- Centre for Infectious Disease Control, National Institute for Public Health and the Environment (RIVM), Bilthoven, Netherlands
Respiratory infectious diseases encountered early in life may result in life-threatening disease in neonates, which is primarily explained by the relatively naive neonatal immune system. Whereas vaccines are not readily available for all infectious diseases, vaccinations have greatly reduced childhood mortality. However, repeated vaccinations are required to reach protective immunity in infants and not all vaccinations are effective at young age. Moreover, protective adaptive immunity elicited by vaccination wanes more rapidly at young age compared to adulthood. The infant adaptive immune system has previously been considered immature but this paradigm has changed during the past years. Recent evidence shows that the early life adaptive immune system is equipped with a strong innate-like effector function to eliminate acute pathogenic threats. These strong innate-like effector capacities are in turn kept in check by a tolerogenic counterpart of the adaptive system that may have evolved to maintain balance and to reduce collateral damage. In this review, we provide insight into these aspects of the early life’s adaptive immune system by addressing recent literature. Moreover, we speculate that this shift from innate-like and tolerogenic adaptive immune features towards formation of immune memory may underlie different efficacy of infant vaccination in these different phases of immune development. Therefore, presence of innate-like and tolerogenic features of the adaptive immune system may be used as a biomarker to improve vaccination strategies against respiratory and other infections in early life.
Introduction
Respiratory infections within the first year of life are common and can evolve into severe disease. Infectious diseases are indeed a major cause of childhood death. For some infectious diseases causing high mortality at early age, such as invasive infection with respiratory syncytial virus (RSV), Klebsiella, and Staphylococcus aureus, vaccines are currently unavailable (1–4). Vaccination against respiratory infectious diseases have greatly reduced childhood mortality. Moreover, maternal vaccination has been implemented to circumvent susceptibility to respiratory disease at early age (5) by boosting the number of maternal antibodies that are transferred to the fetus during pregnancy. Implementation of maternal vaccination in several countries (6) has been shown to protect neonates (<1 month old) against respiratory infectious pathogens such as influenza virus and Bordetella pertussis that may cause life-threatening disease and may therefore provide the neonatal immune system with the necessary time to evolve into a fully protective immune system. However, achieving early-life immune protection against respiratory infectious diseases for which vaccines are available often requires administration of multiple vaccine doses and even then protection elicited can be of shorter duration compared to later in life, potentially leaving infants at risk for respiratory disease.
The innate immune system is an essential part of the early life immune system as it provides a first line of defense at primary pathogenic encounter. Moreover, already in early life the innate immune system starts to develop a form of nonspecific memory that is trained by pathogen exposure, referred to as trained immunity (7). Trained immunity enhances the response of NK cells and monocytes, amongst other cell types, through encountering pathogens and thereby providing nonspecific protection against a broad range of pathogens. For example, vaccination with Bacillus Calmette-Guérin (BCG) has been shown to induce trained immunity in human adults and infants, possibly providing protection not only against disseminated tuberculosis disease but also against other infectious diseases, as reviewed elsewhere (8). However, in contrast to trained immunity, adaptive immune responses that are led by T cells and B cells are vital to provide pathogen-specific protection by building up long-term memory to specifically prevent or limit disease during following encounters.
The neonatal adaptive immune system has long been regarded immature when compared to the adult immune system. Indeed, infants generate suboptimal (memory) T-cell and B-cell responses after vaccination and against acute infection. However, within both the infant T- and B-cell compartment various cell subsets are present that have inspired a change of perspective on the early life adaptive immune system. The view of immune underdevelopment has changed towards a view of immune tolerance: the immune system at early life starts out at being tolerogenic and then slowly adapts to the new environment to become a controlled inflammatory immune system. Moreover, subsets of the early life adaptive immune system appear to be equipped with unique innate-like features to provide rapid protection.
In this review, we assess current literature to find hallmarks of the unique neonatal adaptive immune system that may help to explain timing of (multiple) vaccine doses in order to boost adaptive immune responses against pathogens early in life. We argue that efficacy of vaccination is determined by the rate at which a neonate shifts from an early adaptive immune system that comprises features of tolerance and innate-like capacities towards a mature system of immune memory. We provide future directions for research to characterize whether the immune response to vaccination may be deemed protective or not.
T-cell responses at early age
CD4+ helper and effector T cells at early age: rapid innate-like features, low memory generation
The adult adaptive immune system is poised towards providing protection against current pathogenic threats, while also building long-lasting protection by generation of memory cells. A T-cell response is classically initiated by binding of the T-cell receptor (TCR) to its cognate epitope derived from a pathogen that is presented by antigen presenting cells (APCs) (9). Additionally, interaction of co-stimulatory CD80/CD86 on APCs with CD28 on T cells, amongst other co-stimulatory pathways, provides T cells with the necessary second signal that enhances their response. Once these requirements are met, T cells become activated, secrete cytokines, proliferate, and differentiate into specific subsets to provide acute and long-term protection (10). Of note, several APC subsets show differences in infants compared to adults, mainly comprising decreased functionality of dendritic cells, monocytes, macrophages, and granulocytes at young age, as extensively reviewed elsewhere (11). Consequently, altered APC functionality may in turn affect adaptive immune responses.
In adults, naive CD4+ T cells that encounter pathogen-derived antigens mainly differentiate into type 1 T-helper cells (Th1) that are characterized by secretion of pro-inflammatory interferon-γ (IFN-γ) and tumor necrosis factor-α (TNF-α) and thereby provide protection against viruses, bacteria, and parasites. However, the CD4+ T-cell response to pathogens in neonatal mice and humans has often been reported to be type 2 T-helper cell (Th2)-skewed at the expense of Th1-type responses (12–14). This may in part result in lower levels of Th1-associated cells early in life, whereas the level of Th2-associated cells remains stable with age (15). Th2 responses are characterized by secretion of interleukin (IL-) 4, IL-5, and IL-13 and skewing towards these Th2-type responses may affect vaccine- and infection-related protective T-cell responses. In neonatal mice, antigen recall responses, e.g. elicited by secondary RSV infection (16) or secondary exposure to ovalbumin (14), have been shown to trigger Th2-type responses. However, pioneering studies in neonatal mice have also shown that the shift in balance towards a Th2-type response depends on the type of antigenic stimulation (13, 17). For example, lowering the infectious dose of leukaemia virus resulted in Th1- and cytotoxic T-cell responses in contrast to the non-protective Th2-response with a high viral dose (17). This indicates that the murine neonatal immune system may be poised towards Th2-type responses in case of high infectious dose and/or secondary antigenic encounters.
In human neonates, steady state and polyclonally stimulated CD4+ T cells expressed high intracellular levels of IL-4 (18) and polyclonal stimulation also resulted in less IFN-γ production (19) compared to adult CD4+ T cells. Moreover, skewing towards Th2-type responses in neonates in vaccination settings has also been reported, although outcomes may vary depending on the type of vaccine and/or adjuvant administered, such as Th2-skewing aluminum-containing adjuvants (20), and subunit versus whole cell vaccines. The recall T-cell memory response elicited after hepatitis B subunit vaccination adjuvanted with aluminum hydroxide was primarily of a Th2-type in newborns in contrast to adults, which possibly also resulted in higher antibody responses compared to adults (21). Moreover, vaccination with a conjugate vaccine against pneumococcus (three doses) adjuvanted with aluminum phosphate in neonates has been associated with Th2-type responses (22), although no adult control group was included. In contrast, generation of robust Th1-type responses has been shown in neonates vaccinated with a BCG vaccine (23) and whole-cell pertussis vaccine (24), indicating that vaccines containing Toll-like receptor (TLR)-agonists may elicit substantial Th1 responses in neonates. In an infection setting, Th2 polarization has been reported in RSV-infected infants with acute bronchiolitis that showed an IL-4 skewed IL-4/IFN-γ ratio compared to infants with upper respiratory tract infection, indicating that increased Th2-type and/or decreased Th1-type responses may contribute to severe disease (25). Th2-skewed responses can be counteracted by the administration of type I IFN-α in mice (26). The role of type I IFN in RSV and infants has been reviewed extensively elsewhere (27).
Interestingly, the naive CD4+ T-cell population includes recent thymic emigrants (RTEs) that are highly abundant early in life (15) and express innate-like features such as TLR1 and TLR2 (28–30). Neonatal CD4+ T cells produce equal levels of IFN-γ and IL-2 after TLR2-stimulation compared to adult CD4+ T cells, whereas TCR stimulation alone induced lower IFN-γ and IL-2 production by neonatal compared to adult CD4+ T cells (29). Moreover, neonatal CD4+ T cells have been shown to produce the innate effector cytokine IL-8 in response to polyclonal and TLR stimulation (30) in contrast to adult T cells (31). Based on the findings that neonatal T cells adequately respond to TLR stimulation it may be argued that neonatal T cells preferentially use TLRs to recognize pathogens, resulting in cytokine secretion. This in contrast to the classical view of T-cell activation via recognition of specific epitopes via their TCR (32–34). Innate-like functionality of neonatal CD4+ T cells may also explain why some vaccines elicit robust Th1 responses, such as the BCG vaccine, which can trigger several TLRs, including TLR2 (23).
Innate-like effector capacities of neonatal CD4+ T cells may indicate that T cells generated at early life differ from those generated later in life. It has indeed been shown that neonatal T cells are derived from different progenitor cells compared to adult T cells, indicating the uniqueness of the neonatal immune system (35–37). Whereas the ‘investment’ of the early life adaptive immune system in innate-like properties may provide protection against acute respiratory pathogenic threats, this investment may also be seen to be at the expense of memory cell formation. As proposed previously (38), the innate-like features of neonatal T cells can be explained by the changing environment: e.g. the vast expansion of the neonatal microbiome makes it difficult for TCRs to distinguish between commensal bacteria and pathogenic bacteria. Also, the capacity of building a stable memory T-cell population is also subject to the process of central tolerance, in which self-reactive T cells get eliminated in the thymus to prevent unwanted responses to self-peptides. The T cells are constantly adapting to discriminate between self-antigens, commensal and pathogenic antigens. The potential to detect harmful pathogenic signals via TLRs is therefore highly needed, but at the cost of the capacity to generate a stable memory T-cell population. As a consequence, the downside of investing in innate-like properties may be the diminished response to vaccinations, as well as generation of long-lasting memory response to vaccinations.
Together, these findings indicate that I.) the neonatal T-cell response is not necessarily impaired, but may intrinsically be wired towards induction of Th2 responses during TCR-mediated responses, and II.) aside from Th1/Th2 skewing, the CD4+ T-cell compartment heavily ‘invests’ in innate-like T-cell features for rapid effector responses and immediate protection at the time when the capacity of memory formation is still developing, thereby compromising long-term protection.
CD8+ effector T cells
Effector CD8+ T cells are essential for short and long-term protection against respiratory and other infectious diseases following infection or vaccination. A major part of the T-cell population of neonates consists of RTEs (39), which functionally and epigenetically differ from naive T cells that have matured by circulating for a longer time (40, 41). Neonatal CD8+ T cells have been shown to express innate-associated molecules TLR2 and TLR5 (42). Moreover, neonatal murine CD8+ T cells have been shown to express NK cell-related transcripts and molecules (36, 43, 44) in contrast to adult CD8+ T cells, which again supports the innate-like features of neonatal T cells.
In addition to these innate features, the transcriptional profile of neonatal CD8+ T cells has been shown to be enriched for genes associated with cell cycle and anti-viral innate immune responses compared to adult CD8+ T cells, potentially to compensate for reduced levels of genes associated with cytotoxic T-cell function (45). Enrichment for genes associated with the cell cycle translated into higher proliferative capacity of neonatal CD8+ T cells compared to adult CD8+ T cells (45). The capacity of murine neonatal CD8+ T cells to rapidly expand after stimulation has been shown to result in a pool of short-lived effector cells that became terminally differentiated, whereas stimulation of adult CD8+ T cells resulted in a diverse pool of effector and memory T cells (46). A more recent study indeed showed that CD8+ T cells generated early in life show a more effector-like phenotype before stimulation and respond more rapidly after stimulation, whereas adult-derived CD8+ T cells comprise a pool of naive CD8+ T cells that are capable of forming a stable memory population (36). The difference in generation of CD8+ T cells between neonates and adults in response to infection is that CD8+ T cells of neonates may be derived from a different hematopoietic stem cell lineage (43). Lastly, the decay rate of CD8+ T cells produced at young age is high, which slows down with progressing age (47), indicating rapid turnover of these cells.
Together, it appears that also the neonatal CD8+ T-cell population is poised towards rapid effector and proliferation mechanisms to combat acute potentially harmful pathogens, whereas the capacity to generate memory CD8+ T cells is still developing, which poses a window for potentially life-threatening infections to occur. Moreover, reduced memory generation may also limit long-term vaccine efficacy. CD8+ T-cell proliferation to measles antigens after vaccination are lower in infants (6-12 months of age) compared to those of adults (48). Although these findings do not mean that the CD8+ T-cell response of these infants is not enough to provide protection against measles virus infection, it does indicate that not only neonates but also infants still show altered T-cell responsiveness, which may open a window to infection and/or decreased vaccine efficacy.
Unconventional T cells: γδ T cells and MAIT cells
The group of unconventional T cells are comprised of several subsets of cells, including T cells expressing the γδ T-cell receptor (γδ T cells) and mucosal associated invariant T cells (MAIT cells). γδ T cells bridge the innate and adaptive features of the immune system due to their capacity to act as antigen-presenting cells (49) and their activation is not restricted to MHC (50). It is currently thought that γδ T cells are one of the main lines of defense against pathogens during early life, at the time when protection mediated by conventional αβ T cells is still developing (50, 51). Indeed, a recent study has shown that γδ T cells rapidly expand and functionally develop in preterm and term infants in a short period of time, whereas αβ T cells show little development (52). This again illustrates a possible investment in innate-like T-cell features by the neonatal immune system. T cells expressing the γ-chain variable region 9 (Vγ9) and δ-chain variable region 2 (Vδ2) are most abundant in human peripheral blood, already providing an innate-like T-cell barrier in the developing fetus (53). It has been suggested that γδ T cells are a main line of defense against pathogens during cytomegalovirus infection already in utero (54), and γδ T cells were shown to produce IFN-γ after BCG vaccination in infants (55). Moreover, a recent study shows that shortly after birth (<10 weeks) Vγ9Vδ2 T cells differentiate into cytotoxic effector cells expressing granzymes and perforin similar to adult levels (56). However, their IFN-γ response to bacterial antigens such as the microbial-derived (E)‐4‐hydroxy‐3‐methyl‐but‐2‐enyl pyrophosphate (HMBPP) was lower compared to adults. Indeed, a recent study shows that the a γδ T-cell compartment is not completely established in neonates 14 days after birth, as γδ T cells of older children show higher IFN-γ responses to bacterial-derived antigens (57). Together, these studies indicate the importance of γδ T cells as a first line of defense against infections at early life, highlighting the importance of innate-like functionality at early age.
MAIT cells are a subset of T cells already present in neonatal cord blood that recognize vitamin B metabolites presented by MR1, which is an MHC class I-related protein (58). MAIT cells have been found to recognize cells infected with Mycobacterium tuberculosis (59), a pathogen which may cause severe disease in infants. Similar to γδ T cells, it has recently been shown that the population of MAIT cells rapidly expands and matures after birth (60, 61). Moreover, the functional TNF response of infant MAIT cells to Mycobacterium smegmatis was found to be greater than the response of adult MAIT cells (60). Lastly, the precise role of MAIT cells in vaccination remains to be established, as BCG vaccination in infants did not alter MAIT cell activation or memory phenotype (62), whereas MAIT cells have recently been shown to enhance the efficacy of an adenoviral vector COVID-19 vaccine (ChAdOx1) in humans by improving CD8+ T-cell responses (63). Together, these studies indicate that MAIT cells may play an important role in prevention of infections early in life.
Tolerance by T cells at early age
CD4+ Regulatory T cells
Immune tolerance is needed to prevent inflammatory innate-like immune responses during gestation (64) and to allow establishment of the microbiome after birth. As described above, neonatal T cells have innate-like effector capacities for rapid effector function, whereas the capacity to generate immune memory is still developing. As a consequence of these innate-like effector capacities, a strong, tightly regulated tolerogenic system is needed to dampen excessive inflammatory responses and to thereby prevent collateral immune-mediated damage.
During pregnancy, immune tolerance of the mother towards the fetus ensures fetal growth and development. Maternal tolerogenic M2-like macrophages, natural killer cells (NK cells), and regulatory T cells (Tregs) accumulate within the decidual wall to provide tolerance in order to prevent fetal rejection, but also to limit pathology during infections (65, 66). Similarly, fetal suppressive CD4+ CD25+ Tregs already appear in fetal tissue from 13 weeks of gestational age (67), and these fetal Tregs have been show to play an important role in immune suppression of CD4+ and CD8+ T-cell proliferation and cytokine secretion already in the absence of stimulation (35, 68).
The level of Tregs in neonatal cord blood and peripheral blood compared to adult peripheral blood has been shown to be higher (69–72). Moreover, newborn nonhuman primates also show higher levels of Tregs in the spleen and lungs in addition to peripheral blood (73), suggesting that increased presence of Tregs outside of the blood may also occur in human infants. However, a slight increase of Tregs with age when comparing cord blood with (young) adult peripheral blood has also been reported (74). A reason for these contrasting results is still unknown, although a difference in identification of Tregs by phenotypic markers, as well as definition of Treg subsets may account for these differences. Moreover, it may well be that the pace at which the shift from higher levels of Tregs in the fetus/neonate towards adult levels of Tregs occurs, differs per individual. It is tempting to speculate that this heterogeneity in tolerogenic shift on the individual level could subsequently determine the outcome of immune responses towards infection and vaccination. Thus, it may be valuable to assess Treg levels longitudinally on the individual level to determine whether some infants shift less rapidly than others. This may give an answer to whether heterogeneity in conversion from tolerance to inflammatory and memory formation is a proxy for response to vaccination and/or susceptibility to infection.
Reports on the functionality of neonatal Tregs are contrasting, but may be explained by the different experimental approaches, e.g. cord blood versus peripheral blood, as well as the cell type that is used as a readout in suppression culture assays. Cord blood-derived CD127Lo CD25+ Tregs were found to suppress a mix of CD4+CD25- T cells and dendritic cells as potently as the most suppressive (CD25Hi) adult-derived Tregs (75), or suppress CD4+CD127HiCD25- cells to the same extent as adult Tregs (74). Other studies report ambivalent results: expanded cord blood-derived CD25+ Tregs suppressed CD4+CD25- T cells to a greater level than adult peripheral blood-derived Tregs (76), or, in contrast, report that cord blood-derived CD127Lo CD25+ neonatal Tregs are less functional in suppressing dendritic cells compared to adult Tregs (77). Finally, a recent study showed that CD127Lo CD25+ Tregs in peripheral blood of adults and term neonates suppressed proliferation of CD4+CD25- T cells to the same extent, but that both suppressed these cells to a lesser extent than Tregs of preterm neonates (78), which seems to suggest that the suppressive potential of Tregs seems at its peak during gestation and declines after birth, together with declining Treg numbers.
Possibly, the pace at which the shift from high suppressive capacity to modest suppressive capacity occurs, influences susceptibility to respiratory infection. Presence of FoxP3+ Tregs during viral respiratory infections such as RSV has been shown to limit RSV-induced pathology caused by eosinophilia, CD4+ and CD8+ T cells in wildtype and FoxP3 DTR mice (allowing depletion of FoxP3+ Tregs) (79–81). The importance of Tregs in infants with severe RSV disease has also been shown, as reduced levels of activated Tregs were demonstrated in severe RSV infection (82), which may be needed to counteract pro-inflammatory IL-17-producing T cells (83). Thus, Tregs are important to dampen harmful inflammatory responses. However, this tolerogenic environment may also dampen inflammatory responses to vaccinations, which could be essential to elicit optimal differentiation of the adaptive responses, required for long term protection. Studies investigating the potential suppressive role of Tregs during vaccination against infectious diseases are relatively scarce. In infants, BCG vaccination was shown to induce expression of FoxP3, indicating Treg induction (84). Of these infants, some showed IFN-γ responses whereas other infants showed IL-10 responses to mycobacterial purified protein derivative, indicating immunological variation within the group of infants. Another study indeed suggests that CD25+ Tregs are involved in IL-10 secretion in response to BCG vaccination of neonates (85). In infants vaccinated against measles and Diphteria/Tetanus/Pertussis (DTP), a (relatively weak) negative association between the number of CD127Lo FoxP3+ Tregs present in the periphery and the antibody response to measles vaccination, but not DTP vaccination (Diph-Tet-Pert) was shown (86). Together these data indicate a potential suppressive role for Tregs during infant vaccination, although this may depend on individual Treg variation as well as the type of vaccine.
As neonatal T cells show innate-like characteristics, Tregs may have to deal with these innate-like capacities and respond to innate signals to prevent excessive inflammation. It has been shown that murine and human CD25+ and/or FoxP3+ Tregs express several TLRs (87–90). Exposure to TLR4 and TLR5 ligands, such as lipopolysaccharide (LPS) and flagellin, induces Treg suppressive activity and survival in the absence of TCR-mediated activation (87, 88, 90). TLR2 activation on the other hand, directly promotes Treg proliferation, but limits their suppressive capacity (89, 91–93), indicating that this innate feature is a mechanism to bypass the intrinsically tolerogenic wired neonatal immune system.
CD8+ regulatory T cells
CD8+ Tregs may also be involved in suppression of neonatal T-cell responses. For example, it has been shown that an important role of Qa-1-restricted (HLA-E in humans) CD8+ Tregs is to prevent autoimmune disease in adult mice by suppression of follicular helper T cells (94). Moreover, genetic disruption of these CD8+ Tregs resulted in increased clearance of acute and chronic viral infections in mouse models (95). However, studies addressing the role of CD8+ Tregs in human infants and neonatal mice are scarce. One study in a transplantation model of neonatal mice showed that long-lived CD8+ Tregs could prevent graft rejection by CD8+ T cells and these Tregs maintained tolerance at later life (96). Presence of CD8+ Tregs in human infants requires to be addressed, as this cell type could provide more insight into susceptibility to respiratory infectious disease and vaccination responsiveness.
Virtual memory T cells
Virtual memory CD8+ T cells (TVM cells) were first identified in unimmunized wildtype and germ-free mice and were described as cells that express a memory phenotype without having been exposed to antigen (97). Human TVM cells are characterized by expression of CD45RA and innate-like receptors NKG2A and several KIRs (98). TVM cells most likely develop via cytokine-induced homeostatic proliferation (97). In neonatal mice, TVM cells have been shown to be present in the periphery from two weeks of age, and their expansion was partly dependent on IL-4 (99). As CD4+ T cells of humans and mice show Th2-skewing towards IL-4 at early age, it may well be that the initial expansion of TVM cells is elicited by IL-4 secreted by Th2 cells.
The role of TVM cells in humans and mice is still being elucidated. TVM cells in mice have been shown to provide bystander protection during Listeria monocytogenes infection (100). Moreover, TVM cells have been shown to contribute to the control of HIV-infected CD4+ T cells in humans, likely through KIR-mediated interactions and killing of infected cells (101). Furthermore, our group has recently shown that KIR-expressing TVM cells accumulate in the blood with progressing age and are capable of suppressing proliferation of other CD8+ T cells (102). Based on these findings, TVM cells may also be considered a type of suppressive CD8+ T-cell subset, although their role at early age remains to be elucidated. Moreover, it has been shown previously that CD8+ Tregs expressing the innate receptor NKG2A can recognize HLA-E in an antigen specific manner and can both exert killing as well as regulatory suppressive functions (103, 104), which suggests a link between TVM cells and these HLA-E restricted CD8+ T cells. It would be of interest to address TVM cells in human neonates and infants as these cells may possibly be another feature of the adaptive early age immune system to invest in innate-like T-cell features and with potentially both pathogen-clearing and suppressive capacities.
Immune regulation by co-inhibitory receptors on neonatal T cells
The number of studies addressing expression of co-inhibitory receptors by neonatal T cells is limited. Expression of inhibitory receptors LAIR-1, CD31, and CD200 was increased on neonatal T cells derived from cord blood and peripheral blood compared to adult peripheral blood (105), indicating a potential mechanism for regulation of responsiveness of these T cells. On the other hand, these data could also indicate that excessive T-cell effector capacities are dampened to prevent pathology. Recently, expression of the C-type lectin CD161 by CD4+ T cells was shown to inhibit TCR-dependent induction of IFN-γ and these cells were present at a higher level in cord blood of newborns with chronic inflammation of the intestines as a consequence of gastroschisis (44). Together, expression of co-inhibitory receptors by infant T cells remains a relatively unexplored field which may provide new leads for investigation of reduced vaccine responsiveness.
Collectively on T-cell responses at early age: whilst innate-like and tolerogenic features have developed and as T-cell memory generation is low at early age, we speculate that the shift from innate-like features to generation of immune memory is an important step in the process to confer protection in childhood and beyond (Figure 1).
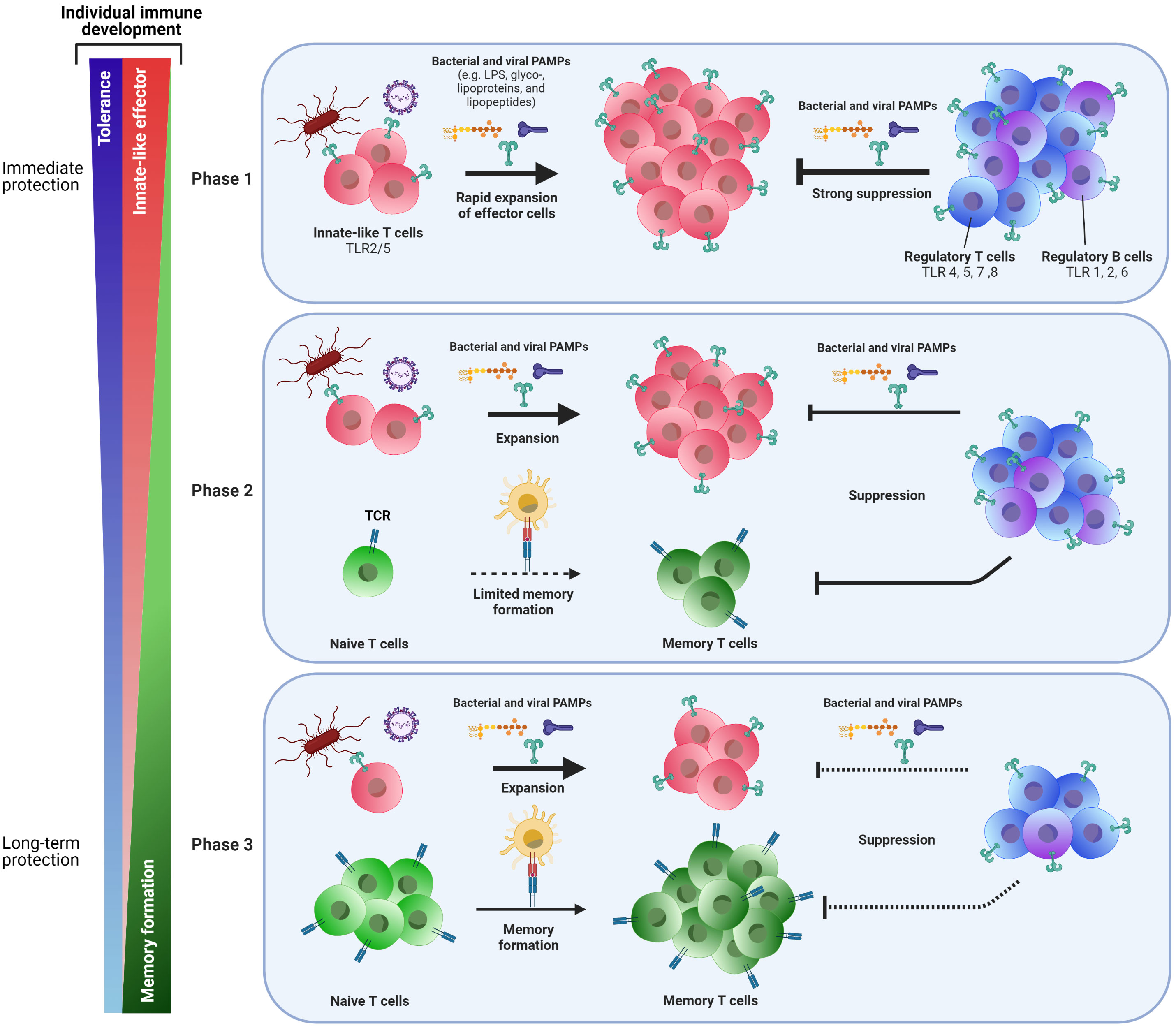
Figure 1 Phasic shift in the adaptive immune response to pathogens early in life: from immediate to long-term protection. Cells of the adaptive immune system change during the course of life. At birth (Phase 1), neonatal T cells express innate-like features such as TLRs 2 and 5, allowing these cells to recognize bacterial and viral PAMPs and act upon pathogenic threats by rapid expansion of these innate-like T cells and by exerting effector functions. To prevent immunopathology, a vast population of regulatory T and B cells can also recognize pathogens via several TLRs and exert suppressive responses to keep innate-like T-cell responses under control. Together, these mechanisms may provide short-term protection against pathogens, but does this at the expense of formation of memory T cells for long-term protection. Moreover, the efficacy of vaccines that do not trigger TLRs may be reduced early in life. With progressing age, the capacity to generate a population of memory T cells through TCR-MHC interactions of naive T cells with antigen presenting cells slowly develops (Phase 2). Innate-like T cells are still present at this stage, as well as suppressive regulatory T and B cells. Finally (Phase 3), formation of memory cells becomes the main pool of cells that confers protection to pathogenic threats and allows for long-lasting vaccine-mediated protection. Suppressive regulatory T and B cells are still present and needed to maintain the immunological balance. Whereas the shift from phase one to phase three may roughly be put alongside aging over time, there still is individual variation at which these changes to immune cells occur. Possibly, the pace at which the early life’s immune system shifts from acute innate-like and tolerogenic responsiveness towards memory generating responses for long term survival may determine whether some infants will be more rapidly protected, while others may be prone to more severe disease and/or reduced vaccination responses.
B-cell responses at early age
Generation and maintenance of protective antibody levels in neonates and infants
Next to T-cell immunity, the adaptive immune response that consists of B cells producing antibodies, seems also be differently regulated at early age. Vaccine- and infection-induced antibody responses in neonates are at a lower level and lower in affinity (106–112). Moreover, antibodies generated in early life wane rapidly, with low or undetectable levels at 6-9 months after primary and booster immunization against diphtheria and pertussis between 2 and 6 months of age (113, 114). It is likely to assume that here suboptimal priming of B cells takes place at an early age, inducing short-lived plasma cells. The insufficient vaccine efficacy by rapid waning of antibody levels in infants may decreased protection to disease.
Whereas extremely premature born neonates (<28 weeks of gestational age) were recently shown to be capable of mounting IgG antibodies against most antigens present in DTaP-IPV-Hib-HepB vaccines comparable to term born neonates (115), generation of protective antibodies is still not optimal at young age. Although neutralizing antibodies elicited by maternal vaccination provide protection for 3-4 months after birth, the levels of these antibodies decline over time (116). For measles, vaccination generally starts at 12 months of age, which leaves a window of opportunity for infection to establish as the capacity for long-lived antibody production in neonates starts to increase gradually from 6 to 9 months of age (107, 117). Earlier vaccination of infants may be a solution to narrow this window for infection. However, recent studies show that earlier vaccination against measles results in a long-term decrease in concentration and avidity of neutralizing antibodies below the cutoff for clinical protection against measles (118), as well as a rapid decay of their polyfunctionality (119). Together, these studies indicate that not only generation of antibodies is reduced early in life, but also the maintenance of antibody levels, which may be caused by less sustained maturation and differentiation of memory B cells and plasma cells at young age, resulting in suboptimal protection later in life.
Follicular helper T cells
Generation of protective antibodies requires a chain of events to occur to elicit robust, protective, and long-lasting antibody responses. Follicular helper T cells (TFH cells) bridge the interaction between T- and B-cell populations (120). TFH cells mediate generation and maintenance of B-cell germinal centers (GCs) where affinity maturation takes place and B-cell differentiation towards long-lived memory B cells and plasma cells producing high-affinity class-switched antibodies (121, 122).
Functional TFH cells are characterized by several factors, including expression of the transcriptional master regulator Bcl-6, as well as IL-21, and IL-4 (120, 121). Impaired TFH cell generation and differentiation may subsequently affect generation of protective B-cell responses. In neonatal mice, vaccine-induced TFH-cell generation is impaired, as characterized by lower expression of Bcl-6 and IL-21, as well as reduced migration into germinal centers, which may be the cause for reduced antibody responses found in these mice (123). Similarly to neonatal CD8+ T cells (45), neonatal mouse TFH cells were shown to be enriched for cell cycle-related genes, indicating that also this T-cell subset in neonates is poised towards rapid action instead of memory generation (124). Moreover, TFH cells elicited in neonatal mice showed a bias to Th2 cytokines, which may negatively affect further generation of TFH cells (124). Adjuvantation of vaccines with CpG oligodeoxynucleotide (CpG-ODN), amongst others, have been shown to reduce Th2 bias and thereby overcome reduced TFH-cell and antibody responses, indicating that providing the right stimulus can result in more optimal responses (125, 126). Whereas circulating CXCR5+ Tfh cells in humans are important for generation of B-cell memory against influenza (127), there currently are, to the best of our knowledge, no studies that address circulating TFH cells at early life. Thus, strong TFH responses are crucial for developing robust antibody and (memory) B-cell responses. In neonates, reduced TFH-cell responses may affect the remaining chain of events that would normally lead to robust B-cell memory and protective antibody responses.
Suppression of antibody production by TFH cells through IL-10
Both IL-21 and IL-6 are important for TFH differentiation and therefore contribute to boosting of antibody responses and B-cell differentiation (121, 128). Whereas IL-21 provides signals to promote lymphocyte differentiation (129) and has been implicated in the development of several autoimmune diseases (130), IL-21 can paradoxically elicit immunosuppressive responses through induction of IL-10 secretion by T cells. Interestingly, polyclonal stimulation of naive CD4+ T cells from cord blood with anti-CD3, anti-CD28, and IL-21 results in more IL-10 production compared to adult naive CD4+ T cells and thereby suppressed proliferation of CD4+ T cells (131). More evidence for TFH/IL-10-mediated suppression may be found from studies investigating the other spectrum of age: old age. At older age it is well known that levels of both pro- and anti-inflammatory cytokine levels increase, one of which is pro-inflammatory IL-6 (132). A recent study has shown a pivotal role for the balance between IL-6, IL-10, and IL-21 in the generation of so-called TFH10 cells in aged mice (133). These TFH10 cells derived their name from their capacity to produce IL-10 which is propagated by production of IL-21 to act as a positive feedback loop for TFH10 generation and maintenance. IL-10 secretion subsequently limited antibody responses to influenza vaccination and the authors speculate that this IL-10 production is established to compensate for the pro-inflammatory micro-environment created by IL-6 (133). Possibly, these findings suggest that neonatal IL-10-producing TFH cells may contribute to decreased B-cell responses and antibody levels found at young age. If IL-10-producing TFH cells are indeed involved in suppressing neonatal B-cell and antibody responses, it would be of high interest to address whether these cells become less tolerogenic as age progresses. Together, they may provide new leads to susceptibility to respiratory infectious disease and reduce vaccine responses early in life.
B cells: Class-switch recombination and somatic hypermutation
As noted above, an impairment in the chain of events that lead to generation of high-affinity antibodies may affect short- and long-term antibody generation and maintenance. B cells of both term and preterm born neonates have been shown to express lower levels of several co-stimulatory receptors, including CD40, CD80, CD86, and some TNFR family receptors (134), which may impede their cross-talk with T cells. Indeed, activation of neonatal B cells with CD40L resulted in lower IgG and IgA production compared to adult B cells (134).
Once antigenic exposure has taken place, interaction with T cells is required for B cells to undergo class-switch recombination (CSR), recently shown to occur before GC formation (135). Subsequent GC formation is required to propagate B-cells and generate high affinity antibody responses. However, impaired formation of GCs and lower presence of TFH cells within GCs of neonatal mice may result in lower antibody generation (123), and may also affect generation of long-lived B-cell memory and plasma cells and thus long-term antibody levels. CSR of B cells is followed by T-cell independent affinity maturation via somatic hypermutation (SHM) of the B cell receptor within the GC. It has recently been shown that B-cell SHM increases during the first three years of life (reaching 60-75% of adult SHM frequencies), mainly due to increasing antigenic exposure (136), indicating that B cells of neonates may not yet be sufficiently capable of generating higher-affinity antibodies.
It has recently been shown that numbers of total memory B cells and plasma cells are low in peripheral blood of newborns, but the number of these cells rapidly increases within 11 months of age, reaching their maximum number at approximately 1 year of age (137). However, these are total levels and may not reflect vaccine-induced responses. Whereas vaccination against meningococcal serogroup C was shown to be effective in producing antibodies in infants after two doses instead of three doses (138), another study showed that multiple doses of a conjugated MenC vaccine were required to induce memory B cells and plasma cells (139), showing suboptimal induction of B-cell response after the first vaccination. This indicates that the short-term induction of antibodies may be adequate in some vaccines, but long-term protection mediated by memory B cells and plasma cells may be suboptimal at early age.
Regulatory B cells (Bregs)
Regulatory B cells (Bregs) secrete high levels of IL-10, transforming growth factor-β (TGF-β), and IL-35 and are thereby capable of suppressing other cell types (140, 141), including various T-cell subsets, as well as the induction of Tregs (142). Differentiation of immature B cells into IL-10-producing Bregs has been shown to be driven by IFN-α secreting plasmacytoid dendritic cells (143). Interestingly, skewing to Th2-type responses in neonatal mice may be explained by IL-10-producing Breg that can be triggered via TLR2, TLR4, and TLR9 (144, 145) as secretion of IL-10 by B cells limits dendritic cell-mediated priming of Th1-type responses (144). There is only a limited number of studies addressing the role of Bregs in human infection and vaccination, especially at early age. The frequency of cord blood Bregs within the B-cell population is higher compared to adult peripheral blood and these Bregs inhibit IFN-γ (Th1) and IL-4 (Th2) responses (146), suggesting that these cells play a role in the Th1/Th2 balance at young age (146). Similarly, the frequency of neonatal Bregs (nBregs) amongst the B-cell population is higher in peripheral blood shortly after birth (1-4weeks) compared to one year after birth and adulthood (147). Infants that suffer from severe RSV infection show RSV-infected nBregs that produce IL-10, which in turn affected protective Th1 responses (147). The frequency of nBregs present in the blood positively correlated with symptom severity, indicating the negative effect of suppression. Interestingly, nBregs have also been addressed as being part of an innate-like B-cell population (148), which indeed produce IL-10 after type I IFN secretion induced by adjuvant-mediated TLR9 stimulation (149). Together, these findings show that innate-like and regulatory cellular characteristics are not only present within the T-cell population, but can also be found within the B-cell population at early life.
Antibody production: Interference by maternal antibodies
It is well known that maternal antibodies provide protection shortly after birth to protect the infant against harmful pathogenic respiratory infections (150–153). Moreover, maternal immunization is now being used as a way to increase protection of the newborn, as extensively reviewed elsewhere (154). Additionally, maternal vaccination may potentially be an important strategy to provide time for the newborn’s immune system to switch from a tolerogenic/innate-like adaptive immune system towards a well-balanced memory generating immune system. Possibly, neonates and infants who are ‘slow switchers’ may especially benefit from maternal vaccination, as it may provide more time to make the shift. However, maternal antibodies (MatAbs) have also been shown to blunt infant immune responses (155–157), which may be an additional explanation for reduced antibody generation in early life. Indeed, a recent study has shown that maternal tetanus, diphteria, and acellular pertussis (Tdap) vaccination elicits MatAbs that interfere with primary and booster vaccination antibody concentrations in newborns (158). As studies have shown that MatAbs can negatively interfere with the neonatal response to vaccines, MatAbs may thus be another hurdle to overcome for the neonate to provide itself with adequate protection. The mechanism behind inhibition of early-life antibody responses by MatAbs remains under debate. MatAbs can interfere with the immune response by binding vaccine-derived immunodominant epitopes, which leaves only non-immunodominant epitopes to be bound by neonatal B cells. This may result in hampered B-cell differentiation and weak antibody responses. A recent study in mice provides evidence that vaccine-induced high levels of MatAbs may also impair expansion of TFH cells and germinal center B cells (159). This study shows that MatAbs impair differentiation of neonatal B cells towards plasma cells and memory B cells, and also alters expression of the B-cell receptor by B cells and thereby the B-cell repertoire (159). Thus, this indicates that future research may search for the fine line between eliciting enough MatAbs through maternal vaccination to protect the neonate, but not in such high levels that MatAbs impede antibody generation by the neonatal immune system. The effect of MatAbs on human B-cell and T-cell differentiation remains to be further investigated.
Heterogeneity in the pace of adaptive-immune shifting from innate-like to long-term memory
Collectively, published evidence shows a previously less recognized role for the shift from an innate-like and tolerogenic-orientated immune system towards a system of memory formation early in life. We speculate that the shift from innate-like features to generation of immune memory is an important step in the process to confer protection in childhood and beyond (Figure 1). However, the speed at which this process takes place may vary between individuals of the same age. Multiple heritable and non-heritable influences may have an impact on the pace of this immunological shift, as indicated by several reports on the variation within the human immune system (15, 160–162). For example, the frequency of TLR-expressing RTEs amongst naive CD4+ T cells varies between ∼10-80% early in life, declining thereafter (15). Based on these reports, we propose that the pace at which a newborn child shifts from its innate-like and tolerogenic adaptive immune features towards formation of immune memory may determine the efficacy of infant vaccination and/or their susceptibility to respiratory infection (Figure 2).
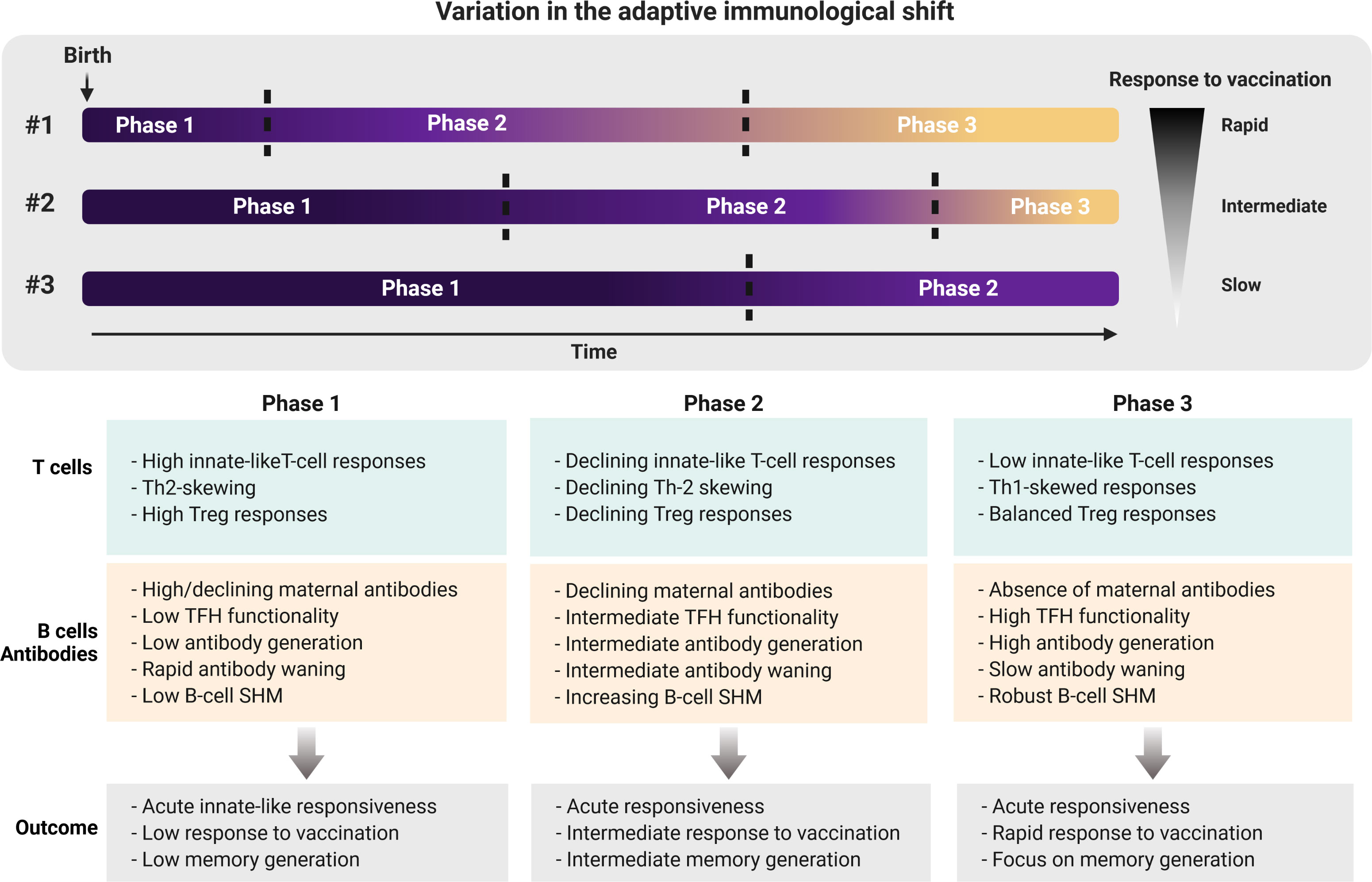
Figure 2 Individual variation in shifting from adaptive innate-like and tolerogenic features early in life towards memory generation later in life. The pace at which the adaptive immune system of newborns shifts from a system with high innate-like and tolerogenic features towards an adult-like memory generating system may determine the susceptibility to infectious disease, as well as the protective efficacy of vaccinations. Neonates who are ‘rapid shifters’ (#1) possibly are better at generating immune memory as a result of infection and/or vaccination for long-lasting protection compared to ‘intermediate shifters’ (#2) and ‘slow shifters’ (#3). Future studies addressing hallmarks of shifting through these phases early in life may therefore provide new insights into whether neonates or infants require multiple doses of vaccinations or not. Th, Thelper; Treg, regulatory T cell; TFH, follicular helper T cell; SHM, somatic hypermutation.
A major question that remains is by what factors the pace of the early life’s immunological shift is driven. Identifying these factors in future research will be challenging, as it most likely will be a multifactorial process. First, immune development over time is an important factor. Many adaptive immune parameters change early in life, including those in the B-cell (137) and T-cell populations (163). Duration of the pregnancy may be an important factor in this, as immune development consists of several layers, first establishing mutual tolerance to prevent damaging alloreactions, and then slowly evolving into functional effector immune capacities (64). Possibly, the speed of this evolvement is a heterogeneous process, and as infants are vaccinated chronologically after birth, and preterm neonates may therefore have a different immune composition compared to term neonates (164). Second, the (microbial) environment may influence the shift, due to the vast perinatal and postnatal exposure to a novel microbiome (38). For example, perinatal inflammation may alter immune development (52), and the mode of delivery determines the composition of the neonatal microbiome and associated with respiratory infections in the first year of life (165). Another environmental example may be the classical hygiene hypothesis, as exposure to pathogens or antibiotics early in life may determine the nature of consecutive immune responses (166, 167). A way to address potential immune modulatory effects may be through administration of a BCG vaccination (168). Lastly, genetics may play a role in the pace of adaptive-immune shifting. As the early life adaptive immune system relies on innate-like characteristics, genetic variation in TLRs (169, 170) may influence the response to vaccination and infection (171). Thus, the pace at which the adaptive immunological shift at early age may be subjected to heritable and non-heritable factors, and we hypothesize that the phase at which a neonate or infant resides in may determine the effectiveness of vaccination and/or the level of protection elicited against infectious pathogens.
Conclusion and future perspectives
Susceptibility to respiratory infectious diseases and reduced response to vaccination in neonates can be ascribed to a differently wired adaptive immune system. The neonatal immune system must be aggressive enough to clear pathogens to ensure survival of the newborn, whereas it should also allow the microbiome to establish. Focusing on comparisons between neonatal adaptive immune capacities by comparing these to those of adults may be a one-dimensional view, and is secondary to the fact that the neonatal adaptive immune system is wired differently: it has characteristic innate-like and tolerogenic features to combat pathogens. The neonatal adaptive immune system should therefore be recognized as a system that can respond to acute pathogenic threats by rapid innate-like effector responsiveness. Concomitantly, this rapid innate-responsiveness is tightly controlled by tolerogenic cells to prevent collateral damage, whilst also allowing the neonatal immune system to invest in more time-consuming processes, such as the generation of naive T cells and the capacity to shift to features that promote memory differentiation. The presence of MatAbs provides protection during the first months after birth and thereby provides the time necessary to shift towards immune memory formation. However, interindividual variation in the development of the adaptive immune system may dictate the speed of this immunological shift and we argue that this may determine the effectiveness of vaccination against respiratory pathogens at young age. Moreover, the pace of adaptive-immune shifting may also account for the susceptibility to infectious diseases, although it may also be that effectiveness of vaccines and susceptibility to infectious diseases are not the same. Based on these notions, the major challenges ahead are to determine whether infants that are susceptible to (severe) infectious diseases are also those infants that respond less effectively to vaccination, or that these are two different processes. Following on this, it would be vital to determine whether the stage at which the adaptive immune system resides in at that moment can be used as a proxy to predict susceptibility to disease and lower effectiveness of vaccination. Together, these insights may aid in vaccination strategies to determine the ideal time for vaccination of neonates and infants and whether adjusting vaccine formulations to specifically boost innate-like adaptive responses may confer greater protection than current strategies. Thus, identification of factors that drive the shift from innate-like to an adaptive immune system that successfully generate long term memory cells should be a primary objective for further research. Moreover, future research should then define whether a rapid shifter is less prone to infectious disease and responds better to vaccination compared to infants who are slower shifters. Collectively, these new insights will help optimizing vaccination strategies to be able to better protect young infants against respiratory infectious diseases.
Author contributions
DP – reviewed literature, conceptualized, wrote, and revised the manuscript. MB – reviewed literature, conceptualized and revised the manuscript. JW – reviewed literature, conceptualized, and revised the manuscript. All authors contributed to the article and approved the submitted version.
Funding
This study was supported by the Dutch Ministry of Health, Welfare and Sport.
Acknowledgments
We thank Willem Luytjes and Teun Guichelaar for critically reviewing the manuscript and useful discussions. Figures were created with BioRender.com.
Conflict of interest
The authors declare that the research was conducted in the absence of any commercial or financial relationships that could be construed as a potential conflict of interest.
Publisher’s note
All claims expressed in this article are solely those of the authors and do not necessarily represent those of their affiliated organizations, or those of the publisher, the editors and the reviewers. Any product that may be evaluated in this article, or claim that may be made by its manufacturer, is not guaranteed or endorsed by the publisher.
References
1. Kollmann TR, Marchant A, Way SS. Vaccination strategies to enhance immunity in neonates. Science (2020) 368(6491):612–5. doi: 10.1126/science.aaz9447
2. Okomo U, Akpalu ENK, Le Doare K, Roca A, Cousens S, Jarde A, et al. Aetiology of invasive bacterial infection and antimicrobial resistance in neonates in sub-Saharan Africa: a systematic review and meta-analysis in line with the STROBE-NI reporting guidelines. Lancet Infect Dis (2019) 19(11):1219–34. doi: 10.1016/S1473-3099(19)30414-1
3. Saha SK, Schrag SJ, El Arifeen S, Mullany LC, Shahidul Islam M, Shang N, et al. Causes and incidence of community-acquired serious infections among young children in south Asia (ANISA): an observational cohort study. Lancet (2018) 392(10142):145–59. doi: 10.1016/S0140-6736(18)31127-9
4. Shi T, McAllister DA, O'Brien KL, Simoes EAF, Madhi SA, Gessner BD, et al. Global, regional, and national disease burden estimates of acute lower respiratory infections due to respiratory syncytial virus in young children in 2015: a systematic review and modelling study. Lancet (2017) 390(10098):946–58. doi: 10.1016/S0140-6736(17)30938-8
5. Saso A, Kampmann B. Vaccine responses in newborns. Semin Immunopathol (2017) 39(6):627–42. doi: 10.1007/s00281-017-0654-9
6. Vojtek I, Dieussaert I, Doherty TM, Franck V, Hanssens L, Miller J, et al. Maternal immunization: where are we now and how to move forward? Ann Med (2018) 50(3):193–208. doi: 10.1080/07853890.2017.1421320
7. Netea MG, Joosten LA, Latz E, Mills KH, Natoli G, Stunnenberg HG, et al. Trained immunity: A program of innate immune memory in health and disease. Science (2016) 352(6284):aaf1098. doi: 10.1126/science.aaf1098
8. Covian C, Fernandez-Fierro A, Retamal-Diaz A, Diaz FE, Vasquez AE, Lay MK, et al. BCG-Induced cross-protection and development of trained immunity: Implication for vaccine design. Front Immunol (2019) 10:2806. doi: 10.3389/fimmu.2019.02806
9. Chen L, Flies DB. Molecular mechanisms of T cell co-stimulation and co-inhibition. Nat Rev Immunol (2013) 13(4):227–42. doi: 10.1038/nri3405
10. Harty JT, Badovinac VP. Shaping and reshaping CD8+ T-cell memory. Nat Rev Immunol (2008) 8(2):107–19. doi: 10.1038/nri2251
11. Tsafaras GP, Ntontsi P, Xanthou G. Advantages and limitations of the neonatal immune system. Front Pediatr (2020) 8:5. doi: 10.3389/fped.2020.00005
12. Barrios C, Brawand P, Berney M, Brandt C, Lambert PH, Siegrist CA. Neonatal and early life immune responses to various forms of vaccine antigens qualitatively differ from adult responses: predominance of a Th2-biased pattern which persists after adult boosting. Eur J Immunol (1996) 26(7):1489–96. doi: 10.1002/eji.1830260713
13. Forsthuber T, Yip HC, Lehmann PV. Induction of TH1 and TH2 immunity in neonatal mice. Science (1996) 271(5256):1728–30. doi: 10.1126/science.271.5256.1728
14. Li L, Lee HH, Bell JJ, Gregg RK, Ellis JS, Gessner A, et al. IL-4 utilizes an alternative receptor to drive apoptosis of Th1 cells and skews neonatal immunity toward Th2. Immunity (2004) 20(4):429–40. doi: 10.1016/S1074-7613(04)00072-X
15. Carr EJ, Dooley J, Garcia-Perez JE, Lagou V, Lee JC, Wouters C, et al. The cellular composition of the human immune system is shaped by age and cohabitation. Nat Immunol (2016) 17(4):461–8. doi: 10.1038/ni.3371
16. You D, Marr N, Saravia J, Shrestha B, Lee GI, Turvey SE, et al. IL-4Ralpha on CD4+ T cells plays a pathogenic role in respiratory syncytial virus reinfection in mice infected initially as neonates. J Leukoc Biol (2013) 93(6):933–42. doi: 10.1189/jlb.1012498
17. Sarzotti M, Robbins DS, Hoffman PM. Induction of protective CTL responses in newborn mice by a murine retrovirus. Science (1996) 271(5256):1726–8. doi: 10.1126/science.271.5256.1726
18. Hebel K, Weinert S, Kuropka B, Knolle J, Kosak B, Jorch G, et al. CD4+ T cells from human neonates and infants are poised spontaneously to run a nonclassical IL-4 program. J Immunol (2014) 192(11):5160–70. doi: 10.4049/jimmunol.1302539
19. White GP, Watt PM, Holt BJ, Holt PG. Differential patterns of methylation of the IFN-gamma promoter at CpG and non-CpG sites underlie differences in IFN-gamma gene expression between human neonatal and adult CD45RO- T cells. J Immunol (2002) 168(6):2820–7. doi: 10.4049/jimmunol.168.6.2820
20. Brewer JM, Conacher M, Satoskar A, Bluethmann H, Alexander J. In interleukin-4-deficient mice, alum not only generates T helper 1 responses equivalent to freund's complete adjuvant, but continues to induce T helper 2 cytokine production. Eur J Immunol (1996) 26(9):2062–6. doi: 10.1002/eji.1830260915
21. Ota MO, Vekemans J, Schlegel-Haueter SE, Fielding K, Whittle H, Lambert PH, et al. Hepatitis b immunisation induces higher antibody and memory Th2 responses in new-borns than in adults. Vaccine (2004) 22(3-4):511–9. doi: 10.1016/j.vaccine.2003.07.020
22. van den Biggelaar AH, Richmond PC, Pomat WS, Phuanukoonnon S, Nadal-Sims MA, Devitt CJ, et al. Neonatal pneumococcal conjugate vaccine immunization primes T cells for preferential Th2 cytokine expression: a randomized controlled trial in Papua new Guinea. Vaccine (2009) 27(9):1340–7. doi: 10.1016/j.vaccine.2008.12.046
23. Marchant A, Goetghebuer T, Ota MO, Wolfe I, Ceesay SJ, De Groote D, et al. Newborns develop a Th1-type immune response to mycobacterium bovis bacillus calmette-guerin vaccination. J Immunol (1999) 163(4):2249–55.
24. Mascart F, Verscheure V, Malfroot A, Hainaut M, Pierard D, Temerman S, et al. Bordetella pertussis infection in 2-month-old infants promotes type 1 T cell responses. J Immunol (2003) 170(3):1504–9. doi: 10.4049/jimmunol.170.3.1504
25. Legg JP, Hussain IR, Warner JA, Johnston SL, Warner JO. Type 1 and type 2 cytokine imbalance in acute respiratory syncytial virus bronchiolitis. Am J Respir Crit Care Med (2003) 168(6):633–9. doi: 10.1164/rccm.200210-1148OC
26. Cormier SA, Shrestha B, Saravia J, Lee GI, Shen L, DeVincenzo JP, et al. Limited type I interferons and plasmacytoid dendritic cells during neonatal respiratory syncytial virus infection permit immunopathogenesis upon reinfection. J Virol (2014) 88(16):9350–60. doi: 10.1128/JVI.00818-14
27. Hijano DR, Vu LD, Kauvar LM, Tripp RA, Polack FP, Cormier SA. Role of type I interferon (IFN) in the respiratory syncytial virus (RSV) immune response and disease severity. Front Immunol (2019) 10:566. doi: 10.3389/fimmu.2019.00566
28. Komai-Koma M, Jones L, Ogg GS, Xu D, Liew FY. TLR2 is expressed on activated T cells as a costimulatory receptor. Proc Natl Acad Sci U S A (2004) 101(9):3029–34. doi: 10.1073/pnas.0400171101
29. Sinnott BD, Park B, Boer MC, Lewinsohn DA, Lancioni CL. Direct TLR-2 costimulation unmasks the proinflammatory potential of neonatal CD4+ T cells. J Immunol (2016) 197(1):68–77. doi: 10.4049/jimmunol.1501297
30. Pekalski ML, Garcia AR, Ferreira RC, Rainbow DB, Smyth DJ, Mashar M, et al. Neonatal and adult recent thymic emigrants produce IL-8 and express complement receptors CR1 and CR2. JCI Insight (2017) 2(16):e93739. doi: 10.1172/jci.insight.93739
31. Gibbons D, Fleming P, Virasami A, Michel ML, Sebire NJ, Costeloe K, et al. Interleukin-8 (CXCL8) production is a signatory T cell effector function of human newborn infants. Nat Med (2014) 20(10):1206–10. doi: 10.1038/nm.3670
32. Whiteside SK, Snook JP, Williams MA, Weis JJ. Bystander T cells: A balancing act of friends and foes. Trends Immunol (2018) 39(12):1021–35. doi: 10.1016/j.it.2018.10.003
33. Kim J, Chang DY, Lee HW, Lee H, Kim JH, Sung PS, et al. Innate-like cytotoxic function of bystander-activated CD8(+) T cells is associated with liver injury in acute hepatitis a. Immunity (2018) 48(1):161–73 e5. doi: 10.1016/j.immuni.2017.11.025
34. Lee HG, Cho MZ, Choi JM. Bystander CD4(+) T cells: crossroads between innate and adaptive immunity. Exp Mol Med (2020) 52(8):1255–63. doi: 10.1038/s12276-020-00486-7
35. Mold JE, Venkatasubrahmanyam S, Burt TD, Michaelsson J, Rivera JM, Galkina SA, et al. Fetal and adult hematopoietic stem cells give rise to distinct T cell lineages in humans. Science (2010) 330(6011):1695–9. doi: 10.1126/science.1196509
36. Smith NL, Patel RK, Reynaldi A, Grenier JK, Wang J, Watson NB, et al. Developmental origin governs CD8(+) T cell fate decisions during infection. Cell (2018) 174(1):117–30 e14. doi: 10.1016/j.cell.2018.05.029
37. Zens KD, Chen JK, Guyer RS, Wu FL, Cvetkovski F, Miron M, et al. Reduced generation of lung tissue-resident memory T cells during infancy. J Exp Med (2017) 214(10):2915–32. doi: 10.1084/jem.20170521
38. Davenport MP, Smith NL, Rudd BD. Building a T cell compartment: how immune cell development shapes function. Nat Rev Immunol (2020) 20(8):499–506. doi: 10.1038/s41577-020-0332-3
39. Opiela SJ, Koru-Sengul T, Adkins B. Murine neonatal recent thymic emigrants are phenotypically and functionally distinct from adult recent thymic emigrants. Blood (2009) 113(22):5635–43. doi: 10.1182/blood-2008-08-173658
40. Berkley AM, Hendricks DW, Simmons KB, Fink PJ. Recent thymic emigrants and mature naive T cells exhibit differential DNA methylation at key cytokine loci. J Immunol (2013) 190(12):6180–6. doi: 10.4049/jimmunol.1300181
41. Friesen TJ, Ji Q, Fink PJ. Recent thymic emigrants are tolerized in the absence of inflammation. J Exp Med (2016) 213(6):913–20. doi: 10.1084/jem.20151990
42. McCarron M, Reen DJ. Activated human neonatal CD8+ T cells are subject to immunomodulation by direct TLR2 or TLR5 stimulation. J Immunol (2009) 182(1):55–62. doi: 10.4049/jimmunol.182.1.55
43. Wang J, Wissink EM, Watson NB, Smith NL, Grimson A, Rudd BD. Fetal and adult progenitors give rise to unique populations of CD8+ T cells. Blood (2016) 128(26):3073–82. doi: 10.1182/blood-2016-06-725366
44. Halkias J, Rackaityte E, Hillman SL, Aran D, Mendoza VF, Marshall LR, et al. CD161 contributes to prenatal immune suppression of IFNgamma-producing PLZF+ T cells. J Clin Invest (2019) 129(9):3562–77. doi: 10.1172/JCI125957
45. Galindo-Albarran AO, Lopez-Portales OH, Gutierrez-Reyna DY, Rodriguez-Jorge O, Sanchez-Villanueva JA, Ramirez-Pliego O, et al. CD8(+) T cells from human neonates are biased toward an innate immune response. Cell Rep (2016) 17(8):2151–60. doi: 10.1016/j.celrep.2016.10.056
46. Smith NL, Wissink E, Wang J, Pinello JF, Davenport MP, Grimson A, et al. Rapid proliferation and differentiation impairs the development of memory CD8+ T cells in early life. J Immunol (2014) 193(1):177–84. doi: 10.4049/jimmunol.1400553
47. Reynaldi A, Smith NL, Schlub TE, Tabilas C, Venturi V, Rudd BD, et al. Fate mapping reveals the age structure of the peripheral T cell compartment. Proc Natl Acad Sci U S A (2019) 116(10):3974–81. doi: 10.1073/pnas.1811634116
48. Gans HA, Yasukawa LL, Alderson A, Rinki M, Dehovitz R, Maldonado Y, et al. T Cell immunity to measles viral proteins in infants and adults after measles immunization. Viral Immunol (2004) 17(2):298–307. doi: 10.1089/0882824041310522
49. Brandes M, Willimann K, Moser B. Professional antigen-presentation function by human gammadelta T cells. Science (2005) 309(5732):264–8. doi: 10.1126/science.1110267
50. Vantourout P, Hayday A. Six-of-the-best: unique contributions of gammadelta T cells to immunology. Nat Rev Immunol (2013) 13(2):88–100. doi: 10.1038/nri3384
51. Gibbons DL, Haque SF, Silberzahn T, Hamilton K, Langford C, Ellis P, et al. Neonates harbour highly active gammadelta T cells with selective impairments in preterm infants. Eur J Immunol (2009) 39(7):1794–806. doi: 10.1002/eji.200939222
52. Kamdar S, Hutchinson R, Laing A, Stacey F, Ansbro K, Millar MR, et al. Perinatal inflammation influences but does not arrest rapid immune development in preterm babies. Nat Commun (2020) 11(1):1284. doi: 10.1038/s41467-020-14923-8
53. Dimova T, Brouwer M, Gosselin F, Tassignon J, Leo O, Donner C, et al. Effector Vgamma9Vdelta2 T cells dominate the human fetal gammadelta T-cell repertoire. Proc Natl Acad Sci U S A (2015) 112(6):E556–65. doi: 10.1073/pnas.1412058112
54. Vermijlen D, Brouwer M, Donner C, Liesnard C, Tackoen M, Van Rysselberge M, et al. Human cytomegalovirus elicits fetal gammadelta T cell responses in utero. J Exp Med (2010) 207(4):807–21. doi: 10.1084/jem.20090348
55. Zufferey C, Germano S, Dutta B, Ritz N, Curtis N. The contribution of non-conventional T cells and NK cells in the mycobacterial-specific IFNgamma response in bacille calmette-guerin (BCG)-immunized infants. PloS One (2013) 8(10):e77334. doi: 10.1371/journal.pone.0077334
56. Papadopoulou M, Dimova T, Shey M, Briel L, Veldtsman H, Khomba N, et al. Fetal public Vgamma9Vdelta2 T cells expand and gain potent cytotoxic functions early after birth. Proc Natl Acad Sci U S A (2020) 117(31):18638–48. doi: 10.1073/pnas.1922595117
57. van der Heiden M, Bjorkander S, Rahman Qazi K, Bittmann J, Hell L, Jenmalm MC, et al. Characterization of the gammadelta T-cell compartment during infancy reveals clear differences between the early neonatal period and 2 years of age. Immunol Cell Biol (2020) 98(1):79–87. doi: 10.1111/imcb.12303
58. Gold MC, Eid T, Smyk-Pearson S, Eberling Y, Swarbrick GM, Langley SM, et al. Human thymic MR1-restricted MAIT cells are innate pathogen-reactive effectors that adapt following thymic egress. Mucosal Immunol (2013) 6(1):35–44. doi: 10.1038/mi.2012.45
59. Harriff MJ, Cansler ME, Toren KG, Canfield ET, Kwak S, Gold MC, et al. Human lung epithelial cells contain mycobacterium tuberculosis in a late endosomal vacuole and are efficiently recognized by CD8(+) T cells. PloS One (2014) 9(5):e97515. doi: 10.1371/journal.pone.0097515
60. Swarbrick GM, Gela A, Cansler ME, Null MD, Duncan RB, Nemes E, et al. Postnatal expansion, maturation, and functionality of MR1T cells in humans. Front Immunol (2020) 11:556695. doi: 10.3389/fimmu.2020.556695
61. Ben Youssef G, Tourret M, Salou M, Ghazarian L, Houdouin V, Mondot S, et al. Ontogeny of human mucosal-associated invariant T cells and related T cell subsets. J Exp Med (2018) 215(2):459–79. doi: 10.1084/jem.20171739
62. Gela A, Murphy M, Rodo M, Hadley K, Hanekom WA, Boom WH, et al. Effects of BCG vaccination on donor unrestricted T cells in two prospective cohort studies. EBioMedicine (2022) 76:103839. doi: 10.1016/j.ebiom.2022.103839
63. Provine NM, Amini A, Garner LC, Spencer AJ, Dold C, Hutchings C, et al. MAIT cell activation augments adenovirus vector vaccine immunogenicity. Science (2021) 371(6528):521–6. doi: 10.1126/science.aax8819
64. Park JE, Jardine L, Gottgens B, Teichmann SA, Haniffa M. Prenatal development of human immunity. Science (2020) 368(6491):600–3. doi: 10.1126/science.aaz9330
65. Mor G, Aldo P, Alvero AB. The unique immunological and microbial aspects of pregnancy. Nat Rev Immunol (2017) 17(8):469–82. doi: 10.1038/nri.2017.64
66. Yockey LJ, Lucas C, Iwasaki A. Contributions of maternal and fetal antiviral immunity in congenital disease. Science (2020) 368(6491):608–12. doi: 10.1126/science.aaz1960
67. Darrasse-Jeze G, Marodon G, Salomon BL, Catala M, Klatzmann D. Ontogeny of CD4+CD25+ regulatory/suppressor T cells in human fetuses. Blood (2005) 105(12):4715–21. doi: 10.1182/blood-2004-10-4051
68. Michaelsson J, Mold JE, McCune JM, Nixon DF. Regulation of T cell responses in the developing human fetus. J Immunol (2006) 176(10):5741–8. doi: 10.4049/jimmunol.176.10.5741
69. Nettenstrom L, Alderson K, Raschke EE, Evans MD, Sondel PM, Olek S, et al. An optimized multi-parameter flow cytometry protocol for human T regulatory cell analysis on fresh and viably frozen cells, correlation with epigenetic analysis, and comparison of cord and adult blood. J Immunol Methods (2013) 387(1-2):81–8. doi: 10.1016/j.jim.2012.09.014
70. Renno C, Nadaf MI, Zago CA, Carneiro-Sampaio M, Palmeira P. Healthy preterm newborns show an increased frequency of CD4(+) CD25(high) CD127(low) FOXP3(+) regulatory T cells with a naive phenotype and high expression of gut-homing receptors. Scand J Immunol (2016) 83(6):445–55. doi: 10.1111/sji.12435
71. van Gent R, van Tilburg CM, Nibbelke EE, Otto SA, Gaiser JF, Janssens-Korpela PL, et al. Refined characterization and reference values of the pediatric T- and b-cell compartments. Clin Immunol (2009) 133(1):95–107. doi: 10.1016/j.clim.2009.05.020
72. Prabhu SB, Rathore DK, Nair D, Chaudhary A, Raza S, Kanodia P, et al. Comparison of human neonatal and adult blood leukocyte subset composition phenotypes. PloS One (2016) 11(9):e0162242. doi: 10.1371/journal.pone.0162242
73. Holbrook BC, Alexander-Miller MA. Higher frequency and increased expression of molecules associated with suppression on T regulatory cells from newborn compared with adult nonhuman primates. J Immunol (2020) 205:2128–36. doi: 10.4049/jimmunol.2000461
74. Santner-Nanan B, Seddiki N, Zhu E, Quent V, Kelleher A, Fazekas de St Groth B, et al. Accelerated age-dependent transition of human regulatory T cells to effector memory phenotype. Int Immunol (2008) 20(3):375–83. doi: 10.1093/intimm/dxm151
75. Godfrey WR, Spoden DJ, Ge YG, Baker SR, Liu B, Levine BL, et al. Cord blood CD4(+)CD25(+)-derived T regulatory cell lines express FoxP3 protein and manifest potent suppressor function. Blood (2005) 105(2):750–8. doi: 10.1182/blood-2004-06-2467
76. Fan H, Yang J, Hao J, Ren Y, Chen L, Li G, et al. Comparative study of regulatory T cells expanded ex vivo from cord blood and adult peripheral blood. Immunology (2012) 136(2):218–30. doi: 10.1111/j.1365-2567.2012.03573.x
77. Rueda CM, Moreno-Fernandez ME, Jackson CM, Kallapur SG, Jobe AH, Chougnet CA. Neonatal regulatory T cells have reduced capacity to suppress dendritic cell function. Eur J Immunol (2015) 45(9):2582–92. doi: 10.1002/eji.201445371
78. Pagel J, Twisselmann N, Rausch TK, Waschina S, Hartz A, Steinbeis M, et al. Increased regulatory T cells precede the development of bronchopulmonary dysplasia in preterm infants. Front Immunol (2020) 11:565257. doi: 10.3389/fimmu.2020.565257
79. Durant LR, Makris S, Voorburg CM, Loebbermann J, Johansson C, Openshaw PJ. Regulatory T cells prevent Th2 immune responses and pulmonary eosinophilia during respiratory syncytial virus infection in mice. J Virol (2013) 87(20):10946–54. doi: 10.1128/JVI.01295-13
80. Fulton RB, Meyerholz DK, Varga SM. Foxp3+ CD4 regulatory T cells limit pulmonary immunopathology by modulating the CD8 T cell response during respiratory syncytial virus infection. J Immunol (2010) 185(4):2382–92. doi: 10.4049/jimmunol.1000423
81. Loebbermann J, Durant L, Thornton H, Johansson C, Openshaw PJ. Defective immunoregulation in RSV vaccine-augmented viral lung disease restored by selective chemoattraction of regulatory T cells. Proc Natl Acad Sci U S A (2013) 110(8):2987–92. doi: 10.1073/pnas.1217580110
82. Christiaansen AF, Syed MA, Ten Eyck PP, Hartwig SM, Durairaj L, Kamath SS, et al. Altered treg and cytokine responses in RSV-infected infants. Pediatr Res (2016) 80(5):702–9. doi: 10.1038/pr.2016.130
83. Stoppelenburg AJ, de Roock S, Hennus MP, Bont L, Boes M. Elevated Th17 response in infants undergoing respiratory viral infection. Am J Pathol (2014) 184(5):1274–9. doi: 10.1016/j.ajpath.2014.01.033
84. Hanekom WA. The immune response to BCG vaccination of newborns. Ann N Y Acad Sci (2005) 1062:69–78. doi: 10.1196/annals.1358.010
85. Akkoc T, Aydogan M, Yildiz A, Karakoc-Aydiner E, Eifan A, Keles S, et al. Neonatal BCG vaccination induces IL-10 production by CD4+ CD25+ T cells. Pediatr Allergy Immunol (2010) 21(7):1059–63. doi: 10.1111/j.1399-3038.2010.01051.x
86. Ndure J, Noho-Konteh F, Adetifa JU, Cox M, Barker F, Le MT, et al. Negative correlation between circulating CD4(+)FOXP3(+)CD127(-) regulatory T cells and subsequent antibody responses to infant measles vaccine but not diphtheria-Tetanus-Pertussis vaccine implies a regulatory role. Front Immunol (2017) 8:921. doi: 10.3389/fimmu.2017.00921
87. Lewkowicz P, Lewkowicz N, Sasiak A, Tchorzewski H. Lipopolysaccharide-activated CD4+CD25+ T regulatory cells inhibit neutrophil function and promote their apoptosis and death. J Immunol (2006) 177(10):7155–63. doi: 10.4049/jimmunol.177.10.7155
88. Crellin NK, Garcia RV, Hadisfar O, Allan SE, Steiner TS, Levings MK. Human CD4+ T cells express TLR5 and its ligand flagellin enhances the suppressive capacity and expression of FOXP3 in CD4+CD25+ T regulatory cells. J Immunol (2005) 175(12):8051–9. doi: 10.4049/jimmunol.175.12.8051
89. Sutmuller RP, den Brok MH, Kramer M, Bennink EJ, Toonen LW, Kullberg BJ, et al. Toll-like receptor 2 controls expansion and function of regulatory T cells. J Clin Invest (2006) 116(2):485–94. doi: 10.1172/JCI25439
90. Caramalho I, Lopes-Carvalho T, Ostler D, Zelenay S, Haury M, Demengeot J. Regulatory T cells selectively express toll-like receptors and are activated by lipopolysaccharide. J Exp Med (2003) 197(4):403–11. doi: 10.1084/jem.20021633
91. Gerriets VA, Kishton RJ, Johnson MO, Cohen S, Siska PJ, Nichols AG, et al. Foxp3 and toll-like receptor signaling balance treg cell anabolic metabolism for suppression. Nat Immunol (2016) 17(12):1459–66. doi: 10.1038/ni.3577
92. Nyirenda MH, Morandi E, Vinkemeier U, Constantin-Teodosiu D, Drinkwater S, Mee M, et al. TLR2 stimulation regulates the balance between regulatory T cell and Th17 function: a novel mechanism of reduced regulatory T cell function in multiple sclerosis. J Immunol (2015) 194(12):5761–74. doi: 10.4049/jimmunol.1400472
93. Oberg HH, Ly TT, Ussat S, Meyer T, Kabelitz D, Wesch D. Differential but direct abolishment of human regulatory T cell suppressive capacity by various TLR2 ligands. J Immunol (2010) 184(9):4733–40. doi: 10.4049/jimmunol.0804279
94. Kim HJ, Verbinnen B, Tang X, Lu L, Cantor H. Inhibition of follicular T-helper cells by CD8(+) regulatory T cells is essential for self tolerance. Nature (2010) 467(7313):328–32. doi: 10.1038/nature09370
95. Holderried TA, Lang PA, Kim HJ, Cantor H. Genetic disruption of CD8+ treg activity enhances the immune response to viral infection. Proc Natl Acad Sci U S A (2013) 110(52):21089–94. doi: 10.1073/pnas.1320999110
96. Reibke R, Garbi N, Ganss R, Hammerling GJ, Arnold B, Oelert T. CD8+ regulatory T cells generated by neonatal recognition of peripheral self-antigen. Proc Natl Acad Sci U S A (2006) 103(41):15142–7. doi: 10.1073/pnas.0602622103
97. Haluszczak C, Akue AD, Hamilton SE, Johnson LD, Pujanauski L, Teodorovic L, et al. The antigen-specific CD8+ T cell repertoire in unimmunized mice includes memory phenotype cells bearing markers of homeostatic expansion. J Exp Med (2009) 206(2):435–48. doi: 10.1084/jem.20081829
98. Jacomet F, Cayssials E, Basbous S, Levescot A, Piccirilli N, Desmier D, et al. Evidence for eomesodermin-expressing innate-like CD8(+) KIR/NKG2A(+) T cells in human adults and cord blood samples. Eur J Immunol (2015) 45(7):1926–33. doi: 10.1002/eji.201545539
99. Akue AD, Lee JY, Jameson SC. Derivation and maintenance of virtual memory CD8 T cells. J Immunol (2012) 188(6):2516–23. doi: 10.4049/jimmunol.1102213
100. White JT, Cross EW, Burchill MA, Danhorn T, McCarter MD, Rosen HR, et al. Virtual memory T cells develop and mediate bystander protective immunity in an IL-15-dependent manner. Nat Commun (2016) 7:11291. doi: 10.1038/ncomms11291
101. Jin JH, Huang HH, Zhou MJ, Li J, Hu W, Huang L, et al. Virtual memory CD8+ T cells restrain the viral reservoir in HIV-1-infected patients with antiretroviral therapy through derepressing KIR-mediated inhibition. Cell Mol Immunol (2020) 17(12):1257–65. doi: 10.1038/s41423-020-0408-9
102. Pieren DKJ, Smits NAM, Hoeboer J, Kandiah V, Postel RJ, Mariman R, et al. Regulatory KIR(+) RA(+) T cells accumulate with age and are highly activated during viral respiratory disease. Aging Cell (2021) 20(6):e13372. doi: 10.1111/acel.13372
103. Joosten SA, van Meijgaarden KE, van Weeren PC, Kazi F, Geluk A, Savage ND, et al. Mycobacterium tuberculosis peptides presented by HLA-e molecules are targets for human CD8 T-cells with cytotoxic as well as regulatory activity. PloS Pathog (2010) 6(2):e1000782. doi: 10.1371/journal.ppat.1000782
104. van Meijgaarden KE, Haks MC, Caccamo N, Dieli F, Ottenhoff TH, Joosten SA. Human CD8+ T-cells recognizing peptides from mycobacterium tuberculosis (Mtb) presented by HLA-e have an unorthodox Th2-like, multifunctional, mtb inhibitory phenotype and represent a novel human T-cell subset. PloS Pathog (2015) 11(3):e1004671. doi: 10.1371/journal.ppat.1004671
105. Walk J, Westerlaken GH, van Uden NO, Belderbos ME, Meyaard L, Bont LJ. Inhibitory receptor expression on neonatal immune cells. Clin Exp Immunol (2012) 169(2):164–71. doi: 10.1111/j.1365-2249.2012.04599.x
106. Adkins B, Leclerc C, Marshall-Clarke S. Neonatal adaptive immunity comes of age. Nat Rev Immunol (2004) 4(7):553–64. doi: 10.1038/nri1394
107. Crowe JE Jr., Williams JV. Immunology of viral respiratory tract infection in infancy. Paediatr Respir Rev (2003) 4(2):112–9. doi: 10.1016/S1526-0542(03)00033-2
108. Wright PF, Karron RA, Belshe RB, Thompson J, Crowe JE Jr., Boyce TG, et al. Evaluation of a live, cold-passaged, temperature-sensitive, respiratory syncytial virus vaccine candidate in infancy. J Infect Dis (2000) 182(5):1331–42. doi: 10.1086/315859
109. Siegrist CA, Aspinall R. B-cell responses to vaccination at the extremes of age. Nat Rev Immunol (2009) 9(3):185–94. doi: 10.1038/nri2508
110. Karron RA, Wright PF, Belshe RB, Thumar B, Casey R, Newman F, et al. Identification of a recombinant live attenuated respiratory syncytial virus vaccine candidate that is highly attenuated in infants. J Infect Dis (2005) 191(7):1093–104. doi: 10.1086/427813
111. Murphy BR, Alling DW, Snyder MH, Walsh EE, Prince GA, Chanock RM, et al. Effect of age and preexisting antibody on serum antibody response of infants and children to the f and G glycoproteins during respiratory syncytial virus infection. J Clin Microbiol (1986) 24(5):894–8. doi: 10.1128/jcm.24.5.894-898.1986
112. Brandenburg AH, Groen J, van Steensel-Moll HA, Claas EC, Rothbarth PH, Neijens HJ, et al. Respiratory syncytial virus specific serum antibodies in infants under six months of age: limited serological response upon infection. J Med Virol (1997) 52(1):97–104. doi: 10.1002/(SICI)1096-9071(199705)52:1<97::AID-JMV16>3.0.CO;2-Y
113. Tiru M, Hallander HO, Gustafsson L, Storsaeter J, Olin P. Diphtheria antitoxin response to DTP vaccines used in Swedish pertussis vaccine trials, persistence and projection for timing of booster. Vaccine (2000) 18(21):2295–306. doi: 10.1016/S0264-410X(99)00539-3
114. Giuliano M, Mastrantonio P, Giammanco A, Piscitelli A, Salmaso S, Wassilak SG. Antibody responses and persistence in the two years after immunization with two acellular vaccines and one whole-cell vaccine against pertussis. J Pediatr (1998) 132(6):983–8. doi: 10.1016/S0022-3476(98)70395-6
115. Rouers EDM, Bruijning-Verhagen PCJ, van Gageldonk PGM, van Dongen JAP, Sanders EAM, Berbers GAM. Association of routine infant vaccinations with antibody levels among preterm infants. JAMA (2020) 324(11):1068–77. doi: 10.1001/jama.2020.12316
116. Niewiesk S. Maternal antibodies: clinical significance, mechanism of interference with immune responses, and possible vaccination strategies. Front Immunol (2014) 5:446. doi: 10.3389/fimmu.2014.00446
117. Gans H, DeHovitz R, Forghani B, Beeler J, Maldonado Y, Arvin AM. Measles and mumps vaccination as a model to investigate the developing immune system: passive and active immunity during the first year of life. Vaccine (2003) 21(24):3398–405. doi: 10.1016/S0264-410X(03)00341-4
118. Brinkman ID, de Wit J, Smits GP, Ten Hulscher HI, Jongerius MC, Abreu TC, et al. Early measles vaccination during an outbreak in the Netherlands: Short-term and long-term decreases in antibody responses among children vaccinated before 12 months of age. J Infect Dis (2019) 220(4):594–602. doi: 10.1093/infdis/jiz159
119. Brinkman ID, Butler AL, de Wit J, van Binnendijk RS, Alter G, van Baarle D. Measles vaccination elicits a polyfunctional antibody response, which decays more rapidly in early vaccinated children. J Infect Dis (2021) 225:1755–64. doi: 10.1093/infdis/jiab318
120. Crotty S. Follicular helper CD4 T cells (TFH). Annu Rev Immunol (2011) 29:621–63. doi: 10.1146/annurev-immunol-031210-101400
121. Vogelzang A, McGuire HM, Yu D, Sprent J, Mackay CR, King C. A fundamental role for interleukin-21 in the generation of T follicular helper cells. Immunity (2008) 29(1):127–37. doi: 10.1016/j.immuni.2008.06.001
122. Hale JS, Ahmed R. Memory T follicular helper CD4 T cells. Front Immunol (2015) 6:16. doi: 10.3389/fimmu.2015.00016
123. Debock I, Jaworski K, Chadlaoui H, Delbauve S, Passon N, Twyffels L, et al. Neonatal follicular Th cell responses are impaired and modulated by IL-4. J Immunol (2013) 191(3):1231–9. doi: 10.4049/jimmunol.1203288
124. Mastelic-Gavillet B, Vono M, Gonzalez-Dias P, Ferreira FM, Cardozo L, Lambert PH, et al. Neonatal T follicular helper cells are lodged in a pre-T follicular helper stage favoring innate over adaptive germinal center responses. Front Immunol (2019) 10:1845. doi: 10.3389/fimmu.2019.01845
125. Mastelic Gavillet B, Eberhardt CS, Auderset F, Castellino F, Seubert A, Tregoning JS, et al. MF59 mediates its b cell adjuvanticity by promoting T follicular helper cells and thus germinal center responses in adult and early life. J Immunol (2015) 194(10):4836–45. doi: 10.4049/jimmunol.1402071
126. Vono M, Eberhardt CS, Mohr E, Auderset F, Christensen D, Schmolke M, et al. Overcoming the neonatal limitations of inducing germinal centers through liposome-based adjuvants including c-type lectin agonists trehalose dibehenate or curdlan. Front Immunol (2018) 9:381. doi: 10.3389/fimmu.2018.00381
127. Koutsakos M, Wheatley AK, Loh L, Clemens EB, Sant S, Nussing S, et al. Circulating TFH cells, serological memory, and tissue compartmentalization shape human influenza-specific b cell immunity. Sci Transl Med (2018) 10(428):eaan8405. doi: 10.1126/scitranslmed.aan8405
128. Eto D, Lao C, DiToro D, Barnett B, Escobar TC, Kageyama R, et al. IL-21 and IL-6 are critical for different aspects of b cell immunity and redundantly induce optimal follicular helper CD4 T cell (Tfh) differentiation. PloS One (2011) 6(3):e17739. doi: 10.1371/journal.pone.0017739
129. Parrish-Novak J, Dillon SR, Nelson A, Hammond A, Sprecher C, Gross JA, et al. Interleukin 21 and its receptor are involved in NK cell expansion and regulation of lymphocyte function. Nature (2000) 408(6808):57–63. doi: 10.1038/35040504
130. Spolski R, Leonard WJ. Interleukin-21: basic biology and implications for cancer and autoimmunity. Annu Rev Immunol (2008) 26:57–79. doi: 10.1146/annurev.immunol.26.021607.090316
131. Doganci A, Birkholz J, Gehring S, Puhl AG, Zepp F, Meyer CU. In the presence of IL-21 human cord blood T cells differentiate to IL-10-producing Th1 but not Th17 or Th2 cells. Int Immunol (2013) 25(3):157–69. doi: 10.1093/intimm/dxs097
132. Morrisette-Thomas V, Cohen AA, Fulop T, Riesco E, Legault V, Li Q, et al. Inflamm-aging does not simply reflect increases in pro-inflammatory markers. Mech Ageing Dev (2014) 139:49–57. doi: 10.1016/j.mad.2014.06.005
133. Almanan M, Raynor J, Ogunsulire I, Malyshkina A, Mukherjee S, Hummel SA, et al. IL-10-producing tfh cells accumulate with age and link inflammation with age-related immune suppression. Sci Adv (2020) 6(31):eabb0806. doi: 10.1126/sciadv.abb0806
134. Kaur K, Chowdhury S, Greenspan NS, Schreiber JR. Decreased expression of tumor necrosis factor family receptors involved in humoral immune responses in preterm neonates. Blood (2007) 110(8):2948–54. doi: 10.1182/blood-2007-01-069245
135. Roco JA, Mesin L, Binder SC, Nefzger C, Gonzalez-Figueroa P, Canete PF, et al. Class-switch recombination occurs infrequently in germinal centers. Immunity (2019) 51(2):337–50.e7. doi: 10.1016/j.immuni.2019.07.001
136. Nielsen SCA, Roskin KM, Jackson KJL, Joshi SA, Nejad P, Lee JY, et al. Shaping of infant b cell receptor repertoires by environmental factors and infectious disease. Sci Transl Med (2019) 11(481):eaat2004. doi: 10.1126/scitranslmed.aat2004
137. Blanco E, Perez-Andres M, Arriba-Mendez S, Contreras-Sanfeliciano T, Criado I, Pelak O, et al. Age-associated distribution of normal b-cell and plasma cell subsets in peripheral blood. J Allergy Clin Immunol (2018) 141(6):2208–19 e16. doi: 10.1016/j.jaci.2018.02.017
138. Southern J, Crowley-Luke A, Borrow R, Andrews N, Miller E. Immunogenicity of one, two or three doses of a meningococcal c conjugate vaccine conjugated to tetanus toxoid, given as a three-dose primary vaccination course in UK infants at 2, 3 and 4 months of age with acellular pertussis-containing DTP/Hib vaccine. Vaccine (2006) 24(2):215–9. doi: 10.1016/j.vaccine.2005.07.060
139. Kelly DF, Snape MD, Perrett KP, Clutterbuck EA, Lewis S, Blanchard Rohner G, et al. Plasma and memory b-cell kinetics in infants following a primary schedule of CRM 197-conjugated serogroup c meningococcal polysaccharide vaccine. Immunology (2009) 127(1):134–43. doi: 10.1111/j.1365-2567.2008.02934.x
140. Mauri C, Bosma A. Immune regulatory function of b cells. Annu Rev Immunol (2012) 30:221–41. doi: 10.1146/annurev-immunol-020711-074934
141. Mauri C, Nistala K. Interleukin-35 takes the 'B' line. Nat Med (2014) 20(6):580–1. doi: 10.1038/nm.3594
142. Flores-Borja F, Bosma A, Ng D, Reddy V, Ehrenstein MR, Isenberg DA, et al. CD19+CD24hiCD38hi b cells maintain regulatory T cells while limiting TH1 and TH17 differentiation. Sci Transl Med (2013) 5(173):173ra23. doi: 10.1126/scitranslmed.3005407
143. Menon M, Blair PA, Isenberg DA, Mauri C. A regulatory feedback between plasmacytoid dendritic cells and regulatory b cells is aberrant in systemic lupus erythematosus. Immunity (2016) 44(3):683–97. doi: 10.1016/j.immuni.2016.02.012
144. Sun CM, Deriaud E, Leclerc C, Lo-Man R. Upon TLR9 signaling, CD5+ b cells control the IL-12-dependent Th1-priming capacity of neonatal DCs. Immunity (2005) 22(4):467–77. doi: 10.1016/j.immuni.2005.02.008
145. Walker WE, Goldstein DR. Neonatal b cells suppress innate toll-like receptor immune responses and modulate alloimmunity. J Immunol (2007) 179(3):1700–10. doi: 10.4049/jimmunol.179.3.1700
146. Esteve-Sole A, Teixido I, Deya-Martinez A, Yague J, Plaza-Martin AM, Juan M, et al. Characterization of the highly prevalent regulatory CD24(hi)CD38(hi) b-cell population in human cord blood. Front Immunol (2017) 8:201. doi: 10.3389/fimmu.2017.00201
147. Zhivaki D, Lemoine S, Lim A, Morva A, Vidalain PO, Schandene L, et al. Respiratory syncytial virus infects regulatory b cells in human neonates via chemokine receptor CX3CR1 and promotes lung disease severity. Immunity (2017) 46(2):301–14. doi: 10.1016/j.immuni.2017.01.010
148. Zhang X. Regulatory functions of innate-like b cells. Cell Mol Immunol (2013) 10(2):113–21. doi: 10.1038/cmi.2012.63
149. Zhang X, Deriaud E, Jiao X, Braun D, Leclerc C, Lo-Man R. Type I interferons protect neonates from acute inflammation through interleukin 10-producing b cells. J Exp Med (2007) 204(5):1107–18. doi: 10.1084/jem.20062013
150. Munoz FM, Jamieson DJ. Maternal immunization. Obstet Gynecol (2019) 133(4):739–53. doi: 10.1097/AOG.0000000000003161
151. Winkelstein JA, Marino MC, Lederman HM, Jones SM, Sullivan K, Burks AW, et al. X-Linked agammaglobulinemia: report on a united states registry of 201 patients. Med (Baltimore) (2006) 85(4):193–202. doi: 10.1097/01.md.0000229482.27398.ad
152. Ochola R, Sande C, Fegan G, Scott PD, Medley GF, Cane PA, et al. The level and duration of RSV-specific maternal IgG in infants in kilifi Kenya. PloS One (2009) 4(12):e8088. doi: 10.1371/journal.pone.0008088
153. Benowitz I, Esposito DB, Gracey KD, Shapiro ED, Vazquez M. Influenza vaccine given to pregnant women reduces hospitalization due to influenza in their infants. Clin Infect Dis (2010) 51(12):1355–61. doi: 10.1086/657309
154. Saso A, Kampmann B. Maternal immunization: Nature meets nurture. Front Microbiol (2020) 11:1499. doi: 10.3389/fmicb.2020.01499
155. Jones C, Pollock L, Barnett SM, Battersby A, Kampmann B. The relationship between concentration of specific antibody at birth and subsequent response to primary immunization. Vaccine (2014) 32(8):996–1002. doi: 10.1016/j.vaccine.2013.11.104
156. Maertens K, Tran TMP, Hens N, Van Damme P, Leuridan E. Effect of prepregnancy pertussis vaccination in young infants. J Infect Dis (2017) 215(12):1855–61. doi: 10.1093/infdis/jix176
157. Ladhani SN, Andrews NJ, Southern J, Jones CE, Amirthalingam G, Waight PA, et al. Antibody responses after primary immunization in infants born to women receiving a pertussis-containing vaccine during pregnancy: single arm observational study with a historical comparator. Clin Infect Dis (2015) 61(11):1637–44. doi: 10.1093/cid/civ695
158. Barug D, Pronk I, van Houten MA, Versteegh FGA, Knol MJ, van de Kassteele J, et al. Maternal pertussis vaccination and its effects on the immune response of infants aged up to 12 months in the Netherlands: an open-label, parallel, randomised controlled trial. Lancet Infect Dis (2019) 19(4):392–401. doi: 10.1016/S1473-3099(18)30717-5
159. Vono M, Eberhardt CS, Auderset F, Mastelic-Gavillet B, Lemeille S, Christensen D, et al. Maternal antibodies inhibit neonatal and infant responses to vaccination by shaping the early-life b cell repertoire within germinal centers. Cell Rep (2019) 28(7):1773–84 e5. doi: 10.1016/j.celrep.2019.07.047
160. Liston A, Carr EJ, Linterman MA. Shaping variation in the human immune system. Trends Immunol (2016) 37(10):637–46. doi: 10.1016/j.it.2016.08.002
161. Orru V, Steri M, Sole G, Sidore C, Virdis F, Dei M, et al. Genetic variants regulating immune cell levels in health and disease. Cell (2013) 155(1):242–56. doi: 10.1016/j.cell.2013.08.041
162. Brodin P, Jojic V, Gao T, Bhattacharya S, Angel CJ, Furman D, et al. Variation in the human immune system is largely driven by non-heritable influences. Cell (2015) 160(1-2):37–47. doi: 10.1016/j.cell.2014.12.020
163. Botafogo V, Perez-Andres M, Jara-Acevedo M, Barcena P, Grigore G, Hernandez-Delgado A, et al. Age distribution of multiple functionally relevant subsets of CD4+ T cells in human blood using a standardized and validated 14-color EuroFlow immune monitoring tube. Front Immunol (2020) 11:166. doi: 10.3389/fimmu.2020.00166
164. Sharma AA, Jen R, Butler A, Lavoie PM. The developing human preterm neonatal immune system: a case for more research in this area. Clin Immunol (2012) 145(1):61–8. doi: 10.1016/j.clim.2012.08.006
165. Reyman M, van Houten MA, van Baarle D, Bosch A, Man WH, Chu M, et al. Impact of delivery mode-associated gut microbiota dynamics on health in the first year of life. Nat Commun (2019) 10(1):4997. doi: 10.1038/s41467-019-13014-7
166. Strachan DP. Hay fever, hygiene, and household size. BMJ (1989) 299(6710):1259–60. doi: 10.1136/bmj.299.6710.1259
167. Guarner F, Bourdet-Sicard R, Brandtzaeg P, Gill HS, McGuirk P, van Eden W, et al. Mechanisms of disease: the hygiene hypothesis revisited. Nat Clin Pract Gastroenterol Hepatol (2006) 3(5):275–84. doi: 10.1038/ncpgasthep0471
168. Moulson AJ, Av-Gay Y. BCG Immunomodulation: From the 'hygiene hypothesis' to COVID-19. Immunobiology (2021) 226(1):152052. doi: 10.1016/j.imbio.2020.152052
169. Henmyr V, Carlberg D, Manderstedt E, Lind-Hallden C, Sall T, Cardell LO, et al. Genetic variation of the toll-like receptors in a Swedish allergic rhinitis case population. BMC Med Genet (2017) 18(1):18. doi: 10.1186/s12881-017-0379-6
170. Netea MG, Wijmenga C, O'Neill LA. Genetic variation in toll-like receptors and disease susceptibility. Nat Immunol (2012) 13(6):535–42. doi: 10.1038/ni.2284
Keywords: adaptive immune system, infants, vaccination, respiratory infectious diseases, heterogeneity, tolerance, immune memory, biomarker
Citation: Pieren DKJ, Boer MC and de Wit J (2022) The adaptive immune system in early life: The shift makes it count. Front. Immunol. 13:1031924. doi: 10.3389/fimmu.2022.1031924
Received: 30 August 2022; Accepted: 31 October 2022;
Published: 17 November 2022.
Edited by:
Kerry M. Empey, University of Pittsburgh, United StatesReviewed by:
Erguang Li, Nanjing University, ChinaSergej Tomić, Institute for the Application of Nuclear Energy (INEP), Serbia
Urban Švajger, Blood Transfusion Centre of Slovenia, Slovenia
Copyright © 2022 Pieren, Boer and de Wit. This is an open-access article distributed under the terms of the Creative Commons Attribution License (CC BY). The use, distribution or reproduction in other forums is permitted, provided the original author(s) and the copyright owner(s) are credited and that the original publication in this journal is cited, in accordance with accepted academic practice. No use, distribution or reproduction is permitted which does not comply with these terms.
*Correspondence: Jelle de Wit, amVsbGUuZGUud2l0QHJpdm0ubmw=
†Present address: Daan K. J. Pieren, Infectious Diseases Department, Vall d’Hebron Research Institute (VHIR), Vall d’Hebron Hospital Universitari, Barcelona, Spain