- 1Dept of Neurology, Division of Movement Disorders, Beth Israel Deaconess Medical Center, Harvard Medical School, Boston, MA, United States
- 2Department of Microbiology and Immunology, College of Medicine and Health Sciences, United Arab Emirates University, Al Ain, United Arab Emirates
- 3Zayed Center for Health Sciences, United Arab Emirates University, Al Ain, United Arab Emirates
Multiple sclerosis (MS) is a chronic disease of the central nervous system (CNS), marked primarily by demyelination, inflammation, and neurodegeneration. While the prevalence and incidence rates of MS are on the rise, the etiology of the disease remains enigmatic. Nevertheless, it is widely acknowledged that MS develops in persons who are both genetically predisposed and exposed to a certain set of environmental factors. One of the most plausible environmental culprits is Epstein-Barr virus (EBV), a common herpesvirus asymptomatically carried by more than 90% of the adult population. How EBV induces MS pathogenesis remains unknown. A comprehensive understanding of the biology of EBV infection and how it contributes to dysfunction of the immune system and CNS, requires an appreciation of the viral dynamics within the host. Here, we aim to outline the different animal models, including nonhuman primates (NHP), rodents, and rabbits, that have been used to elucidate the link between EBV and MS. This review particularly focuses on how the disruption in virus-immune interaction plays a role in viral pathogenesis and promotes neuroinflammation. We also summarize the effects of virus titers, age of animals, and route of inoculation on the neuroinvasiveness and neuropathogenic potential of the virus. Reviewing the rich data generated from these animal models could provide directions for future studies aimed to understand the mechanism(s) by which EBV induces MS pathology and insights for the development of prophylactic and therapeutic interventions that could ameliorate the disease.
Introduction
Multiple sclerosis (MS) is a disease that causes demyelination, or damage to myelin sheaths in the brain, spinal cord and optic nerve (1), leading to disability, particularly among young adults (2). Neuroinflammation is believed to be fundamental to MS pathology (3, 4). T and B lymphocytes infiltrate the central nervous system (CNS) during the early stages of MS, and to a much lesser degree during the late stages (5). Yet, our understanding of what causes pathology in MS is relatively limited.
MS development is believed to be greatly influenced by both genetics and environmental factors. The increased susceptibility to MS in Caucasians compared to other ethnic backgrounds reflects the impact of genes on MS risk (6–8). Among the genes discovered to influence MS risk, HLA class II allele HLADRB1*1501 has been shown to have the greatest odds ratio in conferring susceptibility to MS (9–11). However, the concordance rate for MS is only as high as 25% in genetically identical individuals (12, 13). This implies that other factors are also involved in the development of MS (14). Indeed, immigration studies revealed increased MS risk in immigrants from low MS-risk regions to regions with high MS incidence (15–17). This however, is also impacted by the age at which immigration takes place. Moreover, various environmental factors including infectious agents are thought to modulate susceptibility to MS (18–21).
Substantial evidence from seroepidemiological and pathological studies support the role of Epstein-Barr virus (EBV) infection in MS pathogenesis (22–26). This herpesvirus is one of the most successful pathogens persisting silently in as many as 95% of the human population. It targets circulating B lymphocytes to establish a life-long reservoir in the face of a competent immune surveillance (27, 28). Maintaining a balanced EBV-immune interaction is fundamental to the well-being of the human host. Consequently, several EBV-related pathologies arise in individuals with defects in the immune system (29).
The fact that humans are the only natural host for EBV has made the understanding of how EBV contributes to the development/progression of MS exceptionally challenging. Nevertheless, efforts are continuously made to utilize several animals, including rodents, nonhuman primates (NHP), and rabbits to understand the role of the virus in MS pathogenesis (Table 1). Studies have examined the effect of virus titers, age of animals during primary infection, route of inoculation, the direct use of purified virus versus adoptive transfer of virus infected cells, and the induction of experimental autoimmune encephalitis (EAE) in infected versus naïve hosts on the neuroinvasiveness and neuropathogenic potential of the virus. The rich data generated from these animal models has uncovered various mechanisms that viruses, such as EBV potentially use to promote autoimmunity and/or demyelination in the CNS. This review outlines some of the lessons we have learnt from the studies examining the link between EBV and MS using different in vivo models.
EBV and MS in murine models
Data from Murine γ-herpesvirus 68 infection models
Murine γ-herpesvirus 68 (MHV68, or γHV68) belongs to the gammaherpesvirus subfamily of the Herpesviridae, to which both EBV and human Kaposi’s sarcoma-associated herpesvirus (KSHV, or HHV-8) belong (38). MHV68 is more comparable to KSHV than to EBV in terms of the genomic structure, as both MHV68 and KSHV are gamma-2 viruses while EBV is a gamma-1 virus (38). Nevertheless, MHV68 and EBV share several key biological characteristics during infection of their natural hosts (58) (Table 1). For instance, MHV68 infection in mice results in life-long latency in B cells and macrophages, chiefly in the spleen and lungs, with intermittent lytic infection (32, 59, 60). During chronic latent infection, various immune-related genes are differentially altered in several organs including the brain (61). Furthermore, primary MHV68 infection is associated with a short-lived surge in the frequency of activated CD8+T cells and splenomegaly in what resembles human infectious mononucleosis (IM) caused by primary EBV infection (33, 62, 63). As a result, several studies utilized MHV68 as a surrogate virus to understand the neuropathogenic potential of EBV, and thus the role of the virus in the pathogenesis of MS.
To determine whether primary peripheral MHV68 infection could be neuroinvasive, Terry and colleagues inoculated various mice strains with MHV68 intranasally (30). Although asymptomatic viral infection was established in the periphery, there was no evidence of viral infection in the CNS. However, peripheral infection was found to cause CNS infection in mice that were deficient in interferon type I receptor (IFNAR), which suggests the protective function of IFNAR against the spread of MHV68 from the periphery to the CNS (30).
The study also examined the consequences of a different route of viral inoculation. When MHV68 was introduced directly into the brain, viral infection was detected in the meninges, ependymal cells, oligodendrocytes, cerebellar Bergmann cells, and pyramidal neuron of the hippocampus. Infection of the brain was also accompanied with infiltration of inflammatory cells into the infected areas and damage to white matter tracts. Viral infection and neuroinflammation were most pronounced towards the end of week 1 post infection (30). These animals exhibited signs of ailment such as lethargy and body atrophy. Nevertheless, inhibiting MHV68 replication in these animals led to silent viral persistence in the CNS. Similarly, introducing non-productively MHV68-infected cells into the brain produced long-term viral persistence in the CNS (30). The ability of MHV68 to establish persistent latent infection in the CNS was also demonstrated by Kang and colleagues (31) following intracerebroventricular inoculation of 9-10wk old BALB/c mice with recombinant MHV68-M3/FL. Viral persistence in the CNS resulted in viral dissemination from the brain to peripheral organs including the spleen and lungs, with the spleen being the main peripheral reservoir for latent MHV68. Immunosuppression with cyclosporin A (CsA) of latently infected mice, after the virus levels had become undetectable in the CNS and periphery, caused virus reactivation, and increased viral load in the brain and spleen (31). While these observations imply that latent infection promote viral persistence in the CNS, the implications of latent virus in the brain of an immunocompetent host are currently unclear.
Additionally, the age of the host can be a crucial factor in determining the outcome of MHV68 infection in the CNS. After intracerebral inoculation, older mice are more likely to survive MHV68 infection than younger mice. Younger mice exhibit a more severe MHV68 infection in the CNS, whereas older mice exhibit a decline in MHV68 load to undetectable levels by the second week of infection (30, 34). MHV68 infection in the CNS has been found to involve increased expression of the proinflammatory cytokines, tumor necrosis factor alpha (TNFα), interleukin 1 beta (IL1β), and IL6 in younger mice (34).
Furthermore, the initial viral load introduced into the periphery influences the neuropathogenic potential of MHV68. In wildtype mice, intranasal inoculation using 2*104 plaque-forming units (pfu) of MHV68 was found to be non-neuroinvasive (30), but inoculating animals with 2*105 pfu of MHV68 intranasally produced detectable and presumably productive infection in the CNS (35). The infection was associated with infiltration of CD45+ cells and CD3+ cells into different brain areas resulting in meningitis, cerebellitis and perivascular encephalitis. Severe neuroinflammation was more likely to occur in regions of the brain that had increased expression of viral proteins. Over a third of these animals had ataxia, severe dystrophy, or died early (35).
The fact that the risk of MS increases in EBV-infected individuals has raised questions about how a pre-existing infection promotes autoimmune demyelination. Studying EAE in murine models of MHV68 infection revealed that latent virus could be the culprit to exacerbated EAE pathology (36, 64). A study examined myelin oligodendrocyte glycoprotein (MOG)-induced EAE course in C57BL/6 mice 5 weeks post primary infection with MHV68 (i.e., during latent infection) and compared it to EAE course in non-infected animals and animals previously infected with either lymphocytic choriomeningitis virus (LCMV) or murine cytomegalovirus (MCMV) (36). MHV68-infected animals experienced EAE more rapidly, with more severe morbidity and higher risk of mortality than non-infected, LCMV- or MCMV-infected animals. The aggravated disease in MHV68-infected animals appeared to occur in the absence of any signs of productive infection in the CNS (36). This was further corroborated by the observation that mice infected with latency-deficient MHV68 (MHV68 AC-RTA) had an EAE course comparable to that in noninfected controls, indicating that latent infection - rather than acute infection- plays a role in disease progression (64). Indeed, the neuropathological potential of latent infection was also demonstrated by immunizing mice with latent Epstein-Barr virus nuclear antigen 1 amino acid region 411-426 (EBNA-1411-426) (65). EBNA-1411-426 immunized mice developed neurological signs reminiscent of EAE, and MRI-confirmed cortical lesions (65). This region of EBNA-1 was also found to trigger high antibody response in individuals with relapsing-remitting (RRMS) and secondary progressive MS (SPMS), and these antibodies cross-reacted with myelin-basic protein amino acid region 205-224 (MBP205-224). Similarly, mice immunized with EBNA-1386–405 in combination with proteolipid protein amino acid region 139-151 (PLP139–151) exhibited pronounced EAE course and T helper1 (Th1) phenotype-driven inflammation (66). Notably, immunization with EBNA-1386–405 triggered an antibody response against the CNS-derived antigen, glial cell adhesion molecule (GlialCAM). In MS cases, intrathecal antibody response to EBNA-1386–405 was found to cross-react with GlialCAM, most probably via a molecular mimicry mechanism (66).
EAE pathology in noninfected animals was characterized by infiltration of interferon gamma (IFNγ- and IL17-producing CD4+T cells into the spinal cord, whereas MHV68-infected mice had elevated expression of IFNγ and increased infiltration of T bet+ CD4 T cells, granzyme B-secreting CD8+T cells and F4/80+ macrophages/microglia in the brain and spinal cord. Some of the CNS-infiltrating CD8+T cells were MHV68-specific. In contrast to noninfected controls, MHV68-infected animals showed demyelination in the cerebellum and corpus callosum (36). Additionally, MHV68-infected mice displayed noticeably greater levels of the costimulatory marker CD40, which appears to promote enhanced expression of the Th1 signature cytokine, IFNγ, and reduction of the frequency of regulatory T cells (Tregs) (64). These results suggest that latent MHV68 infection skews the immune response during EAE toward a Th1 response, rather than a Th17 response, via CD40-mediated immune modulation.
How MHV68-infected B cells contribute to EAE pathology was also studied. Animals that received splenic CD19+IgD- B cells derived from MHV68-infected animals, before EAE induction, developed severe EAE pathology, including increased production of IFNγ and infiltration of CD8+T cells in the brain and spinal cord (37). Antibody-mediated depletion of B cells prior to EAE induction alleviated IFNγ production in the CNS, but was ineffective in eliminating detectable virus in the periphery. On the other hand, B cell depletion prior to primary MHV68 infection led to EAE disease similar to that in control animals that received noninfected B cells (37). This implies that B cells are fundamental for MHV68 to establish latency, which in turn contributes to the exacerbation of EAE disease. EAE was also observed to be aggravated in mice that received EBV-immortalized B lymphoblastoid cell lines (BLCLs) derived from patients with SPMS, prior to MOG-EAE induction (67). Furthermore, mice that were given these BLCLs and recovered from the initial EAE course, experienced disease relapses. These animals also exhibited marked changes in the makeup of their gut microbiota (67). This may shed insight into potential mechanisms, by which EBV-infected and transformed B cells could aggravate autoimmunity by interfering in otherwise balanced gut-brain axis.
Data from humanized mice
Humanized mice have also been utilized to examine EBV involvement in MS (Table 1). Humanized mice, such as NOD SCID IL2Rγ- deficient (NSG) mice, are immunodeficient animals that can be successfully engrafted with human hematopoietic stem cells to rebuild functional parts of the human immune system. Studies in humanized mice can help delineate how strictly human pathogens such as EBV, behave in their natural host. EBV infection in humanized mice can result in acute IM-like disease, latent infection, or lymphoproliferative disorders (LPDs), depending on the dose of the inoculum (39, 68, 69).
A recent study explored the association between EBV infection and HLA-DR15 in NSG mice (41) in light of the observation that individuals with a history of IM and carrying the HLA-DRB1*15:01 allele are more likely to develop MS (70). Mice reconstituted with human HLA-DR15 hematopoietic progenitor cells displayed significantly greater numbers of activated CD8+T cells in the periphery (blood and spleen), at 4-6 weeks post EBV infection, compared to controls. EBV-infected mice reconstituted with either HLA-DR15-negative or HLA-DR4-positive immune compartments served as controls. EBV load was also greater in EBV-infected HLA-DR15-carrying animals than in controls. EBV load correlated positively with frequencies and total numbers of activated CD8+T cells in the periphery. Thus, the genetic risk associated with HLA-DR15 may mediate impaired control over EBV infection, and likely contributes to the pathogenesis of IM (41). The study also demonstrated that CD4+T cells derived from HLA-DR15-carrying mice can recognize MBP epitopes and allogeneic targets. HLA-DR15-restricted cells were found to respond more robustly and non-specifically to HLA-mismatched targets than to their HLA-matched targets. This cross-reactivity mechanism, in conjunction with the lack of specificity in the T cell response, may explain how EBV and the HLA-DR15 allele synergistically enhance MS risk (41).
Another study used NSG mice to assess the impact of EBV on EAE course. NSG mice were transplanted with PBMCs isolated from either patients with relapsing-remitting MS (RRMS), EBV-seropositive healthy controls, or EBV- seronegative healthy controls (42). Upon induction of EAE, all mice exhibited EAE signs. Nonetheless, mice engrafted with RRMS PBMCs showed a more pronounced disease course than mice engrafted with non-MS PBMCs. Furthermore, mice engrafted with PBMCs from EBV seropositive donors had more rapid onset of EAE than animals reconstituted with PBMCs from EBV seronegative donors (42). Hence, this model emphasizes the fundamental role of EBV in disease progression.
Studies on NSG mice have also helped us understand factors that promote migration of EBV-infected cells from the periphery to the brain. NSG mice implanted intravenously, intracardially or subcutaneously with the MUN14 cell line derived from an EBV+ Burkitt’s lymphoma, showed increased trafficking of EBV-infected cells to the brain (71). As a result, mice developed neurological signs including gait deficits, tremor and seizures. Epigenetic changes in EBV-infected B cells were found to enhance the neuroinvasiveness of infected cells. Increased expression of viral protein EBNA-1, and cellular phosphoprotein-1/osteopontin were suspected to be involved in driving the epigenetic changes leading to the neuroinvasive phenotype (71).
Nonhuman primate model for EBV infection of the brain
Lymphocryptovirus-infected B cells
Nonhuman primates (NHPs) have also served as an appealing model to better understand how EBV modulates the risk of MS (Table 1). A study on adult rhesus monkeys (Macaca mulatta) examined whether EBV-infected and transformed B cells can (1) present myelin antigen to, and activate myelin-specific T cells, and (2) promote inflammation in the brain (47). Herpesvirus papio (HVP), a baboon-tropic virus that shares biological and genetic similarities with EBV, was used to infect and transform B cells isolated from rhesus monkeys and subsequently generate BLCLs. These cells were then loaded with either MOG34-56, citrullinated MOG34-56, or CMV capsid antigen-derived peptides, and infused back into the monkeys. Animals exhibited only weight loss with no signs of neurological deficits. However, they mounted an immune response made up primarily of CD8+T cells, CD8+CD56+T cells, and CD3-CD56+NK cells against MOG and viral peptides (47). Interestingly, myelin-specific T cells reacted against MOG only when its peptides were presented by BLCLs. Moreover, animals, particularly those infused with auto-BLCLs pulsed with citrullinated MOG34-56 exhibited meningeal inflammation and infiltration of T cells, B cells, and macrophages into the brain, with no major signs of myelin destruction (47). These observations point to the pathogenic role of EBV-infected and transformed B cells in neuroinflammation by serving as efficient APCs and promoting recognition of and response against myelin antigens by myelin-specific T cells. This pathogenic effect of EBV-infected B cells was also demonstrated in another NHP, the marmoset (Callithrix jacchus) (72). Jagessar and coathors proposed an explanation for this. EBV infection of B cells enables productive processing of myelin peptides for presentation to autoreactive T cells (73). B cells in absence of the influence of EBV infection would instead abort antigen processing and opt for the degradation of self-antigen (MOG34-56) which ultimately prevent the presentation of MOG34-56 to their autoreactive T cells (74).
Japanese macaque rhadinovirus and spontaneous onset of encephalomyelitis
NHPs have also emerged as a natural model for herpesvirus-associated autoimmune demyelinating diseases. Japanese macaques (Macaca fuscata) housed at the Oregon National Primate Research Center have been found to naturally develop Japanese macaque encephalomyelitis (JME), an MS-like disease, at a rate of 1-3% annually since 1986 (49). In contrast to MS where females are at higher risk than males, JME is believed to develop in both sexes at comparable rates, with a reported median age at disease onset of ~4.3 years. Furthermore, the majority of animals experience acute neurological signs that are almost immediately followed by rapid progression that necessitates euthanasia within a median time of 6 days. Neurological signs commonly include ataxia, paralysis or paresis of at least one limb, and less commonly ocular paresis, body tremors, and head tilt (49). Antemortem MRI examination of the CNS, using post-gadolinium contrast T1-weighted images and T2-weighted axial images of affected animals showed several conspicuous lesions in the white matter of both the brain and spinal cord. Pathologically, these lesions were restricted to the white matter and characterized by demyelination, disrupted axons and marked influx of immune cells in what resembles chronic active MS plaques (49). Unlike MS, pathological changes in the meninges and cortical grey matter are not characteristics of JME (49). JME white matter lesions are characterized by the infiltration of CD163+ cells, some of which are MBP-reactive, the aggregation of CD20+B cells in periventricular areas, and the presence of CD4+T cells, CD8+T cells, and Th17 cells at variable levels (51). Similar to MS patients, JME-affected animals produce intrathecal oligoclonal bands (51).
Interestingly, a newly characterized gamma-2 herpesvirus was recovered from these lesions (48, 49). The virus termed Japanese macaque rhadinovirus (JMRV) had 89.5% and 47.9% sequence homology with rhesus macaque rhadinovirus and KSHV, respectively (49). However, JMRV does not appear to share considerable genetic homology with EBV (48). Subsequent studies described the complete sequence of the viral genome, which revealed both conserved and unique open-reading frames (ORFs), and viral-encoded miRNAs that may be implicated in disease pathogenesis (48, 75).
In spite of the presence of the virus in JME lesions, the intrathecal humoral response does not appear to be directed against the virus (50). T lymphocytes specific for MBP, MOG, and PLP infiltrate the brain and circulate in peripheral blood of infected animals (50).
Rabbit model of EBV infection
Rabbits are susceptible to EBV infection
A number of reports have shown that New Zealand White (NZW) rabbits are susceptible to EBV, and the infection mimics that observed in humans (52–55, 76) (Table 1). During primary EBV infection, rabbits show no symptoms, but can experience temporarily enlarged lymph nodes and spleens, short-term lymphocytosis, elevated titers of anti-EBV viral capsid antigen antibodies, and detectable levels of EBV genome within the first 2 weeks of infection (57). However, EBV levels vary between different rabbits, and fluctuate overtime in a given animal (57). This suggests that different infection dynamics are influenced by differences in rabbit immune responses to EBV, similar to those observed in humans. Similar to humans, the rabbit immune system does not completely eradicate EBV infection, as EBV can persist latently in these animals (52). EBV infection in rabbits produces a robust humoral response, which helps reduce viral loads below detectable levels in the blood, whereas cyclosporin A (CsA)-mediated suppression of T cells can cause reactivation of the latent virus (53).
Peripheral EBV infection of rabbits can lead to CNS infection
A recent study examined if EBV, following primary infection, could enter the rabbit CNS and by what mechanism (56). The study revealed that, at day 14 of infection, the levels of viral DNA were highest in both peripheral and CNS compartments. Although viremia was pronounced at day 14 of infection, EBV load in the brain did not correlate with the levels of free virus in the circulation. There was, however, a positive correlation between the levels of cell-associated virus (i.e., infected cells) in the periphery and EBV levels in the brain. Thus, we posit that migrating infected cells, presumably B lymphocytes, could be the primary source of EBV infection in the brain (77). Indeed, some brain infiltrating B lymphocytes were infected with EBV, in addition to a few infected astrocytes and microglia.
EBV infection of the CNS induces the formation of inflammatory cellular aggregates
Primary EBV infection has been investigated in healthy rabbits and rabbits immunosuppressed with cyclosporine A (CsA). In line with previous data, EBV load increased significantly when the immune system was suppressed, and this raised the likelihood of virus-infected cells breaching CNS barriers. Despite the absence of overt signs of neurological impairments, peripheral EBV infection induced neuroinflammatory cellular aggregates in some animals within 2 weeks of infection (Figure 1). These animals displayed focal CNS cellular aggregates composed of densely clustered blood-derived macrophages surrounded by activated microglia and astrocytes, neutrophils, CD8+ T lymphocytes, dispersed EBI2+ cells and PCNA+, IgM+, and IgG+ B lymphocytes (Figure 1). Remarkably, the cell aggregates showed myelin damage in the center (Figure 1) (56). This may model the early stages of lesions that progress into MS smoldering active plaques (78). Of interest, some of the immunosuppressed noninfected controls also developed cell aggregates in the CNS, which was probably brought on by the reactivation of opportunistic intrinsic pathogens. This scenario mimics the development of CNS pathology seen in immunosuppressed individuals who experience progressive multifocal leukoencephalopathy as a result of JC virus reactivation (79). Nonetheless, the distinctions between neuroinflammation induced by EBV infection and that attributed to immunosuppression warrants further investigation. Similarly, further investigations are required to understand how the cellular aggregates seen in the rabbits correlates with ectopic lymphoid follicles reported in the brain of MS patients (4, 80, 81).
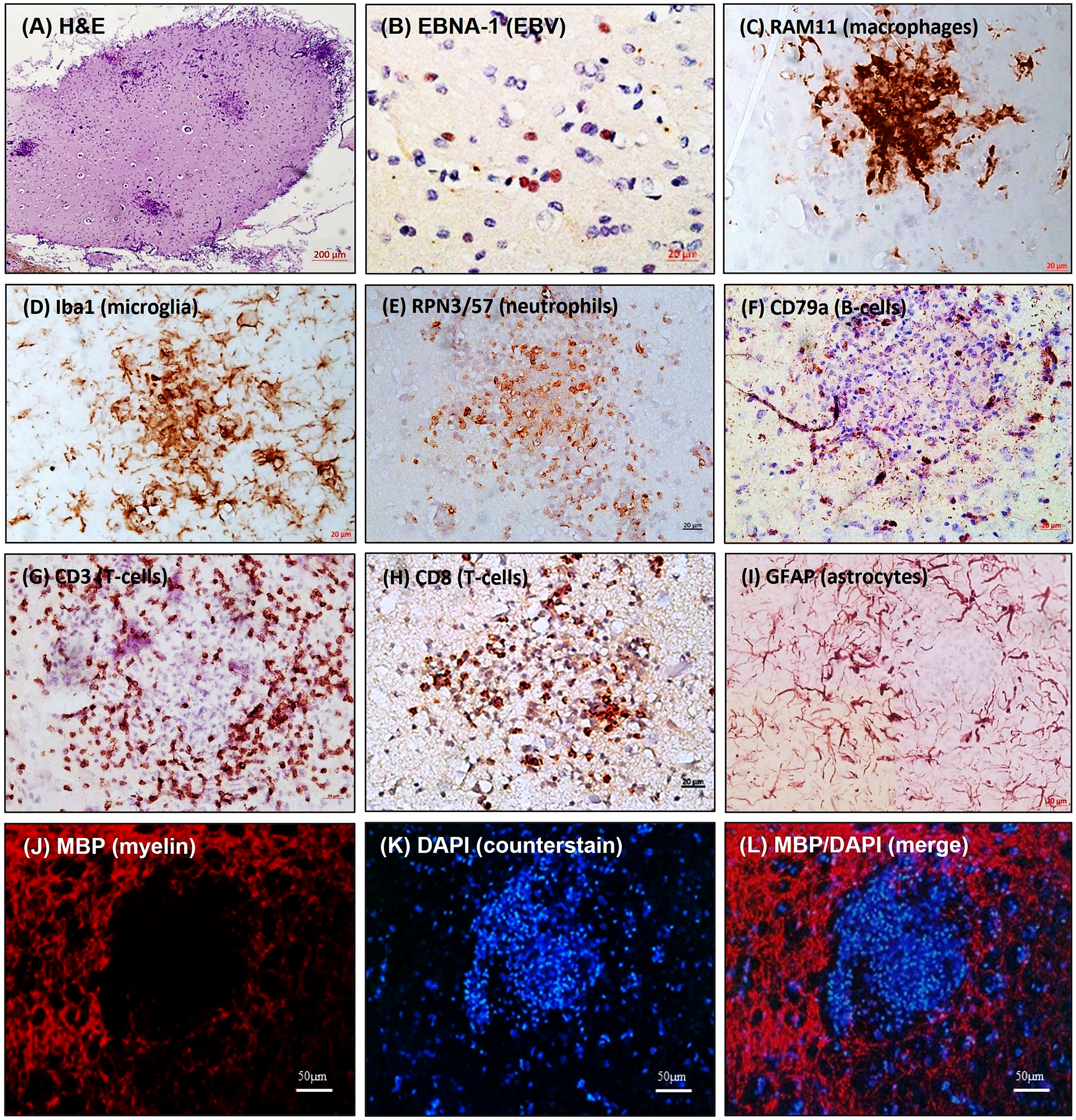
Figure 1 Inflammatory cellular aggregates in brain of EBV-infected rabbits. Brain sections were stained for: (A) H&E; (B) EBV latent nuclear protein, EBNA-1; (C) Blood-derived macrophage marker, RAM11; (D) Microglia marker, Iba1; (E) Neutrophil marker, RPN3/57; (F) B-cell marker, CD79a; (G) pan-T-cell marker, CD3; (H) Cytotoxic T-cell marker, CD8; (I) Astrocyte marker, GFAP; (J) Myelin marker, MBP; (K) Counter stain, DAPI; (L) MBP/DAPI merge showing areas of demyelination [adapted from (56)]. The figure shows non-serial sections from the same block. (Scale bar= 200µm in A, 20µm in B–I, and 50µm in J–L).
Expression of EBV latent transcripts correlates with proinflammatory cytokines
Importantly, EBV latent transcripts, most notably EBV-encoded RNAs (EBERs) correlate positively with the proinflammatory cytokines IL1β and IL6 in the brain and spleen (56). EBER2 expression in vitro is associated with high levels of IL-6 produced by infected B cells (82). This proinflammatory cytokine appears to be instrumental for the activation and expansion of EBV-infected B cells (82, 83). Additionally, proinflammatory IL-6 is markedly elevated in rabbits developing aggregates in the CNS. Thus, it is possible that this cytokine is a major player in the pathogenesis of EBV-associated neuroinflammation.
The mRNA levels of IL-1β, IFN-γ and TNF-α have also been observed to be significantly elevated at day 28 of infection in the spleen, brain and the spinal cord of rabbits. The expression of these cytokines in the CNS is associated with impairment of the brain-blood barrier (BBB) (84–88). CNS viral infections can trigger the production of these inflammatory cytokines, which compromises the integrity of BBB, for example by altering the expression of brain endothelia tight junction proteins (89–92). Thus, BBB breakdown could be both a pre-requisite and a consequence of CNS viral infections (90, 91, 93). One could argue that increased mRNA levels of these cytokines in EBV-infected rabbits may induce BBB leakage and recurrent influx of immune cells into the CNS. Whether EBV infection disrupts BBB integrity warrants further investigation.
Concluding remarks
In spite of substantial efforts over the last 6 decades in studying EBV, there are still many important gaps in our understanding of the details of viral pathogenesis and key aspects of the virus life cycle. There is a pressing need to understand how the virus behaves in the host and how that affects various organ systems. Studies on the link between EBV infection and the pathogenesis of CNS diseases, including MS is enormously expanding. There is now substantial and credible evidence that EBV is involved in the pathogenesis of MS (26, 66, 94, 95). Importantly, a number of studies have demonstrated the presence of EBV-infected cells directly in the white matter lesions in MS tissues (96–99). However, the mechanism by which EBV induces MS remains unclear. To characterize viral dynamics and virus interaction with the immune system, host genetic background and environmental cofactors, it is necessary to develop an in vivo model that captures both EBV biology and MS pathology. This review highlights the lessons we have learnt from several animal models used to understand the link between EBV and MS. While none of the models discussed here is a perfect representation of “EBV-induced MS”, utilizing them has provided insight into a number of potential factors, such as viral latent cycle and viral latent proteins, EBV-infected and transformed B cells, HLA-DR15, and epigenetic regulation of EBV-infected B cells, that may contribute to virus-induced CNS pathology. Exploring the various in vivo models for EBV would not only aid in the early identification of at-risk populations but also provide promising possibilities for therapeutic and prophylactic options for this incurable disease.
Author contributions
Conceptualization, writing the initial draft, and revision was performed by AH; conceptualization, writing part of the first draft and revision was performed by GK. All authors contributed to the article and approved the submitted version.
Funding
This work was funded by UAEU Zayed Centre-Based grants 31R259.
Acknowledgments
We would like to thank the staff in the Animal House, UAE University, College of Medicine and Health Sciences for their continuous support in helping and looking after the rabbits used in the original studies cited here.
Conflict of interest
The authors declare that the research was conducted in the absence of any commercial or financial relationships that could be construed as a potential conflict of interest.
Publisher’s note
All claims expressed in this article are solely those of the authors and do not necessarily represent those of their affiliated organizations, or those of the publisher, the editors and the reviewers. Any product that may be evaluated in this article, or claim that may be made by its manufacturer, is not guaranteed or endorsed by the publisher.
References
1. Reich DS, Lucchinetti CF, Calabresi PA. Multiple sclerosis. N Engl J Med (2018) 378:169–80. doi: 10.1056/NEJMra1401483
2. Dobson R, Giovannoni G. Multiple sclerosis - a review. Eur J Neurol (2019) 26:27–40. doi: 10.1111/ene.13819
3. Bevan RJ, Evans R, Griffiths L, Watkins LM, Rees MI, Magliozzi R, et al. Meningeal inflammation and cortical demyelination in acute multiple sclerosis. Ann Neurol (2018) 84:829–42. doi: 10.1002/ana.25365
4. Pikor NB, Prat A, Bar-Or A, Gommerman JL. Meningeal tertiary lymphoid tissues and multiple sclerosis: A gathering place for diverse types of immune cells during CNS autoimmunity. Front Immunol (2016) 6:657. doi: 10.3389/fimmu.2015.00657
5. Lassmann H. Multiple sclerosis pathology. Cold Spring Harb Perspect Med (2018) 8:a028936. doi: 10.1101/cshperspect.a028936
6. Brum DG, Luizon MR, Santos AC, Lana-Peixoto MA, Rocha CF, Brito ML, et al. European Ancestry predominates in neuromyelitis optica and multiple sclerosis patients from Brazil. PloS One (2013) 8:e58925. doi: 10.1371/journal.pone.0058925
7. Flores J, González S, Morales X, Yescas P, Ochoa A, Corona T. Absence of multiple sclerosis and demyelinating diseases among lacandonians, a pure Amerindian ethnic group in Mexico. Multiple Sclerosis Int (2012) 2012:e292631. doi: 10.1155/2012/292631
8. Hammond SR, de Wytt C, Maxwell IC, Landy PJ, English D, McLeod JG, et al. The epidemiology of multiple sclerosis in Queensland, Australia. J Neurological Sci (1987) 80:185–204. doi: 10.1016/0022-510X(87)90154-7
9. Barcellos LF, Sawcer S, Ramsay PP, Baranzini SE, Thomson G, Briggs F, et al. Heterogeneity at the HLA-DRB1 locus and risk for multiple sclerosis. Hum Mol Genet (2006) 15:2813–24. doi: 10.1093/hmg/ddl223
10. Chao MJ, Barnardo MCNM, Lincoln MR, Ramagopalan SV, Herrera BM, Dyment DA, et al. HLA class I alleles tag HLA-DRB1*1501 haplotypes for differential risk in multiple sclerosis susceptibility. Proc Natl Acad Sci U.S.A. (2008) 105:13069–74. doi: 10.1073/pnas.0801042105
11. Ramagopalan SV, Ebers GC. Multiple sclerosis: major histocompatibility complexity and antigen presentation. Genome Med (2009) 1:105. doi: 10.1186/gm105
12. French Research Group on Multiple Sclerosis Multiple sclerosis in 54 twinships: concordance rate is independent of zygosity. French research group on multiple sclerosis. Ann Neurol (1992) 32:724–7. doi: 10.1002/ana.410320604
13. Willer CJ, Dyment DA, Risch NJ, Sadovnick AD, Ebers GC, Canadian Collaborative Study Group. Twin concordance and sibling recurrence rates in multiple sclerosis. PNAS (2003) 100:12877–82. doi: 10.1073/pnas.1932604100
14. Fagnani C, Neale MC, Nisticò L, Stazi MA, Ricigliano VA, Buscarinu MC, et al. Twin studies in multiple sclerosis: A meta-estimation of heritability and environmentality. Mult Scler (2015) 21:1404–13. doi: 10.1177/1352458514564492
15. Berg-Hansen P, Moen SM, Sandvik L, Harbo HF, Bakken IJ, Stoltenberg C, et al. Prevalence of multiple sclerosis among immigrants in Norway. Mult Scler (2015) 21:695–702. doi: 10.1177/1352458514554055
16. Berg-Hansen P, Celius EG. Socio-economic factors and immigrant population studies of multiple sclerosis. Acta Neurol Scand (2015) 132:37–41. doi: 10.1111/ane.12429
17. Nasr Z, Majed M, Rostami A, Sahraian MA, Minagar A, Amini A, et al. Prevalence of multiple sclerosis in Iranian emigrants: review of the evidence. Neurol Sci (2016) 37:1759–63. doi: 10.1007/s10072-016-2641-7
18. Alharbi FM. Update in vitamin d and multiple sclerosis. Neurosci (Riyadh) (2015) 20:329–35. doi: 10.17712/nsj.2015.4.20150357
19. Hedström AK, Hillert J, Olsson T, Alfredsson L. Smoking and multiple sclerosis susceptibility. Eur J Epidemiol (2013) 28:867–74. doi: 10.1007/s10654-013-9853-4
20. Libbey JE, Cusick MF, Fujinami RS. Role of pathogens in multiple sclerosis. Int Rev Immunol (2014) 33:266–83. doi: 10.3109/08830185.2013.823422
21. Munger KL, Chitnis T, Ascherio A. Body size and risk of MS in two cohorts of US women. Neurology (2009) 73:1543–50. doi: 10.1212/WNL.0b013e3181c0d6e0
22. Ascherio A, Munger KL, Lennette ET, Spiegelman D, Hernán MA, Olek MJ, et al. Epstein-Barr Virus antibodies and risk of multiple sclerosis: a prospective study. JAMA (2001) 286:3083–8. doi: 10.1001/jama.286.24.3083
23. Ascherio A, Munger KL. 99th dahlem conference on infection, inflammation and chronic inflammatory disorders: Epstein–Barr virus and multiple sclerosis: epidemiological evidence. Clin Exp Immunol (2010) 160:120–4. doi: 10.1111/j.1365-2249.2010.04121.x
24. Belbasis L, Bellou V, Evangelou E, Ioannidis JPA, Tzoulaki I. Environmental risk factors and multiple sclerosis: an umbrella review of systematic reviews and meta-analyses. Lancet Neurol (2015) 14:263–73. doi: 10.1016/S1474-4422(14)70267-4
25. Giovannoni G, Cutter GR, Lunemann J, Martin R, Münz C, Sriram S, et al. Infectious causes of multiple sclerosis. Lancet Neurol (2006) 5:887–94. doi: 10.1016/S1474-4422(06)70577-4
26. Bjornevik K, Cortese M, Healy BC, Kuhle J, Mina MJ, Leng Y, et al. Longitudinal analysis reveals high prevalence of Epstein-Barr virus associated with multiple sclerosis. Science (2022) 375:296–301. doi: 10.1126/science.abj8222
28. Young LS, Yap LF, Murray PG. Epstein-Barr Virus: more than 50 years old and still providing surprises. Nat Rev Cancer (2016) 16:789–802. doi: 10.1038/nrc.2016.92
29. Taylor GS, Long HM, Brooks JM, Rickinson AB, Hislop AD. The immunology of Epstein-Barr virus-induced disease. Annu Rev Immunol (2015) 33:787–821. doi: 10.1146/annurev-immunol-032414-112326
30. Terry LA, Stewart JP, Nash AA, Fazakerley JK. Murine gammaherpesvirus-68 infection of and persistence in the central nervous system. J Gen Virol (2000) 81:2635–43. doi: 10.1099/0022-1317-81-11-2635
31. Kang H-R, Cho H-J, Kim S, Song IH, Lee TS, Hwang S, et al. Persistent infection of a gammaherpesvirus in the central nervous system. Virology (2012) 423:23–9. doi: 10.1016/j.virol.2011.11.012
32. Sunil-Chandra NP, Efstathiou S, Nash AA. Murine gammaherpesvirus 68 establishes a latent infection in mouse b lymphocytes in vivo. J Gen Virol (1992) 73(Pt 12):3275–9. doi: 10.1099/0022-1317-73-12-3275
33. Sunil-Chandra NP, Efstathiou S, Arno J, Nash AA. Virological and pathological features of mice infected with murine gamma-herpesvirus 68. J Gen Virol (1992) 73(Pt 9):2347–56. doi: 10.1099/0022-1317-73-9-2347
34. Cho H-J, Kim S, Kwak S-E, Kang T-C, Kim H-S, Kwon H-J, et al. Age-dependent pathogenesis of murine gammaherpesvirus 68 infection of the central nervous system. Mol Cells (2009) 27:105–11. doi: 10.1007/s10059-009-0011-5
35. Häusler M, Sellhaus B, Scheithauer S, Engler M, Alberg E, Teubner A, et al. Murine gammaherpesvirus-68 infection of mice: A new model for human cerebral Epstein–Barr virus infection. Ann Neurol (2005) 57:600–3. doi: 10.1002/ana.20440
36. Casiraghi C, Shanina I, Cho S, Freeman ML, Blackman MA, Horwitz MS. Gammaherpesvirus latency accentuates EAE pathogenesis: Relevance to Epstein-Barr virus and multiple sclerosis. PloS Pathog (2012) 8:e1002715. doi: 10.1371/journal.ppat.1002715
37. Márquez AC, Shanina I, Horwitz MS. Multiple sclerosis-like symptoms in mice are driven by latent γHerpesvirus-68 infected b cells. Front Immunol (2020) 11:584297. doi: 10.3389/fimmu.2020.584297
38. Virgin HW, Latreille P, Wamsley P, Hallsworth K, Weck KE, Dal Canto AJ, et al. Complete sequence and genomic analysis of murine gammaherpesvirus 68. J Virol (1997) 71:5894–904. doi: 10.1128/JVI.71.8.5894-5904.1997
39. Fujiwara S, Imadome K-I, Takei M. Modeling EBV infection and pathogenesis in new-generation humanized mice. Exp Mol Med (2015) 47:e135–5. doi: 10.1038/emm.2014.88
40. Traggiai E, Chicha L, Mazzucchelli L, Bronz L, Piffaretti J-C, Lanzavecchia A, et al. Development of a human adaptive immune system in cord blood cell-transplanted mice. Science (2004) 304:104–7. doi: 10.1126/science.1093933
41. Zdimerova H, Murer A, Engelmann C, Raykova A, Deng Y, Gujer C, et al. Attenuated immune control of Epstein-Barr virus in humanized mice is associated with the multiple sclerosis risk factor HLA-DR15. Eur J Immunol (2021) 51:64–75. doi: 10.1002/eji.202048655
42. Allanach JR, Hardman BK, Fettig NM, Mouat I, Gu Y, Jean-Baptiste V, et al. Insights into the role of Epstein-Barr virus infection in multiple sclerosis using a novel humanized mouse model of disease. J Immunol (2020) 204:58.9–9.
43. Moghaddam A, Rosenzweig M, Lee-Parritz D, Annis B, Johnson RP, Wang F. An animal model for acute and persistent Epstein-Barr virus infection. Science (1997) 276:2030–3. doi: 10.1126/science.276.5321.2030
44. Fujiwara S, Nakamura H. Animal models for gammaherpesvirus infections: Recent development in the analysis of virus-induced pathogenesis. Pathogens (2020) 9(2):116. doi: 10.3390/pathogens9020116
45. Tyler SD, Severini A. The complete genome sequence of herpesvirus papio 2 (Cercopithecine herpesvirus 16) shows evidence of recombination events among various progenitor herpesviruses. J Virol (2006) 80:1214–21. doi: 10.1128/JVI.80.3.1214-1221.2006
46. Haanstra KG, Dijkman K, Bashir N, Bauer J, Mary C, Poirier N, et al. Selective blockade of CD28-mediated T cell costimulation protects rhesus monkeys against acute fatal experimental autoimmune encephalomyelitis. J Immunol (2015) 194:1454–66. doi: 10.4049/jimmunol.1402563
47. Haanstra KG, Wubben JAM, Jonker M, Hart BA‘t. Induction of encephalitis in rhesus monkeys infused with lymphocryptovirus-infected b-cells presenting MOG(34-56) peptide. PloS One (2013) 8:e71549. doi: 10.1371/journal.pone.0071549
48. Estep RD, Hansen SG, Rogers KS, Axthelm MK, Wong SW. Genomic characterization of Japanese macaque rhadinovirus, a novel herpesvirus isolated from a nonhuman primate with a spontaneous inflammatory demyelinating disease. J Virol (2013) 87:512–23. doi: 10.1128/JVI.02194-12
49. Axthelm MK, Bourdette DN, Marracci GH, Su W, Mullaney ET, Manoharan M, et al. Japanese Macaque encephalomyelitis: a spontaneous multiple sclerosis-like disease in a nonhuman primate. Ann Neurol (2011) 70:362–73. doi: 10.1002/ana.22449
50. Govindan AN, Fitzpatrick KS, Manoharan M, Tagge I, Kohama SG, Ferguson B, et al. Myelin-specific T cells in animals with Japanese macaque encephalomyelitis. Ann Clin Transl Neurol (2021) 8:456–70. doi: 10.1002/acn3.51303
51. Blair TC, Manoharan M, Rawlings-Rhea SD, Tagge I, Kohama SG, Hollister-Smith J, et al. Immunopathology of Japanese macaque encephalomyelitis is similar to multiple sclerosis. J Neuroimmunol (2016) 291:1–10. doi: 10.1016/j.jneuroim.2015.11.026
52. Kanai K, Takashima K, Okuno K, Kato K, Sano H, Kuwamoto S, et al. Lifelong persistent EBV infection of rabbits with EBER1-positive lymphocyte infiltration and mild sublethal hemophagocytosis. Virus Res (2010) 153:172–8. doi: 10.1016/j.virusres.2010.07.026
53. Khan G, Ahmed W, Philip PS, Ali MH, Adem A. Healthy rabbits are susceptible to Epstein-Barr virus infection and infected cells proliferate in immunosuppressed animals. Virol J (2015) 12:28. doi: 10.1186/s12985-015-0260-1
54. Okuno K, Takashima K, Kanai K, Ohashi M, Hyuga R, Sugihara H, et al. Epstein-Barr Virus can infect rabbits by the intranasal or peroral route: an animal model for natural primary EBV infection in humans. J Med Virol (2010) 82:977–86. doi: 10.1002/jmv.21597
55. Reguraman N, Hassani A, Philip P, Khan G. Uncovering early events in primary Epstein-Barr virus infection using a rabbit model. Sci Rep (2021) 11:21220. doi: 10.1038/s41598-021-00668-x
56. Hassani A, Reguraman N, Shehab S, Khan G. Primary peripheral Epstein-Barr virus infection can lead to CNS infection and neuroinflammation in a rabbit model: Implications for multiple sclerosis pathogenesis. Front Immunol (2021) 12:764937. doi: 10.3389/fimmu.2021.764937
57. Takashima K, Ohashi M, Kitamura Y, Ando K, Nagashima K, Sugihara H, et al. A new animal model for primary and persistent Epstein-Barr virus infection: human EBV-infected rabbit characteristics determined using sequential imaging and pathological analysis. J Med Virol (2008) 80:455–66. doi: 10.1002/jmv.21102
58. Simas JP, Efstathiou S. Murine gammaherpesvirus 68: a model for the study of gammaherpesvirus pathogenesis. Trends Microbiol (1998) 6:276–82. doi: 10.1016/s0966-842x(98)01306-7
59. Weck KE, Kim SS, Virgin HW IV, Speck SH. Macrophages are the major reservoir of latent murine gammaherpesvirus 68 in peritoneal cells. J Virol (1999) 73:3273–83. doi: 10.1128/JVI.73.4.3273-3283.1999
60. Flaño E, Kim I-J, Moore J, Woodland DL, Blackman MA. Differential gamma-herpesvirus distribution in distinct anatomical locations and cell subsets during persistent infection in mice. J Immunol (2003) 170:3828–34. doi: 10.4049/jimmunol.170.7.3828
61. Canny SP, Goel G, Reese TA, Zhang X, Xavier R, Virgin HW. Latent gammaherpesvirus 68 infection induces distinct transcriptional changes in different organs. J Virol (2014) 88:730–8. doi: 10.1128/JVI.02708-13
62. Cardin RD, Brooks JW, Sarawar SR, Doherty PC. Progressive loss of CD8+ T cell-mediated control of a gamma-herpesvirus in the absence of CD4+ T cells. J Exp Med (1996) 184:863–71. doi: 10.1084/jem.184.3.863
63. Brooks JW, Hamilton-Easton AM, Christensen JP, Cardin RD, Hardy CL, Doherty PC. Requirement for CD40 ligand, CD4(+) T cells, and b cells in an infectious mononucleosis-like syndrome. J Virol (1999) 73:9650–4. doi: 10.1128/JVI.73.11.9650-9654.1999
64. Casiraghi C, Citlali Márquez A, Shanina I, Steven Horwitz M. Latent virus infection upregulates CD40 expression facilitating enhanced autoimmunity in a model of multiple sclerosis. Sci Rep (2015) 5:13995. doi: 10.1038/srep13995
65. Jog NR, McClain MT, Heinlen LD, Gross T, Towner R, Guthridge JM, et al. Epstein Barr Virus nuclear antigen 1 (EBNA-1) peptides recognized by adult multiple sclerosis patient sera induce neurologic symptoms in a murine model. J Autoimmun (2020) 106:102332. doi: 10.1016/j.jaut.2019.102332
66. Lanz TV, Brewer RC, Ho PP, Moon J-S, Jude KM, Fernandez D, et al. Clonally expanded b cells in multiple sclerosis bind EBV EBNA1 and GlialCAM. Nature (2022) 603:321–7. doi: 10.1038/s41586-022-04432-7
67. Polepole P, Bartenslager A, Liu Y, Petro TM, Fernando S, Zhang L. Epstein Barr Virus-immortalized Blymphocytes exacerbate experimental autoimmune encephalomyelitis in xenograft mice. J Med Virol (2021) 93:3813–23. doi: 10.1002/jmv.26188
68. Chen H, Zhong L, Zhang W, Zhang S, Hong J, Zhou X, et al. Dose-dependent outcome of EBV infection of humanized mice based on green raji unit (GRU) doses. Viruses (2021) 13:2184. doi: 10.3390/v13112184
69. Volk V, Theobald SJ, Danisch S, Khailaie S, Kalbarczyk M, Schneider A, et al. PD-1 blockade aggravates Epstein-Barr virus+ post-transplant lymphoproliferative disorder in humanized mice resulting in central nervous system involvement and CD4+ T cell dysregulations. Front Oncol (2020) 10:614876. doi: 10.3389/fonc.2020.614876
70. Nielsen TR, Rostgaard K, Askling J, Steffensen R, Oturai A, Jersild C, et al. Effects of infectious mononucleosis and HLA-DRB1*15 in multiple sclerosis. Mult Scler (2009) 15:431–6. doi: 10.1177/1352458508100037
71. Soldan SS, Su C, Lamontagne RJ, Grams N, Lu F, Zhang Y, et al. Epigenetic plasticity enables CNS-trafficking of EBV-infected b lymphocytes. PloS Pathog (2021) 17:e1009618. doi: 10.1371/journal.ppat.1009618
72. Jagessar SA, Fagrouch Z, Heijmans N, Bauer J, Laman JD, Oh L, et al. The different clinical effects of anti-BLyS, anti-APRIL and anti-CD20 antibodies point at a critical pathogenic role of γ-herpesvirus infected b cells in the marmoset EAE model. J Neuroimmune Pharmacol (2013) 8:727–38. doi: 10.1007/s11481-013-9448-6
73. Jagessar SA, Holtman IR, Hofman S, Morandi E, Heijmans N, Laman JD, et al. Lymphocryptovirus infection of nonhuman primate b cells converts destructive into productive processing of the pathogenic CD8 T cell epitope in myelin oligodendrocyte glycoprotein. J Immunol (2016) 197:1074–88. doi: 10.4049/jimmunol.1600124
74. Hart BA‘t. A tolerogenic role of cathepsin G in a primate model of multiple sclerosis: Abrogation by Epstein-Barr virus infection. Arch Immunol Ther Exp (Warsz) (2020) 68:21. doi: 10.1007/s00005-020-00587-1
75. Skalsky RL, Barr SA, Jeffery AJ, Blair T, Estep R, Wong SW. Japanese Macaque rhadinovirus encodes a viral MicroRNA mimic of the miR-17 family. J Virol (2016) 90:9350–63. doi: 10.1128/JVI.01123-16
76. Osborne AJ, Atkins HM, Balogh KK, Brendle SA, Shearer DA, Hu J, et al. Antibody-mediated immune subset depletion modulates the immune response in a rabbit (Oryctolagus cuniculus) model of Epstein-Barr virus infection. Comp Med (2020) 70:312–22. doi: 10.30802/AALAS-CM-20-000019
77. Meier U-C, Giovannoni G, Tzartos JS, Khan G. Translational mini-review series on b cell subsets in disease. b cells in multiple sclerosis: drivers of disease pathogenesis and Trojan horse for Epstein-Barr virus entry to the central nervous system? Clin Exp Immunol (2012) 167:1–6. doi: 10.1111/j.1365-2249.2011.04446.x
78. Frischer JM, Weigand SD, Guo Y, Kale N, Parisi JE, Pirko I, et al. Clinical and pathological insights into the dynamic nature of the white matter multiple sclerosis plaque. Ann Neurol (2015) 78:710–21. doi: 10.1002/ana.24497
79. Cortese I, Reich DS, Nath A. Progressive multifocal leukoencephalopathy and the spectrum of JC virus-related disease. Nat Rev Neurol (2021) 17:37–51. doi: 10.1038/s41582-020-00427-y
80. Serafini B, Rosicarelli B, Magliozzi R, Stigliano E, Aloisi F. Detection of ectopic b-cell follicles with germinal centers in the meninges of patients with secondary progressive multiple sclerosis. Brain Pathol (2004) 14:164–74. doi: 10.1111/j.1750-3639.2004.tb00049.x
81. Peters A, Pitcher LA, Sullivan JM, Mitsdoerffer M, Acton SE, Franz B, et al. Th17 cells induce ectopic lymphoid follicles in central nervous system tissue inflammation. Immunity (2011) 35:986–96. doi: 10.1016/j.immuni.2011.10.015
82. Wu Y, Maruo S, Yajima M, Kanda T, Takada K. Epstein-Barr Virus (EBV)-encoded RNA 2 (EBER2) but not EBER1 plays a critical role in EBV-induced b-cell growth transformation. J Virol (2007) 81:11236–45. doi: 10.1128/JVI.00579-07
83. Mauray S, Fuzzati-Armentero MT, Trouillet P, Rüegg M, Nicoloso G, Hart M, et al. Epstein-Barr Virus-dependent lymphoproliferative disease: critical role of IL-6. Eur J Immunol (2000) 30:2065–73. doi: 10.1002/1521-4141(200007)30:7<2065::AID-IMMU2065>3.0.CO;2-W
84. Daniels BP, Holman DW, Cruz-Orengo L, Jujjavarapu H, Durrant DM, Klein RS. Viral pathogen-associated molecular patterns regulate blood-brain barrier integrity via competing innate cytokine signals. mBio (2014) 5:e01476–01414. doi: 10.1128/mBio.01476-14
85. Förster C, Burek M, Romero IA, Weksler B, Couraud P-O, Drenckhahn D. Differential effects of hydrocortisone and TNFα on tight junction proteins in an in vitro model of the human blood–brain barrier. J Physiol (2008) 586:1937–49. doi: 10.1113/jphysiol.2007.146852
86. Phares TW, Kean RB, Mikheeva T, Hooper DC. Regional differences in blood-brain barrier permeability changes and inflammation in the apathogenic clearance of virus from the central nervous system. J Immunol (2006) 176:7666–75. doi: 10.4049/jimmunol.176.12.7666
87. Tsao N, Hsu HP, Wu CM, Liu CC, Lei HY. Tumour necrosis factor-alpha causes an increase in blood-brain barrier permeability during sepsis. J Med Microbiol (2001) 50:812–21. doi: 10.1099/0022-1317-50-9-812
88. Wong D, Dorovini-Zis K, Vincent SR. Cytokines, nitric oxide, and cGMP modulate the permeability of an in vitro model of the human blood-brain barrier. Exp Neurol (2004) 190:446–55. doi: 10.1016/j.expneurol.2004.08.008
89. Bonney S, Seitz S, Ryan CA, Jones KL, Clarke P, Tyler KL, et al. Gamma interferon alters junctional integrity via rho kinase, resulting in blood-brain barrier leakage in experimental viral encephalitis. mBio (2019) 10:e01675–19. doi: 10.1128/mBio.01675-19
90. Chai Q, He WQ, Zhou M, Lu H, Fu ZF. Enhancement of blood-brain barrier permeability and reduction of tight junction protein expression are modulated by chemokines/cytokines induced by rabies virus infection. J Virol (2014) 88:4698–710. doi: 10.1128/JVI.03149-13
91. Li F, Wang Y, Yu L, Cao S, Wang K, Yuan J, et al. Viral infection of the central nervous system and neuroinflammation precede blood-brain barrier disruption during Japanese encephalitis virus infection. J Virol (2015) 89:5602–14. doi: 10.1128/JVI.00143-15
92. Minagar A, Long A, Ma T, Jackson TH, Kelley RE, Ostanin DV, et al. Interferon (IFN)-beta 1a and IFN-beta 1b block IFN-gamma-induced disintegration of endothelial junction integrity and barrier. Endothelium (2003) 10:299–307. doi: 10.1080/10623320390272299
93. Cain MD, Salimi H, Gong Y, Yang L, Hamilton SL, Heffernan JR, et al. Virus entry and replication in the brain precedes blood-brain barrier disruption during intranasal alphavirus infection. J Neuroimmunol (2017) 308:118–30. doi: 10.1016/j.jneuroim.2017.04.008
94. Robinson WH, Steinman L. Epstein-Barr Virus and multiple sclerosis. Science (2022) 375:264–5. doi: 10.1126/science.abm7930
95. Pender MP, Csurhes PA, Smith C, Douglas NL, Neller MA, Matthews KK, et al. Epstein-Barr Virus-specific T cell therapy for progressive multiple sclerosis. JCI Insight (2018) 3:e124714. doi: 10.1172/jci.insight.124714
96. Serafini B, Rosicarelli B, Franciotta D, Magliozzi R, Reynolds R, Cinque P, et al. Dysregulated Epstein-Barr virus infection in the multiple sclerosis brain. J Exp Med (2007) 204:2899–912. doi: 10.1084/jem.20071030
97. Tzartos JS, Khan G, Vossenkamper A, Cruz-Sadaba M, Lonardi S, Sefia E, et al. Association of innate immune activation with latent Epstein-Barr virus in active MS lesions. Neurology (2012) 78:15–23. doi: 10.1212/WNL.0b013e31823ed057
98. Lassmann H, Niedobitek G, Aloisi F, Middeldorp JM, NeuroproMiSe EBV Working Group. Epstein-Barr Virus in the multiple sclerosis brain: a controversial issue–report on a focused workshop held in the centre for brain research of the medical university of Vienna, Austria. Brain (2011) 134:2772–86. doi: 10.1093/brain/awr197
Keywords: Epstein-Barr virus, multiple sclerosis, neuroinflammation, animal models, non-human primates (NHP), rodents, rabbits
Citation: Hassani A and Khan G (2022) What do animal models tell us about the role of EBV in the pathogenesis of multiple sclerosis? Front. Immunol. 13:1036155. doi: 10.3389/fimmu.2022.1036155
Received: 04 September 2022; Accepted: 02 November 2022;
Published: 17 November 2022.
Edited by:
Klemens Ruprecht, Charité Universitätsmedizin Berlin, GermanyReviewed by:
Shunbin Ning, East Tennessee State University, United StatesMarco Salvetti, Sapienza University of Rome, Italy
Gunnar Houen, Statens Serum Institut (SSI), Denmark
John Lindsey, University of Texas Health Science Center at Houston, United States
Christoph Friedli, Bern University Hospital and University of Bern, Switzerland
Copyright © 2022 Hassani and Khan. This is an open-access article distributed under the terms of the Creative Commons Attribution License (CC BY). The use, distribution or reproduction in other forums is permitted, provided the original author(s) and the copyright owner(s) are credited and that the original publication in this journal is cited, in accordance with accepted academic practice. No use, distribution or reproduction is permitted which does not comply with these terms.
*Correspondence: Gulfaraz Khan, Z19raGFuQHVhZXUuYWMuYWU=