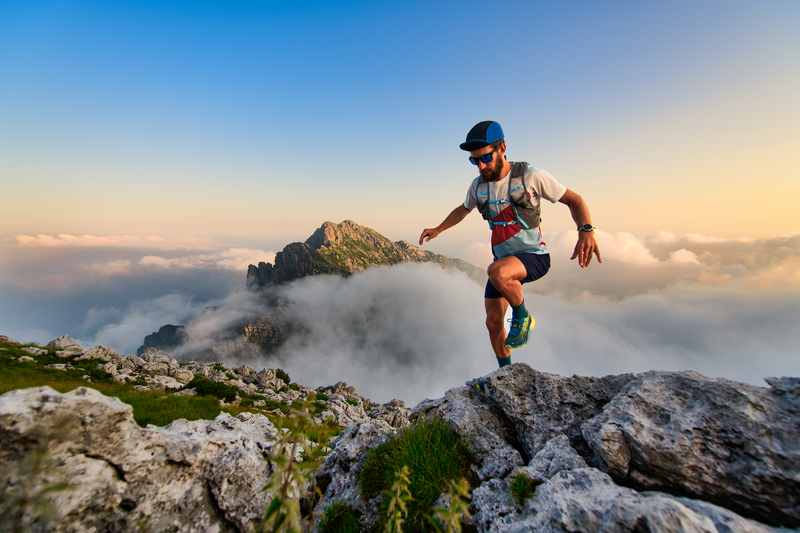
95% of researchers rate our articles as excellent or good
Learn more about the work of our research integrity team to safeguard the quality of each article we publish.
Find out more
REVIEW article
Front. Immunol. , 28 October 2022
Sec. Cancer Immunity and Immunotherapy
Volume 13 - 2022 | https://doi.org/10.3389/fimmu.2022.1018962
This article is part of the Research Topic Adoptive Cellular Therapies in Immunoregulation and Cancer View all 31 articles
Tumor-infiltrating lymphocytes (TILs), frontline soldiers of the adaptive immune system, are recruited into the tumor site to fight against tumors. However, their small number and reduced activity limit their ability to overcome the tumor. Enhancement of TILs number and activity against tumors has been of interest for a long time. A lack of knowledge about the tumor microenvironment (TME) has limited success in primary TIL therapies. Although the advent of engineered T cells has revolutionized the immunotherapy methods of hematologic cancers, the heterogeneity of solid tumors warrants the application of TILs with a wide range of specificity. Recent advances in understanding TME, immune exhaustion, and immune checkpoints have paved the way for TIL therapy regimens. Nowadays, TIL therapy has regained attention as a safe personalized immunotherapy, and currently, several clinical trials are evaluating the efficacy of TIL therapy in patients who have failed conventional immunotherapies. Gaining favorable outcomes following TIL therapy of patients with metastatic melanoma, cervical cancer, ovarian cancer, and breast cancer has raised hope in patients with refractory solid tumors, too. Nevertheless, TIL therapy procedures face several challenges, such as high cost, timely expansion, and technical challenges in selecting and activating the cells. Herein, we reviewed the recent advances in the TIL therapy of solid tumors and discussed the challenges and perspectives.
Tumor-infiltrating lymphocytes (TILs) are naturally-occurring mononuclear cells infiltrating the solid tumor microenvironment (TME), which might be referred all immune cells at the tumor site, too (1, 2). TILs history goes back to more than two centuries. In 1863, Virchow observed that neoplastic tissues contained leukocytes (3). In 1982, Steven Rosenberg, the father of adoptive cell therapy (ACT), isolated TILs from mouse models of tumors for the first time (4). He showed that combining cyclophosphamide, TILs, and interleukin (IL)-2 can improve 50-100% of colon adenocarcinoma-bearing mice with hepatic or pulmonary metastasis (5). This report has underpinned TIL therapy in treating advanced cancers. The first TIL therapy in humans was also conducted by his group in 1988, resulting in a 60% regression in metastatic melanoma (6).
Despite hematologic malignancies with lineage-specific markers, solid tumors are highly heterogeneous and do not possess an ideal tumor marker (7, 8). Targeting a tumor-associated antigen (TAA) leads to the predominance of tumor cells that do not express any special tumor marker (9). TILs are polyclonal cells with diverse receptors capable of detecting a wide range of TAAs, making them superior to genetically-modified immune cells in treating solid tumors. TILs can overcome tumors’ heterogeneity and immune escape and provide better clinical outcomes than chimeric antigen receptor (CAR)-T cells in treating solid tumors with high mutation rates, such as melanoma (10). TILs are mostly tumor-specific and can target even unknown tumor neoantigens within the TME, resolving the necessity of prior knowledge about TAAs or MHC restriction (11).
TILs are generally divided into intratumoral and stromal TILs (iTILs and sTILs). The iTILs are rare lymphocytes within tumor cell clusters, so their detection is complicated, while sTILs are frequently found in the tumor stroma and are easily detectable (2). Most TILs are effector memory T cells with high efficiency in proliferation and antitumor functions, are activated by TAAs in vivo, and can proliferate in vitro up to 105 times (12). TILs are TME-infiltrated cells; therefore, they possess chemokine receptors necessary for migration toward the TME after injection (11). Another advantage of TILs to CAR-T cells is lower off-target toxicity, which probably returns to the negative selection of T cell receptors (TCRs) during T cell maturation (7).
Thus far, TIL therapy has shown significant clinical results in metastatic melanoma (13), cervical squamous cell carcinoma (CSCC) (14, 15), and cholangiocarcinoma (16), and its initial results in non-small cell lung cancer, colorectal cancer (CRC), and breast cancer (BC) have been promising (17–19). Besides, the prognostic role of TILs in multiple tumors has been confirmed and entered into clinical guidelines (20). Herein, we reviewed the latest prognostic and therapeutic advances of TILs in solid tumors and discussed the prospects of TIL therapy in cancer immunotherapy.
The advantages and disadvantages of different types of ACT and their brief protocols have been described in Table 1. TIL therapy depends on some procedures, including nonmyeloablative lymphodepletion and infusion of TILs, which are collected from a tumor mass and expanded ex vivo (Figure 1) (31). Although TILs separated from a resected solid tumor mass can recognize TAAs from their endogenous receptors, the inadequate number of obtained TILs is a limiting factor in cancer immunotherapy. In vitro administration of IL-2 as a T cell growth factor is a well-established protocol for expanding the isolated TILs (6). High-dose IL-2 exposure leads to a rapid proliferation of the lymphocytes, providing enough immune cells for ACT (13). TIL therapy is considered an effective therapeutic strategy in refractory metastatic melanoma, especially with the combination of nonmyeloablative lymphodepletion (13). TIL therapy relies on the infiltration of polyclonal T cells, capable of recognizing multiple TAA or unknown antigens. Recent studies on the TAA-specificity of TILs through peptide-loaded HLA multimers revealed low reactivity of TILs to the specific differentiation antigens.
Figure 1 TIL therapy process. The most common TIL production method is TIL isolation from resected tumor tissue and expansion in vitro using rapid expansion protocol (REP). The tumor is excised by surgery and mechanically cut into small pieces. Then, tumor pieces can be further digested by adding enzymes, such as collagenases, DNAse, hyaluronidase, etc. TILs are isolated via Ficoll density gradient centrifugation or specific cell sorting methods, such as magnetic- or fluorescence-activated cell sorting (MACS/FACS). In the pre-REP phase, TILs undergo primary expansion in the presence of high-dose interleukin-2 (IL-2). In some methods, following pre-REP, the tumor-specific TILs are selected and further expanded (selected TIL method). However, to reduce in vitro culture period and to maintain TILs efficacy, some studies skip the TIL selection process and expand the bulk TILs (young TIL method). In REP, high dose IL-2, anti-CD3, and irradiated allogeneic peripheral blood mononuclear cells (PBMCs) as feeder cells are added to the TIL culture. The expanded cell products that passed the quality controls (sterility, negativity for blood-borne diseases, and phenotype checking) are ready to administer to the patient. Before TIL administration, patients undergo lymphodepletion, and 10-150 billion TILs are infused into patients along with high dose IL-2.
In contrast, the majority of TILs respond to unknown mutated epitopes that would not be a target for central tolerance during T cell differentiation, which is an advantage of TIL therapy compared to other ACT types (32). Following any upcoming therapeutic agent, toxicity is often a critical concern. TIL therapy has shown a favorable safety profile based on early-phase clinical trial studies. However, this form of ACT has significant side effects associated with co-administration protocols, including high-dose IL-2 and different types of chemotherapy regimens (13, 33). Toxicity manifestations might be observed immediately or take time to appear in some patients; however, the application of standard clinical practices can limit these side effects (34).
Altogether, the apparent advantages of TIL therapy are the stable and reproducible clinical outcomes for pre-treated patients with severe tumor progression who have been excluded from other ACT strategies (35). Nevertheless, TIL therapy encounters challenges; for example, it is the most individualized treatment, so a particular infusion product must be prepared for each patient. Good manufacturing practice (GMP) procedures and well-trained personnel are mandatory in this regard. In addition to the costly manufacturing procedure, sometimes the production procedure takes more than one month, which is undesirable for patients with rapid tumor progression (13).
In contrast to TIL therapy, tumor-specific T cell therapy mechanisms are based on developing genetically engineered T cells with accelerated antitumor activity. This is conducted by transferring genetic elements encoding a modified TCR or a synthetic CAR capable of recognizing specific tumor antigens. While different methods are available for developing genetically engineered T cells, the typical approach is based on collecting particular immune cells by leukapheresis before their genetic modification and eventual reinfusion. Similar to TIL therapy, a preconditioning regimen is often used prior to TCR-modified T cell therapy (31).
In TCR-modified T cell therapy, the specificity of T cells depends on modified TCR alpha and beta chains, leading to specific recognition of the tumor antigens (21). The generation of transduced T cells with TAA-specific TCR genes represents many advantages over TIL therapy. In this regard, TME-infiltrated T cells are not always available and would not expand to an adequate number necessary for cancer immunotherapy. On the other hand, the transduction of retrovirus-encoding modified TCR into the isolated peripheral blood lymphocytes can promote rapid access to produce massive TAA-specific T cells (32). Moreover, TCR-modified T cell therapy leads to a greater yield of activated neoantigen-specific T cells and has better proliferative potential than TIL therapy, which may display an exhausted phenotype of lymphocytes because of the frequent stimulation (36). The promising outcomes of the TCR-modified approach with differentiated melanoma/melanocyte antigen in patients with melanoma have been addressed in previous studies (37). Although TCR engineering is a desirable method for cancer immunotherapy, it has more limitations than TIL-based ACT. In this regard, recognizing only one specific tumor antigen allows the tumor cells to escape from TCR-modified T cells through the down-regulation of MHC class I or the tumor antigen, leading to antigen loss. In addition, autoimmune manifestation due to aberrant TCR recognition is a crucial concern. However, normal cells express these antigens at the lower level, but the high-affinity TCRs can represent significant binding to these restricted epitopes (38). Finally, unknown TCR specificity should be considered due to the modified TCR chains mispairing with endogenous TCR alpha and beta chains, leading to the high reactivity against self-antigens (38).
CAR-T cells combine antibody-based recognition with T cell functionality and cytotoxicity. The CAR-T cell structure depends on TCR signaling and the appropriate fragment of an antibody, targeting the molecules of interest expressed on the tumor cell surface. In contrast to TIL therapy and TCR modification strategy, CAR recognition is independent of peptide processing and antigen presentation on MHC molecules. Therefore, many cell surface antigens can be considered a potential CAR-triggering target which is the main advantage of CAR-T cell therapy over the other forms of ACT (39). Similar to transgenic TCR therapy, the source of T cells is not very important for CAR-T cell therapy, and T cells can be isolated from peripheral blood cells (40).
In contrast to the TIL therapy as a safe approach for the ACT, there are several safety risks associated with the CAR-T cell strategy, including I. on-target, off-tumor reactivity due to recognition of the same targeted antigen expressed on normal tissues, II. off-target reactivity due to the cross-reaction of CAR-T cells with non-specific peptides, and III. cytokine-release syndrome and immune effector cell-associated neurotoxicity syndrome, which is characterized by the significant secretion of inflammatory cytokines from non-specific immune cells (41).
The number and functionality of TILs are two critical factors determining the success of TIL therapy. The most common TIL production method is TIL isolation from resected tumor tissue and expansion ex vivo using rapid expansion protocol (REP) (Figure 1) (7). The tumor is excised by surgery and mechanically cut into small pieces. Then, tumor pieces can be further digested by adding enzymes, such as collagenases, DNAse, hyaluronidase, etc. TILs are isolated via Ficoll density gradient centrifugation or specific cell sorting methods, such as magnetic- or fluorescence-activated cell sorting (MACS/FACS). In this step, CD8+ T cells could be enriched, or Tregs could be depleted to enhance the antitumor effects of TIL therapy. Although mechanical and enzymatic digestions rapidly isolate TILs from the tumor tissue, they might damage TILs. Some studies suggest cutting tumor mass into 1 mm3 pieces and putting them into cell-culture media containing high-dose interleukin-2 (IL-2) for two weeks. It lets TILs gradually egress from tissue to the medium. However, this method requires a considerable amount of IL-2 because it should be replenished every 2-3 days.
In the pre-REP phase, TILs undergo primary expansion in the presence of IL-2. In some methods, following pre-REP, the tumor-specific TILs are selected and further expanded (selected TIL method) (11). However, to reduce in vitro culture period and to maintain TILs efficacy, some studies skip the TIL selection process and expand the bulk TILs (young TIL method) (42). In REP, high-dose IL-2, anti-CD3, and irradiated allogeneic peripheral blood mononuclear cells (PBMCs) as feeder cells are added to the TIL culture. Although TIL expansion occurs mainly via treatment with high doses of IL-2, various other in vitro expanding and stimulation methods such as cytokines (such as IL-15 and IL-21), costimulatory molecules, immune-checkpoint inhibitors (ICIs), as well as their co-culture with antigen-presenting cells (APCs) or feeder cells are available (1, 7).
Several methods have been used to detect and remove the residual tumor cells throughout TIL production following isolation from resected tumor tissue. In Rapid Expansion Protocol (REP), residual tumor cells die out after the culture of cells since the culture conditions only support lymphocytes (43). Moreover, obtained TILs by mechanical disaggregation and Ficoll-Hypaque density gradient centrifugation can be filtrated through nylon monofilament mesh to eliminate residual tumor cells (44). According to another study, residual tumor cells may be removed using mononuclear cells stimulated with IL-2 or cultivated in a serum-free environment (45). Besides, residual tumor cells can be removed from TIL products using FACS/MACS.
Generally, several techniques, such as allelic-specific oligonucleotide real-time quantitative polymerase chain reaction, immunohistochemistry, flow cytometry, and fluorescence in situ hybridization, can be employed to determine residual tumor cells (46, 47). Using immunohistochemical staining for S100, gp-100, and tumor markers melanoma-associated antigen recognized by T cells (MART-1), it is possible to detect the presence or absence of residual tumor cells (48). However, evaluating tumor markers by flow cytometry is more common in TIL manufacturing.
The expanded cell products that passed the quality controls (sterility, negativity for blood-borne diseases, and phenotype checking) are ready to administer to the patient. Before TIL administration, patients undergo lymphodepletion via chemotherapy and radiotherapy. Then, 10-150 billion TILs are intravenously infused into patients along with multiple high doses of IL-2. In most clinical trials, TIL therapy has been administered via the intravenous route, although few studies have reported other routes, such as intrapleural, intraperitoneal, intrathoracic, or intratumoral, based on the tumor location (49, 50).
Melanoma, the fifth leading cancer in the USA (51), develops from the malignant transformation of melanocytes, the pigment-producing cells found in the basal epidermis of the skin, the choroidal layer of the eye, inner ear, and leptomeninges (52). Invasive melanoma is one of the most lethal cancers and, despite comprising only 1% of skin cancers, accounts for over 80% of skin cancer deaths. Thanks to recent therapeutic approaches such as combinational ICIs with ipilimumab (anti-CTLA-4) and nivolumab (anti-PD-1), the overall melanoma mortality rate has declined, resulting in an improved 1-year relative survival rate for metastatic melanomas in most patients (53). Although FDA has not approved TIL therapy, it might be an appropriate regimen for ICI-resistant patients.
As mentioned earlier, the clinical development of TIL-based therapy began in 1988 on 20 patients with metastatic melanoma, leading to tumor regression in 40-60% of patients lasting 2-13 months (6). Then, the same group examined the efficacy of TILs in conjunction with high-dose IL-2, with or without cyclophosphamide, in 86 patients with metastatic melanoma (31). With an objective response rate (ORR) of 34%, no significant differences were seen between patients receiving or not receiving cyclophosphamide (35% versus 31%). The frequency of response to treatment has been associated with shorter culture duration, shorter doubling time, and higher lysis activity to autologous tumor targets.
In 2002, Dudley et al. showed that lymphodepletion with cyclophosphamide and fludarabine before TIL re-infusion led to in vivo clonal expansion of TILs and their prolonged response, as well as autoimmune melanocyte destruction in vitiligo and uveitis patients with IL-2 refractory metastatic melanoma. Six out of thirteen patients (47%) in this study had an objective partial response (54). After that, in three sequential clinical trials, they assessed the efficacy of TIL therapy in combination with IL-2 following a non-myeloablative lymphodepleting chemotherapy conducted by the administration of 60 mg/kg cyclophosphamide and 25 mg/m2 fludarabine with or without either 2 or 12 Gy of total-body irradiation, in 93 patients with measurable metastatic melanoma who followed-up for 62 months (55). Data indicated the superiority of total-body irradiation in conferring clinical benefits over chemotherapy alone, such that by adding 2 and 12 Gy of total-body irradiation, the ORR increased from 49% in the chemotherapy alone group to 52% and 72%, respectively. Twenty of the 93 patients (22%) had complete tumor regression lasting beyond three years, with 100% and 93% of 3- and 5-year survival rates, respectively. The high telomere length of the transferred TILs reflects their higher replicative capacity, which is strongly correlated with clinical response.
As previously mentioned, the higher telomere length of the infused TILs and higher expression levels of CD27 and CD28 are associated with survival and clinical efficacy (56, 57). Some studies have tried simplifying and shortening TIL preparation to generate young TILs with higher antitumor activity. In this regard, in a trial, administration of CD8+ enriched young TILs produced by a simplified method, omitting the personalized tumor-reactivity screening step, resulted in an ORR of 48%-58% in melanoma patients (58).
Besser et al. demonstrated that TIL therapy leads to persistent and complete responses in eighty patients with stage IV melanoma who were refractory to IL-2 or ipilimumab (59). Patients received unselected young TILs after a non-myeloablative lymphodepleting standard chemotherapy, followed by bolus high-dose IL-2. Thirty-two patients had been treated with ipilimumab before or after TIL transfer. The ORRs of 40% with five cytokine-release syndromes were seen among 57 evaluated patients. The 3-year survival rate was 78% in responding patients. Despite the lack of association between response to previous immunotherapy and the overall response to TIL therapy, the total count of transferred CD8+ cells, as well as the TIL culture duration independently predicted clinical outcomes.
More recently, Sarnaik et al. reported the safety and efficacy of lifileucel (LN-144), an autologous TIL product, in a phase II study sponsored by Iovance Biotherapeutics, Inc. in 66 patients with advanced melanoma who were refractory to prior treatment with ICI(s) and BRAF ± MEK inhibitors (60). After a non-myeloablative lymphodepletion regimen, patients were administered a single infusion of lifileucel followed by high-dose IL-2. The ORR was 36%, and the overall disease control rate was 80%. The ORR of 41% and the disease control rate of 81% were seen in the subgroup refractory to antibodies against programmed cell death protein 1 (PD-1) or PD-ligand 1 (PD-L1). In a multicenter phase III clinical trial on 186 patients with unresectable stage IIIC-IV melanoma who mainly were (86%) refractory to anti-PD1, the efficacy of 4 doses (3mg/kg) ipilimumab versus one dose (5×109) TIL therapy was evaluated (61). The median PFS for ipilimumab was 3.1 months versus 7.2 months in TIL therapy group. The ORR was 21% and 49% in ipilimumab and TIL therapy groups, respectively. CR was 7% in the ipilimumab group, while in TIL group, it was 20%. Finally, the median OS was 18.9 months in ipilimumab versus 25.8 months in TIL group (61). Noteworthy, the grade ≥ 3 adverse effects were seen in 57% and 100% of ipilimumab and TIL groups, respectively. It suggests that TIL therapy might be a promising option for those unresponsive to ICIs (61).
Lung cancer, the first leading cause of cancer death globally, is divided into two major types, including small cell lung cancer (SCLC) and non-small cell lung cancer (NSCLC), which the latter accounts for ~90% of all cases (62). Although ICIs have revolutionized the treatment of NSCLC, evidence indicates that only a small proportion of patients experience objective responses to ICIs, and most of them show disease progression or grade 3–4 immune-related adverse events on immunotherapy (62). Several studies have demonstrated an association between higher TIL levels, improved recurrence-free survival, and reduced chance of systemic recurrence in NSCLC (17, 63). Also, NSCLC carries a high mutation load (64), leading to a high neoantigen level; furthermore, NSCLC is more likely to respond to ACT, including TIL therapy (17).
The first cancer TIL therapy trial was reported by Kradin et al. in 1987 on NSCLC patients (65). Although five of seven patients experienced cancer reduction, none of them achieved an objective response. The next trial by Kradin et al. on eight NSCLC patients did not result in measurable responses (28). In 2018, Ben-Avi et al. assessed the feasibility of TIL generation according to the well-established melanoma TIL protocol in five patients with advanced-stage NSCLC undergoing thoracic surgery. Despite the small size of the tumors, they reported a successful TIL establishment in all of them (63).
More recently, in a phase I study, Creelan et al. evaluated the safety and efficacy of autologous TILs in combination with nivolumab in twenty patients with advanced NSCLC following disease progression on nivolumab monotherapy (17). Patients received a single TIL infusion preceded by standard lymphodepleting chemotherapy, followed by IL-2, and then nivolumab maintenance. Eleven out of thirteen patients showed tumor regressions with a median best change of 35%, and three had confirmed responses, including two complete responses lasting for 1.5 years. However, none of the patients achieved median OS. Despite these controversial results from very few small studies on NSCLC patients, TIL therapy might still be a promising candidate for the management of lung cancer. It will be clarified upon releasing the results of several ongoing clinical trials exploring the clinical efficacy of TIL therapy alone or in combination with ICIs in lung cancer (NCT04614103, NCT03215810, NCT03903887, NCT04919616, NCT03645928, NCT00019084, NCT03407040, and NCT04677361).
Ovarian cancer is the eighth major cause of cancer-related mortality in women globally. Among different histological subtypes, epithelial ovarian cancer (EOC) accounts for ~90% of ovarian cancers (66). Despite significant advances in therapeutic strategies, the prognosis for EOC remains poor, and disease recurrence occurs in a considerable portion of patients within 2–3 years (67). Indeed, despite the great hope placed on ICIs in cancer therapy, their use in most EOC patients has not yielded clinically meaningful results so far (68). The association of the iTILs with good clinical outcomes has triggered TIL therapy ideas in EOC; nevertheless, the available trial results have not shown any significant clinical efficacy. In 1991, Aoki et al. published the first results of TIL therapy with or without chemotherapy in 17 patients with advanced or recurrent EOC (22). In TIL only group (7 patients), the ORR was 75%, and one patient had a complete response. In TIL plus chemotherapy (10 patients), 90% ORR and seven complete responses were obtained. Two trials on intraperitoneal TIL therapy by Freedman et al. did not result in detectable responses, except for some reduction of CA125 level in ascites and blood of a few patients (69). In a phase I trial (70), Fujita et al. compared the clinical efficacy of TIL therapy in the first-line setting in EOC with standard first-line treatment. Thirteen patients received TIL infusion following primary debulking surgery, then platinum-based adjuvant therapy. As a control group, eleven patients were treated with only standard first-line treatment. The 3-year disease-free survival (DFS) rates of 82.1% against 54.5% were seen in the TIL group and controls, respectively.
In a pilot study at National Center for Cancer Immune Therapy (71), Pedersen et al. treated six platinum-resistant patients with a single TIL infusion after standard lymphodepleting chemotherapy followed by high doses of IL-2. Clinical responses were limited and primarily short-lived, and infused TILs expressed exhaustion markers, including lymphocyte-activation gene-3 (LAG3) and PD-1. More recently, the same group assessed the combination of TIL therapy with ICI sequentially (72). Six patients with late-stage EOC received an infusion of TILs preceded by ipilimumab followed by low-dose IL-2 and nivolumab. Partial response was seen in one patient, which prolonged for 12 months; the other five patients experienced short-lived stable disease. Similar to the previous study, 90-100% of infused TILs expressed LAG3. The engagement of LAG3 on T cells with MHC-II on cancer cells usually results in limited clinical outcomes. Hence, they conducted a phase I/II study on 18 patients with advanced ovarian cancer, in which they added relatlimab (anti-LAG3 antibody) to the TIL therapy regimen (72) to unleash T cell antitumor activity by inhibiting the LAG3-MHC-II interaction (NCT04611126). Preliminary results of phase I/II ongoing clinical trial testing the feasibility and safety of TIL therapy during carboplatin-paclitaxel chemotherapy with or without interferon (IFN)α in 12 patients with recurrent platinum-sensitive EOC showed that ten patients (83%) achieved ORR of 83% and two experienced stable disease (73).
There are several ongoing clinical trials of TIL therapy alone or in combination with other therapeutic strategies in patients with EOC (NCT03412526, NCT03610490, NCT03318900).
In conclusion, despite feasibility and tolerability, TIL therapy in EOC had limited success. Some possible explanations for this low clinical efficacy could be inefficient ex vivo expansion, expression of exhaustion markers, such as PD-1, LAG3, and suboptimal lymphodepleting chemotherapy, or IL-2 support (71, 72).
Head and neck squamous cell carcinoma (HNSCC) is a heterogenic group of cancers developing from the mucosal epithelium in the oral cavity, pharynx, and larynx (74). According to causative factors, HNSCC is classified into two categories: human papillomavirus (HPV)-positive and HPV-negative cancers. Also, the Epstein-Barr virus (EBV) has been associated with a subtype of HNSCC, so-called nasopharyngeal cancer (NPC) (74). Since viral oncoproteins are expressed in HPV and EBV-associated cancers, they are ideal targets for ACT (74).
Despite the great hope placed on ICIs in HNSCC, its response rates remain less than 20% (75). Increasing evidence indicates the feasibility of patient selection in HNSCC for TIL therapy and gives the green light to its clinical testing, confirming a higher TIL number as a significant prognostic factor in the OS of both HPV-positive and HPV-negative patients (76). In addition, HPV-associated oropharyngeal cancers harboring viral oncoproteins such as E6 and E7 as a target for TIL therapy (77). Recently, in a clinical trial study (phase II) by the National Cancer Institute, the clinical efficacy of TIL therapy was evaluated in metastatic HPV-related cancers (25). Patients received a single TIL infusion, preceded by standard lymphodepleting chemotherapy, followed by high-dose IL-2. The ORRs of 28% and 18% have been observed in cervical cancer and non-cervical HPV-related cancer groups, respectively; one of whom has been a patient with HNSCC with lung metastases who experienced a response lasting five months (25).
More recently, in a clinical phase I/II trial, Kverneland et al. evaluated the efficacy of TIL therapy supported by ICIs in 25 patients with different progressive metastatic cancers, one of whom was an HPV-positive HNSCC patient (51). Patients received a single TIL infusion preceded by ipilimumab and nivolumab, as well as chemotherapy, followed by nivolumab and low-dose IL-2. They reported sizeable tumor regressions of 30%-63% in 5 patients, including confirmed partial response (16%) in two patients with HNSCC (51).
Based on these results, a phase II multicenter clinical trial is ongoing to evaluate the efficacy of a single autologous TIL infusion (LN-145/LN-145-S1) followed by IL-2 after a standard lymphodepleting regimen in patients with recurrent and metastatic HNSCC (NCT03083873). Another phase II trial is ongoing to explore the efficacy of combination TIL therapy with pembrolizumab in PD-1-naïve patients with advanced, recurrent, or metastatic HNSCC (NCT03645928), and preliminary results from 12 patients have shown an ORR of 42.9% (78).
Also, there is an ongoing phase I clinical trial of tumor growth factor (TGF)-β resistant, EBV-specific T cells for treating EBV-positive NPC (NCT02065362). The obtained results motivated researchers to evaluate the clinical efficacy of TIL-based ACT in the treatment of NPC. In a phase I study, Jiang et al. assessed TIL therapy’s safety and antitumor activity following concurrent chemoradiotherapy in patients with EBV-induced locoregionally advanced NPC and reported sustained antitumor activity and anti-EBV immune responses following TIL therapy (79). Twenty patients received a single dose of TIL infusion following concurrent chemoradiotherapy. Nineteen patients experienced an ORR and DFS longer than 12 months after TIL infusion (79). The results of these clinical trials will shed light on the potential TIL therapy in HNSCC and might change the landscape of its management, especially in HPV and EBV-positive subgroups.
The first evidence of TILs and their association with better clinical outcomes in BC was reported in 1992 (80). TILs frequency is approximately 10% in luminal BCs, 15% in HER2+ BCs, and 20% in TNBC (2). Moreover, in 20-28% of TNBCs, called lymphocyte-predominant BC (LPBC), TILs constitute >50-60% of the tumor stroma (2). Broadly speaking, lymphocyte infiltration is associated with a better prognosis in all BC types, especially in TNBC and HER2+ BC (81). Studies show that for every 10% increase in breast TILs, there is a 15-20% reduction in recurrence and mortality rate (2). Breast TILs approximately comprise of 70-80% T cells (2/3 are CD4+ and 1/3 are CD8+), 20% B cells, <5% macrophages, <5% NK cells, and 1% DCs (2). Tissue-resident memory T cells (TRMs) are CD8+ CD69+ CD103+ cells with a greater cytotoxic potential than effector CD8+ cells (82). TRMs comprise ≈40% of CD8+ TILs in BCs. TRMs <20% of the CD8+ T cells are associated with poor prognosis, while increasing TRMs to >60% of the CD8+ T population improves the recurrence-free survival (RFS), OS, and treatment responses in TNBC (82). Hence, TRMs have the potential as a predictive marker and a therapeutic target in TNBC (83).
Regulatory T cells (Tregs) are principal immunosuppressive cells in the TME (1). They infiltrated tumors following the PITPNM3 receptor response to CCL18 secreted by tumor-associated macrophages (TAMs) (84). Increased CCL18 levels, along with increased Tregs and follicular regulatory T cells, are strongly associated with increased relapse risk and lower RFS and OS (85, 86). Regarding the fact that the Tregs depend on IL-2, the use of IL-2 superkine (fusion of IL-2 and Fc of IgG2) and PEGylated IL-2 can decrease Tregs and stimulate CD8+ T cells and NK cells (87, 88). In vivo anti-CD25 can also deplete CD25+ Tregs for a long time (89). We have also reported that using pentoxifylline (a methyl xanthine derivative) can reduce the Treg proportion and enhance antitumor responses in an IL-2-mediated expansion of TILs (1). Interestingly, chemotherapy regimens have a more destructive effect on Tregs than CD8+ T cells (90). In contrast, Tregs are radio-resistant and prevail after radiotherapy (91). Cytotoxic T-lymphocyte-associated protein 4 (CTLA4) is a major inhibitory molecule of Tregs. Administration of anti-CTLA4 as monotherapy or combined with anti-PD1 resulted in ORR=12% and 12-month OS in 36% of chemotherapy-resistant patients (92).
Tumor-infiltrated CD57- NK cells, along with the high expression of CD155, can predict the complete pathological response (pCR) after treatment and improve OS in all BC patients (93, 94). Generally, BCs that respond better to trastuzumab (anti-HER2 antibody) have more NK cells and potent antibody-dependent cellular cytotoxicity (ADCC) (95). Activation of NK cells in vivo or ex vivo with IL-2, IL-15, and IL-12, as well as using CAR-NK cells, are NK-based therapeutic methods in BC (96, 97). The safety and efficacy of a CAR-NK cell produced by binding trastuzumab to NK cells are currently under investigation in HER2+ patients (NCT04319757) (98).
B cells are highly infiltrated in 20% of BCs, accounting for about 40% of total TILs (2). Tumor-infiltrating B cells (TIBs) can undergo affinity maturation at the tumor site to secrete high-affinity apoptosis-inducing IgG against tumor antigens (99). B cells also act as APC to stimulate T cells. However, B cells affected by CD40, Toll-like receptor (TLR) ligands, and inflammatory cytokines may become regulatory B cells (Bregs) (100), which are able to suppress immune responses and induce Treg differentiation (100). PD-L1+ TIBs were significantly associated with improved survival and pCR after treatment (101). Contrarily, the increase in CD19+ CD24hi CD38hi Bregs in BC is associated with higher Tregs and lower Progression-free survival (PFS) (102). Using CXCR5-targeted CpG ODN (103), signal transducer and activator of transcription 3 (STAT3)-inactivating resveratrol (104), and IL-10 depletion (100) can reduce Bregs and Tregs. More preclinical and clinical studies are needed to determine the prognostic and therapeutic potentials of TIBs.
Various methods are being studied to improve the amount, composition, and function of TILs. Radiotherapy and chemotherapy improve the infiltration and function of TILs by inducing immunological death (105). Cancer vaccines are also a promising way to strengthen TILs that have been shown to improve the three-year PFS from 31% to 76.9% in phase II/IIIA clinical trial on progesterone receptor (PR-)/estrogen receptor (ER-) patients (106). PD-L1 is expressed in about 60% of BCs and is positively associated with higher TIL levels (107). PD-L1 expression level could be >60% in TNBCs, highlighting the success of ICIs in TNBC (108). Accordingly, anti-PD-L1 with ORR=28% showed better results than anti-PD-1 with ORR=16% and anti-CTLA4 with no significant response (109). Atezolizumab (anti-PD-L1) and pembrolizumab (anti-PD-1) are currently the only FDA-approved ICIs for treating TNBC (110, 111). In the field of CAR-T cells, the use of MUC1-specific CAR-T cells (a protein upregulated in 95% of BCs) exhibited promising results and is currently in phase I clinical trials for treating metastatic BCs (NCT04020575) (112). It has been observed that increased RAS/MAPK signaling is associated with decreased lymphocyte infiltration in TNBC. Accordingly, MEK inhibitors increased lymphocyte infiltration, MHC-I and MHC-II, and PD-L1 expression in the TME (86). This finding suggests that combining MEK inhibitors with ICIs could be an interesting option for synergistic antitumor effects. Additionally, targeting other ICs such as T cell immunoglobulin and mucin-domain containing 3 (TIM3), indoleamine 2,3-dioxygenase (IDO), and the adenosinergic pathway has shown promising results in improving the function of TILs in TNBC, which should be the subject of future investigations (113–115).
CRC is the third most common cancer worldwide. The type and density of TILs are important histopathological features of CRC and strongly affect the tumor progression and OS rate (116). Several studies showed that high levels of TILs are associated with a better prognosis in CRC. On the other hand, reduced TIL infiltration is associated with metastasis and the spreading of the tumor cells into the blood, lymphatic vessels, and the perineural space (117, 118).
TILs in CRC are a mixture of T cells, B cells, NK cells, macrophages, and other immune cells that impact the prognosis of CRC. Thus, their population can serve as a prognostic biomarker, and CRC could be an attractive target for immunotherapy (119).
High frequency of T cells (CD4+ or CD8+) in CRC tissue TILs is associated with a lower risk of metastasis, significantly improved prognosis, reduced relapse rate, and longer DFS and OS (120). CD8+cytotoxic T cells in the tumor epithelium can destroy tumor cells by recognizing the tumor antigens and directly suppressing metastasis. The existing studies showed a correlation between the prognosis of CRC and the number of CD8+ T cells. Therefore, a lower CD8+ cell number is associated with lower DFS and relapse rates (121). CD8+ T cell frequency predicts an effective response to chemotherapy, and also, there is a positive correlation between high pretreatment CD8+ T cell density and response to neoadjuvant chemoradiotherapy (122). In patients with high microsatellite instability CRCs, the high numbers of CD8+ TILs due to their specific neoantigen load were accompanied by an improved response to anti-PD-1 antibodies (123).
Effector memory T cells are responsible for long-lasting protection against tumors and are defined by the presence of CD3, CD8, CCR7, CD45RO, CD27, and CD28 markers. Infiltration of memory T cells is related to the absence of metastatic invasion and improved clinical outcomes (124). Intriguingly, the accumulation of Tregs in the CRC is associated with a favorable prognosis, while a higher ratio of CD8+ cells to FOXP3 seems to improve the prognosis (125).
The presence of TIBs is accompanied by infiltration of CD8+ T lymphocytes and has a positive prognostic role in CRC (126). CD20+ TIBs play different roles in antitumor immune response, such as the production of anti-TAA antibodies, cooperation with cytotoxic T lymphocytes by producing cytokines that can support T cell responses, and antigen-presentation to T cells to induce a cellular immune response (127). The favorable prognostic value of CD20+ TIBs in CRC synergizes with the prognostic effects of CD8+ T lymphocytes (128). Contrastingly, there is a negative correlation between tumor-associated neutrophils (TANs) and TAMs density and CRC patient prognosis (129).
These findings suggest that an active immune response correlates with favorable survival and provides a rationale for TIL therapy in CRC. Previous reports demonstrated the possibility of isolating and expanding sufficient numbers of TILs from CRC patients, which provides a rationale for advancing personalized immunotherapy in CRC (130). In a clinical trial (NCT01373047) of 16 patients with CRC, infusion of expanded sentinel lymph node (SLN)-derived CD4+ T helper 1 (Th1) cells induced an antitumor response, and complete tumor regression occurred in four of nine stage IV patients with distant metastases (131).
In another clinical trial conducted by Gardini et al. in the 1990s, 14 CRC patients with positive carcinoembryonic antigen (CEA) and liver metastases were treated with IL-2-expanded TILs. Findings showed no significant difference in DFS between the conventional chemotherapy and TILs group (132). A phase I/II study of SLN T cell-based therapy in patients with stage IV CRC showed that the survival rate of patients who received SLN T cell transfusion was significantly higher than the controls (19).
Recently, the successful application of TILs in CRC patients was reported in 2016 by Rosenberg’s team. In this study, KRAS G12D-specific CD8+ T cells were expanded from metastatic lung lesions of a CRC patient, and following TIL therapy, results showed eradication of 6 of 7 lung metastases. One tumor-progressing patient expressed the mutated KRAS G12D and did not genetically encode the HLA-C*08:02 allele. Harvesting sufficient TILs from CRC samples is challenging because relatively few effector cells infiltrate CRC tumors (133). Currently, CD8+ T cell therapy with pembrolizumab is an active clinical trial to treat CRC.
The most common type of liver cancer (90%) is hepatocellular carcinoma (HCC). TILs play a major role in the prognosis and immunotherapy of HCC. Some TIL subsets show significant prognostic values on treatment and survival outcomes so that they can serve as prognostic biomarkers. Foxp3+, CD8+, and CD4+ T cells are the most widely analyzed subgroups of TILs in HCC (134). The high frequency of TILs in the invasive margin, intratumoral, and perivascular areas is associated with improved OS and RFS in HCC patients. In addition, TILs within the tumor or perivascular area are positively associated with the outcome of HCC patients, and deleting the exhausted effector T cells expressing PD-1 reduces the incidence of HCC so that the density of CD8+ cells can be a prognostic marker and can predict the treatment outcome in HCC (135).
Increased density of CD4+ TILs is also associated with better outcomes and acts as a protective factor in HCC. Disruption of CD4+ cells impairs CTL activation and correlates with poor prognosis and high recurrence of HCC (136). Contrarily, the frequency of Foxp3+ Tregs in peripheral blood and tumor tissue is associated with poor prognosis and invasiveness of HCC (137). The decreased frequency of PD-1+ Foxp3+ Tregs after administration of sorafenib, a multikinase inhibitor, enhances the antitumor immune responses. In addition, ICIs targeting CCR4, PD-1, LAG3, TIM3, and glucocorticoid-induced TNFR-related protein (GITR) on the Treg surface enhance the TILs’ antitumor function in HCC (138).
B lymphocyte subtypes, including naive B cells, CD20+ B cells, CD27- isotype-switched memory B cells, IgM+ memory B cells, and plasma cells, are present in HCC, defined as TIBs, all of which show reduced count and functionality compared to normal tissue (139). A reduced number of naive B cells and CD27- isotype-switched memory B cells are predictive factors for the progression of HCC. TIBs secrete IL-12 and IFN-γ and increase the infiltration of CD8+ T cells and NK cells to eliminate tumor cells (140).
The density of NK cells and macrophages is also associated with the prognosis of HCC. A low intratumoral CD56+ NK cell subset correlates with shorter DFS and OS outcomes (141). Peritumor-activated hepatic stellate cells predict poor clinical outcomes in HCC after therapeutic resection (142).
HCC patients with prominent lymphocytic infiltration who were surgically resected had a 38.6% lower recurrence rate and a 34.9% higher five-year OS than patients with weak lymphocytic infiltration. Considering the significant correlation between TILs and HCC prognosis, using TILs expanded from HCC can be a promising treatment (143). The feasibility of TIL therapy was demonstrated in phase I clinical trials in HCC patients. In a randomized clinical trial, TIL infusion improved RFS after liver resection in 150 HCC patients (144). Jiang et al. conducted the only phase I clinical trial of TIL therapy as a new treatment strategy for HCC patients. In this study, 15 HCC patients received autologous TILs after tumor resection. After a median follow-up of 14 months, 15 patients (100%) were alive, 12 patients (80%) showed no evidence of disease, and three patients had tumor recurrence. Despite this report of relatively high antitumor efficacy and low toxicity of TILs, there are no other clinical studies involving TIL therapy in HCC (145).
The critical application of TIL therapy was also reported in other types of solid tumors, including gastric carcinomas, gynecologic, and urological cancers. High infiltration of CXCR3+ immune cells in EBV-associated gastric carcinomas is associated with improved prognosis and is considered a discrete prognostic factor for RFS but not OS (146, 147). In contrast, the expression of CCR7 and the presence of PD-L1+ exhausted T cells are associated with a poor prognosis of gastric carcinomas (148).
Few studies report the presence of tertiary lymphoid structures in pancreatic ductal adenocarcinoma (PDAC) and their correlation with OS and PFS (149). In these patients, the immunosuppressive features of Th2 cells contribute to the rapid progression of tumors and reduction in OS (150). Some immunohistochemical studies reported that high recruitment of TAMs, TANs, and FOXP3+ Tregs was associated with a worse prognosis in PDAC patients (151, 152). However, similar to gastric carcinoma, infiltration of CD8+ T cells and CD20+ B cells is correlated with improved PFS in PDAC (152, 153).
There is not enough knowledge about the prognostic value of TILs in gynecologic cancers. Intra-epithelial CD8+ T cells and stromal CD3+ T cells may have prognostic significance (154). Workel and colleagues reported that intra-epithelial PD-1+ CD8+ T cells were associated with a favorable prognosis in vulnerable patients to endometrial carcinoma (155). A comprehensive systematic review of the ACT in gynecologic cancers has reported 41.4% ORR, 57.6% of disease control rate, 31.4% of disease stability rate, and 46.0% toxicity rate for TIL therapy in 238 patients with grade III/IV gynecologic cancers (156).
The prognostic significance of TILs in three urological tumors, including renal cell carcinoma (RCC), prostate cancer, and urothelial bladder carcinoma (the most common type of bladder cancer), is still unclear. Early reports in RCC indicated that TILs mainly included functional CD4+ T cells, effector memory CD8+ T cells, and NK cells with a limited population of B cells (157). Several studies have shown that increased T cell numbers are associated with tumor recurrence and a worse prognosis of RCC (158, 159). Moreover, Tregs’ presence can dampen T cells’ antitumor function (160). The studies documented before 2009 about TIL therapy in RCC seems disappointing because of the lack of reactivity of TILs against RCC (161). However, in recent years, several published studies demonstrated that manipulation of TILs can result in the generation of TILs with high tumor reactivity in recognizing or killing autologous tumors (162–164). Nevertheless, additional clinical studies are warranted.
The constituent of TILs in prostate cancer is still questioned and sometimes conflicting. Usually, TILs are scarce in the prostate TME. Although several studies have reported that CD8+ T cells are the predominant TIL population in prostate cancer, some studies reported a high proportion of CD4+ T cells and Tregs in prostate cancer (165, 166). Despite reports showing that high TIL infiltration is associated with recurrence, metastasis, and poor OS (167, 168) in prostate cancer, Yang et al. reported an improved 5-year OS in patients with a high number of TILs compared to patients with low TILs (169). The prognostic value of TILs on urothelial bladder cancer depends on the status of the disease and the region where they were measured (170). The increase in T cell frequency is correlated with worse prognosis and poor OS in non-muscle-invasive bladder cancer (171).
In contrast, a high proportion of T cells in muscle-invasive bladder cancer is associated with better clinical outcomes (172). Moreover, Wahlin et al. reported that CD8+ T cells and Tregs are associated with improved outcomes (173). The inconsistent findings of such studies indicate the necessity of further investigations.
ACT faces obstacles, including immunosuppressive TME, tumor heterogeneity, antigen escape, and ineffective trafficking to the tumor site; nonetheless, TILs have overcome some of these challenges because they are isolated from the tumor site (174). Despite TIL therapy’s benefits in treating solid cancers, this therapeutic method is associated with challenges and limitations (175) (Figure 2). The first step in TIL therapy is isolating TILs, which requires surgery to resect tumor tissue, and this invasive method can be distressing and even risky for patients with cancer (21). Furthermore, the tumor is not always accessible for resection, such as a subcutaneous nodule or deposit (176). Determining the exact location of lesions for tumor resection sometimes requires radiological interventions, the equipment for which is not available everywhere. On the other hand, following tumor resection, only a part of the TILs can be isolated and expanded. In the case of melanoma, about one-third of the isolated TILs fail to expand (177). In addition, preparing an exclusive TIL culture with antitumor activity for each patient and the necessity to access special and well-equipped centers, as well as technical expertise for TIL culture and expansion, are other obstacles in TIL therapy (58, 178). Another limitation of TIL therapy is the 6–8-week period for expanding and preparing these cells, which regarding the rapid progress of the tumor in the patient’s body, is considered a long time.
Figure 2 Challenges and limitations of TIL therapy. Immunosuppressive TME, tumor heterogeneity and immune system escape mechanisms are considered the main challenges to immunotherapy and cell therapy of human malignancies. Resection of the tumor mass may be difficult due to the inaccessibility of the tumor tissue. Surgery is considered an invasive method for the patient. Moreover, expanding TILs requires appropriate equipment and technical experience after separating them from the tumor tissue. Despite these cases, in vitro expanding TILs sometimes fail, and high doses of IL-2 for expansion may also be associated with toxicity. On the other hand, the preparation time of TILs is about 6 to 8 weeks, which affects the effectiveness of treatment in highly progressive tumors. Additionally, the low affinity of TILs isolated from tumor tissue, low persistence, and defects in co-stimulator molecules are other problems with TIL therapy.
For this reason, many patients miss the optimal treatment window, or their treatment is not completed (55). Therefore, designing protocols to reduce TILs production time is also imperative. In this regard, “CD8+ enriched young TILs” were employed to overcome this problem and give a comparable response rate to conventional TIL therapy. In this method, TILs are briefly grown and reinfused into the patient, ignoring the personalized tumor-reactivity assessment phase, which requires the co-culture of TILs with their autologous tumor cells. Furthermore, these tumor cells must be freshly cryopreserved following tumor resection (58). Additionally, genetic engineering approaches can help to produce tumor-reactive T cells and compensate for the lack of TIL availability in solid tumors (174).
Rapid expansion protocol, which uses IL-2, OKT-3, and irradiated feeder cells isolated from the autologous patient or multiple donors, is another way of expanding TILs (Figure 1). Nonetheless, this method can achieve TILs with different potency due to variations in the expression of co-stimulatory molecules on the surface of personalized T cells in different donors (178). Genetically-enhanced artificial APCs were also fabricated to develop a standardized “off-the-shelf” platform for the fast expansion of tumor-reactive TILs from patients with limited TIL numbers (179). Evidence revealed that the low persistence and reduced function of reinfused TILs are also considered other factors of the failure of TIL therapy because most of the TCRs isolated from patients with cancer have a relatively low affinity for tumor antigens (37). In this context, researchers have used high-avidity engineered T cells to enhance antitumor responses, which can be associated with undesirable adverse effects (180). Therefore, finding a way to enhance the function of low-affinity T cells without TCR genetic manipulation is still a necessity.
Co-stimulatory signals are often defective in TILs, and their persistence and degranulation capability are affected in the TME (181). Using co-stimulators could be beneficial for strengthening and sustaining the cytotoxic effects of TILs (182). However, studies show that co-stimulation is challenging because not all effector T cells express CD28 and will be regarded as CD28- TIL subsets (183). On the other hand, tumor cells also reduce the expression of CD80 and CD86 and increase the expression of inhibitory molecules such as PDL-1, which neutralizes TIL-dependent antitumor responses (184, 185). The importance of using molecules such as tumor necrosis factor receptor superfamily-9 (TNFRSF-9, 4-1-BB) as a co-stimulator in CAR-T cells is determined here (186). A look at the protocols of TIL therapy showed that administrating IL-2 to expand TILs is a common regimen. However, administering high doses of this cytokine in TIL therapy is associated with several side effects. In this context, studies have claimed that with low doses of IL-2 or other cytokines, patients under TIL therapy experienced fewer side effects and preserved effective partial or complete responses (13, 33). Another challenge of TIL therapy is the speed of the emergence of novel immunotherapeutic methods, such as ICIs, which can lead to the reprogramming of the TME by inhibiting immunosuppressive signals. As a result, infiltrated T cells can have constant and improved antitumor activity, along with less exhaustion (187).
Collectively, it can be argued that despite the advantages of TIL therapy, finding solutions to optimize protocols related to the expansion of tumor-reactive TILs and reduced production time can improve the capabilities of this therapeutic method. Correspondingly, combining therapies can undoubtedly increase cancer therapy’s effectiveness in a synergistic fashion.
Despite promising clinical findings following TIL therapy in solid tumors, many patients do not respond to the treatment (188). Therefore, researchers are looking for alternative therapeutic options using combination therapies, the most important of which are mentioned in this section (Table 2).
Different forms of therapies, including conventional chemo/radiotherapy, cytokine therapy (IL-2, IL-15, IL-12, GM-CSF, TNF-α, IFN-α, IFN-γ), ICIs (antibodies against PD-1, PD-L1, TIM-3, OX40, CTLA-4, LAG3), vaccines (DC-based vaccines, neoadjuvant) and chemokines (CXCR2, CXCR4) in combination with TILs could be effective strategies to improve TIL infiltration and function in solid tumors (200). Furthermore, by infecting tumor cells with the virus, oncolytic virotherapy causes the emergence of new tumor antigens and creates local signals for optimal activation of T cells and polarization of M2 macrophages in the TME (193). These combination therapies enhance TIL therapy’s effectiveness and require more clinical trials.
Chemotherapy has been accepted as a standard conventional method for treating all cancers. However, various adverse effects, poor bioavailability, high-dose requirements, low therapeutic indexes, multiple drug resistance, and non-specific targeting have limited the effectiveness of chemotherapy (201). Additionally, several experimental studies have demonstrated that the immunogenicity of resistant tumor cells and the host’s immune response are crucial factors in chemotherapy’s efficacy (202). Therefore, combining chemotherapeutics with immunotherapy is a promising approach for improving the clinical outcomes of cancer patients.
Study on patients with epithelial ovarian cancer, scientists compared the effects of the adoptive transfer of TILs alone with those of TILS therapy and a cisplatin-containing chemotherapeutic regimen. They found that patients’ complete response rate (CRR) was 30% and 70%, respectively. Interestingly, the second group showed no relapse after 15 months of monitoring (22). In another clinical study of osteosarcoma, patients with poor responses to chemotherapy were treated via chemotherapy plus TILs therapy (189). This study showed that the rate of DFS and overall survival (OS) were significantly increased in those who received TILs plus chemotherapy. Moreover, no significant TIL-related adverse effects were seen in the group treated with TIL plus chemotherapy. Therefore, combining TIL and chemotherapy may increase the survival of patients and reduce the defects of chemotherapy.
Although the clinical outcomes of combining radiotherapy and TIL therapy have not yet been reported, there is evidence that radiotherapy enhances the number of TILs recruited into the TME, boosting the anti-tumor response and prolonging patient survival. For instance, a study on head and neck cancer observed that the frequency of infiltrated TILs into the tumor site increased following radiotherapy and chemo/radiotherapy. Furthermore, this investigation reported that the expression of HIF-α as a hypoxia indicator decreased following the combination therapy (203).
Another clinical trial investigated the relationship between TILs and the effects of radiotherapy. The findings showed that radiotherapy causes an increase in the TILs frequency in patients with relapsed breast cancer (204). Another study compared the combination of chemo and radiotherapy with drug therapy in non-small cell lung cancer. The researchers observed that following chemo/radiotherapy, the expression of PD-L1 molecules in tumor cells and the frequency of CD8+ TILs were significantly increased (105). This study suggested that using PD-L1 monoclonal antibody can improve the effects of this treatment.
Collectively, it can be concluded that radiotherapy alone or in combination with chemotherapy or immune checkpoints causes an increase in the number of TILs. As a result, it changes the immunosuppressive space to the antitumoral space and, consequently, increases the survival of patients.
The immunosuppressive nature of the TME caused by its components, including Tregs, M2 macrophages, and MDSCs, leads to the inactivation of TILs in vivo (205). Additionally, co-inhibitory molecules and signals in the TME promote angiogenesis and suppress antitumor T cell responses (113). Activated T cells express multiple co-inhibitory receptors, including LAG3, B and T lymphocyte attenuator (BTLA), CTLA-4, and PD- 1 (113). On the other hand, MDSCs and Tregs, through producing molecules such as TGF-β, IL-10, IL-35, and IDO, can promote the expression of co-inhibitory molecules on TILs (1, 113). Therefore, the blockade of co-inhibitory immune checkpoints could be an effective strategy to improve TIL infiltration and function.
Anti-PD1 is a frequent ICI combined with TIL therapy. Patients with metastatic cervical cancer with low microsatellite instability and negative PD-L1 were examined for anti-PD1 plus TIL therapy (14). Their findings demonstrated that the prognosis for metastatic cervical cancer is greatly improved by combining TILs and anti-PD1. It has already been shown that increased expression of PD-L1 on tumor and immune cells. The high microsatellite instability is associated with favorable responses to immunotherapy (206). In another study, researchers investigated whether TILs plus anti-PD1 improve the prognosis of patients with chemotherapy-resistant metastatic osteosarcoma. They reported that combined therapy was safe and improved the efficacy of TIL therapy. Besides, all treatment-related adverse events were reversible or manageable (190).
A study showed that combining TILs with ipilimumab in metastatic melanoma improved antitumor responses and increased survival time (191). In a phase I/II clinical trial, the co-administration of ipilimumab and nivolumab in combination with TIL therapy in different solid tumors was studied. Ipilimumab was administered before tumor resection, and nivolumab was used along with TIL infusion. Preconditioning chemotherapy was given before TIL infusion, followed by a low-dose stimulation with IL-2. They showed that adding ICIs before and during TIL infusion with low-dose IL-2 resulted in manageable toxicity and sizeable tumor regressions (51).
Taken together, TIL therapy combined with ICIs is more beneficial than monotherapy, leading to lower toxicity, improved prognosis, increased survival rate, and enhanced antitumor responses.
Oncolytic viruses induce immunogenic cell death and indirectly increase T cells’ effectiveness through manipulating the immunosuppressive condition of the TME, releasing TAAs, inflammatory cytokines, and chemokine. In addition, oncolytic viruses directly lysis and destroy tumor cells (207, 208). In a unique design, Haminki et al. investigated the dual administration of TIL and virus in immunocompromised animals to induce virus-mediated tumor lysis along with enhanced TIL infiltration. They showed increased infiltration of TILs coupled with tumor regression and enhanced antitumor activity following combination therapy compared to the controls (192).
In another study, herpes simplex virus 1 (HSV-1), an oncolytic virus, was engineered to express OX40L and IL-12 (OV-OX40L/IL12). Infection of tumor cells with the engineered virus provided activation signals for T cells. Co-culture of the virus-infected cells with TILs, upregulation of MHC I, MHC II, and costimulatory receptors, such as CD80 and CD86 on tumor cells and increased the IFN-γ-secreting cells, leading to delayed tumor growth and improved survival time (193). In another approach, local administration of oncolytic poxvirus enhanced the activity of tumor-specific TILs by altering the immunosuppressive TME to an immunogenic milieu, leading to a significant reduction in tumor size and enhanced survival in mice harboring MC38 tumors (194). Determining the ideal oncolytic virus in each tumor type is necessary, and efforts to achieve this goal are ongoing. Studies on mouse models of tumors suggest that adenovirus might be the most effective virus for enhancing TIL therapy. Adenovirus, vaccinia virus, HSV, and reovirus are four oncolytic viruses currently being evaluated in clinical trials. Focus on virus engineering by arming with transgenes can provide potent antitumor effects, especially in combinational therapies (195).
Cancer neoantigens, the product of chromosomal changes, have unique amino acid sequences capable of inducing a potent and long-lasting immunological response. A high mutational and neoantigen burden in melanoma patients receiving TIL is substantially linked to increased PFS and OS (196). For patients with advanced solid tumors, therapeutic targeting of neoantigens using either ACT or vaccines has shown some early promise (209). At the National Cancer Institute, scientists have developed an approach to identify applicable immunogenic mutations by directly presentation of the tumor’s mutation profile to the patient’s APC, creating a renewable target for testing TILs’ reactivity. They showed immune recognition of the mutated intracellular proteins by patients’ TILs in metastatic melanoma (197). Taken together, the combination of genomics and cellular immunotherapy permits the identification of somatic alterations and the prediction of potential neoantigens that could be utilized as targets in cancer vaccines and ACT with TILs or engineered T cells (210).
DCs are professional APCs that can be employed as powerful inducers of tumor-specific immune responses in cancer vaccines. Monocytes can be used to generate DCs in vitro, which can then be transfected with RNA encoding tumor-specific epitopes or pulsed with proteins, peptides, or whole-tumor lysates (211). There is a justification for mixing ACT and DC vaccines. Poschke et al. showed the viability and safety of this treatment strategy in a pilot phase I clinical study combining whole-tumor lysate DC vaccination with TILs in eight recruited metastatic melanoma patients (212).
An improved protocol for producing DC vaccines could induce more robust IL-12 production and T cell activation (198). Five patients received TIL therapy alone in an initial cohort to evaluate vaccine safety and optimize TIL expansion protocol. Five other patients received TILs combined with autologous tumor lysate-loaded DC vaccine in the second cohort. All patients received cyclophosphamide/fludarabine preconditioning and intravenous IL-2 after TIL transfer. In the safety/optimization cohort, all patients had a mixed response or stable disease, but none were durable. In the combination cohort, some patients experienced complete responses while others had partial responses.
In summary, they reported clinical responses by TIL therapy combined with DC vaccination in all treated metastatic melanoma patients who previously failed with ICIs (199). Using a modified DC vaccine, the authors investigated the efficacy of combined DC vaccination with CD40 agonistic antibodies in immune-competent mice with PDAC (213). Mice were vaccinated with syngeneic bone marrow-derived DCs loaded with either pancreatic cancer (in KPC mice) or mesothelioma (in AE17 mice) lysate and consequently treated with FGK45 as a CD40 agonist. Mesothelioma-lysate-loaded DCs combined with CD40 agonist-induced tumor growth reduction and improved survival time rather than anti-CD40 alone.
Together, combination therapies with TIL are more successful than TIL monotherapy in decreasing tumor development, improving patients’ clinical conditions and survival, and lowering adverse effects.
Although clinical trials have shown that polyclonal tumor-reactive T cells can mediate antitumor responses and are effective in patients with metastatic tumors, most patients did not experience a successful outcome (214).
Several key factors influence treatment efficacy with polyclonal TILs. TIL phenotype profoundly affects the efficacy of the anticancer response. Pre-selection of CD39- CD69- T cells exhibiting a stem-like phenotype with self-renewal and proliferation capacities, resulting in effective antitumor responses compared to terminally differentiated T cells (215). Therefore, a potential approach that promotes the stemness phenotype could enhance the antitumor potency. Also, inhibition of metabolism/anabolism, such as glycolysis and amino acid synthesis and acquisition, and blocking the signaling cascades that promote cell differentiation and growth, such as the PI3K/AKT/mTOR and MAPK pathways, may enhance T cell stemness and antitumor potency (216, 217). Another approach that enhances the survival and performance of traditional TIL therapy in cancer patients, when T cells are already highly differentiated with lacking stemness potential, is next-generation strategies (214). Such strategies include gene-editing technologies such as CRISPR and transcription-activator-like effector nuclease (TALEN) to genetically transfer and permanently modify the polyclonal TILs, to overexpress an interesting gene by viral transduction or knockout of the target gene (164). PDCD1 gene knockout of the TILs using CRISPR-Cas9 gene editing and TALEN technology prevents its binding to PD-L1 in the TME and increases TILs functionality (163). PD-1 knockout in TILs is also an alternative to combining TIL-based therapy with systemic ICIs, significantly reducing the unwanted side effects and toxicity associated with systemic ICIs (218). Another CRISPR approach that can be exploited to enhance T cell effector function is using CRISPR/Cas9 gene editing to abolish the expression of GATA3 transcription factors. GATA3 is highly expressed in CD8+ TIM3+ TILs and is involved in T cell dysfunction and inhibition of IFN-γ and IL-2 production upon stimulation (219). Also, CRISPR-mediated deletion of TILs cytokine-induced SH2 (CISH) gene leads to favorable outcomes and actively inhibits TCR signaling in CD8+ T cells (220).
In the clinic, the main focus of next-generation TIL is on engineering TIL to overexpress cytokines such as IL-2 and IL-12. T cell genetic modifications to secrete cytokines or express tethered cytokines can increase the antitumor activity and the lifespan of TILs by maintaining high cytokine levels preferentially at the tumor site. In addition, avoiding the systemic side effects of IL-2 administration during TIL treatment is necessary for T cells’ survival. TILs transduced with the gene encoding recombinant IL-2 showed promising results in vitro. Six of eight transduced patient samples produced IL-2 upon autologous tumor stimulation and survived longer than non-transduced TILs. Unfortunately, in clinical trials, poor in vivo responses were inconsistent with in vitro findings (221). IL-12 is a vital cytokine in perpetuating Th1 antitumor responses. TIL transduced with IL-12 under the regulation of nuclear factor of activated T cells (NFAT)-inducible promoter revealed favorable clinical effects in a phase I trial (222). Another manipulation that improves TIL therapy and is currently in clinical trials at MD Anderson Cancer Center (NCT01740557) is CXCR2 retrovirally transduced TILs. This TIL chemotactically localizes the tumor and ensures that the infused cells are localized to the tumor sites (223).
The high diversity of TCR, excellent ability to infiltrate into the tumor site, and low toxicity of TILs are the advantages of TIL therapy over other ACTs. TIL therapy is generally performed as a second-line treatment. The number of clinical trials on TIL therapy is increasing. Melanoma is still at the top of the number of TIL therapy clinical trials, followed by NSCLC, ovarian, and head and neck cancers. The success of two TIL products, LN144 and LN145, by Iovance in 2018 has paved the way for commercializing TIL therapy. However, TIL therapy still faces serious challenges. The most widespread method of TIL production is to isolate it from tumor tissue and then expand it in vitro. The process of selected TIL production usually takes 6-8 weeks. This long period causes TIL exhaustion. Besides, patients might be unable to wait for such a long time. Preparing young TILs without selection with antitumor reactivity is much faster than selecting the TILs. However, their tumor reactivity is questionable.
Additionally, the immunosuppressive mechanisms in the TME limit the TIL function. Also, injection of high-dose IL-2 as a standard method to support the growth and activity of injected TILs has several adverse effects. Combining TIL therapy with ICIs, modified cytokines (superkine), cancer vaccines, and next-generation TILs could minimize the limitations and maximize the efficacy of TIL therapy. Finally, developing multi-omics and sequencing techniques could help us set up a standard platform for rapidly expanding and selecting tumor-reactive TILs for each patient as personalized immunotherapy.
MK. Conceived and designed the study, drafted the manuscript; MS. Contributed in manuscript drafting and critically evaluate the article; AN. Prepared Table 2 and contributed in manuscript drafting; AR. Participated in the manuscript drafting and revision; ZB. Prepared Table 1 and contributed in manuscript drafting; HK. Contributed in study design and manuscript drafting, prepared figures, revised the manuscript; RF. Designed and supervised the project, revised and finalized the manuscript; All authors contributed to the article and approved the submitted version.
Iran University of Medical Sciences supported this study.
The authors declare that the research was conducted in the absence of any commercial or financial relationships that could be construed as a potential conflict of interest.
All claims expressed in this article are solely those of the authors and do not necessarily represent those of their affiliated organizations, or those of the publisher, the editors and the reviewers. Any product that may be evaluated in this article, or claim that may be made by its manufacturer, is not guaranteed or endorsed by the publisher.
1. Kazemi MH, Barough MS, Ghanavatinejad A, Momeni-Varposhti Z, Khorrami S, Sadeghi B, et al. Decrease of tumor-infiltrating regulatory T cells using pentoxifylline: An ex vivo analysis in triple-negative breast cancer mouse model. Iranian J Allergy Asthma Immunol (2022) 21:1–11. doi: 10.18502/ijaai.v21i2.9224
2. Savas P, Salgado R, Denkert C, Sotiriou C, Darcy PK, Smyth MJ, et al. Clinical relevance of host immunity in breast cancer: From tils to the clinic. Nat Rev Clin Oncol (2016) 13(4):228–41. doi: 10.1038/nrclinonc.2015.215
3. Virchow R. Cellular pathology as based upon physiological and pathological histology. Nutr Rev (1863) 47:23–5. doi: 10.5962/bhl.title.32770
4. Eberlein TJ, Rosenstein M, Rosenberg SA. Regression of a disseminated syngeneic solid tumor by systemic transfer of lymphoid cells expanded in interleukin 2. J Exp Med (1982) 156(2):385–97. doi: 10.1084/jem.156.2.385
5. Rosenberg SA, Spiess P, Lafreniere R. A new approach to the adoptive immunotherapy of cancer with tumor-infiltrating lymphocytes. Science (1986) 233(4770):1318–21. doi: 10.1126/science.3489291
6. Rosenberg SA, Packard BS, Aebersold PM, Solomon D, Topalian SL, Toy ST, et al. Use of tumor-infiltrating lymphocytes and interleukin-2 in the immunotherapy of patients with metastatic melanoma. New Engl J Med (1988) 319(25):1676–80. doi: 10.1056/NEJM198812223192527
7. Wang S, Sun J, Chen K, Ma P, Lei Q, Xing S, et al. Perspectives of tumor-infiltrating lymphocyte treatment in solid tumors. BMC Med (2021) 19(1):1–7. doi: 10.1186/s12916-021-02006-4
8. Roshandel E, Noorazar L, Farhadihosseinabadi B, Mehdizadeh M, Kazemi MH, Parkhideh S. Pi3 kinase signaling pathway in hematopoietic cancers: A glance in mirna’s role. J Clin Lab Anal (2021) 35(4):e23725. doi: 10.1002/jcla.23725
9. Majzner RG, Mackall CL. Clinical lessons learned from the first leg of the car T cell journey. Nat Med (2019) 25(9):1341–55. doi: 10.1038/s41591-019-0564-6
10. Titov A, Zmievskaya E, Ganeeva I, Valiullina A, Petukhov A, Rakhmatullina A, et al. Adoptive immunotherapy beyond car T-cells. Cancers (2021) 13:743. doi: 10.3390/cancers13040743
11. Fernandez-Poma SM, Salas-Benito D, Lozano T, Casares N, Riezu-Boj J-I, Mancheño U, et al. Expansion of tumor-infiltrating Cd8+ T cells expressing pd-1 improves the efficacy of adoptive T-cell Therapypd-1–selected tils improve the efficacy of act. Cancer Res (2017) 77(13):3672–84. doi: 10.1158/0008-5472.CAN-17-0236
12. Sun X, Zhai J, Sun B, Parra ER, Jiang M, Ma W, et al. Effector memory cytotoxic Cd3+/Cd8+/Cd45ro+ T cells are predictive of good survival and a lower risk of recurrence in triple-negative breast cancer. Modern Pathol (2022) 35(5):601–8. doi: 10.1038/s41379-021-00973-w
13. Dafni U, Michielin O, Lluesma SM, Tsourti Z, Polydoropoulou V, Karlis D, et al. Efficacy of adoptive therapy with tumor-infiltrating lymphocytes and recombinant interleukin-2 in advanced cutaneous melanoma: A systematic review and meta-analysis. Ann Oncol (2019) 30(12):1902–13. doi: 10.1093/annonc/mdz398
14. Yin H, Guo W, Sun X, Li R, Feng C, Tan Y. Tils and anti-Pd1 therapy: An alternative combination therapy for Pdl1 negative metastatic cervical cancer. J Immunol Res (2020) 2020:1–11. doi: 10.1155/2020/8345235
15. Jazaeri AA, Zsiros E, Amaria RN, Artz AS, Edwards RP, Wenham RM, et al. Safety and efficacy of adoptive cell transfer using autologous tumor infiltrating lymphocytes (Ln-145) for treatment of recurrent, metastatic, or persistent cervical carcinoma. Am Soc Clin Oncol (2019) 15:2538–2538. doi: 10.1016/j.ygyno.2018.04.274
16. Liu D, Heij LR, Czigany Z, Dahl E, Lang SA, Ulmer TF, et al. The role of tumor-infiltrating lymphocytes in cholangiocarcinoma. J Exp Clin Cancer Res (2022) 41(1):1–18. doi: 10.1186/s13046-022-02340-2
17. Creelan BC, Wang C, Teer JK, Toloza EM, Yao J, Kim S, et al. Tumor-infiltrating lymphocyte treatment for anti-Pd-1-Resistant metastatic lung cancer: A phase 1 trial. Nat Med (2021) 27(8):1410–8. doi: 10.1038/s41591-021-01462-y
18. Zacharakis N, Huq LM, Seitter SJ, Kim SP, Gartner JJ, Sindiri S, et al. Breast cancers are immunogenic: Immunologic analyses and a phase ii pilot clinical trial using mutation-reactive autologous lymphocytes. J Clin Oncol (2022) 40(16):1741–54. doi: 10.1200/JCO.21.02170
19. Zhen Y-H, Liu X-H, Yang Y, Li B, Tang J-L, Zeng Q-X, et al. Phase I/Ii study of adjuvant immunotherapy with sentinel lymph node T lymphocytes in patients with colorectal cancer. Cancer Immunol Immunother (2015) 64(9):1083–93. doi: 10.1007/s00262-015-1715-3
20. Borsetto D, Tomasoni M, Payne K, Polesel J, Deganello A, Bossi P, et al. Prognostic significance of Cd4+ and Cd8+ tumor-infiltrating lymphocytes in head and neck squamous cell carcinoma: A meta-analysis. Cancers (2021) 13(4):781. doi: 10.3390/cancers13040781
21. Park TS, Rosenberg SA, Morgan RA. Treating cancer with genetically engineered T cells. Trends Biotechnol (2011) 29(11):550–7. doi: 10.1016/j.tibtech.2011.04.009
22. Aoki Y, Takakuwa K, Kodama S, Tanaka K, Takahashi M, Tokunaga A, et al. Use of adoptive transfer of tumor-infiltrating lymphocytes alone or in combination with cisplatin-containing chemotherapy in patients with epithelial ovarian cancer. Cancer Res (1991) 51(7):1934–9.
23. Amaria RN, Vining DJ, Kopetz S, Overman MJ, Javle MM, Antonoff M, et al. Efficacy and safety of autologous expanded tumor infiltrating lymphocytes (Tils) in multiple solid tumors. Am Soc Clin Oncol (2022) 40:2536–2536. doi: 10.1200/JCO.2022.40.16_suppl.2536
24. Johnson CB, Win SY. Combination therapy with pd-1/Pd-L1 blockade: An overview of ongoing clinical trials. Oncoimmunology (2018) 7(4):e1408744. doi: 10.1080/2162402X.2017.1408744
25. Stevanović S, Helman SR, Wunderlich JR, Langhan MM, Doran SL, Kwong MLM, et al. A phase ii study of tumor-infiltrating lymphocyte therapy for human papillomavirus–associated epithelial cancerstil therapy for hpv-associated cancers. Clin Cancer Res (2019) 25(5):1486–93. doi: 10.1158/1078-0432.CCR-18-2722
26. Figlin RA, Thompson JA, Bukowski RM, Vogelzang NJ, Novick AC, Lange P, et al. Multicenter, randomized, phase iii trial of Cd8+ tumor-infiltrating lymphocytes in combination with recombinant interleukin-2 in metastatic renal cell carcinoma. J Clin Oncol (1999) 17(8):2521–. doi: 10.1200/JCO.1999.17.8.2521
27. Topalian SL, Solomon D, Avis FP, Chang AE, Freerksen DL, Linehan WM, et al. Immunotherapy of patients with advanced cancer using tumor-infiltrating lymphocytes and recombinant interleukin-2: A pilot study. J Clin Oncol (1988) 6(5):839–53. doi: 10.1200/JCO.1988.6.5.839
28. Kradin R, Lazarus D, Dubinett S, Gifford J, Grove B, Kurnick J, et al. Tumour-infiltrating lymphocytes and interleukin-2 in treatment of advanced cancer. Lancet (1989) 333(8638):577–80. doi: 10.1016/S0140-6736(89)91609-7
29. Figlin RA, Pierce WC, Kaboo R, Tso CL, Moldawer N, Gitlitz B, et al. Treatment of metastatic renal cell carcinoma with nephrectomy, interleukin-2 and cytokine-primed or Cd8 (+) selected tumor infiltrating lymphocytes from primary tumor. J Urol (1997) 158(3):740–5. doi: 10.1016/S0022-5347(01)64304-0
30. Thiounn N, Mathiot C, Flam T, Tartour E, Peyret C, Joyeux I, et al. Cd4 til (Tumor infiltrating lymphocytes) induce complete response in patients treated with il-2 (Interleukin-2). Prelim Study J D’urol (1994) 100(4):185–8.
31. Rosenberg SA, Yannelli JR, Yang JC, Topalian SL, Schwartzentruber DJ, Weber JS, et al. Treatment of patients with metastatic melanoma with autologous tumor-infiltrating lymphocytes and interleukin 2. JNCI: J Natl Cancer Inst (1994) 86(15):1159–66. doi: 10.1093/jnci/86.15.1159
32. Wu R, Forget M-A, Chacon J, Bernatchez C, Haymaker C, Chen JQ, et al. Adoptive T-cell therapy using autologous tumor-infiltrating lymphocytes for metastatic melanoma: Current status and future outlook. Cancer J (Sudbury Mass) (2012) 18(2):160. doi: 10.1097/PPO.0b013e31824d4465
33. Ellebaek E, Iversen TZ, Junker N, Donia M, Engell-Noerregaard L, Met Ö, et al. Adoptive cell therapy with autologous tumor infiltrating lymphocytes and low-dose interleukin-2 in metastatic melanoma patients. J Trans Med (2012) 10(1):1–12. doi: 10.1186/1479-5876-10-169
34. Haanen J, Carbonnel F, Robert C, Kerr K, Peters S, Larkin J, et al. Management of toxicities from immunotherapy: Esmo clinical practice guidelines for diagnosis, treatment and follow-up. Ann Oncol (2017) 28:iv119–iv42. doi: 10.1093/annonc/mdx225
35. Kumar A, Watkins R, Vilgelm AE. Cell therapy with tils: Training and taming T cells to fight cancer. Front Immunol (2021) 12:690499. doi: 10.3389/fimmu.2021.690499
36. Presotto D, Erdes E, Duong MN, Allard M, Regamey P-O, Quadroni M, et al. Fine-tuning of optimal tcr signaling in tumor-redirected Cd8 T cells by distinct tcr affinity-mediated mechanisms. Front Immunol (2017) 8:1564. doi: 10.3389/fimmu.2017.01564
37. Johnson LA, Morgan RA, Dudley ME, Cassard L, Yang JC, Hughes MS, et al. Gene therapy with human and mouse T-cell receptors mediates cancer regression and targets normal tissues expressing cognate antigen. Blood J Am Soc Hematol (2009) 114(3):535–46. doi: 10.1182/blood-2009-03-211714
38. Bendle GM, Linnemann C, Hooijkaas AI, Bies L, de Witte MA, Jorritsma A, et al. Lethal graft-Versus-Host disease in mouse models of T cell receptor gene therapy. Nat Med (2010) 16(5):565–70. doi: 10.1038/nm.2128
39. Zhang C, Liu J, Zhong JF, Zhang X. Engineering car-T cells. biomark Res (2017) 5(1):1–6. doi: 10.1186/s40364-017-0102-y
40. Magalhaes I, Carvalho-Queiroz C, Hartana CA, Kaiser A, Lukic A, Mints M, et al. Facing the future: Challenges and opportunities in adoptive T cell therapy in cancer. Expert Opin Biol Ther (2019) 19(8):811–27. doi: 10.1080/14712598.2019.1608179
41. Mehrabadi AZ, Ranjbar R, Farzanehpour M, Shahriary A, Dorostkar R, Hamidinejad MA, et al. Therapeutic potential of car T cell in malignancies: A scoping review. Biomed Pharmacother (2022) 146:112512. doi: 10.1016/j.biopha.2021.112512
42. Tran KQ, Zhou J, Durflinger KH, Langhan MM, Shelton TE, Wunderlich JR, et al. Minimally cultured tumor-infiltrating lymphocytes display optimal characteristics for adoptive cell therapy. J Immunother (Hagerstown Md: 1997) (2008) 31(8):742. doi: 10.1097/CJI.0b013e31818403d5
43. Hopewell EL, Cox C, Pilon-Thomas S, Kelley LL. Tumor-infiltrating lymphocytes: Streamlining a complex manufacturing process. Cytotherapy (2019) 21(3):307–14. doi: 10.1016/j.jcyt.2018.11.004
44. Niitsuma M, Golub SH, Edelstein R, Holmes EC. Lymphoid cells infiltrating human pulmonary tumors: Effect of intralesional bcg injection. J Natl Cancer Inst (1981) 67(5):997–1003. doi: 10.1093/jnci/67.5.997
45. Malone CC, Schiltz PM, MacKintosh AD, Beutel LD, Heinemann FS, Dillman RO. Characterization of human tumor-infiltrating lymphocytes expanded in hollow-fiber bioreactors for immunotherapy of cancer. Cancer Biother Radiopharmaceut (2001) 16(5):381–90. doi: 10.1089/108497801753354285
46. Okcu MF, Wang RY, Bueso-Ramos C, Schober W, Weidner D, Andrassy R, et al. Flow cytometry and fluorescence in situ hybridization to detect residual neuroblastoma cells in bone marrow. Pediatr Blood Cancer (2005) 45(6):787–95. doi: 10.1002/pbc.20428
47. Sarasquete ME, García-Sanz R, González D, Martínez J, Mateo G, Martínez P, et al. Minimal residual disease monitoring in multiple myeloma: A comparison between allelic-specific oligonucleotide real-time quantitative polymerase chain reaction and flow cytometry. haematologica (2005) 90(10):1365–72. doi: 10.3324/%25x
48. van den Berg JH, Heemskerk B, van Rooij N, Gomez-Eerland R, Michels S, van Zon M, et al. Tumor infiltrating lymphocytes (Til) therapy in metastatic melanoma: Boosting of neoantigen-specific T cell reactivity and long-term follow-up. J Immunother Cancer (2020) 8(2):1–11. doi: 10.1136/jitc-2020-000848
49. Chu H, Du F, Gong Z, Lian P, Wang Z, Li P, et al. Better clinical efficiency of tils for malignant pleural effusion and ascites than cisplatin through intrapleural and intraperitoneal infusion. Anticancer Res (2017) 37(8):4587–91. doi: 10.21873/anticanres.11857
50. Quattrocchi KB, Miller CH, Cush S, Bernard SA, Dull ST, Smith M, et al. Pilot study of local autologous tumor infiltrating lymphocytes for the treatment of recurrent malignant gliomas. J Neuro-oncol (1999) 45(2):141–57. doi: 10.1023/A:1006293606710
51. Kverneland AH, Chamberlain CA, Borch TH, Nielsen M, Mørk SK, Kjeldsen JW, et al. Adoptive cell therapy with tumor-infiltrating lymphocytes supported by checkpoint inhibition across multiple solid cancer types. J Immunother Cancer (2021) 9(10):1–11. doi: 10.1136/jitc-2021-003499
52. Duc GHT. Melanoma: From early detection to treatment: BoD–books on demand. Florida, USA: Florida Medical Association, (2013).
53. Carlino MS, Larkin J, Long GV. Immune checkpoint inhibitors in melanoma. Lancet (2021) 398(10304):1002–14. doi: 10.1016/S0140-6736(21)01206-X
54. Dudley ME, Wunderlich JR, Robbins PF, Yang JC, Hwu P, Schwartzentruber DJ, et al. Cancer regression and autoimmunity in patients after clonal repopulation with antitumor lymphocytes. Science (2002) 298(5594):850–4. doi: 10.1126/science.1076514
55. Rosenberg SA, Yang JC, Sherry RM, Kammula US, Hughes MS, Phan GQ, et al. Durable complete responses in heavily pretreated patients with metastatic melanoma using T-cell transfer immunotherapycomplete regressions in melanoma. Clin Cancer Res (2011) 17(13):4550–7. doi: 10.1158/1078-0432.CCR-11-0116
56. Powell DJ Jr., Dudley ME, Robbins PF, Rosenberg SA. Transition of late-stage effector T cells to Cd27+ Cd28+ tumor-reactive effector memory T cells in humans after adoptive cell transfer therapy. Blood (2005) 105(1):241–50. doi: 10.1182/blood-2004-06-2482
57. Shen X, Zhou J, Hathcock KS, Robbins P, Powell DJ Jr., Rosenberg SA, et al. Persistence of tumor infiltrating lymphocytes in adoptive immunotherapy correlates with telomere length. J Immunother (Hagerstown Md: 1997) (2007) 30(1):123. doi: 10.1097/01.cji.0000211321.07654.b8
58. Dudley ME, Gross CA, Langhan MM, Garcia MR, Sherry RM, Yang JC, et al. Cd8+ enriched “Young” tumor infiltrating lymphocytes can mediate regression of metastatic Melanomacd8+ enriched young til. Clin Cancer Res (2010) 16(24):6122–31. doi: 10.1158/1078-0432.CCR-10-1297
59. Besser MJ, Shapira-Frommer R, Itzhaki O, Treves AJ, Zippel DB, Levy D, et al. Adoptive transfer of tumor-infiltrating lymphocytes in patients with metastatic melanoma: Intent-to-Treat analysis and efficacy after failure to prior immunotherapiesintent-to-Treat analysis of til act and impact of ipilimumab. Clin Cancer Res (2013) 19(17):4792–800. doi: 10.1158/1078-0432.CCR-13-0380
60. Sarnaik AA, Hamid O, Khushalani NI, Lewis KD, Medina T, Kluger HM, et al. Lifileucel, a tumor-infiltrating lymphocyte therapy, in metastatic melanoma. J Clin Oncol (2021) 39(24):2656–66. doi: 10.1200/JCO.21.00612
61. Haanen J, Rohaan M, Borch TH, van den Berg JH, Met Ö, Geukes Foppen M, et al. LBA3 Treatment with tumor-infiltrating lymphocytes (TIL) versus ipilimumab for advanced melanoma: Results from a multicenter, randomized phase III trial. Annals of Oncology (2022) 33:1406. doi: 10.1016/j.annonc.2022.08.036
62. Wills B, Brahmer JR, Naidoo J. Treatment of complications from immune checkpoint inhibition in patients with lung cancer. Curr Treat Options Oncol (2018) 19(9):1–21. doi: 10.1007/s11864-018-0562-9
63. Ben-Avi R, Farhi R, Ben-Nun A, Gorodner M, Greenberg E, Markel G, et al. Establishment of adoptive cell therapy with tumor infiltrating lymphocytes for non-small cell lung cancer patients. Cancer Immunol Immunother (2018) 67(8):1221–30. doi: 10.1007/s00262-018-2174-4
64. Lawrence MS, Stojanov P, Polak P, Kryukov GV, Cibulskis K, Sivachenko A, et al. Mutational heterogeneity in cancer and the search for new cancer-associated genes. Nature (2013) 499(7457):214–8. doi: 10.1038/nature12213
65. Kradin RL, Boyle LA, Preffer FI, Callahan RJ, Barlai-Kovach M, Strauss HW, et al. Tumor-derived interleukin-2-Dependent lymphocytes in adoptive immunotherapy of lung cancer. Cancer Immunol Immunother (1987) 24(1):76–85. doi: 10.1007/BF00199837
66. Quirk JT, Natarajan N. Ovarian cancer incidence in the united states, 1992–1999. Gynecol Oncol (2005) 97(2):519–23. doi: 10.1016/j.ygyno.2005.02.007
67. Lheureux S, Gourley C, Vergote I, Oza AM. Epithelial ovarian cancer. Lancet (2019) 393(10177):1240–53. doi: 10.1016/S0140-6736(18)32552-2
68. Borella F, Ghisoni E, Giannone G, Cosma S, Benedetto C, Valabrega G, et al. Immune checkpoint inhibitors in epithelial ovarian cancer: An overview on efficacy and future perspectives. Diagnostics (2020) 10(3):146. doi: 10.3390/diagnostics10030146
69. Freedman RS, Kudelka AP, Kavanagh JJ, Verschraegen C, Edwards CL, Nash M, et al. Clinical and biological effects of intraperitoneal injections of recombinant interferon-Γ and recombinant interleukin 2 with or without tumor-infiltrating lymphocytes in patients with ovarian or peritoneal carcinoma. Clin Cancer Res (2000) 6(6):2268–78.
70. Fujita K, Ikarashi H, Takakuwa K, Kodama S, Tokunaga A, Takahashi T, et al. Prolonged disease-free period in patients with advanced epithelial ovarian cancer after adoptive transfer of tumor-infiltrating lymphocytes. Clin Cancer Res (1995) 1(5):501–7.
71. Pedersen M, Westergaard MCW, Milne K, Nielsen M, Borch TH, Poulsen LG, et al. Adoptive cell therapy with tumor-infiltrating lymphocytes in patients with metastatic ovarian cancer: A pilot study. Oncoimmunology (2018) 7(12):e1502905. doi: 10.1080/2162402X.2018.1502905
72. Kverneland AH, Pedersen M, Westergaard MCW, Nielsen M, Borch TH, Olsen LR, et al. Adoptive cell therapy in combination with checkpoint inhibitors in ovarian cancer. Oncotarget (2020) 11(22):2092. doi: 10.18632/oncotarget.27604
73. Kroep J, Visser M, van der Minne L, de Bruin L, Roozen I, Meij P, et al. 729p adoptive T-cell therapy during chemotherapy with or without peginterferon-A (Ifnα) in patients with platinum sensitive recurrent epithelial ovarian cancer (Eoc). Ann Oncol (2021) 32:S732–S3. doi: 10.1016/j.annonc.2021.08.1172
74. Hinrichs CS, Restifo NP. Reassessing target antigens for adoptive T-cell therapy. Nat Biotechnol (2013) 31(11):999–1008. doi: 10.1038/nbt.2725
75. Ferris RL, Blumenschein G, Fayette J, Guigay J, Colevas AD, Licitra L, et al. Nivolumab for recurrent squamous-cell carcinoma of the head and neck. N Engl J Med (2016) 375:1856–67. doi: 10.1056/NEJMoa1602252
76. Kong CS, Narasimhan B, Cao H, Kwok S, Erickson JP, Koong A, et al. The relationship between human papillomavirus status and other molecular prognostic markers in head and neck squamous cell carcinomas. Int J Radiat Oncol Biol Phys (2009) 74(2):553–61. doi: 10.1016/j.ijrobp.2009.02.015
77. Berman TA, Schiller JT. Human papillomavirus in cervical cancer and oropharyngeal cancer: One cause, two diseases. Cancer (2017) 123(12):2219–29. doi: 10.1002/cncr.30588
78. O’Malley D, Lee S, Psyrri A, Sukari A, Thomas S, Wenham R, et al. 492 phase 2 efficacy and safety of autologous tumor-infiltrating lymphocyte (Til) cell therapy in combination with pembrolizumab in immune checkpoint inhibitor-naïve patients with advanced cancers. BMJ Specialist J (2021) 9:523–24. doi: 10.1136/jitc-2021-SITC2021.492
79. Li J, Chen Q-Y, He J, Li Z-L, Tang X-F, Chen S-P, et al. Phase I trial of adoptively transferred tumor-infiltrating lymphocyte immunotherapy following concurrent chemoradiotherapy in patients with locoregionally advanced nasopharyngeal carcinoma. Oncoimmunology (2015) 4(2):e976507. doi: 10.4161/23723556.2014.976507
80. Sistrunk WE, MacCarty WC. Life expectancy following radical amputation for carcinoma of the breast: A clinical and pathologic study of 218 cases. Ann Surg (1922) 75(1):61.
81. Barzaman K, Moradi-Kalbolandi S, Hosseinzadeh A, Kazemi MH, Khorramdelazad H, Safari E, et al. Breast cancer immunotherapy: Current and novel approaches. Int Immunopharmacol (2021) 98:107886. doi: 10.1016/j.intimp.2021.107886
82. Savas P, Virassamy B, Ye C, Salim A, Mintoff CP, Caramia F, et al. Single-cell profiling of breast cancer T cells reveals a tissue-resident memory subset associated with improved prognosis. Nat Med (2018) 24(7):986–93. doi: 10.1038/s41591-018-0078-7
83. Park SL, Buzzai A, Rautela J, Hor JL, Hochheiser K, Effern M, et al. Tissue-resident memory Cd8+ T cells promote melanoma–immune equilibrium in skin. Nature (2019) 565(7739):366–71. doi: 10.1038/s41586-018-0812-9
84. Su S, Liao J, Liu J, Huang D, He C, Chen F, et al. Blocking the recruitment of naive Cd4+ T cells reverses immunosuppression in breast cancer. Cell Res (2017) 27(4):461–82. doi: 10.1038/cr.2017.34
85. Song H, Liu A, Liu G, Wu F, Li Z. T Follicular regulatory cells suppress tfh-mediated b cell help and synergistically increase il-10-Producing b cells in breast carcinoma. Immunol Res (2019) 67(4):416–23. doi: 10.1007/s12026-019-09090-y
86. Sun J, Fan N, Zhang Y. Correlation between serum level of chemokine (Cc motif) ligand 18 and poor prognosis in breast cancer. Genet Mol Res (2016) 15(3):1–6. doi: 10.4238/gmr.15038632
87. Charych DH, Hoch U, Langowski JL, Lee SR, Addepalli MK, Kirk PB, et al. Nktr-214, an engineered cytokine with biased Il2 receptor binding, increased tumor exposure, and marked efficacy in mouse tumor models. Clin Cancer Res (2016) 22(3):680–90. doi: 10.1158/1078-0432.CCR-15-1631
88. Levin AM, Bates DL, Ring AM, Krieg C, Lin JT, Su L, et al. Exploiting a natural conformational switch to engineer an interleukin-2 ‘Superkine’. Nature (2012) 484(7395):529–33. doi: 10.1038/nature10975
89. Rech AJ, Mick R, Martin S, Recio A, Aqui NA, Powell DJ Jr., et al. Cd25 blockade depletes and selectively reprograms regulatory T cells in concert with immunotherapy in cancer patients. Sci Trans Med (2012) 4(134):134ra62–ra62. doi: 10.1126/scitranslmed.3003330
90. Maj T, Wang W, Crespo J, Zhang H, Wang W, Wei S, et al. Oxidative stress controls regulatory T cell apoptosis and suppressor activity and pd-L1-Blockade resistance in tumor. Nat Immunol (2017) 18(12):1332–41. doi: 10.1038/ni.3868
91. Qu Y, Zhang B, Liu S, Zhang A, Wu T, Zhao Y. 2-gy whole-body irradiation significantly alters the balance of Cd4+ Cd25– T effector cells and Cd4+ Cd25+ Foxp3+ T regulatory cells in mice. Cell Mol Immunol (2010) 7(6):419–27. doi: 10.1038/cmi.2010.45
92. Adams S, Othus M, Patel SP, Chae YK, Miller K, Chugh R, et al. Dual anti-Ctla-4 and anti-Pd-1 blockade in metaplastic carcinoma of the breast: Dart (Swog S1609, cohort 36). Am Soc Clin Oncol (2020) 38:1073–1073. doi: 10.1200/JCO.2020.38.15_suppl.1073
93. Björkström NK, Riese P, Heuts F, Andersson S, Fauriat C, Ivarsson MA, et al. Expression patterns of Nkg2a, kir, and Cd57 define a process of Cd56dim nk-cell differentiation uncoupled from nk-cell education. Blood J Am Soc Hematol (2010) 116(19):3853–64. doi: 10.1182/blood-2010-04-281675
94. Triki H, Charfi S, Bouzidi L, Kridis WB, Daoud J, Chaabane K, et al. Cd155 expression in human breast cancer: Clinical significance and relevance to natural killer cell infiltration. Life Sci (2019) 231:116543. doi: 10.1016/j.lfs.2019.116543
95. Beano A, Signorino E, Evangelista A, Brusa D, Mistrangelo M, Polimeni MA, et al. Correlation between nk function and response to trastuzumab in metastatic breast cancer patients. J Trans Med (2008) 6(1):1–10. doi: 10.1186/1479-5876-6-25
96. Knudson KM, Hicks KC, Alter S, Schlom J, Gameiro SR. Mechanisms involved in il-15 superagonist enhancement of anti-Pd-L1 therapy. J Immunother Cancer (2019) 7(1):1–16. doi: 10.1186/s40425-019-0551-y
97. Gillgrass AE, Chew MV, Krneta T, Ashkar AA. Overexpression of il-15 promotes tumor destruction Via Nk1. 1+ cells in a spontaneous breast cancer model. BMC Cancer (2015) 15(1):1–15. doi: 10.1186/s12885-015-1264-3
98. Li H-KH, Wu T-SE, Hsiao C-WS, Yang S-HS, Lee C-YS, Lin Y-LJ, et al. Ace1702: A potent and off-the-Shelf onk cell therapy product. Cancer Res (2020) 80(16_Supplement):2169–. doi: 10.1158/1538-7445.AM2020-2169
99. Coronella JA, Spier C, Welch M, Trevor KT, Stopeck AT, Villar H, et al. Antigen-driven oligoclonal expansion of tumor-infiltrating b cells in infiltrating ductal carcinoma of the breast. J Immunol (2002) 169(4):1829–36. doi: 10.4049/jimmunol.169.4.1829
100. Tao H, Lu L, Xia Y, Dai F, Wang Y, Bao Y, et al. Antitumor effector b cells directly kill tumor cells Via the Fas/Fasl pathway and are regulated by il-10. Eur J Immunol (2015) 45(4):999–1009. doi: 10.1002/eji.201444625
101. Arias-Pulido H, Cimino-Mathews A, Chaher N, Qualls C, Joste N, Colpaert C, et al. The combined presence of Cd20+ b cells and pd-L1+ tumor-infiltrating lymphocytes in inflammatory breast cancer is prognostic of improved patient outcome. Breast Cancer Res Treat (2018) 171(2):273–82. doi: 10.1007/s10549-018-4834-7
102. Gheybi MK, Farrokhi S, Ravanbod MR, Ostovar A, Mehrzad V, Nematollahi P. The correlation of Cd19+ Cd24+ Cd38+ b cells and other clinicopathological variables with the proportion of circulating tregs in breast cancer patients. Breast Cancer (2017) 24(6):756–64. doi: 10.1007/s12282-017-0775-y
103. Bodogai M, Lee Chang C, Wejksza K, Lai J, Merino M, Wersto RP, et al. Anti-Cd20 antibody promotes cancer escape Via enrichment of tumor-evoked regulatory b cells expressing low levels of Cd20 and Cd137l. Cancer Res (2013) 73(7):2127–38. doi: 10.1158/0008-5472.CAN-12-4184
104. Lee-Chang C, Bodogai M, Martin-Montalvo A, Wejksza K, Sanghvi M, Moaddel R, et al. Inhibition of breast cancer metastasis by resveratrol-mediated inactivation of tumor-evoked regulatory b cells. J Immunol (2013) 191(8):4141–51. doi: 10.4049/jimmunol.1300606
105. Yoneda K, Kuwata T, Kanayama M, Mori M, Kawanami T, Yatera K, et al. Alteration in tumoural pd-L1 expression and stromal Cd8-positive tumour-infiltrating lymphocytes after concurrent chemo-radiotherapy for non-small cell lung cancer. Br J Cancer (2019) 121(6):490–6. doi: 10.1038/s41416-019-0541-3
106. Lowenfeld L, Zaheer S, Oechsle C, Fracol M, Datta J, Xu S, et al. Addition of anti-estrogen therapy to anti-Her2 dendritic cell vaccination improves regional nodal immune response and pathologic complete response rate in patients with Erpos/Her2pos early breast cancer. Oncoimmunology (2017) 6(9):e1207032. doi: 10.1080/2162402X.2016.1207032
107. Wimberly H, Brown JR, Schalper K, Haack H, Silver MR, Nixon C, et al. Pd-L1 expression correlates with tumor-infiltrating lymphocytes and response to neoadjuvant chemotherapy in breast cancerpd-L1 and response to neoadjuvant therapy in breast cancer. Cancer Immunol Res (2015) 3(4):326–32. doi: 10.1158/2326-6066.CIR-14-0133
108. Cortés J, Lipatov O, Im S-A, Gonçalves A, Lee K, Schmid P, et al. Keynote-119: Phase iii study of pembrolizumab (Pembro) versus single-agent chemotherapy (Chemo) for metastatic triple negative breast cancer (Mtnbc). Ann Oncol (2019) 30:v859–v60. doi: 10.1093/annonc/mdz394.010
109. Zou Y, Zou X, Zheng S, Tang H, Zhang L, Liu P, et al. Efficacy and predictive factors of immune checkpoint inhibitors in metastatic breast cancer: A systematic review and meta-analysis. Ther Adv Med Oncol (2020) 12:1758835920940928. doi: 10.1177/1758835920940928
110. Heeke AL, Tan AR. Checkpoint inhibitor therapy for metastatic triple-negative breast cancer. Cancer Metastasis Rev (2021) 40(2):537–47. doi: 10.1007/s10555-021-09972-4
111. Narayan P, Wahby S, Gao JJ, Amiri-Kordestani L, Ibrahim A, Bloomquist E, et al. Fda approval summary: Atezolizumab plus paclitaxel protein-bound for the treatment of patients with advanced or metastatic tnbc whose tumors express pd-L1fda approval: Atezolizumab plus paclitaxel protein-bound. Clin Cancer Res (2020) 26(10):2284–9. doi: 10.1158/1078-0432.CCR-19-3545
112. Bamdad CC, Stewart AK, Huang P, Smagghe BJ, Moe ST, Swanson TE, et al. Abstract P3-11-11: First-in-Human car T for solid tumors targets the Muc1 transmembrane cleavage product. Cancer Res (2020) 80(4_Supplement):P3-11–P3–. doi: 10.1158/1538-7445.SABCS19-P3-11-11
113. Kazemi MH, Najafi A, Karami J, Ghazizadeh F, Yousefi H, Falak R, et al. Immune and metabolic checkpoints blockade: Dual wielding against tumors. Int Immunopharmacol (2021) 94:107461. doi: 10.1016/j.intimp.2021.107461
114. Barzaman K, Karami J, Zarei Z, Hosseinzadeh A, Kazemi MH, Moradi-Kalbolandi S, et al. Breast cancer: Biology, biomarkers, and treatments. Int Immunopharmacol (2020) 84:106535. doi: 10.1016/j.intimp.2020.106535
115. Kazemi MH, Raoofi Mohseni S, Hojjat-Farsangi M, Anvari E, Ghalamfarsa G, Mohammadi H, et al. Adenosine and adenosine receptors in the immunopathogenesis and treatment of cancer. J Cell Physiol (2018) 233(3):2032–57. doi: 10.1002/jcp.25873
116. Mlecnik B, Tosolini M, Kirilovsky A, Berger A, Bindea G, Meatchi T, et al. Histopathologic-based prognostic factors of colorectal cancers are associated with the state of the local immune reaction. J Clin Oncol (2011) 29(6):610–8. doi: 10.1200/JCO.2010.30.5425
117. Zhao Y, Ge X, He J, Cheng Y, Wang Z, Wang J, et al. The prognostic value of tumor-infiltrating lymphocytes in colorectal cancer differs by anatomical subsite: A systematic review and meta-analysis. World J Surg Oncol (2019) 17(1):1–11. doi: 10.1186/s12957-019-1621-9
118. Barzaman K, Vafaei R, Samadi M, Kazemi MH, Hosseinzadeh A, Merikhian P, et al. Anti-cancer therapeutic strategies based on Hgf/Met, epcam, and tumor-stromal cross talk. Cancer Cell Int (2022) 22(1):1–20. doi: 10.1186/s12935-022-02658-z
119. Ko YS, Pyo J-S. Clinicopathological significance and prognostic role of tumor-infiltrating lymphocytes in colorectal cancer. Int J Biol Markers (2019) 34(2):132–8. doi: 10.1177/1724600818817320
120. Väyrynen J, Tuomisto A, Klintrup K, Mäkelä J, Karttunen T, Mäkinen MJ. Detailed analysis of inflammatory cell infiltration in colorectal cancer. Br J Cancer (2013) 109(7):1839–47. doi: 10.1038/bjc.2013.508
121. Zou Q, Hu B, Yu H, Ren D. Characteristics of Cd8+ T cell infiltration in colorectal cancer and their correlation with prognosis. Chin J Dig Dis (2021) 24(12):1086–92. doi: 10.3760/cma.j.cn441530-20210402-00144
122. Akiyoshi T, Gotoh O, Tanaka N, Kiyotani K, Yamamoto N, Ueno M, et al. -cell complexity and density are associated with sensitivity to neoadjuvant chemoradiotherapy in patients with rectal cancer. T (2021) 70(2):509–18. doi: 10.1007/s00262-020-02705-6
123. Yao W, He J-c, Yang Y, Wang J-m, Qian Y-w, Yang T, et al. The prognostic value of tumor-infiltrating lymphocytes in hepatocellular carcinoma: A systematic review and meta-analysis. Sci Reps (2017) 7(1):1–11. doi: 10.1038/s41598-017-08128-1
124. Lee WS, Park S, Lee WY, Yun SH, Chun HK. Clinical impact of tumor-infiltrating lymphocytes for survival in stage ii colon cancer. Cancer (2010) 116(22):5188–99. doi: 10.1002/cncr.25293
125. Nosho K, Baba Y, Tanaka N, Shima K, Hayashi M, Meyerhardt JA, et al. Tumour-infiltrating T-cell subsets, molecular changes in colorectal cancer, and prognosis: Cohort study and literature review. J Pathol (2010) 222(4):350–66. doi: 10.1002/path.2774
126. Berntsson J, Nodin B, Eberhard J, Micke P, Jirström K. Prognostic impact of tumour-infiltrating b cells and plasma cells in colorectal cancer. Int J Cancer (2016) 139(5):1129–39. doi: 10.1002/ijc.30138
127. Maletzki C, Jahnke A, Ostwald C, Klar E, Prall F, Linnebacher M. Ex-vivo clonally expanded b lymphocytes infiltrating colorectal carcinoma are of mature immunophenotype and produce functional igg. PLOS ONE (2012) 7(2):. doi: 10.1371/journal.pone.0032639
128. Edin S, Kaprio T, Hagström J, Larsson P, Mustonen H, Böckelman C, et al. The prognostic importance of Cd20+ b lymphocytes in colorectal cancer and the relation to other immune cell subsets. Sci Refs (2019) 9(1):1–9. doi: 10.1038/s41598-019-56441-8
129. Wang H, Tian T, Zhang J. Tumor-associated macrophages (Tams) in colorectal cancer (Crc): From mechanism to therapy and prognosis. Int J Mol Sci (2021) 22(16):8470. doi: 10.3390/ijms22168470
130. Rodrigo BN, Viganò S, Gannon P, Baumgartner P, Maisonneuve C, Sempoux C, et al. Comprehensive assessment of the feasibility of adoptive cell therapy in colorectal carcinoma. Ann Oncol (2016) 27:vi372. doi: 10.1093/annonc/mdw378.42
131. Karlsson M, Marits P, Dahl K, Dagöö T, Enerbäck S, Thörn M, et al. Pilot study of sentinel-Node-Based adoptive immunotherapy in advanced colorectal cancer. Ann Surg Oncol (2010) 17(7):1747–57. doi: 10.1245/s10434-010-0920-8
132. Gardini A, Ercolani G, Riccobon A, Ravaioli M, Ridolfi L, Flamini E, et al. Adjuvant, adoptive immunotherapy with tumor infiltrating lymphocytes plus interleukin-2 after radical hepatic resection for colorectal liver metastases: 5-year analysis. J Surg Oncol (2004) 87(1):46–52. doi: 10.1002/jso.20066
133. Tran E, Robbins PF, Lu Y-C, Prickett TD, Gartner JJ, Jia L, et al. T-Cell transfer therapy targeting mutant kras in cancer. N England J Med (2016) 375(23):2255–62. doi: 10.1056/NEJMoa1609279
134. Hiraoka N. Tumor-infiltrating lymphocytes and hepatocellular carcinoma: Molecular biology. Int J Clin Oncol (2010) 15(6):544–51. doi: 10.1007/s10147-010-0130-1
135. O’Leary K. T Cell drivers in Nash-hcc. Nature Reviews Cancer (2021) 21(6):341–. doi: 10.1038/s41568-021-00362-0
136. Fu J, Zhang Z, Zhou L, Qi Z, Xing S, Lv J, et al. Impairment of Cd4+ cytotoxic T cells predicts poor survival and high recurrence rates in patients with hepatocellular carcinoma. Hepatology (2013) 58(1):139–49. doi: 10.1002/hep.26054
137. Katz SC, Bamboat ZM, Maker AV, Shia J, Pillarisetty VG, Yopp AC, et al. Regulatory T cell infiltration predicts outcome following resection of colorectal cancer liver metastases. Ann Surg Oncol (2013) 20(3):946–55. doi: 10.1245/s10434-012-2668-9
138. Kalathil SG, Lugade AA, Miller A, Iyer R, Thanavala Y. Pd-1+ and Foxp3+ T cell reduction correlates with survival of hcc patients after sorafenib therapy. JCI insight (2016) 1(11):1–12. doi: 10.1172/jci.insight.86182
139. Zhang Z, Ma L, Goswami S, Ma J, Zheng B, Duan M, et al. Landscape of infiltrating b cells and their clinical significance in human hepatocellular carcinoma. Oncoimmunology (2019) 8(4):e1571388. doi: 10.1080/2162402X.2019.1571388
140. Shi J-Y, Gao Q, Wang Z-C, Zhou J, Wang X-Y, Min Z-H, et al. Margin-infiltrating Cd20+ b cells display an atypical memory phenotype and correlate with favorable prognosis in hepatocellular carcinomamargin-infiltrating b cells in hepatocellular carcinoma. Clin Cancer Res (2013) 19(21):5994–6005. doi: 10.1158/1078-0432.CCR-12-3497
141. Zhu L-Y, Zhou J, Liu Y-Z, Pan W-D. Prognostic significance of natural killer cell infiltration in hepatocellular carcinoma. Chin J Cancer (2009) 28(11):1198–202. doi: 10.5732/cjc.009.10284
142. Ju M-J, Qiu S-J, Fan J, Xiao Y-S, Gao Q, Zhou J, et al. Peritumoral activated hepatic stellate cells predict poor clinical outcome in hepatocellular carcinoma after curative resection. Am J Clin Pathol (2009) 131(4):498–510. doi: 10.1309/AJCP86PPBNGOHNNL
143. Liu Z, Liu X, Liang J, Liu Y, Hou X, Zhang M, et al. Immunotherapy for hepatocellular carcinoma: Current status and future prospects. Front Immunol (2021) 12:4165. doi: 10.3389/fimmu.2021.765101
144. Takayama T, Sekine T, Makuuchi M, Yamasaki S, Kosuge T, Yamamoto J, et al. Adoptive immunotherapy to lower postsurgical recurrence rates of hepatocellular carcinoma: A randomised trial. Lancet (2000) 356(9232):802–7. doi: 10.1016/S0140-6736(00)02654-4
145. Jiang S-S, Tang Y, Zhang Y-J, Weng D-S, Zhou Z-G, Pan K, et al. A phase I clinical trial utilizing autologous tumor-infiltrating lymphocytes in patients with primary hepatocellular carcinoma. Oncotarget (2015) 6(38):41339. doi: 10.18632/oncotarget.5463
146. Kang B, Seo A, Yoon S, Bae H, Jeon S, Kwon O, et al. Prognostic value of tumor-infiltrating lymphocytes in Epstein–Barr virus-associated gastric cancer. Ann Oncol (2016) 27(3):494–501. doi: 10.1093/annonc/mdv610
147. Hennequin A, Derangere V, Boidot R, Apetoh L, Vincent J, Orry D, et al. Tumor infiltration by tbet+ effector T cells and Cd20+ b cells is associated with survival in gastric cancer patients. Oncoimmunology (2016) 5(2):e1054598. doi: 10.1080/2162402X.2015.1054598
148. Solinas C, Pusole G, Demurtas L, Puzzoni M, Mascia R, Morgan G, et al. Tumor infiltrating lymphocytes in gastrointestinal tumors: Controversies and future clinical implications. Crit Rev Oncology/hematol (2017) 110:106–16. doi: 10.1016/j.critrevonc.2016.11.016
149. Hendry S, Salgado R, Gevaert T, Russell PA, John T, Thapa B, et al. Assessing tumor infiltrating lymphocytes in solid tumors: A practical review for pathologists and proposal for a standardized method from the international immuno-oncology biomarkers working group: Part 2: Tils in melanoma, gastrointestinal tract carcinomas, non-small cell lung carcinoma and mesothelioma, endometrial and ovarian carcinomas, squamous cell carcinoma of the head and neck, genitourinary carcinomas, and primary brain tumors. Adv Anatomic Pathol (2017) 24(6):311. doi: 10.1097/PAP.0000000000000161
150. Protti MP, De Monte L. Immune infiltrates as predictive markers of survival in pancreatic cancer patients. Front Physiol (2013) 4:210. doi: 10.3389/fphys.2013.00210
151. Hiraoka N, Ino Y, Yamazaki-Itoh R, Kanai Y, Kosuge T, Shimada K. Intratumoral tertiary lymphoid organ is a favourable prognosticator in patients with pancreatic cancer. Br J Cancer (2015) 112(11):1782–90. doi: 10.1038/bjc.2015.145
152. Miksch RC, Schoenberg MB, Weniger M, Bösch F, Ormanns S, Mayer B, et al. Prognostic impact of tumor-infiltrating lymphocytes and neutrophils on survival of patients with upfront resection of pancreatic cancer. Cancers (2019) 11(1):39. doi: 10.3390/cancers11010039
153. Shamohammadi FN, Yazdanifar M, Oraei M, Kazemi MH, Roohi A, Rezaei F, et al. Controversial role of Γδ T cells in pancreatic cancer. Int Immunopharmacol (2022) 108:108895. doi: 10.1016/j.intimp.2022.108895
154. De Jong R, Leffers N, Boezen H, Ten Hoor K, van der Zee A, Hollema H, et al. Presence of tumor-infiltrating lymphocytes is an independent prognostic factor in type I and ii endometrial cancer. Gynecol Oncol (2009) 114(1):105–10. doi: 10.1016/j.ygyno.2009.03.022
155. Workel HH, Komdeur FL, Wouters MC, Plat A, Klip HG, Eggink FA, et al. Cd103 defines intraepithelial Cd8+ Pd1+ tumour-infiltrating lymphocytes of prognostic significance in endometrial adenocarcinoma. Eur J Cancer (2016) 60:1–11. doi: 10.1016/j.ejca.2016.02.026
156. Son J, George GC, Nardo M, Krause KJ, Jazaeri AA, Biter AB, et al. Adoptive cell therapy in gynecologic cancers: A systematic review and meta-analysis. Gynecol Oncol (2022) 165:664–70. doi: 10.1016/j.ygyno.2022.03.013
157. Attig S, Hennenlotter J, Pawelec G, Klein G, Koch SD, Pircher H, et al. Simultaneous infiltration of polyfunctional effector and suppressor T cells into renal cell carcinomast lymphocytes in renal cell carcinoma. Cancer Res (2009) 69(21):8412–9. doi: 10.1158/0008-5472.CAN-09-0852
158. Bromwich E, McArdle P, Canna K, McMillan D, McNicol A, Brown M, et al. The relationship between T-lymphocyte infiltration, stage, tumour grade and survival in patients undergoing curative surgery for renal cell cancer. Br J Cancer (2003) 89(10):1906–8. doi: 10.1038/sj.bjc.6601400
159. Remark R, Alifano M, Cremer I, Lupo A, Dieu-Nosjean M-C, Riquet M, et al. Characteristics and clinical impacts of the immune environments in colorectal and renal cell carcinoma lung metastases: Influence of tumor origin in situ immune reaction in lung metastases. Clin Cancer Res (2013) 19(15):4079–91. doi: 10.1158/1078-0432.CCR-12-3847
160. Siddiqui SA, Frigola X, Bonne-Annee S, Mercader M, Kuntz SM, Krambeck AE, et al. Tumor-infiltrating Foxp3– Cd4+ Cd25+ T cells predict poor survival in renal cell carcinoma. Clin Cancer Res (2007) 13(7):2075–81. doi: 10.1158/1078-0432.CCR-06-2139
161. Shablak A, Hawkins RE, Rothwell DG, Elkord E. T Cell–based immunotherapy of metastatic renal cell carcinoma: Modest success and future perspectivet–cell immunotherapy of mrcc. Clin Cancer Res (2009) 15(21):6503–10. doi: 10.1158/1078-0432.CCR-09-1605
162. Baldan V, Griffiths R, Hawkins RE, Gilham DE. Efficient and reproducible generation of tumour-infiltrating lymphocytes for renal cell carcinoma. Br J Cancer (2015) 112(9):1510–8. doi: 10.1038/bjc.2015.96
163. Chamberlain CA, Bennett EP, Kverneland AH, Svane IM, Donia M, Met ÖJMT-O. Highly efficient pd-1-Targeted crispr-Cas9 for tumor-infiltrating lymphocyte-based adoptive T cell therapy. Mol Ther Oncolytics (2022) 24:417–28. doi: 10.1016/j.omto.2022.01.004
164. Singh N, Shi J, June CH, Ruella M. Genome-editing technologies in adoptive T cell immunotherapy for cancer. Curr Hematol Malig Rep (2017) 12(6):522–9. doi: 10.1007/s11899-017-0417-7
165. Ebelt K, Babaryka G, Figel AM, Pohla H, Buchner A, Stief CG, et al. Dominance of Cd4+ lymphocytic infiltrates with disturbed effector cell characteristics in the tumor microenvironment of prostate carcinoma. Prostate (2008) 68(1):1–10. doi: 10.1002/pros.20661
166. Yang Y, Attwood K, Bshara W, Mohler JL, Guru K, Xu B, et al. High intratumoral Cd8+ T-cell infiltration is associated with improved survival in prostate cancer patients undergoing radical prostatectomy. Prostate (2021) 81(1):20–8. doi: 10.1002/pros.24068
167. Zeigler-Johnson C, Morales KH, Lal P, Feldman M. The relationship between obesity, prostate tumor infiltrating lymphocytes and macrophages, and biochemical failure. PloS One (2016) 11(8):e0159109. doi: 10.1371/journal.pone.0159109
168. McArdle P, Canna K, McMillan D, McNicol A, Campbell R, Underwood M. The relationship between T-lymphocyte subset infiltration and survival in patients with prostate cancer. Br J Cancer (2004) 91(3):541–3. doi: 10.1038/sj.bjc.6601943
169. Yang Y, Attwood K, Versaggi C, Omilian A, Bshara W, Xu B, et al. Association of high Cd8+ tumor infiltrating lymphocytes at prostatectomy with improved survival of prostate cancer patients. Am Soc Clin Oncol (2018) 36:5068–5068. doi: 10.1200/JCO.2018.36.15_suppl.5068
170. Krpina K, Babarović E, Jonjić N. Correlation of tumor-infiltrating lymphocytes with bladder cancer recurrence in patients with solitary low-grade urothelial carcinoma. Virchows Archiv (2015) 467(4):443–8. doi: 10.1007/s00428-015-1808-6
171. Zhang Q, Hao C, Cheng G, Wang L, Wang X, Li C, et al. High Cd4+ T cell density is associated with poor prognosis in patients with non-Muscle-Invasive bladder cancer. Int J Clin Exp Pathol (2015) 8(9):11510.
172. Horn T, Laus J, Seitz A, Maurer T, Schmid S, Wolf P, et al. The prognostic effect of tumour-infiltrating lymphocytic subpopulations in bladder cancer. World J Urol (2016) 34(2):181–7. doi: 10.1007/s00345-015-1615-3
173. Wahlin S, Nodin B, Leandersson K, Boman K, Jirström K. Clinical impact of T cells, b cells and the pd-1/Pd-L1 pathway in muscle invasive bladder cancer: A comparative study of transurethral resection and cystectomy specimens. Oncoimmunology (2019) 8(11):e1644108. doi: 10.1080/2162402X.2019.1644108
174. Kirtane K, Elmariah H, Chung CH, Abate-Daga D. Adoptive cellular therapy in solid tumor malignancies: Review of the literature and challenges ahead. J Immunother Cancer (2021) 9(7):1–11. doi: 10.1136/jitc-2021-002723
175. Hershkovitz L, Schachter J, Treves AJ, Besser MJ. Focus on adoptive T cell transfer trials in melanoma. Clin Dev Immunol (2010) 2010:1–12. doi: 10.1155/2010/260267
176. Mills J, Darcy P, Gyorki DE. Adoptive cell therapy for melanoma. Melanoma Springer (2018) . p:549–65. doi: 10.1007/978-3-319-78310-9_34
177. Goff SL, Smith FO, Klapper JA, Sherry R, Wunderlich JR, Steinberg SM, et al. Tumor infiltrating lymphocyte therapy for metastatic melanoma: Analysis of tumors resected for til. J Immunother (Hagerstown Md: 1997) (2010) 33(8):840. doi: 10.1097/CJI.0b013e3181f05b91
178. Sim GC, Chacon J, Haymaker C, Ritthipichai K, Singh M, Hwu P, et al. Tumor-infiltrating lymphocyte therapy for melanoma: Rationale and issues for further clinical development. BioDrugs (2014) 28(5):421–37. doi: 10.1007/s40259-014-0097-y
179. Ye Q, Loisiou M, Levine BL, Suhoski MM, Riley JL, June CH, et al. Engineered artificial antigen presenting cells facilitate direct and efficient expansion of tumor infiltrating lymphocytes. J Trans Med (2011) 9(1):1–13. doi: 10.1186/1479-5876-9-131
180. Morgan RA, Chinnasamy N, Abate-Daga DD, Gros A, Robbins PF, Zheng Z, et al. Cancer regression and neurologic toxicity following anti-Mage-A3 tcr gene therapy. J Immunother (Hagerstown Md: 1997) (2013) 36(2):133. doi: 10.1007/s13181-021-00835-6
181. Prinz PU, Mendler AN, Masouris I, Durner L, Oberneder R, Noessner E. High dgk-A and disabled mapk pathways cause dysfunction of human tumor-infiltrating Cd8+ T cells that is reversible by pharmacologic intervention. J Immunol (2012) 188(12):5990–6000. doi: 10.4049/jimmunol.1103028
182. Krummel MF, Heath WR, Allison J. Differential coupling of second signals for cytotoxicity and proliferation in Cd8+ T cell effectors: Amplification of the lytic potential by B7. J Immunol (1999) 163(6):2999–3006.
183. Weng N-p, Akbar AN, Goronzy J. Cd28– T cells: Their role in the age-associated decline of immune function. Trends Immunol (2009) 30(7):306–12. doi: 10.1016/j.it.2009.03.013
184. Tirapu I, Huarte E, Guiducci C, Arina A, Zaratiegui M, Murillo O, et al. Low surface expression of B7-1 (Cd80) is an immunoescape mechanism of colon carcinoma. Cancer Res (2006) 66(4):2442–50. doi: 10.1158/0008-5472.CAN-05-1681
185. Afreen S, Dermime S. The immunoinhibitory B7-H1 molecule as a potential target in cancer: Killing many birds with one stone. Hematology/oncol Stem Cell Ther (2014) 7(1):1–17. doi: 10.1016/j.hemonc.2013.09.005
186. Guedan S, Posey AD Jr., Shaw C, Wing A, Da T, Patel PR, et al. Enhancing car T cell persistence through icos and 4-1bb costimulation. JCI Insight (2018) 3(1):1–17. doi: 10.1172/jci.insight.96976
187. Radvanyi LG. Tumor-infiltrating lymphocyte therapy: Addressing prevailing questions. Cancer J (2015) 21(6):450–64. doi: 10.1097/PPO.0000000000000162
188. Granhøj JS, Witness Præst Jensen A, Presti M, Met Ö, Svane IM, Donia M. Tumor-infiltrating lymphocytes for adoptive cell therapy: Recent advances, challenges, and future directions. Expert Opin Biol Ther (2022) 22(5):627–41. doi: 10.1080/14712598.2022.2064711
189. Shi J, Li M, Yang R. Tumor-infiltrating lymphocytes as a feasible adjuvant immunotherapy for osteosarcoma with a poor response to neoadjuvant chemotherapy. Immunotherapy (2020) 12(9):641–52. doi: 10.2217/imt-2020-0107
190. Zhou X, Wu J, Duan C, Liu Y. Retrospective analysis of adoptive til therapy plus anti-Pd1 therapy in patients with chemotherapy-resistant metastatic osteosarcoma. J Immunol Res (2020) 2020:1–12. doi: 10.1155/2020/7890985
191. Mullinax JE, Hall M, Prabhakaran S, Weber J, Khushalani N, Eroglu Z, et al. Combination of ipilimumab and adoptive cell therapy with tumor-infiltrating lymphocytes for patients with metastatic melanoma. Front Oncol (2018) 8:44. doi: 10.3389/fonc.2018.00044
192. Santos J, Heiniö C, Quixabeira D, Zafar S, Clubb J, Pakola S, et al. Systemic delivery of oncolytic adenovirus to tumors using tumor-infiltrating lymphocytes as carriers. Cells (2021) 10(5):978. doi: 10.3390/cells10050978
193. Ye K, Li F, Wang R, Cen T, Liu S, Zhao Z, et al. An armed oncolytic virus enhances the efficacy of tumor-infiltrating lymphocyte therapy by converting tumors to artificial antigen presenting cells in situ. Mol Ther (2022) 30:1–19. doi: 10.1016/j.ymthe.2022.06.010
194. Feist M, Zhu Z, Dai E, Ma C, Liu Z, Giehl E, et al. Oncolytic virus promotes tumor-reactive infiltrating lymphocytes for adoptive cell therapy. Cancer Gene Ther (2021) 28(1):98–111. doi: 10.1038/s41417-020-0189-4
195. Cervera-Carrascon V, Quixabeira DC, Havunen R, Santos JM, Kutvonen E, Clubb JH, et al. Comparison of clinically relevant oncolytic virus platforms for enhancing T cell therapy of solid tumors. Mol Therapy-Oncol (2020) 17:47–60. doi: 10.1016/j.omto.2020.03.003
196. Lauss M, Donia M, Harbst K, Andersen R, Mitra S, Rosengren F, et al. Mutational and putative neoantigen load predict clinical benefit of adoptive T cell therapy in melanoma. Nat Commun (2017) 8(1):1–11. doi: 10.1038/s41467-017-01460-0
197. Lu Y-C, Yao X, Crystal JS, Li YF, El-Gamil M, Gross C, et al. Efficient identification of mutated cancer antigens recognized by T cells associated with durable tumor regressions. Clin Cancer Res (2014) 20(13):3401–10. doi: 10.1158/1078-0432.CCR-14-0433
198. Lövgren T, Sarhan D, Truxová I, Choudhary B, Maas R, Melief J, et al. Enhanced stimulation of human tumor-specific T cells by dendritic cells matured in the presence of interferon-Γ and multiple toll-like receptor agonists. Cancer Immunol Immunother (2017) 66(10):1333–44. doi: 10.1007/s00262-017-2029-4
199. Lövgren T, Wolodarski M, Wickström S, Edbäck U, Wallin M, Martell E, et al. Complete and long-lasting clinical responses in immune checkpoint inhibitor-resistant, metastasized melanoma treated with adoptive T cell transfer combined with dc vaccination. Oncoimmunology (2020) 9(1):1792058. doi: 10.1080/2162402X.2020.1792058
200. Foley KC, Nishimura MI, Moore TV. Combination immunotherapies implementing adoptive T cell transfer for advanced-stage melanoma. Melanoma Res (2018) 28(3):171. doi: 10.1097/CMR.0000000000000436
201. Senapati S, Mahanta AK, Kumar S, Maiti P. Controlled drug delivery vehicles for cancer treatment and their performance. Signal Transduct Targeted Ther (2018) 3(1):1–19. doi: 10.1038/s41392-017-0004-3
202. Aoto K, Mimura K, Okayama H, Saito M, Chida S, Noda M, et al. Immunogenic tumor cell death induced by chemotherapy in patients with breast cancer and esophageal squamous cell carcinoma. Oncol Rep (2018) 39(1):151–9. doi: 10.3892/or.2017.6097
203. Koukourakis IM, Gkegka AG, Xanthopoulou E, Nanos C, Giatromanolaki A, Koukourakis MI. Prognostic and predictive relevance of tumor-infiltrating lymphocytes in squamous cell head–neck cancer patients treated with radical Radiotherapy/Chemo-radiotherapy. Curr Oncol (2022) 29(6):4274–84. doi: 10.3390/curroncol29060342
204. Kovács A, Stenmark Tullberg A, Werner Rönnerman E, Holmberg E, Hartman L, Sjöström M, et al. Effect of radiotherapy after breast-conserving surgery depending on the presence of tumor-infiltrating lymphocytes: A long-term follow-up of the Swebcg91rt randomized trial. J Clin Oncol (2019) 37(14):1179–87. doi: 10.1200/JCO.18.02157
205. Kodumudi KN, Siegel J, Weber AM, Scott E, Sarnaik AA, Pilon-Thomas S. Immune checkpoint blockade to improve tumor infiltrating lymphocytes for adoptive cell therapy. PloS One (2016) 11(4):e0153053. doi: 10.1371/journal.pone.0153053
206. Goodman AM, Kato S, Bazhenova L, Patel SP, Frampton GM, Miller V, et al. Tumor mutational burden as an independent predictor of response to immunotherapy in diverse cancerstmb predicts response to immunotherapy in diverse cancers. Mol Cancer Ther (2017) 16(11):2598–608. doi: 10.1158/1535-7163.MCT-17-0386
207. Cervera-Carrascon V, Quixabeira DC, Santos JM, Havunen R, Zafar S, Hemminki O, et al. Tumor microenvironment remodeling by an engineered oncolytic adenovirus results in improved outcome from pd-L1 inhibition. Oncoimmunology (2020) 9(1):1761229. doi: 10.1080/2162402X.2020.1761229
208. Kazemi MH, Dehaghi BK, Roshandel E, Parkhideh S, Mehdizadeh M, Salimi M, et al. Oncolytic virotherapy in hematopoietic stem cell transplantation. Hum Immunol (2021) 82(9):640–8. doi: 10.1016/j.humimm.2021.05.007
209. Ott PA, Dotti G, Yee C, Goff SL. An update on adoptive T-cell therapy and neoantigen vaccines. Am Soc Clin Oncol Educ Book (2019) 39:e70–e8. doi: 10.1200/EDBK_238001
210. Tran E, Robbins PF, Rosenberg SA. ‘Final common pathway’of human cancer immunotherapy: Targeting random somatic mutations. Nat Immunol (2017) 18(3):255–62. doi: 10.1038/ni.3682
211. Garg AD, Coulie PG, Van den Eynde BJ, Agostinis P. Integrating next-generation dendritic cell vaccines into the current cancer immunotherapy landscape. Trends Immunol (2017) 38(8):577–93. doi: 10.1016/j.it.2017.05.006
212. Poschke I, Lövgren T, Adamson L, Nyström M, Andersson E, Hansson J, et al. A phase I clinical trial combining dendritic cell vaccination with adoptive T cell transfer in patients with stage iv melanoma. Cancer Immunol Immunother (2014) 63(10):1061–71. doi: 10.1007/s00262-014-1575-2
213. Anderson EM, Thomassian S, Gong J, Hendifar A, Osipov A. Advances in pancreatic ductal adenocarcinoma treatment. Cancers (2021) 13(21):5510. doi: 10.3390/cancers13215510
214. Yamamoto TN, Kishton RJ, Restifo NP. Developing neoantigen-targeted T cell–based treatments for solid tumors. Nature Med (2019) 25(10):1488–99. doi: 10.1038/s41591-019-0596-y
215. Krishna S, Lowery FJ, Copeland AR, Bahadiroglu E, Mukherjee R, Jia L, et al. Stem-like Cd8 T cells mediate response of adoptive cell immunotherapy against human cancer. Science (2020) 370(6522):1328–34. doi: 10.1126/science.abb9847
216. Sukumar M, Liu J, Ji Y, Subramanian M, Crompton JG, Yu Z, et al. Inhibiting glycolytic metabolism enhances Cd8+ T cell memory and antitumor function. J Clin Investig (2013) 123(10):4479–88. doi: 10.1172/JCI69589
217. Johnson MO, Wolf MM, Madden MZ, Andrejeva G, Sugiura A, Contreras DC, et al. Distinct regulation of Th17 and Th1 cell differentiation by glutaminase-dependent metabolism. Cell (2018) 175(7):1780–95. e19. doi: 10.1016/j.cell.2018.10.001
218. Martins F, Sofiya L, Sykiotis GP, Lamine F, Maillard M, Fraga M, et al. Adverse effects of immune-checkpoint inhibitors: Epidemiology, management and surveillance. Nat Rev Clin Oncol (2019) 16(9):563–80. doi: 10.1038/s41571-019-0218-0
219. Singer M, Wang C, Cong L, Marjanovic ND, Kowalczyk MS, Zhang H, et al. A distinct gene module for dysfunction uncoupled from activation in tumor-infiltrating T cells. Cell (2016) 166(6):1500–11. e9. doi: 10.1016/j.cell.2016.08.052
220. Palmer DC, Webber BR, Patel Y, Johnson MJ, Kariya CM, Lahr WS, et al. Internal checkpoint regulates T cell neoantigen reactivity and susceptibility to Pd1 blockade. Med (2020) 3:682–704. doi: 10.1016/j.medj.2022.07.008
221. Heemskerk B, Liu K, Dudley ME, Johnson LA, Kaiser A, Downey S, et al. Adoptive cell therapy for patients with melanoma, using tumor-infiltrating lymphocytes genetically engineered to secrete interleukin-2. Hum Gene Ther (2008) 19(5):496–510. doi: 10.1089/hum.2007.0171
222. Zhang L, Morgan RA, Beane JD, Zheng Z, Dudley ME, Kassim SH, et al. Tumor-infiltrating lymphocytes genetically engineered with an inducible gene encoding interleukin-12 for the immunotherapy of metastatic melanomanfat–Il12 in til trial. Clin Cancer Res (2015) 21(10):2278–88. doi: 10.1158/1078-0432.CCR-14-2085
Keywords: combination therapy, immunotherapy, solid tumor, tumor-infiltrating lymphocyte (TIL), TIL therapy
Citation: Kazemi MH, Sadri M, Najafi A, Rahimi A, Baghernejadan Z, Khorramdelazad H and Falak R (2022) Tumor-infiltrating lymphocytes for treatment of solid tumors: It takes two to tango? Front. Immunol. 13:1018962. doi: 10.3389/fimmu.2022.1018962
Received: 14 August 2022; Accepted: 14 October 2022;
Published: 28 October 2022.
Edited by:
Anna Pasetto, Oslo University Hospital, NorwayReviewed by:
David Akhavan, University of Kansas Medical Center, United StatesCopyright © 2022 Kazemi, Sadri, Najafi, Rahimi, Baghernejadan, Khorramdelazad and Falak. This is an open-access article distributed under the terms of the Creative Commons Attribution License (CC BY). The use, distribution or reproduction in other forums is permitted, provided the original author(s) and the copyright owner(s) are credited and that the original publication in this journal is cited, in accordance with accepted academic practice. No use, distribution or reproduction is permitted which does not comply with these terms.
*Correspondence: Reza Falak, RmFsYWsuckBpdW1zLmFjLmly; Hossein Khorramdelazad, SC5raG9yYW1kZWxAcnVtcy5hYy5pcg==
Disclaimer: All claims expressed in this article are solely those of the authors and do not necessarily represent those of their affiliated organizations, or those of the publisher, the editors and the reviewers. Any product that may be evaluated in this article or claim that may be made by its manufacturer is not guaranteed or endorsed by the publisher.
Research integrity at Frontiers
Learn more about the work of our research integrity team to safeguard the quality of each article we publish.