45, CD3ε, and CD2 proteins
Porcine IELs can be identified by IHC using antibodies to label cluster of differentiation (CD) proteins expressed on the cell surface of lymphocytes within the epithelium. In pigs, a definitive pan-IEL protein marker has not been established, though the majority of porcine IELs appear to express CD45, CD3ε, and CD2 proteins when labeled in situ (as described below).
CD45 is a phosphatase that regulates cellular signaling/activation [reviewed in (53, 54)] and is considered a pan-leukocyte marker expressed by >95% of circulating lymphocytes in pigs (55, 56). Though the percentage of CD45+ intestinal IELs in pigs has not been quantified to our knowledge, most IELs are likely CD45+ (similar to circulating lymphocytes). However, non-lymphocytes in the epithelial layer, such as eosinophils and dendritic cells (DCs), likely also express CD45 (55, 56), limiting the ability of CD45 to serve as a marker for only lymphocytes. We’ve established CD45 as an unsuitable pan-innate lymphoid cell (ILC) marker in the pig intestine, as PTPRC (gene encoding CD45) has decreased expression in some intestinal ILC populations, and not all intestinal ILCs express CD45 protein (57).
CD3ε is an accessory molecule to the T cell receptor (TCR) and is considered a pan-T cell marker in pigs (55, 58), unlike additional TCR accessory molecules, CD3δ, CD3γ, and CD3ζ, that are detected in both porcine T cells and ILCs at the transcriptional level (57, 59). On the other hand, CD2 is a cell adhesion molecule and co-stimulatory receptor that is expressed by both T cells and ILCs in pigs, humans, and mice (55, 58–61). Therefore, CD3ε can be used to detect T-IELs but not ILC-IELs in pigs, while CD2 might serve as a reliable marker to identify both T-IELs and ILC-IELs in the intestinal epithelium. However, a small subset of γδTCR T cells in the porcine intestinal tract are CD2- (62), leaving the potential of some intestinal T-IELs being CD2- in pigs. Identification of CD2- γδTCR T cells in the porcine intestine by staining with antibody clone MAC320 [reactive to swine workshop cluster 6 (SWC6) protein expressed by porcine CD2- γδTCR T cells (63, 64); to-date, SWC6 protein has not been fully characterized] demonstrated very few SWC6+ cells (inferred to be CD2- γδTCR T cells) present, and none were detected in the epithelium (65). In addition, there are very few CD2- γδTCR T cells in small or large intestinal epithelium (66, 67), confirming CD2- γδTCR T-IEL absence or rarity throughout the porcine intestine. Therefore, CD2 still remains a potential marker for identifying the majority of IELs in pigs.
Previous in situ work found most but not all CD2 staining colocalizes with CD3ε staining (68). Moreover, recent work identified CD2+CD3ε- cells in porcine ileum (57), suggesting the presence of CD2+CD3ε- ILC-IELs. Collectively, CD3ε appears to be a reliable marker for detecting T-IELs, while CD2 remains a more reliable in situ marker to encompass T-IEL and ILC-IEL detection at the protein level, though additional biological contexts (e.g. age, disease state) warrant further exploration for more comprehensive validation.
Utilizing RNA in situ hybridization (RNA-ISH) to further delineate IEL populations
mRNA-reactive reagents can more easily be synthesized and optimized for in situ detection than can antibodies reactive to targeted proteins (69). Given the paucity of porcine-specific immunoreagents, RNA-ISH has become an important tool for identifying and characterizing porcine cell types. Recent work documents discovery of ILC-IELs in the porcine ileum based on dual labeling for ITGAE mRNA [encoding the integrin α subunit of CD103 (aka αEβ7), considered a pan-marker of IELs and tissue residency in humans and mice (70–72)] and CD3ε protein [a porcine T cell marker (55, 58)]. Based on dual ITGAE/CD3ε staining, ILC-IELs are identified as ITGAE+CD3ε- cells, while T-IELs are identified as ITGAE+CD3ε+ cells (57). Based on transcriptional properties, ILC-IELs are further classified as group 1 ILCs, which includes both ILC1s and natural killer (NK) cells. However, group 1 ILCs in the porcine intestine differ transcriptionally from NK cells, which are infrequent in the porcine intestinal tract (57), thus suggesting a non-NK cell identity for porcine intestinal ILC-IELs. Since ITGAE is expressed by both CD3ε+ and CD3ε- cells within the epithelial layer of porcine ileum, ITGAE might be a pan-IEL marker for both T cells and ILCs in pigs. ITGAE+CD3ε+ vastly outnumber ITGAE+CD3ε- IELs in the ileal epithelium of 7.5-week-old pigs (57), indicating T-IELs (ITGAE+CD3ε+) are more abundant than ILC-IELs (ITGAE+CD3ε-) in pigs at the only age and intestinal location thus far analyzed. Findings are similar to humans, where >90% of intestinal IELs are T-IELs (1), but further probing into additional ages, intestinal locations, and biological contexts in pigs remains to be studied.
CD69, a marker associated with a tissue resident phenotype within the epithelial layer, is used to identify IELs in other species (1). Our lab investigated the potential use of CD69 transcript as another IEL marker in pigs but determined, unlike ITGAE, CD69 is not largely expressed by cells in the epithelial layer of the porcine intestinal tract (Figures 2A,B). Additional markers with known expression by IELs in other species include NCR1 [encoding NKp46 (73)], KLRB1 [encoding CD161 (74)], and CCR9 (75), all of which did not appear to be appropriate pan-IEL markers in pigs due to low/sporadic expression in the epithelium (Figures 2C–E). RNA-ISH detection of cytokine-encoding genes IFNG, IL10, and TGFB1 were also tested, but positive staining was sporadic or non-specific to IELs (Figures 2F–H). Of mRNA targets evaluated thus far via RNA-ISH, only ITGAE presents as a promising pan-IEL gene marker to capture both T-IELs and ILC-IELs that likely encompass the total IEL community in the intestinal epithelium of pigs. However, CD103+ DCs in the epithelial layer (intraepithelial DCs; not reported in pigs thus far) would likely express ITGAE as well. Therefore, further methods to differentiate between ITGAE+ lymphocytes and non-lymphocytes may be beneficial for more accurate identification of porcine IELs at the mRNA level, but reliable in situ markers for many non-lymphocyte immune cells also remain to be developed in pigs.
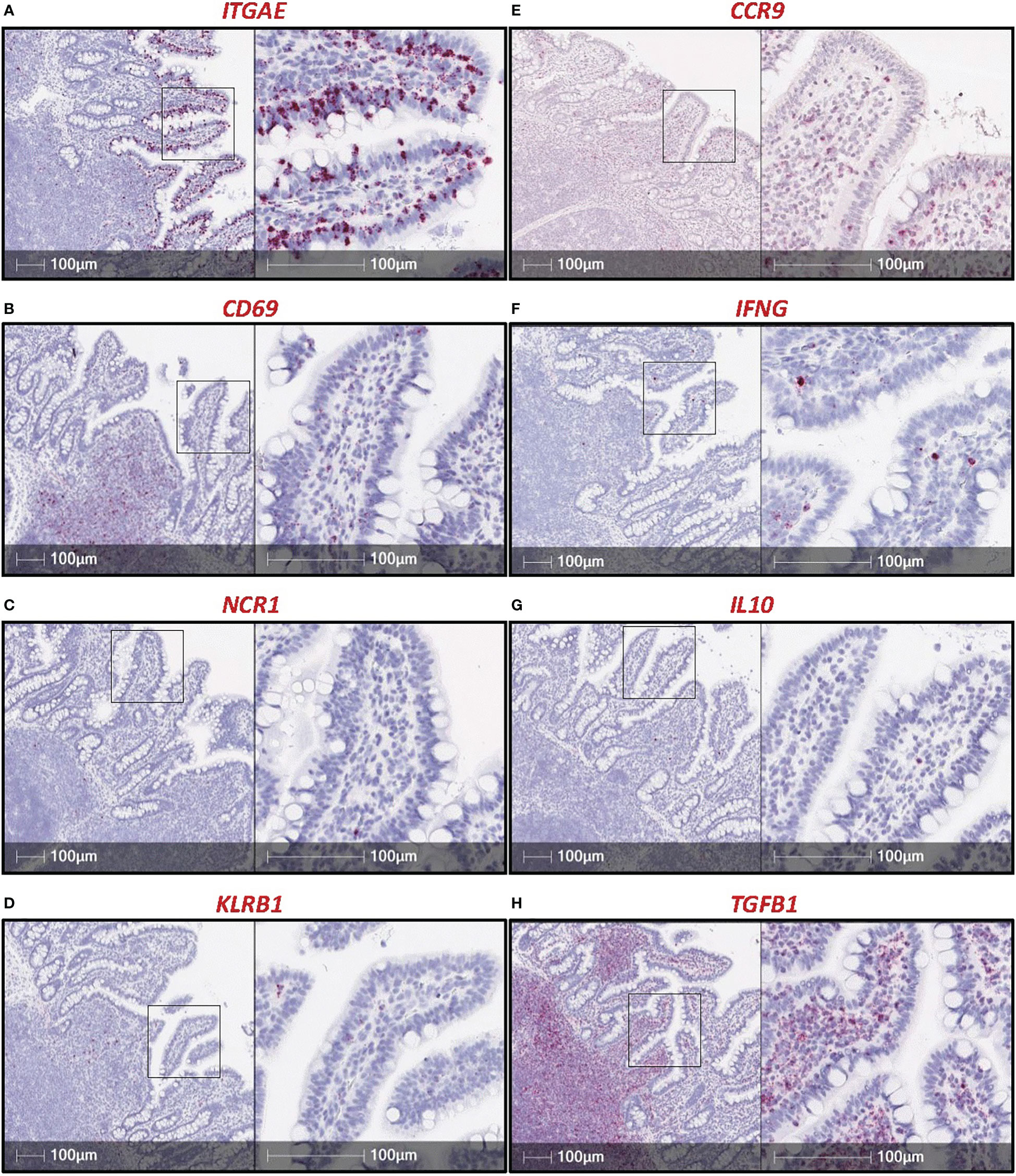
Figure 2 RNA-ISH staining of potential IEL markers in porcine ileum. Sections of ileum from a ~7.5-week-old pig stained for mRNA transcripts (red staining): ITGAE (A), CD69 (B), NCR1 (C), CD161 (D), CCR9 (E), IFNG (F), IL10 (G), TGFB1 (H). Probes were custom-designed from Advanced Cell Diagnostics with the following catalog numbers: ITGAE (#590611); CD69 (#590601); NCR1 (#553131); KLRB1 (#490871, product labeled CD161); CCR9 (#1062631); IFNG (#490821); IL10 (#442791); TGFB1 (#444951). A protocol for RNA-ISH staining is described previously (57). RNA-ISH (RNA in situ hybridization).
In situ identification of T-IEL subsets
Porcine T cells are further broken down into subsets of CD4 αβTCR T cells, CD8 αβTCR T cells, and γδTCR T cells based on expression of different cell surface molecules. Consequently, it has been attempted to differentiate between CD4 αβTCR, CD8 αβTCR, and γδTCR T-IEL subsets through in situ staining for CD4 and CD8α proteins. CD4+ cells are found in the intestinal lamina propria and surrounding Peyer’s patches but are completely absent or extremely rare in the intestinal epithelium of pigs (76–80), indicating CD4 αβTCR T cells are not major contributors to the IEL community in the pig intestine. CD8α+ cells are localized preferentially to the epithelium (76, 78–81), but porcine αβTCR T , γδTCR T, and NK cells can all express CD8α (58). While NK cells (CD3ε-CD8α+) are not highly prevalent in the porcine intestinal tract, both γδTCR and CD8 αβTCR T cells occur in the intestine of pigs at regular frequencies (62), and most γδTCR T-IELs are CD8α+ (66, 67). Thus, CD8α+ IELs mostly includes both γδTCR and αβTCR lineage T cells. A protocol for in situ detection of γδTCR-associated protein is required to detect γδTCR T-IELs but has not been established in pigs to our knowledge, and thus porcine γδTCR T cells have not been exclusively identified in situ at the protein level. Previously documented attempts for in situ detection of porcine γδTCR T cells included staining for SWC6 protein to detect CD2- γδTCR T cells (65) and use of an anti-mouse γδ antibody with unverified reactivity in pigs (82).
To overcome issues of αβTCR versus γδTCR T cell differentiation in pigs, a dual staining method was established (combining IHC and RNA-ISH), where IELs expressing TRDC (encoding the δ constant chain of the γδTCR) are identified as γδTCR T cells, and TRDC- IELs expressing CD3ε protein (TRDC-CD3ε+) are inferred as αβTCR T cells (Figure 3). Using this method, γδTCR T cells were identified in both the small and large intestine and were mainly concentrated to the epithelium, indicating most intestinal γδTCR T cells are intraepithelial in pigs (66). Moreover, RNA-ISH staining for CD4 and CD8B transcripts to detect CD4 αβTCR and CD8 αβTCR T cells, respectively, further supports in situ protein staining, showing CD4 is not expressed in the epithelium, while CD8B staining is present in the epithelium (57). Unlike the protein marker CD8α, the RNA marker CD8B is exclusive to CD8 αβTCR T cells, as CD8B is not expressed in transcriptomes of ILCs (including NK cells), CD4 αβTCR T cells, or γδTCR T cells in pigs (57, 59). Collectively, IHC and RNA-ISH staining support ILCs, γδ TCR T cells, and CD8 αβTCR T cells preferentially located in the intestinal epithelium of pigs, while CD4 αβTCR T cells are sub-epithelial.
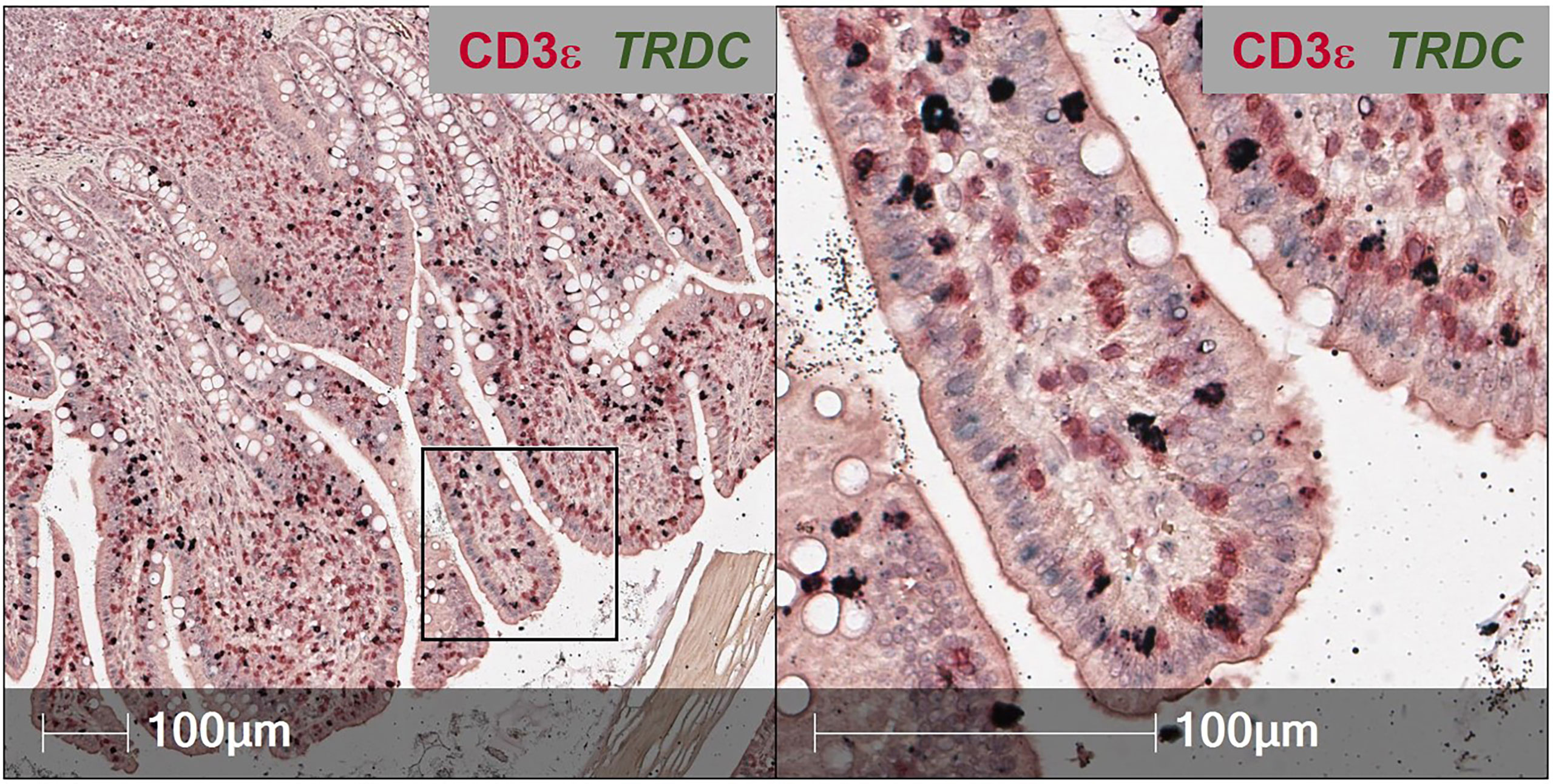
Figure 3 Dual in situ detection of αβ and γδ T cells in pig intestine. Section of ileum from a ~6-week-old pig stained for CD3ε protein (red) and TRDC transcript (dark green). TRDC+ cells (dark green) are identified as γδ T cells, while TRDC-CD3e+ cells (red) are identified as αβ T cells. The staining protocol was further optimized from previous work (57, 66) and is available at dx.doi.org/10.17504/protocols.io.q26g7yro1gwz/v1.
Abundance and distribution of IELs throughout the intestine
To understand how IEL communities mature as the porcine intestinal tract develops, a baseline for IEL distribution within the intestinal tract of aging, healthy, conventional pigs must be established. Newborn pigs have very few IELs in small intestinal villi (65, 77, 79, 80, 83). At birth, only 38% of CD2+ cells are intraepithelial but increase to >50% in jejunum and >60% in ileum by seven weeks of age (77). In jejunum and ileum, total IEL numbers increase from ~2 cells per 100 enterocytes near birth to between 20 and 30 cells by nine weeks of age (65). Results thus indicate preferential localization of CD2+ cells in epithelium over lamina propria and expansion and/or recruitment of the intestinal IEL compartment with age.
IEL distributions within conventional, age-matched pigs differ by regional locality across proximal versus distal intestinal locations. In the large intestine, between 3.5-4 times as many CD3ε+ cells are located within the epithelium compared to lamina propria (84), while in the small intestine, approximately equal numbers of CD2+ lymphocytes are distributed between the epithelium and lamina propria (76, 77, 79). Though measurements of CD2+ and CD3ε+ cells are not necessarily equivalent, comparison suggests more preferential localization of lymphocytes to the epithelium of the large compared to small intestine. However, overall IEL abundance is greater in the porcine small than large intestine (66, 67, 85–90).
Though the largest differences in IEL distributions occur when comparing small versus large intestinal locations, comparisons between locations within small intestine or within large intestine also reveal important distinctions. However, study of porcine IELs has been largely focused to the small intestine, with very few comparisons of IEL distributions between large intestinal locations. In the large intestine, IELs are more abundant in cecum than in colon [per 100 colonocytes or percentage surface area (66, 91)]. In small intestine, IEL abundance across proximal and distal locations differ across studies; however, quantification of IELs are made with different methods that yield varying results where intestinal morphology need be considered. Quantification of total CD2+ intraepithelial cells per villus revealed greater IEL numbers in proximal over distal small intestine (76). Quantification of total CD3ε+ intraepithelial cells per villus revealed greater IEL numbers in jejunum over duodenum and ileum (87). Conversely, quantification of total IELs per 100 enterocytes revealed approximately equal or higher numbers in distal compared to proximal small intestine (65, 88, 90, 92–95), as did quantifying the number of CD2+ intraepithelial cells per a set number of microscopic fields (79) or the percentage of CD3ε+-stained intraepithelial surface area per villi (66). Coupled with knowledge that epithelial area per villus is greater in the proximal versus distal small intestine (96), results suggest more IELs are present in each of the larger villi of the proximal small intestine, such as the jejunum, but IELs in the distal small intestine, such as the ileum, are more concentrated within smaller villi. Additional biological and/or technical factors may also contribute to the variable findings across studies and might be indicative of functional roles across the intestinal tract yet to be understood.
IEL distributions also vary by locality within crypts and villi and in relation to luminal proximity, including location in the basement membrane, enterocyte nuclear level, or apical epithelium (Figure 1). In porcine small intestine, IELs preferentially congregate to intestinal villi over crypts at up to a four-to-one ratio (65–67, 77, 78, 83, 97, 98). Preferential accumulation of IELs in villi suggests IEL effector functions may involve close interactions with signals derived from the intestinal lumen, as villi are more intimately associated with the lumen compared to shielded niches of crypts. Similarly, reduced numbers of IELs in large compared to small intestine may be due to lack of luminal signals occurring in large intestinal crypts, supported by preferential congregation of large intestinal IELs to the upper third of the crypt that is in closest proximity to the lumen (84). Reduced numbers of IELs in the large intestine might also be attributed to differences in mucosal barriers, such as a double mucus layer providing enhanced separation between lumen and epithelium in the large intestine compared to only a single mucus layer in the small intestine (99, 100). IELs in small intestinal villi also differ in distribution across the epithelial layer in relation to luminal proximity. While the majority of IELs are located near the basement membrane, some IELs are in closer proximity to the lumen, including at the enterocyte nuclear level, apically, or even freely within the lumen (65, 67, 78, 79, 87, 97, 101). However, IEL detection within the free lumen must be cautiously interpreted, as technical variables of tissue processing may compromise tissue integrity. IELs in closer proximity to the lumen vary in phenotypes and potential effector functions compared to those located at the basement membrane, as is discussed in later sections of this review.
IEL isolation for ex vivo phenotyping via flow cytometry
Further resolution of IELs into inferred functional subsets can be performed through flow cytometry, where a larger selection of antibodies is available for simultaneous cell labeling compared to in situ detection methods. Cell staining for flow cytometry requires cells to be dissociated from tissue into a single-cell suspension. Isolation of IELs relies on precise and accurate removal of only cells from the epithelial layer of intestinal tissues, so as not to obtain contaminating sub-epithelial cells that can confound biological interpretations. Epithelial cell removal is performed by incubating tissues in solution containing the chelating agent, ethylenediamine tetraacetic acid (EDTA), which dissociates epithelial cells from tissue, though protocols need to be catered to particular intestinal samples in different biological contexts. For example, in jejunum and ileum of 5-day-old pigs, incubation in EDTA solution for two sequential one hour incubations resulted in near complete epithelial removal from both crypts and villi, plus dissociation of a sizeable portion of non-epithelial cells (83). The same incubations performed on jejunum and ileum of 14-month-old pigs resulted in incomplete removal of epithelial crypt cells but less sub-epithelial contamination (83). Thus, biological context of intestinal samples influences which epithelial regions are removed and the degree of sub-epithelial contamination. Technical parameters must also be considered for efficient epithelial isolation. For example, duration of incubation in EDTA solution impacts epithelial recovery and purity. As seen in Figure 4, extended EDTA incubation times result in greater epithelial liberation but at the expense of increased potential for sub-epithelial contamination and alterations to cellular profiles associated with isolation procedures, including increased cell activation or death. Additional factors including epithelial integrity, epithelial surface area, tissue handling, tissue size, reagent volume, incubation temperatures, use of freshly prepared solutions, and EDTA concentrations must also be considered and optimized, as these variables can affect the duration of EDTA incubation required for efficient epithelial removal.
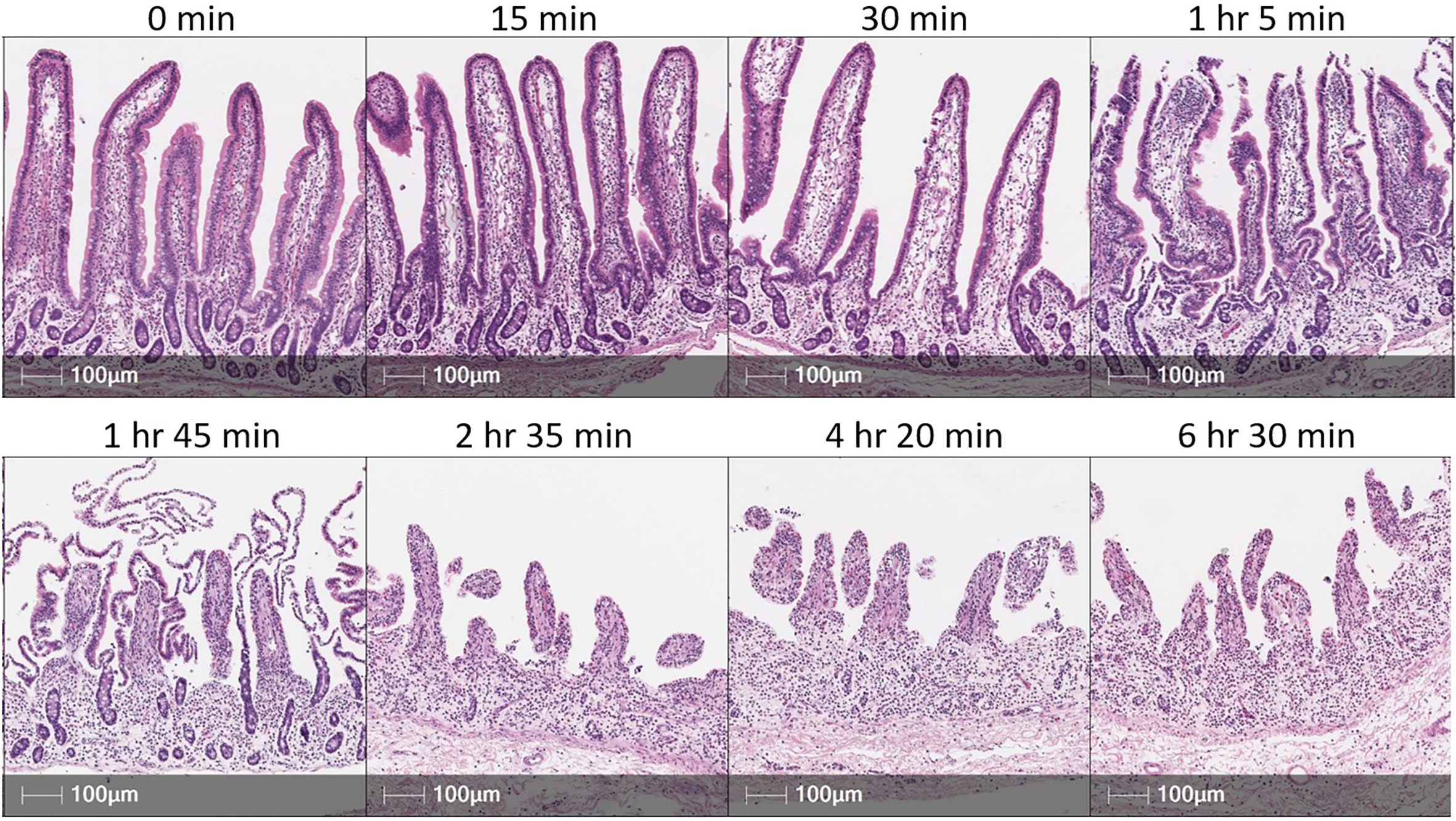
Figure 4 EDTA incubation time influences epithelial cell removal in porcine intestinal tissues. H&E sections of porcine small intestinal tissue subjected to incubation in EDTA solution for various amounts of time. Tissue was derived from terminal ileum of a ~3-month-old pig. After dissociating mucus from tissue [described elsewhere (66)], ~2 grams of tissue was placed into 200 mL of epithelial removal solution (5mM EDTA and 2% FCS in HBSS) and incubated at 37°C, 200 rpm for amounts of time specified above each image panel. Tissues were not transferred into fresh EDTA solution throughout the duration of specified incubation time. Cell recovery did not substantially increase from 2 hr 35 min to either 4 hr 20 min or 6 hr 30 min incubations. This figure is not intended as a reference for an EDTA incubation time for optimal epithelial removal but to emphasize that individual optimization of methods for epithelial cell removal will be necessary based on technical and biological contexts of an experiment. EDTA (ethylenediamine tetraacetic acid); FCS (fetal calf serum); H&E (hematoxylin and eosin); HBSS (Hank’s balanced salt solution); hr (hour); min (minute); mL (milliliters); mM (millimolar); rpm (rotations per minute).
Since porcine ILC-IELs are only recently characterized via flow cytometry, most in-depth ex vivo phenotyping of porcine intestinal IELs pertains to T cell subsets, which we focus on for this review. While ILCs were identified as CD2+CD3ε-CD79α-CD172α- cells in porcine intestine via flow cytometry (57), porcine T-IELs are more simply identified as CD3ε+ lymphocytes, and detection of additional phenotypic markers is implemented for in-depth phenotyping. Though a small fraction of CD4+ T cells are detected via flow cytometry in preparations of porcine IELs, in situ staining mentioned previously has validated CD4+ T cells are contaminating sub-epithelial cells and are thus not discussed further.
IEL subsets: Phenotypes, inferred functions, and proportional distributions
Porcine IELs include major subsets of CD8 αβTCR T-IELs (further broken down into CD8αα+ and CD8αβ+ populations), γδ TCR T-IELs, and ILC-IELs. A summarization of major porcine intestinal IEL subsets and findings discussed in subsequent subsections is available in Table 1.
CD8 αβTCR T-IELs
In pigs, CD8 αβTCR T-IELs are labeled as CD3ε+γδTCR-CD4- lymphocytes expressing CD8α and/or CD8β (67). In rodents, two subsets of CD8 αβTCR T-IELs exist: (1) ‘induced’ T-IELs expressing the traditional heterodimeric CD8αβ co-receptor and (2) ‘natural’ T-IELs expressing CD8αα homodimers. Induced CD8αβ+ αβTCR T-IELs are recruited to the intestine in an antigen-dependent manner by TCR-mediated antigen recognition (109, 110). Conversely, CD8αα+ αβTCR T-IELs occur naturally, migrating directly to the intestine from the thymus without TCR-mediated recruitment (102–104), and CD8αα acts as an inhibitory receptor rather than an activating TCR co-receptor (1, 105–108). In the small intestine of pigs, the majority of T-IELs are CD8 αβTCR T cells (γδTCR-CD4-CD8α+) and proportionally increase with age, whereas in the large intestine, only about half of T-IELs are CD8 αβTCR T cells (66). However, whether γδTCR-CD4-CD8α+ T-IELs expressed CD8β was not determined. We have since identified both CD8β+ and CD8β- populations within γδTCR-CD4-CD8α+ T-IELs, indicating the presence of both innate-like CD8αα+ αβTCR T-IELs and traditional CD8αβ+ αβTCR T-IELs in pigs (67). Though the majority of porcine CD8α+ αβTCR T-IELs also express CD8β throughout the intestinal tract, CD8αα+ αβTCR T-IELs are found at lower frequencies (67). CD8αα+ αβTCR T-IELs have the highest proportional abundance in the large intestine (2-15% of T-IELs in cecum), are rare in the jejunum, and have intermediate proportions in the ileum (67). Moreover, porcine CD8αα+ αβTCR T-IELs have increased expression of CD16 [Fcγ receptor able to activate T cells independent of TCR engagement (114, 115)] and lower expression of CD27 [co-stimulatory receptor downregulated on effector and memory T cells (116, 117) and used as a marker of effector/memory T cells in pigs (118–124)] compared to porcine CD8αβ+ αβTCR T-IELs (67). Based on the limited phenotyping that has been performed, we speculate an innate-like, effector role for porcine CD8αα+ αβTCR T-IELs. CD8αα+ αβTCR T-IELs in pigs might be similar to natural as opposed to induced T-IELs described in other species, though further research is warranted to confirm or disprove. In further support of CD8αα+ αβTCR T-IELs being naturally-occurring T-IELs, others reported in review that CD4-CD8α+CD8β- αβTCR T cells are some of the earliest T-IELs present in the porcine intestine, before antigen has largely been encountered (125), though we were unable to identify the original research these conclusions are derived from.
Though CD8αβ+ αβTCR T cells are traditionally considered conventional T cells that rely on TCR-mediated activation, we speculate functional properties of CD8αβ+ αβTCR T-IELs is influenced by intestinal location of cells being assessed. Increased proportions of CD8αβ+ αβTCR T-IELs express CD16 in the cecum compared to jejunum and ileum, albeit at lower levels than that of cecal CD8αα+ αβTCR T-IELs [15-55% of cecal CD8αβ+ αβTCR T-IELs versus 35-80% of cecal CD8αα+ αβTCR T-IELs (67)]. CD16 expression on a subset of large intestinal CD8αβ+ αβTCR T-IELs therefore suggests at least some CD8αβ+ αβTCR T-IELs might also have innate-like functions. However, no functional studies of CD8αβ+ αβTCR T-IELs have yet been conducted in the porcine large intestine to our knowledge.
CD8αβ+ αβTCR T-IELs from porcine small intestine have been isolated as CD8β+ cells and studied at a functional level in vitro. NKG2D is an NK activating receptor that recognizes major histocompatibility complex (MHC) class I-related molecules (MICs) expressed by stressed epithelial cells. Activation of NKG2D initiates TCR-independent cell activation (126) or costimulation to amplify TCR-mediated T cell activation and augment cellular activation and cytotoxicity (127–129).While small intestinal CD8β+ IELs in pigs have only moderate expression of KLRK1 (encoding NKG2D), KLRK1 expression is increased more than two-fold with anti-CD3ε stimulation (111, 112). Thus, small intestinal CD8αβ+ αβTCR T-IELs are activated in an adaptive, TCR-dependent manner (via anti-CD3ε stimulation). Moreover, anti-CD3ε stimulation of peripheral CD8β+ T cells and γδTCR+ IELs (γδ T-IELs; from similar small intestinal locations as CD8β+ IELs) did not result in increased KLRK1 expression (112), indicating TCR-dependent NKG2D upregulation is not generalized across anatomical locations or T cell lineages. Therefore, CD8αβ+ αβTCR T-IELs mirror induced IELs in their patterns of TCR-mediated activation but also had different activation dynamics compared to peripheral CD8β+ T cells. The use of single-cell RNA sequencing (scRNA-seq) to compare CD8αβ+ αβTCR T cells from porcine ileum and blood further supports the notion of functional distinctions, as intestinal CD8αβ+ αβTCR T cells are transcriptionally distinct from cells in the blood (57).
γδTCR T-IELs
Due to previous inabilities for in situ detection of γδTCR+ T cells described earlier, knowledge of γδTCR T-IEL distributions is derived from flow cytometry data that reports only proportional rather than absolute cell abundances. The proportion of γδTCR+ within total T-IELs generally increases from proximal to distal small intestine and from small to large intestine, with similar proportions in both the cecum and colon of the large intestine (66). Similar to γδTCR T cells in circulation (58, 124, 130–133), there are decreased proportions of small intestinal γδTCR T-IELs as pigs age (66), presumably due to recruitment/expansion primarily of CD8αβ+ αβTCR T-IELs that mirror descriptions of induced T-IELs in humans and mice (109, 110). Thus, though it is unknown whether absolute numbers of γδTCR T-IELs are altered with age (due to lack of in situ quantification), proportions of γδTCR T-IELs decrease with age at the expense of increasing proportions of other T-IELs.
Limited functional studies of intestinal γδTCR T-IELs in pigs indicate innate-like roles. γδTCR+ IELs have higher expression of KLRK1 (encoding NKG2D, functionally described in previous section) than do CD8β+ IELs, indicating their enhanced ability to mediate epithelial stress signals by recognizing MICs on IECs (111, 112). Moreover, more porcine γδTCR T-IELs express CD16 than do CD8β+ IELs (67). In humans, CD16 on γδTCR T cells binds to opsonizing antibodies to facilitate phagocytosis, followed by presentation of ingested antigen on MHC-II molecules (134–136). It’s plausible that porcine γδTCR T-IELs might have similar capacities for CD16-mediated phagocytosis and antigen presentation, as circulating γδTCR T cells can act as antigen presenting cells in pigs (137). Most CD16+ γδTCR T-IELs are also MHC-II+ in the porcine jejunum (Figures 5A, B), supporting the idea of dual ability to phagocytose and present antigens. Compared to CD8β+ IELs, porcine γδTCR+ IELs are more susceptible to Salmonella infection in vitro, indicating γδTCR T-IELs as a potential target for pathogenic infection (138), perhaps related to phagocytic abilities of γδTCR T-IELs.
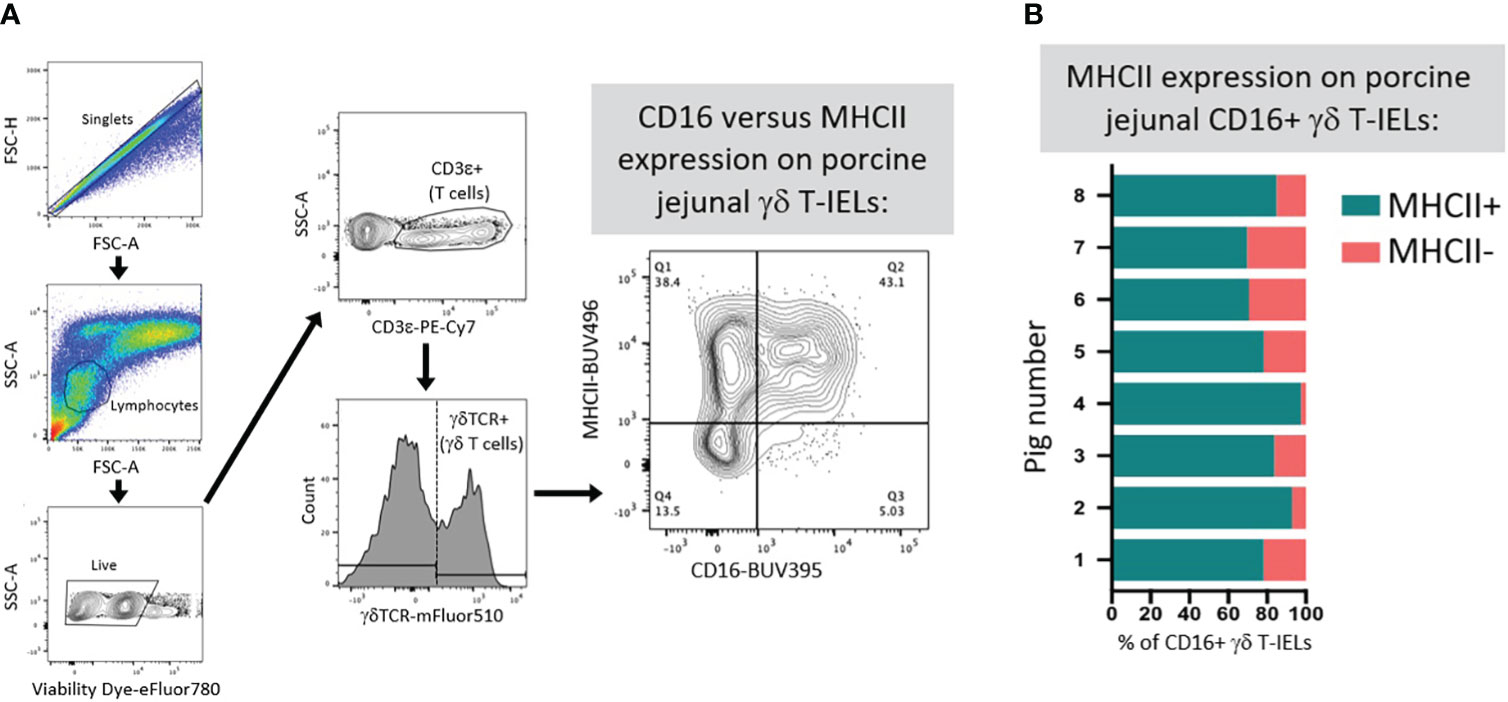
Figure 5 Co-expression of CD16 and MHC-II on γδ T-IELs in porcine jejunum. (A) Representative flow cytometry gating showing the expression of CD16 and MHC-II on gated γδ T-IELs in epithelial-enriched cell fractions from jejunum of a 6-week-old pig. (B) Stacked bar plot of the percentage of CD16+ γδ T-IELs that are MHC-II+ (turquoise) versus MHC-II- (salmon). Data are derived from jejunum of eight 6-week-old pigs. Epithelial-enriched cell fractions were isolated from jejunum and stained for flow cytometry as previously described (67). Detection reagents used for flow cytometry staining are as follows: Fixable Viability Dye eFluor780 (ThermoFisher Scientific 65-0865-14); anti-CD3ε-PE-Cy7 (clone BB23-8E6-8C8; BD 561477); anti-γδTCR (clone PGBL22A; Washington State University PG2032; conjugated to mFluor510 fluorophore by Caprico Biotechnologies); anti-CD16 (clone G7; BioRad MCA1971GA) detected with anti-mouse IgG1 (clone A85-1; BD 740234); MHC-II (clone 2E9/13; BioRad MCA2314GA) detected with anti-mouse IgG2b-BUV496 (clone R12-3; BD 750517). FSC-A (forward-scatter area); FSC-H (forward-scatter height); Ig (immunoglobulin); MHC (major histocompatibility complex); SSC-A (side-scatter area); T-IEL (intraepithelial T lymphocyte); TCR (T cell receptor).
CD8α expression in the absence of CD8β (presumably expressed as CD8αα homodimers) is considered a marker of differentiation for CD4 αβTCR T cells in pigs (121, 139–145) but may also serve as a marker to differentiate porcine γδTCR T-IEL populations and functions. Most γδTCR T-IELs are CD2+CD8α+ (presumably expressed as CD8αα homodimers), with a smaller proportion of CD2+CD8α- γδTCR T-IELs primarily located in the proximal small intestine (66, 67). Fewer porcine CD2+CD8α- γδTCR T-IELs express CD27 compared to CD2+CD8α+ γδTCR T-IELs, indicating a potential difference in effector capacity between the two γδTCR T-IEL subsets (66, 67). Thus, CD8α expression by CD2+ γδTCR T-IELs likely correlates with altered functional capacities, though these functional differences are incompletely understood. Comparison of porcine CD2+CD8α- and CD2+CD8α+ γδTCR T cells completed outside of the context of the intestine supports CD8α as a marker gained upon activation/differentiation (120, 123, 124, 132, 137, 146–151). However, we are cautious to apply such findings to γδTCR T-IELs, as IELs represent a unique compartment of immune cells that may have different functional dynamics. In other work directly applied to γδTCR T-IELs in porcine ileum, CD2+CD8α+ γδTCR T-IELs were proposed as anti-inflammatory and CD2+CD8α- γδTCR T-IELs as pro-inflammatory mediators, albeit findings result from studies in gnotobiotic pigs infected with human rotavirus (113). Interestingly, findings draw parallels to documented inhibitory functions of CD8αα homodimers expressed by IELs of other species (1, 105–108). Wen et al. (113) describe higher frequencies of Foxp3+ cells within CD2+CD8α+ γδTCR T-IELs in comparison to both CD2+CD8α- γδTCR T-IELs and circulating CD2+CD8α+ γδTCR T cells, indicating enrichment of regulatory T cells within the CD2+CD8α+ γδTCR T-IEL compartment, though percentages were consistently low (<0.4%). When cultured, CD2+CD8α+ γδTCR T-IELs had greater spontaneous expression of IL10 mRNA. Following stimulation with the phosphoantigen, isopentenyl pyrophosphate, in combination with IL-2, greater expression of IL10 was induced in CD2+CD8α+ γδTCR T-IELs, and greater expression of IFNG was induced in CD2+CD8α- γδTCR T-IELs. Thus, analysis of IL10 and IFNG expression indicated propensity of anti-inflammatory cytokine production in CD2+CD8α+ γδTCR T-IELs and pro-inflammatory cytokine production in CD2+CD8α- γδTCR T-IELs (113). Therefore, results bring forward the question of whether CD8α expression might be associated with pro- versus anti-inflammatory bias in γδTCR T-IELs, but again, whether results are applicable to conventional pigs remains unknown. As CD2+CD8α- γδTCR T-IELs with inferred pro-inflammatory capacity are preferentially located in the proximal small intestine and have decreased expression of CD27 in conventional pigs (66, 67), we speculate γδ T-IEL responses are compartmentalized by intestinal region.
ILC-IELs
Current knowledge of intestinal ILC-IELs in pigs is severely limited due to their very recent discovery (57). However, study of ILC-IELs in the porcine intestine is an exciting new research frontier, as methods for their identification via in situ and ex vivo staining are now available (57). ILC-IELs are identified as group 1 ILCs in pigs (57), which encompasses both NK cells and ILC1s by classical definitions. However, porcine intestinal ILC-IELs are difficult to discretely classify as NK cells or ILC1s due to tissue-specific characteristics, and this remains an issue even in humans and rodents (15, 152–155). Porcine NK cells are CD3ε-CD2+CD8α+ cells (55, 58, 142, 156, 157) and are scarce throughout the porcine intestine (62, 82, 158–161). However, group 1 ILCs in porcine ileum are transcriptionally distinct from porcine peripheral NK cells, including lack of CD8A expression, the gene encoding for CD8α and used to identify porcine NK cells (57). Group 1 ILCs located in the ileal epithelium instead have gene expression profiles supporting tissue residency, cell activation, and altered metabolic states compared to peripheral NK cells. The majority of ileal group 1 ILCs have transcriptional profiles supporting cytotoxic functions, while smaller subsets express genes indicating cellular division/replication or other effector cell profiles that is characterized by high expression of activation-associated genes, including CTSW, XCL1, CCR9, and genes encoding MHC-II (57). Recent work described above provides only the first established evidence of non-NK intestinal ILC-IELs in pigs, and further work assessing phenotype, function, and distributions is warranted to understand their biological functions.
Though research on porcine intestinal ILC-IELs is only recently emerging, we can cautiously extrapolate ILC-IEL functions from past studies. Previous research hints at the potential presence of ILCs as CD3ε-CD2+CD8α- cells in the pig intestinal tract, which excludes both T cell (CD3ε+) and NK cell (CD3ε-CD2+CD8α+) phenotypes. Shortly after birth, most CD2+ cells in the porcine intestine are CD4-CD8α- (77, 79, 80, 83) and might represent naturally-occurring populations of ILCs that predominate in the intestinal tract prior to antigen-dependent lymphocyte recruitment/expansion. Most but not all CD2+ staining in small intestinal epithelium colocalizes with CD3ε+ staining (68), giving another potential indication of a minority of intestinal CD3ε-CD2+ ILCs in pigs. Within the duodenal and ileal epithelium, IELs located near the basement membrane are primarily CD2+CD4-CD8α+ (likely NK cells or T cells) and morphologically consistent with small, resting lymphocytes; however, IELs located in epithelial nuclear and apical zones that are in closer proximity to the lumen are mostly CD2+CD4-CD8α- and have enlarged cytoplasm with granules and ribosomes (79), thus leaving the possibility for existence of intraepithelial ILCs in epithelial nuclear and apical zones that act as first-line effector cells with cytotoxic capabilities at epithelial barriers. Though the CD3ε-CD2+ phenotype excludes T cells and the CD2+CD4-CD8α- phenotype excludes NK cells, expression of specific NK, B, or myeloid lineage leukocyte markers was not fully assessed in a single study, making it impossible to definitively discern the cell lineage of either CD3ε-CD2+ or CD2+CD4-CD8α- cells. Thus, recent identification of porcine intestinal ILCs and development of tools for their detection present exciting opportunities for future research to better understand biological importance of non-NK ILCs in pigs, including intestinal ILC-IELs.
Indications of IEL effector functions and tissue residency
Tissue resident lymphocytes represent effector immune cells found at local, non-lymphoid tissue sites and can act as first-line immune responders, thus comprising an important component of immune defense often targeted via immunomodulatory strategies [reviewed in (70, 162–164)]. Without deciphering between particular IEL subsets, several studies have indicated IELs are resident, effector cells in the porcine small intestine. One indicator of tissue residency for porcine intestinal IELs is expression of ITGAE (57), which encodes for a subunit of CD103, a marker of tissue residency in humans and rodents (70). Another indicator of tissue residency for porcine intestinal IELs is an observed low exchange rate of IELs with lymphocytes recirculating through the lymph (165, 166), suggesting IELs are largely self-replenishing/long-lived resident cells, at least under steady state conditions. IEL self-replenishment is supported by observing most bromodeoxyuridine (BrdU)+CD2+ cells (indicating proliferating lymphocytes) in the intestinal tract are intraepithelial (65, 83).
Molecular characterization and spatial context of IELs also provides relevant functional insight. About half of porcine small intestinal IELs contain cytoplasmic granules accompanied by prominent mitochondria, ribosomes, nuclei, and nucleoli (167) that are indicative of cytotoxic capacity and elevated metabolic/protein production. IELs with enlarged cytoplasm, granules, and prominent organelles are preferentially found in apical epithelial locations in closer proximity to the lumen, whereas the majority of total IELs are located in the basement membrane, do not have enlarged cytoplasm, and have dense, round nuclei (79, 97). From these observations, we speculate a correlation between luminal proximity and IEL effector function, where IELs in the basement membrane are naïve or patrolling IELs that require additional activating signals to infiltrate the epithelium. In support, inoculating isolated porcine jejunal loops with porcine epidemic diarrhea virus (PEDV) or Bacillus subtilis resulted in overall increases in IELs. Specifically, a proportional increase of apically-located IELs and an increase in proliferating IELs that were mostly apically located occurred after inoculation (87), indicating both pathogenic and non-pathogenic microbial cues derived from the lumen can shape IEL responses associated with their localization.
Though IEL responses to infectious challenge are not a focus of this review, we discuss the recent work by Li and colleagues (87) in greater detail to conclude this section due to implications on porcine IEL immunosurveillance, migratory behaviors, and effector functions. Li et al. recently studied the dynamics of isolated small intestinal porcine IELs in co-culture systems with epithelial cell lines in the context of PEDV infection and pre-exposure. Treatment of co-cultures with live PEDV resulted in enhanced migration of IELs across the epithelial monolayer, which was inhibited in the presence of a CCL2 receptor inhibitor. As CCL2 protein expression was mainly concentrated to the apical epithelial membrane, it appears that IEL migratory responsiveness is reliant on epithelial-IEL crosstalk, by which IELs follow a CCL2 chemotactic gradient to gain closer proximity to the lumen, all without deterioration to the epithelial barrier. Moreover, treatment of PEDV-infected epithelial cells with IELs pre-stimulated with whole-inactivated PEDV resulted in decreased viral titers and increased IEL cytotoxicity, perforin expression, IFN-γ expression, STAT1 phosphorylation, interferon-stimulated gene (ISG) mRNA expression, and targeted apoptosis of virally-infected epithelial cells. Lastly, the ability of IELs to exert effector functions contributing to anti-viral immunity was dependent on IFN-γ activity but was independent of direct cell-cell contact, perforin, and granzymes (87). Collectively, results emphasize the importance of cell contact-independent activation of IELs resulting in migration towards epithelial-derived chemotactic signals at the apical epithelium surface. IEL activation also results in exertion of effector functions including proliferation, targeted killing of infected (and perhaps stressed) epithelial cells, cytotoxicity, and cytokine production. Interestingly, trans-epithelial migration of porcine IELs mediated by IEC-derived signaling in response to microbial stimuli mirrors IEL surveillance and migration dynamics observed in mice (7, 23). Effector functions including porcine IEL cytotoxicity, antiviral activity, and cytokine production (TGF-β) are also reported for porcine intestinal IELs (167), although outside of the context of epithelial co-cultures systems. While TCR-mediated activation (anti-CD3ε stimulation) did not induce an anti-viral response in work by Li et al. (87), TCR stimulation can induce changes in expression of activating receptor NKG2D on subsets of porcine IELs [discussed in earlier sections (112)]. Different anti-CD3ε clones were used to stimulate cells across studies, and anti-CD3ε antibodies used by Li et al. were not verified to activate T cells via TCR stimulation. Overall, further investigation is required to better understand dynamics of porcine intestinal IEL activation that occurs with or without TCR stimulation and how these dynamics tie into IEL roles of immunosurveillance, migratory behaviors, and effector functions in different biological contexts.
Antigen exposure influences IEL recruitment and maturation
From the preceding section, we can gather porcine intestinal IELs have functional roles as tissue resident, effector cells. However, IEL maturation into functionally capable cells is dependent on antigen exposure, as discussed in this section. In the next three subsections, we discuss how microbial, dietary/oral, and environmental antigen exposures affect T-IEL maturation in the developing intestinal immune system.
Microbial colonization
The influence of microbial exposure on intestinal IEL development in pigs is best demonstrated by comparing germ-free (GF) and conventional animals. Intestinal IEL communities in GF pigs resemble those of very young animals in terms of both cell quantities and phenotypes (65, 80, 83), indicating intestinal IEL maturation is dependent on microbial exposure accrued with age when reared in a conventional environment. GF pigs have fewer CD2+ lymphocytes present in the intestinal tract, including less than one-third as many IELs in ileum or jejunum of GF versus conventional pigs aged one to two months old (65, 83). A large percentage of CD2+ cells remain CD4-CD8α- in the epithelium of GF compared to conventional, age-matched animals (80, 83, 168), similar to high frequencies of CD2+CD4-CD8α- IELs in young animals. Context of colonization also influences IEL abundances in the intestinal tract, as the number of IELs in the jejunum and ileum of specific pathogen-free (SPF) animals is intermediate compared to high IEL numbers in conventional pigs and low IEL numbers in GF pigs (65). Thus, intestinal IEL abundance and phenotype in pigs depends on both the presence and context of microbial colonization.
It also remains likely that proximity to luminal microbiota influences effector potential of intestinal IELs. As mentioned in preceding sections, IELs preferentially congregate to intestinal villi that are more intimately associated with the lumen over intestinal crypts that provide a more shielded niche (65). IELs located closer to the lumen have enlarged cytoplasm containing granules, ribosomes, and mitochondria, consistent with increased effector functions (79), and IELs migrate across the epithelium in response to pathogenic and non-pathogenic microbial stimuli (87).
Dietary/oral antigen
Close proximity of IELs with the intestinal lumen likely result in intimate interactions with dietary/orally-administered antigens that also shape functions and behaviors of IELs. For example, seven-day-old pigs given dietary nutrients intravenously to bypass the intestinal tract (total parenteral nutrition [TPN]) have nearly three-fold fewer IELs per villus in jejunum compared to conventionally-fed animals (169), indicating exposure to dietary antigens at the intestine-lumen interface is critical for IEL recruitment/expansion.
Increases in IEL numbers are generally associated with enhanced immune activation of IELs, while decreased IEL numbers are associated with reduced immune activation. For example, pigs fed bacteria producing the immune enhancer, 4,4’-diaponeurosporene, have increased IEL numbers in ileum (170), while 57% fewer jejunal IELs are observed in pigs fed the immunosuppressor, dexamethasone (171), compared to control animals. Though IEL abundances in the intestine are the primary readout for potential immunomodulatory effects of dietary additives, phenotypic and functional studies of porcine IELs in response to dietary immunomodulators are hugely lacking. Thus, IEL abundances cannot be linked directly to any stimulated or suppressed behaviors and are probably largely dependent on more specific biological context, such as pig age, environment, health status, and potential mechanistic outcomes of intervention strategies being implemented. Regardless, we summarize our current knowledge on the effects a subset of dietary immunomodulators have on porcine IEL abundances in the intestinal tract. However, we again emphasize the need for deeper phenotypic and functional analyses of IELs in future research.
Recruitment and maturation of intestinal IELs is largely dependent on the microbiota, and dietary additives affecting the microbiota, such as probiotics and prebiotics, also exert immunomodulatory effects on porcine intestinal IELs. Oral administration of various probiotics leads to increased numbers of IELs in the small and large intestine (86, 97, 101, 172, 173), including increased numbers of CD3ε+CD8α+ cells (174) that might represent induced T-IELs recruited to the intestinal tract. Feeding prebiotics can also have similar effects on intestinal IELs, where feeding rice bran (175), yeast products (95), plant-derived polysaccharides (173), and chito-oligosaccharides (176) results in increased numbers of IELs in the porcine small and/or large intestine. However, yeast product and chito-oligosaccharide feeding causes increases in IELs of the proximal small intestine (duodenum, jejunum) but decreases in distal small intestine [ileum (95, 176)], suggesting regionally-specific immunomodulatory roles. Other dietary additives decrease the number of IELs in the small and/or large intestine of pigs. Plant extracts decrease IEL numbers in jejunum but not ileum or colon (88, 90), whereas spray-dried porcine plasma decreases IEL numbers in jejunum and colon (89, 90).
Oral antibiotic administration has variable effects on IEL numbers. Avilamycin, olaquindox, and cyadox administration result in decreased small intestinal IEL numbers (88, 92). However, others have reported increased numbers of IELs in small intestine after feeding chlorotetracycline (173) or a combination of chlorotetracycline, sulfamethazine, and penicillin (175). Thus, the effect of oral antibiotic administration on IELs is probably dependent on the specific antibiotic being used and the intestinal region being assessed.
Recent limitations on the use of antibiotics as growth promoters has resulted in the need to identify dietary alternatives that may not only promote animal growth but also improve gut health through modulation of the intestinal immune system. As IELs at the epithelial-lumen interface are in close contact with dietary substances and the microbiota, IELs are a prime target to study for the effects of dietary amendment with antibiotic alternatives. As demonstrated above, many dietary additives affect the intestinal microbiota, which likely leads to production of microbial products that contribute to pig intestinal and overall health outcomes. Therefore, understanding the mode of action for various dietary immunomodulators on intestinal health is crucial for developing mechanistic understandings that can be applied through targeted approaches for improving pig health in the future.
Environment
Environmental hygiene influences development of intestinal IELs. Weaned pigs transported to separate facilities have greater numbers of small intestinal IELs and earlier expansion of CD8α+ and γδTCR+ IELs compared to weaned pigs reared on the same farm (177, 178). Though other variables associated with transport cannot be excluded, results suggest IEL recruitment, expansion, and/or maturation in the post-weaning period is accelerated by diverse antigen exposure encountered upon introduction to new environments.
IEL dynamics associated with age and weaning
Weaning is a major stressor that occurs in early life for pigs (~3 or 4 weeks of age), prior to complete development of the intestinal immune system [5-8 weeks of age (46, 77, 80, 125)]. Industry-standard weaning occurs at ~21 days of life in the United States and includes removal of piglets from the sow, introduction to a new environment, abrupt conversion to a solid food diet, intermingling with non-littermates, and often transportation over long distances. We collectively refer to all of these events as ‘weaning’ for the rest of this review. Weaning results in stress and intestinal distress characterized by a combination of inflammation, diarrhea, disease susceptibility, weight loss, increased epithelial permeability, and decreased nutrient absorption (47, 48). Thus, conventional pig weaning represents a major early-life stressor that is detrimental to intestinal health and may impact subsequent intestinal immune system development and intestinal immune cell maturation. Weaning pigs at an earlier age can exacerbate negative weaning impacts and also affects intestinal IELs. Weaning is generally associated with a post-weaning increase in IEL abundances of both small and large intestine that is most notable in the first few days after weaning (76, 77, 81, 93, 97, 179–181). However, detailed phenotypic analyses of IELs in age-matched weaned and non-weaned animals are extremely limited.
Though intestinal IEL populations expand in weaned pigs, full capacity to mount immune responses geared towards intestinal defense or tolerance are not achieved until a later age (46). IELs from pigs weaned at 21 days of age undergo metabolic shifts that increase substrate metabolism and adenosine triphosphate (ATP) production within the first week post-weaning, followed by resuming lower baseline values as post-weaning time increases (182). Moreover, IELs from pigs weaned at 21 days of age cannot proliferate in response to stimulation with T cell mitogens, phytohaemagglutinin (PHA) or concavalin A (ConA), until 11 weeks of age (183). Results suggest an enhanced metabolic state for porcine intestinal IELs but limited effector response shortly after weaning, as the intestinal immune system is not fully developed and IELs continue to mature. Though weaning accelerates the recruitment/expansion of intestinal IELs, as indicated by their increased frequencies in weaned versus non-weaned pigs, weaning may delay the ability of IELs to form an appropriate effector response. As mentioned above, IELs from pigs weaned at 21 days of age do not respond to in vitro PHA or ConA stimulation until 11 weeks of age (183). In contrast, IELs from pigs that are not weaned are able to proliferate in response to PHA or ConA starting at 8 weeks of age (183), indicating weaning delayed the ability of intestinal IELs to mount an effector response.
Results in the preceding paragraph are from functional study of IELs derived from small intestine of pigs, and functions of large intestinal IELs are unresolved in pigs. Our group has assessed expression of CD27 by T-IELs derived from both small and large intestine at one, three, and five weeks post-weaning (66), which can lend inference into functional capacities of T-IELs. A similar majority percentage of CD4-CD8α+ αβTCR, CD2+CD8α+ γδTCR, and CD2+CD8α- γδTCR T-IELs (making up the majority of total T-IELs) express CD27 one week post-weaning in jejunum, ileum, cecum, and colon. However, the percentage of CD27+ cells significantly drops in ileum and to a more drastic extent in cecum and colon by three weeks post-weaning while staying constant in the jejunum (66). By using loss of CD27 as an inferred readout of increased T cell activation, results suggest T-IELs in the large intestine and distal small intestine may be activated at an earlier age. Functional studies in the preceding paragraph were executed using IELs from jejunum (182) and an unspecified small intestinal location (183). Lack of study for T-IELs from the distal intestinal tract, particularly the large intestine, leaves the question of whether IEL activation and ability to mount immune responses might be achieved at an earlier post-weaning age at alternate intestinal locations. Moreover, proportions of different T-IEL subsets (primarily CD4-CD8α+CD8β+ αβTCR, CD4-CD8α+CD8β- αβTCR, CD2+CD8α+ γδTCR, and CD2+CD8α- γδTCR T-IELs) vary by time post-weaning across different intestinal location and have different inferred functional capacities (66, 67), again emphasizing the importance of considering locational context of IELs used in previous functional studies.
Though IEL populations begin to rapidly expand in the porcine small intestine within the first few weeks of life in accordance with antigen exposure, CD8α+ IELs don’t begin appearing with regular frequency until around five weeks of age, two weeks into the post-weaning period for pigs weaned at 3 weeks of age (77). As the post-weaning period provides exposure to new antigens, it’s possible that the majority of CD8α+ IELs are recruited to the intestinal epithelium in response to increased antigen exposure that occurs after weaning. In preceding sections, we established the majority of CD8α+ IELs in the porcine small intestine are CD8αβ+ αβTCR T-IELs, and CD8αβ+ αβTCR T-IELs proportionally increase to make up the largest majority of porcine intestinal T-IELs with post-weaning age (66, 67). In mice, CD8αβ+ αβTCR T-IELs are termed induced IELs because they are recruited to the intestinal epithelium in response to age-associated, antigen-specific activation (184–187). Thus, it’s possible that similar phenomena occur in the pig post-weaning period, where post-weaning expansion of the IEL community is largely due to antigen-specific recruitment of CD8αβ+ αβTCR T-IELs.
Collectively, weaning and age-associated changes in IELs of the porcine intestine are intercalated with events facilitating antigen exposure, including microbial, environmental, and dietary/oral antigen exposure described above. As we can now appreciate, intestinal IELs take time to mature and are influenced by the factors of the developing intestinal tract in which they reside. Thus, results show the importance of stressful early life events and antigenic exposure in shaping the intestinal immune system, including that of porcine intestinal IELs.
Pigs as biomedical models for intestinal IEL research
Throughout previous sections, porcine IEL cross-species parallels were mainly drawn to mice, largely because mice are the primary model species from which IELs are studied. However, major differences between humans and mice exist (discussed below), indicating mice may be a suboptimal model for biomedical intestinal IEL research. Compared to rodents, pigs are promising biomedical models for intestinal research due to greater intestinal physiological, nutritional, and microbial similarities to humans (186–192). Comparability of the intestinal immune system in pigs and humans still requires further investigation to understand key early life differences, including placental transfer of maternal immunity present in humans and mice but not pigs (42). However, due to lack of placentally-transferred maternal immunity, pigs serve as an excellent model to study the impact of maternal immune transfer on subsequent immune system development due to the ability to derive agammaglobulinemic animals. Overall, present knowledge of porcine intestinal IELs presented in this review gives initial indication for suitability of pigs as biomedical models for intestinal IEL research, as summarized in Table 2 and discussed below.
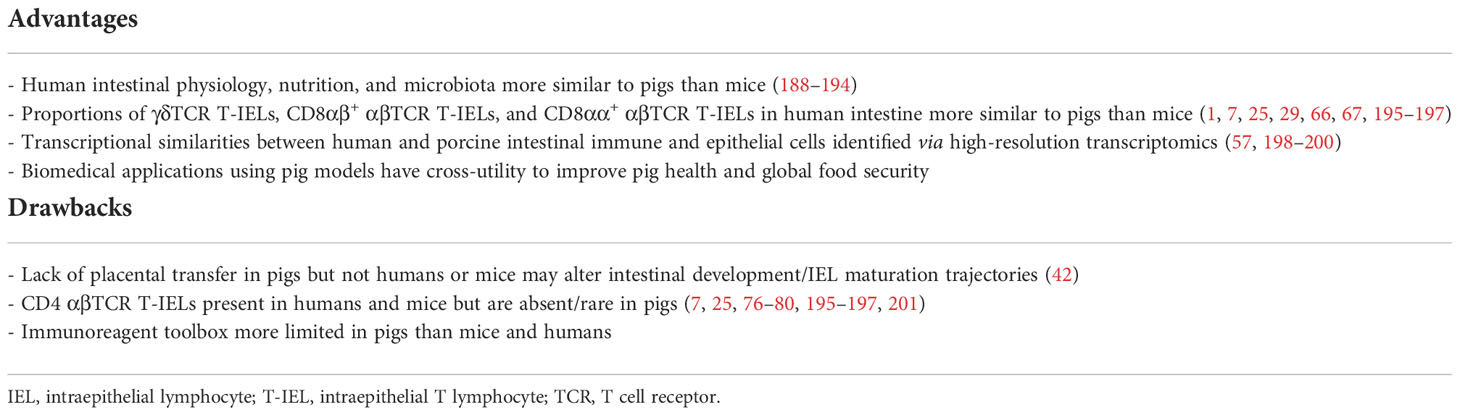
Table 2 Advantages and drawbacks on utility of pigs as biomedical models for intestinal IEL research.
Immune responses and health outcomes are results of culminating signals derived from the overall cellular community of a tissue (202–207). Thus, the proportional composition of cellular constituents is a major factor to consider when selecting a biomedical model to accurately recapitulate human biological systems. Proportions of specific intestinal T-IEL subsets are vastly different between humans and mice while being more similar to pigs in several ways. In the small intestine, over half of human T-IELs are CD8αβ+ αβTCR T-IELs [γδTCR-αβTCR+CD4-CD8α+CD8β+ (25)], whereas most small intestinal T-IELs in mice are instead γδTCR T-IELs [γδTCR+ (7, 25, 195–197)]. Similar to humans, most porcine T-IELs are CD8αβ+ αβTCR T-IELs in the small intestine [γδTCR-CD4-CD8α+CD8β+ (67)]. In humans, the proportion of γδTCR T-IELs (γδTCR+) increases from proximal to distal intestine, whereas γδTCR T-IEL proportions have a proximal-to-distal decline in mice (7, 25, 195–197). Again similar to humans, porcine γδTCR T-IELs (γδTCR+) make up increasing proportions of total intestinal T-IELs along a proximal-to-distal gradient (66, 67). Another key difference in cellular compositions of T-IELs in human and mice is the presence of CD8αα+ αβTCR T-IELs (γδTCR-αβTCR+CD4-CD8α+CD8β-), which make up ~20-40% of total T-IELs in both small and large intestine of mice (7, 25). In contrast, CD8αα+ αβTCR T-IELs are extremely rare or absent from humans (1, 25, 29). Though CD8αα+ αβTCR T-IELs (γδTCR-CD4-CD8α+CD8β-) were recently discovered in the porcine intestinal tract, CD8αα+ αβTCR T-IELs make up only a small fraction of small intestinal (<5%) and large intestinal (average 5-10%) T-IELs (67), thus representing a rare T-IEL subset in pigs more closely mirroring compositional proportions in humans than mice. Though T-IEL compositions between humans and pigs are more similar than human-to-mouse comparisons regarding γδTCR, CD8αβ+ αβTCR, and CD8αα+ αβTCR T-IEL proportions, differences in human versus pig T-IEL compositions do arise, largely from the presence of CD4 αβTCR T-IELs in humans (γδTCR-αβTCR+CD4+) but not pigs. While up to 20% of small intestinal and ~35-45% of large intestinal T-IELs are CD4+ in humans (25, 201), CD4 αβTCR T-IELs have been validated through in situ staining to be absent or extremely rare in porcine intestinal epithelium (addressed in preceding sections). However, similar to pigs (addressed in preceding sections) some human CD4 αβTCR T cells identified in epithelial cell fractions via ex vivo phenotyping could be contaminating sub-epithelial cells. Collectively, community compositions of IELs in pigs and humans share proportional similarities, especially in the small intestine where IELs are more abundant and the majority of IEL research is conducted. Therefore, pigs may be more suitable for the study of intestinal T-IELs than mice, as the overall cellular community from which culminating signals are derived are similar in regards to proportions of γδTCR T-IELs, CD8αβ+ αβTCR T-IELs, and CD8αα+ αβTCR T-IELs; however, the absence of CD4 αβTCR T-IELs in pigs provides a limitation for modeling intestinal CD4 αβTCR T-IELs found in human intestinal epithelium.
In addition to phenotyping a limited number of cellular markers at the protein level to identify proportions of specific IEL populations, high-resolution transcriptional studies suggest pig-to-human similarities of intestinal cells. A recent scRNA-seq study identified conserved expression of several cell type-canonical genes between pig and human epithelial and immune cells that were sparsely expressed in mice (198). An additional scRNA-seq study defining lymphocyte subsets (including T cells and ILCs) at enhanced resolution also identified transcriptional similarities, similar localization patterns, and conserved inferred functions of lymphocytes from porcine and human ileum (57). Yet another scRNA-seq study highlights conserved functions and compositions of epithelial cells between human and pig ileum; however, compositional similarities should be interpreted with caution due to vast differences in cell isolation procedures used between species (199). One final scRNA-seq study expanded analysis to assess transcriptomes of epithelial cells in duodenum, jejunum, and ileum of the porcine small intestine, finding again transcriptional similarities between humans and pigs for the majority of epithelial cells (200). Thus, high-resolution transcriptomic analyses support transcriptional conservation between immune and epithelial cells of human and porcine intestine, but scRNA-seq studies have so far been limited to only the small intestine. Therefore, further research is warranted to expand understanding of potential regional specialization and/or species overlap at additional intestinal locations. No scRNA-seq study has explicitly differentiated between intraepithelial and subepithelial lymphocytes in pigs, as the exclusive study of IELs is complicated by necessity of epithelial cell isolation that is plagued by contamination with sub-epithelial cells (discussed in preceding sections), though this is a species-agnostic issue. Regardless, high-resolution transcriptional profiling has revealed indications of transcriptional conservation across intestinal cells in humans and pigs, which encompasses IELs.
Altogether, preliminary phenotypic research at the protein and gene level indicates porcine IELs more closely mirror human IELs than do IELs from mice. Though immunoreagent availability in pigs is more limited than mice or humans, the toolbox of porcine-specific immunoreagents is rapidly expanding. Breakthroughs in fundamental porcine immunology and intestinal biology are also constantly occurring, leaving the study of porcine IELs, including both T-IELs and ILC-IELs, as an exciting, emerging research frontier. Besides phenotypic descriptions of pigs that draw parallels to humans, functional studies mentioned in preceding sections also highlight the versatility of pigs to study IEL functions that might more closely recapitulate human biology, though further investigation is still required. Unlike most model species, biomedical applications of pigs for intestinal IEL research falls under the One Health initiative, as biomedical research utilizing porcine models has cross-utility for improving pig health related to global food security, a growing world-wide crisis (208). Further research into porcine intestinal IEL functions is thus advantageous for multiple realms of research, emphasizing an integrated research approach producing applicational duality for human and animal health.
Author contributions
JW wrote the manuscript and performed experiments. CL edited the manuscript. All authors contributed to the article and approved the submitted version.
Funding
Work was funded by USDA-ARS CRIS project 5030-3200-225-00D and USDA-NIFA predoctoral grant 2021-67011-35228.
Acknowledgments
We thank the following: (1) Judith Stasko, Adrienne Shircliff, Hannah Mazon, and Ashley Budde for histology assistance; (2) Samuel Humphrey for flow cytometry assistance; (3) Dr. Julian Trachsel and Dr. Christopher Anderson for expert consultation; (4) Celeste Gutierrez for proof-reading.
Conflict of interest
The authors declare that the research was conducted in the absence of any commercial or financial relationships that could be construed as a potential conflict of interest.
Publisher’s note
All claims expressed in this article are solely those of the authors and do not necessarily represent those of their affiliated organizations, or those of the publisher, the editors and the reviewers. Any product that may be evaluated in this article, or claim that may be made by its manufacturer, is not guaranteed or endorsed by the publisher.
Abbreviations
- ^ ATP, adenosine triphosphate; BrdU, bromodeoxyuridine; CD, cluster of differentiation; ConA, concavalin A; DC, dendritic cell; EDTA, ethylenediamine tetraacetic acid; GF, germ-free; H&E, hematoxylin & eosin; IEC, intestinal epithelial cell; IEL, intraepithelial lymphocyte; IHC, immunohistochemistry; ILC, innate lymphoid cell; ILC-IEL, intraepithelial innate lymphoid cell; ISG, interferon-stimulated gene; MHC, major histocompatibility complex; MIC, MHC class I-related molecules; NK, natural killer; PEDV, porcine epidemic diarrhea virus; PHA, phytohaemagglutinin; RNA-ISH, RNA in situ hybridization; scRNA-seq, single-cell RNA sequencing; SPF, specific pathogen-free; SWC6, swine workshop cluster 6; T-IEL, intraepithelial T lymphocyte; TCR, T cell receptor; TPN, total parenteral nutrition.
References
1. Olivares-Villagómez D, Van Kaer L. Intestinal intraepithelial lymphocytes: sentinels of the mucosal barrier. Trends Immunol (2018) 39(4):264–75. doi: 10.1016/j.it.2017.11.003
2. Chen Y, Chou K, Fuchs E, Havran WL, Boismenu R. Protection of the intestinal mucosa by intraepithelial T cells. Proc Natl Acad Sci USA (2002) 99(22):14338–43. doi: 10.1073/pnas.212290499
3. Ismail AS, Behrendt CL, Hooper LV. Reciprocal interactions between commensal bacteria and γδ intraepithelial lymphocytes during mucosal injury. J Immunol (2009) 182(5):3047. doi: 10.4049/jimmunol.0802705
4. Ismail AS, Severson KM, Vaishnava S, Behrendt CL, Yu X, Benjamin JL, et al. γδ intraepithelial lymphocytes are essential mediators of host–microbial homeostasis at the intestinal mucosal surface. Proc Natl Acad Sci USA (2011) 108(21):8743–8. doi: 10.1073/pnas.1019574108
5. Kühl AA, Pawlowski NN, Grollich K, Loddenkemper C, Zeitz M, Hoffmann JC. Aggravation of intestinal inflammation by depletion/deficiency of γδ T cells in different types of IBD animal models. J Leukoc Biol (2007) 81(1):168–75. doi: 10.1189/jlb.1105696
6. Boismenu R, Havran WL. Modulation of epithelial cell growth by intraepithelial γδ T cells. Science (1994) 266(5188):1253–5. doi: 10.1126/science.7973709
7. Hoytema van Konijnenburg DP, Reis BS, Pedicord VA, Farache J, Victora GD, Mucida D. Intestinal epithelial and intraepithelial T cell crosstalk mediates a dynamic response to infection. Cell (2017) 171(4):783–94.e13. doi: 10.1016/j.cell.2017.08.046
8. Dalton JE, Cruickshank SM, Egan CE, Mears R, Newton DJ, Andrew EM, et al. Intraepithelial γδ+ lymphocytes maintain the integrity of intestinal epithelial tight junctions in response to infection. Gastroenterology (2006) 131(3):818–29. doi: 10.1053/j.gastro.2006.06.003
9. Hu MD, Golovchenko NB, Burns GL, Nair PM, Kelly TJ, Agos J, et al. γδ intraepithelial lymphocytes facilitate pathological epithelial cell shedding via CD103-mediated granzyme release. Gastroenterology (2022) 162(3):877–89.e7. doi: 10.1053/j.gastro.2021.11.028
10. Sullivan ZA, Khoury-Hanold W, Lim J, Smillie C, Biton M, Reis BS, et al. γδ T cells regulate the intestinal response to nutrient sensing. Science (2021) 371(6535):eaba8310. doi: 10.1126/science.aba8310
11. Denning TL, Granger S, Mucida D, Graddy R, Leclercq G, Zhang W, et al. Mouse TCRαβ+CD8αα intraepithelial lymphocytes express genes that down-regulate their antigen reactivity and suppress immune responses. J Immunol (2007) 178(7):4230. doi: 10.4049/jimmunol.178.7.4230
12. Edelblum KL, Singh G, Odenwald MA, Lingaraju A, El Bissati K, McLeod R, et al. γδ intraepithelial lymphocyte migration limits transepithelial pathogen invasion and systemic disease in mice. Gastroenterology (2015) 148(7):1417–26. doi: 10.1053/j.gastro.2015.02.053
13. Inagaki-Ohara K, Sakamoto Y, Dohi T, Smith AL. γδ T cells play a protective role during infection with nippostrongylus brasiliensis by promoting goblet cell function in the small intestine. Immunology (2011) 134(4):448–58. doi: 10.1111/j.1365-2567.2011.03503.x
14. Fuchs A, Vermi W, Lee JS, Lonardi S, Gilfillan S, Newberry RD, et al. Intraepithelial type 1 innate lymphoid cells are a unique subset of IL-12- and IL-15-responsive IFN-γ-producing cells. Immunity (2013) 38(4):769–81. doi: 10.1016/j.immuni.2013.02.010
15. Van Acker A, Gronke K, Biswas A, Martens L, Saeys Y, Filtjens J, et al. A murine intestinal intraepithelial NKp46-negative innate lymphoid cell population characterized by group 1 properties. Cell Rep (2017) 19(7):1431–43. doi: 10.1016/j.celrep.2017.04.068
16. Simpson SJ, Holländer GA, Mizoguchi E, Allen D, Bhan AK, Wang B, et al. Expression of pro-inflammatory cytokines by TCRαβ+ T and TCRγδ+ T cells in an experimental model of colitis. Eur J Immunol (1997) 27(1):17–25. doi: 10.1002/eji.1830270104
17. Kumar AA, Delgado AG, Piazuelo MB, Van Kaer L, Olivares-Villagómez D. Innate CD8αα+ lymphocytes enhance anti-CD40 antibody-mediated colitis in mice. Immun Inflammation Dis (2017) 5(2):109–23. doi: 10.1002/iid3.146
18. Abadie V, Discepolo V, Jabri B. Intraepithelial lymphocytes in celiac disease immunopathology. Semin Immunopathol (2012) 34(4):551–66. doi: 10.1007/s00281-012-0316-x
19. Jabri B, Sollid LM. T Cells in celiac disease. J Immunol (2017) 198(8):3005. doi: 10.4049/jimmunol.1601693
20. Kawaguchi-Miyashita M, Shimada S-i, Kurosu H, Kato-Nagaoka N, Matsuoka Y, Ohwaki M, et al. An accessory role of TCRγ δ + cells in the exacerbation of inflammatory bowel disease in TCRα mutant mice. Eur J Immunol (2001) 31(4):980–8. doi: 10.1002/1521-4141(200104)31:4<980::AID-IMMU980>3.0.CO;2-U
21. Vandereyken M, James OJ, Swamy M. Mechanisms of activation of innate-like intraepithelial T lymphocytes. Mucosal Immunol (2020) 13(5):721–31. doi: 10.1038/s41385-020-0294-6
22. Cheroutre H, Lambolez F, Mucida D. The light and dark sides of intestinal intraepithelial lymphocytes. Nat Rev Immunol (2011) 11(7):445–56. doi: 10.1038/nri3007
23. Fischer MA, Golovchenko NB, Edelblum KL. γδ T cell migration: separating trafficking from surveillance behaviors at barrier surfaces. Immunol Rev (2020) 298(1):165–80. doi: 10.1111/imr.12915
24. Hoytema van Konijnenburg DP, Mucida D. Intraepithelial lymphocytes. Curr Biol (2017) 27(15):R737–R9. doi: 10.1016/j.cub.2017.05.073
25. Mayassi T, Jabri B. Human intraepithelial lymphocytes. Mucosal Immunol (2018) 11(5):1281–9. doi: 10.1038/s41385-018-0016-5
26. Qiu Y, Yang H. Effects of intraepithelial lymphocyte-derived cytokines on intestinal mucosal barrier function. J Interferon Cytokine Res (2013) 33(10):551–62. doi: 10.1089/jir.2012.0162
27. Sheridan BS, Lefrançois L. Intraepithelial lymphocytes: to serve and protect. Curr Gastroenterol Rep (2010) 12(6):513–21. doi: 10.1007/s11894-010-0148-6
28. Sumida H. Dynamics and clinical significance of intestinal intraepithelial lymphocytes. Immunol Med (2019) 42(3):117–23. doi: 10.1080/25785826.2019.1658516
29. Van Kaer L, Olivares-Villagómez D. Development, homeostasis, and functions of intestinal intraepithelial lymphocytes. J Immunol (2018) 200(7):2235–44. doi: 10.4049/jimmunol.1701704
30. Wyatt CR, Brackett EJ, Perryman LE, Davis WC. Identification of γδ T lymphocyte subsets that populate calf ileal mucosa after birth. Vet Immunol Immunopathol (1996) 52(1):91–103. doi: 10.1016/0165-2427(95)05535-5
31. Nagi AM, Babiuk LA. Characterization of surface markers of bovine gut mucosal leukocytes using monoclonal antibodies. Vet Immunol Immunopathol (1989) 22(1):1–14. doi: 10.1016/0165-2427(89)90159-1
32. Waters WR, Harp JA, Nonnecke BJ. Phenotypic analysis of peripheral blood lymphocytes and intestinal intra-epithelial lymphocytes in calves. Vet Immunol Immunopathol (1995) 48(3):249–59. doi: 10.1016/0165-2427(95)05430-E
33. Gorrell MD, Willis G, Brandon MR, Lascelles AK. Lymphocyte phenotypes in the intestinal mucosa of sheep infected with trichostrongylus colubriformis. Clin Exp Immunol (1988) 72(2):274–9.
34. Navarro JA, Seva J, Caro MR, Sánchez J, Gómez MA, Bernabé A. Postnatal development of lymphocyte subpopulations in the intestinal mucosa in goat. Vet Immunol Immunopathol (1997) 55(4):303–11. doi: 10.1016/S0165-2427(96)05625-5
35. Ma M, Bai X, Wang Z, Dong Y, Chen Y, Cao J. Distribution of intraepithelial lymphocytes, mast cells, and goblet cells in the intestine of alpaca. Anat Histol Embryol (2022) 51(4):501–8. doi: 10.1111/ahe.12823
36. Lowden S, Heath T. Lymphoid tissues of the ileum in young horses: distribution, structure, and epithelium. Anat Embryol (1995) 192(2):171–9. doi: 10.1007/BF00186005
37. Rocchigiani G, Ricci E, Navarro MA, Samol MA, Uzal FA. Leukocyte numbers and intestinal mucosal morphometrics in horses with no clinical intestinal disease. J Vet Diagn (2022) 34(3):389–95. doi: 10.1177/10406387211031944
38. Chai JY, Lillehoj HS. Isolation and functional characterization of chicken intestinal intra-epithelial lymphocytes showing natural killer cell activity against tumour target cells. Immunology (1988) 63(1):111–7.
39. Lawn AM, Rose ME, Bradley JW, Rennie MC. Lymphocytes of the intestinal mucosa of chickens. Cell Tissue Res (1988) 251(1):189–95. doi: 10.1007/BF00215464
40. Agrawal PK, Reynolds DL. A comparative analysis of the mitogenic response of intestinal intraepithelial lymphocytes in various age groups of turkeys. Avian Dis (1999) 43(3):376–83. doi: 10.2307/1592634
41. United States Department of Agriculture FAS. . Available at: https://apps.fas.usda.gov/psdonline/app/index.html#/app/advQuery.
42. Poonsuk K, Zimmerman J. Historical and contemporary aspects of maternal immunity in swine. Anim Health Res Rev (2018) 19(1):31–45. doi: 10.1017/S1466252317000123
43. Al Nabhani Z, Dulauroy S, Marques R, Cousu C, Al Bounny S, Déjardin F, et al. A weaning reaction to microbiota is required for resistance to immunopathologies in the adult. Immunity (2019) 50(5):1276–88.e5. doi: 10.1016/j.immuni.2019.02.014
44. Rogier EW, Frantz AL, Bruno MEC, Wedlund L, Cohen DA, Stromberg AJ, et al. Secretory antibodies in breast milk promote long-term intestinal homeostasis by regulating the gut microbiota and host gene expression. Proc Natl Acad Sci USA (2014) 111(8):3074–9. doi: 10.1073/pnas.1315792111
45. Salmon H, Berri M, Gerdts V, Meurens F. Humoral and cellular factors of maternal immunity in swine. Dev Comp Immunol (2009) 33(3):384–93. doi: 10.1016/j.dci.2008.07.007
46. Stokes CR, Bailey M, Haverson K, Harris C, Jones P, Inman C, et al. Postnatal development of intestinal immune system in piglets: implications for the process of weaning. Anim Res (2004) 53(4):325–34. doi: 10.1051/animres:2004020
47. Moeser AJ, Pohl CS, Rajput M. Weaning stress and gastrointestinal barrier development: Implications for lifelong gut health in pigs. Anim Nutr (2017) 3(4):313–21. doi: 10.1016/j.aninu.2017.06.003
48. Campbell JM, Crenshaw JD, Polo J. The biological stress of early weaned piglets. J Anim Sci Biotechnol (2013) 4(1):19. doi: 10.1186/2049-1891-4-19
49. Sauls RS, McCausland C, Taylor BN eds. Histology, T-cell lymphocyte (2019). (Treasure Island (FL): StatPearls Publishing).
51. Hudacko R, Kathy Zhou X, Yantiss RK. Immunohistochemical stains for CD3 and CD8 do not improve detection of gluten-sensitive enteropathy in duodenal biopsies. Mod Pathol (2013) 26(9):1241–5. doi: 10.1038/modpathol.2013.57
52. Cooper R, Papworth NJ, Harris C, Horne J, Lai J, Lai J, et al. Counting intraepithelial lymphocytes: A comparison between routine staining and CD3 immunohistochemistry. Int J Surg Pathol (2020) 28(4):367–70. doi: 10.1177/1066896919894644
53. Hermiston ML, Xu Z, Weiss A. CD45: a critical regulator of signaling thresholds in immune cells. Annu Rev Immunol (2003) 21(1):107–37. doi: 10.1146/annurev.immunol.21.120601.140946
54. Courtney AH, Shvets AA, Lu W, Griffante G, Mollenauer M, Horkova V, et al. CD45 functions as a signaling gatekeeper in T cells. Sci Signal (2019) 12(604):eaaw8151. doi: 10.1126/scisignal.aaw8151
55. Piriou-Guzylack L, Salmon H. Membrane markers of the immune cells in swine: an update. Vet Res (2008) 39(6):54. doi: 10.1051/vetres:2008030
56. Zuckermann FA, Peavey C, Schnitzlein WM, Schabacker D, Husmann RJ, Yang H, et al. Definition of the specificity of monoclonal antibodies against porcine CD45 and CD45R: report from the CD45/CD45R and CD44 subgroup of the second international swine CD workshop. Vet Immunol Immunopathol (1998) 60(3):367–87. doi: 10.1016/S0165-2427(97)00112-8
57. Wiarda JE, Trachsel JM, Sivasankaran SK, Tuggle CK, Loving CL. Intestinal single-cell atlas reveals novel lymphocytes in pigs with similarities to human cells. Life Sci Alliance (2022) 5(10):e202201442. doi: 10.26508/lsa.202201442
58. Gerner W, Käser T, Saalmüller A. Porcine T lymphocytes and NK cells – an update. Dev Comp Immunol (2009) 33(3):310–20. doi: 10.1016/j.dci.2008.06.003
59. Herrera-Uribe J, Wiarda JE, Sivasankaran SK, Daharsh L, Liu H, Byrne KA, et al. Reference transcriptomes of porcine peripheral immune cells created through bulk and single-cell RNA sequencing. Front Genet (2021) 12:689406. doi: 10.3389/fgene.2021.689406
60. Bromberg JS. The biology of CD2: adhesion, transmembrane signal, and regulatory receptor of immunity. J Surg Res (1993) 54(3):258–67. doi: 10.1006/jsre.1993.1041
61. Binder C, Cvetkovski F, Sellberg F, Berg S, Paternina Visbal H, Sachs DH, et al. CD2 immunobiology. Front Immunol (2020) 11:1090. doi: 10.3389/fimmu.2020.01090
62. Potockova H, Sinkorova J, Karova K, Sinkora M. The distribution of lymphoid cells in the small intestine of germ-free and conventional piglets. Dev Comp Immunol (2015) 51(1):99–107. doi: 10.1016/j.dci.2015.02.014
63. Davis WC, Zuckermann FA, Hamilton MJ, Barbosa JIR, Saalmüller A, Binns RM, et al. Analysis of monoclonal antibodies that recognize γδ t/null cells. Vet Immunol Immunopathol (1998) 60(3):305–16. doi: 10.1016/S0165-2427(97)00107-4
64. Binns R, Duncan I, Powis S, Hutchings A, Butcher G. Subsets of null and gamma delta T-cell receptor+ T lymphocytes in the blood of young pigs identified by specific monoclonal antibodies. Immunol (1992) 77(2):219.
65. Rothkötter H, Möllhoff S, Pabst R. The influence of age and breeding conditions on the number and proliferation of intraepithelial lymphocytes in pigs. Scand J Immunol (1999) 50(1):31–8. doi: 10.1046/j.1365-3083.1999.00557.x
66. Wiarda JE, Trachsel JM, Bond ZF, Byrne KA, Gabler NK, Loving CL. Intraepithelial T cells diverge by intestinal location as pigs age. Front Immunol (2020) 11:1139. doi: 10.3389/fimmu.2020.01139
67. Wiarda JE, Watkins HR, Gabler NK, Anderson CL, Loving CL. Intestinal location- and age-specific variation of intraepithelial T lymphocytes and mucosal microbiota in pigs. Res Square (2022) 139:104590. doi: 10.1016/j.dci.2022.104590
68. Haverson K, Rehakova Z, Sinkora J, Sver L, Bailey M. Immune development in jejunal mucosa after colonization with selected commensal gut bacteria: A study in germ-free pigs. Vet Immunol Immunopathol (2007) 119(3):243–53. doi: 10.1016/j.vetimm.2007.05.022
69. Wang F, Flanagan J, Su N, Wang L-C, Bui S, Nielson A, et al. RNAscope: a novel in situ RNA analysis platform for formalin-fixed, paraffin-embedded tissues. J Mol Diagn (2012) 14(1):22–9. doi: 10.1016/j.jmoldx.2011.08.002
70. Szabo PA, Miron M, Farber DL. Location, location, location: tissue resident memory T cells in mice and humans. Sci Immunol (2019) 4(34):eaas9673. doi: 10.1126/sciimmunol.aas9673
71. Kilshaw P, Baker K. A unique surface antigen on intraepithelial lymphocytes in the mouse. Immunol Lett (1988) 18(2):149–54. doi: 10.1016/0165-2478(88)90056-9
72. Cerf-Bensussan N, Jarry A, Brousse N, Lisowska-Grospierre B, Guy-Grand D, Griscelli C. A monoclonal antibody (HML-1) defining a novel membrane molecule present on human intestinal lymphocytes. Eur J Immunol (1987) 17(9):1279–85. doi: 10.1002/eji.1830170910
73. Ettersperger J, Montcuquet N, Malamut G, Guegan N, Lopez-Lastra S, Gayraud S, et al. Interleukin-15-dependent T-cell-like innate intraepithelial lymphocytes develop in the intestine and transform into lymphomas in celiac disease. Immunity (2016) 45(3):610–25. doi: 10.1016/j.immuni.2016.07.018
74. Iiai T, Watanabe H, Suda T, Okamoto H, Abo T, Hatakeyama K. CD161+ T (NT) cells exist predominantly in human intestinal epithelium as well as in liver. Clin Exp Immunol (2002) 129(1):92–8. doi: 10.1046/j.1365-2249.2002.01886.x
75. Ericsson A, Svensson M, Arya A, Agace WW. CCL25/CCR9 promotes the induction and function of CD103 on intestinal intraepithelial lymphocytes. Eur J Immunol (2004) 34(10):2720–9. doi: 10.1002/eji.200425125
76. Brown D, Maxwell C, Erf G, Davis M, Singh S, Johnson Z. The influence of different management systems and age on intestinal morphology, immune cell numbers and mucin production from goblet cells in post-weaning pigs. Vet Immunol Immunopathol (2006) 111(3-4):187–98. doi: 10.1016/j.vetimm.2005.12.006
77. Vega-Lopez M, Bailey M, Telemo E, Stokes C. Effect of early weaning on the development of immune cells in the pig small intestine. Vet Immunol Immunopathol (1995) 44(3-4):319–27. doi: 10.1016/0165-2427(94)05309-G
78. Vega-Lopez M, Telemo E, Bailey M, Stevens K, Stokes C. Immune cell distribution in the small intestine of the pig: immunohistological evidence for an organized compartmentalization in the lamina propria. Vet Immunol Immunopathol (1993) 37(1):49–60. doi: 10.1016/0165-2427(93)90015-V
79. Vega-Lopez M, Arenas-Contreras G, Bailey M, Gonzalez-Pozos S, Stokes C, Ortega M, et al. Development of lntraepithelial cells in the porcine small intestine. Dev Immunol (2001) 8(2):147–58. doi: 10.1155/2001/25301
80. Rothkötter H, Ulbrich H, Pabst R. The postnatal development of gut lamina propria lymphocytes: number, proliferation, and T and b cell subsets in conventional and germ-free pigs. Pediatr Res (1991) 29(3):237–42. doi: 10.1203/00006450-199103000-00004
81. Solano-Aguilar GI, Vengroski KG, Beshah E, Douglass LW, Lunney JK. Characterization of lymphocyte subsets from mucosal tissues in neonatal swine. Dev Comp Immunol (2001) 25(3):245–63. doi: 10.1016/S0145-305X(00)00053-7
82. Wasowicz K, Winnicka A, Kaleczyc J, Zalecki M, Podlasz P, Pidsudko Z. Neuropeptides and lymphocyte populations in the porcine ileum and ileocecal lymph nodes during postnatal life. PloS One (2018) 13(5):e0196458. doi: 10.1371/journal.pone.0196458
83. Rothkötter H, Kirchhoff T, Pabst R. Lymphoid and non-lymphoid cells in the epithelium and lamina propria of intestinal mucosa of pigs. Gut (1994) 35(11):1582–9. doi: 10.1136/gut.35.11.1582
84. Nofrarías M, Martínez-Puig D, Pujols J, Majó N, Pérez JF. Long-term intake of resistant starch improves colonic mucosal integrity and reduces gut apoptosis and blood immune cells. Nutrition (2007) 23(11-12):861–70. doi: 10.1016/j.nut.2007.08.016
85. Ayuso M, Michiels J, Wuyts S, Yan H, Degroote J, Lebeer S, et al. Short-chain fructo-oligosaccharides supplementation to suckling piglets: assessment of pre-and post-weaning performance and gut health. PloS One (2020) 15(6):e0233910. doi: 10.1371/journal.pone.0233910
86. Gāliņa D, Ansonska L, Valdovska A. Effect of probiotics and herbal products on intestinal histomorphological and immunological development in piglets. Vet Med Int (2020) 2020:3461768. doi: 10.1155/2020/3461768
87. Li Y, Ma Y, Jin Y, Peng X, Wang X, Zhang P, et al. Porcine intraepithelial lymphocytes undergo migration and produce an antiviral response following intestinal virus infection. Commun Biol (2022) 5(1):1–16. doi: 10.1038/s42003-022-03205-2
88. Manzanilla E, Nofrarias M, Anguita M, Castillo M, Perez J, Martin-Orue S, et al. Effects of butyrate, avilamycin, and a plant extract combination on the intestinal equilibrium of early-weaned pigs. J Anim Sci (2006) 84(10):2743–51. doi: 10.2527/jas.2005-509
89. Nofrarías M, Manzanilla E, Pujols J, Gibert X, Majó N, Segalés J, et al. Spray-dried porcine plasma affects intestinal morphology and immune cell subsets of weaned pigs. Livest Sci (2007) 108(1-3):299–302. doi: 10.1016/j.livsci.2007.01.103
90. Nofrarias M, Manzanilla EG, Pujols J, Gibert X, Majo N, Segalés J, et al. Effects of spray-dried porcine plasma and plant extracts on intestinal morphology and on leukocyte cell subsets of weaned pigs. J Anim Sci (2006) 84(10):2735–42. doi: 10.2527/jas.2005-414
91. Barszcz M, Taciak M, Skomiał J. The effects of inulin, dried Jerusalem artichoke tuber and a multispecies probiotic preparation on microbiota ecology and immune status of the large intestine in young pigs. Arch Anim Nutr (2016) 70(4):278–92. doi: 10.1080/1745039X.2016.1184368
92. Ding M, Yuan Z, Wang Y, Zhu H, Fan S. Olaquindox and cyadox stimulate growth and decrease intestinal mucosal immunity of piglets orally inoculated with escherichia coli. J Anim Physiol Anim Nutr (2006) 90(5-6):238–43. doi: 10.1111/j.1439-0396.2005.00593.x
93. Kelly D, Smyth J, McCracken K. Effect of creep feeding on structural and functional changes of the gut of early weaned pigs. Res Vet Sci (1990) 48(3):350–6. doi: 10.1016/S0034-5288(18)31026-9
94. Wang J, Huang L, Mou C, Zhang E, Wang Y, Cao Y, et al. Mucosal immune responses induced by oral administration recombinant bacillus subtilis expressing the COE antigen of PEDV in newborn piglets. Biosci Rep (2019) 39(3):BSR20182028. doi: 10.1042/BSR20182028
95. Yang H-s, Wu F, Long L-n, Li T-J, Xiong X, Liao P, et al. Effects of yeast products on the intestinal morphology, barrier function, cytokine expression, and antioxidant system of weaned piglets. J Zhejiang Univ Sci B (2016) 17(10):752–62. doi: 10.1631/jzus.B1500192
96. Lærke HN, Hedemann MS. The digestive system of the pig. Nutritional physiology of pigs (Chapter 5). Foulumn, Denmark: Videncenter for Svineproduktion, (2012).
97. Kalita A, Talukdar M, Sarma K, Kalita P, Roychoudhury P, Kalita G, et al. Small intestinal mucosal cells in piglets fed with probiotic and zinc: A qualitative and quantitative microanatomical study. Folia Morphol (2021) 80(3):605–17.
98. Torrallardona D, Andrés-Elias N, López-Soria S, Badiola I, Cerdà-Cuéllar M. Effect of feeding piglets with different extruded and nonextruded cereals on the gut mucosa and microbiota during the first postweaning week. J Anim Sci (2012) 90(suppl_4):7–9. doi: 10.2527/jas.53910
99. Johansson ME, Phillipson M, Petersson J, Velcich A, Holm L, Hansson GC. The inner of the two Muc2 mucin-dependent mucus layers in colon is devoid of bacteria. Proc Natl Acad Sci USA (2008) 105(39):15064–9. doi: 10.1073/pnas.0803124105
100. Atuma C, Strugala V, Allen A, Holm L. The adherent gastrointestinal mucus gel layer: thickness and physical state in vivo. Am J Physiol Gastrointest (2001) 280(5):G922–G9. doi: 10.1152/ajpgi.2001.280.5.G922
101. Rieger J, Janczyk P, Hünigen H, Neumann K, Plendl J. Intraepithelial lymphocyte numbers and histomorphological parameters in the porcine gut after Enterococcus faecium NCIMB 10415 feeding in a salmonella typhimurium challenge. Vet Immunol Immunopathol (2015) 164(1-2):40–50. doi: 10.1016/j.vetimm.2014.12.013
102. McDonald BD, Bunker JJ, Ishizuka IE, Jabri B, Bendelac A. Elevated T cell receptor signaling identifies a thymic precursor to the TCRαβ+ CD4– CD8β– intraepithelial lymphocyte lineage. Immunity (2014) 41(2):219–29. doi: 10.1016/j.immuni.2014.07.008
103. Pobezinsky LA, Angelov GS, Tai X, Jeurling S, Van Laethem F, Feigenbaum L, et al. Clonal deletion and the fate of autoreactive thymocytes that survive negative selection. Nat Immunol (2012) 13(6):569–78. doi: 10.1038/ni.2292
104. Ruscher R, Kummer RL, Lee YJ, Jameson SC, Hogquist KA. CD8αα intraepithelial lymphocytes arise from two main thymic precursors. Nat Immunol (2017) 18(7):771–9. doi: 10.1038/ni.3751
105. Denning TL, Granger S, Mucida D, Graddy R, Leclercq G, Zhang W, et al. Mouse TCRαβ+ CD8αα intraepithelial lymphocytes express genes that down-regulate their antigen reactivity and suppress immune responses. J Immunol (2007) 178(7):4230–9. doi: 10.4049/jimmunol.178.7.4230
106. Van Oers N, Teh SJ, Garvin AM, Forbush KA, Perlmutter RM, Teh HS. CD8 inhibits signal transduction through the T cell receptor in CD4-CD8-thymocytes from T cell receptor transgenic mice reconstituted with a transgenic CD8 alpha molecule. J Immunol (1993) 151(2):777–90.
107. Cheroutre H, Lambolez F. Doubting the TCR coreceptor function of CD8αα. Immunity (2008) 28(2):149–59. doi: 10.1016/j.immuni.2008.01.005
108. Geng J, Raghavan M. CD8αα homodimers function as a coreceptor for KIR3DL1. Proc Natl Acad Sci USA (2019) 116(36):17951–6. doi: 10.1073/pnas.1905943116
109. Masopust D, Vezys V, Marzo AL, Lefrançois L. Preferential localization of effector memory cells in nonlymphoid tissue. Science (2001) 291(5512):2413–7. doi: 10.1126/science.1058867
110. Montufar-Solis D, Garza T, Klein JR. T-Cell activation in the intestinal mucosa. Immunol Rev (2007) 215(1):189–201. doi: 10.1111/j.1600-065X.2006.00471.x
111. Altmeyer S, Kröger S, Vahjen W, Zentek J, Scharek-Tedin L. Impact of a probiotic Bacillus cereus strain on the jejunal epithelial barrier and on the NKG2D expressing immune cells during the weaning phase of piglets. Vet Immunol Immunopathol (2014) 161(1-2):57–65. doi: 10.1016/j.vetimm.2014.07.001
112. Altmeyer S, Zentek J, Vahjen W, Scharek-Tedin L. The expression of NKG2D on porcine IEL and its possible relation to the adaptive intestinal immune system. Vet Immunol Immunopathol (2017) 187:89–95. doi: 10.1016/j.vetimm.2017.03.005
113. Wen K, Bui T, Li G, Liu F, Li Y, Kocher J, et al. Characterization of immune modulating functions of γδ T cell subsets in a gnotobiotic pig model of human rotavirus infection. Comp immunol Microbiol Infect Dis (2012) 35(4):289–301. doi: 10.1016/j.cimid.2012.01.010
114. Couzi L, Pitard V, Sicard X, Garrigue I, Hawchar O, Merville P, et al. Antibody-dependent anti-cytomegalovirus activity of human γδ T cells expressing CD16 (FcγRIIIa). Blood (2012) 119(6):1418–27. doi: 10.1182/blood-2011-06-363655
115. Farrington LA, Callaway PC, Vance HM, Baskevitch K, Lutz E, Warrier L, et al. Opsonized antigen activates Vδ2+ T cells via CD16/FCγRIIIa in individuals with chronic malaria exposure. PloS Pathog (2020) 16(10):e1008997. doi: 10.1371/journal.ppat.1008997
116. Mahnke YD, Brodie TM, Sallusto F, Roederer M, Lugli E. The who’s who of T-cell differentiation: human memory T-cell subsets. Eur J Immunol (2013) 43(11):2797–809. doi: 10.1002/eji.201343751
117. Van De Ven K, Borst J. Targeting the T-cell co-stimulatory CD27/CD70 pathway in cancer immunotherapy: rationale and potential. Immunotherapy (2015) 7(6):655–67. doi: 10.2217/imt.15.32
118. Gerner W, Mair KH, Schmidt S. Local and systemic T cell immunity in fighting pig viral and bacterial infections. Annu Rev Anim Biosci (2022) 10:349–72. doi: 10.1146/annurev-animal-013120-044226
119. Lagumdzic E, Pernold C, Viano M, Olgiati S, Schmitt MW, Mair KH, et al. Transcriptome profiling of porcine naïve, intermediate and terminally differentiated CD8+ T cells. Front Immunol (2022) 13:849922. doi: 10.3389/fimmu.2022.849922
120. Reutner K, Leitner J, Essler SE, Witter K, Patzl M, Steinberger P, et al. Porcine CD27: identification, expression and functional aspects in lymphocyte subsets in swine. Dev Comp Immunol (2012) 38(2):321–31. doi: 10.1016/j.dci.2012.06.011
121. Reutner K, Leitner J, Müllebner A, Ladinig A, Essler SE, Duvigneau JC, et al. CD27 expression discriminates porcine T helper cells with functionally distinct properties. Vet Res (2013) 44(1):1–14. doi: 10.1186/1297-9716-44-18
122. Rodríguez-Gómez IM, Talker SC, Käser T, Stadler M, Hammer SE, Saalmüller A, et al. Expression of T-bet, eomesodermin and GATA-3 in porcine αβ T cells. Dev Comp Immunol (2016) 60:115–26. doi: 10.1016/j.dci.2016.02.022
123. Rodríguez-Gómez IM, Talker SC, Käser T, Stadler M, Reiter L, Ladinig A, et al. Expression of T-bet, eomesodermin, and GATA-3 correlates with distinct phenotypes and functional properties in porcine γδ T cells. Front Immunol (2019) 10:396. doi: 10.3389/fimmu.2019.00396
124. Talker SC, Käser T, Reutner K, Sedlak C, Mair KH, Koinig H, et al. Phenotypic maturation of porcine NK-and T-cell subsets. Dev Comp Immunol (2013) 40(1):51–68. doi: 10.1016/j.dci.2013.01.003
125. Stokes C. The development and role of microbial-host interactions in gut mucosal immune development. J Anim Sci Biotechnol (2017) 8(1):1–10. doi: 10.1186/s40104-016-0138-0
126. Meresse B, Chen Z, Ciszewski C, Tretiakova M, Bhagat G, Krausz TN, et al. Coordinated induction by IL15 of a TCR-independent NKG2D signaling pathway converts CTL into lymphokine-activated killer cells in celiac disease. Immunity (2004) 21(3):357–66. doi: 10.1016/j.immuni.2004.06.020
127. Verneris MR, Karami M, Baker J, Jayaswal A, Negrin RS. Role of NKG2D signaling in the cytotoxicity of activated and expanded CD8+ T cells. Blood (2004) 103(8):3065–72. doi: 10.1182/blood-2003-06-2125
128. Jamieson AM, Diefenbach A, McMahon CW, Xiong N, Carlyle JR, Raulet DH. The role of the NKG2D immunoreceptor in immune cell activation and natural killing. Immunity (2002) 17(1):19–29. doi: 10.1016/S1074-7613(02)00333-3
129. Karimi M, Cao TM, Baker JA, Verneris MR, Soares L, Negrin RS. Silencing human NKG2D, DAP10, and DAP12 reduces cytotoxicity of activated CD8+ T cells and NK cells. J Immunol (2005) 175(12):7819–28. doi: 10.4049/jimmunol.175.12.7819
130. Borghetti P, De Angelis E, Saleri R, Cavalli V, Cacchioli A, Corradi A, et al. Peripheral T lymphocyte changes in neonatal piglets: relationship with growth hormone (GH), prolactin (PRL) and cortisol changes. Vet Immunol Immunopathol (2006) 110(1-2):17–25. doi: 10.1016/j.vetimm.2005.09.001
131. Stepanova H, Samankova P, Leva L, Sinkora J, Faldyna M. Early postnatal development of the immune system in piglets: the redistribution of T lymphocyte subsets. Cell Immunol (2007) 249(2):73–9. doi: 10.1016/j.cellimm.2007.11.007
132. Stepanova K, Sinkora M. The expression of CD25, CD11b, SWC1, SWC7, MHC-II, and family of CD45 molecules can be used to characterize different stages of γδ T lymphocytes in pigs. Dev Comp Immunol (2012) 36(4):728–40. doi: 10.1016/j.dci.2011.11.003
133. Yang H, Parkhouse R. Phenotypic classification of porcine lymphocyte subpopulations in blood and lymphoid tissues. Immunology (1996) 89(1):76–83. doi: 10.1046/j.1365-2567.1996.d01-705.x
134. Brandes M, Willimann K, Moser B. Professional antigen-presentation function by human γδ T cells. Science (2005) 309(5732):264–8. doi: 10.1126/science.1110267
135. Zhu Y, Wang H, Xu Y, Hu Y, Chen H, Cui L, et al. Human γδ T cells augment antigen presentation in listeria monocytogenes infection. Mol Med (2016) 22(1):737–46. doi: 10.2119/molmed.2015.00214
136. Wu Y, Wu W, Wong WM, Ward E, Thrasher AJ, Goldblatt D, et al. Human γδ T cells: a lymphoid lineage cell capable of professional phagocytosis. J Immunol (2009) 183(9):5622–9. doi: 10.4049/jimmunol.0901772
137. Takamatsu H-H, Denyer M, Wileman T. A sub-population of circulating porcine γδ T cells can act as professional antigen presenting cells. Vet Immunol Immunopathol (2002) 87(3-4):223–4. doi: 10.1016/S0165-2427(02)00083-1
138. Scharek-Tedin L, Pieper R, Vahjen W, Tedin K, Neumann K, Zentek J. Bacillus cereus var. toyoi modulates the immune reaction and reduces the occurrence of diarrhea in piglets challenged with salmonella typhimurium DT104. J Anim Sci (2013) 91(12):5696–704. doi: 10.2527/jas.2013-6382
139. Saalmüller A, Werner T, Fachinger V. T-Helper cells from naive to committed. Vet Immunol Immunopathol (2002) 87(3-4):137–45. doi: 10.1016/S0165-2427(02)00045-4
140. Summerfield A, Rziha H-J, Saalmüller A. Functional characterization of porcine CD4+ CD8+ extrathymic T lymphocytes. Cell Immunol (1996) 168(2):291–6. doi: 10.1006/cimm.1996.0078
141. Saalmüller A, Reddehase MJ, Bühring HJ, Jonjić S, Koszinowski UH. Simultaneous expression of CD4 and CD8 antigens by a substantial proportion of resting porcine T lymphocytes. Eur J Immunol (1987) 17(9):1297–301. doi: 10.1002/eji.1830170912
142. Pescovitz M, Sakopoulos AG, Gaddy JA, Husmann R, Zuckermann F. Porcine peripheral blood CD4+/CD8+ dual expressing T-cells. Vet Immunol Immunopathol (1994) 43(1-3):53–62. doi: 10.1016/0165-2427(94)90120-1
143. Revilla C, Chamorro S, Alvarez B, Perez C, Ezquerra A, Alonso F, et al. Analysis of functional heterogeneity of porcine memory CD4+ T cells. Dev Comp Immunol (2005) 29(5):479–88. doi: 10.1016/j.dci.2004.08.006
144. Ober BT, Summerfield A, Mattlinger C, Wiesmüller K-H, Jung GN, Pfaff E, et al. Vaccine-induced, pseudorabies virus-specific, extrathymic CD4+ CD8+ memory T-helper cells in swine. J Virol (1998) 72(6):4866–73. doi: 10.1128/JVI.72.6.4866-4873.1998
145. Zuckermann FA, Husmann R. Functional and phenotypic analysis of porcine peripheral blood CD4/CD8 double-positive T cells. Immunology (1996) 87(3):500.
146. De Bruin M, Van Rooij E, Voermans J, De Visser Y, Bianchi A, Kimman T. Establishment and characterization of porcine cytolytic cell lines and clones. Vet Immunol Immunopathol (1997) 59(3-4):337–47. doi: 10.1016/S0165-2427(97)00085-8
147. Kim S, Lim B, Mattoo S-u, Oh E-Y, Jeong C-G, Kim W-I, et al. Comprehensive transcriptomic comparison between porcine CD8– and CD8+ gamma delta T cells revealed distinct immune phenotype. Animals (2021) 11(8):2165. doi: 10.3390/ani11082165
148. Mair KH, Stadler M, Talker SC, Forberg H, Storset AK, Müllebner A, et al. Porcine CD3+ NKp46+ lymphocytes have NK-cell characteristics and are present in increased frequencies in the lungs of influenza-infected animals. Front Immunol (2016) 7:263. doi: 10.3389/fimmu.2016.00263
149. Sedlak C, Patzl M, Saalmüller A, Gerner W. CD2 and CD8α define porcine γδ T cells with distinct cytokine production profiles. Dev Comp Immunol (2014) 45(1):97–106. doi: 10.1016/j.dci.2014.02.008
150. Stepanova K, Sinkora M. Porcine γδ T lymphocytes can be categorized into two functionally and developmentally distinct subsets according to expression of CD2 and level of TCR. J Immunol (2013) 190(5):2111–20. doi: 10.4049/jimmunol.1202890
151. Yang H, Parkhouse R. Differential expression of CD8 epitopes amongst porcine CD8-positive functional lymphocyte subsets. Immunology (1997) 92(1):45–52. doi: 10.1046/j.1365-2567.1997.00308.x
152. Meininger I, Carrasco A, Rao A, Soini T, Kokkinou E, Mjösberg J. Tissue-specific features of innate lymphoid cells. Trends Immunol (2020) 41(10):902–17. doi: 10.1016/j.it.2020.08.009
153. Robinette ML, Fuchs A, Cortez VS, Lee JS, Wang Y, Durum SK, et al. Transcriptional programs define molecular characteristics of innate lymphoid cell classes and subsets. Nat Immunol (2015) 16(3):306–17. doi: 10.1038/ni.3094
154. Simoni Y, Fehlings M, Kløverpris HN, McGovern N, Koo S-L, Loh CY, et al. Human innate lymphoid cell subsets possess tissue-type based heterogeneity in phenotype and frequency. Immunity (2017) 46(1):148–61. doi: 10.1016/j.immuni.2016.11.005
155. Simoni Y, Newell EW. Toward meaningful definitions of innate-lymphoid-cell subsets. Immunity (2017) 46(5):760–1. doi: 10.1016/j.immuni.2017.04.026
156. Mair K, Sedlak C, Käser T, Pasternak A, Levast B, Gerner W, et al. The porcine innate immune system: an update. Dev Comp Immunol (2014) 45(2):321–43. doi: 10.1016/j.dci.2014.03.022
157. Saalmüller A, Hirt W, Maurer S, Weiland E. Discrimination between two subsets of porcine CD8+ cytolytic T lymphocytes by the expression of CD5 antigen. Immunology (1994) 81(4):578.
158. Annamalai T, Lu Z, Jung K, Langel SN, Tuggle CK, Dekkers JC, et al. Infectivity of GII. 4 human norovirus does not differ between TB-NK+ severe combined immunodeficiency (SCID) and non-SCID gnotobiotic pigs, implicating the role of NK cells in mediation of human norovirus infection. Virus Res (2019) 267:21–5. doi: 10.1016/j.virusres.2019.05.002
159. Annamalai T, Saif LJ, Lu Z, Jung K. Age-dependent variation in innate immune responses to porcine epidemic diarrhea virus infection in suckling versus weaned pigs. Vet Immunol Immunopathol (2015) 168(3-4):193–202. doi: 10.1016/j.vetimm.2015.09.006
160. Haverson K, Bailey M, Stokes C. T-Cell populations in the pig intestinal lamina propria: memory cells with unusual phenotypic characteristics. Immunology (1999) 96(1):66. doi: 10.1046/j.1365-2567.1999.00658.x
161. Sinkora M, Stepanova K, Butler JE, Francis D, Santiago-Mateo K, Potockova H, et al. Ileal peyer’s patches are not necessary for systemic b cell development and maintenance and do not contribute significantly to the overall b cell pool in swine. J Immunol (2011) 187(10):5150–61. doi: 10.4049/jimmunol.1101879
162. Shin H, Iwasaki A. Tissue-resident memory T cells. Immunol Rev (2013) 255(1):165–81. doi: 10.1111/imr.12087
163. Sasson S, Gordon C, Christo S, Klenerman P, Mackay L. Local heroes or villains: tissue-resident memory T cells in human health and disease. Cell Mol Immunol (2020) 17(2):113–22. doi: 10.1038/s41423-019-0359-1
164. Baker JR Jr., Farazuddin M, Wong PT, O’Konek JJ. The unfulfilled potential of mucosal immunization. J Allergy Clin Immunol (2022) 150(1):1–11. doi: 10.1016/j.jaci.2022.05.002
165. Rothkötter HJ, Hriesik C, Barman NN, Pabst R. B and also T lymphocytes migrate via gut lymph to all lymphoid organs and the gut wall, but only IgA+ cells accumulate in the lamina propria of the intestinal mucosa. Eur J Immunol (1999) 29(1):327–33. doi: 10.1002/(SICI)1521-4141(199901)29:01<327::AID-IMMU327>3.0.CO;2-K
166. Thielke KH, Hoffmann-Moujahid A, Weisser C, Waldkirch E, Pabst R, Holtmeier W, et al. Proliferating intestinal γ/δ T cells recirculate rapidly and are a major source of the γ/δ T cell pool in the peripheral blood. Eur J Immunol (2003) 33(6):1649–56. doi: 10.1002/eji.200323442
167. Wilson A, Stokes C, Bourne F. Morphology and functional characteristics of isolated porcine intraepithelial lymphocytes. Immunology (1986) 59(1):109.
168. Rothkötter H, Pabst R. Lymphocyte subsets in jejunal and ileal peyer’s patches of normal and gnotobiotic minipigs. Immunology (1989) 67(1):103.
169. Kansagra K, Stoll B, Rognerud C, Niinikoski H, Ou C-N, Harvey R, et al. Total parenteral nutrition adversely affects gut barrier function in neonatal piglets. Am J Physiol Gastrointest (2003) 285(6):G1162–G70. doi: 10.1152/ajpgi.00243.2003
170. Jing Y, Liu H, Xu W, Yang Q. 4, 4′-Diaponeurosporene-Producing bacillus subtilis promotes the development of the mucosal immune system of the piglet gut. Anat Rec (2019) 302(10):1800–7. doi: 10.1002/ar.24102
171. Schwarz E, Saalmüller A, Gerner W, Claus R. Intraepithelial but not lamina propria lymphocytes in the porcine gut are affected by dexamethasone treatment. Vet Immunol Immunopathol (2005) 105(1-2):125–39. doi: 10.1016/j.vetimm.2004.12.019
172. Barba-Vidal E, Castillejos L, Roll VF, Cifuentes-Orjuela G, Moreno Munoz JA, Martín-Orúe SM. The probiotic combination of bifidobacterium longum subsp. infantis CECT 7210 and bifidobacterium animalis subsp. lactis BPL6 reduces pathogen loads and improves gut health of weaned piglets orally challenged with salmonella typhimurium. Front Microbiol (2017) 8:1570. doi: 10.3389/fmicb.2017.01570
173. Hou G, Peng W, Wei L, Li R, Huang X, Yin Y. Probiotics and achyranthes bidentata polysaccharides improve growth performance via promoting intestinal nutrient utilization and enhancing immune function of weaned pigs. Animals (2021) 11(9):2617. doi: 10.3390/ani11092617
174. Scharek L, Altherr B, Tölke C, Schmidt M. Influence of the probiotic bacillus cereus var. toyoi on the intestinal immunity of piglets. Vet Immunol Immunopathol (2007) 120(3-4):136–47. doi: 10.1016/j.vetimm.2007.07.015
175. Herfel T, Jacobi S, Lin X, Van Heugten E, Fellner V, Odle J. Stabilized rice bran improves weaning pig performance via a prebiotic mechanism. J Anim Sci (2013) 91(2):907–13. doi: 10.2527/jas.2012-5287
176. Xiong X, Yang H, Wang X, Hu Q, Liu C, Wu X, et al. Effect of low dosage of chito-oligosaccharide supplementation on intestinal morphology, immune response, antioxidant capacity, and barrier function in weaned piglets. J Anim Sci (2015) 93(3):1089–97. doi: 10.2527/jas.2014-7851
177. Brown D, Maxwell C, Davis M, Erf G, Singh S. Effects of segregated early weaning on systemic and enteric T lymphocyte subpopulations in pigs. J Anim Sci (2002) 80:30–1.
178. Tang M, Laarveld B, Van Kessel A, Hamilton D, Estrada A, Patience J. Effect of segregated early weaning on postweaning small intestinal development in pigs. J Anim Sci (1999) 77(12):3191–200. doi: 10.2527/1999.77123191x
179. Castillo M, Martín-Orúe SM, Nofrarías M, Manzanilla EG, Gasa J. Changes in caecal microbiota and mucosal morphology of weaned pigs. Vet Microbiol (2007) 124(3-4):239–47. doi: 10.1016/j.vetmic.2007.04.026
180. García GR, Dogi CA, Ashworth GE, Berardo D, Godoy G, Cavaglieri LR, et al. Effect of breast feeding time on physiological, immunological and microbial parameters of weaned piglets in an intensive breeding farm. Vet Immunol Immunopathol (2016) 176:44–9. doi: 10.1016/j.vetimm.2016.02.009
181. Orgeur P, Hay M, Mormède P, Salmon H, Le Dividich J, Nowak R, et al. Behavioural, growth and immune consequences of early weaning in one-week-old Large-white piglets. Reprod Nutr Dev (2001) 41(4):321–32. doi: 10.1051/rnd:2001134
182. Dugan M, Knabe D, Wu G. Glutamine and glucose metabolism in intraepithelial lymphocytes from pre-and post-weaning pigs. Comp Biochem Physiol B Biochem (1994) 109(4):675–81. doi: 10.1016/0305-0491(94)90130-9
183. Wilson A, Stokes C, Bourne F. Responses of intraepithelial lymphocytes to T-cell mitogens: a comparison between murine and porcine responses. Immunology (1986) 58(4):621.
184. Maloy K, Mowat A, Zamoyska R, Crispe I. Phenotypic heterogeneity of intraepithelial T lymphocytes from mouse small intestine. Immunology (1991) 72(4):555.
185. Manzano M, Abadiía-Molina AC, Garciía-Olivares E, Gil A, Rueda R. Absolute counts and distribution of lymphocyte subsets in small intestine of BALB/c mice change during weaning. J Nutr (2002) 132(9):2757–62. doi: 10.1093/jn/132.9.2757
186. Ter Steege JC, Buurman WA, Forget P-P. The neonatal development of intraepithelial and lamina propria lymphocytes in the murine small intestine. Dev Immunol (1997) 5(2):121–8. doi: 10.1155/1997/34891
187. Umesaki Y, Setoyama H, Matsumoto S, Okada Y. Expansion of alpha beta T-cell receptor-bearing intestinal intraepithelial lymphocytes after microbial colonization in germ-free mice and its independence from thymus. Immunology (1993) 79(1):32.
188. Aluthge ND, Tom WA, Bartenslager AC, Burkey TE, Miller PS, Heath KD, et al. Differential longitudinal establishment of human fecal bacterial communities in germ-free porcine and murine models. Commun Biol (2020) 3(1):1–14. doi: 10.1038/s42003-020-01477-0
189. Gonzalez LM, Moeser AJ, Blikslager AT. Porcine models of digestive disease: the future of large animal translational research. Transl Res (2015) 166(1):12–27. doi: 10.1016/j.trsl.2015.01.004
190. Käser T. Swine as biomedical animal model for T-cell research–success and potential for transmittable and non-transmittable human diseases. Mol Immunol (2021) 135:95–115. doi: 10.1016/j.molimm.2021.04.004
191. Lunney JK, Van Goor A, Walker KE, Hailstock T, Franklin J, Dai C. Importance of the pig as a human biomedical model. Sci Transl Med (2021) 13(621):eabd5758. doi: 10.1126/scitranslmed.abd5758
192. Rose EC, Blikslager AT, Ziegler AL. Porcine models of the intestinal microbiota: the translational key to understanding how gut commensals contribute to gastrointestinal disease. Front Vet Sci (2022) 9:834598. doi: 10.3389/fvets.2022.834598
193. Roura E, Koopmans S-J, Lallès J-P, Le Huerou-Luron I, de Jager N, Schuurman T, et al. Critical review evaluating the pig as a model for human nutritional physiology. Nutr Res Rev (2016) 29(1):60–90. doi: 10.1017/S0954422416000020
194. Ziegler A, Gonzalez L, Blikslager A. Large Animal models: the key to translational discovery in digestive disease research. Cell Mol Gastroenterol (2016) 2(6):716–24. doi: 10.1016/j.jcmgh.2016.09.003
195. Beagley KW, Fujihashi K, Lagoo AS, Lagoo-Deenadaylan S, Black CA, Murray AM, et al. Differences in intraepithelial lymphocyte T cell subsets isolated from murine small versus large intestine. J Immunol (1995) 154(11):5611–9.
196. Boll G, Rudolphi A, Spieβ S, Reimann J. Regional specialization of intraepithelial T cells in the murine small and large intestine. Scand J Immunol (1995) 41(2):103–13. doi: 10.1111/j.1365-3083.1995.tb03541.x
197. Camerini V, Panwala C, Kronenberg M. Regional specialization of the mucosal immune system. intraepithelial lymphocytes of the large intestine have a different phenotype and function than those of the small intestine. J Immunol (1993) 151(4):1765–76.
198. Tang W, Zhong Y, Wei Y, Deng Z, Mao J, Liu J, et al. Ileum tissue single-cell mRNA sequencing elucidates the cellular architecture of pathophysiological changes associated with weaning in piglets. BMC Biol (2022) 20(1):1–24. doi: 10.1186/s12915-022-01321-3
199. Li H, Wang X, Wang Y, Zhang M, Hong F, Wang H, et al. Cross-species single-cell transcriptomic analysis reveals divergence of cell composition and functions in mammalian ileum epithelium. Cell Regener (2022) 11(1):1–16. doi: 10.1186/s13619-022-00118-7
200. Wiarda JE, Becker SR, Sivasankaran SK, Loving CL. Regional epithelial cell diversity in the small intestine of pigs. J Anim Sci (2022), skac318. doi: 10.1093/jas/skac318
201. Jarry A, Cerf-bensussan N, Brousse N, Selz F, Guy-grand D. Subsets of CD3+ (T cell receptor α/β or γ/δ) and CD3– lymphocytes isolated from normal human gut epithelium display phenotypical features different from their counterparts in peripheral blood. Eur J Immunol (1990) 20(5):1097–103. doi: 10.1002/eji.1830200523
202. Kaczorowski KJ, Shekhar K, Nkulikiyimfura D, Dekker CL, Maecker H, Davis MM, et al. Continuous immunotypes describe human immune variation and predict diverse responses. Proc Natl Acad Sci USA (2017) 114(30):E6097–E106. doi: 10.1073/pnas.1705065114
203. Glastonbury CA, Alves AC, Moustafa JSE-S, Small KS. Cell-type heterogeneity in adipose tissue is associated with complex traits and reveals disease-relevant cell-specific eQTLs. Am J Hum Genet (2019) 104(6):1013–24. doi: 10.1016/j.ajhg.2019.03.025
204. Naoun AA, Raphael I, Forsthuber TG. Immunoregulation via cell density and quorum sensing-like mechanisms: an underexplored emerging field with potential translational implications. Cells (2022) 11(15):2442. doi: 10.3390/cells11152442
205. Antonioli L, Blandizzi C, Pacher P, Guilliams M, Haskó G. Quorum sensing in the immune system. Nat Rev Immunol (2018) 18(9):537–8. doi: 10.1038/s41577-018-0040-4
206. Kennedy RB, Simon WL, Gibson MJ, Goergen KM, Grill DE, Oberg AL, et al. The composition of immune cells serves as a predictor of adaptive immunity in a cohort of 50-to 74-year-old adults. Immunology (2016) 148(3):266–75. doi: 10.1111/imm.12599
207. Bardou M, Postat J, Loaec C, Lemaître F, Ronteix G, Garcia Z, et al. Quorum sensing governs collective dendritic cell activation in vivo. EMBO J (2021) 40(15):e107176. doi: 10.15252/embj.2020107176
Keywords: porcine, biomedical model, intestinal lymphocyte, swine, epithelial barrier, intestinal epithelium, T lymphocyte, innate lymphoid cell 1
Citation: Wiarda JE and Loving CL (2022) Intraepithelial lymphocytes in the pig intestine: T cell and innate lymphoid cell contributions to intestinal barrier immunity. Front. Immunol. 13:1048708. doi: 10.3389/fimmu.2022.1048708
Received: 19 September 2022; Accepted: 25 November 2022;
Published: 08 December 2022.
Edited by:
Noemi Sevilla, Centro de Investigación en Sanidad Animal (CISA)-INIA-CSIC, SpainReviewed by:
Tobias Kaeser, North Carolina State University, United StatesWilhelm Gerner, The Pirbright Institute, United Kingdom
Copyright © 2022 Wiarda and Loving. This is an open-access article distributed under the terms of the Creative Commons Attribution License (CC BY). The use, distribution or reproduction in other forums is permitted, provided the original author(s) and the copyright owner(s) are credited and that the original publication in this journal is cited, in accordance with accepted academic practice. No use, distribution or reproduction is permitted which does not comply with these terms.
*Correspondence: Crystal L. Loving, Y3J5c3RhbC5sb3ZpbmdAdXNkYS5nb3Y=