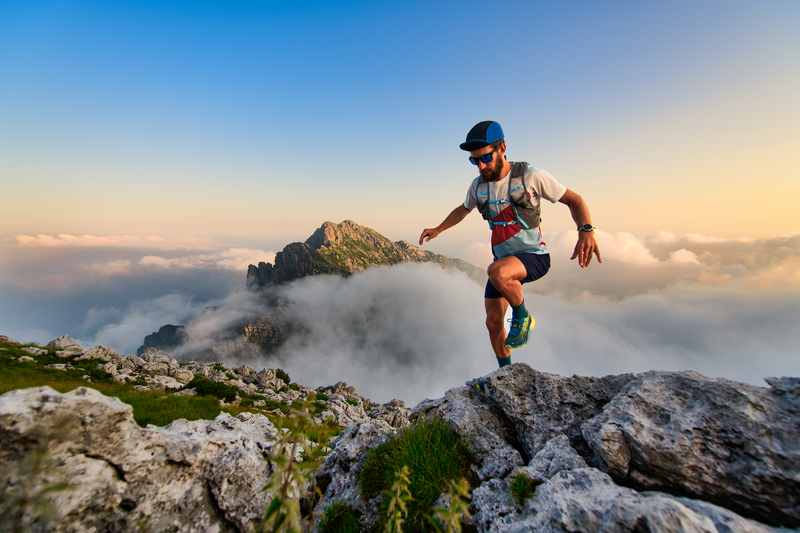
95% of researchers rate our articles as excellent or good
Learn more about the work of our research integrity team to safeguard the quality of each article we publish.
Find out more
REVIEW article
Front. Immunol. , 21 November 2022
Sec. Cancer Immunity and Immunotherapy
Volume 13 - 2022 | https://doi.org/10.3389/fimmu.2022.1037124
This article is part of the Research Topic Genome Editing Applications of CRISPR/Cas9 in Metabolic Diseases, Hormonal System and Cancer Research View all 5 articles
Human papillomaviruses (HPVs) have been recognized as the etiologic agents of various cancers and are called HPV-driven cancers. Concerning HPV-mediated carcinogenic action, gene therapy can cure cancer at the molecular level by means of the correction of specific genes or sites. CRISPR-Cas9, as a novel genetic editing technique, can correct errors in the genome and change the gene expression and function in cells efficiently, quickly, and with relative ease. Herein, we overviewed studies of CRISPR-mediated gene remedies for HPV-driven cancers and summarized the potential applications of CRISPR-Cas9 in gene therapy for cancer.
Human papillomaviruses (HPVs) are nonenveloped epitheliotropic viruses with eight coding genes and a circular double-stranded DNA genome (1). HPVs are important human pathogens, and many viral diseases, such as cervical cancer caused by HPVs, have received immense attention as a result of their high transmission rates and difficulty in curing (2). During HPV-driven cancer development, viral DNA is frequently integrated into host cell chromosomes, and the proteins encoded by viral genes play a critical role in carcinogenesis (3, 4).
Collectively, HPV-driven cancers include cervical cancer, anal cancer, oral cancer, oropharyngeal cancer, and other cancers. In recent decades, many studies have reported various breakthroughs in the field of cervical cancer, with the earliest and deepest progress on the HPV-relevant mechanism. A further study explored the prevention and treatment of cervical cancer and used it as the breach point of other HPV-driven cancers (5).
The CRISPR-Cas9 system is an adaptive defense network in microorganisms and has become the leading technology for genome editing (6). Compared with other genome editing technologies, such as ZFNs and TALENs, it has the advantage of being programmable with short RNAs, which makes it easier to use (7). There is already a study indicating that CRISPR-Cas9 has a role in targeting DNA (8) and was subsequently proven to be able to edit human chromosomal DNA (9). Building on these studies, in 2020, a phase I clinical trial of CRISPR-Cas9 was completed in patients with advanced non-small cell lung cancer (10), which opened the beginning of the technology for the clinical treatment of oncology. In recent years, CRISPR-Cas9 has been increasingly used to target the HPV gene that induces most cervical cancer tumors. In 2014, the E6 and E7 genes of the HPV virus were targeted by using CRISPR-Cas9, leading to the death of tumor cells (11). This study provides a theoretical basis for the treatment of HPV-induced cancers. Studies on the stability and safety of CRISPR-Cas9 are still ongoing, as preclinical and clinical studies have been conducted.
In this review, we discuss the current state of development of CRISPR-Cas9 for HPV clinical treatment based on a summary of the theoretical rationale and relevant trials. We also highlight the potential application of this technology for HPV clinical treatment.
CRISPR-Cas9 systems are divided into two primary classes. CRISPR-Cas9 belongs to type II in Class 2 (12). It consists of a CRISPR array in the middle and several Cas genes on both sides, including the specific cas9 gene. The CRISPR RNA transcribed by the CRISPR array (gRNA) acts to direct the nuclease Cas9 to generate a double-strand break (DSB) at the target site (13). (Figure 1)
First, after invading the host, exogenous DNA is cleaved into several DNA fragments called protospacers. Protospacers will then be inserted between the high-frequency repeat fragments to form the CRISPR array. The proteins Cas1 and Cas2 play an important role in this process (12, 14, 15). The Cas1 protein has been proven to have endonuclease activity and directs protospacer insertion between repetitive fragments (16). However, how the Cas2 protein works remains unclear.
Subsequently, the CRISPR array will be transcribed to generate precrRNAs that match the target gene. The CRISPR site located upstream of the CRISPR array is transcribed to generate tracrRNA. TracrRNA has a fragment homologous to precrRNA and therefore can bind to it to form a precrRNA/tracrRNA complex. This complex is further processed to form the mature tracrRNA-crRNA complex known as gRNA. The gRNA will then bind to Cas9 and direct Cas9 to the target site for DNA cleavage to generate DSBs. Every single strand is cleaved by a different structural domain of Cas (17). Whether the intended site can be targeted depends on a short sequence called the Protospacer Adjacent Motif (PAM) located downstream of the intended target site. PAM which controls the targeting specification is recognized by Cas9 and is specific to each subgroup of the CRISPR-Cas9 system (18, 19).
Finally, cutting target DNA to produce DSBs by the nuclease Cas9 initiates the host self-repair machinery, including nonhomologous end joining (NHEJ) and homology-directed repair (HDR) (20). These two restoration mechanisms mediate mutations such as substitutions, deletions, and insertions in the target DNA (17, 21, 22).
Off-target effects are one of the biggest problems facing CRISPR-Cas9 in clinical applications. A study demonstrated that Cas9 nucleic acid endonuclease has high activity even in the presence of gRNA bootstrap mismatch, which would greatly reduce the safety of its clinical application (23). But it has been demonstrated that CRISPR-Cas9 has higher cleavage efficiency and lower off-target rates than TALEN and ZFN gene therapy technologies in the treatment of HPV infection (24). On the other hand, it has been reported that off-target can be minimized by optimizing gRNA sequences as well as Cas9, among other ways (25). For example, Komor et al. significantly improved targeting rates using Cas9-nickase, a D10 mutant of Cas9 (26). And many CRIPSR-Cas9-based editors such as cytosine base-editors (CBEs), adenine base-editors (ABEs) and Prime-editors (PEs) have also been designed to achieve smaller insertion or deletion of mutations (27). More variants of CRISPR and Cas9 are being investigated to optimize this technique. Therefore, although the clinical application of CRISPR-Cas9 still needs to overcome many difficulties, it is still the optimal choice for gene therapy of HPV virus infection.
To apply CRISPR-Cas9 to tumor therapy, in 2012, a single-guide RNA (gRNA) was first designed to contain all the components needed for prerRNA/tracrRNA to guide the Cas9 nuclease (8). Soon after, in 2014, Zhen et al. reported for the first time that targeting the HPV E6/E7 gene using CRISPR-Cas could inhibit tumor cell growth. Researches on CRISPR-Cas for HPV-associated cancers have increased rapidly since then (28).
Studies have proven that over 99% of cervical cancers are directly related to high-risk HPV infection (29). Two subtypes, HPV16 and HPV18, are predominant. The genome of the HPV virus consists of two main parts. The first part is the early region (E), whose main function is to participate in the regulation of virus replication and life cycle; the other part is the late region (L), whose main function is to encode the capsid protein that forms the virus (30). In the genome of HPV, the E6 and E7 genes are the major oncogenes. The E6 oncogene functions by inhibiting the p53 cancer suppressor pathway and blocking the RIG-I signaling pathway (immune escape mechanism), and the E7 oncogene suppresses retinoblastoma protein (Rb) and affects p21 and other pathways (31–33). (Figure 2) A growing number of studies have demonstrated that the E6 and E7 genes play a critical role in enabling the induction of apoptosis and cell cycle arrest (34). Therefore, the E6 and E7 genes have also been widely studied by many scholars as to the main target loci (Table 1).
In 2014, HPV16-E7 was first recognized as a goal of motion of the CRISPR-Cas9 machine for gene remedying HPV virus-positive cervical cancer (31). In the same year, another study also demonstrated that the CRISPR-Cas9 system targeting the E6 and E7 loci led to a significant accumulation of p53 and p21, which significantly reduced the proliferation of cervical cancer cells in vitro, and this finding was also demonstrated in vivo in a mouse model (28). In subsequent studies, it was found that knocking out the E6 and E7 genes could inhibit cervical cancer cell proliferation, in addition to several other effects. In 2016, a study confirmed that focused inactivation of the HPV16 E6/E7 gene might also be a high-quality sensitizer of CDDP chemotherapy in cervical cancer (36), providing new ideas for additional gene therapy strategies. The same research team again found that blocking the PD-1 pathway and the HPV16 E6/E7 gene may have a synergistic effect and together enhance the antitumor effect in 2019 (46).
In addition to the treatment targeting the E6/E7 gene, some scholars have also turned their attention to investigating other HPV oncogenic mechanisms using the CRISPR-Cas9 system. In 2017, the SIRT1 gene was knocked out by CRISPR-Cas9, demonstrating the important regulatory role of the cytosolic enzyme SIRT1 in HPV16 replication (37). Subsequently, in 2020, the same research team continued to investigate the regulatory role of the SIRT1-WRN axis using CRISPR-Cas9 technology and noted the dependence of the viral replication cycle on WRN (47). Some scholars have also started to study the host proteins that interact with the transcription products of E6/E7 genes. For example, in 2020, a study found that binding of the E7 oncoprotein of HPV16 and HPV18 to the host tumor suppressor PTPN14 would inhibit the expression of differentiation genes and demonstrated experimentally that mutating the PTPN14 gene in cervical cancer cells by CRISPR-Cas9 would significantly reduce the oncogenic activity of HPV viruses (50). Many studies on CRISPR-Cas9 system-targeted gene therapy are underway.
As a therapeutic technique, gene therapy can work by replacing specific molecular defects of genes that contribute to the development or progression of cancer. This therapy has been widely applied, including in cardiovascular diseases, vaccination, and cancers in which conventional therapies have failed. For HPV-driven cancer, various gene therapy approaches have been developed and verified. CRISPR-Cas9, unlike traditional gene-editing technology, can provide an easy way to edit specific sites in the genome and thus offers tremendous opportunities for more diseases. We briefly summarized the CRISPR-Cas9 in various potential applications in HPV (Table 2). Detailed information will be introduced below.
There is a common etiologic feature in HPV-driven cancer that the emergence and function of viral oncogene expression (E6/E7) are related to the tumor cells, far away from stromal cells. Relevant research confirmed that most HPV-driven cancers can classify the oncogenic proteins E6/E7 to inactivate the host tumor suppressors p53 and RB, respectively. In the process of cell oncogenesis, E6 and E7 promote the replication of the viral genome and induce malignant biological properties, including uncontrolled cellular proliferation, angiogenesis, invasion, and metastasis (75) (Figure 3). A previous study showed that E6/E7-inactivated HeLa cells displayed distinctive senescence markers, such as an enlarged cell surface area (1).
E6/E7 and their complex have deserved as specific targets in gene therapy. Several strategies targeting E6/E7 have been reported, including cytotoxic drugs and zinc-ejecting inhibitors of the viral E6 oncoprotein (76–78), E7 antagonist peptide (79), and HSP90 and GRP78 inhibitors targeting E6 and E7 (80). Recently, CRISPR-Cas9 has entered into clinical trials as a novel therapeutic strategy. The therapeutic mechanism of CRISPR-Cas9-mediated downregulation of E6 and E7 normally attributes to the inactivation of p53 and RB to set off apoptosis and mobile senescence.
High-risk HPVs (such as HPV-16 and HPV-18) can encode viral oncoproteins E6 and E7 in tumor cells, most common in cervical and penile cancers. The targeted gene by CRISPR-Cas9 performed well in a large number of studies. In 2014, the first CRISPR-Cas9 system featuring HPV16-E7 single-guide RNA (gRNA) showed disruption of HPV16-E7 DNA at specific sites induced apoptosis and growth inhibition of HPV-driven cancer cells (31). Further study conducted gene therapy in the K14-HPV16 transgenic mouse model, which had a favorable effect on cervical precancer (53). In 2015, the HPV16-E6 oncogene was cleaved by the customized CRISPR gRNA/Cas9, which demonstrated for the first time that it could be a therapeutic approach to reverse the malignant phenotype and increase the expression of p53 (55). Both viral oncoproteins are therefore regarded as promising targets for gene therapy. The knockdown of E6/E7 has been pronounced in in vivo and vitro trials alongside an accumulation of p53 and p21 protein and should result in remarkably issue of the proliferation of HPV-driven most cancers cells (36, 56).
In addition, other HPV types were also studied for their biological properties and use in genetic therapy with CRISPR-Cas9. As an HR-HPV, HPV-18 has been targeted and tested in vitro and in vivo. Previous studies showed that oncogenes E6 and E7 of HPV18 could be successfully inhibited by the CRISPR-Cas9 system (39, 40, 57, 58). However, HPV-6/11, which are low-risk HPV types most common in anogenital warts and laryngeal papillomatosis, also encode the oncoproteins E6/E7. Thus, a CRISPR−Cas9 system centered on HPV6/11 has been stated as a novel and fairly effective molecular purpose for the treatment and prevention of low-risk HPV-driven diseases (35).
With the deepening look up on HPV, more gene sequences will flip out to be ambitions of gene treatment for HPV illness or remedy of HPV-driven cancer. CRISPR-Cas9 can additionally be used in the preparation of vaccines to prevent HPV infection (81). Nonetheless, the pleasant transport of developed Cas9 plasmids in vivo remains a challenge. Further look up should be carried out to transport Cas9 and gRNA into the intention cell of the human physique and to make positive the biosafety need.
As a promising progressive technological know-how in gene editing, CRISPR-Cas9 provides scientists with a number of alternatives to manipulate the genome of cancers and trade the DNA structure.
Oncogenes are a group of mutated genes that may cause cancer, such as JunB and PIM1. JunB is a unique factor of activator protein-1 transcription factors, appearing both as a tumor suppressor or as an oncogene relying on the cell context (82). JunB knockdown and knockout limited the progression of tumor migration and invasion, suggesting that the downregulation of JunB expression might be a potential therapeutic strategy for inhibiting distant metastasis in patients with HPV-driven cancer (59). Another oncogene, PIM1, encoding a constitutively active serine/threonine protein kinase, was investigated for its functional roles in the viability and growth of HPV-driven cancer cells (83). CRISPR-Cas9-mediated exchange of PIM1 resulted in cell cycle arrest and apoptosis in HPV-driven most cancers (62). In addition, the genetic depletion of CDK7 using the CRISPR−Cas9 system exhibited great cell growth inhibition in cervical cancer cell lines (43). Additionally, HPV16-transformed cells with CRISPR−Cas9-mediated loss of p53 were inclined to lose dependence on the continuous expression of HPV oncogenes for proliferation (63).
The identification of cell surface markers in cancer can establish differentiation to target specific sites. Some research confirmed a novel affiliation between HPV-E6 oncoprotein expression and the increase in the CD55 and CD71 floor markers in most cervical cancer cells (60, 61). The HPV-E6 oncoprotein enriched the CD55 and CD71 populations, which increased cell proliferation, cell self-renewal ability, cell migration, radioresistance, and tumorigenicity. The knockdown or knockout of CD55 and CD71 expression in HPV-E6-expressing cells should reverse the tumorigenic phenotypes of most cervical cancer cells.
HPV plays a role in the pathologic process of HPV-driven cancers. This finding suggests that HPV prophylactic vaccines will have a wider range of protection, making us far from a series of HPV-driven cancers, especially in head and neck cancer and cervical cancer, where the expression of HPV is relatively high.
With the advent of immune checkpoint inhibitors (ICIs), immunotherapy has emerged as one of the most promising therapeutic strategies for cancer. CRISPR−Cas9, as a versatile and easily used genetic enhancing technology, is frightening a progressive change in most cancer immunotherapies. Recently, CRISPR−Cas9 has been carried out to incite the improvement of therapeutic immune agents, such as chimeric antigen receptor T (CAR-T) cells and the programmed cell dying protein-1 (PD-1) or its ligand (PD-L1) (84).
PD-1 and PD-L1 are negative regulators of the immune responses of T cells (85). Expressed on cervical T cells and DCs. PD-1 and PD-L1 have been lately pronounced to be related to excessive risk-HPV positivity and to be increased along with growing cervical intraepithelial neoplasia (CIN) grade (86). Furthermore, the researcher established a C33 cell line stably expressing HPV16 E6/E7 (SiHa-E6/E7) to verify the promotive association between HPV16 E6 and the expression of PD-1. Based on this, they tested whether combined targeted therapy with immunotherapy can 1 + 1 equal more than 2 in the SiHa cervical cancer mouse model. Synergistic effects have been reported for combination therapy targeting HPV16 E6/E7 and PD-1 blockade using CRISPR-Cas9 (46).
EZH2, a catalytic subunit of polycomb repressive complex 2 (PRC2), was reported to block PD-1/PD-L1 axis downregulation. High expression of EZH2 was also associated with tumor cell proliferation, invasion, and metastasis and has important clinicopathologic significance (87). EZH2 knockdown or inhibition has been tested in mice with induced endometriosis and prompted EZH2-induced epithelial-mesenchymal transition (EMT) in cancers (88). Further research identified EZH2 as a potential therapeutic target for encouraging antigen presentation and antitumor immunity in head and neck squamous cell carcinoma (HNSCC) (64). The combination of EZH2 inhibition and anti-PD-1 therapy may be beneficial for patients with HNSCC, which requires further preclinical studies.
Academic clinical trials have investigated T cells with PD-1 knocked out by CRISPR-Cas9 for the treatment of multiple types of cancer. Among the trials, it has recently attained a significant therapeutic effect on NSCLC (10). However, we have not found any research directly exploring novel and promising cancer immunotherapy with the knockout of CAR-T or PD-1 by CRISPR−Cas9 in HPV-driven cancers.
For patients with HPV-driven cancers, conventional therapy includes chemotherapy, radiotherapy, or subsequent chemoradiotherapy (CCRT). One of the major issues in clinical oncology is the ability of cancer cells to resist chemotherapy drugs, which leads to chemotherapy failure.
In 2016, the first record of HPV16 E6/E7 focused on CRISPR-Cas9 was published, in which the method was once described as a nice sensitizer for bettering CDDP chemotherapy in cervical cancer (36). It ought to efficiently and especially coordinate with CDDP for HPV16 fantastic cervical cancer. This further indicated the position of the blended lineage leukemia 5 (MLL5) in the carcinogenesis of most HPV-positive cervical cancer cells (48). Knockout of MLL5 greatly impacted the chemotherapeutic effectivity of cisplatin in HPV-18-positive cells. Additionally, the finding that MLL5 has a higher anticancer effect than E6 by means of CRISPR-Cas9 has an impact on the disruption of MLL5.
For head and neck squamous cell carcinoma (HNSCC), radiotherapy is one of the most commonly used and effective treatments. However, different HPV genotypes of HPV-driven cancers, such as cervical cancer patients with HPV-18 DNA, have significantly different responses to radiotherapy (89). Recently, a novel screen based on a targeted CRISPR−Cas9 system was applied to identify optimal radiosensitization approaches for HPV-positive/negative HNSCC (90). The combination of radiotherapy and CRISPR−Cas9-mediated inhibition of genetic repair pathways could improve the therapeutic response in patients with HNSCC.
Nucleic acid detection techniques are always crucial to diagnosis, especially in the background of the present coronavirus disease 2019 pandemic. Despite a wide range of applications of genetic testing tools, the CRISPR-Cas9 system has advantages in the detection field and has been applied in gene editing and regulation. We know that polymerase chain reaction (PCR) is widely applied due to the high sensitivity of the exponential amplification of target DNA. Therefore, PCR has been the most popular DNA detection and genotyping technique, such as detecting SARS-CoV-2 and diagnosing the current COVID-19 pandemic. To date, the combination of PCR and CRISPR techniques provides a new chance for developing new nucleic acid detection and typing techniques.
In 2018, Qiao and Beibei first developed CRISPR-Cas9-associated reverse PCR (CARP), in which Cas9-cut target DNA was cyclized and detected by reverse PCR amplification (71). Due to the reverse PCR amplification of the DNA of interest that was performed in detection, this method had high sensitivity. Based on the technique, they then developed a new method for detecting and typing target DNA based on Cas9 nuclease, which was named ctPCR (72). By using qPCR machines, the whole ctPCR detection process can be finished in as little as 3 to 4 hours. Thus, ctPCR should be useful in DNA detection and genotyping. However, the target DNA was detected and genotyped based on comparing the Ct values and DNA copies of two qPCRs. Afterward, a new version of ctPCR was developed to avoid this comparison step, which symbolized one-pot detection (73). The whole detection process can be finished on PCR instruments without further tube opening.
Outside of the PCR technique, another CRISPR-Cas9-mediated DNA detection method called CRISPR−Cas9-assisted DNA detection (CADD) was developed (74). The detection of target DNA could be completed in less than 30 min, according to the unique advantages over current methods, such as being simple, rapid, and free of preamplification and the application of fluorescent hybridization chain reaction (HCR).
The delivery system of CRISPR-Cas9 applied in the human body remains a challenge. Even when many specific molecular targets are available to select for tumor cells, it is quite controversial to identify an effective and safe transport system. To overcome this issue, researchers have exploited several kinds of carriers, from viral delivery systems to liposomes and from plasmids to nanoparticles. We discussed the pros and cons of each delivery system based on current research reports.
Among the viral shipping vectors, high-capacity adenoviral vectors (HCAdV) have the potential for packaging up to 35 kb, permitting handing over the entire CRISPR-Cas9 equipment inclusive of numerous gRNAs (68). In contrast to adenoviral vectors (AdV), they had no threat of expressing AdV genes with much less immunogenic properties (91). The proof-of-concept for the use of CRISPR-Cas9 delivered by the most superior adenoviral vector (HCAdV) has a considerable impact in treating HPV-derived tumors (57). Nevertheless, manufacturing of HCAdV is intensive in time and work when compared with AAV-vector platforms, hampering their exploration for unique applications. Different from the normal viral shipping system, AAV-based shipping structures have shown predominant benefits (92), which have attracted much attention, especially for therapeutic purposes. Additionally, AAV can have stable transgene expression with long-term existence as a concatemer in nondividing cells (93). The AAV-based CRISPR-Cas9 machine has been used for disruption of the E6 gene in HeLa, which emphasized AAV-based viral vectors as one of the most sensitive viral vectors for gene remedy and gene switch in vivo (40, 58).
However, the viable cytotoxicity, immunogenic response, and long-term expression of viral vectors continue to be issues of scientific application (94). Several nonviral transport techniques have been reported. In 2019, a study indicated the viability of endogenous exosomes as a protected and fantastic transport carrier of the purposeful gRNA and Cas9 protein (70).Scientific hobby in nanoparticles (NPs) is on the rise due to their versatility and, in particular, their large applicability (95). A study has developed NPs consisting of PBAE546 and CRISPR-Cas9 for the treatment of HPV infection, which provides new hope for the clinical transformation of nanomedicine to treat cervical lesions, thereby preventing cervical cancer (52). Other research also confirmed this genetic strategy effectively (49, 67, 96).
CRISPR-Cas9 technology presents a new device for the genetic detection and remedy of most HPV-driven cancers from a specific aspect. With numerous benefits over traditional methods, such as being simple to design, easy to use, and efficient to edit, the remedy affords a promising method for medical applications. However, nearly complete gene treatment plans associated with CRISPR-Cas9 continue to be in the experimental phase, with current off-target consequences and other safety perils. Collectively, the novel genetic cure of HPV-driven most cancers can be anticipated with the leap forward of CRISPR-Cas9 technology.
YW and ZZ contributed equally to this work. YW and ZZ participated in the literature search and data collection. YW wrote the manuscript in consultation with ZZ. XM participated in the study conception and manuscript revision. All authors discussed the results and contributed to the final manuscript.
The authors declare that the research was conducted in the absence of any commercial or financial relationships that could be construed as a potential conflict of interest.
All claims expressed in this article are solely those of the authors and do not necessarily represent those of their affiliated organizations, or those of the publisher, the editors and the reviewers. Any product that may be evaluated in this article, or claim that may be made by its manufacturer, is not guaranteed or endorsed by the publisher.
1. Inturi R, Jemth P. CRISPR/Cas9-based inactivation of human papillomavirus oncogenes E6 or E7 induces senescence in cervical cancer cells. Virology (2021) 562:92–102. doi: 10.1016/j.virol.2021.07.005
2. Choi JH, Shin M, Yang L, Conley B, Yoon J, Lee SN, et al. Clustered regularly interspaced short palindromic repeats-mediated amplification-free detection of viral DNAs using surface-enhanced raman spectroscopy-active nanoarray. ACS Nano (2021) 15: 13475–85. doi: 10.1021/acsnano.1c03975
3. Cain JM, Howett MK. Preventing cervical cancer. Science (2000) 288(5472):1753–5. doi: 10.1126/science.288.5472.1753
4. Integrated genomic and molecular characterization of cervical cancer. Nature (2017) 543(7645):378–84. doi: 10.1038/nature21386
5. Alsbeih G. HPV infection in cervical and other cancers in Saudi Arabia: Implication for prevention and vaccination. Front Oncol (2014) 4:65. doi: 10.3389/fonc.2014.00065
6. Zhang Y, Li M. Genome editing technologies as cellular defense against viral pathogens. Front Cell Dev Biol (2021) 9:716344. doi: 10.3389/fcell.2021.716344
7. Batista AC, Pacheco LGC. Detecting pathogens with zinc-finger, TALE and CRISPR- based programmable nucleic acid binding proteins. J Microbiol Methods (2018) 152:98–104. doi: 10.1016/j.mimet.2018.07.024
8. Jinek M, Chylinski K, Fonfara I, Hauer M, Doudna JA, Charpentier E. A programmable dual-RNA-guided DNA endonuclease in adaptive bacterial immunity. Science (2012) 337(6096):816–21. doi: 10.1126/science.1225829
9. Jinek M, East A, Cheng A, Lin S, Ma E, Doudna J. RNA-Programmed genome editing in human cells. Elife (2013) 2:e00471. doi: 10.7554/eLife.00471
10. Lu Y, Xue J, Deng T, Zhou X, Yu K, Deng L, et al. Safety and feasibility of CRISPR-edited T cells in patients with refractory non-small-cell lung cancer. Nat Med (2020) 26(5):732–40. doi: 10.1038/s41591-020-0840-5
11. Kennedy EM, Kornepati AV, Goldstein M, Bogerd HP, Poling BC, Whisnant AW, et al. Inactivation of the human papillomavirus E6 or E7 gene in cervical carcinoma cells by using a bacterial CRISPR/Cas RNA-guided endonuclease. J Virol (2014) 88(20):11965–72. doi: 10.1128/jvi.01879-14
12. Karimian A, Azizian K, Parsian H, Rafieian S, Shafiei-Irannejad V, Kheyrollah M, et al. CRISPR/Cas9 technology as a potent molecular tool for gene therapy. J Cell Physiol (2019) 234(8):12267–77. doi: 10.1002/jcp.27972
13. Zhang F, Wen Y, Guo X. CRISPR/Cas9 for genome editing: progress, implications and challenges. Hum Mol Genet (2014) 23(R1):R40–6. doi: 10.1093/hmg/ddu125
14. Makarova KS, Wolf YI, Alkhnbashi OS, Costa F, Shah SA, Saunders SJ, et al. An updated evolutionary classification of CRISPR-cas systems. Nat Rev Microbiol (2015) 13(11):722–36. doi: 10.1038/nrmicro3569
15. Yosef I, Goren MG, Qimron U. Proteins and DNA elements essential for the CRISPR adaptation process in escherichia coli. Nucleic Acids Res (2012) 40(12):5569–76. doi: 10.1093/nar/gks216
16. Nuñez JK, Kranzusch PJ, Noeske J, Wright AV, Davies CW, Doudna JA. Cas1-Cas2 complex formation mediates spacer acquisition during CRISPR-cas adaptive immunity. Nat Struct Mol Biol (2014) 21(6):528–34. doi: 10.1038/nsmb.2820
17. Kim EJ, Kang KH, Ju JH. CRISPR-Cas9: a promising tool for gene editing on induced pluripotent stem cells. Korean J Internal Med (2017) 32(1):42–61. doi: 10.3904/kjim.2016.198
18. Rodríguez-Rodríguez DR, Ramírez-Solís R, Garza-Elizondo MA, Garza-Rodríguez ML, Barrera-Saldaña HA. Genome editing: A perspective on the application of CRISPR/Cas9 to study human diseases (Review). Int J Mol Med (2019) 43(4):1559–74. doi: 10.3892/ijmm.2019.4112
19. Zhan T, Rindtorff N, Betge J, Ebert MP, Boutros M. CRISPR/Cas9 for cancer research and therapy. Semin Cancer Biol (2019) 55:106–19. doi: 10.1016/j.semcancer.2018.04.001
20. Wyman C, Kanaar R. DNA Double-strand break repair: all’s well that ends well. Annu Rev Genet (2006) 40:363–83. doi: 10.1146/annurev.genet.40.110405.090451
21. Ratan ZA, Son YJ, Haidere MF, Uddin BMM, Yusuf MA, Haidere SB, et al. CRISPR-Cas9: a promising genetic engineering approach in cancer research. Ther Adv Med Oncol (2018) 10:1758834018755089. doi: 10.1177/1758834018755089
22. Tiruneh GMM, Chekol Abebe E, Sisay T, Berhane N, Bekele T, Asmamaw Dejenie T. Current applications and future perspectives of CRISPR-Cas9 for the treatment of lung cancer. Biologics: Targets Ther (2021) 15:199–204. doi: 10.2147/btt.S310312
23. Fu Y, Foden JA, Khayter C, Maeder ML, Reyon D, Joung JK, et al. High-frequency off-target mutagenesis induced by CRISPR-cas nucleases in human cells. Nat Biotechnol (2013) 31(9):822–6. doi: 10.1038/nbt.2623
24. Cui Z, Liu H, Zhang H, Huang Z, Tian R, Li L, et al. The comparison of ZFNs, TALENs, and SpCas9 by GUIDE-seq in HPV-targeted gene therapy. Mol Ther Nucleic Acids (2021) 26:1466–78. doi: 10.1016/j.omtn.2021.08.008
25. Zhang XH, Tee LY, Wang XG, Huang QS, Yang SH. Off-target effects in CRISPR/Cas9-mediated genome engineering. Mol Ther Nucleic Acids (2015) 4(11):e264. doi: 10.1038/mtna.2015.37
26. Komor AC, Kim YB, Packer MS, Zuris JA, Liu DR. Programmable editing of a target base in genomic DNA without double-stranded DNA cleavage. Nature (2016) 533(7603):420–4. doi: 10.1038/nature17946
27. Kantor A, McClements ME, MacLaren RE. CRISPR-Cas9 DNA base-editing and prime-editing. Int J Mol Sci (2020) 21(17):6240. doi: 10.3390/ijms21176240
28. Zhen S, Hua L, Takahashi Y, Narita S, Liu YH, Li Y. In vitro and in vivo growth suppression of human papillomavirus 16-positive cervical cancer cells by CRISPR/Cas9. Biochem Biophys Res Commun (2014) 450(4):1422–6. doi: 10.1016/j.bbrc.2014.07.014
29. Münger K, Phelps WC, Bubb V, Howley PM, Schlegel R. The E6 and E7 genes of the human papillomavirus type 16 together are necessary and sufficient for transformation of primary human keratinocytes. J Virol (989) 63(10):4417–21. doi: 10.1128/jvi.63.10.4417-4421.1989
30. Zhen S, Li X. Oncogenic human papillomavirus: Application of CRISPR/Cas9 therapeutic strategies for cervical cancer. Cell Physiol Biochem (2017) 44(6):2455–66. doi: 10.1159/000486168
31. Hu Z, Yu L, Zhu D, Ding W, Wang X, Zhang C, et al. Disruption of HPV16-E7 by CRISPR/Cas system induces apoptosis and growth inhibition in HPV16 positive human cervical cancer cells. BioMed Res Int (2014) 2014:612823. doi: 10.1155/2014/612823
32. Thatte J, Banks L. Human papillomavirus 16 (HPV-16), HPV-18, and HPV-31 E6 override the normal phosphoregulation of E6AP enzymatic activity. J Virol (2017) 91(22). doi: 10.1128/jvi.01390-17
33. Chiang C, Pauli EK, Biryukov J, Feister KF, Meng M, White EA, et al. The human papillomavirus E6 oncoprotein targets USP15 and TRIM25 to suppress RIG-I-Mediated innate immune signaling. J Virol (2018) 92(6). doi: 10.1128/jvi.01737-17
34. Mittal S, Banks L. Molecular mechanisms underlying human papillomavirus E6 and E7 oncoprotein-induced cell transformation. Mutat Res Rev Mutat Res (2017) 772:23–35. doi: 10.1016/j.mrrev.2016.08.001
35. Liu YC, Cai ZM, Zhang XJ. Reprogrammed CRISPR-Cas9 targeting the conserved regions of HPV6/11 E7 genes inhibits proliferation and induces apoptosis in E7-transformed keratinocytes. Asian J Androl (2016) 18(3):475–9. doi: 10.4103/1008-682x.157399
36. Zhen S, Lu JJ, Wang LJ, Sun XM, Zhang JQ, Li X, et al. In vitro and In vivo synergistic therapeutic effect of cisplatin with human Papillomavirus16 E6/E7 CRISPR/Cas9 on cervical cancer cell line. Transl Oncol (2016) 9(6):498–504. doi: 10.1016/j.tranon.2016.10.002
37. Das D, Smith N, Wang X, Morgan IM. The deacetylase SIRT1 regulates the replication properties of human papillomavirus 16 E1 and E2. J Virol (2017) 91(10). doi: 10.1128/jvi.00102-17
38. Sun YZ, Ren Y, Zhang YJ, Han Y, Yang Y, Gao YL, et al. DNAJA4 deficiency enhances NF-kappa b-related growth arrest induced by hyperthermia in human keratinocytes. J Dermatol Sci Sep (2018) 91(3):256–67. doi: 10.1016/j.jdermsci.2018.05.006
39. Wang J, Guo M, Wang Q, Dang J, Liu X, Jin Z. Blocking activity of the HPV18 virus in cervical cancer cells using the CRISPR/Cas9 system. Int J Clin Exp Pathol (2018) 11(8):4230–5.
40. Yoshiba T, Saga Y, Urabe M, Uchibori R, Matsubara S, Fujiwara H, et al. CRISPR/Cas9-mediated cervical cancer treatment targeting human papillomavirus E6. Oncol Lett (2019) 17(2):2197–206. doi: 10.3892/ol.2018.9815
41. Jubair L, Fallaha S, McMillan NAJ. Systemic delivery of CRISPR/Cas9 targeting HPV oncogenes is effective at eliminating established tumors. Mol Ther (2019) 27(12):2091–9. doi: 10.1016/j.ymthe.2019.08.012
42. James CD, Prabhakar AT, Otoa R, Evans MR, Wang X, Bristol ML, et al. SAMHD1 regulates human papillomavirus 16-induced cell proliferation and viral replication during differentiation of keratinocytes. mSphere (2019) 4(4). doi: 10.1128/mSphere.00448-19
43. Zhong S, Zhang Y, Yin X, Di W. CDK7 inhibitor suppresses tumor progression through blocking the cell cycle at the G2/M phase and inhibiting transcriptional activity in cervical cancer. Onco Targets Ther (2019) 12:2137–47. doi: 10.2147/ott.S195655
44. Imahorn E, Aushev M, Herms S, Hoffmann P, Cichon S, Reichelt J, et al. Gene expression is stable in a complete CIB1 knockout keratinocyte model. Sci Rep (2020) 10(1):14952. doi: 10.1038/s41598-020-71889-9
45. Broutian TR, Jiang B, Li J, Akagi K, Gui S, Zhou Z, et al. Human papillomavirus insertions identify the PIM family of serine/threonine kinases as targetable driver genes in head and neck squamous cell carcinoma. Cancer Lett (2020) 476:23–33. doi: 10.1016/j.canlet.2020.01.012
46. Zhen S, Lu J, Liu YH, Chen W, Li X. Synergistic antitumor effect on cervical cancer by rational combination of PD1 blockade and CRISPR-Cas9-mediated HPV knockout. Cancer Gene Ther (2020) 27(3-4):168–78. doi: 10.1038/s41417-019-0131-9
47. James CD, Das D, Morgan EL, Otoa R, Macdonald A, Morgan IM. Werner Syndrome protein (WRN) regulates cell proliferation and the human papillomavirus 16 life cycle during epithelial differentiation. mSphere (2020) 5(5). doi: 10.1128/mSphere.00858-20
48. Pirouzfar M, Amiri F, Dianatpour M, Takhshid MA. CRISPR/Cas9-mediated knockout of MLL5 enhances apoptotic effect of cisplatin in HeLa cells in vitro. Excli J (2020) 19:170–82. doi: 10.17179/excli2019-1957
49. Ling K, Yang L, Yang N, Chen M, Wang Y, Liang S, et al. Gene targeting of HPV18 E6 and E7 synchronously by nonviral transfection of CRISPR/Cas9 system in cervical cancer. Hum Gene Ther (2020) 31(5-6):297–308. doi: 10.1089/hum.2019.246
50. Hatterschide J, Brantly AC, Grace M, Munger K. White EA. a conserved amino acid in the c terminus of human papillomavirus E7 mediates binding to PTPN14 and repression of epithelial differentiation. J Virol (2020) 94(17). doi: 10.1128/jvi.01024-20
51. Bortnik V, Wu M, Julcher B, Salinas A, Nikolic I, Simpson KJ, et al. Loss of HPV type 16 E7 restores cGAS-STING responses in human papilloma virus-positive oropharyngeal squamous cell carcinomas cells. J Microbiol Immunol Infect (2021) 54(4):733–9. doi: 10.1016/j.jmii.2020.07.010
52. Xiong J, Tan S, Yu L, Shen H, Qu S, Zhang C, et al. E7-targeted nanotherapeutics for key HPV afflicted cervical lesions by employing CRISPR/Cas9 and poly (Beta-amino ester). Int J Nanomed (2021) 16:7609–22. doi: 10.2147/ijn.S335277
53. Gao C, Wu P, Yu L, Liu L, Liu H, Tan X, et al. The application of CRISPR/Cas9 system in cervical carcinogenesis. Cancer Gene Ther (2021) 29(5):625–6 doi: 10.1038/s41417-021-00366-w
54. Jubair L, Lam AK, Fallaha S, McMillan NAJ. CRISPR/Cas9-loaded stealth liposomes effectively cleared established HPV16-driven tumours in syngeneic mice. PloS One (2021) 16(1):e0223288. doi: 10.1371/journal.pone.0223288
55. Yu L, Wang X, Zhu D, Ding W, Wang L, Zhang C, et al. Disruption of human papillomavirus 16 E6 gene by clustered regularly interspaced short palindromic repeat/Cas system in human cervical cancer cells. Onco Targets Ther (2015) 8:37–44. doi: 10.2147/ott.S64092
56. Hsu DS, Kornepati AV, Glover W, Kennedy EM, Cullen BR. Targeting HPV16 DNA using CRISPR/Cas inhibits anal cancer growth in vivo. Future Virol (2018) 13(7):475–82. doi: 10.2217/fvl-2018-0010
57. Ehrke-Schulz E, Heinemann S, Schulte L, Schiwon M, Ehrhardt A. Adenoviral vectors armed with PAPILLOMAVIRUs oncogene specific CRISPR/Cas9 kill human-Papillomavirus-Induced cervical cancer cells. Cancers (Basel) (2020) 12(7):1934. doi: 10.3390/cancers12071934
58. Noroozi Z, Shamsara M, Valipour E, Esfandyari S, Ehghaghi A, Monfaredan A, et al. Antiproliferative effects of AAV-delivered CRISPR/Cas9-based degradation of the HPV18-E6 gene in HeLa cells. Sci Rep (2022) 12(1):2224. doi: 10.1038/s41598-022-06025-w
59. Hyakusoku H, Sano D, Takahashi H, Hatano T, Isono Y, Shimada S, et al. JunB promotes cell invasion, migration and distant metastasis of head and neck squamous cell carcinoma. J Exp Clin Cancer Res (2016) 35:6. doi: 10.1186/s13046-016-0284-4
60. Leung TH, Tang HW, Siu MK, Chan DW, Chan KK, Cheung AN, et al. Human papillomavirus E6 protein enriches the CD55(+) population in cervical cancer cells, promoting radioresistance and cancer aggressiveness. J Pathol (2018) 244(2):151–63. doi: 10.1002/path.4991
61. Leung TH, Tang HW, Siu MK, Chan DW, Chan KK, Cheung AN, et al. CD71(+) population enriched by HPV-E6 protein promotes cancer aggressiveness and radioresistance in cervical cancer cells. Mol Cancer Res (2019) 17(9):1867–80. doi: 10.1158/1541-7786.Mcr-19-0068
62. Broutian TR, Jiang B, Li J, Akagi K, Gui S, Zhou Z, et al. Human papillomavirus insertions identify the PIM family of serine/threonine kinases as targetable driver genes in head and neck squamous cell carcinoma. Cancer Lett (2020) 476:23–33. doi: 10.1016/j.canlet.2020.01.012
63. Abboodi F, Buckhaults P, Altomare D, Liu C, Hosseinipour M, Banister CE, et al. HPV-inactive cell populations arise from HPV16-transformed human keratinocytes after p53 knockout. Virology (2021) 554:9–16. doi: 10.1016/j.virol.2020.12.005
64. Zhou L, Mudianto T, Ma X, Riley R, Uppaluri R. Targeting EZH2 enhances antigen presentation, antitumor immunity, and circumvents anti-PD-1 resistance in head and neck cancer. Clin Cancer Res (2020) 26(1):290–300. doi: 10.1158/1078-0432.Ccr-19-1351
65. Dok R, Bamps M, Glorieux M, Zhao P, Sablina A, Nuyts S. Radiosensitization approaches for HPV-positive and HPV-negative head and neck squamous carcinomas. Int J Cancer (2020) 146(4):1075–85. doi: 10.1002/ijc.32558
66. Lao YH, Li M, Gao MA, Shao D, Chi CW, Huang D, et al. HPV oncogene manipulation using nonvirally delivered CRISPR/Cas9 or natronobacterium gregoryi argonaute. Adv Sci (Weinh) (2018) 5(7):1700540. doi: 10.1002/advs.201700540
67. Gao X, Jin Z, Tan X, Zhang C, Zou C, Zhang W, et al. Hyperbranched poly(β-amino ester) based polyplex nanopaticles for delivery of CRISPR/Cas9 system and treatment of HPV infection associated cervical cancer. J Control Release (2020) 321:654–68. doi: 10.1016/j.jconrel.2020.02.045
68. Ehrke-Schulz E, Schiwon M, Leitner T, Dávid S, Bergmann T, Liu J, et al. CRISPR/Cas9 delivery with one single adenoviral vector devoid of all viral genes. Sci Rep (2017) 7(1):17113. doi: 10.1038/s41598-017-17180-w
69. Cheng YX, Chen GT, Yang X, Wang YQ, Hong L. Effects of HPV pseudotype virus in cutting E6 gene selectively in SiHa cells. Curr Med Sci (2018) 38(2):212–21. doi: 10.1007/s11596-018-1868-3
70. Chen R, Huang H, Liu H, Xi J, Ning J, Zeng W, et al. Friend or foe? evidence indicates endogenous exosomes can deliver functional gRNA and Cas9 protein. Small (2019) 15(38):e1902686. doi: 10.1002/smll.201902686
71. Zhang B, Wang Q, Xu X, Xia Q, Long F, Li W, et al. Detection of target DNA with a novel Cas9/gRNAs-associated reverse PCR (CARP) technique. Anal Bioanal Chem (2018) 410(12):2889–900. doi: 10.1007/s00216-018-0873-5
72. Wang Q, Zhang B, Xu X, Long F, Wang J. CRISPR-typing PCR (ctPCR), a new Cas9-based DNA detection method. Sci Rep (2018) 8(1):14126. doi: 10.1038/s41598-018-32329-x
73. Gao J, Wu L, Yang D, Gong W, Wang J. A one-pot CRISPR/Cas9-typing PCR for DNA detection and genotyping. J Mol Diagn (2021) 23(1):46–60. doi: 10.1016/j.jmoldx.2020.10.004
74. Xu X, Luo T, Gao J, Lin N, Li W, Xia X, et al. CRISPR-assisted DNA detection: A novel dCas9-based DNA detection technique. Crispr J (2020) 3(6):487–502. doi: 10.1089/crispr.2020.0041
75. Pal A, Kundu R. Human papillomavirus E6 and E7: The cervical cancer hallmarks and targets for therapy. Front Microbiol (2019) 10:3116. doi: 10.3389/fmicb.2019.03116
76. Beerheide W, Bernard HU, Tan YJ, Ganesan A, Rice WG, Ting AE. Potential drugs against cervical cancer: zinc-ejecting inhibitors of the human papillomavirus type 16 E6 oncoprotein. J Natl Cancer Inst (1999) 91(14):1211–20. doi: 10.1093/jnci/91.14.1211
77. Beerheide W, Sim MM, Tan YJ, Bernard HU, Ting AE. Inactivation of the human papillomavirus-16 E6 oncoprotein by organic disulfides. Bioorg Med Chem (2000) 8(11):2549–60. doi: 10.1016/s0968-0896(00)00193-0
78. Butz K, Denk C, Ullmann A, Scheffner M, Hoppe-Seyler F. Induction of apoptosis in human papillomaviruspositive cancer cells by peptide aptamers targeting the viral E6 oncoprotein. Proc Natl Acad Sci U.S.A. (2000) 97(12):6693–7. doi: 10.1073/pnas.110538897
79. Guo CP, Liu KW, Luo HB, Chen HB, Zheng Y, Sun SN, et al. Potent anti-tumor effect generated by a novel human papillomavirus (HPV) antagonist peptide reactivating the pRb/E2F pathway. PloS One (2011) 6(3):e17734. doi: 10.1371/journal.pone.0017734
80. Ajiro M, Zheng ZM. E6^E7, a novel splice isoform protein of human papillomavirus 16, stabilizes viral E6 and E7 oncoproteins via HSP90 and GRP78. mBio (2015) 6(1):e02068–14. doi: 10.1128/mBio.02068-14
81. Teng M, Yao Y, Nair V, Luo J. Latest advances of virology research using CRISPR/Cas9-based gene-editing technology and its application to vaccine development. Viruses (2021) 13(5):779. doi: 10.3390/v13050779
82. Pérez-Benavente B, García JL, Rodríguez MS, Pineda-Lucena A, Piechaczyk M, Font de Mora J, et al. GSK3-SCF(FBXW7) targets JunB for degradation in G2 to preserve chromatid cohesion before anaphase. Oncogene (2013) 32(17):2189–99. doi: 10.1038/onc.2012.235
83. Wu W, Yu A, Chen K, Lu P, Yang J, Liu K, et al. The oncogene PIM1 contributes to cellular senescence by phosphorylating staphylococcal nuclease domain-containing protein 1 (SND1). Med Sci Monit (2019) 25:8651–9. doi: 10.12659/msm.917867
84. Xia AL, He QF, Wang JC, Zhu J, Sha YQ, Sun B, et al. Applications and advances of CRISPR-Cas9 in cancer immunotherapy. J Med Genet (2019) 56(1):4–9. doi: 10.1136/jmedgenet-2018-105422
85. Ishida Y. PD-1: Its discovery, involvement in cancer immunotherapy, and beyond. Cells (2020) 9(6):1376. doi: 10.3390/cells9061376
86. Yang W, Song Y, Lu YL, Sun JZ, Wang HW. Increased expression of programmed death (PD)-1 and its ligand PD-L1 correlates with impaired cell-mediated immunity in high-risk human papillomavirus-related cervical intraepithelial neoplasia. Immunology (2013) 139(4):513–22. doi: 10.1111/imm.12101
87. Fang J, Zhang M, Li Q. Enhancer of zeste homolog 2 expression is associated with tumor cell proliferation and invasion in cervical cancer. Am J Med Sci (2011) 342(3):198–204. doi: 10.1097/MAJ.0b013e31821335a9
88. Zhang Q, Dong P, Liu X, Sakuragi N, Guo SW. Enhancer of zeste homolog 2 (EZH2) induces epithelial-mesenchymal transition in endometriosis. Sci Rep (2017) 7(1):6804. doi: 10.1038/s41598-017-06920-7
89. Bachtiary B, Obermair A, Dreier B, Birner P, Breitenecker G, Knocke TH, et al. Impact of multiple HPV infection on response to treatment and survival in patients receiving radical radiotherapy for cervical cancer. Int J Cancer (2002) 102(3):237–43. doi: 10.1002/ijc.10708
90. Tian R, Liu J, Fan W, Li R, Cui Z, Jin Z, et al. Gene knock-out chain reaction enables high disruption efficiency of HPV18 E6/E7 genes in cervical cancer cells. Mol Ther Oncol (2022) 24:171–9. doi: 10.1016/j.omto.2021.12.011
91. Ehrhardt A, Kay MA. A new adenoviral helper-dependent vector results in long-term therapeutic levels of human coagulation factor IX at low doses in vivo. Blood (2002) 99(11):3923–30. doi: 10.1182/blood.v99.11.3923
92. Zheng R, Fang X, Chen X, Huang Y, Xu G, He L, et al. Knockdown of lactate dehydrogenase by adeno-associated virus-delivered CRISPR/Cas9 system alleviates primary hyperoxaluria type 1. Clin Transl Med (2020) 10(8):e261. doi: 10.1002/ctm2.261
93. Mingozzi F, High KA. Therapeutic in vivo gene transfer for genetic disease using AAV: progress and challenges. Nat Rev Genet (2011) 12(5):341–55. doi: 10.1038/nrg2988
94. Lin Y, Wu J, Gu W, Huang Y, Tong Z, Huang L, et al. Exosome-liposome hybrid nanoparticles deliver CRISPR/Cas9 system in MSCs. Adv Sci (Weinh) (2018) 5(4):1700611. doi: 10.1002/advs.201700611
95. Gonçalves BC, Lopes Barbosa MG, Silva Olak AP, Belebecha Terezo N, Nishi L, Watanabe MA, et al. Antiviral therapies: advances and perspectives. Fundam Clin Pharmacol (2021) 35(2):305–20. doi: 10.1111/fcp.12609
Keywords: human papillomavirus, clustered regularly interspaced short palindromic repeat/CRISPR-associated nuclease 9, gene editing, cancer treatment, tumor microenvironment
Citation: Wei Y, Zhao Z and Ma X (2022) Description of CRISPR-Cas9 development and its prospects in human papillomavirus-driven cancer treatment. Front. Immunol. 13:1037124. doi: 10.3389/fimmu.2022.1037124
Received: 05 September 2022; Accepted: 17 October 2022;
Published: 21 November 2022.
Edited by:
Pengpeng Liu, University of Massachusetts Medical School, United StatesReviewed by:
Haridha Shivram, Genentech, Inc., United StatesCopyright © 2022 Wei, Zhao and Ma. This is an open-access article distributed under the terms of the Creative Commons Attribution License (CC BY). The use, distribution or reproduction in other forums is permitted, provided the original author(s) and the copyright owner(s) are credited and that the original publication in this journal is cited, in accordance with accepted academic practice. No use, distribution or reproduction is permitted which does not comply with these terms.
*Correspondence: Xuelei Ma, ZHJtYXh1ZWxlaUBnbWFpbC5jb20=
†These authors have contributed equally to this work
Disclaimer: All claims expressed in this article are solely those of the authors and do not necessarily represent those of their affiliated organizations, or those of the publisher, the editors and the reviewers. Any product that may be evaluated in this article or claim that may be made by its manufacturer is not guaranteed or endorsed by the publisher.
Research integrity at Frontiers
Learn more about the work of our research integrity team to safeguard the quality of each article we publish.