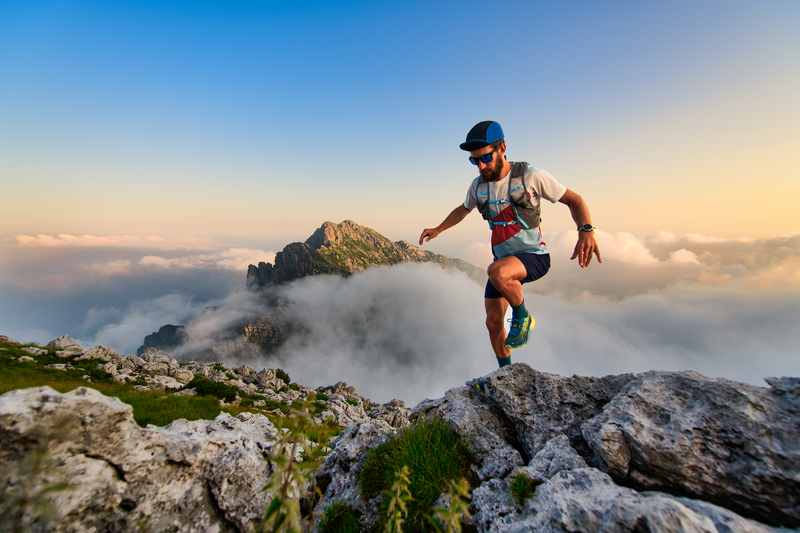
95% of researchers rate our articles as excellent or good
Learn more about the work of our research integrity team to safeguard the quality of each article we publish.
Find out more
REVIEW article
Front. Immunol. , 20 October 2022
Sec. Cytokines and Soluble Mediators in Immunity
Volume 13 - 2022 | https://doi.org/10.3389/fimmu.2022.1042983
This article is part of the Research Topic Role of Stem Cell Derivatives in Inflammatory Diseases View all 13 articles
Organ fibrosis is a serious health challenge worldwide, and its global incidence and medical burden are increasing dramatically each year. Fibrosis can occur in nearly all major organs and ultimately lead to organ dysfunction. However, current clinical treatments cannot slow or reverse the progression of fibrosis to end-stage organ failure, and thus advanced anti-fibrotic therapeutics are urgently needed. As a type of naturally derived nanovesicle, native extracellular vesicles (EVs) from multiple cell types (e.g., stem cells, immune cells, and tissue cells) have been shown to alleviate organ fibrosis in many preclinical models through multiple effective mechanisms, such as anti-inflammation, pro-angiogenesis, inactivation of myofibroblasts, and fibrinolysis of ECM components. Moreover, the therapeutic potency of native EVs can be further enhanced by multiple engineering strategies, such as genetic modifications, preconditionings, therapeutic reagent-loadings, and combination with functional biomaterials. In this review, we briefly introduce the pathology and current clinical treatments of organ fibrosis, discuss EV biology and production strategies, and particularly focus on important studies using native or engineered EVs as interventions to attenuate tissue fibrosis. This review provides insights into the development and translation of EV-based nanotherapies into clinical applications in the future.
Organ fibrosis is a serious and unsolved health problem worldwide. The main pathological feature of fibrosis is the abnormal formation and deposition of excessive extracellular matrix (ECM), which eventually results in disrupted architectural remodeling and progressive organ dysfunction. Fibrosis can occur in nearly all major organs (Figure 1), such as liver, heart, lung, and kidney, and it is attributed to ~45% of all deaths in the world (1). For example, more than 800 million patients were affected by chronic liver disease (liver fibrosis); about 1.5% of global deaths were caused by chronic kidney disease (CKD, renal fibrosis) in 2012 (2, 3). The mechanism of fibrosis is extremely complicated, and many pathological factors, such as infections, immune reactions, chemical insults, oxidative stress, and hazardous agents, have been proved to be involved with fibrosis (4). In recent decades, many single factor-targeted treatments have been developed and have shown certain beneficial effects in preclinical studies, but most of them fail to achieve clinical approval. Thus, novel and advanced antifibrotic therapeutics are desired in the clinic (5).
Figure 1 Pathological causes of organ fibrosis. Fibrosis can occur in most organs or tissues, such as the heart, liver, lung, kidney, tendon, intrauterine and intervertebral disc, due to complicated pathological causes.
In recent years, extracellular vesicle (EV)-based therapies have emerged as a potent strategy for resolving organ fibrosis. EVs are nanoscale bilayer vesicles secreted by live cells that exert similar functions as parental cells by delivering various types of cargoes, such as lipids, proteins, nucleic acids, and metabolites (6). As a type of naturally derived nanomaterial, EVs have multiple advantages, such as abundant cell sources, intrinsic bioactive properties, low immunogenicity, rare toxicity, flexibility to modify, and ability to cross biological barriers compared to synthetic materials (7). In many preclinical studies, EV-based nanomedicines have been shown to prevent multiple types of organ fibrosis through complicated mechanisms, such as promoting the recovery of damaged tissues, resolution of inflammation, inactivation of myofibroblasts, and fibrinolysis of excess ECM components (5). Although the results are encouraging, some important problems, such as proper cell sources, EV engineering strategies, detailed mechanisms, and possible limitations, remain elusive and need to be comprehensively reviewed. This will be helpful for the improvement and clinical translation of EV-based antifibrotic therapies.
In this review, we briefly introduce the key pathology of organ fibrosis as well as EV biology and production and emphasize the important studies that are highly relevant to using native or engineered EVs for decreasing organ fibrosis. We also discuss the possible limitations in this field and provide insights into developing advanced EV therapeutics for the treatment of fibrotic diseases.
Fibrosis, characterized by the activation of myofibroblasts, excessive deposition of ECM, and inhibition of ECM degradation, is a common adverse outcome of many etiological conditions after acute or chronic organ injury (8, 9). A variety of cell types and signaling pathways synergistically regulate the occurrence and progression of organ fibrosis (Figure 2). In this section, we briefly review the critical cellular events, signaling pathways and current clinical strategies for fibrotic disease treatment.
Figure 2 (A) Key cellular events of organ fibrosis. Organ damage can trigger infiltration of immune cells, followed by secretion of excessive cytokines/chemokines to activate myofibroblasts. Myofibroblasts are originated from many cell types, such as epithelial cells, mesothelial cells and endothelial cells, through EMT, MMT or EndoMT. Activated myofibroblasts promote organ ECM synthesis/deposition. (B) Key signaling pathway of organ fibrosis. TGF-β participates in the molecular mechanism of fibrosis in Smad-dependent and Smad-independent manner such as MAPK families. Moreover, TGF-β also interacts with other profibrotic pathways, such as the Wnt/β-catenin, Hedgehog and BMP-7 pathways. (TGF-β, transforming growth factor-β; ILs, interleukins; PDGF, platelet-derived growth factor; EMT, epithelial to mesenchymal transition; MMT, mesothelial to mesenchymal transition; EndoMT, endothelial to mesenchymal transition; MMP, matrix metalloproteinase; TIMPs, tissue inhibitors of matrix metalloproteinases; PA, plasminogen activator; PAI, plasminogen activator-inhibitor 1; MAPK, mitogen-activated protein kinase; JNK, JUN amino-terminal kinase; ERK, extracellular signal-regulated kinase; GSK-3β, glycogen synthase kinase-3β; FN, fibronectin).
In the early phases after organ injury, persistent stress may induce parenchymal cell death (e.g., necrosis, pyroptosis, and ferroptosis) and trigger abnormal activation and infiltration of multiple types of immune cells (e.g., macrophages) and inflammation (10). Subsequently, cytokines and chemokines secreted by these infiltrated immune cells, such as transforming growth factor-β (TGF-β), interleukins (ILs), and platelet-derived growth factor (PDGF), further amplify the severity of inflammation and organ damage (11). The activation of myofibroblasts is the central event mediating ECM synthesis and deposition in the later phases after organ injury. Activated myofibroblasts can be identified by several marker proteins, such as α-smooth muscle actin (α-SMA) and PDGFβR (12). However, the origins of myofibroblasts remain incompletely understood, but multiple cell sources have been reported (Figure 2A), such as resident fibroblasts, mesothelial cells, circulating fibroblasts, epithelial cells, endothelial cells, pericytes, vascular smooth muscle cells, and other specialized tissue cells (e.g., hepatic stellate cells). Additionally, many possible mechanisms, such as cell proliferation, epithelial to mesenchymal transition (EMT), mesothelial to mesenchymal transition (MMT) and endothelial to mesenchymal transition (EndoMT), are involved in this process (5, 13).
The imbalance between ECM deposition and ECM degradation is another vital event during fibrosis. Briefly, the degradation of ECM proteins (e.g., collagens) is mainly controlled by matrix metalloproteinase (MMP)-mediated proteolysis but is antagonized by multiple tissue inhibitors of matrix metalloproteinases (TIMPs), which are the endogenous inhibitors of MMPs (14, 15). MMPs can be classified into different subtypes, such as interstitial collagenases, gelatinases, metalloelastases and membrane-type MMPs, according to their enzyme-substrate specificity and subcellular locations (16). For example, interstitial collagenases (e.g., MMP-1, MMP-13, and MMP-18) can proteolyze type I, II, III interstitial collagens, while gelatinases (e.g., MMP-2 and MMP-9) can cleave the denatured collagens and basement membrane ECM (16, 17). Conversely, TIMPs can blunt ECM proteolysis by inhibiting the activity of MMPs, and the upregulation of TIMPs is commonly associated with an accumulation of ECM (18). In addition, the dysregulation of serine proteases and their inhibitors, such as urokinase plasminogen activator (uPA) and plasminogen activator-inhibitor 1 (PAI-1), is also associated with abnormal ECM remodeling and fibrosis (14).
Although many possible pathways have been revealed in the pathogenesis of organ fibrosis in recent decades, TGF-β has been recognized as a master regulator of myofibroblast activation and fibrotic processes (Figure 2B). TGF-β has three isoforms (TGFβ1-3) and can stimulate the biosynthesis and accumulation of ECM components, such as collagen I and fibronectin (FN), in epithelial cells and mesenchymal cells (19, 20). TGF-β1 can be secreted by many types of cells, such as macrophages, lymphocytes, epithelial cells, fibroblasts, pericytes, endothelial cells and platelets, at injured tissue sites (19). Once activated after secretion, TGF-β1 binds to its receptor (TGF-βR) and activates downstream profibrotic pathways in a Smad-dependent (classical) or Smad-independent (nonclassical) manner (21). In the Smad-dependent pathway, TGF-β1 induces phosphorylated Smad2/3 proteins, and then Smad4 binds to the Smad2/3 complex and translocates the complex into the nucleus to induce the transcription of many essential profibrotic genes, such as collagens, FN and PAI-1 (9). Smad7 is a negative regulator of this process, which can compete with Smad2/3 for binding to activated TGF-βR and thus block TGF-β/Smad signaling (22). Moreover, TGF-β1 can also interact with other Smad-independent pathways, such as the mitogen-activated protein kinase (MAPK) family p38 MAPK, JUN amino-terminal kinase (JNK) and extracellular signal-regulated kinase (ERK) pathways. MAPKs further phosphorylate the linker region of Smad2/3 and thus modulate Smad3 transcriptional activity (9).
Furthermore, TGF-β has potential crosstalks with other profibrotic pathways, such as the Wnt/β-catenin, Jagged1/Notch, Hedgehog, and bone morphogenic protein-7 (BMP-7) pathways (23). For example, TGF-β activates Wnt signaling by inhibiting glycogen synthase kinase-3β (GSK-3β), thereby disrupting the stabilization of β-catenin or suppressing Wnt inhibitors and enhancing the transcription of β-catenin-targeted profibrotic genes (e.g., FN, PAI-1, Snail and MMP-7) in injured kidneys (24, 25). TGF-β can also induce the transcription of GLI2 (an activator of Hedgehog signaling), which subsequently upregulates Hedgehog-targeted profibrotic gene (e.g., α-SMA) expression (26–28). In contrast, BMP-7 activates Smad1/5/8, which can block the nuclear translocation of phosphorylated receptor-Smad2/3 and thus inhibit TGF-β signaling (29). Due to the complicated mechanisms of organ fibrosis, it can be speculated that single pathway-targeted antifibrotic strategies may not be efficient.
Since fibrosis is a long-term outcome of persistent organ damage, it can be assumed that the resolution of fibrosis may be achieved when the pathological causes of injuries are eliminated. Based on previous studies of fibrogenesis, several therapeutic strategies (Table 1) are proposed (30), such as immunoregulation, degradation of ECM and elimination of myofibroblasts. Currently, some potential targets or medicines for fibrosis in different organs have been reported, and some of them have progressed to clinical trials or clinical phases (Table 1). For example, pirfenidone and nintedanib (NIN), two compounds with pleiotropic mechanisms of action, are approved for the management of idiopathic pulmonary fibrosis (IPF) due to their effects on slowing lung functional decline. Pirfenidone may inhibit TGF-β, inflammatory cytokines (e.g., tumor necrosis factor-α, TNF-α) or oxidative stress, and NIN may inhibit tyrosine kinase receptors such as PDGFR, vascular endothelial growth factor receptor (VEGFR) and fibroblast growth factor receptor (FGFR) (31). However, the two drugs cannot prevent or reverse the existing organ fibrosis according to physiological, histological, and radiological examination results (31). Thus, more advanced antifibrotic treatments are urgently required in the clinic.
EVs are a group of nanoscale bilayer vesicles and are widely distributed in the cultured medium of almost all cell types and biofluids, such as blood, urine, saliva, and breast milk (32). Numerous evidence indicates that EVs are key mediators of cell-to-cell or organ-to-organ communication since they can deliver multiple types of bioactive cargoes to regulate the signaling of the recipient cells under physiological or pathophysiological conditions (33). In this section, we briefly introduce the biological properties of EVs, such as biogenesis, uptake, production, and stability.
The current classification of EVs is mainly based on their cellular origin and/or biological function. According to their sizes or biogenesis routes, EVs can be divided into many subtypes, such as exosomes (~30-200 nm), microvesicles (MVs, ~200-1000 nm) and apoptotic bodies (~800-5000 nm) (34, 35). Among these EV subtypes, exosomes and MVs are frequently reported in most of the previous studies related to fibrosis. Thus, we will focus on these two types of EVs in the following sections (Figure 3). Briefly, the first step of exosome biogenesis is that the plasma membrane is endocytosed to form early endosomes, which then mature into late endosomes, also known as multivesicular bodies (MVBs). The delimiting membrane of MVBs can be invaginated to form intraluminal vesicles (ILVs), followed by the endosomal sorting complex required for transport (ESCRT)-dependent or ESCRT-independent (tetraspanins related) steps. In the ESCRT-dependent process, ESCRT-0 binds to the ubiquitination sites of early endosomes, ESCRT-I and ESCRT-II induce the formation of MVBs, and ESCRT-III promotes intraluminal budding of endosomal vesicles and scissors ILVs into the MVB lumen. The last step of exosome formation is the binding of some MVBs to the cell membrane to release ILVs, whereas other ILVs binds to lysosomes and are degraded (36). Unlike exosomes, MVs are mainly originated by outward budding at the cell plasma membrane, which is regulated by several rearrangements within the plasma membrane, such as altered lipid/protein components and Ca2+ levels. Although exosomes and MVs may have different biogenesis routes, they still share some common pathways, such as ESCRT and the conversion of ceramide from sphingomyelinas (37). However, the detailed mechanisms of other EV subpopulations remain elusive and need to be explored in future studies.
Figure 3 Biogenesis and uptake of EVs. During exosome biogenesis, the plasma membrane is endocytosed to form early endosomes and then matures into MVBs. The delimiting membrane of MVBs invaginates from the ILVs. Finally, some MVBs bind to the cell membrane to release ILVs. MVs are mainly originated by outward budding at the cell plasma membrane. For cellular uptake, EVs can be taken up by recipient cells via direct membrane fusion or endocytosis by interacting with receptors on the surface. (Extracellular vesicles: EVs, microvesicles: MVs, multivesicular body: MVB, intraluminal vesicles: ILV, microRNA: miRNAs, long noncoding RNA: lncRNA, mitochondrial DNA: mtDNA).
EVs can participate in intracellular communication by delivering multiple types of bioactive contents into recipient cells (Figure 3). EVs are enriched in lipid contents, such as cholesterol, sphingomyelin, and hexosylceramides (38), which contribute to the in vivo stability of EVs. Due to their biogenesis routes, EVs carry many proteins originated from the cell plasma membrane, cytoskeleton and cytoplasm, as well as other proteins involved in EV sorting and secretion, such as tetraspanins and proteins from the ESCRT-dependent pathway (39). In addition, EVs also contain large amounts of RNAs and DNAs, among which microRNAs (miRNAs) are the most abundantly studied to explain the effective mechanisms of EVs. Other types of RNAs, such as mRNAs, long noncoding RNAs (lncRNAs), tRNAs, Y-RNAs and ribosomal RNAs, have also been observed in EVs (40, 41). Interestingly, we and others had found the appearance of mitochondrial DNA (mtDNA), electron transport chain proteins and even fragmented mitochondria in EVs (42).
As a result, the composition of proteins and lipids on the surface of EVs may influence the efficiency of cell uptake (Figure 3). In brief, EVs can be taken up by target cells via direct membrane fusion or endocytosis, which has been well reviewed (43). Direct fusion relies on the lipid composition of EVs and target cells, and the fusion efficacy of EVs to the plasma membrane may be enhanced by acidic pH in the extracellular environment (44). In the case of endocytosis, EVs are docked by interacting with proteins, glycoproteins or lipids exposed on the cell membrane and then internalized by recipient cells (45). Overall, the surface signature of EVs can influence the pattern of EV uptake in target cells.
EVs can be isolated from various types of samples (e.g., conditioned medium (CM) and body fluids) using different methods, such as ultracentrifugation (UC), density gradient ultracentrifugation, size-exclusive chromatography (SEC), immunoaffinity, ultrafiltration, coprecipitation (polyethylene glycol-based) and newly developed microfluidics (46), and each method has its advantages and shortcomings. For example, UC, including differential centrifugation and sucrose density gradient ultracentrifugation, remains the most widely used method which relies on the size and/or density of EVs. However, the production efficiency of UC is limited by long time consumption, operator dependence and rupture of EVs (47, 48). Methods based on EV size, such as SEC, can improve the purity and stability of EVs, while EV yield is limited (49, 50). The immunoaffinity method can selectively enrich EVs with high purity, but it is unsuitable for large volume samples due to its high cost (51). For future clinical applications, a large amount of therapeutic EVs with high purity is needed, and thus, a major issue in this field is how to overcome the current problems of high cost and low yield for larger-scale production of EVs.
According to the minimal information for studies of extracellular vesicles (MISEV) guidelines, isolated EVs should be characterized at least by morphology, particle size and marker proteins (52, 53). The morphology of EVs is commonly observed using transmission electron microscopy or scanning electron microscopy (53). The particle sizes and numbers of EVs can be measured using nanoparticle analysis technology or dynamic light scattering (54, 55). Some key proteins involved in EV biogenesis or function, such as tetraspanins (e.g., CD9, CD63, CD81, CD82) or Alix, heat shock protein70 (HSP70), TSG101, and syntenin-1 associated with ESCRT, have been proposed as common EV markers (56, 57). However, the truth is that there are no specific markers for the identification of all EV subtypes until now (39). More importantly, for therapeutic purposes, the possible biosafety issues of EVs should be carefully considered, since it has been reported that some EVs from immortalized cell lines may carry oncogenic materials or endotoxins, while some EVs from infected cells may carry viral-derived proteins (34, 58). Thus, it is necessary to establish standardized and reproductive operating procedures, quality control criteria, and strictly sterile conditions during EV production (59).
For future clinical translation, a good manufacturing practice (GMP)-compliant protocol for EV production should be developed. The manufacturing of EV products can be briefly divided into upstream and downstream processings and subsequent quality control (60). In upstream processing, the large-scale expansion of EV-producing cells mainly depends on bioreactors, such as hollow fiber and stirred suspension bioreactors (using microcarriers) (61). Hollow fibers culture the cells in the hollow and semipermeable fibers and has less risk of contamination, while microcarriers are tiny beads with many varying features and require changing of culture media frequently (62). A recent study reported a turbulence strategy integrated into the cell culture in a stirred tank incompatible with a GMP bioreactor, which could obtain ~1013 EV particles from the supernatant of the one-liter bioreactor (63). In downstream processing, traditional isolation methods are not suitable for large-volume samples. Among the different methods, tangential flow filtration (TFF) may be one of the most powerful tools for industrial-scale EV manufacture (64) since it has a superior yield and bioactivity of isolated EVs compared to UC (65). Furthermore, TFF can be combined with other isolation approaches, such as SEC, to achieve higher scalability and reproducibility (66).
Stability is an important parameter for biological reagents, and the lipid bilayer structure of EVs provides unique protection to their bioactive cargoes (67). Some storage techniques that can long-term preserve EV bioactivities, such as freezing, lyophilization and spray-drying, have also been developed (68). For example, EVs suspended in buffered saline solution are stable for up to 2 years at -80°C without significant changes in properties (69), and the bioactivities of lyophilized EVs are similar to those of frozen EVs even stored at room temperature for 4 weeks (70). Therefore, the stability of EVs makes them ideal therapeutics in future clinical applications.
In recent decades, increasing numbers of studies have reported the potential of native EVs in preventing fibrosis via multiple mechanisms (Supplementary Table 1). Here, we briefly introduce the important studies regarding the anti-fibrotic effects (in major organs) of native EVs from various cell sources, such as stem cells, immune cells, tissue cells and blood (Figure 4).
Figure 4 Therapeutic potentials of native EVs. Native EVs isolated from multiple cell sources (e.g., stem cells, immune cells, tissue cells, and plasma) have exerted therapeutic efficacy on the alleviation of fibrosis in some major organs (e.g., heart, lung, liver, and kidney) and other tissues (e.g., IUA, IDD, and urethral stricture), and their therapeutic role may be due to multiple mechanisms, such as anti-inflammation, EMT inactivation, ECM degradation, and pro-angiogenesis. (Mesenchymal stem cells, MSCs; iPSCs, induced pluripotent stem cells; natural killer, NK; bronchial epithelial cell, BECs; tubular epithelial cell, TECs; epithelial to mesenchymal transition, EMT; extracellular matrix, ECM; intrauterine adhesion, IUA; intervertebral disc degeneration, IDD).
Cardiovascular disease (CVD) is one of the leading causes of death globally, and more than 17 million deaths worldwide are due to CVD per year (109, 110). Cardiac fibrosis can occur after almost all types of heart diseases, such as myocardial infarction (MI), hypertrophic cardiomyopathy (HCM), dilated cardiomyopathy (DCM), aortic stenosis (AOS), and diabetic cardiomyopathy (111, 112). Although some potential medicines, such as angiotensin (AT)-converting enzyme and angiotensin II receptor antagonists, β-blockers, endothelin antagonists, and statins have been used in the clinic to protect cardiac function, their direct antifibrotic efficacy remains debated (113, 114). Thus, new and advanced antifibrotic therapies are urgently needed.
Increasing evidence shows that native EVs isolated from stem cells may have the potential to prevent cardiac fibrosis (Figure 4). Mesenchymal stem cells (MSCs) are a type of adult stem cell with immunoregulatory and tissue repair potentials that can be isolated from multiple tissue types, such as bone marrow (BMSCs), umbilical cord tissue (ucMSCs) and adipose tissue (ADMSCs) (115). The possible antifibrotic effects of MSC-derived EVs (MSC-EVs) have been reported in multiple models of cardiac damage (such as hypertension and MI), and the underlying protective mechanism is linked to the inhibition of EMT (miR-200, etc.), anti-apoptosis, induction of M2 macrophages or pro-angiogenesis (Supplementary Table 1) (71, 72, 116, 117). Induced pluripotent stem cells (iPSCs) are a new source of embryonic-like stem cells obtained by reprogramming of somatic cells (e.g., skin fibroblasts), which can further differentiate into many different cell types. iPSC-derived EVs reduced ECM deposition in the aortas of aged mice by inhibiting MMPs and elastase activity while enhancing the endothelial nitric oxide synthase (eNOS) pathway (73). Another therapeutic strategy is using EVs produced by iPSC-derived cardiac cells. For instance, iPSCs were first differentiated into contractile cardiomyocytes (iCMs), then iCM-derived EVs reduced the fibrotic areas of the left ventricle (LV) by enhancing autophagic flux after ischemic heart injury (74). EVs isolated from human embryonic stem cell (ESC)-derived cardiovascular progenitor cells recovered heart function (LV ejection fraction values/LV systolic dimensions) and reduced fibrotic areas after MI by promoting angiogenesis and inhibiting cardiomyocyte death (75).
In addition, native EVs from heart tissue cells also displayed certain antifibrotic effects in cardiac injury models (Figure 4; Supplementary Table 1) (118). The cardiosphere is a cluster of endogenous cardiac stem cells that forms when they are cloned in suspension, and cardiosphere-derived cells (CDCs) have been shown to have regenerative potential in cardiac injuries such as MI (119). CDC-EV treatment was shown to reduce the levels of cardiac hypertrophy, inflammation, and interstitial cardiac fibrosis in an Ang II-induced hypertension model by delivering Y RNA fragments to induce IL-10 expression (76). DCM is a serious pediatric cardiomyopathy, and ~50~60% of children with DCM will die in 5 years (120). In a swine model of DCM, CDC-EV treatment decreased myocardial fibrosis by shedding proangiogenic and cardioprotective miR-146a-5p to suppress inflammation (77). Regeneration-associated cells (RACs) are a group of heterogeneous cells (e.g., endothelial progenitor cells, M2 macrophages and regulatory T cells) under vasculogenic conditions after heart injury (121). RACs-derived EVs could reduce interstitial fibrosis after myocardial ischemic injury by shedding functional miRNAs (e.g., miR-150-5p, miR-195 and miR-142-3p) to promote angiogenesis and cardiomyogenesis while reducing the inflammatory response (78). Altogether, these findings indicate that native EVs from multiple cell types may mitigate cardiac fibrosis via immunological regulation, pro-angiogenesis or suppression of cell death.
Chronic lung diseases are highly associated with progressive lung fibrosis, resulting in poor quality of life and high mortality of patients (122). For example, IPF, an aggressive lung disease with an uncertain cause, has a poor prognosis with a median survival time of ~2-5 years after diagnosis (123) and ∼3 million patients worldwide suffer from IPF (124). However, the therapeutic efficacies of current treatments are not ideal in the clinic (125). For example, IPF patients receiving pirfenidone still have a high mortality rate and uncertain survival times (126). Therefore, advanced treatments for the resolution of lung fibrosis are also needed.
Native EVs from stem cells (e.g., MSCs) have shown therapeutic potency in preclinical models of lung injury, and immunoregulation may be one of the main mechanisms involved (Supplementary Table 1). For example, BMSC-EV treatment attenuated profibrotic factor (TGF-β1, α-SMA, collagen I/III) expressions as well as fibrotic areas after lipopolysaccharide-induced acute lung injury, and this effect may be due to the inhibitory role of miRNAs (miR-23a-3p and miR-182-5p) on the NF-κB/Hedgehog pathways (79). In a silicosis model, ADMSC-EV intervention reduced the collagen contents and F4/80+ macrophage numbers and suppressed NF-κB/TLR activation in lungs by delivering functional miRNAs (e.g., miR-146b) (80). In addition, the direct impact of MSC-EVs on profibrotic pathways had been reported. In a bleomycin (BLM)-induced IPF model, hucMSC-EV treatment inhibited myofibroblast differentiation and collagen deposition by delivering miR-21-5p/miR-23-3p to suppress the TGF-β pathway (81). BMSC-EV treatments also ameliorated fibroblast activation and α-SMA/collagen I expression in a BLM model, and miR-186 of EVs may inhibit SRY-related HMG box transcription factor 4 (SOX4), a regulator of lung development and monocyte infiltration (82, 83).
In addition, EVs from healthy lung tissue cells may be a possible therapy for the treatment of lung fibrosis (Supplementary Table 1). Airway epithelial cells (AECs) play regulatory roles in the development of lung fibrosis, and damaged bronchial epithelial cell (HBEC)-derived EVs can induce myofibroblast differentiation associated with airway remodeling (127). However, healthy AEC-EVs may have the opposite anti‐fibrotic effects. A recent study found that EVs from healthy HBECs could reduce TGF-β-induced myofibroblast differentiation in vitro, and intratracheal administration of these EVs promoted collagen degradation in BLM-induced IPF by inhibiting the crosstalks between the TGF-β and Wnt pathways (84). Lung spheroid cells (LSCs), an intrinsic source of lung stem cells, have been shown to mitigate fibrosis development in a rat BLM model (128). Similarly, human LSC-derived EVs (hLSC-EVs) also inhibited myofibroblast proliferation and collagen production by shedding miRNAs (miR-30a, miR-99 and let-7), and the inhalation of these EVs decreased BLM- or silica-induced rat lung fibrosis (85), suggesting that inhalation of EVs is a promising route for treating lung fibrosis. Altogether, these reports suggest that stem cells and healthy lung tissue cells are potential EV sources for mitigating lung fibrosis with inflammation and myofibroblasts as the possible targets.
Chronic liver diseases are also a major health issue globally, with more than 800 million patients affected and ~2 million deaths per year (2). Liver fibrosis can be triggered by many causes, such as alcohol, nonalcoholic hepatic steatohepatitis (NASH), and biliary atresia, and the fibrosis is associated with cirrhosis, liver failure and portal hypertension (11). Current clinical therapies for patients with chronic liver diseases are including the removal of the pathological cause, management of the complications, and final liver transplantation (11, 129). However, these strategies are not efficient due to the continued growth of cirrhosis and the paucity of available donor livers (130). Thus, the development of novel treatments to lighten the burden of patients with liver fibrosis is necessary.
Native EVs from MSCs and iPSCs are also major players in studies related to liver fibrosis therapy (Supplementary Table 1). For instance, human amnion MSC-derived EV treatment reduced α-SMA expression and fibrotic areas in a rat CCl4-induced liver fibrosis model by inhibiting Kupffer cells and hepatic stellate cells (HSCs) activations (86). Chemokines, such as CXC chemokine ligands (CXCLs), are involved in the activation of HSCs through autocrine and fibrogenesis (131). Allogenic ADMSC-EVs reduced the CCl4-induced collagen volume fraction and α-SMA/collagen I/III expression by transferring miR-150-5p to inhibit CXCL1 signaling in a mouse CCl4 model (87). Chronic graft-versus-host disease (cGVHD) is a common complication of allogeneic hematopoietic stem cell transplantation and is highly associated with major organ damage, such as liver damage (132, 133). In cGVHD mice, hBMSC-EVs alleviated the degree of liver fibrosis and prolonged animal survival by inducing IL-10+ regulatory cells and inhibiting IL-17+ pathogenic T cells (88). In another study, human skin fibroblast-derived iPSC-secreted EVs decreased HSC activation and liver fibrotic areas by shuttling functional miRNAs (e.g., miR-92a-3p) in a CCl4- or bile duct ligation-induced mouse model (89).
In addition, EVs from liver or other tissue-derived stem cells also showed potential anti-fibrotic effects (Supplementary Table 1). Human liver stem cells (hLSCs) are a stem cell population derived from human adult liver cells that exhibit antifibrotic effects in a murine model of NASH (134). A recent study found that hLSC-derived EVs could attenuate plasma alanine aminotransferase (ALT) and liver fibrosis in a murine model of NASH by delivering miRNAs (miR-29a, miR-30a and let-7) to inhibit collagen I and Snail expression (90). Tonsils are an alternative source of MSCs since tonsil-derived MSCs (T-MSCs) can be readily obtained from surgically removed tonsil tissues. T-MSC-EV treatment reduced the expression of α-SMA, TGF-β, Vimentin and connective tissue growth factor (CTGF) in the liver of the CCl4 model by delivering miR-486-5p to inhibit Hedgehog signaling (91).
Interestingly, EVs from immune cells or healthy liver cells may have a potential role in preventing liver fibrosis (Supplementary Table 1). As a type of innate immune cell, natural killer (NK) cells are important regulators of HSC activation (135, 136). NK-cell line (NK-92MI)-derived EVs reduce TGF-β-induced HSC activation and α-SMA/collagen expression by transferring miR-223 to inhibit the autophagy-related (ATG7) pathway (92). EVs from primary human hepatocytes could also attenuate CCl4-induced hepatocyte injury and profibrotic factor (α-SMA, collagen and CTGF) expression (93). In addition to eukaryotes, EVs isolated from prokaryotes may also have antifibrotic effects. Akkermansia muciniphila is a probiotic with beneficial effects on the host metabolic system and immune response. In a high-fat diet (HFD)- and CCl4-induced mouse liver injury model, probiotic-derived EVs reduced liver functional damage and fibrotic factor (TGF-β, α-SMA and collagen I) expressions by normalizing gut microbiota composition disorders (94).
Chronic kidney disease (CKD) is a huge public health issue that attributed to ~1.5% of deaths worldwide in 2012 (3). Renal fibrosis is the key feature of CKD and the leading cause of the end-stage renal disease (ESRD) and renal failure (137). Current clinical CKD therapeutic strategies mainly focus on controlling ongoing nephron injury, hyperfiltration and renal complications. Once CKD patients progress to ESRD, the only treatment is kidney replacement therapy or kidney transplantation (138). Thus, more efficient therapeutics to prevent renal fibrosis are needed.
As shown in Supplementary Table 1, EVs isolated from stem cells remain as a major candidate for antifibrotic therapy in kidneys (139). For example, hBMSC-EV treatment reduced α-SMA and ECM (collagen I) expressions in a mouse aristolochic acid nephropathy (AAN) model (95). Yes-associated protein (YAP) plays a vital role in fibrogenesis by retaining activated Smad2/3 in the cell nucleus (140). EVs from hucMSCs alleviated interstitial fibrosis by promoting YAP degradation in a rat UUO model (96). Autophagy is a conserved cellular process that removes unnecessary or damaged components, while it may be impaired in the diabetic nephropathy (DN) state (141). In a rat DN model, BMSC-EVs were found to reduce collagen accumulation by inducing mTOR-mediated autophagy (97). Importantly, the antifibrotic effects of MSC-EVs can be replicated in large animal models. In a porcine model of metabolic syndrome (MetS), the systemic administration of ADMSC-EVs restored kidney function and reduced tubulointerstitial fibrosis by IL-10-dependent immunoregulation (98). Additionally, intrarenal injection of ADMSC-EVs decreased ECM expression in another swine MetS model by inducing regulatory T cells (99). These findings suggest that in situ administration of EVs may be an efficient way to improve their therapeutic potency.
Renovascular disease (RVD), represented by the narrowing of one or both renal arteries, can cause high blood pressure and renal dysfunction in patients. In a swine atherosclerotic RVD model, intrarenal-injected ADMSC-EVs decreased interstitial fibrosis while improving the glomerular filtration rate (GFR) and peritubular capillary density by shedding some vasculo-protective genes (e.g., HGF and VEGFR) (100). In addition, EVs isolated from other stem cells may also have therapeutic effects. For example, mouse ESC-derived EVs reduced renal dysfunction (creatinine and blood urea nitrogen) and α-SMA expression by promoting tubular epithelial cell (TEC) proliferation and angiogenesis in a mouse model of ischemic acute kidney injury (AKI) (101). In a mouse AAN, hLSC-derived EVs reduced renal interstitial fibrosis via miR-29b-mediated inhibition of the Wnt/β-catenin pathway (102).
In addition to stem cells, EVs from healthy kidney tissue cells may also have an antifibrotic role (Supplementary Table 1). For example, in a rat ischemic AKI model, renal tubular cell-derived EV treatment reduced collagen I/II/IV/V deposition, α-SMA/FN expression and neutrophil infiltration by shuttling mRNAs encoding cytoplasmic ribosomal proteins (Rps6 and Rps13) (103). Interestingly, EVs isolated from the plasma of MI could inhibit the apoptosis and autophagy of TECs (NRK-52E) in vitro, and decrease the fibrotic area in a contrast-induced nephropathy (CIN) model by delivering miR-1-3p to target ATG13 and activate the AKT pathway (104). These studies suggest the potential of native EVs in attenuating renal fibrosis through a variety of mechanisms.
In addition to these major organs, many other organs or tissues can also undergo fibrosis (Figure 4). For example, in the reproductive system, intrauterine adhesion (IUA) is a serious fibrotic disease due to disordered repair after endometrial basal layer damage. Rabbit BMSC-derived EV treatment inhibited endometrial fibrosis and the TGF-β1/Smad2 pathway in a rabbit model of endometrial injury (105). Subretinal fibrosis is a common complication of macular neovascularization and causes irreversible loss of central vision (142). hucMSC-EV treatment was found to suppress the EMT of retinal pigment epithelial cells in vitro and reduce laser-induced subretinal fibrosis in mice by shedding miR-27-3p to inhibit the homeobox protein Hox-C6 (HOXC6) (106). Intervertebral disc degeneration (IDD) is a major cause of chronic discogenic back pain/sciatica which results in poor quality of life in patients. Fibrosis progression of nucleus pulposus (NP) cells plays a vital role in the pathology of IDD. Rat BMSC-EVs reduced TNF-α-induced ECM (collagen I) expression in NP cells by inhibiting proapoptotic RASSF5 signaling via miR-532-5p (107). Urethral stricture (abnormal narrowing of the urethra) is a complication of urological surgery and is caused by fibrosis of the urethral epithelium due to damage or infection. In a rat urethral injury model, hucMSC-EV treatment inhibited myofibroblast activation by transferring anti-inflammatory miR-146a (108). These reports indicate that MSC-EVs may exert antifibrotic effects in many types of organs by inhibiting EMT, inflammation or apoptosis.
Although native EVs isolated from multiple cell types have shown certain antifibrotic effects, their therapeutic efficiency may be restricted by some intrinsic limitations, such as short half-life, low specific organ retention, possible off-target effects, and insufficient cell sources. Therefore, the development of engineered EVs may resolve these issues and enhance their therapeutic potency (143). Abundant evidence shows that native EVs can be re-engineered by biological or chemical methods and serve as advanced nanomedicines for the resolution of organ fibrosis. In the following sections, we briefly discuss the current modification strategies and therapeutic outcomes of engineered EVs in different organ fibrosis models (Figure 5 and Table 2).
Figure 5 Engineering strategies of EV-based anti-fibrotic therapies. Native EVs can be re-engineered by multiple strategies, such as genetic modifications, preconditions, loading with therapeutic reagents, and incorporation with functional materials. Engineered EVs can exhibit additional beneficial effects, such as enhanced drug payload, longer half-life, better organ targeting capability and bioavailability, and serve as advanced nanomedicines for the resolution of organ fibrosis. (Antisense oligonucleotide: ASO, pneumatic microextrusion: PME).
Genetic engineering of parental cells is a common strategy to produce functional EVs. Engineered EVs with enhanced therapeutic properties or organ targeting efficacy can be obtained from donor cells with genetic modifications, such as gene or protein (e.g., miRNAs and cytokines) overexpression or knockdown (7). Vector transfection and transduction are the main methods used for the genetic engineering of EV donor cells (66). For example, mouse ADMSC-derived EVs were engineered to overexpress miRNA-181-5p, a regulator of hepatic progenitor cell differentiation and autophagy (144). As a result, these engineered EVs had high potency to rescue liver function and reduce ECM (collagen I and FN) and vimentin expression in a CCl4-induced liver fibrosis model (144). miRNA-21a-5p can synchronize with NF-κB activation and it is abundant in fibrotic tissues. Engineered hucMSC-EVs with antagomir-21a-5p showed higher potential in inhibiting fibroblast activation in vitro and α-SMA and collagen III expression in a rat tendon adhesion model than native EVs (145).
EVs can also be engineered with therapeutic proteins (e.g., cytokines). Glial cell line-derived neurotrophic factor (GDNF) is a member of the TGF-β superfamily and plays a vital role in renal morphogenesis and angiogenesis (160). In a UUO renal fibrosis model, GDNF-overexpressing hADMSC-EVs exhibited higher potency to reduce peritubular capillary rarefaction and renal fibrosis score/α-SMA levels than native EVs (146). Disruption of the human leukocyte antigen (HLA) light chain β2-microglobulin (B2 M) gene may disable the function of HLA-I molecules and thus prevent hucMSC-mediated immune rejection (161). hucMSCs with B2 M deletion (B2 M-hucMSCs) were generated using a CRISPR/Cas9 method, and these modified EVs were more efficient in reducing fibrotic areas and restoring cardiac function than native EVs in a rat model of MI (147).
Furthermore, the organ targeting capability of native EVs can be improved by genetic modifications. Fusion of targeting ligands (e.g., peptides) into EV membrane proteins (e.g., Lamp2b and CD63) is a common strategy to produce EVs with specific cell- or tissue-targeting ability (162). For example, a recent study showed that engineered mouse satellite cell-derived EVs using Lamp2b fused with a rabies viral glycoprotein peptide (RVG) had higher renal targeting efficacy. In a UUO model, delivery of miR-29 (a potent inhibitor of TGF-β3) by these modified EVs had higher antifibrotic potency (reducing renal α-SMA, collagen, vimentin and FN levels) than unmodified EVs (148). Altogether, these studies indicate that genetic engineering is a potent strategy to enhance the organ targeting and therapeutic effects of EVs.
Due to their bilayer structure and cargo transferring capacity, EVs can serve as natural carriers of therapeutic agents and protect them against in vivo degradation. The current techniques of loading cargoes into EVs can be divided into exogenous loading (coincubation, electroporation, extrusion or sonication of drugs with isolated EVs) and endogenous loading (genetic modification of EV donor cells) (66, 163). Antisense oligonucleotide (ASO)/siRNA is a type of nucleic acid drug that can silence targeted genes, but its therapeutic potency is largely limited by off-target effects and liver toxicity (164, 165). To overcome these limitations, ASO or siRNA targeting STAT3 (a key regulator of inflammation) was loaded into BMSC-EVs, and the engineered iExosiRNA-STAT3 or iExomASO-STAT3 treatment showed higher potential to reduce collagen I deposition and α-SMA, vimentin and FN expression in a CCl4-induced liver fibrosis model (149).
In addition, EVs can also be used to deliver chemical drugs to enhance their therapeutic index in vivo (Table 2). The anti-inflammatory drug, dexamethasone (DEX), has been used for fibrosis treatment (166). In a recent study, RAW 264.7 macrophage-derived DEX-packaging EVs exhibited a superior capacity to suppress renal inflammation and interstitial fibrosis without apparent glucocorticoid adverse effects in Adriamycin-induced nephropathy in mice (150). These studies suggest that engineered EVs are potent carriers for the delivery of therapeutic agents to alleviate fibrosis.
In addition to the mentioned EV modification methods, parental cells can also be primed/preconditioned with pathological stimuli to produce EVs with desirable profiles or contents useful for fibrosis resolution (Table 2). MSCs are usually preconditioned with hypoxia or cytokines to acquire and retain phenotypes relevant for therapeutic applications (167). For instance, EVs isolated from BMSCs under hypoxic conditions (0.5% O2 for 24 h) had superior therapeutic ability in restoring cardiac function and ameliorating fibrotic scar area after MI, because those EVs had enriched miRNAs such as the anti-apoptotic miR-210 (151). Pretreatment of donor cells with cytokines can augment the immunomodulatory properties of the produced EVs. For example, TNF-α-preconditioned hucMSC-EVs reduced α-SMA and collagen expression in a rat urethral fibrosis model due to the enriched anti-inflammatory miR-146a in those EVs (108). EVs from mouse BMSCs primed with a cocktail of cytokines (IL-1β, IL-6 and TNF-α) had higher efficacy in reducing the necrotic mucosal surface and collagen deposition in a dextran sulfate sodium-induced colitis model (152).
In addition, pretreatment of donor cells with bioactive molecules may also enhance the therapeutic effects of EVs (Table 2). Relaxin (RLN) is an antifibrotic peptide hormone that has been shown to reduce liver fibrosis by reversing the activation of HSCs (168). In a mouse CCl4 liver fibrosis model, EVs from RLN-preconditioned mouse macrophage cell lines (Raw264.7) showed higher potency to lower serum ALT/aspartate aminotransferase (AST) and reduced liver fibrosis (hydroxyproline and α-SMA levels) than native EVs (153). These studies suggest that the preconditioning of parental cells may be an efficient method to produce functionalized EVs.
Current evidence indicates that systemically administered EVs have a short half-life and can be rapidly cleared in vivo. To resolve this problem, an innovative strategy that encapsulates EVs with functional biomaterials has been proposed (Figure 5 and Table 2). EVs delivered by biomaterials such as hydrogels and scaffolds may have enhanced therapeutic efficacy due to prolonged EV release and improved bioavailability (169). Hydrogels are three-dimensional hydrophilic networks with advanced properties, such as tunability, good biocompatibility and biodegradability, and high tissue retention, therefore, they have been widely used to encapsulate cells or drugs. This strategy can also be applied in the local delivery of EVs to improve their stability and half-life (170). Self-assembling peptide (SAP) is a type of biomaterial made of natural amino acids, and some of them can rapidly form a nanoscale hydrogel in ionic saline conditions (171). An injectable SAP hydrogel was used to encapsulate BMSC-EVs, which enabled a sustained release of EVs with preserved biofunction. In a mouse model of ischemic AKI, local delivery of BMSC-EVs by SAP hydrogels showed better efficacy in decreasing renal damage, inflammation and subsequent renal fibrosis (α-SMA and FN) than EVs alone (154).
Similarly, human ESC-EVs were encapsulated into a polyethylene glycol (PEG) hydrogel through a click reaction. The resulting EV-loaded hydrogel showed higher antifibrotic effects in a thioacetamide-induced liver fibrosis model, as indicated by lower expression of MMP-9/13, collagen I and α-SMA than EVs alone (155). The fibrin scaffold is a degradable biopolymer made of fibrinogen and can provide binding sites for cell migration and proliferation to promote tissue regeneration (172). In another study, an invasive EV spray was prepared by incorporating MSC-EVs with fibrin scaffold materials. In a mouse or a swine model of MI, EV spray treatment led to smaller infarct size, thicker left ventricular wall, and enhanced angiomyogenesis than EVs alone in the postinjury heart (156). Altogether, these findings suggest that biomaterial-based EV engineering is an efficient strategy for enhanced antifibrotic therapy.
Moreover, the combination of multiple EV modifications may be an attractive strategy for advanced anti-fibrotic therapy (Table 2). For example, cytokine (TNF-α and IFN-γ)-preconditioned hucMSC-EVs were loaded into a hybrid scaffold made of pneumatic microextrusion (PME, consisting of PLGA, magnesium hydroxide and decellularized porcine kidney extracellular matrix) and polydeoxyribonucleotide (PDRN). This combined treatment showed a synergistic effect of reducing renal inflammation (IL-1RA and TNF-α) and fibrosis (α-SMA, vimentin and Snail) in a mouse model of partial nephrectomy (157). NIN is a tyrosine kinase receptor inhibitor for the treatment of lung fibrosis in the clinic, but its therapeutic efficiency is not ideal due to nonspecific organ distribution. In a recent study, NIN was loaded into hybrid nanovesicles made of clodronate disodium (CLD)-loaded liposomes and fibroblast cell line (L-929)-derived EVs. In a BLM-induced mouse lung fibrosis model, this engineered hybrid EVs showed higher lung retention than unmodified EVs and thus decreased macrophage-mediated inflammation and ECM deposition in the lungs (158). In addition, the combination of stimuli and genetic modification may enhance the therapeutic potency of native EVs. For example, EVs from human dental pulp MSCs with HIF-1α (a master regulator of hypoxia) overexpression and cytokine (IFN-γ, IL-1β and TNF-α) preconditioning were produced, and these EVs had higher potency to alleviate the fibrillar collagen proportion and colon length shortening in a mouse colitis model than native EVs (159). Altogether, the combination of multiple engineering strategies may further enhance the anti-fibrotic potency of EV-based therapies.
To date, few clinical trials have been conducted associated with direct organ fibrosis, and most of them are still in the early stages. For example, a randomized, placebo-controlled, phase 2/3 clinical pilot study was performed to investigate the safety and therapeutic efficacy of cord-blood MSC-EVs in preventing the progression of grade III and IV CKD. The results showed that intra-arterial and intravenous EV injections reduced the inflammatory immune reaction and improved kidney function (173). Recent studies suggest that severe acute respiratory syndrome coronavirus 2 (SARS-CoV-2) infection may cause severe lung damage and substantial lung fibrotic consequences in patients (174). To protect the damaged lungs, several EV-based clinical trials are already underway or seem to be underway. A multicenter, double-blind, randomized controlled trial (RCT) phase 2/3 trial is recruiting, and it aims to evaluate the efficacy and safety of MSC-EVs on reducing inflammation in moderate COVID-19 patients (NCT05216562). An open-label phase 1 study was conducted to evaluate the safety and immunoregulation of EVs carrying CD24 in patients with moderate/severe COVID-19 (NCT04747574). These modified EVs are produced from T-REx™-293 cells engineered to express CD24 (a vital immunomodulator), and its phase II trial is currently active, not recruiting (NCT04969172). Although the current state is still far from clinical applications, increasing evidence suggests that EVs are a potent cell-free, off-the-shelf antifibrotic strategy.
Notably, a large number of studies indicate that EVs may be promising means to mitigate organ fibrosis. Moreover, as a type of naturally derived nanomaterial, EVs have several advantages, such as intrinsic biological properties, the ability to cross biological barriers, and minimal immunogenicity or toxicity in vivo (7, 51). However, there are still some limitations that needed to be well resolved before further translation of EVs into clinical applications.
A primary challenge in this field is how to standardize the production of EVs on a large-scale during isolation, purification, and scalability. For research purposes, small amounts of EVs can be readily isolated by common techniques, such as UC, SEC and immunoaffinity (47), but the yields are far below the clinical requirement. For small animal models, such as rodents, the medium EV dosage is ~80-100 μg/mouse with systematic injection, while it dramatically increases to 75 mg/swine in situ in large animal models (175, 176). In this case, it can be assumed that the required EV amounts for human patients with fibrotic diseases are much larger. Thus, it is urgent to optimize the process of large-scale EV production with high yield and purity, as well as retain integrity and biofunction. More importantly, for clinical applications, it is strictly required to define the bioactive components, standard operating procedures, quality control criteria, virulence and sterility of EV products.
Native EVs usually exhibit a short half-life and insufficient organ retention in vivo. The distribution of EVs can be affected by many factors, such as parental cell types or administration routes (177). To improve the specific organ targeting efficiency of EVs, it is possible to select the appropriate cell source according to the therapeutic intent, such as bronchial epithelial cell-derived EVs for lung fibrosis and cardiosphere-derived EVs for cardiac fibrosis (77, 84). Another strategy is surface modification of EVs with targeting ligands, thereby enhancing the uptake of therapeutic EVs in targeted organs. The administration routes of EVs may also affect their therapeutic potency. For instance, i.v. injected EVs showed higher retention in the liver and spleen and lower retention in the pancreas compared to i.p. and subcutaneous injection (177). Thus, the selection of a proper delivery route should also be considered in the treatment of fibrosis in different organs.
Since the mechanism of fibrosis is complicated and many types of cells and pathways are involved, it seems that single-target therapy may not be efficient. In fact, many antifibrotic strategies, such as cessation of chronic tissue injury, resolution of local inflammation, deactivation or elimination of myofibroblasts, and degradation of ECM, have been evaluated individually or combined in preclinical studies or clinical trials (5). However, many current EV-based studies are still focusing on targeting a single cause (e.g., inflammation and angiogenesis), and the direct antifibrotic potency of EVs needs to be further enhanced. To address this limitation, a better strategy is to develop engineered EVs that can target multiple essential cells or pathways involved in fibrosis, which may exhibit advanced therapeutic effects.
Another important limitation is that current findings related to the anti-fibrotic effects of EVs are mainly based on small animal (mouse and rat) models, while there have been only a small number of large animal (e.g., swine) model-based studies (156). Moreover, these models may not fully mimic the pathology of human patients due to the large genetic and/or physiological differences between humans and small animals. For example, rodent models of liver fibrosis are commonly induced by toxic reagents (e.g., CCl4) or bile duct ligation, while virus (hepatitis B virus) infection, alcoholic liver disease, and nonalcoholic fatty liver disease are the main causes of liver fibrosis in the clinic (2, 178). Thus, standard and large animal models that can better mimic the fibrosis of patients are needed in this field.
In summary, EV-based therapeutics have shown promising effects in mitigating multiple types of organ fibrosis in many preclinical studies. Moreover, the therapeutic potential of EVs can be further improved using multiple modification strategies. To better translate EV-based therapies into clinical applications, more research is required to clarify the direct antifibrotic role of EVs and to establish large-scale production and efficient EV engineering strategies in the future.
KL wrote the manuscript and prepared for visualization. YW, PL, SL and PZ prepared for drawing elements, LY and YL played as supervision roles. JC provided financial support. JL is corresponding author and review the manuscript. All authors contributed to manuscript revision, read, and approved the submitted version. All authors contributed to the article and approved the submitted version.
This study was partly supported by the National Natural Science Foundation of China (32071453, 31871001, 81571808, 32271438, 32001011), 1.3.5 Project for Disciplines of Excellence (ZYGD18014), the Fundamental Research Funds for the Central Universities (2020SCU12040), and the PostDoctor Research Project of West China Hospital of Sichuan University (2020HXBH047).
We would like to thank Wenshu Dai from Sichuan University for language editing.
The authors declare that the research was conducted in the absence of any commercial or financial relationships that could be construed as a potential conflict of interest.
All claims expressed in this article are solely those of the authors and do not necessarily represent those of their affiliated organizations, or those of the publisher, the editors and the reviewers. Any product that may be evaluated in this article, or claim that may be made by its manufacturer, is not guaranteed or endorsed by the publisher.
The Supplementary Material for this article can be found online at: https://www.frontiersin.org/articles/10.3389/fimmu.2022.1042983/full#supplementary-material
1. Henderson NC, Rieder F, Wynn TA. Fibrosis: From mechanisms to medicines. Nature (2020) 587(7835):555–66. doi: 10.1038/s41586-020-2938-9
2. Parola M, Pinzani M. Liver fibrosis: Pathophysiology, pathogenetic targets and clinical issues. Mol Aspects Med (2019) 65:37–55. doi: 10.1016/j.mam.2018.09.002
3. Webster AC, Nagler EV, Morton RL, Masson P. Chronic kidney disease. Lancet (2017) 389(10075):1238–52. doi: 10.1016/s0140-6736(16)32064-5
4. Wynn TA. Cellular and molecular mechanisms of fibrosis. J Pathol (2008) 214(2):199–210. doi: 10.1002/path.2277
5. Weiskirchen R, Weiskirchen S, Tacke F. Organ and tissue fibrosis: Molecular signals, cellular mechanisms and translational implications. Mol Aspects Med (2019) 65:2–15. doi: 10.1016/j.mam.2018.06.003
6. Teng F, Fussenegger M. Shedding light on extracellular vesicle biogenesis and bioengineering. Advanced Sci (Weinheim Baden-Wurttemberg Germany) (2020) 8(1):2003505. doi: 10.1002/advs.202003505
7. de Abreu RC, Fernandes H, da Costa Martins PA, Sahoo S, Emanueli C, Ferreira L. Native and bioengineered extracellular vesicles for cardiovascular therapeutics. Nat Rev Cardiol (2020) 17(11):685–97. doi: 10.1038/s41569-020-0389-5
8. Parola M, Pinzani M. Pathophysiology of organ and tissue fibrosis. Mol Aspects Med (2019) 65:1. doi: 10.1016/j.mam.2019.02.001
9. Meng XM, Nikolic-Paterson DJ, Lan HY. Tgf-B: The master regulator of fibrosis. Nat Rev Nephrol (2016) 12(6):325–38. doi: 10.1038/nrneph.2016.48
10. Chung AC, Lan HY. Chemokines in renal injury. J Am Soc Nephrol (2011) 22(5):802–9. doi: 10.1681/asn.2010050510
11. Kisseleva T, Brenner D. Molecular and cellular mechanisms of liver fibrosis and its regression. Nat Rev Gastroenterol Hepatol (2021) 18(3):151–66. doi: 10.1038/s41575-020-00372-7
12. Sun YB, Qu X, Caruana G, Li J. The origin of renal Fibroblasts/Myofibroblasts and the signals that trigger fibrosis. Differentiation (2016) 92(3):102–7. doi: 10.1016/j.diff.2016.05.008
13. Nieto MA, Huang RY, Jackson RA, Thiery JP. Emt: 2016. Cell (2016) 166(1):21–45. doi: 10.1016/j.cell.2016.06.028
14. Horowitz JC, Thannickal VJ. Mechanisms for the resolution of organ fibrosis. Physiol (Bethesda) (2019) 34(1):43–55. doi: 10.1152/physiol.00033.2018
15. Masuzaki R, Kanda T, Sasaki R, Matsumoto N, Ogawa M, Matsuoka S, et al. Noninvasive assessment of liver fibrosis: Current and future clinical and molecular perspectives. Int J Mol Sci (2020) 21(14):4906. doi: 10.3390/ijms21144906
16. Mahalanobish S, Saha S, Dutta S, Sil PC. Matrix metalloproteinase: An upcoming therapeutic approach for idiopathic pulmonary fibrosis. Pharmacol Res (2020) 152:104591. doi: 10.1016/j.phrs.2019.104591
17. Craig VJ, Zhang L, Hagood JS, Owen CA. Matrix metalloproteinases as therapeutic targets for idiopathic pulmonary fibrosis. Am J Respir Cell Mol Biol (2015) 53(5):585–600. doi: 10.1165/rcmb.2015-0020TR
18. Arpino V, Brock M, Gill SE. The role of timps in regulation of extracellular matrix proteolysis. Matrix Biol (2015) 44-46:247–54. doi: 10.1016/j.matbio.2015.03.005
19. Frangogiannis N. Transforming growth factor-B; in tissue fibrosis. J Exp Med (2020) 217(3):e20190103. doi: 10.1084/jem.20190103
20. Kim KK, Sheppard D, Chapman HA. Tgf-B1 signaling and tissue fibrosis. Cold Spring Harb Perspect Biol (2018) 10(4):a022293. doi: 10.1101/cshperspect.a022293
21. Derynck R, Zhang YE. Smad-dependent and smad-independent pathways in tgf-beta family signalling. Nature (2003) 425(6958):577–84. doi: 10.1038/nature02006
22. Györfi AH, Matei AE, Distler JHW. Targeting tgf-B signaling for the treatment of fibrosis. Matrix Biol (2018) 68-69:8–27. doi: 10.1016/j.matbio.2017.12.016
23. Zhao X, Kwan JYY, Yip K, Liu PP, Liu FF. Targeting metabolic dysregulation for fibrosis therapy. Nat Rev Drug Discovery (2020) 19(1):57–75. doi: 10.1038/s41573-019-0040-5
24. Schunk SJ, Floege J, Fliser D, Speer T. Wnt-B-Catenin signalling - a versatile player in kidney injury and repair. Nat Rev Nephrol (2021) 17(3):172–84. doi: 10.1038/s41581-020-00343-w
25. Akhmetshina A, Palumbo K, Dees C, Bergmann C, Venalis P, Zerr P, et al. Activation of canonical wnt signalling is required for tgf-B-Mediated fibrosis. Nat Commun (2012) 3:735. doi: 10.1038/ncomms1734
26. Distler JHW, Györfi AH, Ramanujam M, Whitfield ML, Königshoff M, Lafyatis R. Shared and distinct mechanisms of fibrosis. Nat Rev Rheumatol (2019) 15(12):705–30. doi: 10.1038/s41584-019-0322-7
27. Kramann R. Hedgehog gli signalling in kidney fibrosis. Nephrol Dial Transplant (2016) 31(12):1989–95. doi: 10.1093/ndt/gfw102
28. Effendi WI, Nagano T. The hedgehog signaling pathway in idiopathic pulmonary fibrosis: Resurrection time. Int J Mol Sci (2021) 23(1):171. doi: 10.3390/ijms23010171
29. Boon MR, van der Horst G, van der Pluijm G, Tamsma JT, Smit JW, Rensen PC. Bone morphogenetic protein 7: A broad-spectrum growth factor with multiple target therapeutic potency. Cytokine Growth Factor Rev (2011) 22(4):221–9. doi: 10.1016/j.cytogfr.2011.08.001
30. Jun JI, Lau LF. Resolution of organ fibrosis. J Clin Invest (2018) 128(1):97–107. doi: 10.1172/jci93563
31. Spagnolo P, Kropski JA, Jones MG, Lee JS, Rossi G, Karampitsakos T, et al. Idiopathic pulmonary fibrosis: Disease mechanisms and drug development. Pharmacol Ther (2021) 222:107798. doi: 10.1016/j.pharmthera.2020.107798
32. Armstrong JP, Holme MN, Stevens MM. Re-engineering extracellular vesicles as smart nanoscale therapeutics. ACS nano (2017) 11(1):69–83. doi: 10.1021/acsnano.6b07607
33. Stahl PD, Raposo G. Exosomes and extracellular vesicles: The path forward. Essays Biochem (2018) 62(2):119–24. doi: 10.1042/ebc20170088
34. Elsharkasy OM, Nordin JZ, Hagey DW, de Jong OG, Schiffelers RM, Andaloussi SE, et al. Extracellular vesicles as drug delivery systems: Why and how? Adv Drug Delivery Rev (2020) 159:332–43. doi: 10.1016/j.addr.2020.04.004
35. van Niel G, Carter DRF, Clayton A, Lambert DW, Raposo G, Vader P. Challenges and directions in studying cell-cell communication by extracellular vesicles. Nat Rev Mol Cell Biol (2022) 23(5):369–82. doi: 10.1038/s41580-022-00460-3
36. Merchant ML, Rood IM, Deegens JKJ, Klein JB. Isolation and characterization of urinary extracellular vesicles: Implications for biomarker discovery. Nat Rev Nephrol (2017) 13(12):731–49. doi: 10.1038/nrneph.2017.148
37. van Niel G, D'Angelo G, Raposo G. Shedding light on the cell biology of extracellular vesicles. Nat Rev Mol Cell Biol (2018) 19(4):213–28. doi: 10.1038/nrm.2017.125
38. Raposo G, Stoorvogel W. Extracellular vesicles: Exosomes, microvesicles, and friends. J Cell Biol (2013) 200(4):373–83. doi: 10.1083/jcb.201211138
39. Sharma S, Masud MK, Kaneti YV, Rewatkar P, Koradia A, Hossain MSA, et al. Extracellular vesicle nanoarchitectonics for novel drug delivery applications. Small (Weinheim an der Bergstrasse Germany) (2021) 17(42):e2102220. doi: 10.1002/smll.202102220
40. Jiang L, Vader P, Schiffelers RM. Extracellular vesicles for nucleic acid delivery: Progress and prospects for safe rna-based gene therapy. Gene Ther (2017) 24(3):157–66. doi: 10.1038/gt.2017.8
41. Schulz-Siegmund M, Aigner A. Nucleic acid delivery with extracellular vesicles. Adv Drug Deliv Rev (2021) 173:89–111. doi: 10.1016/j.addr.2021.03.005
42. Zhao M, Liu S, Wang C, Wang Y, Wan M, Liu F, et al. Mesenchymal stem cell-derived extracellular vesicles attenuate mitochondrial damage and inflammation by stabilizing mitochondrial DNA. ACS Nano (2021) 15(1):1519–38. doi: 10.1021/acsnano.0c08947
43. Mulcahy LA, Pink RC, Carter DR. Routes and mechanisms of extracellular vesicle uptake. J Extracellular Vesicles (2014) 3:24641. doi: 10.3402/jev.v3.24641
44. Maas SLN, Breakefield XO, Weaver AM. Extracellular vesicles: Unique intercellular delivery vehicles. Trends Cell Biol (2017) 27(3):172–88. doi: 10.1016/j.tcb.2016.11.003
45. Mathieu M, Martin-Jaular L, Lavieu G, Théry C. Specificities of secretion and uptake of exosomes and other extracellular vesicles for cell-to-Cell communication. Nat Cell Biol (2019) 21(1):9–17. doi: 10.1038/s41556-018-0250-9
46. Gardiner C, Di Vizio D, Sahoo S, Théry C, Witwer KW, Wauben M, et al. Techniques used for the isolation and characterization of extracellular vesicles: Results of a worldwide survey. J Extracellular Vesicles (2016) 5:32945. doi: 10.3402/jev.v5.32945
47. Sidhom K, Obi PO, Saleem A. A review of exosomal isolation methods: Is size exclusion chromatography the best option? Int J Mol Sci (2020) 21(18):6466. doi: 10.3390/ijms21186466
48. Taylor DD, Shah S. Methods of isolating extracellular vesicles impact down-stream analyses of their cargoes. Methods (2015) 87:3–10. doi: 10.1016/j.ymeth.2015.02.019
49. Monguió-Tortajada M, Gálvez-Montón C, Bayes-Genis A, Roura S, Borràs FE. Extracellular vesicle isolation methods: Rising impact of size-exclusion chromatography. Cell Mol Life Sci (2019) 76(12):2369–82. doi: 10.1007/s00018-019-03071-y
50. Willms E, Cabañas C, Mäger I, Wood MJA, Vader P. Extracellular vesicle heterogeneity: Subpopulations, isolation techniques, and diverse functions in cancer progression. Front Immunol (2018) 9:738. doi: 10.3389/fimmu.2018.00738
51. Vader P, Mol EA, Pasterkamp G, Schiffelers RM. Extracellular vesicles for drug delivery. Adv Drug Deliv Rev (2016) 106(Pt A):148–56. doi: 10.1016/j.addr.2016.02.006
52. Wang Y, Zhao M, Liu S, Guo J, Lu Y, Cheng J, et al. Macrophage-derived extracellular vesicles: Diverse mediators of pathology and therapeutics in multiple diseases. Cell Death Dis (2020) 11(10):924. doi: 10.1038/s41419-020-03127-z
53. Russell AE, Sneider A, Witwer KW, Bergese P, Bhattacharyya SN, Cocks A, et al. Biological membranes in ev biogenesis, stability, uptake, and cargo transfer: An isev position paper arising from the isev membranes and evs workshop. J Extracellular Vesicles (2019) 8(1):1684862. doi: 10.1080/20013078.2019.1684862
54. Soo CY, Song Y, Zheng Y, Campbell EC, Riches AC, Gunn-Moore F, et al. Nanoparticle tracking analysis monitors microvesicle and exosome secretion from immune cells. Immunology (2012) 136(2):192–7. doi: 10.1111/j.1365-2567.2012.03569.x
55. Sitar S, Kejžar A, Pahovnik D, Kogej K, Tušek-Žnidarič M, Lenassi M, et al. Size characterization and quantification of exosomes by asymmetrical-flow field-flow fractionation. Anal Chem (2015) 87(18):9225–33. doi: 10.1021/acs.analchem.5b01636
56. Gandham S, Su X, Wood J, Nocera AL, Alli SC, Milane L, et al. Technologies and standardization in research on extracellular vesicles. Trends Biotechnol (2020) 38(10):1066–98. doi: 10.1016/j.tibtech.2020.05.012
57. Salunkhe S, Dheeraj, Basak M, Chitkara D, Mittal A. Surface functionalization of exosomes for target-specific delivery and in vivo imaging & tracking: Strategies and significance. J Control Release (2020) 326:599–614. doi: 10.1016/j.jconrel.2020.07.042
58. Herrmann IK, Wood MJA, Fuhrmann G. Extracellular vesicles as a next-generation drug delivery platform. Nat Nanotechnology (2021) 16(7):748–59. doi: 10.1038/s41565-021-00931-2
59. Reiner AT, Witwer KW, van Balkom BWM, de Beer J, Brodie C, Corteling RL, et al. Concise review: Developing best-practice models for the therapeutic use of extracellular vesicles. Stem Cells Trans Med (2017) 6(8):1730–9. doi: 10.1002/sctm.17-0055
60. Staubach S, Bauer FN, Tertel T, Börger V, Stambouli O, Salzig D, et al. Scaled preparation of extracellular vesicles from conditioned media. Adv Drug Deliv Rev (2021) 177:113940. doi: 10.1016/j.addr.2021.113940
61. Panchalingam KM, Jung S, Rosenberg L, Behie LA. Bioprocessing strategies for the Large-scale production of human mesenchymal stem cells: A review. Stem Cell Res Ther (2015) 6:225. doi: 10.1186/s13287-015-0228-5
62. Phan J, Kumar P, Hao D, Gao K, Farmer D, Wang A. Engineering mesenchymal stem cells to improve their exosome efficacy and yield for cell-free therapy. J Extracellular Vesicles (2018) 7(1):1522236. doi: 10.1080/20013078.2018.1522236
63. Berger A, Araújo-Filho I, Piffoux M, Nicolás-Boluda A, Grangier A, Boucenna I, et al. Local administration of stem cell-derived extracellular vesicles in a thermoresponsive hydrogel promotes a pro-healing effect in a rat model of colo-cutaneous post-surgical fistula. Nanoscale (2021) 13(1):218–32. doi: 10.1039/d0nr07349k
64. Lee JH, Ha DH, Go HK, Youn J, Kim HK, Jin RC, et al. Reproducible Large-scale isolation of exosomes from adipose tissue-derived mesenchymal Stem/Stromal cells and their application in acute kidney injury. Int J Mol Sci (2020) 21(13):4774. doi: 10.3390/ijms21134774
65. Haraszti RA, Miller R, Stoppato M, Sere YY, Coles A, Didiot MC, et al. Exosomes produced from 3d cultures of mscs by tangential flow filtration show higher yield and improved activity. Mol Ther (2018) 26(12):2838–47. doi: 10.1016/j.ymthe.2018.09.015
66. Wiklander OPB, Brennan M, Lötvall J, Breakefield XO, El Andaloussi S. Advances in therapeutic applications of extracellular vesicles. Sci Transl Med (2019) 11(492):eaav8521. doi: 10.1126/scitranslmed.aav8521
67. Lou P, Liu S, Xu X, Pan C, Lu Y, Liu J. Extracellular vesicle-based therapeutics for the regeneration of chronic wounds: Current knowledge and future perspectives. Acta Biomater (2021) 119:42–56. doi: 10.1016/j.actbio.2020.11.001
68. Zhang Y, Bi J, Huang J, Tang Y, Du S, Li P. Exosome: A review of its classification, isolation techniques, storage, diagnostic and targeted therapy applications. Int J Nanomedicine (2020) 15:6917–34. doi: 10.2147/ijn.S264498
69. Jeyaram A, Jay SM. Preservation and storage stability of extracellular vesicles for therapeutic applications. AAPS J (2017) 20(1):1. doi: 10.1208/s12248-017-0160-y
70. Charoenviriyakul C, Takahashi Y, Nishikawa M, Takakura Y. Preservation of exosomes at room temperature using lyophilization. Int J Pharm (2018) 553(1-2):1–7. doi: 10.1016/j.ijpharm.2018.10.032
71. Lindoso RS, Lopes JA, Binato R, Abdelhay E, Takiya CM, Miranda KR, et al. Adipose mesenchymal cells-derived evs alleviate doca-Salt-Induced hypertension by promoting cardio-renal protection. Mol Ther Methods Clin Dev (2020) 16:63–77. doi: 10.1016/j.omtm.2019.11.002
72. Deng S, Zhou X, Ge Z, Song Y, Wang H, Liu X, et al. Exosomes from adipose-derived mesenchymal stem cells ameliorate cardiac damage after myocardial infarction by activating S1p/Sk1/S1pr1 signaling and promoting macrophage M2 polarization. Int J Biochem Cell Biol (2019) 114:105564. doi: 10.1016/j.biocel.2019.105564
73. Feng R, Ullah M, Chen K, Ali Q, Lin Y, Sun Z. Stem cell-derived extracellular vesicles mitigate ageing-associated arterial stiffness and hypertension. J Extracellular Vesicles (2020) 9(1):1783869. doi: 10.1080/20013078.2020.1783869
74. Santoso MR, Ikeda G, Tada Y, Jung JH, Vaskova E, Sierra RG, et al. Exosomes from induced pluripotent stem cell-derived cardiomyocytes promote autophagy for myocardial repair. J Am Heart Assoc (2020) 9(6):e014345. doi: 10.1161/jaha.119.014345
75. Wu Q, Wang J, Tan WLW, Jiang Y, Wang S, Li Q, et al. Extracellular vesicles from human embryonic stem cell-derived cardiovascular progenitor cells promote cardiac infarct healing through reducing cardiomyocyte death and promoting angiogenesis. Cell Death Dis (2020) 11(5):354. doi: 10.1038/s41419-020-2508-y
76. Cambier L, Giani JF, Liu W, Ijichi T, Echavez AK, Valle J, et al. Angiotensin ii-induced end-organ damage in mice is attenuated by human exosomes and by an exosomal y rna fragment. Hypertension (2018) 72(2):370–80. doi: 10.1161/hypertensionaha.118.11239
77. Hirai K, Ousaka D, Fukushima Y, Kondo M, Eitoku T, Shigemitsu Y, et al. Cardiosphere-derived exosomal micrornas for myocardial repair in pediatric dilated cardiomyopathy. Sci Transl Med (2020) 12(573):eabb3336. doi: 10.1126/scitranslmed.abb3336
78. Salybekov AA, Salybekova A, Sheng Y, Shinozaki Y, Yokoyama K, Kobayashi S, et al. Extracellular vesicles derived from regeneration associated cells preserve heart function after ischemia-induced injury. Front Cardiovasc Med (2021) 8:754254. doi: 10.3389/fcvm.2021.754254
79. Xiao K, He W, Guan W, Hou F, Yan P, Xu J, et al. Mesenchymal stem cells reverse emt process through blocking the activation of nf-Kb and hedgehog pathways in lps-induced acute lung injury. Cell Death Dis (2020) 11(10):863. doi: 10.1038/s41419-020-03034-3
80. Bandeira E, Oliveira H, Silva JD, Menna-Barreto RFS, Takyia CM, Suk JS, et al. Therapeutic effects of adipose-Tissue-Derived mesenchymal stromal cells and their extracellular vesicles in experimental silicosis. Respir Res (2018) 19(1):104. doi: 10.1186/s12931-018-0802-3
81. Shi L, Ren J, Li J, Wang D, Wang Y, Qin T, et al. Extracellular vesicles derived from umbilical cord mesenchymal stromal cells alleviate pulmonary fibrosis by means of transforming growth factor-B signaling inhibition. Stem Cell Res Ther (2021) 12(1):230. doi: 10.1186/s13287-021-02296-8
82. Zhou J, Lin Y, Kang X, Liu Z, Zhang W, Xu F. Microrna-186 in extracellular vesicles from bone marrow mesenchymal stem cells alleviates idiopathic pulmonary fibrosis Via interaction with Sox4 and Dkk1. Stem Cell Res Ther (2021) 12(1):96. doi: 10.1186/s13287-020-02083-x
83. Mansouri N, Willis GR, Fernandez-Gonzalez A, Reis M, Nassiri S, Mitsialis SA, et al. Mesenchymal stromal cell exosomes prevent and revert experimental pulmonary fibrosis through modulation of monocyte phenotypes. JCI Insight (2019) 4(21):e128060. doi: 10.1172/jci.insight.128060
84. Kadota T, Fujita Y, Araya J, Watanabe N, Fujimoto S, Kawamoto H, et al. Human bronchial epithelial cell-derived extracellular vesicle therapy for pulmonary fibrosis Via inhibition of tgf-B-Wnt crosstalk. J extracellular vesicles (2021) 10(10):e12124. doi: 10.1002/jev2.12124
85. Dinh PC, Paudel D, Brochu H, Popowski KD, Gracieux MC, Cores J, et al. Inhalation of lung spheroid cell secretome and exosomes promotes lung repair in pulmonary fibrosis. Nat Commun (2020) 11(1):1064. doi: 10.1038/s41467-020-14344-7
86. Ohara M, Ohnishi S, Hosono H, Yamamoto K, Yuyama K, Nakamura H, et al. Extracellular vesicles from amnion-derived mesenchymal stem cells ameliorate hepatic inflammation and fibrosis in rats. Stem Cells Int (2018) 2018:3212643. doi: 10.1155/2018/3212643
87. Du Z, Wu T, Liu L, Luo B, Wei C. Extracellular vesicles-derived mir-150-5p secreted by adipose-derived mesenchymal stem cells inhibits Cxcl1 expression to attenuate hepatic fibrosis. J Cell Mol Med (2021) 25(2):701–15. doi: 10.1111/jcmm.16119
88. Lai P, Chen X, Guo L, Wang Y, Liu X, Liu Y, et al. A potent immunomodulatory role of exosomes derived from mesenchymal stromal cells in preventing cgvhd. J Hematol Oncol (2018) 11(1):135. doi: 10.1186/s13045-018-0680-7
89. Povero D, Pinatel EM, Leszczynska A, Goyal NP, Nishio T, Kim J, et al. Human induced pluripotent stem cell-derived extracellular vesicles reduce hepatic stellate cell activation and liver fibrosis. JCI Insight (2019) 5(14):e125652. doi: 10.1172/jci.insight.125652
90. Bruno S, Pasquino C, Herrera Sanchez MB, Tapparo M, Figliolini F, Grange C, et al. Hlsc-derived extracellular vesicles attenuate liver fibrosis and inflammation in a murine model of non-alcoholic steatohepatitis. Mol Ther (2020) 28(2):479–89. doi: 10.1016/j.ymthe.2019.10.016
91. Kim J, Lee C, Shin Y, Wang S, Han J, Kim M, et al. Sevs from tonsil-derived mesenchymal stromal cells alleviate activation of hepatic stellate cells and liver fibrosis through mir-486-5p. Mol Ther (2021) 29(4):1471–86. doi: 10.1016/j.ymthe.2020.12.025
92. Wang L, Wang Y, Quan J. Exosomal mir-223 derived from natural killer cells inhibits hepatic stellate cell activation by suppressing autophagy. Mol Med (2020) 26(1):81. doi: 10.1186/s10020-020-00207-w
93. Li X, Chen R, Kemper S, Brigstock DR. Extracellular vesicles from hepatocytes are therapeutic for toxin-mediated fibrosis and gene expression in the liver. Front Cell Dev Biol (2019) 7:368. doi: 10.3389/fcell.2019.00368
94. Keshavarz Azizi Raftar S, Ashrafian F, Yadegar A, Lari A, Moradi HR, Shahriary A, et al. The protective effects of live and pasteurized akkermansia muciniphila and its extracellular vesicles against Hfd/Ccl4-induced liver injury. Microbiol Spectr (2021) 9(2):e0048421. doi: 10.1128/Spectrum.00484-21
95. Kholia S, Herrera Sanchez MB, Cedrino M, Papadimitriou E, Tapparo M, Deregibus MC, et al. Mesenchymal stem cell derived extracellular vesicles ameliorate kidney injury in aristolochic acid nephropathy. Front Cell Dev Biol (2020) 8:188. doi: 10.3389/fcell.2020.00188
96. Ji C, Zhang J, Zhu Y, Shi H, Yin S, Sun F, et al. Exosomes derived from hucmsc attenuate renal fibrosis through Ck1δ/B-Trcp-Mediated yap degradation. Cell Death Dis (2020) 11(5):327. doi: 10.1038/s41419-020-2510-4
97. Ebrahim N, Ahmed IA, Hussien NI, Dessouky AA, Farid AS, Elshazly AM, et al. Mesenchymal stem cell-derived exosomes ameliorated diabetic nephropathy by autophagy induction through the mtor signaling pathway. Cells (2018) 7(12):226. doi: 10.3390/cells7120226
98. Eirin A, Zhu XY, Puranik AS, Tang H, McGurren KA, van Wijnen AJ, et al. Mesenchymal stem cell-derived extracellular vesicles attenuate kidney inflammation. Kidney Int (2017) 92(1):114–24. doi: 10.1016/j.kint.2016.12.023
99. Song T, Eirin A, Zhu X, Zhao Y, Krier JD, Tang H, et al. Mesenchymal stem cell-derived extracellular vesicles induce regulatory T cells to ameliorate chronic kidney injury. Hypertension (2020) 75(5):1223–32. doi: 10.1161/hypertensionaha.119.14546
100. Ferguson CM, Farahani RA, Zhu XY, Tang H, Jordan KL, Saadiq IM, et al. Mesenchymal Stem/Stromal cell-derived extracellular vesicles elicit better preservation of the intra-renal microvasculature than renal revascularization in pigs with renovascular disease. Cells (2021) 10(4):763. doi: 10.3390/cells10040763
101. Yu L, Liu S, Wang C, Zhang C, Wen Y, Zhang K, et al. Embryonic stem cell-derived extracellular vesicles promote the recovery of kidney injury. Stem Cell Res Ther (2021) 12(1):379. doi: 10.1186/s13287-021-02460-0
102. Kholia S, Herrera Sanchez MB, Deregibus MC, Sassoè-Pognetto M, Camussi G, Brizzi MF. Human liver stem cell derived extracellular vesicles alleviate kidney fibrosis by interfering with the B-catenin pathway through Mir29b. Int J Mol Sci (2021) 22(19):10780. doi: 10.3390/ijms221910780
103. Dominguez JH, Liu Y, Gao H, Dominguez JM 2nd, Xie D, Kelly KJ. Renal tubular cell-derived extracellular vesicles accelerate the recovery of established renal ischemia reperfusion injury. J Am Soc Nephrol (2017) 28(12):3533–44. doi: 10.1681/asn.2016121278
104. Zhao P, Zhu Y, Sun L, Zhu W, Lu Y, Zhang J, et al. Circulating exosomal mir-1-3p from rats with myocardial infarction plays a protective effect on contrast-induced nephropathy Via targeting Atg13 and activating the akt signaling pathway. Int J Biol Sci (2021) 17(4):972–85. doi: 10.7150/ijbs.55887
105. Yao Y, Chen R, Wang G, Zhang Y, Liu F. Exosomes derived from mesenchymal stem cells reverse emt Via tgf-B1/Smad pathway and promote repair of damaged endometrium. Stem Cell Res Ther (2019) 10(1):225. doi: 10.1186/s13287-019-1332-8
106. Li D, Zhang J, Liu Z, Gong Y, Zheng Z. Human umbilical cord mesenchymal stem cell-derived exosomal mir-27b attenuates subretinal fibrosis Via suppressing epithelial-mesenchymal transition by targeting Hoxc6. Stem Cell Res Ther (2021) 12(1):24. doi: 10.1186/s13287-020-02064-0
107. Zhu G, Yang X, Peng C, Yu L, Hao Y. Exosomal mir-532-5p from bone marrow mesenchymal stem cells reduce intervertebral disc degeneration by targeting Rassf5. Exp Cell Res (2020) 393(2):112109. doi: 10.1016/j.yexcr.2020.112109
108. Liang YC, Wu YP, Li XD, Chen SH, Ye XJ, Xue XY, et al. Tnf-A-Induced exosomal mir-146a mediates mesenchymal stem cell-dependent suppression of urethral stricture. J Cell Physiol (2019) 234(12):23243–55. doi: 10.1002/jcp.28891
109. Townsend N, Kazakiewicz D, Lucy Wright F, Timmis A, Huculeci R, Torbica A, et al. Epidemiology of cardiovascular disease in Europe. Nat Rev Cardiol (2022) 19(2):133–43. doi: 10.1038/s41569-021-00607-3
110. Simon ST, Kini V, Levy AE, Ho PM. Medication adherence in cardiovascular medicine. BMJ (2021) 374:n1493. doi: 10.1136/bmj.n1493
111. Tallquist MD. Cardiac fibroblast diversity. Annu Rev Physiol (2020) 82:63–78. doi: 10.1146/annurev-physiol-021119-034527
112. Liu M, López de Juan Abad B, Cheng K. Cardiac fibrosis: Myofibroblast-mediated pathological regulation and drug delivery strategies. Adv Drug Delivery Rev (2021) 173:504–19. doi: 10.1016/j.addr.2021.03.021
113. Hinderer S, Schenke-Layland K. Cardiac fibrosis - a short review of causes and therapeutic strategies. Adv Drug Deliv Rev (2019) 146:77–82. doi: 10.1016/j.addr.2019.05.011
114. Ma ZG, Yuan YP, Wu HM, Zhang X, Tang QZ. Cardiac fibrosis: New insights into the pathogenesis. Int J Biol Sci (2018) 14(12):1645–57. doi: 10.7150/ijbs.28103
115. Weng Z, Zhang B, Wu C, Yu F, Han B, Li B, et al. Therapeutic roles of mesenchymal stem cell-derived extracellular vesicles in cancer. J Hematol Oncol (2021) 14(1):136. doi: 10.1186/s13045-021-01141-y
116. Xiong M, Jiang L, Zhou Y, Qiu W, Fang L, Tan R, et al. The mir-200 family regulates tgf-B1-Induced renal tubular epithelial to mesenchymal transition through smad pathway by targeting Zeb1 and Zeb2 expression. Am J Physiol Renal Physiol (2012) 302(3):F369–79. doi: 10.1152/ajprenal.00268.2011
117. Zhao Y, Sun X, Cao W, Ma J, Sun L, Qian H, et al. Exosomes derived from human umbilical cord mesenchymal stem cells relieve acute myocardial ischemic injury. Stem Cells Int (2015) 2015:761643. doi: 10.1155/2015/761643
118. Jung JH, Fu X, Yang PC. Exosomes generated from ipsc-derivatives: New direction for stem cell therapy in human heart diseases. Circ Res (2017) 120(2):407–17. doi: 10.1161/circresaha.116.309307
119. Smith RR, Barile L, Cho HC, Leppo MK, Hare JM, Messina E, et al. Regenerative potential of cardiosphere-derived cells expanded from percutaneous endomyocardial biopsy specimens. Circulation (2007) 115(7):896–908. doi: 10.1161/circulationaha.106.655209
120. Lipshultz SE, Law YM, Asante-Korang A, Austin ED, Dipchand AI, Everitt MD, et al. Cardiomyopathy in children: Classification and diagnosis: A scientific statement from the American heart association. Circulation (2019) 140(1):e9-68. doi: 10.1161/cir.0000000000000682
121. Salybekov AA, Kawaguchi AT, Masuda H, Vorateera K, Okada C, Asahara T. Regeneration-associated cells improve recovery from myocardial infarction through enhanced vasculogenesis, anti-inflammation, and cardiomyogenesis. PloS One (2018) 13(11):e0203244. doi: 10.1371/journal.pone.0203244
122. Parimon T, Hohmann MS, Yao C. Cellular senescence: Pathogenic mechanisms in lung fibrosis. Int J Mol Sci (2021) 22(12):6214. doi: 10.3390/ijms22126214
123. Kapnadak SG, Raghu G. Lung transplantation for interstitial lung disease. Eur Respir Rev (2021) 30(161):210017. doi: 10.1183/16000617.0017-2021
124. Martinez FJ, Collard HR, Pardo A, Raghu G, Richeldi L, Selman M, et al. Idiopathic pulmonary fibrosis. Nat Rev Dis Primers (2017) 3:17074. doi: 10.1038/nrdp.2017.74
125. Wong AW, Ryerson CJ, Guler SA. Progression of fibrosing interstitial lung disease. Respir Res (2020) 21(1):32. doi: 10.1186/s12931-020-1296-3
126. Richeldi L, Collard HR, Jones MG. Idiopathic pulmonary fibrosis. Lancet (2017) 389(10082):1941–52. doi: 10.1016/s0140-6736(17)30866-8
127. Fujita Y, Araya J, Ito S, Kobayashi K, Kosaka N, Yoshioka Y, et al. Suppression of autophagy by extracellular vesicles promotes myofibroblast differentiation in copd pathogenesis. J extracellular vesicles (2015) 4:28388. doi: 10.3402/jev.v4.28388
128. Cores J, Hensley MT, Kinlaw K, Rikard SM, Dinh PU, Paudel D, et al. Safety and efficacy of allogeneic lung spheroid cells in a mismatched rat model of pulmonary fibrosis. Stem Cells Trans Med (2017) 6(10):1905–16. doi: 10.1002/sctm.16-0374
129. Bataller R, Brenner DA. Liver fibrosis. J Clin Invest (2005) 115(2):209–18. doi: 10.1172/jci24282
130. Ramachandran P, Henderson NC. Antifibrotics in chronic liver disease: Tractable targets and translational challenges. Lancet Gastroenterol Hepatol (2016) 1(4):328–40. doi: 10.1016/s2468-1253(16)30110-8
131. Shi WP, Ju D, Li H, Yuan L, Cui J, Luo D, et al. Cd147 promotes Cxcl1 expression and modulates liver fibrogenesis. Int J Mol Sci (2018) 19(4):1145. doi: 10.3390/ijms19041145
132. Modi D, Ye JC, Surapaneni M, Singh V, Chen W, Jang H, et al. Liver graft-Versus-Host disease is associated with poor survival among allogeneic hematopoietic stem cell transplant recipients. Am J Hematol (2019) 94(10):1072–80. doi: 10.1002/ajh.25575
133. Socié G. Treating chronic gvhd-induced fibrosis? Blood (2018) 131(13):1396–7. doi: 10.1182/blood-2018-02-830505
134. Bruno S, Herrera Sanchez MB, Pasquino C, Tapparo M, Cedrino M, Tetta C, et al. Human liver-derived stem cells improve fibrosis and inflammation associated with nonalcoholic steatohepatitis. Stem Cells Int (2019) 2019:6351091. doi: 10.1155/2019/6351091
135. Fasbender F, Widera A, Hengstler JG, Watzl C. Natural killer cells and liver fibrosis. Front Immunol (2016) 7:19. doi: 10.3389/fimmu.2016.00019
136. Neviani P, Wise PM, Murtadha M, Liu CW, Wu CH, Jong AY, et al. Natural killer-derived exosomal mir-186 inhibits neuroblastoma growth and immune escape mechanisms. Cancer Res (2019) 79(6):1151–64. doi: 10.1158/0008-5472.Can-18-0779
137. Nastase MV, Zeng-Brouwers J, Wygrecka M, Schaefer L. Targeting renal fibrosis: Mechanisms and drug delivery systems. Adv Drug Deliv Rev (2018) 129:295–307. doi: 10.1016/j.addr.2017.12.019
138. Romagnani P, Remuzzi G, Glassock R, Levin A, Jager KJ, Tonelli M, et al. Chronic kidney disease. Nat Rev Dis Primers (2017) 3:17088. doi: 10.1038/nrdp.2017.88
139. Aghajani Nargesi A, Lerman LO, Eirin A. Mesenchymal stem cell-derived extracellular vesicles for kidney repair: Current status and looming challenges. Stem Cell Res Ther (2017) 8(1):273. doi: 10.1186/s13287-017-0727-7
140. Varelas X, Samavarchi-Tehrani P, Narimatsu M, Weiss A, Cockburn K, Larsen BG, et al. The crumbs complex couples cell density sensing to hippo-dependent control of the tgf-B-Smad pathway. Dev Cell (2010) 19(6):831–44. doi: 10.1016/j.devcel.2010.11.012
141. Deng X, Xie Y, Zhang A. Advance of autophagy in chronic kidney diseases. Ren Fail (2017) 39(1):306–13. doi: 10.1080/0886022x.2016.1274662
142. Ma X, Takahashi Y, Wu W, Chen J, Dehdarani M, Liang W, et al. Soluble very low-density lipoprotein receptor (Svldlr) inhibits fibrosis in neovascular age-related macular degeneration. FASEB J (2021) 35(12):e22058. doi: 10.1096/fj.202101334R
143. Wu P, Zhang B, Ocansey DKW, Xu W, Qian H. Extracellular vesicles: A bright star of nanomedicine. Biomaterials (2021) 269:120467. doi: 10.1016/j.biomaterials.2020.120467
144. Qu Y, Zhang Q, Cai X, Li F, Ma Z, Xu M, et al. Exosomes derived from mir-181-5p-Modified adipose-derived mesenchymal stem cells prevent liver fibrosis Via autophagy activation. J Cell Mol Med (2017) 21(10):2491–502. doi: 10.1111/jcmm.13170
145. Yao Z, Li J, Wang X, Peng S, Ning J, Qian Y, et al. Microrna-21-3p engineered umbilical cord stem cell-derived exosomes inhibit tendon adhesion. J Inflamm Res (2020) 13:303–16. doi: 10.2147/jir.S254879
146. Chen L, Wang Y, Li S, Zuo B, Zhang X, Wang F, et al. Exosomes derived from gdnf-modified human adipose mesenchymal stem cells ameliorate peritubular capillary loss in tubulointerstitial fibrosis by activating the Sirt1/Enos signaling pathway. Theranostics (2020) 10(20):9425–42. doi: 10.7150/thno.43315
147. Shao L, Zhang Y, Pan X, Liu B, Liang C, Zhang Y, et al. Knockout of beta-2 microglobulin enhances cardiac repair by modulating exosome imprinting and inhibiting stem cell-induced immune rejection. Cell Mol Life Sci (2020) 77(5):937–52. doi: 10.1007/s00018-019-03220-3
148. Wang H, Wang B, Zhang A, Hassounah F, Seow Y, Wood M, et al. Exosome-mediated mir-29 transfer reduces muscle atrophy and kidney fibrosis in mice. Mol Ther (2019) 27(3):571–83. doi: 10.1016/j.ymthe.2019.01.008
149. Tang M, Chen Y, Li B, Sugimoto H, Yang S, Yang C, et al. Therapeutic targeting of Stat3 with small interference rnas and antisense oligonucleotides embedded exosomes in liver fibrosis. FASEB J (2021) 35(5):e21557. doi: 10.1096/fj.202002777RR
150. Tang TT, Lv LL, Wang B, Cao JY, Feng Y, Li ZL, et al. Employing macrophage-derived microvesicle for kidney-targeted delivery of dexamethasone: An efficient therapeutic strategy against renal inflammation and fibrosis. Theranostics (2019) 9(16):4740–55. doi: 10.7150/thno.33520
151. Zhu J, Lu K, Zhang N, Zhao Y, Ma Q, Shen J, et al. Myocardial reparative functions of exosomes from mesenchymal stem cells are enhanced by hypoxia treatment of the cells Via transferring microrna-210 in an Nsmase2-dependent way. Artif cells nanomedicine Biotechnol (2018) 46(8):1659–70. doi: 10.1080/21691401.2017.1388249
152. Tolomeo AM, Castagliuolo I, Piccoli M, Grassi M, Magarotto F, De Lazzari G, et al. Extracellular vesicles secreted by mesenchymal stromal cells exert opposite effects to their cells of origin in murine sodium dextran sulfate-induced colitis. Front Immunol (2021) 12:627605. doi: 10.3389/fimmu.2021.627605
153. Hu M, Wang Y, Liu Z, Yu Z, Guan K, Liu M, et al. Hepatic macrophages act as a central hub for relaxin-mediated alleviation of liver fibrosis. Nat Nanotechnology (2021) 16(4):466–77. doi: 10.1038/s41565-020-00836-6
154. Zhou Y, Liu S, Zhao M, Wang C, Li L, Yuan Y, et al. Injectable extracellular vesicle-released self-assembling peptide nanofiber hydrogel as an enhanced cell-free therapy for tissue regeneration. J Control Release (2019) 316:93–104. doi: 10.1016/j.jconrel.2019.11.003
155. Mardpour S, Ghanian MH, Sadeghi-Abandansari H, Mardpour S, Nazari A, Shekari F, et al. Hydrogel-mediated sustained systemic delivery of mesenchymal stem cell-derived extracellular vesicles improves hepatic regeneration in chronic liver failure. ACS Appl Materials Interfaces (2019) 11(41):37421–33. doi: 10.1021/acsami.9b10126
156. Yao J, Huang K, Zhu D, Chen T, Jiang Y, Zhang J, et al. A minimally invasive exosome spray repairs heart after myocardial infarction. ACS Nano (2021). doi: 10.1021/acsnano.1c00628
157. Ko KW, Park SY, Lee EH, Yoo YI, Kim DS, Kim JY, et al. Integrated bioactive scaffold with polydeoxyribonucleotide and stem-Cell-Derived extracellular vesicles for kidney regeneration. ACS nano (2021) 15(4):7575–85. doi: 10.1021/acsnano.1c01098
158. Sun L, Fan M, Huang D, Li B, Xu R, Gao F, et al. Clodronate-loaded liposomal and fibroblast-derived exosomal hybrid system for enhanced drug delivery to pulmonary fibrosis. Biomaterials (2021) 271:120761. doi: 10.1016/j.biomaterials.2021.120761
159. Gómez-Ferrer M, Amaro-Prellezo E, Dorronsoro A, Sánchez-Sánchez R, Vicente Á, Cosín-Roger J, et al. Hif-overexpression and pro-inflammatory priming in human mesenchymal stromal cells improves the healing properties of extracellular vesicles in experimental crohn's disease. Int J Mol Sci (2021) 22(20):11269. doi: 10.3390/ijms222011269
160. Tao L, Ma W, Wu L, Xu M, Yang Y, Zhang W, et al. Glial cell line-derived neurotrophic factor (Gdnf) mediates hepatic stellate cell activation Via Alk5/Smad signalling. Gut (2019) 68(12):2214–27. doi: 10.1136/gutjnl-2018-317872
161. Wang D, Quan Y, Yan Q, Morales JE, Wetsel RA. Targeted disruption of the B2-microglobulin gene minimizes the immunogenicity of human embryonic stem cells. Stem Cells Trans Med (2015) 4(10):1234–45. doi: 10.5966/sctm.2015-0049
162. Liang Y, Duan L, Lu J, Xia J. Engineering exosomes for targeted drug delivery. Theranostics (2021) 11(7):3183–95. doi: 10.7150/thno.52570
163. Walker S, Busatto S, Pham A, Tian M, Suh A, Carson K, et al. Extracellular vesicle-based drug delivery systems for cancer treatment. Theranostics (2019) 9(26):8001–17. doi: 10.7150/thno.37097
164. Chi X, Gatti P, Papoian T. Safety of antisense oligonucleotide and sirna-based therapeutics. Drug Discovery Today (2017) 22(5):823–33. doi: 10.1016/j.drudis.2017.01.013
165. Saw PE, Song EW. Sirna therapeutics: A clinical reality. Sci China Life Sci (2020) 63(4):485–500. doi: 10.1007/s11427-018-9438-y
166. Yuan P, Qiu X, Liu T, Tian R, Bai Y, Liu S, et al. Substrate-independent polymer coating with stimuli-responsive dexamethasone release for on-demand fibrosis inhibition. J Materials Chem B (2020) 8(34):7777–84. doi: 10.1039/d0tb01127d
167. Yin JQ, Zhu J, Ankrum JA. Manufacturing of primed mesenchymal stromal cells for therapy. Nat Biomed Eng (2019) 3(2):90–104. doi: 10.1038/s41551-018-0325-8
168. Hu M, Wang Y, Xu L, An S, Tang Y, Zhou X, et al. Relaxin gene delivery mitigates liver metastasis and synergizes with check point therapy. Nat Commun (2019) 10(1):2993. doi: 10.1038/s41467-019-10893-8
169. Wang C, Wang M, Xu T, Zhang X, Lin C, Gao W, et al. Engineering bioactive self-healing antibacterial exosomes hydrogel for promoting chronic diabetic wound healing and complete skin regeneration. Theranostics (2019) 9(1):65–76. doi: 10.7150/thno.29766
170. Murali VP, Holmes CA. Biomaterial-based extracellular vesicle delivery for therapeutic applications. Acta Biomater (2021) 124:88–107. doi: 10.1016/j.actbio.2021.01.010
171. Lou P, Liu S, Wang Y, Pan C, Xu X, Zhao M, et al. Injectable self-assembling peptide nanofiber hydrogel as a bioactive 3d platform to promote chronic wound tissue regeneration. Acta Biomater (2021) 135:100–12. doi: 10.1016/j.actbio.2021.08.008
172. Li Y, Meng H, Liu Y, Lee BP. Fibrin gel as an injectable biodegradable scaffold and cell carrier for tissue engineering. TheScientificWorldJournal (2015) 2015:685690. doi: 10.1155/2015/685690
173. Nassar W, El-Ansary M, Sabry D, Mostafa MA, Fayad T, Kotb E, et al. Umbilical cord mesenchymal stem cells derived extracellular vesicles can safely ameliorate the progression of chronic kidney diseases. Biomaterials Res (2016) 20:21. doi: 10.1186/s40824-016-0068-0
174. John AE, Joseph C, Jenkins G, Tatler AL. Covid-19 and pulmonary fibrosis: A potential role for lung epithelial cells and fibroblasts. Immunol Rev (2021) 302(1):228–40. doi: 10.1111/imr.12977
175. Gupta D, Zickler AM, El Andaloussi S. Dosing extracellular vesicles. Adv Drug Delivery Rev (2021) 178:113961. doi: 10.1016/j.addr.2021.113961
176. Gao L, Wang L, Wei Y, Krishnamurthy P, Walcott GP, Menasché P, et al. Exosomes secreted by hipsc-derived cardiac cells improve recovery from myocardial infarction in swine. Sci Transl Med (2020) 12(561):eaay1318. doi: 10.1126/scitranslmed.aay1318
177. Wiklander OP, Nordin JZ, O'Loughlin A, Gustafsson Y, Corso G, Mäger I, et al. Extracellular vesicle in vivo biodistribution is determined by cell source, route of administration and targeting. J Extracellular Vesicles (2015) 4:26316. doi: 10.3402/jev.v4.26316
Keywords: fibrosis, extracellular vesicles, exosomes, nanomedicine, biomaterials
Citation: Lv K, Wang Y, Lou P, Liu S, Zhou P, Yang L, Lu Y, Cheng J and Liu J (2022) Extracellular vesicles as advanced therapeutics for the resolution of organ fibrosis: Current progress and future perspectives. Front. Immunol. 13:1042983. doi: 10.3389/fimmu.2022.1042983
Received: 13 September 2022; Accepted: 30 September 2022;
Published: 20 October 2022.
Edited by:
Cuiping Zhang, The Military General Hospital of Beijing PLA, ChinaReviewed by:
Sara Shamdani, INSERM UMR-S-MD 1197, Hôpital Paul Brousse, FranceCopyright © 2022 Lv, Wang, Lou, Liu, Zhou, Yang, Lu, Cheng and Liu. This is an open-access article distributed under the terms of the Creative Commons Attribution License (CC BY). The use, distribution or reproduction in other forums is permitted, provided the original author(s) and the copyright owner(s) are credited and that the original publication in this journal is cited, in accordance with accepted academic practice. No use, distribution or reproduction is permitted which does not comply with these terms.
*Correspondence: Jingping Liu, bGl1amluZ3BpbmdAc2N1LmVkdS5jbg==
Disclaimer: All claims expressed in this article are solely those of the authors and do not necessarily represent those of their affiliated organizations, or those of the publisher, the editors and the reviewers. Any product that may be evaluated in this article or claim that may be made by its manufacturer is not guaranteed or endorsed by the publisher.
Research integrity at Frontiers
Learn more about the work of our research integrity team to safeguard the quality of each article we publish.