- 1The Second Clinical Medical College, Zhejiang Chinese Medical University, Hangzhou, China
- 2The First Clinical Medical College, Zhejiang Chinese Medical University, Hangzhou, China
- 3The Second Affiliated Hospital of Zhejiang Chinese Medical University, Hangzhou, China
Immune cells and immune molecules in the intestinal mucosa participate in innate and adaptive immunity to maintain local and systematic homeostasis. With aging, intestinal mucosal immune dysfunction will promote the emergence of age-associated diseases. Although there have been a number of studies on the impact of aging on systemic immunity, relatively fewer studies have been conducted on the impact of aging on the intestinal mucosal immune system. In this review, we will briefly introduce the impact of aging on the intestinal mucosal barrier, the impact of aging on intestinal immune cells as well as immune molecules, and the process of interaction between intestinal mucosal immunity and gut microbiota during aging. After that we will discuss potential strategies to slow down intestinal aging in the elderly.
1 Introduction
With aging, aging cells accumulate extensively, and utilize a senescence-associated secretory phenotype to secrete a variety of extracellular regulators (1). These abnormal accumulations of aging cells will lead to potentially deleterious effects, especially a dysfunctional immune system. The dysfunctional immune system in the elderly is associated with two processes – the decline of immune function, commonly termed the “ immunosenescence” process (2), and the dysregulation and hyperactivation of inflammation, leading to a persistent chronic low-grade inflammatory state in aging individuals, commonly termed the “inflammatory aging “ process (3). As the largest immune compartment, intestinal mucosal immune function is strongly influenced by aging, which will promote the emergence of diseases, including intestinal infections, intestinal tumors, malnutrition, chronic constipation, and other age-related diseases (4).
A critical function of the intestinal mucosa is to form a barrier that separates harmful antigens of the luminal content from the body. A monolayer of polarized intestinal epithelial cells (IECs) plays a physical isolation role and constitutes the last line of defense. Meanwhile, an apical junctional complex confers the property of selective permeability to the intestine (5). Apart from the last line of defense, the extracellular components of the barrier play an important role in maintaining the integrity of the intestine (6). For example, mucus, secreted by specialized epithelial cells, prevents large particles from directly contacting with IECs (5). Antimicrobial peptides (AMPs) have broad-spectrum and high-efficiency bactericidal activity (7). Additionally, gut microbiota influences the balance between pro-inflammatory and regulatory responses. Certain members of the microbiota colonizing the intestine stimulate the production of microbicidal peptides and secretory immunoglobulin A (sIgA) and are endowed with anti-inflammatory properties. Others promote effector immune responses and are endowed with pro-inflammatory properties (8).
The gut-associated lymphoid tissue along with immune molecules are the major participants of both innate and adaptive immune responses. The gut-associated lymphoid tissue mainly includes immune cells, either interspersed between epithelial cells or present in the lamina propria, scattered or grouped in lymphoid follicles, either isolated or aggregated to form Peyers’patches in the ileum (9). Intestinal innate immune system includes intrinsic lymphocytes, macrophages, dendritic cells (DC), eosinophils, IEC, etc. (10). Cells from the immune system spectrum along with IEC are capable of using pattern recognition receptors(PRR) to recognize highly conserved pathogen-associated molecular patterns. Compared with adaptive immune system, it provides an acute nonspecific immune defense. Moreover, the PRR family links the innate and adaptive immune system by producing cytokines and costimulatory molecules (11). By contrast, the intestinal adaptive immune system utilizes highly mutated receptors that recognize specific microbial antigens for the activation and differentiation of T cells. Then, naive T cells can differentiate into either effector T cells to fight pathogens and toxins or regulatory T (Treg) cells to tolerate presence of specific antigens. In addition, B cells and their antibodies (mainly IgA) constitute the other half of adaptive immune response. B cells produce high-affinity, monoreactive antibodies primarily against pathogens and toxins through a T cell-dependent pathway. By contrary, they produce low-affinity, polyreactive antibodies primarily against commensal microbiota through a T cell-independent mechanism (12).
Aging impairs the intestinal mucosal barrier, which brings about the intestinal mucosal immune dysfunction. Also, aging adversely affects the function of intestinal mucosal immune system – protective immune response and induction of immune tolerance. Moreover, the gut microbiota in the elderly is closely associated with the aging intestinal mucosal immune system. Understanding the age-related process of intestinal mucosal immune dysfunction may help to slow down intestinal aging in the elderly, and further help to reduce the risk of age-associated diseases.
2 The impact of aging on the intestinal mucosal barrier
2.1 The impact of aging on intestinal stem cells
Intestinal stem cells (ISCs) located at the base of the intestinal crypt differentiate to produce all cell lineages of the intestinal epithelium, including enterocytes, goblet cells, Paneth cells, enteroendocrine cells, and Tuft cells. ISCs complete the renewal of the intestinal epithelium and are essential for maintaining the intestinal mucosal barrier (13).
Aging affects the external structure of crypt and the number of total cells in crypt. In small intestine of aging mice, researchers observed a decrease in the number of crypts, an increase in crypt length and width, an increase in the number of cells in per crypt and an increase in villus length (14). In colon of aging human, researchers observed increased percentage of Ki67 positive cells and decreased number of cell divisions (15). Ki67 is a widely-used proliferation marker, whose effect on cell proliferation varies by cell type (16). This suggests that increased Ki67 index in aging crypt is associated with decreased cell divisions. In addition, further study found that the expression of the cell cycle regulator CDKN1C in aging crypt is reduced, at the same time, terminal deoxynucleotidyl transferase dUTP nick end labeling (TUNEL) positive cells are increased (14). Consequently, the mechanism for the reduced cell proliferation may be associated with the reduced cell division and the reduced survival of aging cells. Cell proliferation within crypts is the principal driving force for cell migration along the villus (17). So the impact of aging on the mitotic process of crypt cells is likely to reduce the rate of IEC migration along the villi.
Aging affects the capacity of ISCs in crypt. Researchers have assessed the number of ISCs during aging in different subjects using different ISC markers and derived different results. Researchers studied on aging human using the well-established ISC marker – Olfm4, and found an increase in the number of ISCs (15). A study on Drosophila found similar results – aging ISCs are activated in response to tissue damage or infection and then become highly proliferative (18). However, researchers studied on aging mice using a variety of markers specific to ISC, including Lgr5 and Olfm4, and found no significant change (14). Overall, these results at least suggest that ISC number do not decrease with aging. Thus, although the nature of mitotic cells in the crypts has not been clarified currently, the above results suggest that the cells with reduced mitosis in the crypts are not dominated by ISC.
Aging affects signaling within the ISCs. First, the Wnt pathway is closely associated with stem cell maintenance and differentiation in the intestinal epithelium. Aging impairs the regenerative and self-renewal capacity of ISCs, which is closely associated with a decline in the canonical Wnt signaling (19, 20). On the one hand, with aging, the expression of canonical Wnt is reduced, including the expression of Wnt3 in Paneth cells, mesenchymal cells and ISCs. On the other hand, with aging, the expression of target genes of canonical Wnt signaling and genes regulating ISC function, including β-catenin, Ascl2, and Lgr5, in ISCs or crypt is decreased as well (21). The mechanism of the decreased Wnt signaling is associated with aging Paneth cells. Increased mTORC1 activity in aging Paneth cells alters the expression of Notum through the mTORC1-PPAR-α axis (Figure 1). Notum is a secreted Wnt deacylase that disengages Wnt ligands from receptors on ISCs and reduces Wnt activity during development. Hence the increased expression of Notum can impair function of aging IECs (22). Moreover, the reduced function of ISC may be related to the activity of Cdc42. The increased activity of Cdc42 in aging crypt and ISC is associated with the reduced expression of Ascl2(achaete scute like 2) (23). Ascl2 is a β-catenin-dependent transcription factor that controls ISC function, so the reduced expression of Ascl2 is closely associated with the aging of ISCs (14). Second, three major signaling pathways, Wnt, Notch, and MAPK, determine the spectrum of ISC differentiation (24). Several reports have suggested changes in the composition of IECs during aging, which will be analyzed in detail below. In addition, the stress response signaling pathway to tissue injury or infection is involved in the heterotypic proliferation of ISC. During aging, ISCs exhibit up-regulated JNK signaling as well as up-regulated platelet-derived growth factor (PDGF)/vascular endothelial growth factor (VEGF) signaling, and normal Nrf2 signaling is interrupted (25, 26). As a result, ISCs are hyperactivated, highly proliferative and eventually heterogeneous. This seems to explain the increased incidence of intestinal tumors in the elderly.
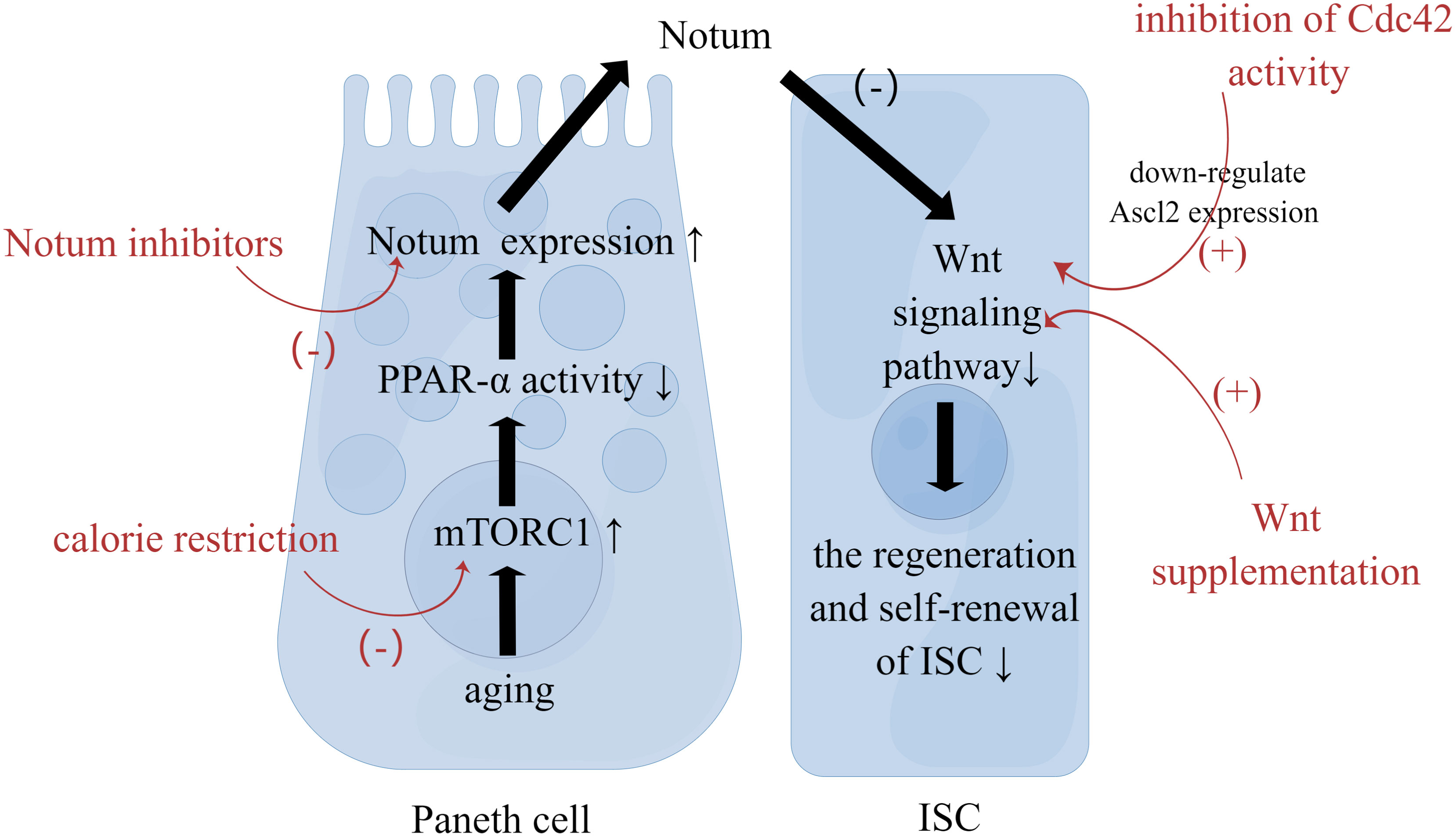
Figure 1 Strategies to Restore the Function of ISC. Paneth cells reside in proximity to ISCs. With aging, increased mTORC1 activity in Paneth cells alters the expression of Notum. Then Notum can diminish Wnt activity in ISC to impair function of aging IEC. Calorie restriction, Wnt supplementation, Notum inhibitors, and inhibition of Cdc42 activity can restore ISC function through the mechanism mentioned above.
2.2 The impact of aging on IECs
Several reports showed that the composition of IEC changed with aging for the reason that aging affects the differentiation of ISCs. Of note, many investigators have reported increased number of secretory cell lineages in aging individuals, including Paneth cells, goblet cells, enteroendocrine cells (14, 27, 28). Some of them speculated that it may be related to persistent damage to the epithelium (28), while others suggested that this alteration may be driven by changes in spectrum of ISC differentiation due to signaling pathways (14). Notch pathway is one of possible signaling pathways. Notch signaling affects ISC differentiation by regulating helix-loop-helix transcription factor Atonal homolog 1 (ATOH1). It was found that Notch1, one of the Notch receptors, and the Atoh1 gene exhibit reduced expression in aging ISCs. ATOH1 can regulate lateral inhibition through Delta-like notch ligand and secretory lineage genes (14). Paradoxically, distinct age-related changes in the secretory cell lineage have also been reported by investigators. Investigators have successively observed a decreased number of Paneth cells in aging mice and humans (29, 30). It was also found that no significant changes in enteroendocrine cells with aging (31). As for goblet cells, a decrease in the number of goblet cells has been observed at the end of the ileum and in the colon (31–33). These changes may be due to the decreased function of ISCs and increased apoptosis of IECs. Overall, more experimental data are needed to clarify the impact of aging on IEC composition, especially in different regions.
The entry of antigens from the intestine into host tissue is restricted by the IECs. M cells, as the specialized epithelial cells, can sample luminal antigens across the IECs (34). M cells develop from ISCs and their differentiation requires NF-κB receptor activator ligand/NF-κB receptor activator signaling pathway activation, or RANKL/RANK signaling pathway activation as it is often called. Also, their differentiation requires chemokine CCL20-mediated chemical attraction (35). Their maturation is mediated by the expression of E26-transformation-specific-family transcription factor Spi-B. And, glycoprotein 2 (GP2), as one of the target genes of Spi-B, has an extremely important role in the maturation of M cells (36, 37). Aging affects the differentiation and maturation of M cells. First, the overall size of follicle-associated epithelium, density of M cells in follicle-associated epithelium, expression of CCL20, and M cell-specific expressed genes are significantly reduced in Peyer’s patches. Additionally, the expression of RANKL and RANK as well as their signaling are not altered. These results suggest that the mechanisms of the impact of aging on M cell differentiation are related to actors downstream of RANKL-RANK signaling and changes in the chemokine CCL20. Second, the number of Spi-B+ cells, Spi-B expression, and the number of GP2+ cells are also significantly reduced (38, 39), suggesting that aging affects M cell maturation due to the changes in the expression of Spi-B and its target genes. Moreover, the density of mature M cells in follicle-associated epithelium of Spi-B-deficient mice and CCR6-deficient mice is significantly reduced (40, 41), resulting in reduced antigen-specific mucosal immune responses (42). It supports the above view further. In conclusion, aging adversely affects the differentiation and maturation of M cells, hindering the process of sampling and uptaking luminal antigens.
Aging affects the function of Paneth cells. On the one hand, Paneth cells are the main source of AMPs, which include defensin peptides, lysozyme C, phospholipases, C-type lectins (43). Aging affects the production of AMPs. It was shown that the expression of α-defensin (α-defensin) and lysozyme in aging mouse Paneth cells is significantly reduced, suggesting that Paneth cell dysfunction affects AMP expression. Paradoxically, this experiment found that the expression of C-type lectins Reg3b and Reg3g, β-defensin 1, and Relmb, was significantly increased at the same time. The researchers reckoned that the change of the latter may be related to age-associated inflammation and persistent damage to the aging intestinal epithelium (28). On the other hand, Paneth cells produce multiple signaling factors, including EGF, TGF-a, Wnt3, Delta-like ligands and cyclic ADP ribose (cADPR), to maintain ISC capacity and regulate ISC function (44). It was found that with aging, Paneth cells could not support the function of young ISCs as well as before. Moreover, co-culture of aging ISCs with young Paneth cells could partially recover from age-related ISC dysfunction (22). This suggests that, in addition to Notum expression, aging Paneth cells can also regulate ISC capacity and function through some signaling factors. Notably, researchers observed no change in the expression of Wnt3 or EGF in aging Paneth cells, while the expression of cADPR was reduced (22). More experiments are needed to clarify the effects of other signaling factors.
2.3 The impact of aging on apical junctional complex
The apical junctional complex includes tight junctions (TJs) and adherens junctions (21). TJ proteins are multiprotein complexes composed of transmembrane proteins (including claudins, ocludins, TAMPs, and JAMs), scaffolding proteins (including ZO-1/2/3 and cingulin), and regulatory molecules (45). The TJ is the principal determinant of mucosal permeability. The primary function of TJs is to strictly regulate the passage of ions through small pores that show size and charge selectivity, and macromolecules through leak pathways that do not show charge selectivity (5). Recently, numerous researchers have assessed changes in intestinal physical barrier on human, nonhuman primates, and rodents during aging. They obtained roughly the same results – the permeability of aging intestine increases, which is associated with changes in the expression of key TJ proteins (21, 34, 35, 46). Nevertheless, there are slight differences in details in these researches above. One is that there are different results about the impact on macromolecular permeability: the intestinal permeability of aging rodents and non-human primates to macromolecules increases, while aging human’s increased intestinal permeability is limited to solutes rather. In other words, aging human’s intestinal permeability to macromolecular is not affected by aging. It suggests that human’s increased intestinal permeability is not due to disturbances to the overall morphology of the TJ. The other is that the key TJ proteins affected by aging of different species are completely different: reduced expression of JAM, ZO-1 and ocludin is observed in the intestine of aging rodents and non-human primates, while no changes about the expression of the above proteins is observed in the intestine of aging human. Only increased expression of claudin-2 is observed in human. Claudin protein, as one type of TJ proteins, can be divided into sealing proteins that reduce permeability and pore-forming proteins that increase permeability. Claudin-2 belongs to the latter (36), so the increased expression of claudin-2 in the aging human intestine can increase the intestinal permeability to pathogens and toxins, which is associated with the chronic low-grade inflammatory state and higher risk of intestinal infection in the elderly. In conclusion, the intestinal permeability of human is less affected by aging than other animals. It seems that the overall morphology of the TJ suffers less for the reason that TJ protein expression changes less.
2.4 The impact of aging on extracellular components
As mentioned above, aging affects the production of AMP. In addition, aging affects the thickness and function of mucus. Goblet cells are the main source of mucus, which includes mucins (major components), immune mediators that regulate the intestinal microbiome, and other molecules with unknown function (37). Aging affects the thickness and function of mucus. First, aging affects the thickness of the mucus layer. In aging mice, the thickness of the mucus layer in colon is reduced (32, 33, 47). Supplementation of different gut microbiota may prevent or exacerbate such changes in aging mice, suggesting that aging may affect mucus through the gut microbiota (47). In aging human, the mucus layer thickness of stomach and duodenum in H. pylori-positive individuals is reduced, yet aging does not seem to affect H. pylori-negative individuals’ (48). The intestinal mucus plays an important role in protecting the epithelial surface from pathogens. Overall, these changes may imply a loss of mucus protection. Second, aging affects the adhesion of mucus to the gut microbiota. The adhesion of mucus to the gut microbiota, considered as a precondition for the initial colonization and subsequent proliferation of the gut microbiome, is associated with the glycan-rich structural domain of mucins (30). Unfortunately, some researchers found that the mucosal adhesion to Bifidobacterium decreases with aging in human (49, 50), which well explains the reduced abundance of Bifidobacterium in the gut microbiota of the elderly. The exact mechanism is not clear. It may be due to the changes in chemical structure of mucin, but there is no evidence that the chemical structure of mucin is impaired during aging. Bifidobacterium, as probiotics in the intestine, play an important role in intestinal homeostasis and health (51). Therefore, the reduced abundance of Bifidobacterium in the gut microbiome of the elderly is universally considered as an undesirable change that adversely affects intestinal mucosal immune function.
3 The impact of aging on the intestinal immune system
3.1 The impact of aging on immune cells
3.1.1 The impact of aging on DC
DCs induce a balance of regulatory and effector T cell responses and play a critical role in intestinal immune regulation. The DC-induced balance of T cells is finely regulated by immune mediators (52). At steady state, intestinal DCs can induce regulatory T cells (Treg) to tolerate presence of soluble antigens and commensal microbiota (53). TGF-β, IL-10, Retinoic Acid and other immune mediators are required for the differentiation process to Treg (54). For example, the exposure of Retinoic Acid can lead to the upregulation of CD141 and GARP on DCs, to enhance the ability for inducing Treg. It was found that aging DCs in human show an impaired response to Retinoic Acid, resulting in reduced upregulation of CD141 and GARP on DCs and defects in the induction of Treg (55). In a word, aging affects the process of introducing Treg for tolerance of antigens and increases the susceptibility to diseases ultimately. However, upon pathogen stimulation, antigen-presenting cells recognize antigens through pattern recognition receptors and transfer them to gut-associated lymphoid tissue. Then, intestinal pathogens and their metabolites can provide immunostimulatory signals to DCs (56). Hence, previous balance is altered and DCs induce a series of effector T cells, providing antigen-specific protective immune responses against pathogens and toxins (52). During this process, CTLA-4 expression in DCs regulates CD80/86 costimulatory molecules to activate immune responses (57). In aging mouse, DCs produce less IL-12p70 and IL-15 and express less CD80/CD86 costimulatory signals, resulting in reduced antigen-specific T-cell responses (58). In contrast, providing DCs from young mice to T cells originally from older animals can introduce optimal expression of CD80 and CD86 costimulatory molecules, restoring their original ability to introduce antigen-specific T-cell responses (59). This also proves that aging affects the process of inducing effector T cells for antigen-specific protective immune responses, and enhances inflammation ultimately.
3.1.2 The impact of aging on T cells
Aging diminishes the immune function of T cells, which adversely affects mucosal immune function (60). First, aging affects the proliferative response of CD4+ T cells in Peyer’s patches. The underlying mechanisms may be that aging altered CD4+ T cell responsiveness to T cell growth factors and decreased cytokine synthesis by CD4+ T cells (61). For example, IL-4, as one of B cell stimulation and growth factors, is secreted less by CD4+ T cells in aging nasal mucosa (62). Second, aging affects the expression of immunomodulatory molecules in LP CD4+ T cells. PD-1 and CTLA-4, as the inhibitory moleculules, show reduced expression. Also, the expression of Ki67 is decreased, which is associated with steady-state proliferation and renewal of cells (63, 64). The inhibitory molecules CTLA-4 and PD-1 can inhibit T cell activation and T cell responses through different mechanisms (65, 66). Therefore, age-associated low CTLA-4 and PD-1 expression in LP CD4+ T cells may be associated with CD4+ T cell hyperactivation as well as mucosal inflammatory environment in aging individuals. However, Ki67 is a widely-used proliferation marker, whose effect on cell proliferation varies by cell type (16). Also, Ki67 expression is generally low in LP CD4+ T cells from young individuals as well. So, it is difficult to determine the significance of further reduction of Ki67 in aging LP CD4+ T cells (63, 64). In addition, aging reduces the frequency and function of LP CD4 Th17 cell, which is important to antigen-specific immune responses and mucosal barrier integrity. LP CD4 Th1 and Th17 cells produce IFN-γ (produced mainly by Th1) and IL-17, which are critical cytokines driving immune responses against pathogenic microorganisms (67). Also LP CD4 Th1 and Th17 cells produce IL-22 (produced mainly by Th22 but also by Th1 and Th17 cells) which acts on epithelial cells to maintain the integrity of the epithelial barrier (68). It was found that the capability of Th17 and Th1 cells from the elderly is defective, including responding to gut microbiota and the proliferation after exposure to antigens (63). Considering that the absence of intestinal Th17 cells is associated with epithelial barrier damage and microbial translocation (69, 70), it is likely that defects in Th17 and Th1 cells in the elderly play an important role in the aging of the gut mucosal immune system.
3.2 The impact of aging on cytokine production
During aging, cytokine expression patterns are remodeled and gradually tend to a pro-inflammatory phenotype, which is referred to as “inflammatory aging” (67).
Several studies have evaluated the secretion of anti-inflammatory and pro-inflammatory cytokines in the circulation during aging. Several evidences suggested that the age-related elevations in the circulation of the pro-inflammatory cytokines IL-1, IL-2, IL-6, IL-18, and TNF-α as well as anti-inflammatory TGF-β, which are associated with various age-related diseases (67). The dysregulation of pro-inflammatory and anti-inflammatory cytokines in the circulation is undoubtedly directly related to systemic inflammation and systemic immunity. Moreover, recent studies have suggested that this dysregulation is also closely linked to intestinal mucosal immune function. Fist, the age-related elevations in the circulation of TNF, IL-2, IL-6 and IL-8 directly affect the gut microbiome in aging individuals. The link between cytokines and gut microbiome may be related to potential pathways associated with amino acid (68). In addition, the age-related elevations in the circulation of IL-6, TNF-α, IL-1β and IL-12 appear to be associated with increased intestinal permeability (69).
Currently, many studies on mice, rats, and baboons have evaluated the secretion of cytokines in the intestinal mucosa during aging. These studies have found that the secretion of pro-inflammatory cytokines IL-6, TNF-α, IFN-γ, IL-1β increases, the secretion of anti-inflammatory cytokines TGF-β, IL-4 decreases, and the secretion of IL-10 shows opposite results in rats and mice (58, 69, 70). In the intestinal mucosal immune system, cytokines are involved in the regulation of intestinal mucosal immune function. First, aging affect production of cytokines from IECs, some of which can remodel TJ proteins. It impairs the integrity of TJs, which could be a potential mechanism for increased permeability. For example, IL-1β can increase TJ permeability through activation of the standard NF-kB pathway, activation of MLCK gene and post-transcriptional degradation of ocludin mRNA (71). And, IL-6 can increase TJ permeability by upregulating claudin-2 expression. The underlying mechanism may be that IL-6 induces claudin-2 gene expression through activating the JNK signaling pathway to upregulate the expression of transcription factor AP-1 or through the MEK/ERK and PI3K pathways to upregulate the expression of transcription factor Cdx2 (72, 73). Also, TNF-α can increase TJ permeability by upregulating claudin-2 expression and downregulating occludin expression. The underlying mechanism may be that TNF-α upregulates MLCK expression, promotes MLC phosphorylation levels, activates hypoxia-inducible factor-1α, NF-κB as well as PI3K/Akt (74), and diminishes occludin promoter activity (75). In addition, IFN-γ can increase TJ permeability by upregulating claudin-2 expression and downregulating occludin expression, which may be associated with serine protease (76). So, serine protease inhibitor could be a potential strategy to slow down intestinal aging. And IFN-γ can decrease ocludin promoter activity as well (77). Second, age-related cytokines affect the regulation of epithelial cell dynamics. For example, TNF-α can inhibit cell proliferation, inhibit cell migration and induce apoptosis to interfere with the inherent recovery potential of IECs (78). Also TNF-α can synergize with IFN-γ to induce caspase-8-JAK1/2-STAT1-dependent death of IECs (79). Finally, age-related cytokines influence the regulation of the immune response. Cytokines, as the key modulators of immunity, participate in nearly all aspects of immunity. Cells from the immune system spectrum and IEC secrete pro-inflammatory cytokines linking innate and adaptive immune responses. And thereafter, these cytokines play a decisive role in the activation, differentiation, maturation and function of the immune cells (Table 1).
3.3 The impact of aging on immunoglobulins
On the mucosal surface, secretory immunoglobulin M (sIgM) and sIgA play a significant role in promoting mucosal tolerance and shaping the microbiome (94).
There are fewer studies on sIgM. An older study showed that sIgM secretion in the intestinal mucosa appears to not correlate with aging (95). Compared to specific sIgA secretion upon antigen stimulation, nonspecific sIgA secretion in intestinal mucosa seems to be a poor indicator of mucosal immunity function (77). Considering this, more researchers focus on specific sIgA secretion. A couple of studies showed reduced antigen-specific sIgA secretion in the intestinal mucosa (77, 96, 97), whose mechanism involves the immune cells and immune molecules during sIgA production (Figure 2). sIgA serves as the first line of defense in the intestinal mucosa, the reduced secretion of which will do harm to antigen-specific immune responses.
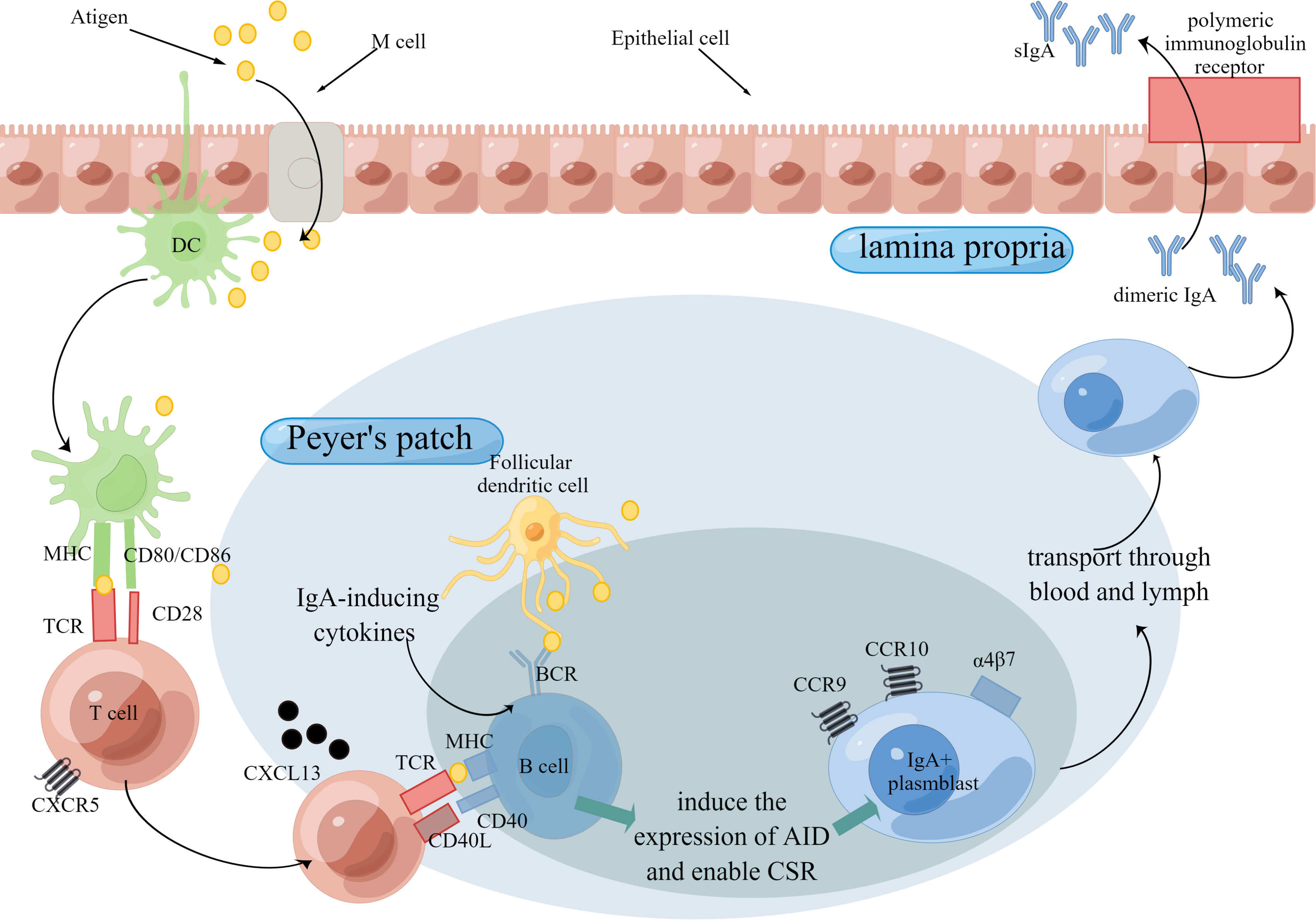
Figure 2 Production of IgA Through T cell-dependent Pathway. The intestinal mucosal immune function is mainly organized by the induction sites and effector sites. Peyer’s patch is the major induction site of immune responses. Antigens are delivered to Peyer’s patch by M cells and then may be captured and presented by DCs. In T cell-dependent pathway, B cells is stimulated by T cells, which release IgA-inducing cytokines, and then express CD40 ligand (CD40L) and cytokines that induce the expression of activation-induced cytidine deaminase (AID) and thereby enable class-switch recombination (CSR). After that, IgA+ plasmblast is transported to effector sites, such as lamina propria. Aging is detrimental to the production of IgA for the reason that aging adversely influences the number or/and function of immune cells as well as immune molecules. Abbreviations: TCR, T cell receptors; BCR, B cell receptors.
4 Interaction between gut microbiota and mucosal immunity during aging
4.1 The impact of microbiome in the aging gut on mucosal immunity
With aging, the age-related changes of the intestinal microbial composition, called microbial dysbiosis, are mainly characterized in the elderly. The abundance of core commensal bacteria decreases, while the abundance of opportunistic and pathogenic microorganisms increases (98). This change implies that the aging intestine loses protection from commensal bacteria and at the same time the growth and colonization of pathogens increase. It can impair the intestinal mucosal barrier by directly damaging the TJ or modulating signaling pathways related to the TJ (99), leading to leakage of pathogens, metabolites of pathogens, and pro-inflammatory cytokines. Further, it increases the risk of intestinal infections, triggers chronic intestinal inflammation, and exacerbates the process of aging (100, 101). There have been some researches on the underlying mechanisms of the impact of specific microorganisms. For example, Strains Lactobacillus reuteri 5454 can induce Tregs and Reg3b expression. Bifidobacterium animalis spp. lactis 5764 can promote the maturation of DCs and the secretion of IL-17A (102). And Bifidobacterium lactis Bi-07 can enhance phagocytic activity of monocytes and granulocytes (103). Also, Bifidobacterium lactis HN019 can enhance the function of natural killer cells and polymorphonuclear cells (104).
In addition, the intestinal microbiota can affect the intestinal mucosal immune function through its metabolism. With aging, the potential of saccharolytic decreases, the ability of the colon to utilize lactate decreases, the catabolism of creatine increases, and the production of cobalamin, biotin as well as short chain fatty acids (SCFAs) decreases. Also, the bile acid pool composition is affected (105, 106). These microbial metabolites play a key role in regulating immune responses. For instance, SCFAs can inhibit NF-kB, stimulate IL-8 secretion (107), induce Treg differentiation (108), enhance regulatory B cell differentiation, and inhibit differentiation of plasma cells (109). Consequently, SCFAs have an anti-inflammatory effect and the reduced SCFAs can increase the intestinal inflammation. Bile acids can induce monocyte differentiation to IL-12 hypo-producing DCs and inhibit the production of pro-inflammatory cytokines and chemokines in monocytes and macrophages (110, 111). Furthermore, high fecal lactate is associated with ulcerative colitis and other inflammatory bowel diseases (112). In conclusion, the intestinal microbiota, through changes in its composition and metabolism, can influence the immune function of the intestinal mucosa.
4.2 The impact of mucosal “immunosenescence” on intestinal microbiome
The mucosal immune system shapes the intestinal microbiome by allowing commensal bacteria to occupy mucosal ecological niches, while selectively eliminating or neutralizing harmful microorganisms. The mucosal “immunosenescence” can directly affect intestinal recognition and processing of gut microbiota (113, 114). Also, it promotes intestinal microbial dysbiosis by other mechanisms. For example, the responses of sIgA to Clostridiaceae and Enterobacteriaceae decrease with aging (115). Additionally, the increased intestinal inflammation in aging individuals can promote microbial dysbiosis. For instance, in the aging TNF-deficient mice, protected from age-associated inflammation, researchers did not observe age-related microbiome changes (116).
5 Strategies to slow down intestinal aging
“immunosenescence”, state of immune dysfunction, results in an increased susceptibility to infections, reduced abilities to heal, and altered homeostasis (2). As the largest immune compartment, intestinal mucosal immune function is strongly influenced by aging.
Aging impairs the intestinal mucosal barrier, which brings about the intestinal mucosal immune dysfunction. First, aging affects the number of ISCs, which is related to changes of crypt. Also, aging adversely influences the regeneration and self-renewal of intestinal epithelium. Second, aging affects the differentiation of ISCs. That is to say, aging affects the composition of IECs. Further, aging the function of IECs. Also, aging increases intestinal permeability by changing the expression of key TJ proteins, resulting in the impairment in the physical barrier. At last, Aging affects extracellular components of intestinal mucosal barrier, including the production and function of mucus and AMP. Understanding the impact of aging on the intestinal mucosal barrier will help explore strategies to slow down intestinal aging. Low caloric states can enhance ISC function during aging by inducing fatty acid oxidation (31). And calorie restriction can inhibit mTORC1 to promote epithelial regeneration (117). Additionally, Wnt supplementation, Notum inhibitors, and inhibition of Cdc42 activity can restore ISC function as well (Figure 1). Blocking pro-inflammatory cytokines associated with increased permeability is one of the strategies. For instance, Nicotinamide mononucleotide supplementation can downregulate the TNF-α expression and upregulates the ocludin as well as claudin-1 expression (118).
Aging can affect the intestinal mucosal innate and adaptive immune function through immune cells and immune molecules. On the one hand, aging affects intestinal mucosal immune function through immune cells. First, aging affects the ability of DCs to induce a balance of regulatory and effector T cell response through the impaired response to Retinoic Acid, the reduced cytokine secretion, and the costimulatory signal expression. It is detrimental to antigen-specific immunity and the tolerance of intestinal harmless antigen. In addition, aging diminishes the immune function of T cells. Aging affects the proliferative response of PP CD4+ T cells, which is disadvantageous to antigen-specific immunity. Aging affects the expression of immunomodulatory molecules in LP CD4+ T cells, which may be associated with CD4+ T cells hyperactivation as well as mucosal inflammatory environment. Aging affects the frequency and function of Th17 cell, which is disadvantageous to antigen-specific immunity and mucosal barrier integrity. Understanding the impact of aging on immune cells can help explore strategies targeting immune cells to slow down intestinal aging. Here are some examples. Exogenous addition of IL-15 can enhance the antigen-presenting ability of DCs by upregulating the costimulatory molecules CD80 and CD86, which introduce effector T cells to recognize antigens and promote pathogen-specific immune responses (59). Increasing the response of CD4+ T-cell to cytokines, such as adipose-derived mesenchymal stem cell transplantation, can improve the response of CD4+ T cells to cytokine and increase the antigen-specific sIgA (119). And Exogenous addition of cytokines synthesized by aging CD4+ T cells, such as IL-4, IL-5, and IL-2, may improve immune function (120, 121). In addition, it is found that CTLA-4 and PD-1 blockers widely used for cancer treatment could lead to unintended immune-mediated intestinal inflammation (122). And anti-CTLA-4 treatment confers the anti-inflammatory property to the colitis model mouse by inducing IL-10-producing Tregs (123). However, there is a lack of studies on intestinal aging. So the effect of CTLA-4 and PD-1 blockers to intestinal aging remains unknown. On the other hand, aging affects intestinal mucosal immune function through immune molecules. Aging remodels cytokine expression patterns, tending to a pro-inflammatory phenotype of the intestinal and systemic immune system. These cytokines play important roles in nearly all aspects of immunity. So, the age-related changes of cytokines will cause adverse effects on intestinal mucosal immune function. Recombinant cytokines and cytokine blockers are potential strategies to slow down intestinal aging. Although studies on aging are lacking, the widespread use of cytokines in IBD suggests that they are very promising strategies (124). Additionally, aging affects the secretion of antigen-specific IgA in the intestine, which does harm to intestinal adaptive immunity. Targeting cells and chemokines, involved with the production and transport processes of antigen-specific sIgA, can promote pathogen-specific immune function.
At last, the microbiome in the aging gut and the aging mucosal immune system are closely related. The altered composition and metabolism of the gut microbiome adversely affect the intestinal mucosal immunity. Conversely, the aging mucosal immune system directly and also indirectly affects the composition of the gut microbiota. Therefore, modulation of gut microbiota is an important strategy to slow down intestinal aging, including dietary interventions, exercise interventions, drugs and bacterial therapies (125).
Author contributions
SZ devised the study. HZ, CZ, and QW were involved in the conception of the study and critically revised the manuscript. SF and YF were involved in writing the article. All authors agree to be accountable for the content of the work.
Funding
This work was supported by the National Natural Science Foundation of China (82074214). Funding was also provided by the Research Fund Project of Zhejiang Chinese Medical University (2019ZY02).
Conflict of interest
The authors declare that the research was conducted in the absence of any commercial or financial relationships that could be construed as a potential conflict of interest.
Publisher’s note
All claims expressed in this article are solely those of the authors and do not necessarily represent those of their affiliated organizations, or those of the publisher, the editors and the reviewers. Any product that may be evaluated in this article, or claim that may be made by its manufacturer, is not guaranteed or endorsed by the publisher.
References
1. Malaquin N, Martinez A, Rodier F. Keeping the senescence secretome under control: Molecular reins on the senescence-associated secretory phenotype. Exp Gerontol (2016) 82:39–49. doi: 10.1016/j.exger.2016.05.010
2. Borgoni S, Kudryashova KS, Burka K, de Magalhaes JP. Targeting immune dysfunction in aging. Ageing Res Rev (2021) 70:101410. doi: 10.1016/j.arr.2021.101410
3. Chen Y, Liu S, Leng SX. Chronic low-grade inflammatory phenotype (Clip) and senescent immune dysregulation. Clin Ther (2019) 41(3):400–9. doi: 10.1016/j.clinthera.2019.02.001
4. Dumic I, Nordin T, Jecmenica M, Stojkovic Lalosevic M, Milosavljevic T, Milovanovic T. Gastrointestinal tract disorders in older age. Can J Gastroenterol Hepatol (2019) 2019:6757524. doi: 10.1155/2019/6757524
5. Turner JR. Intestinal mucosal barrier function in health and disease. Nat Rev Immunol (2009) 9(11):799–809. doi: 10.1038/nri2653
6. Ohman L, Tornblom H, Simren M. Crosstalk at the mucosal border: Importance of the gut microenvironment in ibs. Nat Rev Gastroenterol Hepatol (2015) 12(1):36–49. doi: 10.1038/nrgastro.2014.200
7. An J, Liu Y, Wang Y, Fan R, Hu X, Zhang F, et al. The role of intestinal mucosal barrier in autoimmune disease: A potential target. Front Immunol (2022) 13:871713. doi: 10.3389/fimmu.2022.871713
8. Cerf-Bensussan N, Gaboriau-Routhiau V. The immune system and the gut microbiota: Friends or foes? Nat Rev Immunol (2010) 10(10):735–44. doi: 10.1038/nri2850
9. Mörbe UM, Jørgensen PB, Fenton TM, von Burg N, Riis LB, Spencer J, et al. Human gut-associated lymphoid tissues (Galt); diversity, structure, and function. Mucosal Immunol (2021) 14(4):793–802. doi: 10.1038/s41385-021-00389-4
10. Shemtov SJ, Emani R, Bielska O, Covarrubias AJ, Verdin E, Andersen JK, et al. The intestinal immune system and gut barrier function in obesity and ageing. FEBS J (2022). doi: 10.1111/febs.16558
11. Yuan Q, Walker WA. Innate immunity of the gut: Mucosal defense in health and disease. J Pediatr Gastroenterol Nutr (2004) 38(5):463–73. doi: 10.1097/00005176-200405000-00001
12. Zhao Q, Elson CO. Adaptive immune education by gut microbiota antigens. Immunology (2018) 154(1):28–37. doi: 10.1111/imm.12896
13. Zhao Q, Guan J, Wang X. Intestinal stem cells and intestinal organoids. J Genet Genomics (2020) 47(6):289–99. doi: 10.1016/j.jgg.2020.06.005
14. Nalapareddy K, Nattamai KJ, Kumar RS, Karns R, Wikenheiser-Brokamp KA, Sampson LL, et al. Canonical wnt signaling ameliorates aging of intestinal stem cells. Cell Rep (2017) 18(11):2608–21. doi: 10.1016/j.celrep.2017.02.056
15. Mitchell EM, Parris A, Bigwood L, Scobioala-laker N, Reynolds A, Lewis MP, et al. Compromised tissue renewal in the ageing human colonic epithelium. Gastroenterology (2011) 5(140):S–321. doi: 10.1016/s0016-5085(11)61298-5
16. Miller I, Min M, Yang C, Tian C, Gookin S, Carter D, et al. Ki67 is a graded rather than a binary marker of proliferation versus quiescence. Cell Rep (2018) 24(5):1105–12. doi: 10.1016/j.celrep.2018.06.110
17. Parker A, Maclaren OJ, Fletcher AG, Muraro D, Kreuzaler PA, Byrne HM, et al. Cell proliferation within small intestinal crypts is the principal driving force for cell migration on villi. FASEB J (2016) 31(2):636–49. doi: 10.1096/fj.201601002
18. Chandel NS, Jasper H, Ho TT, Passegué E. Metabolic regulation of stem cell function in tissue homeostasis and organismal ageing. Nat Cell Biol (2016) 18(8):823–32. doi: 10.1038/ncb3385
19. Rodriguez-Fernandez IA, Tauc HM, Jasper H. Hallmarks of aging drosophila intestinal stem cells. Mech Ageing Dev (2020) 190:111285. doi: 10.1016/j.mad.2020.111285
20. Nalapareddy K, Zheng Y, Geiger H. Aging of intestinal stem cells. Stem Cell Rep (2022) 17(4):734–40. doi: 10.1016/j.stemcr.2022.02.003
21. Mabbott NA. A breakdown in communication? understanding the effects of aging on the human small intestine epithelium. Clin Sci (Lond) (2015) 129(7):529–31. doi: 10.1042/CS20150364
22. Pentinmikko N, Iqbal S, Mana M, Andersson S, Cognetta AB, 3rd, Suciu RM, et al. Notum produced by paneth cells attenuates regeneration of aged intestinal epithelium. Nature (2019) 571(7765):398–402. doi: 10.1038/s41586-019-1383-0
23. Nalapareddy K, Hassan A, Sampson LL, Zheng Y, Geiger H. Suppression of elevated Cdc42 activity promotes the regenerative potential of aged intestinal stem cells. iScience (2021) 24(4):102362. doi: 10.1016/j.isci.2021.102362
24. Gehart H, Clevers H. Tales from the crypt: New insights into intestinal stem cells. Nat Rev Gastroenterol Hepatol (2019) 16(1):19–34. doi: 10.1038/s41575-018-0081-y
25. Biteau B, Hochmuth CE, Jasper H. Jnk activity in somatic stem cells causes loss of tissue homeostasis in the aging drosophila gut. Cell Stem Cell (2008) 3(4):442–55. doi: 10.1016/j.stem.2008.07.024
26. Choi NH, Kim JG, Yang DJ, Kim YS, Yoo MA. Age-related changes in drosophila midgut are associated with Pvf2, a Pdgf/Vegf-like growth factor. Aging Cell (2008) 7(3):318–34. doi: 10.1111/j.1474-9726.2008.00380.x
27. Igarashi M, Miura M, Williams E, Jaksch F, Kadowaki T, Yamauchi T, et al. Nad+ supplementation rejuvenates aged gut adult stem cells. Aging Cell (2019) 18(3):e12935. doi: 10.1111/acel.12935
28. Tremblay S, Cote NML, Grenier G, Duclos-Lasnier G, Fortier LC, Ilangumaran S, et al. Ileal antimicrobial peptide expression is dysregulated in old age. Immun Ageing (2017) 14:19. doi: 10.1186/s12979-017-0101-8
29. Valenkevich IN, Zhukova NM. The structure of the mucous membrane of the human duodenum with aging. Arkhiv patologii (1976) 38(3):58–61.
30. Juge N. Microbial adhesins to gastrointestinal mucus. Trends Microbiol (2012) 20(1):30–9. doi: 10.1016/j.tim.2011.10.001
31. Mihaylova MM, Cheng C-W, Cao AQ, Tripathi S, Mana MD, Bauer-Rowe KE, et al. Fasting activates fatty acid oxidation to enhance intestinal stem cell function during homeostasis and aging. Cell Stem Cell (2018) 22(5):769–78.e4. doi: 10.1016/j.stem.2018.04.001
32. Sovran B, Hugenholtz F, Elderman M, Van Beek AA, Graversen K, Huijskes M, et al. Age-associated impairment of the mucus barrier function is associated with profound changes in microbiota and immunity. Sci Rep (2019) 9(1):1437. doi: 10.1038/s41598-018-35228-3
33. Elderman M, Sovran B, Hugenholtz F, Graversen K, Huijskes M, Houtsma E, et al. The effect of age on the intestinal mucus thickness, microbiota composition and immunity in relation to sex in mice. PloS One (2017) 12(9):e0184274. doi: 10.1371/journal.pone.0184274
34. Tran L, Greenwood-Van Meerveld B. Age-associated remodeling of the intestinal epithelial barrier. J Gerontol A Biol Sci Med Sci (2013) 68(9):1045–56. doi: 10.1093/gerona/glt106
35. Man AL, Bertelli E, Rentini S, Regoli M, Briars G, Marini M, et al. Age-associated modifications of intestinal permeability and innate immunity in human small intestine. Clin Sci (Lond) (2015) 129(7):515–27. doi: 10.1042/CS20150046
36. Angelow S, Ahlstrom R, Yu AS. Biology of claudins. Am J Physiol Renal Physiol (2008) 295(4):F867–76. doi: 10.1152/ajprenal.90264.2008
37. Johansson ME, Hansson GC. Immunological aspects of intestinal mucus and mucins. Nat Rev Immunol (2016) 16(10):639–49. doi: 10.1038/nri.2016.88
38. Kobayashi A, Donaldson DS, Erridge C, Kanaya T, Williams IR, Ohno H, et al. The functional maturation of m cells is dramatically reduced in the peyer's patches of aged mice. Mucosal Immunol (2013) 6(5):1027–37. doi: 10.1038/mi.2012.141
39. Donaldson DS, Shih BB, Mabbott NA. Aging-related impairments to m cells in peyer's patches coincide with disturbances to paneth cells. Front Immunol (2021) 12:761949. doi: 10.3389/fimmu.2021.761949
40. Kanaya T, Hase K, Takahashi D, Fukuda S, Hoshino K, Sasaki I, et al. The ets transcription factor spi-b is essential for the differentiation of intestinal microfold cells. Nat Immunol (2012) 13(8):729–36. doi: 10.1038/ni.2352
41. Cook DN, Prosser DM, Forster R, Zhang J, Kuklin NA, Abbondanzo SJ, et al. Ccr6 mediates dendritic cell localization, lymphocyte homeostasis, and immune responses in mucosal tissue. Immunity (2000) 12(5):495–503. doi: 10.1016/s1074-7613(00)80201-0
42. Ebisawa M, Hase K, Takahashi D, Kitamura H, Knoop KA, Williams IR, et al. Ccr6hicd11c(Int) b cells promote m-cell differentiation in peyer's patch. Int Immunol (2011) 23(4):261–9. doi: 10.1093/intimm/dxq478
43. Bevins CL, Salzman NH. Paneth cells, antimicrobial peptides and maintenance of intestinal homeostasis. Nat Rev Microbiol (2011) 9(5):356–68. doi: 10.1038/nrmicro2546
44. Sato T, van Es JH, Snippert HJ, Stange DE, Vries RG, van den Born M, et al. Paneth cells constitute the niche for Lgr5 stem cells in intestinal crypts. Nature (2011) 469(7330):415–8. doi: 10.1038/nature09637
45. Tsukita S, Tanaka H, Tamura A. The claudins: From tight junctions to biological systems. Trends Biochem Sci (2019) 44(2):141–52. doi: 10.1016/j.tibs.2018.09.008
46. Ren WY, Wu KF, Li X, Luo M, Liu HC, Zhang SC, et al. Age-related changes in small intestinal mucosa epithelium architecture and epithelial tight junction in rat models. Aging Clin Exp Res (2014) 26(2):183–91. doi: 10.1007/s40520-013-0148-0
47. van Beek AA, Sovran B, Hugenholtz F, Meijer B, Hoogerland JA, Mihailova V, et al. Supplementation with lactobacillus plantarum Wcfs1 prevents decline of mucus barrier in colon of accelerated aging Ercc1(-/Delta7) mice. Front Immunol (2016) 7:408. doi: 10.3389/fimmu.2016.00408
48. Newton JL, Jordan N, Pearson J, Williams GV, Allen A, James OF. The adherent gastric antral and duodenal mucus gel layer thins with advancing age in subjects infected with helicobacter pylori. Gerontology (2000) 46(3):153–7. doi: 10.1159/000022151
49. Ouwehand AC, Isolauri E, Kirjavainen PV, Salminen SJ. Adhesion of four bifidobacterium strains to human intestinal mucus from subjects in different age groups. FEMS Microbiol Lett (1999) 172(1):61–4. doi: 10.1111/j.1574-6968.1999.tb13450.x
50. He F, Ouwehand AC, Isolauri E, Hosoda M, Benno Y, Salminen S. Differences in composition and mucosal adhesion of bifidobacteria isolated from healthy adults and healthy seniors. Curr Microbiol (2001) 43(5):351–4. doi: 10.1007/s002840010315
51. Arboleya S, Watkins C, Stanton C, Ross RP. Gut bifidobacteria populations in human health and aging. Front Microbiol (2016) 7:1204. doi: 10.3389/fmicb.2016.01204
52. Stagg AJ. Intestinal dendritic cells in health and gut inflammation. Front Immunol (2018) 9:2883. doi: 10.3389/fimmu.2018.02883
53. Coombes JL, Powrie F. Dendritic cells in intestinal immune regulation. Nat Rev Immunol (2008) 8(6):435–46. doi: 10.1038/nri2335
54. Coombes JL, Siddiqui KRR, Arancibia-Cárcamo CV, Hall J, Sun C-M, Belkaid Y, et al. A functionally specialized population of mucosal Cd103+ dcs induces Foxp3+ regulatory T cells via a tgf-beta and retinoic acid-dependent mechanism. J Exp Med (2007) 204(8):1757–64. doi: 10.1084/jem.20070590
55. Agrawal S, Ganguly S, Tran A, Sundaram P, Agrawal A. Retinoic acid treated human dendritic cells induce T regulatory cells via the expression of Cd141 and garp which is impaired with age. Aging-US (2016) 8(6):1223. doi: 10.18632/aging.100973
56. Omenetti S, Pizarro TT. The Treg/Th17 axis: A dynamic balance regulated by the gut microbiome. Front Immunol (2016) 6:639. doi: 10.3389/fimmu.2015.00639
57. Kornete M, Piccirillo CA. Functional crosstalk between dendritic cells and Foxp3(+) regulatory T cells in the maintenance of immune tolerance. Front Immunol (2012) 3:165. doi: 10.3389/fimmu.2012.00165
58. Santiago AF, Alves AC, Oliveira RP, Fernandes RM, Paula-Silva J, Assis FA, et al. Aging correlates with reduction in regulatory-type cytokines and T cells in the gut mucosa. Immunobiology (2011) 216(10):1085–93. doi: 10.1016/j.imbio.2011.05.007
59. Moretto MM, Lawlor EM, Khan IA. Aging mice exhibit a functional defect in mucosal dendritic cell response against an intracellular pathogen. J Immunol (2008) 181(11):7977–84. doi: 10.4049/jimmunol.181.11.7977
60. Koga T, McGhee JR, Kato H, Kato R, Kiyono H, Fujihashi K. Evidence for early aging in the mucosal immune system. J Immunol (2000) 165(9):5352–9. doi: 10.4049/jimmunol.165.9.5352
61. Hagiwara Y, McGhee JR, Fujihashi K, Kobayashi R, Yoshino N, Kataoka K, et al. Protective mucosal immunity in aging is associated with functional Cd4+ T cells in nasopharyngeal-associated lymphoreticular tissue. J Immunol (2003) 170(4):1754–62. doi: 10.4049/jimmunol.170.4.1754
62. Xiong N, Hu S. Regulation of intestinal iga responses. Cell Mol Life Sci (2015) 72(14):2645–55. doi: 10.1007/s00018-015-1892-4
63. Dillon SM, Liu J, Purba CM, Christians AJ, Kibbie JJ, Castleman MJ, et al. Age-related alterations in human gut Cd4 T cell phenotype, T helper cell frequencies, and functional responses to enteric bacteria. J Leukoc Biol (2020) 107(1):119–32. doi: 10.1002/JLB.5A0919-177RR
64. Dillon SM, Thompson TA, Christians AJ, McCarter MD, Wilson CC. Reduced immune-regulatory molecule expression on human colonic memory Cd4 T cells in older adults. Immun Ageing (2021) 18(1):6. doi: 10.1186/s12979-021-00217-0
65. Fuertes Marraco SA, Neubert NJ, Verdeil G, Speiser DE. Inhibitory receptors beyond T cell exhaustion. Front Immunol (2015) 6:310. doi: 10.3389/fimmu.2015.00310
66. Parry RV, Chemnitz JM, Frauwirth KA, Lanfranco AR, Braunstein I, Kobayashi SV, et al. Ctla-4 and pd-1 receptors inhibit T-cell activation by distinct mechanisms. Mol Cell Biol (2005) 25(21):9543–53. doi: 10.1128/mcb.25.21.9543-9553.2005
67. Rea IM, Gibson DS, McGilligan V, McNerlan SE, Alexander HD, Ross OA. Age and age-related diseases: Role of inflammation triggers and cytokines. Front Immunol (2018) 9:586. doi: 10.3389/fimmu.2018.00586
68. Gou W, Fu Y, Yue L, Chen G-D, Cai X, Shuai M, et al. Gut microbiota, inflammation, and molecular signatures of host response to infection. J Genet Genomics (2021) 48(9):792–802. doi: 10.1016/j.jgg.2021.04.002
69. Walker EM, Slisarenko N, Gerrets GL, Kissinger PJ, Didier ES, Kuroda MJ, et al. Inflammaging phenotype in rhesus macaques is associated with a decline in epithelial barrier-protective functions and increased pro-inflammatory function in Cd161-expressing cells. GeroScience (2019) 41(6):739–57. doi: 10.1007/s11357-019-00099-7
70. Xu H-J, Hao X-L, Qiao D-L, Xia L-B, Chen R, He X-M, et al. Effects of dendrobium huoshanense polysaccharides on antioxidant capacity, mucosal barrier integrity and inflammatory responses in an aging rat ileal model. Biotechnol Biotechnol Equip (2019) 33(1):1444–52. doi: 10.1080/13102818.2019.1674187
71. Kaminsky LW, Al-Sadi R, Ma TY. Il-1beta and the intestinal epithelial tight junction barrier. Front Immunol (2021) 12:767456. doi: 10.3389/fimmu.2021.767456
72. Van Itallie CM, Holmes J, Bridges A, Gookin JL, Coccaro MR, Proctor W, et al. The density of small tight junction pores varies among cell types and is increased by expression of claudin-2. J Cell Sci (2008) 121(Pt 3):298–305. doi: 10.1242/jcs.021485
73. Suzuki T, Yoshinaga N, Tanabe S. Interleukin-6 (Il-6) regulates claudin-2 expression and tight junction permeability in intestinal epithelium. J Biol Chem (2011) 286(36):31263–71. doi: 10.1074/jbc.M111.238147
74. Amasheh M, Fromm A, Krug SM, Amasheh S, Andres S, Zeitz M, et al. Tnfalpha-induced and berberine-antagonized tight junction barrier impairment Via tyrosine kinase, akt and nfkappab signaling. J Cell Sci (2010) 123(Pt 23):4145–55. doi: 10.1242/jcs.070896
75. Tavalali S, Schmitz H, Fromm M, Schulzke J-D, Mankertz J. Expression from the human occludin promoter is affected by tumor necrosis factor alpha and interferon gamma. Gastroenterology (2000) 4(118):A602. doi: 10.1016/s0016-5085(00)84547-3
76. Willemsen LE, Hoetjes JP, van Deventer SJ, van Tol EA. Abrogation of ifn-gamma mediated epithelial barrier disruption by serine protease inhibition. Clin Exp Immunol (2005) 142(2):275–84. doi: 10.1111/j.1365-2249.2005.02906.x
77. Schmucker DL, Thoreux K, Owen RL. Aging impairs intestinal immunity. Mech Ageing Dev (2001) 122(13):1397–411. doi: 10.1016/s0047-6374(01)00276-7
78. Waseem T, Duxbury M, Ito H, Rocha F, Lautz D, Whang E, et al. Ghrelin ameliorates tnf-a induced anti-proliferative and pro-apoptotic effects and promotes intestinal epithelial restitution. J Am Coll Surgeons (2004) 199(3):16. doi: 10.1016/j.jamcollsurg.2004.05.018
79. Woznicki JA, Saini N, Flood P, Rajaram S, Lee CM, Stamou P, et al. Tnf-alpha synergises with ifn-gamma to induce caspase-8-Jak1/2-Stat1-Dependent death of intestinal epithelial cells. Cell Death Dis (2021) 12(10):864. doi: 10.1038/s41419-021-04151-3
80. Guo Y, Wang B, Wang T, Gao L, Yang ZJ, Wang FF, et al. Biological characteristics of il-6 and related intestinal diseases. Int J Biol Sci (2021) 17(1):204–19. doi: 10.7150/ijbs.51362
81. Drastich P, Frolova-Brizova L, Zanvit P, Spicak J, Tlaskalova-Hogenova H. Spontaneous in vitro il-6 production in various intestinal segments in patients with inflammatory bowel disease. Folia Microbiol (2011) 56(3):185–90. doi: 10.1007/s12223-011-0018-0
82. Mitoma H, Horiuchi T, Tsukamoto H, Ueda N. Molecular mechanisms of action of anti-Tnf-A agents – comparison among therapeutic tnf-A antagonists. Cytokine (2018) 101:56–63. doi: 10.1016/j.cyto.2016.08.014
83. Leppkes M, Roulis M, Neurath MF, Kollias G, Becker C. Pleiotropic functions of tnf-alpha in the regulation of the intestinal epithelial response to inflammation. Int Immunol (2014) 26(9):509–15. doi: 10.1093/intimm/dxu051
84. Ye L, Schnepf D, Staeheli P. Interferon-lambda orchestrates innate and adaptive mucosal immune responses. Nat Rev Immunol (2019) 19(10):614–25. doi: 10.1038/s41577-019-0182-z
85. Broggi A, Tan Y, Granucci F, Zanoni I. Ifn-Λ suppresses intestinal inflammation by non-translational regulation of neutrophil function. Nat Immunol (2017) 18(10):1084–93. doi: 10.1038/ni.3821
86. Eriguchi Y, Nakamura K, Yokoi Y, Sugimoto R, Takahashi S, Hashimoto D, et al. Essential role of ifn-Γ in T cell-associated intestinal inflammation. JCI Insight (2018) 3(18). doi: 10.1172/jci.insight.121886
87. Ihara S, Hirata Y, Koike K. Tgf-beta in inflammatory bowel disease: A key regulator of immune cells, epithelium, and the intestinal microbiota. J Gastroenterol (2017) 52(7):777–87. doi: 10.1007/s00535-017-1350-1
88. Zhang N, Bevan MJ. Transforming growth factor-B signaling controls the formation and maintenance of gut-resident memory T cells by regulating migration and retention. Immunity (2013) 39(4):687–96. doi: 10.1016/j.immuni.2013.08.019
89. Monteleone G, Stolfi C, Marafini I, Atreya R, Neurath MF. Smad7 antisense oligonucleotide-based therapy in crohn's disease: Is it time to re-evaluate? Mol Diag Ther (2022) 26(5):477–81. doi: 10.1007/s40291-022-00606-1
90. Urban JF, Fayer R, Sullivan C, Goldhill J, Shea-Donohue T, Madden K, et al. Local Th1 and Th2 responses to parasitic infection in the intestine: Regulation by ifn-gamma and il-4. Veterinary Immunol Immunopathol (1996) 54(1–4):337–44. doi: 10.1016/s0165-2427(96)05708-x
91. Tu L, Chen J, Zhang H, Duan L. Interleukin-4 inhibits regulatory T cell differentiation through regulating Cd103+ dendritic cells. Front Immunol (2017) 8:214. doi: 10.3389/fimmu.2017.00214
92. Mantovani A, Marchesi F. Il-10 and macrophages orchestrate gut homeostasis. Immunity (2014) 40(5):637–9. doi: 10.1016/j.immuni.2014.04.015
93. Wang X, Wong K, Ouyang W, Rutz S. Targeting il-10 family cytokines for the treatment of human diseases. Cold Spring Harbor Perspect Biol (2019) 11(2):a028548. doi: 10.1101/cshperspect.a028548
94. Turula H, Wobus CE. The role of the polymeric immunoglobulin receptor and secretory immunoglobulins during mucosal infection and immunity. Viruses (2018) 10(5):237. doi: 10.3390/v10050237
95. Senda S, Cheng E, Kawanishi H. Aging-associated changes in murine intestinal immunoglobulin a and m secretions. Scandinavian J Immunol (1988) 27(2):157–64. doi: 10.1111/j.1365-3083.1988.tb02334.x
96. Fulton JR, Cuff CF. Mucosal and systemic immunity to intestinal reovirus infection in aged mice. Exp Gerontol (2004) 39(9):1285–94. doi: 10.1016/j.exger.2004.06.013
97. Thoreux K, Owen RL, Schmucker DL. Intestinal lymphocyte number, migration and antibody secretion in young and old rats. Immunology (2000) 101(1):161–7. doi: 10.1046/j.1365-2567.2000.00095.x
98. Conway J, Duggal NA. Ageing of the gut microbiome: Potential influences on immune senescence and inflammageing. Ageing Res Rev (2021) 68:101323. doi: 10.1016/j.arr.2021.101323
99. Paradis T, Begue H, Basmaciyan L, Dalle F, Bon F. Tight junctions as a key for pathogens invasion in intestinal epithelial cells. Int J Mol Sci (2021) 22(5):2506. doi: 10.3390/ijms22052506
100. Fransen F, van Beek AA, Borghuis T, Aidy SE, Hugenholtz F, van der Gaast-de Jongh C, et al. Aged gut microbiota contributes to systemical inflammaging after transfer to germ-free mice. Front Immunol (2017) 8:1385. doi: 10.3389/fimmu.2017.01385
101. Thevaranjan N, Puchta A, Schulz C, Naidoo A, Szamosi JC, Verschoor CP, et al. Age-associated microbial dysbiosis promotes intestinal permeability, systemic inflammation, and macrophage dysfunction. Cell Host Microbe (2017) 21(4):455–66 e4. doi: 10.1016/j.chom.2017.03.002
102. Hrdy J, Alard J, Couturier-Maillard A, Boulard O, Boutillier D, Delacre M, et al. Lactobacillus reuteri 5454 and bifidobacterium animalis ssp. lactis 5764 improve colitis while differentially impacting dendritic cells maturation and antimicrobial responses. Sci Rep (2020) 10(1):5345. doi: 10.1038/s41598-020-62161-1
103. Maneerat S, Lehtinen MJ, Childs CE, Forssten SD, Alhoniemi E, Tiphaine M, et al. Consumption of bifidobacterium lactis bi-07 by healthy elderly adults enhances phagocytic activity of monocytes and granulocytes. J Nutr Sci (2013) 2:e44. doi: 10.1017/jns.2013.31
104. Miller L, Lehtoranta L, Lehtinen M. The effect of bifidobacterium animalis ssp. lactis Hn019 on cellular immune function in healthy elderly subjects: Systematic review and meta-analysis. Nutrients (2017) 9(3):191. doi: 10.3390/nu9030191
105. Langille MG, Meehan CJ, Koenig JE, Dhanani AS, Rose RA, Howlett SE, et al. Microbial shifts in the aging mouse gut. Microbiome (2014) 2(1):1–12. doi: 10.1186/s40168-014-0050-9
106. Vemuri R, Gundamaraju R, Shastri MD, Shukla SD, Kalpurath K, Ball M, et al. Gut microbial changes, interactions, and their implications on human lifecycle: An ageing perspective. BioMed Res Int (2018) 2018:4178607. doi: 10.1155/2018/4178607
107. Asarat M, Vasiljevic T, Apostolopoulos V, Donkor O. Short-chain fatty acids regulate secretion of il-8 from human intestinal epithelial cell lines in vitro. Immunol Invest (2015) 44(7):678–93. doi: 10.3109/08820139.2015.1085389
108. Furusawa Y, Obata Y, Fukuda S, Endo TA, Nakato G, Takahashi D, et al. Commensal microbe-derived butyrate induces the differentiation of colonic regulatory T cells. Nature (2013) 504(7480):446–50. doi: 10.1038/nature12721
109. Rosser EC, Piper CJM, Matei DE, Blair PA, Rendeiro AF, Orford M, et al. Microbiota-derived metabolites suppress arthritis by amplifying aryl-hydrocarbon receptor activation in regulatory b cells. Cell Metab (2020) 31(4):837–51 e10. doi: 10.1016/j.cmet.2020.03.003
110. Gadaleta RM, van Erpecum KJ, Oldenburg B, Willemsen EC, Renooij W, Murzilli S, et al. Farnesoid X receptor activation inhibits inflammation and preserves the intestinal barrier in inflammatory bowel disease. Gut (2011) 60(4):463–72. doi: 10.1136/gut.2010.212159
111. Ichikawa R, Takayama T, Yoneno K, Kamada N, Kitazume MT, Higuchi H, et al. Bile acids induce monocyte differentiation toward interleukin-12 hypo-producing dendritic cells Via a Tgr5-dependent pathway. Immunology (2012) 136(2):153–62. doi: 10.1111/j.1365-2567.2012.03554.x
112. Vernia P, Caprilli R, Latella G, Barbetti F, Magliocca FM, Cittadini M. Fecal lactate and ulcerative colitis. Gastroenterology (1988) 95(6):1564–8. doi: 10.1016/s0016-5085(88)80078-7
113. Zhang CX, Wang HY, Chen TX. Interactions between intestinal Microflora/Probiotics and the immune system. BioMed Res Int (2019) 2019:6764919. doi: 10.1155/2019/6764919
114. Yoo JY, Groer M, Dutra SVO, Sarkar A, Mcskimming DI. Gut microbiota and immune system interactions. Microorganisms (2020) 8(10):1587. doi: 10.3390/microorganisms8101587
115. Sugahara H, Okai S, Odamaki T, Wong CB, Kato K, Mitsuyama E, et al. Decreased taxon-specific iga response in relation to the changes of gut microbiota composition in the elderly. Front Microbiol (2017) 8:1757. doi: 10.3389/fmicb.2017.01757
116. Thevaranjan N, Puchta A, Schulz C, Naidoo A, Szamosi JC, Verschoor CP, et al. Age-associated microbial dysbiosis promotes intestinal permeability, systemic inflammation, and macrophage dysfunction. Cell Host Microbe (2018) 21(4):455–66. doi: 10.1016/j.chom.2018.03.006
117. Yousefi M, Nakauka-Ddamba A, Berry CT, Li N, Schoenberger J, Simeonov KP, et al. Calorie restriction governs intestinal epithelial regeneration through cell-autonomous regulation of Mtorc1 in reserve stem cells. Stem Cell Rep (2018) 10(3):703–11. doi: 10.1016/j.stemcr.2018.01.026
118. Ru M, Wang W, Zhai Z, Wang R, Li Y, Liang J, et al. Nicotinamide mononucleotide supplementation protects the intestinal function in aging mice and d-galactose induced senescent cells. Food Funct (2022) 13(14):7507–19. doi: 10.1039/d2fo00525e
119. Boyaka PN, Aso K, Tsuruhara A, Takagaki K, Oki K, Ota M, et al. Adipose-derived mesenchymal stem cells restore impaired mucosal immune responses in aged mice. PloS One (2016) 11(2):e0148185. doi: 10.1371/journal.pone.0148185
120. Ajitsu S, Mirabella S, Kawanishi H. In vivo immunologic intervention in age-related T cell defects in murine gut-associated lymphoid tissues by Il2. Mech Ageing Dev (1990) 54(2):163–83. doi: 10.1016/0047-6374(90)90063-l
121. Roberts AI, O'Connell SM, Biancone L, Brolin RE, Ebert EC. Spontaneous cytotoxicity of intestinal intraepithelial lymphocytes: Clues to the mechanism. Clin Exp Immunol (1993) 94(3):527–32. doi: 10.1111/j.1365-2249.1993.tb08229.x
122. De Velasco G, Je Y, Bossé D, Awad MM, Ott PA, Moreira RB, et al. Comprehensive meta-analysis of key immune-related adverse events from ctla-4 and pd-1/Pd-L1 inhibitors in cancer patients. Cancer Immunol Res (2017) 5(4):312–8. doi: 10.1158/2326-6066.cir-16-0237
123. Coquerelle C, Oldenhove G, Acolty V, Denoeud J, Vansanten G, Verdebout JM, et al. Anti-Ctla-4 treatment induces il-10-Producing icos+ regulatory T cells displaying ido-dependent anti-inflammatory properties in a mouse model of colitis. Gut (2009) 58(10):1363–73. doi: 10.1136/gut.2008.162842
124. Yamamoto-Furusho JK. Inflammatory bowel disease therapy: Blockade of cytokines and cytokine signaling pathways. Curr Opin Gastroenterol (2018) 34(4):187–93. doi: 10.1097/MOG.0000000000000444
Keywords: aging, intestinal mucosal immune, mucosal barrier, cytokine, gut microbiota
Citation: Zheng H, Zhang C, Wang Q, Feng S, Fang Y and Zhang S (2022) The impact of aging on intestinal mucosal immune function and clinical applications. Front. Immunol. 13:1029948. doi: 10.3389/fimmu.2022.1029948
Received: 28 August 2022; Accepted: 09 November 2022;
Published: 28 November 2022.
Edited by:
Javier Ochoa-Repáraz, Boise State University, United StatesReviewed by:
Anne Jarry, Institut National de la Santé et de la Recherche Médicale (INSERM), FranceClaudio Nicoletti, University of Florence, Italy
Copyright © 2022 Zheng, Zhang, Wang, Feng, Fang and Zhang. This is an open-access article distributed under the terms of the Creative Commons Attribution License (CC BY). The use, distribution or reproduction in other forums is permitted, provided the original author(s) and the copyright owner(s) are credited and that the original publication in this journal is cited, in accordance with accepted academic practice. No use, distribution or reproduction is permitted which does not comply with these terms.
*Correspondence: Shuo Zhang, emhhbmdzaHVvdGNtQDE2My5jb20=
†These authors have contributed equally to this work and share first authorship