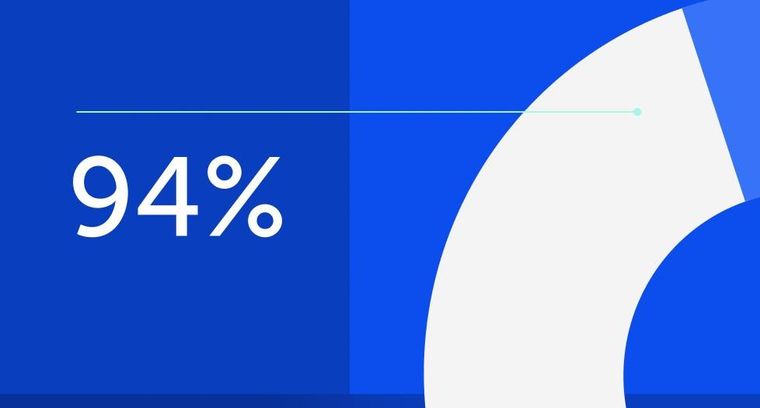
94% of researchers rate our articles as excellent or good
Learn more about the work of our research integrity team to safeguard the quality of each article we publish.
Find out more
PERSPECTIVE article
Front. Immunol., 04 October 2022
Sec. Mucosal Immunity
Volume 13 - 2022 | https://doi.org/10.3389/fimmu.2022.1039194
This article is part of the Research TopicAdaptive Immunity to Respiratory PathogensView all 7 articles
Historically, the gold-standard benchmark for vaccine immunogenicity has been the induction of neutralizing antibodies detectable in the serum of peripheral blood. However, in recent years there has been a new appreciation for the mucosa as an important site for vaccine induced immunity. As a point of first contact, the mucosal tissue represents a major site of immune based detection and restriction of pathogen entry and dissemination. Tissue resident memory T cells (Trm) are one of the critical cell types involved in this early detection and restriction of mucosal pathogens. Following tissue-specific infection or vaccination, Trm lodge themselves within tissues and can perform rapid sensing and alarm functions to control local re-infections, in an effort that has been defined as important for restriction of a number of respiratory pathogens including influenza and respiratory syncytial virus. Despite this characterized importance, only minor attention has been paid to the importance of Trm as a benchmark for vaccine immunogenicity. The purpose of this review is to highlight the functions of Trm with particular emphasis on respiratory infections, and to suggest the inclusion of Trm elicitation as a benchmark for vaccine immunogenicity in animal models, and where possible, human samples.
Throughout history, respiratory infections have been a global scourge on human health and many respiratory infections we have yet to effectively control through vaccination (1). Seasonal influenza virus infects between 5-20% of the population annually and was estimated to cause more than 20,000 deaths in the U.S. in the 2019/2020 season (2). While seasonal influenza vaccines are produced and administered annually, they vary substantially in efficacy each year depending upon a number of factors including the accuracy of algorithms designed to predict the likely circulating strains (3). Speaking to this, is the additional challenge that the seasonal influenza vaccine must be reformulated annually to account for the virus constantly mutating to evade existing humoral immunity. Outside of seasonal influenza, looms the constant threat of pandemic influenza, which can emerge from a reassortment event with a non-human reservoir influenza strain. Examples of this include the 2009 H1N1 pandemic that resulted in nearly 300,000 deaths and the 1918 H1N1 pandemic that resulted in an estimated death toll of 20-40 million people (4, 5). Since its emergence in 2019, the novel coronavirus SARS-CoV-2 spread explosively throughout the world. At the time of this review there have been an estimated 600 million cases and more than 6 million deaths due to SARS-CoV-2 worldwide (both numbers are likely underestimates) (6). The deployment of multiple COVID vaccines including the Moderna and Pfizer mRNA-based vaccines and the Johnson & Johnson and AstraZeneca adenovirus-vector-based vaccines contributed to curbing severe disease associated with COVID-19 (7). However, the virus continues to spread as new viral variants that are poorly controlled by pre-existing immunity are generated, posing a roadblock to controlling this virus (8). Despite these substantial burdens to public health, effective vaccination strategies to control many respiratory infections have continued to elude us.
Barrier sites such as the respiratory epithelial interface represent the first site of encounter for pathogens. T cells have been demonstrated to play a critical role in the control of a number of respiratory infections, particularly when antibody neutralization fails (9). Following infection, a subset of memory T cells can be retained in the tissue of immunological insult and are termed “tissue resident memory T cells” (Trm) and have been demonstrated to play an important role in protection from a number of respiratory pathogens including influenza virus (9, 10), respiratory syncytial virus (RSV) (11), and mycobacterium tuberculosis(mTB) (12). In addition to being poised to respond directly within the tissue of initial infection, Trm display unique transcriptional and functional profiles relative to other memory T cell subsets that permit them to maintain tissue residence, sense pathogens early after reinfection and activate a local inflammatory state within the tissue to restrict dissemination of the pathogen (13–16). Despite their recognized importance in a number of infection models, the elicitation of antigen-specific Trm to barrier sites is not typically used as a benchmark for immunogenicity of candidate vaccines. This is likely due to a number of reasons, including accessibility of the tissue and specific challenges in mucosal vaccine design such as lack of effective adjuvant and delivery platforms. To aid in the design and testing of more effective mucosal vaccines, much work still needs to be done to overcome these barriers, including the optimization of mucosal delivery platforms to overcome immune tolerance at mucosal sites and the inclusion of Trm as a benchmark for immunogenicity in pre-clinical model testing of mucosal vaccine candidates.
Following resolution of infection, subsets of T cells can be retained in memory at a higher frequency and lower activation threshold relative to naïve T cells. These memory T cell subsets are defined by expression of specific homing receptors and their functions (17). Central memory T cells (Tcm) patrol secondary lymphoid organs and are characterized by rapid proliferation upon antigen re-encounter, while T effector memory (Tem) recirculate between blood and non-lymphoid tissues (18). In the last decade, it has begun to be appreciated that another subset of memory T cells exist, termed tissue resident memory T cells (Trm), which lodge into non-lymphoid tissues as a result of distinct cellular signals controlling their transcriptional profiles, allowing them to perform functions distinct from Tem and Tcm (19).
CD8+ T cells primed in the draining lymph nodes migrate to the tissue of immunological insult (20, 21). After entry, some of these cells undergo transcriptional changes including upregulation of HOBIT, BLIMP1 and RUNX3 and downregulation of KLF2 (15), resulting in upregulation of Trm hallmark proteins such as CD69, and depending upon the tissue, upregulation of the αeβ7 integrin CD103 [e.g. in epitheliod tissues such as the skin (22), lungs (23), and gut (24)]. As an antagonist of S1PR1, CD69 expression is thought to prevent Trm egress from the tissues (25). CD103 expressed on Trm can interact with E-cadherin on epithelial cells (26) supporting their tissue retention (22, 27) and potentially supporting their survival through the upregulation of Bcl-2 (19, 22). Multiple studies support these proposed roles of CD103 and CD69, but it is still unclear exactly how each is necessary or sufficient to promote Trm retention in tissues (19, 22, 28). It is highly likely that the cytokine milieu within specific tissues may drive unique Trm generation and maintenance (29). Multiple studies have established an importance in the pleiotropic cytokine TGF-β in CD103 upregulation (22, 27, 30). There is evidence that CD69 expression can be induced by IL-33, type 1 interferons and TNF-α (31).
There is understandably substantial interest in the longevity of Trm particularly as it pertains to vaccines. In animal models (including rhesus macaques and mice), Trm have been reported as a highly stable population at least out to 300 days post infection in tissues like the skin (16, 22, 32–34). Importantly, this appears to be a tissue-dependent phenomenon. Trm in the lung begin to numerically wane via apoptosis and potentially draining lymph node egress by 100 days post infection with influenza virus (33, 35). This loss of Trm has been shown to correlate with the loss of heterosubtypic immunity to influenza virus (33, 36). Therefore, a significant challenge in optimal mucosal vaccine design, specifically as it relates to respiratory pathogens, is to determine why lung Trm are relatively short-lived. In a model of influenza infection, it was shown that repeated antigen exposure increased the longevity of Trm in the lung, likely through stepwise transcriptional alterations including the upregulation of survival signals like Bcl-2 (16, 35). This repeated antigen stimulation as a means to enhance Trm longevity in the lung could have substantial implications for vaccine design and deployment, though more mechanistic work needs to be done.
Because Trm occupy the first exposure sites of infection, they represent a powerful and rapid mechanism for restriction of pathogen replication. Indeed, this appears to be the case for a number of infections in the skin (37), liver (38), female reproductive tract (39), and lungs (11, 36). In addition to being in a locally advantageous position to restrict pathogen entry and replication, Trm possess unique functional characteristics that also support this effort. In terms of patrolling function, Trm in the skin appear to have a dynamic morphology that includes projections, which can potentially assist in facilitating antigen encounters in the tissue (40, 41). Particularly in the skin, Trm have been confirmed to display “crawling behavior” between keratinocytes as they patrol for virally infected cells (40, 42). In the liver, Trm have also been reported to patrol within the sinusoids, facilitating close interactions with infected hepatocytes and liver resident immune cells (43). This motile behavior is likely to occur within the respiratory tissues, but has yet to be elucidated.
In addition to unique motility and projections, CD8+ Trm upregulate the cytolytic molecules perforin and granzyme B to quickly control infection through direct cytolysis of infected cells (16, 44, 45). In addition to this, these cells can potentially contribute to pathogen restriction through non-cytolytic means such as IFN-γ production (46) or production of chemokines to attract other immune cell populations (13). This swift cytokine and chemokine production can trigger an antiviral state in the infected tissue, facilitating the recruitment of additional T and B cells (13), triggering the induction of antiviral genes (47), and driving dendritic cell maturation (13). In this way, Trm serve as a means to regulate both innate and adaptive immune responses at the site of pathogen entry to quickly limit pathogen invasion.
Perhaps one of the most well-studied systems in relation to Trm and respiratory infections is that of influenza virus. It has been appreciated that in the absence of cross-reactive antibodies (as is the case often times when antigenic drift occurs with seasonal flu) CD8+ Trm specific for conserved influenza antigens can facilitate strain transcending heterosubtypic (but non-sterilizing) immunity to influenza in mouse models (33, 36). The Kohlmeier group found that specifically airway-resident CD8+ T cells contribute to this protection in a mechanism that is dependent on IFN-γ production (48, 49). It is less clear how Trm found in the lung parenchyma can facilitate protection. Prior to the formal description of Trm in the literature, studies identified perforin, FasL and IFN-γ as being important mediators for CD8+ T cell dependent protection against heterosubtypic influenza challenge (50, 51). As it is known that Trm are the predominant drivers of heterosubtypic influenza virus immunity in murine models, it is likely that these mechanisms are important for protection driven by Trm in the parenchyma. Importantly, several studies of cells from human lung donors have identified influenza-specific Trm that were cross-reactive against multiple influenza strains (49, 52–54). While CD4+ Trm are an under-appreciated population, multiple studies have found them sufficient for protection in a mouse model of influenza challenge (55, 56). Indeed, one study from the Sant group utilized a nanoparticle vaccine linked to the influenza nucleoprotein (NP) which was administered intranasally, and found to elicit persistent and polyfunctional influenza specific CD4+ Trm responses in the lung that protected from severe disease in mice (56). This highlights forward development in terms of a novel delivery platform for mucosal vaccines and the notion that multiple T cell populations can contribute to mucosal protection.
Injectable vaccines against influenza virus have been approved for use for many years and have some success at eliciting protective effects in the lower respiratory tract, mainly in the form of secretory IgA and IgG (57). More recently, the temperature-sensitive live attenuated intranasal influenza vaccine (LAIV, FluMist) was introduced into the United States and found to be immunogenic and protective against antigenically matched influenza strains in children (58). Perhaps unsurprisingly, the intranasally administered live attenuated vaccine was more similar to natural immunity elicited from infection compared to the injectable vaccine (59). This was supported when controlled comparisons were made in murine vaccination models, finding that LAIV was capable of generating influenza-specific CD4+ and CD8+ Trm that facilitated cross-strain protection, while the injectable inactivated vaccine did not generate Trm nor facilitate cross-strain protection, highlighting the potential importance of route of immunization in eliciting T cells resident to the respiratory tract (10).
Studies involving human Trm in protection from respiratory pathogens are less common due to the invasiveness surrounding Trm isolation. However, one human study focusing on respiratory syncytial virus (RSV) investigated the local and systemic CD8+ T cell response by serial bronchoscopy and blood sampling, respectively, and found that the abundance of RSV-specific CD8+ T cells within the bronchial washes correlated with reduced symptoms and viral load during infection, while the abundance of peripheral CD8+ T cells did not (60). This study indicated a potential importance for Trm in protection from RSV in humans, which had not been previously shown.
Multiple murine studies have also demonstrated that CD4+ and CD8+ lung Trm rather than peripheral T cells contribute to protection from RSV (11, 61). Importantly, it was found that while both CD4+ and CD8+ Trm contributed to disease reduction (as measured by weight loss), only CD8+ Trm contributed to the reduction of viral load in the lungs (61). IFN-γ produced by these CD8+ T cells was proposed to be the likely mechanism for this protection. These studies assessed different mechanisms of vaccine-induced immunity that warrant discussion. The study by Kinnear et al. utilized a DNA vaccine encoding the RSV M2 protein which was administered intramuscularly. This vaccine has previously been shown to induce robust anti-RSV T cells in the periphery (62). In contrast, the Luangrath et al. study utilized a recombinant influenza virus expressing the RSV H2-D restricted CD4+ T cell epitope F51 and CD8+ T cell epitope M282 to intranasally challenge RSV immune mice (11). The Kinnear study very clearly found that while the DNA based intramuscular vaccination led to RSV-specific CD8+ T cells that were highly inflammatory, there was not substantial numerical induction of CD8+ Trm relative to prior RSV exposure. As such, vaccination did not lead to a reduction in disease or viral burden in the lungs (61). In the Luangrath study, mice with prior RSV intranasal exposure had significantly reduced viral burden when challenged with the recombinant influenza virus expressing RSV antigen (11). These two studies nicely highlight that different routes of immunization will lead to different levels of induction of Trm in the lungs and it will be critical to address the mechanism by which this occurs in order to aid in effective vaccine design.
The literature surrounding the nature of Trm as they relate to SARS-CoV-2 is still a rapidly developing field, though some information does exist. Human studies from the Zhang lab and Farber lab have identified and characterized Trm in bronchoalveolar flushes from healthy controls and COVID-19 patients (63–65). It was found that mild COVID-19 cases were more associated with a robust expansion of CD8+ Trm compared to more severe cases, suggesting a role for SARS-CoV-2-specific Trm in the mitigation of COVID-19 pathogenesis (64). Despite having changed the trajectory of the COVID-19 pandemic, there is little information regarding the propensity for the currently approved COVID vaccines to elicit SARS-CoV-2 specific Trm in the lungs. Somewhat justifiably, these early immunogenicity studies relied on measuring antigen-specific circulating memory T and B cell populations, and the elicitation of neutralizing antibodies (66–70). While Trm can be generated from site-specific vaccination (e.g. intranasal vaccination to elicit Trm in the lungs) it is unknown whether the currently approved COVID vaccines elicit lung Trm when administered intramuscularly, as is current practice. As has been the case historically with other emerging viral pathogens, murine studies will likely be important in furthering our mechanistic understanding of the role of Trm during SARS-CoV-2 infection.
While limited studies have been completed delineating the role of lung Trm in response to SARS-CoV-2 infection or vaccination, we can, however draw some information from studies involving SARS-CoV-1 infection. SARS-CoV-1 specific CD4+ and CD8+ Trm have been defined as necessary for vaccine-mediated protection from SARS-CoV-1 in a mouse model of infection (71, 72). By using a dendritic cell pulsed peptide immunization followed by an intranasal boost with recombinant vaccinia virus expressing the H2-B restricted epitopes S436/525, the authors were able to induce rapid production of IFN-γ, TNF-α, IL-2, and granzyme B by antigen-specific CD8+ T cells, which was shown to contribute to reduced viral loads; again highlighting the potential value of the advancement of mucosal vaccines to elicit Trm populations in the lung (71). The robustness and conserved nature of this type of immunity has the potential to be a highly effective strategy for the design of broadly protective respiratory pathogen vaccines. However, one must acknowledge the necessity of further development of more effective mucosal vaccines to elicit pulmonary Trm.
While Trm have been linked to protective immune responses against a number of pathogens, barriers still exist to their successful use as a benchmark for vaccine immunogenicity. One such obvious barrier is the challenge of tissue access to study Trm. By nature, these cells are lodged within their tissue of origin which often requires invasive techniques to extricate them for study. To move the study of vaccine induced Trm forward, the substantial power of animal models of pre-clinical vaccine testing will continue to be an invaluable resource to rational vaccine design. In this way, murine or non-human primate tissues can be analyzed for effective and optimal elicitation of mucosal Trm by vaccine candidates. Moreover, several human studies have been described in this review that utilized longitudinal bronchoalveolar sampling to assess Trm induction in airways in response to infection (63, 65). While this technique is relatively invasive compared to peripheral blood sampling, it still represents a viable option to study respiratory Trm in humans.
While the protective capacity of antiviral CD8+ Trm in the respiratory tract have been heavily described in the literature, it is important to note that a balance between viral clearance and immune driven pathology is critical for an effective response against any invading pathogen. CD8+ T cells clearing virally infected cells have the capacity to cause damage and subsequently fibrosis within the tissue (73). Of particular relevance to this point are studies from the Jie Sun lab which defined a role for PD-L1 in limiting post-infection inflammatory damage mediated by CD8+ Trm in the lung following influenza infection (74). It is clear that while antiviral Trm can be used as a benchmark for protection, context is highly important and should be taken into consideration to promote a balance between protection and pathogenesis.
As noted earlier in the review, Trm in the respiratory tract have been characterized as more short-lived in comparison to other memory cell populations, waning numerically by approximately 100 days after a single antigen exposure (33, 35). This represents an interesting and unique challenge to mucosal T cell biology that will require further mechanistic studies into the underlying cause of lung Trm loss. Interestingly, our lab has previously found that repetitive encounters with their cognate antigen can drive increased Trm longevity in the lungs, representing a potentially fruitful mechanism to address this question (35). Specifically, Trm stimulated in vivo via a single infection begin to wane by 100 days. However, Trm that had experienced antigen in vivo four times were numerically maintained out to at least 150 days. This is likely due to the stepwise transcriptional changes that have been demonstrated to occur when memory T cells have multiple encounters with antigen, which support survival signals such as Bcl-2 expression (16, 75). It is certainly possible that mucosal vaccines designed to elicit Trm in the lung may have to be administered multiple times (boosting) to have full long-term protective effects.
Respiratory pathogens have had catastrophic impacts on human health and economic development throughout history. The explosive spread of SARS-CoV-2 over the past 2 years, has highlighted the continued threat of emerging respiratory pathogens. Within the devastation of COVID-19 has also been a reminder that vaccination against respiratory pathogens remains a highly effective strategy to curb the disease and community spread and an impetus for the further development of novel vaccine strategies. Over the past 10 years, Trm have emerged as a newly appreciated memory T cell subset, particularly in the case of respiratory infection biology. Being poised at the site of pathogen entry and possessing unique transcriptional and functional profiles makes this cell type a prime candidate to capitalize on for the next generation of vaccines. Multiple human and animal studies have demonstrated an importance for these cells in protection from disease including influenza virus, respiratory syncytial virus, and coronaviruses. While these findings have been clear, the challenges described in this review must be addressed to most effectively design mucosal vaccines to elicit protective Trm mediated responses.
The original contributions presented in the study are included in the article/Supplementary Material. Further inquiries can be directed to the corresponding author.
MH and JTH wrote and edited the manuscript. All authors contributed to the article and approved the submitted version.
This work was supported by grants from the National Institutes of Health (National Institute of Allergy and Infectious Diseases) AI042767, AI114543 (to JTH), and T32 AI007260 (to MH).
The authors declare that the research was conducted in the absence of any commercial or financial relationships that could be construed as a potential conflict of interest.
All claims expressed in this article are solely those of the authors and do not necessarily represent those of their affiliated organizations, or those of the publisher, the editors and the reviewers. Any product that may be evaluated in this article, or claim that may be made by its manufacturer, is not guaranteed or endorsed by the publisher.
1. Esparza J. Lessons from history: What can we learn from 300 years of pandemic flu that could inform the response to COVID-19? Am J Public Health (2020) 110(8):1160–1. doi: 10.2105/AJPH.2020.305761
2. (CDC) CfDCaP. Estimated flu-related illnesses, medical visits, hospitalizations, and deaths in the united states — 2019–2020 flu season cdc.gov. CDC (2021). (the Centers for Disease Control and Prevention) Available at: https://www.cdc.gov/flu/about/burden/2019-2020.html.
3. Center for Disease control and Prevention (2022). (Centers for Disease Control and Prevention and National Center for Immunization and Respiratory Diseases (NCIRD) Available at: https://www.cdc.gov/flu/weekly/flusight/index.html
4. Prevention CfDCa. The burden of the influenza a H1N1pdm09 virus since the 2009 pandemic cdc.gov. CDC (2019).The Centers for Disease Control and Prevention Available at: https://www.cdc.gov/flu/pandemic-resources/burden-of-h1n1.html.
5. Taubenberger JK, Morens DM. 1918 influenza: the mother of all pandemics. Emerg Infect diseases (2006) 12(1):15–22. doi: 10.3201/eid1209.05-0979
6. WHO. WHO coronavirus (COVID-19) dashboard covid19.who.int. World Health Organization (WHO World Health Organization) (2022).
7. Santacatterina M, Sanders JW, Weintraub WS. Prevention of covid-19 with the BNT162b2 and mRNA-1273 vaccines. New Engl J Med (2021) 385(19):1817–8. doi: 10.1056/NEJMc2113575
8. Fernandes Q, Inchakalody VP, Merhi M, Mestiri S, Taib N, Moustafa Abo El-Ella D, et al. Emerging COVID-19 variants and their impact on SARS-CoV-2 diagnosis, therapeutics and vaccines. Ann Med (2022) 54(1):524–40. doi: 10.1080/07853890.2022.2031274
9. Liang S, Mozdzanowska K, Palladino G, Gerhard W. Heterosubtypic immunity to influenza type a virus in mice. effector mechanisms and their longevity. J Immunol (1994) 152(4):1653–61.
10. Zens KD, Chen JK, Farber DL. Vaccine-generated lung tissue-resident memory T cells provide heterosubtypic protection to influenza infection. JCI Insight (2016) 1(10)1–13. doi: 10.1172/jci.insight.85832
11. Luangrath MA, Schmidt ME, Hartwig SM, Varga SM. Tissue-resident memory T cells in the lungs protect against acute respiratory syncytial virus infection. ImmunoHorizons. (2021) 5(2):59–69. doi: 10.4049/immunohorizons.2000067
12. Sakai S, Kauffman KD, Schenkel JM, McBerry CC, Mayer-Barber KD, Masopust D, et al. Cutting edge: control of mycobacterium tuberculosis infection by a subset of lung parenchyma-homing CD4 T cells. J Immunol (2014) 192(7):2965–9. doi: 10.4049/jimmunol.1400019
13. Schenkel JM, Fraser KA, Beura LK, Pauken KE, Vezys V, Masopust D. T Cell memory. resident memory CD8 T cells trigger protective innate and adaptive immune responses. Science (2014) 346(6205):98–101. doi: 10.1126/science.1254536
14. Kumar BV, Ma W, Miron M, Granot T, Guyer RS, Carpenter DJ, et al. Human tissue-resident memory T cells are defined by core transcriptional and functional signatures in lymphoid and mucosal sites. Cell Rep (2017) 20(12):2921–34. doi: 10.1016/j.celrep.2017.08.078
15. Milner JJ, Goldrath AW. Transcriptional programming of tissue-resident memory CD8(+) T cells. Curr Opin Immunol (2018) 51:162–9. doi: 10.1016/j.coi.2018.03.017
16. Anthony SM, Van Braeckel-Budimir N, Moioffer SJ, van de Wall S, Shan Q, Vijay R, et al. Protective function and durability of mouse lymph node-resident memory CD8(+) T cells. eLife (2021) 10:1–28. doi: 10.7554/eLife.68662
17. Sallusto F, Lenig D, Förster R, Lipp M, Lanzavecchia A. Two subsets of memory T lymphocytes with distinct homing potentials and effector functions. Nature. (1999) 401(6754):708–12. doi: 10.1038/44385
18. Butcher EC, Picker LJ. Lymphocyte homing and homeostasis. Science (1996) 272(5258):60–6. doi: 10.1126/science.272.5258.60
19. Schenkel JM, Masopust D. Tissue-resident memory T cells. Immunity. (2014) 41(6):886–97. doi: 10.1016/j.immuni.2014.12.007
20. Harty JT, Badovinac VP. Shaping and reshaping CD8+ T-cell memory. Nat Rev Immunol (2008) 8(2):107–19. doi: 10.1038/nri2251
21. Hofmann M, Pircher H. E-cadherin promotes accumulation of a unique memory CD8 T-cell population in murine salivary glands. Proc Natl Acad Sci United States America (2011) 108(40):16741–6. doi: 10.1073/pnas.1107200108
22. Mackay LK, Rahimpour A, Ma JZ, Collins N, Stock AT, Hafon M-L, et al. The developmental pathway for CD103+CD8+ tissue-resident memory T cells of skin. Nat Immunol (2013) 14(12):1294–301. doi: 10.1038/ni.2744
23. Richter MV, Topham DJ. The alpha1beta1 integrin and TNF receptor II protect airway CD8+ effector T cells from apoptosis during influenza infection. J Immunol (2007) 179(8):5054–63. doi: 10.4049/jimmunol.179.8.5054
24. Masopust D, Choo D, Vezys V, Wherry EJ, Duraiswamy J, Akondy R, et al. Dynamic T cell migration program provides resident memory within intestinal epithelium. J Exp Med (2010) 207(3):553–64. doi: 10.1084/jem.20090858
25. Masopust D, Vezys V, Wherry EJ, Barber DL, Ahmed R. Cutting edge: gut microenvironment promotes differentiation of a unique memory CD8 T cell population. J Immunol (2006) 176(4):2079–83. doi: 10.4049/jimmunol.176.4.2079
26. Cepek KL, Shaw SK, Parker CM, Russell GJ, Morrow JS, Rimm DL, et al. Adhesion between epithelial cells and T lymphocytes mediated by e-cadherin and the alpha e beta 7 integrin. Nature. (1994) 372(6502):190–3. doi: 10.1038/372190a0
27. Casey KA, Fraser KA, Schenkel JM, Moran A, Abt MC, Beura LK, et al. Antigen-independent differentiation and maintenance of effector-like resident memory T cells in tissues. J Immunol (2012) 188(10):4866–75. doi: 10.4049/jimmunol.1200402
28. Lee YT, Suarez-Ramirez JE, Wu T, Redman JM, Bouchard K, Hadley GA, et al. Environmental and antigen receptor-derived signals support sustained surveillance of the lungs by pathogen-specific cytotoxic T lymphocytes. J virol (2011) 85(9):4085–94. doi: 10.1128/JVI.02493-10
29. Milner JJ, Toma C, He Z, Kurd NS, Nguyen QP, McDonald B, et al. Heterogenous populations of tissue-resident CD8(+) T cells are generated in response to infection and malignancy. Immunity. (2020) 52(5):808–24.e7. doi: 10.1016/j.immuni.2020.04.007
30. Zhang N, Bevan MJ. Transforming growth factor-β signaling controls the formation and maintenance of gut-resident memory T cells by regulating migration and retention. Immunity. (2013) 39(4):687–96. doi: 10.1016/j.immuni.2013.08.019
31. Skon CN, Lee JY, Anderson KG, Masopust D, Hogquist KA, Jameson SC. Transcriptional downregulation of S1pr1 is required for the establishment of resident memory CD8+ T cells. Nat Immunol (2013) 14(12):1285–93. doi: 10.1038/ni.2745
32. Masopust D, Vezys V, Marzo AL, Lefrançois L. Preferential localization of effector memory cells in nonlymphoid tissue. Science (2001) 291(5512):2413–7. doi: 10.1126/science.1058867
33. Slütter B, Van Braeckel-Budimir N, Abboud G, Varga SM, Salek-Ardakani S, Harty JT. Dynamics of influenza-induced lung-resident memory T cells underlie waning heterosubtypic immunity. Sci Immunol (2017) 2(7):eaag2031. doi: 10.1126/sciimmunol.aag2031
34. Pan Y, Tian T, Park CO, Lofftus SY, Mei S, Liu X, et al. Survival of tissue-resident memory T cells requires exogenous lipid uptake and metabolism. Nature. (2017) 543(7644):252–6. doi: 10.1038/nature21379
35. Van Braeckel-Budimir N, Varga SM, Badovinac VP, Harty JT. Repeated antigen exposure extends the durability of influenza-specific lung-resident memory CD8+ T cells and heterosubtypic immunity. Cell Rep (2018) 24(13):3374–82.e3. doi: 10.1016/j.celrep.2018.08.073
36. Wu T, Hu Y, Lee YT, Bouchard KR, Benechet A, Khanna K, et al. Lung-resident memory CD8 T cells (TRM) are indispensable for optimal cross-protection against pulmonary virus infection. J leuk Biol (2014) 95(2):215–24. doi: 10.1189/jlb.0313180
37. Gebhardt T, Wakim LM, Eidsmo L, Reading PC, Heath WR, Carbone FR. Memory T cells in nonlymphoid tissue that provide enhanced local immunity during infection with herpes simplex virus. Nat Immunol (2009) 10(5):524–30. doi: 10.1038/ni.1718
38. Fernandez-Ruiz D, Ng WY, Holz LE, Ma JZ, Zaid A, Wong YC, et al. Liver-resident memory CD8(+) T cells form a front-line defense against malaria liver-stage infection. Immunity. (2016) 45(4):889–902. doi: 10.1016/j.immuni.2016.08.011
39. Iijima N, Iwasaki A. T Cell memory. a local macrophage chemokine network sustains protective tissue-resident memory CD4 T cells. Science (2014) 346(6205):93–8. doi: 10.1126/science.1257530
40. Ariotti S, Beltman JB, Chodaczek G, Hoekstra ME, van Beek AE, Gomez-Eerland R, et al. Tissue-resident memory CD8+ T cells continuously patrol skin epithelia to quickly recognize local antigen. Proc Natl Acad Sci United States America (2012) 109(48):19739–44. doi: 10.1073/pnas.1208927109
41. Reilly EC, Lambert Emo K, Buckley PM, Reilly NS, Smith I, Chaves FA, et al. TRM integrins CD103 and CD49a differentially support adherence and motility after resolution of influenza virus infection. Proc Natl Acad Sci (2020) 117(22):12306–14. doi: 10.1073/pnas.1915681117
42. Corgnac S, Boutet M, Kfoury M, Naltet C, Mami-Chouaib F. The emerging role of CD8(+) tissue resident memory T (T(RM)) cells in antitumor immunity: A unique functional contribution of the CD103 integrin. Front Immunol (2018) 9:1904. doi: 10.3389/fimmu.2018.01904
43. Bartsch LM, Damasio MPS, Subudhi S, Drescher HK. Tissue-resident memory T cells in the liver-unique characteristics of local specialists. Cells. (2020) 9(11):1–22. doi: 10.3390/cells9112457
44. Cheuk S, Schlums H, Gallais Sérézal I, Martini E, Chiang SC, Marquardt N, et al. CD49a expression defines tissue-resident CD8+ T cells poised for cytotoxic function in human skin. Immunity. (2017) 46(2):287–300. doi: 10.1016/j.immuni.2017.01.009
45. Kim SK, Reed DS, Olson S, Schnell MJ, Rose JK, Morton PA, et al. Generation of mucosal cytotoxic T cells against soluble protein by tissue-specific environmental and costimulatory signals. Proc Natl Acad Sci United States America (1998) 95(18):10814–9. doi: 10.1073/pnas.95.18.10814
46. Zheng MZM, Wakim LM. Tissue resident memory T cells in the respiratory tract. Mucosal Immunol (2022) 15(3):379–88. doi: 10.1038/s41385-021-00461-z
47. Ariotti S, Hogenbirk MA, Dijkgraaf FE, Visser LL, Hoekstra ME, Song JY, et al. T Cell memory. skin-resident memory CD8⁺ T cells trigger a state of tissue-wide pathogen alert. Science (2014) 346(6205):101–5. doi: 10.1126/science.1254803
48. McMaster SR, Wilson JJ, Wang H, Kohlmeier JE. Airway-resident memory CD8 T cells provide antigen-specific protection against respiratory virus challenge through rapid IFN-γ production. J Immunol (2015) 195(1):203–9. doi: 10.4049/jimmunol.1402975
49. Pizzolla A, Nguyen TH, Sant S, Jaffar J, Loudovaris T, Mannering SI, et al. Influenza-specific lung-resident memory T cells are proliferative and polyfunctional and maintain diverse TCR profiles. J Clin Invest (2018) 128(2):721–33. doi: 10.1172/JCI96957
50. Topham DJ, Tripp RA, Doherty PC. CD8+ T cells clear influenza virus by perforin or fas-dependent processes. J Immunol (1997) 159(11):5197–200.
51. Moskophidis D, Kioussis D. Contribution of Virus-specific CD8+ cytotoxic T cells to virus clearance or pathologic manifestations of influenza virus infection in a T cell receptor transgenic mouse model. J Exp Med (1998) 188(2):223–32. doi: 10.1084/jem.188.2.223
52. de Bree GJ, van Leeuwen EM, Out TA, Jansen HM, Jonkers RE, van Lier RA. Selective accumulation of differentiated CD8+ T cells specific for respiratory viruses in the human lung. J Exp Med (2005) 202(10):1433–42. doi: 10.1084/jem.20051365
53. Piet B, de Bree GJ, Smids-Dierdorp BS, van der Loos CM, Remmerswaal EB, von der Thüsen JH, et al. CD8⁺ T cells with an intraepithelial phenotype upregulate cytotoxic function upon influenza infection in human lung. J Clin Invest (2011) 121(6):2254–63. doi: 10.1172/JCI44675
54. Koutsakos M, Illing PT, Nguyen THO, Mifsud NA, Crawford JC, Rizzetto S, et al. Human CD8(+) T cell cross-reactivity across influenza a, b and c viruses. Nat Immunol (2019) 20(5):613–25. doi: 10.1038/s41590-019-0320-6
55. Teijaro JR, Turner D, Pham Q, Wherry EJ, Lefrançois L, Farber DL. Cutting edge: Tissue-retentive lung memory CD4 T cells mediate optimal protection to respiratory virus infection. J Immunol (2011) 187(11):5510. doi: 10.4049/jimmunol.1102243
56. Nelson SA, Dileepan T, Rasley A, Jenkins MK, Fischer NO, Sant AJ. Intranasal nanoparticle vaccination elicits a persistent, polyfunctional CD4 T cell response in the murine lung specific for a highly conserved influenza virus antigen that is sufficient to mediate protection from influenza virus challenge. J virol (2021) 95(16):e0084121. doi: 10.1128/JVI.00841-21
57. Holmgren J, Czerkinsky C. Mucosal immunity and vaccines. Nat Med (2005) 11(4):S45–53. doi: 10.1038/nm1213
58. Belshe R, Lee MS, Walker RE, Stoddard J, Mendelman PM. Safety, immunogenicity and efficacy of intranasal, live attenuated influenza vaccine. Expert Rev Vaccines (2004) 3(6):643–54. doi: 10.1586/14760584.3.6.643
59. Cox RJ, Brokstad KA, Ogra P. Influenza virus: immunity and vaccination strategies. comparison of the immune response to inactivated and live, attenuated influenza vaccines. Scand J Immunol (2004) 59(1):1–15. doi: 10.1111/j.0300-9475.2004.01382.x
60. Jozwik A, Habibi MS, Paras A, Zhu J, Guvenel A, Dhariwal J, et al. RSV-Specific airway resident memory CD8+ T cells and differential disease severity after experimental human infection. Nat Commun (2015) 6(1):10224. doi: 10.1038/ncomms10224
61. Kinnear E, Lambert L, McDonald JU, Cheeseman HM, Caproni LJ, Tregoning JS. Airway T cells protect against RSV infection in the absence of antibody. Mucosal Immunol (2018) 11(1):249–56. doi: 10.1038/mi.2017.46
62. Tregoning J, Kinnear E. Using plasmids as DNA vaccines for infectious diseases. Microbiol Spectr (2014) 2. doi: 10.1128/microbiolspec
63. Szabo PA, Dogra P, Gray JI, Wells SB, Connors TJ, Weisberg SP, et al. Longitudinal profiling of respiratory and systemic immune responses reveals myeloid cell-driven lung inflammation in severe COVID-19. Immunity. (2021) 54(4):797–814.e6. doi: 10.1016/j.immuni.2021.03.005
64. Liao M, Liu Y, Yuan J, Wen Y, Xu G, Zhao J, et al. Single-cell landscape of bronchoalveolar immune cells in patients with COVID-19. Nat Med (2020) 26(6):842–4. doi: 10.1038/s41591-020-0901-9
65. Grau-Expósito J, Sánchez-Gaona N, Massana N, Suppi M, Astorga-Gamaza A, Perea D, et al. Peripheral and lung resident memory T cell responses against SARS-CoV-2. Nat Commun (2021) 12(1):3010. doi: 10.1038/s41467-021-23333-3
66. Liu X, Shaw RH, Stuart ASV, Greenland M, Aley PK, Andrews NJ, et al. Safety and immunogenicity of heterologous versus homologous prime-boost schedules with an adenoviral vectored and mRNA COVID-19 vaccine (Com-COV): a single-blind, randomised, non-inferiority trial. Lancet (London England) (2021) 398(10303):856–69. doi: 10.1016/S0140-6736(21)01694-9
67. Stuart ASV, Shaw RH, Liu X, Greenland M, Aley PK, Andrews NJ, et al. Immunogenicity, safety, and reactogenicity of heterologous COVID-19 primary vaccination incorporating mRNA, viral-vector, and protein-adjuvant vaccines in the UK (Com-COV2): a single-blind, randomised, phase 2, non-inferiority trial. Lancet (2022) 399(10319):36–49. doi: 10.1016/S0140-6736(21)02718-5
68. Wang Z, Schmidt F, Weisblum Y, Muecksch F, Barnes CO, Finkin S, et al. mRNA vaccine-elicited antibodies to SARS-CoV-2 and circulating variants. Nature. (2021) 592(7855):616–22. doi: 10.1038/s41586-021-03324-6
69. Jain S, Venkataraman A, Wechsler ME, Peppas NA. Messenger RNA-based vaccines: Past, present, and future directions in the context of the COVID-19 pandemic. Advanced Drug delivery Rev (2021) 179:114000. doi: 10.1016/j.addr.2021.114000
70. Teijaro JR, Farber DL. COVID-19 vaccines: modes of immune activation and future challenges. Nat Rev Immunol (2021) 21(4):195–7. doi: 10.1038/s41577-021-00526-x
71. Channappanavar R, Fett C, Zhao J, Meyerholz DK, Perlman S. Virus-specific memory CD8 T cells provide substantial protection from lethal severe acute respiratory syndrome coronavirus infection. J virol (2014) 88(19):11034–44. doi: 10.1128/JVI.01505-14
72. Zhao J, Zhao J, Mangalam AK, Channappanavar R, Fett C, Meyerholz DK, et al. Airway memory CD4(+) T cells mediate protective immunity against emerging respiratory coronaviruses. Immunity. (2016) 44(6):1379–91. doi: 10.1016/j.immuni.2016.05.006
73. Moskophidis D, Kioussis D. Contribution of virus-specific CD8+ cytotoxic T cells to virus clearance or pathologic manifestations of influenza virus infection in a T cell receptor transgenic mouse model. J Exp Med (1998) 188(2):223–32. doi: 10.1084/jem.188.2.223
74. Wang Z, Wang S, Goplen NP, Li C, Cheon IS, Dai Q, et al. PD-1hi CD8+ resident memory T cells balance immunity and fibrotic sequelae. Sci Immunol (2019) 4(36):eaaw1217. doi: 10.1126/sciimmunol.aaw1217
Keywords: TRM, respiratory pathogens, mucosal immunity, mucosal vaccines, T Cell immunity, influenza, COVID-19
Citation: Hassert M and Harty JT (2022) Tissue resident memory T cells- A new benchmark for the induction of vaccine-induced mucosal immunity. Front. Immunol. 13:1039194. doi: 10.3389/fimmu.2022.1039194
Received: 07 September 2022; Accepted: 21 September 2022;
Published: 04 October 2022.
Edited by:
Anukul T. Shenoy, Boston University, United StatesCopyright © 2022 Hassert and Harty. This is an open-access article distributed under the terms of the Creative Commons Attribution License (CC BY). The use, distribution or reproduction in other forums is permitted, provided the original author(s) and the copyright owner(s) are credited and that the original publication in this journal is cited, in accordance with accepted academic practice. No use, distribution or reproduction is permitted which does not comply with these terms.
*Correspondence: John T. Harty, am9obi1oYXJ0eUB1aW93YS5lZHU=
Disclaimer: All claims expressed in this article are solely those of the authors and do not necessarily represent those of their affiliated organizations, or those of the publisher, the editors and the reviewers. Any product that may be evaluated in this article or claim that may be made by its manufacturer is not guaranteed or endorsed by the publisher.
Research integrity at Frontiers
Learn more about the work of our research integrity team to safeguard the quality of each article we publish.