- 1State Key Laboratory of Animal Nutrition, Beijing Engineering Technology Research Center of Raw Milk Quality and Safety Control, College of Animal Science and Technology, China Agricultural University, Beijing, China
- 2Faculty of Veterinary and Animal Sciences, the University of Agriculture, Dera Ismail Khan, Pakistan
- 3Anhui Province Key Laboratory of Embryo Development and Reproduction Regulation, Anhui Province Key Laboratory of Environmental Hormone and Reproduction, School of Biological and Food Engineering, Fuyang Normal University, Fuyang, China
- 4Genome Analysis Laboratory of the Ministry of Agriculture, Agricultural Genomics Institute at Shenzhen, Chinese Academy of Agricultural Sciences, Shenzhen, China
Overproduction of reactive oxygen species (ROS) is a well-known phenomenon experienced by ruminants, especially during the transition from late gestation to successful lactation. This overproduction of ROS may lead to oxidative stress (OS), which compromises the immune and anti-inflammatory systems of animals, thus predisposing them to health issues. Besides, during the periparturient period, metabolic stress is developed due to a negative energy balance, which is followed by excessive fat mobilization and poor production performance. Excessive lipolysis causes immune suppression, abnormal regulation of inflammation, and enhanced oxidative stress. Indeed, OS plays a key role in regulating the metabolic activity of various organs and the productivity of farm animals. For example, rapid fetal growth and the production of large amounts of colostrum and milk, as well as an increase in both maternal and fetal metabolism, result in increased ROS production and an increased need for micronutrients, including antioxidants, during the last trimester of pregnancy and at the start of lactation. Oxidative stress is generally neutralized by the natural antioxidant system in the body. However, in some special phases, such as the periparturient period, the animal’s natural antioxidant system is unable to cope with the situation. The effect of rumen-protected limiting amino acids and choline on the regulation of immunity, antioxidative, and anti-inflammatory status and milk production performance, has been widely studied in ruminants. Thus, in the current review, we gathered and interpreted the data on this topic, especially during the perinatal and lactational stages.
1 Introduction
Under normal physiological conditions, the antioxidant system’s capacity to neutralize and eliminate reactive oxygen species (ROS) produced during metabolic activities is usually sufficient. Metabolic alterations during pregnancy and calving have been shown to increase ROS generation above the level that the antioxidant system can cope with (1, 2). When there is an imbalance between the generation of ROS and the availability of antioxidant molecules, oxidative stress arises, exposing cattle to a variety of illnesses (3, 4). An excessive generation of ROS results in lipid peroxidation, oxidative stress, tissue damage, and changes in the quantity of reduced glutathione (GSH), a key component of glutathione metabolism (3, 5). When the pro/antioxidant balance is disrupted, damage to the structure and function of cellular macromolecules (lipids, proteins, and nucleic acids) occurs, resulting in oxidative stress. A preponderance of oxidation over reduction processes leads to metabolic disorders and diseases in dairy cows (6). Maintaining redox homeostasis in dairy cows during the periparturient and peak lactation stages is therefore critical (4, 7, 8). Parturition-related oxidative stress may contribute to immunological and inflammatory abnormalities, which increase the risk of metabolic and infectious disorders (2, 9).
Metabolic stress during the periparturient period is another key factor that exposes animals to immune depression, abnormal regulation of the inflammatory response, and oxidative stress. During the periparturient period, metabolic stress causes excessive mobilization of lipids followed by oxidative stress (10). The oxidative stress compromises the immunity and inflammatory status in dairy cattle, as shown in Figure 1.
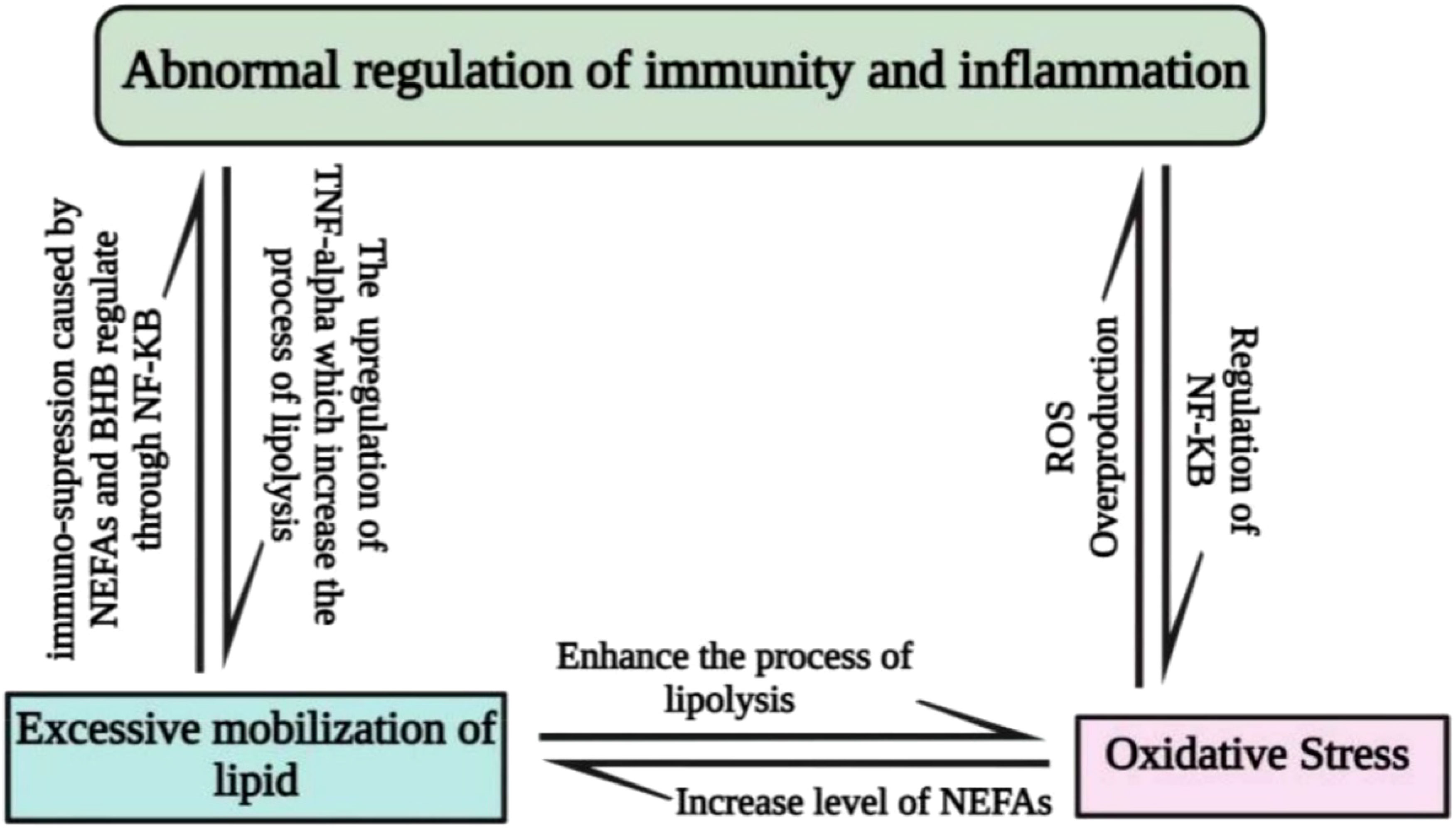
Figure 1 Interlink between metabolic stress, oxidative stress, immunity, and inflammation; nuclear factor kappa-B (NF-kB) signaling is used by oxidative stress to promote inflammation and immune system malfunction. Improper inflammatory regulation promotes the synthesis of tumor necrosis factor-alpha in non-phagocytic cells, leading to an excess of OS and excessive lipolysis. Oxidative stress increases the level of NEFAs, which is correlated with excessive lipid mobilization. The excessive lipid mobilization may elevate the level of NEFAs and BHB, which are the key factors in the abnormal regulation of the immune and inflammatory response.
Another critical factor is the abnormal regulation of immunity and inflammation caused by metabolic and oxidative stress (11–14), which predisposes dairy animals to various infections. In addition, the productive efficiency of animals is compromised by oxidative and metabolic stress, resulting in negative energy balance, immune suppression, and low productive efficiency (15–17). Several nutritional strategies have been adopted to overcome these issues during the periparturient period in ruminants.
The role of amino acids in the regulation of intestinal immunity and inflammation has been discussed previously (18). In brief, He et al. (18) reported that amino acid supplementation regulates the expression of anti-inflammatory cytokines and suppresses the apoptosis of enterocytes, as well as the expression of pro-inflammatory cytokines in intestinal inflammation. Furthermore, it was noted that several anti-inflammatory-related pathways, such as nuclear factor-kappa-B (NF-ϰB), mitogen-activated protein kinase (MAPK), inducible nitric oxide synthase (iNOS), mechanistic target of rapamycin (mTOR), nuclear erythroid-related factor 2 (Nrf2), and angiotensin-converting enzyme 2 (ACE2), are regulated by amino acid supplementation (18, 19).
Rumen-protected amino acid (methionine and lysine) and choline supplementation has been widely studied for its role in the maintenance of the antioxidant status, immunity status, and anti-inflammatory ability of dairy cattle (11, 20–25). Amino acids such as lysine, choline, and methionine are considered important sources of antioxidants in the diets of ruminants because of their positive role in relieving oxidative stress, which is associated with better health and productive efficiency (22, 23, 26). Thus, in the current review, we summarize the effect of rumen-protected first-limiting amino acids (methionine and lysine) and choline on the regulation of immunity, antioxidative, and anti-inflammatory status, especially during the periparturient period in dairy cattle in which they experience severe oxidative stress followed by depressed immunity and anti-inflammatory status.
2 Effect of rumen-protected first-limiting amino acids (methionine and lysine) and choline on the antioxidant, immunity, anti-inflammatory, and health status of ruminants
2.1. Effect of rumen-protected first-limiting amino acids (methionine and lysine) and choline supplementation on the antioxidant status of ruminants
2.1.1. Effect of methionine and choline supplementation on the antioxidant status of ruminants
The availability of key methyl donors, such as methionine and choline, is limited due to extensive microbial degradation in the peripartal dairy cow (27). If, for any reason, an imbalance of negative methyl donor occurs it will compromise the synthesis of critical compounds, such as carnitine and phosphatidylcholine (PC), which are required for methyl donors in cells and tissues (28). The biosynthesis of PC from endogenous supplementation of methionine and choline could prevent the incoming fatty acids produced from the lipolysis of adipose tissue. It has been documented that the disproportionate infiltration of hepatic fatty acid can negatively influence the normal function of the liver (29). Moreover, the excessive fat infiltration in the liver may suppress intracellular antioxidants, such as glutathione (GSH) and alternatively oxidative stress, which may lead to extensive tissue damage (11, 30, 31). The methyl donors are considered the most important extracellular source of the antioxidants glutathione (GSH) and taurine (32). During the periparturient period, the increased oxidative stress enhances inflammation, resulting in suppressed leukocyte responses. To overcome these challenges and for cells to synthesize sulfur-containing antioxidants, the supplementation of methionine and choline could be beneficial.
Rumen-protected limiting amino acids, such as methionine, are necessary for the biosynthesis of S-adenosylmethionine (SAM) (33). SAM is required for many biological processes, such as transsulfuration, polyamine biosynthesis, and DNA methylation (34). Thus, the need for methyl donors, including choline and methionine, is increased at the onset of the lactation period (35, 36). It has been well-documented that DNA methylation regions are associated with the regulation of gene expression. These epigenetic changes are partly driven by betaine and methionine through SAM. A study documented that rumen-protected choline enhances the expression of genes associated with the synthesis of betaine, phosphatidylcholine, acetylcholine, muscarinic, and nicotinic acetylcholine receptors (37). Interestingly, betaine has been found to work as a methyl donor and help in the regeneration of methionine and SAM (38). In addition, phosphatidylcholine has been found to be involved in the export of low-density protein, which is followed by a decrease in hepatic fat accumulation (39). Furthermore, a study found that methionine supplementation is necessary for the regulation of the nuclear receptor peroxisome proliferator-activated receptor alpha (PPARα), which plays a key role in reducing the inflammatory response, improving fatty acid oxidation, and preventing liver triacylglycerol (TAG) accumulation (40).
According to the available literature, rumen-protected choline and methionine are considered as valuable antioxidants in ruminant nutrition (41). Recent evidence suggests that methionine plays a role in glutathione formation (42), which could improve the antioxidant status of both animals and their products. Consistently, a study reported that methionine alone or in combination with choline alleviates oxidative stress by enhancing glutathione transferase (GST) activity in the plasma of periparturient ewes (43). Furthermore, another study documented that methionine in combination with choline enhances glutathione and amino acid levels in dairy cows during the perinatal period (44).
2.1.2. Effect of methionine and lysine supplementation on the antioxidant status of ruminants
Methionine is attacked by many of the ROS generated in biological systems and is considered one of the more readily oxidized residues in proteins (45–47). Methionine has a scavenging ability, and various ROS combine with methionine to form methionine sulfoxide. The cells contain methionine sulfoxide reductases, which catalyze the thioredoxin-dependent reduction of methionine sulfoxide back to methionine (47). Methionine acts as a catalytic antioxidant and protects protein and macromolecules from the action of ROS in which they are present.
The antioxidant role of lysine and methionine has been widely studied in ruminants (48–51). Furthermore, it has been highlighted that amino acids enhance metabolism, antioxidant status, and immunity to improve production and disease resistance. Consistent with this, it has been well established that amino acids play a key role in cellular oxidative balance (52) because they participate in taurine and glutathione (GSH) biosynthesis (53–55). GSH using glutathione transferase (GST) and hydrogen peroxide neutralization via glutathione peroxidase (GSH-Px) causes cellular detoxification. Glutathione transferases (GSTs, EC 2.5.1.18) are some of the most important antioxidant enzymes for regulating the cell’s redox state (56–58). Similarly, a reduced level of glutathione (a potent intracellular antioxidant) was reported in liver tissue in response to methionine treatment (59).
It has been reported that methionine supplementation enhances very low-density lipoprotein (VLDL) to promote vitamin E in circulation (60). Consequently, the detrimental effect of lipid peroxidation by-products, such as malondialdehyde (MDA), can be suppressed with supplementation with rumen-protected amino acids (60). Furthermore, antioxidant systems, which can be split into enzymatic and non-enzymatic (e.g., metabolites), control ROS (56, 61). The effect of methionine and lysine supplementation on oxidative stress has been studied in ewes (21). It was further noted that methionine supplementation reduces the expression of MDA and improves the level of superoxide dismutase (SOD), CAT, GPH, and GST in the plasma of ewes (21).
A few studies, through in vitro experiments, reported that methionine supplementation decreases the process of apoptosis and necrosis and inhibits lipid peroxidation in the bovine mammary glands (62, 63). Moreover, they documented that methionine supplementation enhances the level of antioxidant-associated genes (superoxide dismutase and glutathione peroxidase) and the cytoprotective effect against hyperthermia (62, 63).
2.2. Effect of methionine and lysine supplementation on inflammation and immunity in ruminants
Parturition has been characterized as the stage of inflammatory change during which the level of haptoglobin is elevated, thus decreasing the concentration of albumin (64). Moreover, albumin levels have been reported to decrease around parturition and are also considered as a key biomarker for inflammation in dairy cattle. The higher the level of albumin, especially during the periparturient period, the healthier the animal (65). A higher concentration of albumin has been reported in response to methionine supplementation in dairy cattle (64). Moreover, by binding to non-esterified fatty acids and bilirubin, albumin takes on a detoxifying function and alleviates inflammation (66), which is the critical factor that exposes periparturient dairy cattle to infections. Haptoglobin is another key acute-phase protein, which usually increases around parturition because of inflammatory events and parenchymal cell stimulation by fatty acid infiltration of the liver. Furthermore, it has been demonstrated that methionine supplementation significantly downregulates the level of haptoglobin, which is associated with improved antioxidant and anti-inflammatory levels during parturition in dairy cattle (64). Furthermore, Zhou et al. (64) found that dairy cattle supplemented with methionine were less susceptible to infections during the periparturient period because of suppressed inflammatory changes and improved antioxidant status.
Previous studies have proved experimentally that methionine supplementation enhances the anti-inflammation and anti-oxidative status in periparturient dairy cattle (59, 67, 68) and neonatal calves (69). In a recent study, Hu et al. reported that methionine and arginine significantly regulates milk protein synthesis, thereby alleviating a potential inflammatory and pro-oxidant state in transitioning dairy cattle (70). In addition, a few studies have reported that the supplementation of methionine with arginine plays an important role in anti-inflammation and improves the antioxidant status of transition dairy cattle (68, 71). Moreover, methionine and arginine supplementation has been associated with the regulation of immunity and relief of oxidative stress caused by bacterial lipopolysaccharide (LPS) in bovine mammary cells (71).
It has been reported that rumen-protected lysine and methionine feeding before parturition to transition dairy cows may affect the immunity of calves (69). In an experimental trial, it has been reported that the offspring (calves) of rumen-protected methionine and lysine-fed cows show higher passive immunity, including a higher concentration of immunoglobulin G, higher serum total protein, and a higher growth rate than an unsupplemented group of dairy cows (69). Lee et al. (72) reported that supplementation with lysine and methionine positively reduces milk somatic cell count (SCC) and improves the immunity and health status of dairy cattle. Consistent with this, a study proved experimentally that methionine supplementation tends to decrease metabolic stress and milk SCC and improve udder health in goats (73).
2.3. Effect of methionine and choline supplementation on the inflammation and immunity of ruminants
It has been well established through experimental trials that treatment with a combination of choline and methionine can regulate the antioxidative state, thereby increasing the anti-inflammatory and cytoprotective effect against oxidative stress in neonatal Holstein calves (74). Consistent with this, a study reported that supplementation with choline and methionine improves the immunometabolic state, blood polymorphonuclear leukocyte phagocytosis capacity, and the anti-inflammatory effect upon pathogen challenge, and enhances the antioxidative capacity of peripartal cows (75). During the periparturient period, the liver functionality index (LFI) is the key indicator that is used to assess immune and inflammatory status, as well as metabolic profiles in dairy cattle (44, 59, 76). In addition, a study reported that a low LFI and low amino acids in circulating plasma indicates a difficult transition from gestation to lactation (30). The supplementation of a combination of choline and methionine enhances the level of the LFI, resulting in an improved immunity and anti-inflammatory status in dairy cattle (44). Moreover, ketosis was also found to be positively associated with a low LFI, while combined supplementation of choline and methionine significantly reduces the chances of ketosis by alleviating oxidative stress in dairy cattle (77).
Choline supplementation in the transition diet may be partially useful for reducing the deleterious effects of an inflammatory-like condition on the hepatic function of transition cows. The non-esterified fatty acids (NEFA) and β-hydroxybutyric acid (BHB) may lead to oxidative stress followed by an enhancement of the lipolysis process (78). Additionally, rumen-protected choline supplementation has been found to reduce the concentration of liver triacylglycerol and metabolic stress, resulting in an improved immune and antioxidative state (79, 80). Consistent with this, choline supplementation also lowers BHBH levels and the body condition score, which is the best indicator of health in dairy cattle during the periparturient period (81, 82). Furthermore, it has been reported that excessive production of NEFA and BHB may compromise immunity, resulting in abnormal regulation of immune and inflammation responses (83). The high levels of NEFA and BHB followed by oxidative stress and dysfunctional immunity have been reported as major contributory factors of mastitis in dairy cattle (10). Several studies have demonstrated that choline supplementation significantly reduces the level of NEFA and BHB, resulting in enhanced immunity and maintenance of the anti-inflammatory state in dairy cows (84–87).
During early lactation, catabolic changes are initiated and lead to the utilization of body energy reserves (non-esterified fatty acids and amino acids). The excessive utilization of NEFA is incorporated into VLDL by the liver. A high level of VLDL is associated with oxidative stress and fatty liver syndrome (88). Furthermore, supplementation of rumen-protected choline enhances the function of the liver by improving VLDL exportation from the liver and relieving oxidative stress (88). By increasing the expression of fatty acid transport protein 5 and carnitine transporter SLC22A5 in the liver and endorsing apo B-containing lipoprotein assembly, rumen-protected choline supplementation reduces the harmful effects of hepatic lipidosis in periparturient dairy cattle (89). Consistent with this, a study reported that choline significantly decreases the level of postpartal liver TAG and enhances the biosynthesis of VLDL through the regulation of PPARα targets apolipoprotein B (APOB) and microsomal triglyceride transfer protein (MTTP) (89).
It has been well demonstrated that heat stress compromises immunity and regulates the inflammatory system in an abnormal way, affecting the production performance of dairy cattle (90, 91). Nutritional intervention with methionine and choline has been reported to enhance the immune response to heat stress and play a key role in improving the health of animals (92–94). Furthermore, choline supplementation improves lipid and energy metabolism and alleviates the inflammatory response (95). Rumen-protected choline feeding during the periparturient period is the key focus because of its key role in preventing liver lipid accumulation through VLDL export. Moreover, it has been reported that choline plays an important role in the regulation of immune function and also mitigates inflammatory changes in transition dairy cows (93), enhances immune function in calves (94), and adjusts the responses of immune cells to LPS ex vivo (37). Consequently, it has been documented that choline deficiency may lead to intestinal morphology and lipid metabolism impairment in rats (96). The antioxidant, anti-inflammatory and immune regulatory role of limiting amino acids (methionine and lysine) and choline has been summarized in Table 1. Based on the available data, we concluded that choline supplementation maintains the inflammatory response in immune cells and relieves metabolic stress during the periparturient period in dairy cattle.
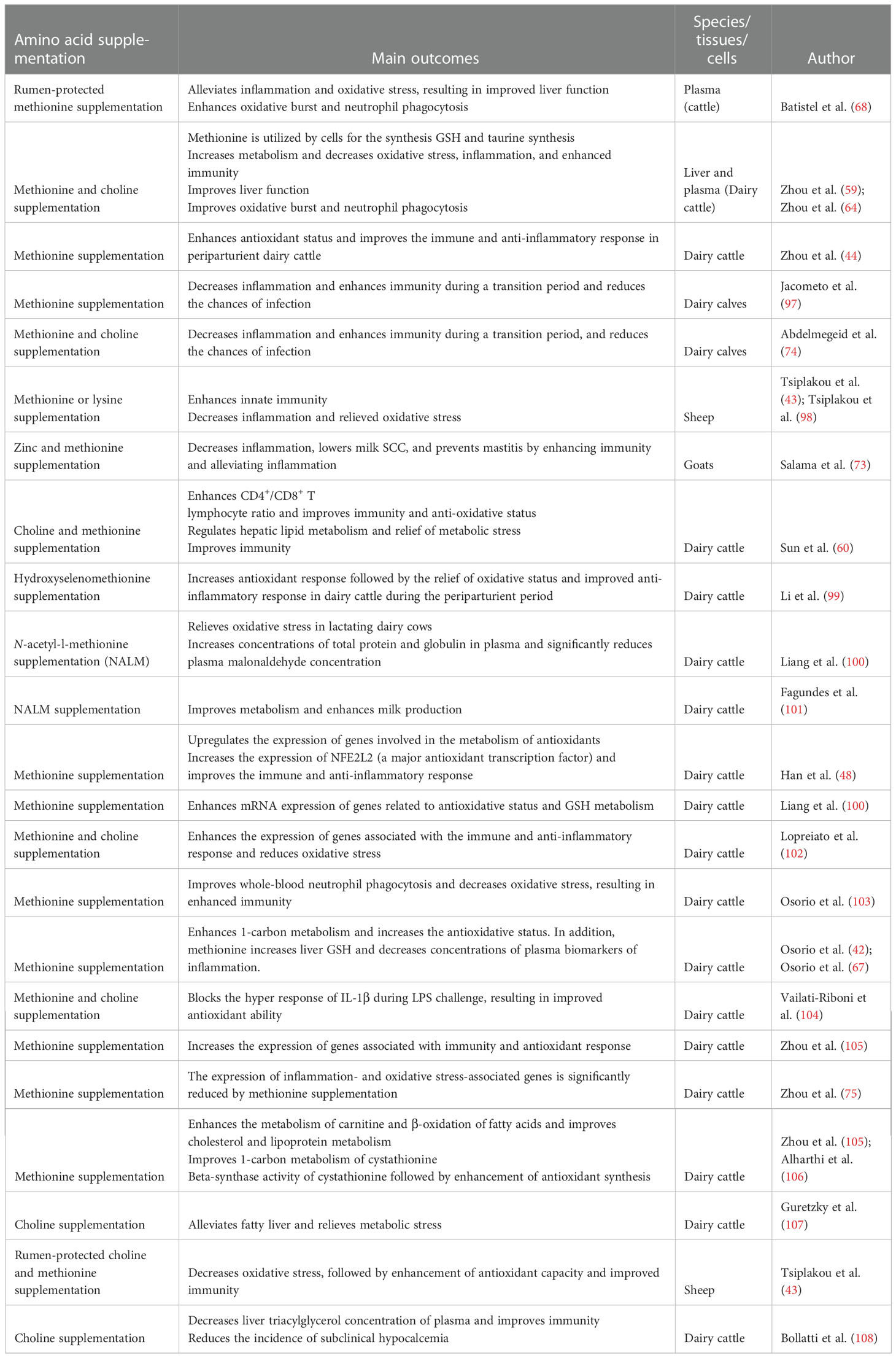
Table 1 Summary of studies in dairy ruminants investigating the influence of rumen-protected limiting amino acids (methionine and lysine) and choline on immune function and the oxidative and anti-inflammatory status of ruminants.
2.4. Rumen-protected limiting amino acids (methionine and lysine) and choline-regulated genes are associated with the immunity, antioxidant, and anti-inflammatory status of ruminants
The molecular response associated with health regulation in response to rumen-protected limiting amino acids in dairy ruminants has been well documented (21). It has been reported that heat stress upregulates miR-34a, miR-92a, miR-99, and miR-184. In addition, the upregulation of microRNA in the mammary gland by heat stress is related to cell growth arrest and apoptosis and inhibition of fat synthesis (109). Consistent with this, it has been documented that miR-34a overexpression induces apoptosis in Hep2 cells (110) and mammary cells (111) and inhibits cell proliferation (110). Salama et al. proved through experimental trials that methionine and arginine supplementation downregulates the expression of miR-34a, miR-92a, miR-99, and miR-184 in the mammary gland. Furthermore, they documented that methionine and arginine treatment regulates most of the genes that are associated with insulin signaling (AKT2 and IRS1), transcription and translation (MAPK1, MTOR, SREBF1, RPS6KB1, and JAK2), amino acid transport (SLC1A5 and SLC7A1), and cell proliferation (MKI67) in the mammary glands of dairy cattle (109). Heat stress also upregulates genes associated with apoptosis (BAX), translation inhibition (EIF4EBP1), and lipogenesis (PPARG, FASN, and ACACA) and decreases the expression of the anti-apoptotic gene BCL2L1 in the mammary glands of dairy cattle (109). The above effects caused by heat were reversed with methionine and arginine supplementation in dairy cattle (109).
71, reported that bacterial LPS significantly downregulates the expression of SLC36A1 and SLC7A1 and genes associated with antioxidant response (NFE2L2, NQO1, GPX1, ATG7, and GPX3), and upregulates SOD2 and NOS2 (71). Furthermore, they noticed that arginine and methionine supplementation enhances antioxidative gene expression and increases the signaling level of NFE2L2 in mammary gland cells. Ma et al. (112) found that NFE2L2 signaling plays a critical role in the cellular antioxidant defense system. It might be possible that arginine and methionine treatment significantly enhances the antioxidative response in mammary gland cells via the NFE2L2 pathway (112). Consistent with this, it has been found that methionine-treated mammary gland cells show increased expression of CSN1S1, CSN1S2, CSN2, CSN3, LALBA, JAK2, STAT5, and MTOR, which are positively linked to milk protein synthesis (113, 114). Methionine supplementation significantly regulates the transsulfuration pathways that play a key role in taurine and glutathione biosynthesis. Taurine and glutathione biosynthesis alleviates the oxidative stress that is caused by negative energy balance in neonatal Holstein calves (115), as demonstrated in Figure 2.
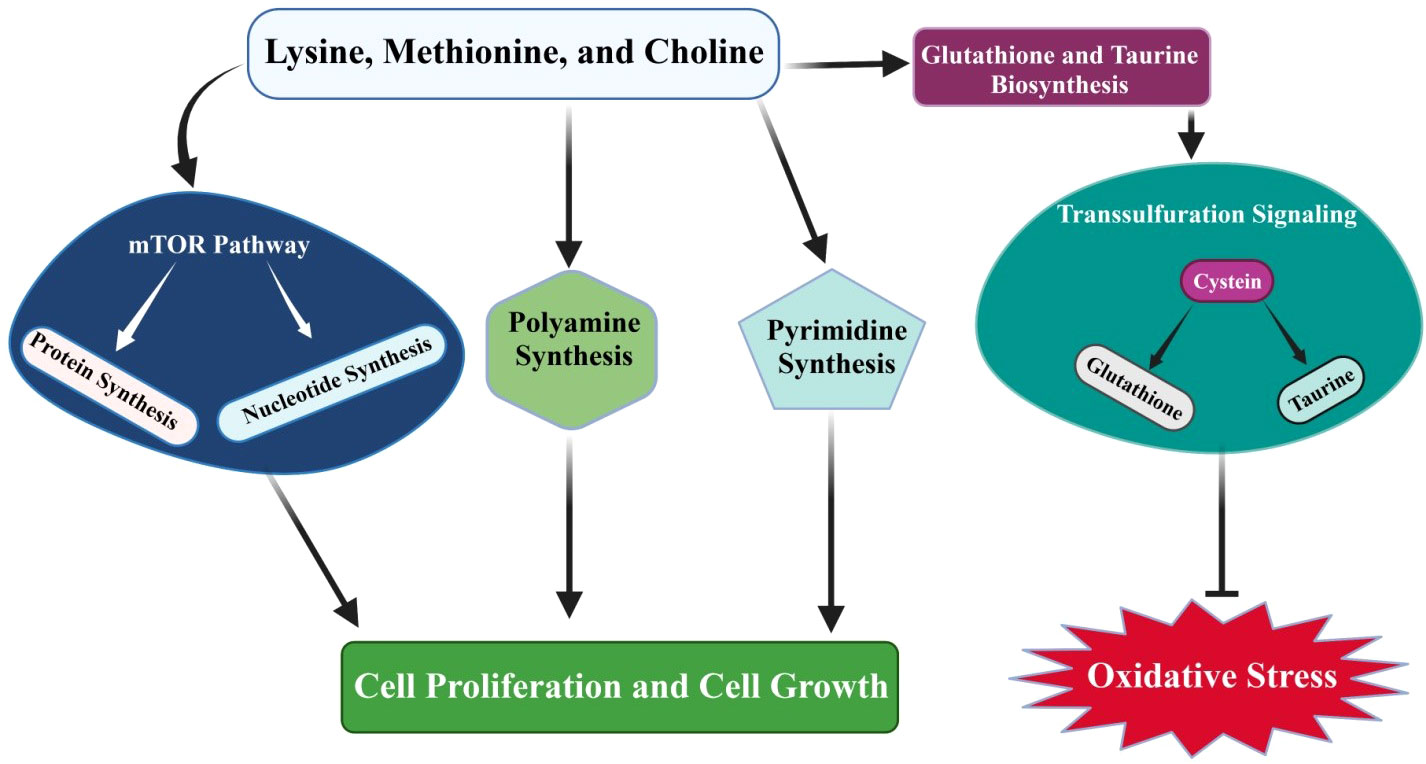
Figure 2 Rumen-protected limiting amino acids (lysine and methionine) and choline supplementation regulate mTOR signaling, which controls protein and nucleotide synthesis. In addition, amino acids mediate transsulfuration pathways that further facilitate taurine and glutathione biosynthesis. Taurine and glutathione biosynthesis plays a key role in alleviating oxidative stress in dairy cattle. Additionally, amino acid supplementation regulates mTOR signaling and pyrimidine and polyamine synthesis, and thus plays an essential role in controlling cell proliferation and growth processes.
Garcia et al. experimentally proved that choline supplementation regulates the genes that are associated with muscarinic and nicotinic acetylcholine (37). The muscarinic and nicotinic acetylcholine receptors have been detected in innate and adaptive immune cells (116). Garcia et al. identified several genes involved in choline metabolism (SLC5A7, CHDH, CHKA, ACHE, CHRM5, and CHRNA7) and inflammatory responses (TLR4, NFKB1, TNFA, ELANE, H2A, CASP3, and CASP7) in the neutrophils and monocytes of lactating dairy cattle, which are regulated in response to rumen-protected choline supplementation (37). Inflammatory genes (TLR4, NFKB1, and TNFA) are significantly upregulated in LPS-challenged immune cells; however, the opposite trend is observed for these genes in choline-treated immune cells (37).
Methionine supplementation elevates the expression of genes associated with inflammation (IL1B, TLR2, NF-κB, and STAT3) and oxidative stress (glutathione synthase, GPX1, CBS, and SOD2) in polymorphonuclear leukocytes (PMNLs) and enhances taurine in the plasma of dairy cattle (75). These findings suggest that methionine supplementation improves the anti-inflammatory and antioxidative status of periparturient dairy cattle. Furthermore, PMNLs treated with LPS reveal the hyper response of IL-1β, resulting in oxidative stress (104). Rumen-protected methionine supplementation suppresses the hyper response of IL-1β, resulting in a decreased inflammatory response and oxidative stress (104). Consistent with this, it has been documented that the combination of methionine and choline significantly upregulates the expression of genes involved in pathogen recognition mechanisms (TLR2 and L-selectin [SELL]) and lowers the expression of genes associated with inflammation (cysteine sulfinic acid decarboxylase [CSAD], cystathionine gammalyase [CTH], myeloperoxidase [MPO], glutathione reductase [GSR], GSS, IL6, IL10, and IL1B), resulting in an improved anti-inflammatory and antioxidative status in dairy cattle (102). Additionally, methionine downregulates the expression of several genes potentially associated with oxidative stress (SOD1, GSS, and GCLC) (42). Furthermore, Osorio et al. (42) reported higher expression of haptoglobin (HP), S-adenosylhomocysteine hydrolase (SAHH), adenosyltransferase 1A (MAT1A) cytosine-5-methyltransferase 3 alpha (DNMT3A), and DNMT1 in response to methionine supplementation. Furthermore, they reported lowered oxidative stress and a mild inflammatory status in dairy cattle (42). Consistent with this, methionine and lysine combination treatment significantly reduces the level of TLR4, pro-inflammatory cytokines (TNF-α and IL-1β), chemokine (CXCL-16), and BHBA content in ewes (117). The summary of the genetic response to rumen-protected limiting amino acids (lysine and methionine) and choline is shown in Table 2.
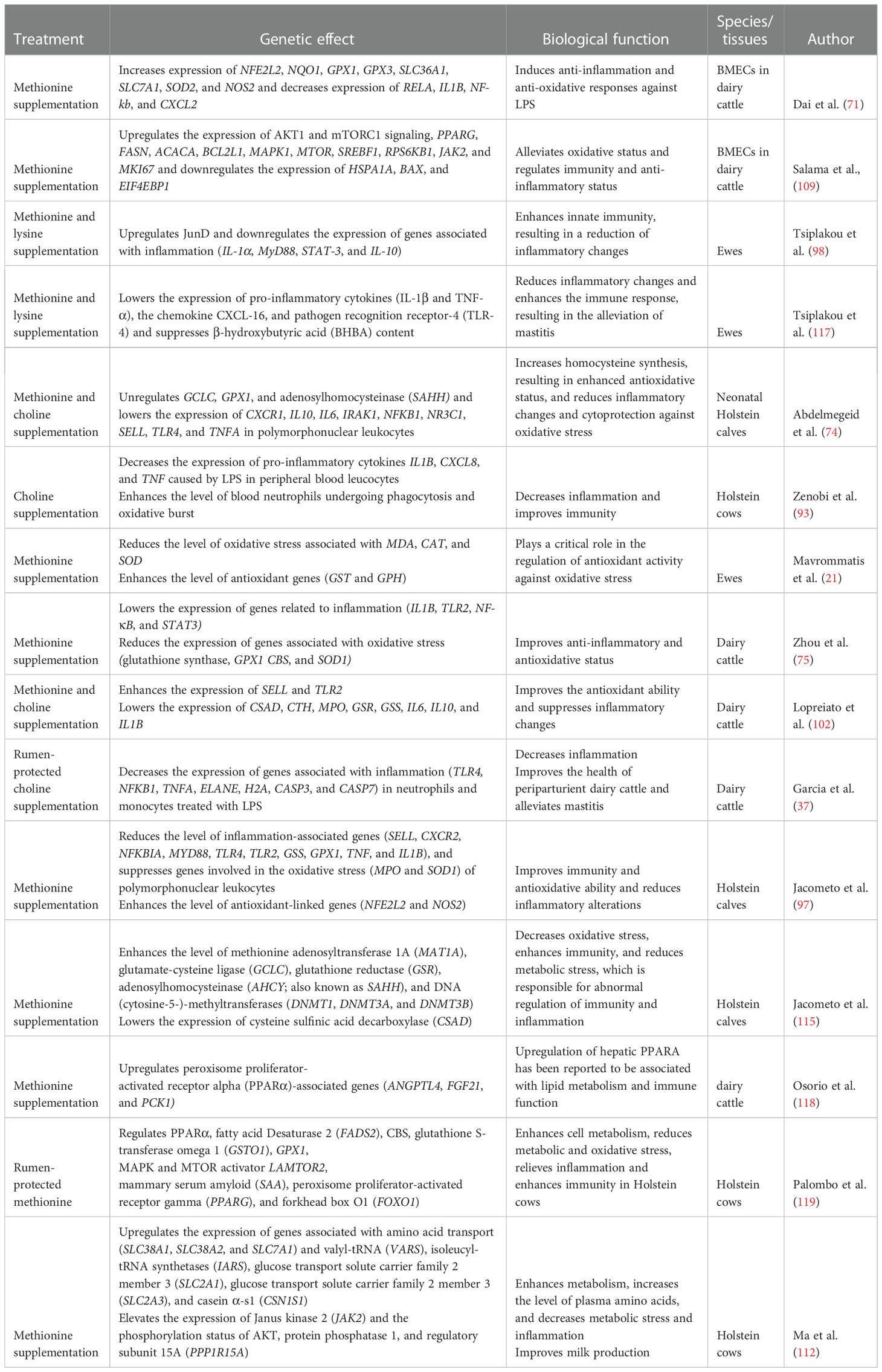
Table 2 Summary of studies that investigated the rumen-protected limiting amino acid (methionine and lysine)- and choline-regulated genes associated with the immunity, antioxidative, and anti-inflammatory status of dairy ruminants.
3 Conclusions
Based on published data, we conclude that rumen-protected limiting amino acids (lysine and methionine) and choline supplementation alleviate oxidative stress, which is the primary cause of several diseases in ruminants. Moreover, oxidative stress, particularly during the periparturient period, compromises immunity, production performance, and metabolism in dairy ruminants. The supplementation of rumen-protected amino acids and choline, especially during the periparturient period, enhances antioxidative ability, resulting in the regulation of immunity and anti-inflammation status in dairy ruminants. Thus, supplementation with a sufficient quantity of rumen-protected amino acids (lysine and methionine) and choline is highly recommended for protecting animals from diseases and enhancing their productive abilities.
Author contributions
MK, MM, and ZC designed the study and wrote the manuscript; ZC supervised the manuscript; MM, YM, JW, QU, JX, TC, SL, IMK and AK helped in collection of data resources and editing of final version of manuscript. All authors contributed to the article and approved the submitted version.
Funding
This work was supported by the China Animal Husbandry Group (DR201905) and the 2115 Talent Development Program of China Agricultural University (2115). The funders had no role in the study design, data collection, analysis, decision to publish, or preparation of the manuscript.
Acknowledgments
We are thankful to the 2115 Talent Development Program of China Agricultural University (Beijing, P. R. China) and the China Animal Husbandry Group (DR201905) for their financial support.
Conflict of interest
The authors declare that the research was conducted in the absence of any commercial or financial relationships that could be construed as a potential conflict of interest.
Publisher’s note
All claims expressed in this article are solely those of the authors and do not necessarily represent those of their affiliated organizations, or those of the publisher, the editors and the reviewers. Any product that may be evaluated in this article, or claim that may be made by its manufacturer, is not guaranteed or endorsed by the publisher.
References
1. Sordillo LM. Factors affecting mammary gland immunity and mastitis susceptibility. Livest Prod Sci (2005) 98:89–99. doi: 10.1016/j.livprodsci.2005.10.017
2. Mavangira V, Sordillo LM. Role of lipid mediators in the regulation of oxidative stress and inflammatory respons-es in dairy cattle. Res Vet Sci (2018) 116:4–14. doi: 10.1016/j.rvsc.2017.08.002
3. Mikulková K, Kadek R, Filípek J, Illek J. Evaluation of oxidant/antioxidant status, metabolic profile and milk production in cows with metritis. Irish Vet J (2020) 73(2020):1–11. doi: 10.1186/s13620-020-00161-3
4. Castillo C, Hernandez J, Valverde I, Pereira V, Sotillo J, Alonso ML, et al. Plasma malonaldehyde (MDA) and total antioxidant status (TAS) during lactation in dairy cows. Res vet Sci (2006) 80(2):133–9. doi: 10.1016/j.rvsc.2005.06.003
5. Wullepit N, Raes K, Beerda B, Veerkamp RF, Fremaut D, De Smet S. Influence of management and genetic merit for milk yield on the oxidative status of plasma in heifers. Livest Sci (2009) 123(2-3):276–82. doi: 10.1016/j.livsci.2008.11.013
6. Sharma N, Singh N, Singh O, Pandey V, Verma P. Oxidative stress and antioxidant status during transition period in dairy cows. Asian-Australas J Anim Sci (2011) 24:479–84. doi: 10.5713/ajas.2011.10220
7. Kowalska J, Jankowiak D. Changes of reduction-oxidation balance in pregnant ruminants. Postepy biochemii (2009) 55(3):323–8.
8. Markiewicz H, Gehrke M, Malinowski E, Kaczmarowski M. Evaluating the antioxidant potential in the blood of tran-sition cows. Med Weterynaryjna (2005) 61:1382–4.
9. Mavangira VMJ, Mangual JC, Gandy L, Sordillo M. 15-F2t-Isoprostane concentrations and oxidant status in lactating dairy cattle with acute coliform mastitis. J Vet Intern Med (2016) 30:339–47. doi: 10.1111/jvim.13793
10. Khan MZ, Ma Y, Xiao J, Chen T, Ma J, Liu S, et al. Role of selenium and vitamins e and B9 in the alleviation of bovine mastitis during the periparturient period. Antioxidants (2022) 11(4):657. doi: 10.3390/antiox11040657
11. Sordillo LM, Aitken SL. Impact of oxidative stress on the health and immune function of dairy cattle. Vet Immunol Immunopathol (2009) 128:104–9. doi: 10.1016/j.vetimm.2008.10.305
12. Agrawal A, Khan MJ, Graugnard DE, Vailati-Riboni M, Rodriguez-Zas SL, Osorio JS, et al. Prepartal energy intake alters blood polymorphonuclear leukocyte transcriptome during the peripartal period in Holstein cows. Bioinform Biol Insights (2017) 11:1177932217704667. doi: 10.1177/1177932217704667
13. Mann S, Sipka AS, Grenier JK. The degree of postpartum metabolic challenge in dairy cows is associated with peripheral blood mononuclear cell transcriptome changes of the innate immune system. Dev Comp Immunol (2019) 93:28–36. doi: 10.1016/j.dci.2018.11.021
14. Mann S, Sipka A, Leal Yepes FA, Nydam DV, Overton TR, Wakshlag JJ. Nutrient-sensing kinase signaling in bovine immune cells is altered during the postpartum nutrient deficit: a possible role in transition cow inflammatory response. J Dairy Sci (2018) 101:9360–70. doi: 10.3168/jds.2018-14549
15. Yin FG, Zhang ZZ, Ju H, Yin YL. Digestion rate of dietary starch affects systemic circulation of amino acids in weaned pigs. Br J Nutr (2010) 103(10):1404. doi: 10.1017/S0007114509993321
16. Zhou XH, He LQ, Wan D, Yang HS, Yao K, Wu GY, et al. Methionine restriction on lipid metabolism and its possible mechanisms. Amino Acids (2016) 48(7):1533–40. doi: 10.1007/s00726-016-2247-7
17. Xiao J, Khan MZ, Ma Y, Alugongo GM, Ma J, Chen T, et al. The antioxidant properties of selenium and vitamin e; their role in periparturient dairy cattle health regulation. Antioxidants (2021) 10:1555. doi: 10.3390/antiox10101555
18. He F, Wu C, Li P, Li N, Zhang D, Zhu Q, et al. Functions and signaling pathways of amino acids in intestinal inflammation. BioMed Res Int (2018) 2018:13. doi: 10.1155/2018/9171905
19. Andou A, Hisamatsu T, Okamoto S, Chinen H, Kamada N, Kobayashi T, et al. Dietary histidine ameliorates murine colitis by inhibition of pro-inflammatory cytokine production from macrophages. Gastroenterology (2009) 136(2):564–74. doi: 10.1053/j.gastro.2008.09.062
20. Catanesi M, Brandolini L, d’Angelo M, Benedetti E, Tupone MG, Alfonsetti M, et al. L-methionine protects against oxidative stress and mitochondrial dysfunction in an In vitro model of Parkinson ‘s disease. Antioxidants (2021) 10(9):1467.
21. Mavrommatis A, Mitsiopoulou C, Christodoulou C, Kariampa P, Simoni M, Righi F, et al. Effects of supplementing rumen-protected methionine and lysine on milk performance and oxidative status of dairy ewes. Antioxidants (2021) 10(5):654. doi: 10.3390/antiox10050654
22. Ratika K, James Singh KR, Dahiya SS. Methionine, lysine and choline in dairy cows: a review article. Int J Curr Microbiol App Sci (2018) 7(7):3921–34. doi: 10.20546/ijcmas.2018.707.456
23. Cho I-J, Kim D, Kim E-O, Jegal K-H, Kim J-K, Park S-M, et al. Cystine and methionine deficiency promotes ferroptosis by inducing b-cell translocation gene 1. Antioxidants (2021) 10:1543. doi: 10.3390/antiox10101543
24. Kung L Jr., Rode LM. Amino acid metabolism in ruminants. Anim Feed Sci Technol (1996) 59:167–72. doi: 10.1016/0377-8401(95)00897-7
25. Castillo C, Pereira V, Abuelo A, Hernández J. Effect of supplementation with antioxidants on the quality of bovine milk and meat production. Sci World J (2013), 616098. doi: 10.1155/2013/616098
26. Martínez Y, Li X, Liu G, Peng Bin P, Yan W, Dairon M, et al. The role of methionine on metabolism, oxidative stress, and diseases. Amino Acids (2017) 49(12):2091–8. doi: 10.1007/s00726-017-2494-2
27. Girard CL, Matte JJ. Effects of intramuscular injections of vitamin B12 on lactation performance of dairy cows fed dietary supplements of folic acid and rumen-protected methionine. J dairy Sci (2005) 88(2):671–6. doi: 10.3168/jds.S0022-0302(05)72731-4
28. Pinotti L, Baldi A, Dell'Orto V. Comparative mammalian choline metabolism with emphasis on the high-yielding dairy cow. Nutr Res Rev (2002) 15(2):315–32. doi: 10.1079/NRR200247
29. Drackley JK. Biology of dairy cows during the transition period: The final frontier? J dairy Sci (1999) 82(11):2259–73. doi: 10.3168/jds.S0022-0302(99)75474-3
30. Trevisi E, Amadori M, Cogrossi S, Razzuoli E, Bertoni G. Metabolic stress and inflammatory response in high-yielding, periparturient dairy cows. Res Vet Sci (2012) 93:695–704. doi: 10.1016/j.rvsc.2011.11.008
31. Bertoni G, Trevisi E. Use of the liver activity index and other metabolic variables in the assessment of metabolic health in dairy herds. Vet Clin North Am Food Anim Pract (2013) 29(2):413–31. doi: 10.1016/j.cvfa.2013.04.004
32. Brosnan JT, Brosnan ME. The sulfur-containing amino acids: An overview. J Nutr (2006) 136:1636S–40S. doi: 10.1093/jn/136.6.1636S
33. Martinov MV, Vitvitsky VM, Banerjee R, Ataullakhanov FI. The logic of the hepatic methionine metabolic cycle. Biochim Biophys Acta (2010) 1804:89–96. doi: 10.1016/j.bbapap.2009.10.004
34. Lu SC, Mato JM. S-adenosylmethionine in liver health, injury, and cancer. Physiol Rev (2012) 92:1515–42. doi: 10.1152/physrev.00047.2011
35. Preynat A, Lapierre H, Thivierge MC, Palin MF, Matte JJ, Desrochers A, et al. Effects of supplements of folic acid, vitamin B12, and rumen protected methionine on whole body metabolism of methionine and glucose in lactating dairy cows. J dairy sci (2009) 92:677–89. doi: 10.3168/jds.2008-1525
36. Preynat A, Lapierre H, Thivierge MC, Palin MF, Cardinault N, Matte JJ, et al. Effects of supplementary folic acid and vitamin b (12) on hepatic metabolism of dairy cows according to methionine supply. J Dairy Sci (2010) 93:2130–42. doi: 10.3168/jds.2009-2796
37. Garcia M, Mamedova LK, Barton B, Bradford BJ. Choline regulates the function of bovine immune cells and alters the mRNA abundance of enzymes and receptors involved in its metabolism in vitro. Front Immunol (2018) 9:2448. doi: 10.3389/fimmu.2018.02448
38. Barak AJ, Beckenhauer HC, Junnila M, Tuma DJ. Dietary betaine promotes generation of hepatic s-adenosylmethionine and protects the liver from ethanol-induced fatty infiltration. Alcohol Clin Exp Res (1993) 17:552–5. doi: 10.1111/j.1530-0277.1993.tb00798.x
39. Fujii T, Watanabe Y, Fujimoto K, Kawashima K. Expression of acetylcholine in lymphocytes and modulation of an independent lymphocytic cholinergic activity by immunological stimulation. Biog Amines (2002) 17:373–86. doi: 10.1163/15693910260698320
40. Bionaz M, Chen S, Khan MJ, Loor JJ. Functional role of PPARs in ruminants: Potential targets for fine-tuning metabolism during growth and lactation. PPAR Res (2013) 2013:684159. doi: 10.1155/2013/684159
41. Lopreiato V, Mezzetti M, Cattaneo L, Ferronato G, Minuti A, Trevisi E. Role of nutraceuticals during the transition period of dairy cows: A review. J Anim Sci Biotechnol (2020) 11(1):1–18. doi: 10.1186/s40104-020-00501-x
42. Osorio JS, Ji P, Drackley JK, Luchini D, Loor JJ. Smartamine m and MetaSmart supplementation during the peripartal period alter hepatic expression of gene networks in 1-carbon metabolism, inflammation, oxidative stress, and the growth hormone-insulin-like growth factor 1 axis pathways. J Dairy Sci (2014) 97:7451–64. doi: 10.3168/jds.2014-8680
43. Tsiplakou E, Mavrommatis A, Kalogeropoulos T, Chatzikonstantinou M, Koutsouli P, Sotirakoglou K, et al. The effect of dietary supplementation with rumen-protected methionine alone or in combination with rumen-protected choline and betaine on sheep milk and antioxidant capacity. J Anim Physiol Anim Nutr (2017) 101(5):1004–13. doi: 10.1111/jpn.12537
44. Zhou Z, Trevisi E, Luchini DN, Loor JJ. Differences in liver functionality indexes in peripartal dairy cows fed rumen-protected methionine or choline are associated with performance, oxidative stress status, and plasma amino acid profiles. J Dairy Sci (2017) 100(8):6720–32. doi: 10.3168/jds.2016-12299
45. Vogt W. Oxidation of methionine residues in proteins: Tools, targets, and reversal. Free Rad Biol Med (1995) 18:93–105. doi: 10.1016/0891-5849(94)00158-G
46. Stadtman ER, Moskovitz J, Berlett BS, Levine RL. Cyclic oxidation and reduction of protein methionine residues is an important antioxidant mechanism. Mol Cell Biochem (2002) 234–235:3–9. doi: 10.1023/A:1015916831583
47. Luo S, Levine RL. Methionine in proteins defends against oxidative stress. FASEB J (2009) 23(2):464–72. doi: 10.1096/fj.08-118414
48. Han L, Batistel F, Ma Y, Alharthi ASM, Parys C, Loor JJ. Methionine supply alters mammary gland antioxidant gene networks via phosphorylation of nuclear factor erythroid 2-like 2 (NFE2L2) protein in dairy cows during the periparturient period. J Dairy Sci (2018) 101:8505–12. doi: 10.3168/jds.2017-14206
49. Coleman DN, Lopreiato V, Alharthi A, Loor JJ. Amino acids and the regulation of oxidative stress and immune function in dairy cattle. J Anim Sci (2020) 98:S175–93. doi: 10.1093/jas/skaa138
50. Abbasi IHR, Abbasi F, Wang L, Abd El Hack ME, Swelum AA, Hao R, et al. Folate promotes s-adenosyl methionine reactions and the microbial methylation cycle and boosts ruminants production and reproduction. Amb Express (2018) 8(1):1–10. doi: 10.1186/s13568-018-0592-5
51. Abbasi IHR, Abbasi F, Soomro RN, Abd El-Hack ME, Abdel-Latif MA, Li W, et al. Considering choline as methionine precursor, lipoproteins transporter, hepatic promoter and antioxidant agent in animals. AMB Expr (2017) 7:214. doi: 10.1186/s13568-017-0513-z
52. Yin J, Li T, Yin Y. Methionine and antioxidant potential. J Antioxid Act (2016) 1:12–7. doi: 10.14302/issn.2471-2140.jaa-16-1378
53. Li P, Yin YL, Li D, Kim SW, Wu G. Amino acids and immune function. Br J Nutr (2007) 98:237–52. 12. doi: 10.1017/S000711450769936X
54. Atmaca G. Antioxidant effects of sulfur-containing amino acids. Yonsei Med J (2004) 45(5):776–88. doi: 10.3349/ymj.2004.45.5.776
55. Colovic MB, Vasic VM, Djuric DM, Krstic DZ. Sulphur-containing amino acids: protective role against free radicals and heavy metals. Curr med Chem (2018) 25(3):324–35. doi: 10.2174/0929867324666170609075434
56. Ye ZW, Zhang J, Townsend DM, Tew KD. Oxidative stress, redox regulation and diseases of cellular differentiation. Biochim Biophys Acta (2015) 1850:1607–21. doi: 10.1016/j.bbagen.2014.11.010
57. Singhal SS, Singh SP, Singhal P, Horne D, Singhal J, Awasthi S. Antioxidant role of glutathione stransferases: 4-hydroxynonenal, a key molecule in stress-mediated signaling. Toxicol Appl Pharmacol (2015) 289:361–70. doi: 10.1016/j.taap.2015.10.006
58. Galal AM, Walker LA, Khan IA. Induction of GST and related events by dietary phytochemicals: sources, chemistry, and possible contribution to chemoprevention. Curr Top Med Chem (2015) 14:2802–21. doi: 10.2174/1568026615666141208110721
59. Zhou Z, Loor JJ, Piccioli-Cappelli F, Librandi F, Lobley GE, Trevisi E. Circulating amino acids in blood plasma during the peripartal period in dairy cows with different liver functionality index. J Dairy Sci (2016) 99:2257–67. doi: 10.3168/jds.2015-9805
60. Sun F, Cao Y, Cai C, Li S, Yu C, Yao J. Regulation of nutritional metabolism in transition dairy cows: Energy homeostasis and health in response to post-ruminal choline and methionine. PloS One (2016) 11(8):e0160659. doi: 10.1371/journal.pone.0160659
61. Bartosz G. Non-enzymatic antioxidant capacity assays: limitations of use in biomedicine. Free Radical Res (2010) 44:711–20. doi: 10.3109/10715761003758114
62. Mu T, Kong GH, Han ZY, Li HX. Cytoprotection of methionine on hyperthermia-induced damage in bovine mammary epithelial cells. Cell Biol Int (2014) 38:971–6. doi: 10.1002/cbin.10271
63. Han ZY, Mu T, Yang Z. Methionine protects against hyperthermia-induced cell injury in cultured bovine mammary epithelial cells. Cell Stress Chaperones (2015) 20:109–20. doi: 10.1007/s12192-014-0530-7
64. Zhou Z, Bulgari O, Vailati-Riboni M, Trevisi E, Ballou MA, Cardoso FC, et al. Rumen-protected methionine compared with rumen-protected choline improves immunometabolic status in dairy cows during the peripartal period. J dairy Sci (2016) 99(11):pp.8956–8969. doi: 10.3168/jds.2016-10986
65. Ceciliani F, Ceron JJ, Eckersall PD, Sauerwein H. Acute phase proteins in ruminants. J Proteomics (2012) 75:4207–31. doi: 10.1016/j.jprot.2012.04.004
66. van der Vusse GJ. Albumin as fatty acid transporter. Drug Metab Pharmacokinet (2009) 24:300–7. doi: 10.2133/dmpk.24.300
67. Osorio JS, Trevisi E, Ji P, Drackley JK, Luchini D, Bertoni G, et al. Biomarkers of inflammation, metabolism, and oxidative stress in blood, liver, and milk reveal a better immunometabolic status in peripartal cows supplemented with smartamine m or MetaSmart. J Dairy Sci (2014) 97:7437–50. doi: 10.3168/jds.2013-7679
68. Batistel F, Arroyo JM, Garces CIM, Trevisi E, Parys C, Ballou MA, et al. Ethyl-cellulose rumen-protected methionine alleviates inflammation and oxidative stress and improves neutrophil function during the periparturient period and early lactation in Holstein dairy cows. J Dairy Sci (2018) 101:480–90. doi: 10.3168/jds.2017-13185
69. Wang H, Elsaadawy SA, Wu Z, Bu DP. Maternal supply of ruminally-protected lysine and methionine during close-up period enhances immunity and growth rate of neonatal calves. Front Vet Sci (2021) 8:780731. doi: 10.3389/fvets.2021.780731
70. Hu L, Chen Y, Cortes IM, Coleman DN, Dai H, Liang Y, et al. Supply of methionine and arginine alters phosphorylation of mechanistic target of rapamycin (mTOR), circadian clock proteins, and α-s1-casein abundance in bovine mammary epithelial cells. Food Funct (2020) 11(1):883–94. doi: 10.1039/C9FO02379H
71. Dai H, Coleman DN, Hu L, Martinez-Cortés I, Wang M, Parys C, et al. Methionine and arginine supplementation alter inflammatory and oxidative stress responses during lipopolysaccharide challenge in bovine mammary epithelial cells in vitro. J dairy Sci (2020) 103(1):676–89. doi: 10.3168/jds.2019-16631
72. Lee C, Lobos NE, Weiss WP. Effects of supplementing rumen-protected lysine and methionine during prepartum and postpartum periods on performance of dairy cows. J dairy Sci (2019) 102(12):11026–39. doi: 10.3168/jds.2019-17125
73. Salama AA, Caja G, Albanell E, Such X, Casals R, Plaixats J. Effects of dietary supplements of zinc-methionine on milk production, udder health and zinc metabolism in dairy goats. J Dairy Res (2003) 70(1):9–17. doi: 10.1017/S0022029902005708
74. Abdelmegeid MK, Vailati-Riboni M, Alharthi A, Batistel F, Loor JJ. Supplemental methionine, choline, or taurine alter in vitro gene network expression of polymorphonuclear leukocytes from neonatal Holstein calves. J Dairy Sci (2017) 100:3155–65. doi: 10.3168/jds.2016-12025
75. Zhou Z, Ferdous F, Montagner P, Luchini DN, Corrêa MN, Loor JJ. Methionine and choline supply during the peripartal period alter polymorphonuclear leukocyte immune response and immunometabolic gene expression in Holstein cows. J Dairy Sci (2018) 101:10374–82. doi: 10.3168/jds.2018-14972
76. Calamari L, Soriani N, Panella G, Petrera F, Minuti A, Trevisi E. Rumination time around calving: An early signal to detect cows at greater risk of disease. J Dairy Sci (2014) 97:3635–47. doi: 10.3168/jds.2013-7709
77. Zhou Z, Vailati-Riboni M, Luchini D, Loor J. Methionine and choline supply during the periparturient period alter plasma amino acid and one-carbon metabolism profiles to various extents: Potential role in hepatic metabolism and antioxidant status. Nutrients (2016) 9:10. doi: 10.3390/nu9010010
78. Ospina PA, McArt JA, Overton TR, Stokol T, Nydam DV. Using nonesterified fatty acids and β-hydroxybutyrate concentrations during the transition period for herd-level monitoring of increased risk of disease and decreased reproductive and milking performance. Vet Clinics: Food Anim Pract (2013) 29(2):387–412. doi: 10.1016/j.cvfa.2013.04.003
79. Zom RLG, Van Baal J, Goselink RMA, Bakker JA, De Veth MJ, Van Vuuren AM. Effect of rumen-protected choline on performance, blood metabolites, and hepatic triacylglycerols of periparturient dairy cattle. J dairy Sci (2011) 94(8):4016–27. doi: 10.3168/jds.2011-4233
80. Morrison EI, Reinhardt H, Leclerc H, DeVries TJ, LeBlanc SJ. Effect of rumen-protected b vitamins and choline supplementation on health, production, and reproduction in transition dairy cows. J dairy Sci (2018) 101(10):9016–27. doi: 10.3168/jds.2018-14663
81. Bollatti JM, Zenobi MG, Barton BA, Staples CR, Santos JEP. Responses to rumen-protected choline in transition cows do not depend on prepartum body condition. J dairy Sci (2020) 103(3):2272–86. doi: 10.3168/jds.2019-17302
82. Chung YH, Brown NE, Martinez CM, Cassidy TW, Varga GA. Effects of rumen-protected choline and dry propylene glycol on feed intake and blood parameters for Holstein dairy cows in early lactation. J Dairy Sci (2009) 92(6):2729–36. doi: 10.3168/jds.2008-1299
83. Ster C, Loiselle MC, Lacasse P. Effect of postcalving serum nonesterified fatty acids concentration on the functionality of bovine immune cells. J Dairy Sci (2012) 95:708–17. doi: 10.3168/jds.2011-4695
84. Pinotti L, Polidori C, Campagnoli A, Dell’Orto V, Baldi A. A meta-analysis of the effects of rumen protected choline supplementation on milk production in dairy cows. EEAP Sci Ser (2010) 127:321–2.
85. Pinotti L, Baldi A, Politis I, Rebucci R, Sangalli L, Dell’Orto V. Rumen protected choline administration to transition cows: effects on milk production and vitamin e status. J Vet Med Ser A (2003) 50:18–21. doi: 10.1046/j.1439-0442.2003.00502.x
86. Cooke RF, Silva Del Río N, Caraviello DZ, Bertics SJ, Ramos MH, Grummer RR. Supplemental choline for prevention and alleviation of fatty liver in dairy cattle. J Dairy Sci (2007) 90:2413–8. doi: 10.3168/jds.2006-028
87. Elek P, Gaál T, Husvéth F. Influence of rumen-protected choline on liver composition and blood variables indicating energy balance in periparturient dairy cows. Acta Vet Hungarica (2013) 61(1):59–70. doi: 10.1556/avet.2012.053
88. Shahsavari A, Michael JD, Al Jassim R. The role of rumen-protected choline in hepatic function and performance of transition dairy cows. Br J Nutr (2016) 116(1):35–44. doi: 10.1017/S0007114516001641
89. Goselink RMA, van Baal J, Widjaja HCA, Dekker RA, Zom RLG, de Veth MJ, et al. Effect of rumen-protected choline supplementation on liver and adipose gene expression during the transition period in dairy cattle. J Dairy Sci (2013) 96(2):1102–16. doi: 10.3168/jds.2012-5396
90. Baumgard LH, Rhoads RP Jr. Effects of heat stress on postabsorptive metabolism and energetics. Annu Rev Anim Biosci (2013) 1(1):311–37. doi: 10.1146/annurev-animal-031412-103644
91. Ouellet V, Cabrera VE, Fadul-Pacheco L, Charbonneau É. The relationship between the number of consecutive days with heat stress and milk production of Holstein dairy cows raised in a humid continental climate. J dairy Sci (2019) 102(9):8537–45. doi: 10.3168/jds.2018-16060
92. Holdorf HT, White HM. Effects of rumen-protected choline supplementation in Holstein dairy cows during electric heat blanket-induced heat stress. J Dairy Sci (2021) 104(9):9715–25. doi: 10.3168/jds.2020-19794
93. Zenobi MG, Gardinal R, Zuniga JE, Mamedova LK, Driver JP, Barton BA, et al. Effect of prepartum energy intake and supplementation with ruminally protected choline on innate and adaptive immunity of multiparous Holstein cows. J dairy Sci (2020) 103(3):2200–16. doi: 10.3168/jds.2019-17378
94. Zenobi MG, Scheffler TL, Zuniga JE, Poindexter MB, Campagna SR, Gonzalez HC, et al. Feeding increasing amounts of ruminally protected choline decreased fatty liver in nonlactating, pregnant Holstein cows in negative energy status. J dairy Sci (2018) 101(7):5902–23. doi: 10.3168/jds.2017-13973
95. McFadden JW, Girard CL, Tao S, Zhou Z, Bernard JK, Duplessis M, et al. Symposium review: One-carbon metabolism and methyl donor nutrition in the dairy cow. J dairy Sci (2020) 103(6):5668–83. doi: 10.3168/jds.2019-17319
96. da Silva RP, Kelly KB, Lewis ED, Leonard KA, Goruk S, Curtis JM, et al. Choline deficiency impairs intestinal lipid metabolism in the lactating rat. J Nutr Biochem (2015) 26(10):1077–83. doi: 10.1016/j.jnutbio.2015.04.015
97. Jacometo CB, Alharthi AS, Zhou Z, Luchini D, Loor JJ. Maternal supply of methionine during late pregnancy is associated with changes in immune function and abundance of microRNA and mRNA in Holstein calf polymorphonuclear leukocytes. J dairy Sci (2018) 101(9):8146–58. doi: 10.3168/jds.2018-14428
98. Tsiplakou E, Mavrommatis A, Skliros D, Righi F, Flemetakis E. The impact of rumen-protected amino acids on the expression of key-genes involved in the innate immunity of dairy sheep. PloS One (2020) 15(5):e0233192. doi: 10.1371/journal.pone.0233192
99. Li Y, Bi Y, Diao Q, Piao M, Wang B, Kong F, et al. The limiting sequence and appropriate amino acid ratio of lysine, methionine, and threonine for seven-to nine-Month-Old Holstein heifers fed corn–soybean m-based diet. Animals (2019) 9(10):750. doi: 10.3390/ani9100750
100. Liang Y, Batistel F, Parys C, Loor JJ. Glutathione metabolism and nuclear factor erythroid 2-like 2 (NFE2L2)- related proteins in adipose tissue are altered by supply of ethyl-cellulose rumen-protected methionine in peripartal Holstein cows. J Dairy Sci (2019) 102:5530–41. doi: 10.3168/jds.2018-15687
101. Fagundes MA, Yang SY, Eun JS, Hall JO, Moon JO, Park JS. Influence of supplementing a methionine derivative, n-acetyl-l-methionine, in dairy diets on production and ruminal fermentation by lactating cows during early to mid lactation. J dairy Sci (2018) 101(8):7082–94. doi: 10.3168/jds.2017-14130
102. Lopreiato V, Vailati-Riboni M, Bellingeri A, Khan I, Farina G, Parys C, et al. Inflammation and oxidative stress transcription profiles due to in vitro supply of methionine with or without choline in unstimulated blood polymorphonuclear leukocytes from lactating Holstein cows. J Dairy Sci (2019) 102(1):10395–410. doi: 10.3168/jds.2019-16413
103. Osorio JS, Ji P, Drackley JK, Luchini D, Loor JJ. Supplemental smartamine m or MetaSmart during the transition period benefits postpartal cow performance and blood neutrophil function. J Dairy Sci (2013) 96:6248–63. doi: 10.3168/jds.2012-5790
104. Vailati-Riboni M, Zhou Z, Jacometo CB, Minuti A, Trevisi E, Luchini DN, et al. Supplementation with rumen-protected methionine or choline during the transition period influences whole-blood immune response in periparturient dairy cows. J Dairy Sci (2017) 100:3958–68. doi: 10.3168/jds.2016-11812
105. Zhou Z, Garrow TA, Dong X, Luchini DN, Loor JJ. Hepatic activity and transcription of betaine-homocysteine methyltransferase, methionine synthase, and cystathionine synthase in periparturient dairy cows are altered to different extents by supply of methionine and choline. J Nutr (2017) 147:11–9. doi: 10.3945/jn.116.240234
106. Alharthi AS, Coleman DN, Liang Y, Batistel F, Elolimy AA, Yambao RC, et al. Hepatic 1-carbon metabolism enzyme activity, intermediate metabolites, and growth in neonatal Holstein dairy calves are altered by maternal supply of methionine during late pregnancy. J Dairy Sci (2019) 102:10291–303. doi: 10.3168/jds.2019-16562
107. Guretzky NJ, Carlson DB, Garrett JE, Drackley JK. Lipid metabolite profiles and milk production for Holstein and Jersey cows fed rumen-protected choline during the periparturient period. J dairy Sci (2006) 89(1):188–200. doi: 10.3168/jds.S0022-0302(06)72083-5
108. Bollatti JM, Zenobi MG, Artusso NA, Alfaro GF, Lopez AM, Barton BA, et al. Timing of initiation and duration of feeding rumen-protected choline affects performance of lactating Holstein cows. J dairy Sci (2020) 103(5):4174–91. doi: 10.3168/jds.2019-17293
109. Salama AA, Duque M, Wang L, Shahzad K, Olivera M, Loor JJ. Enhanced supply of methionine or arginine alters mechanistic target of rapamycin signaling proteins, messenger RNA, and microRNA abundance in heat-stressed bovine mammary epithelial cells in vitro. Journal of dairy science (2019) 102(3):2469–80. doi: 10.3168/jds.2018-15219
110. Wang JX, Zhang Q, Pei S, Yang B. Effect and mechanism of miR-34a on proliferation, apoptosis and invasion of laryngeal carcinoma cells. Asian Pac J Trop Med (2016) 9:494–8. doi: 10.1016/j.apjtm.2016.03.018
111. Jena MK. MicroRNAs in the development and neoplasia of the mammary gland. F1000Res (2017) 6:1018. doi: 10.12688/f1000research.12005.2.
112. Ma YF, Batistel F, Xu TL, Han LQ, Bucktrout R, Liang Y, et al. Phosphorylation of AKT serine/threonine kinase and abundance of milk protein synthesis gene networks in mammary tissue in response to supply of methionine in periparturient Holstein cows. J dairy Sci (2019) 102(5):4264–74. doi: 10.3168/jds.2018-15451
113. Nan X, Bu D, Li X, Wang J, Wei H, Hu H, et al. Ratio of lysine to methionine alters expression of genes involved in milk protein transcription and translation and mTOR phosphorylation in bovine mammary cells. Physiol Genomics (2014) 46:268–75. doi: 10.1152/physiolgenomics.00119.2013
114. Dong X, Zhou Z, Saremi B, Helmbrecht A, Wang Z, Loor JJ. Varying the ratio of lys: Met while maintaining the ratios of thr: Phe, lys: Thr, lys: His, and lys: Val alters mammary cellular metabolites, mammalian target of rapamycin signaling, and gene transcription. J Dairy Sci (2018) 101:1708–18. doi: 10.3168/jds.2017-13351
115. Jacometo CB, Zhou Z, Luchini D, Corrêa MN, Loor JJ. Maternal supplementation with rumen-protected methionine increases prepartal plasma methionine concentration and alters hepatic mRNA abundance of 1-carbon, methionine, and transsulfuration pathways in neonatal Holstein calves. J Dairy Sci (2017) 100(4):3209–19. doi: 10.3168/jds.2016-11656
116. Neumann S, Razen M, Habermehl P, Meyer CU, Zepp F, Kirkpatrick CJ, et al. The non-neuronal cholinergic system in peripheral blood cells: effects of nicotinic and muscarinic receptor antagonists on phagocytosis, respiratory burst and migration. Life Sci (2007) 80:2361–4. doi: 10.1016/j.lfs.2007.01.010
117. Tsiplakou E, Mavrommatis A, Skliros D, Sotirakoglou K, Flemetakis E, Zervas G. The effects of dietary supplementation with rumen-protected amino acids on the expression of several genes involved in the immune system of dairy sheep. J Anim Physiol Anim Nutr (Berl) (2018) 102(6):1437–49. doi: 10.1111/jpn.12968
118. Osorio JS, Jacometo CB, Zhou Z, Luchini D, Cardoso FC, Loor JJ. Hepatic global DNA and peroxisome proliferator-activated receptor alpha promoter methylation are altered in peripartal dairy cows fed rumen-protected methionine. J dairy Sci (2016) 99(1):234–44. doi: 10.3168/jds.2015-10157
119. Palombo V, Alharthi A, Batistel F, Parys C, Guyader J, Trevisi E, et al. Unique adaptations in neonatal hepatic transcriptome, nutrient signaling, and one-carbon metabolism in response to feeding ethyl cellulose rumen-protected methionine during late-gestation in Holstein cows. BMC Genomics (2021) 22(1):1–24. doi: 10.1186/s12864-021-07538-w
Keywords: oxidative stress, periparturient period, ruminants, antioxidants, immunity, limiting amino acids
Citation: Khan MZ, Liu S, Ma Y, Ma M, Ullah Q, Khan IM, Wang J, Xiao J, Chen T, Khan A and Cao Z (2023) Overview of the effect of rumen-protected limiting amino acids (methionine and lysine) and choline on the immunity, antioxidative, and inflammatory status of periparturient ruminants. Front. Immunol. 13:1042895. doi: 10.3389/fimmu.2022.1042895
Received: 13 September 2022; Accepted: 08 December 2022;
Published: 12 January 2023.
Edited by:
Sergey Ponomarev, Institute of Biomedical Problems (RAS), RussiaReviewed by:
Emmanuel Asiamah, University of Arkansas at Pine Bluff, United StatesShanshan Yang, Jiangxi Agricultural University, China
Copyright © 2023 Khan, Liu, Ma, Ma, Ullah, Khan, Wang, Xiao, Chen, Khan and Cao. This is an open-access article distributed under the terms of the Creative Commons Attribution License (CC BY). The use, distribution or reproduction in other forums is permitted, provided the original author(s) and the copyright owner(s) are credited and that the original publication in this journal is cited, in accordance with accepted academic practice. No use, distribution or reproduction is permitted which does not comply with these terms.
*Correspondence: Zhijun Cao, Y2FvemhpanVuQGNhdS5lZHUuY24=
†These authors have contributed equally to this work