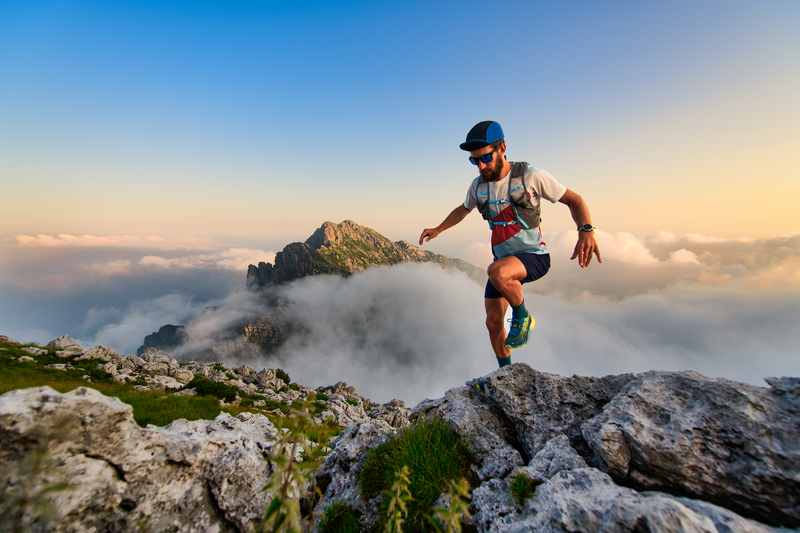
95% of researchers rate our articles as excellent or good
Learn more about the work of our research integrity team to safeguard the quality of each article we publish.
Find out more
REVIEW article
Front. Immunol. , 23 November 2022
Sec. Viral Immunology
Volume 13 - 2022 | https://doi.org/10.3389/fimmu.2022.1016304
This article is part of the Research Topic A year in review: Coronavirus infection in Humans and Viral Immunology View all 21 articles
The general immune state plays important roles against severe acute respiratory syndrome coronavirus 2 (SARS-CoV-2) infection. Cells of the immune system are encountering rapid changes during the acute phase of SARS-CoV-2-induced disease. Reduced fraction of functional CD8+ T cells, disrupted cross-talking between CD8+ T cells with dendritic cells (DCs), and impaired immunological T-cell memory, along with the higher presence of hyperactive neutrophils, high expansion of myeloid-derived suppressor cells (MDSCs) and non-classical monocytes, and attenuated cytotoxic capacity of natural killer (NK) cells, are all indicative of low efficient immunity against viral surge within the body. Immune state and responses from pro- or anti-inflammatory cells of the immune system to SARS-CoV-2 are discussed in this review. We also suggest some strategies to enhance the power of immune system against SARS-CoV-2-induced disease.
•Impaired pDCs and exhaustive T cells occur in severe SARS-CoV-2-induced disease.
•Tregs act differently in acute vs. chronic phase of the disease.
•MDSC expansion causes T-cell dysfunction in severe disease.
•Low Treg fraction causes more severe disease in adults vs. children.
•Co-adjuvant with O2 boosts immunity against SARS-CoV-2.
Severe acute respiratory syndrome coronavirus 2 (SARS-CoV-2) is a beta coronavirus that emerged first in China. SARS-CoV-2 is regarded as the etiological factor of coronavirus disease 2019 (COVID-19). The pandemic of SARS-CoV-2-induced disease is one of the current health issues causing a huge number of deaths worldwide (1). Clinical presentations show high variations in symptoms and severity of the disease (2). Patients with SARS-CoV-2-induced disease may be asymptomatic or show severe pneumonia (3). SARS-CoV-2-induced disease varies from mild to acute respiratory disease syndrome (ARDS), which is life threatening. Dysregulated immune response is behind the pathogenesis of ARDS (4).
An urgent priority is the understanding of protective responses from immune system against SARS-CoV-2 infection (1). SARS-CoV-2 induces unrestrained generation and secretion of different cytokines into the bloodstream. A consequence of this surge of inflammatory mediators is the systemic inflammation and dysregulation of responses from innate and adaptive immunity and further infiltration of different immune effector cells into various organ tissues. Overexpression of cytokines and exaggerated immune responses finally cause organ dysfunction and cytotoxicity (2). In this review, we aimed to discuss the immune state and responses from pro- or anti-inflammatory cells of the immune system to SARS-CoV-2 infection and suggest some potential strategies for strengthening the activity of immune system against this virus. Here, we have made all our effort for gathering and interpreting information about the position of immune cells in SARS-CoV-2-induced disease. We described controversies in this context and gave a reasonable answer to them. Referring also to our background knowledge over immune cells and immune state and conditions, such as hypoxia and its impact on cellular immunity in other diseases, we have made our interpretations more justifiable to the whole immune state affected by SARS-CoV-2 infection. The novelty of this study is the whole overview on cellular immune states and conditions affecting functionality of different immune cells in patients with SARS-CoV-2-induced disease.
SARS-CoV-2 is a single-strand RNA enveloped virus with a diameter of 40–60 nm and the largest genome compared with other RNA viruses (5). SARS-CoV-2 RNA encodes four structural proteins: membrane, nucleocapsid, envelope, and spike. The structural membrane, nucleocapsid, and envelope proteins are important for genomic stability and replication of the virus, and the spike protein includes S1 and S2 subunits that allow viral fusion to the membrane of target cells and its further cellular entry (6). S1 subunit contains a receptor-binding domain (RBD) for allowing engagement of the virus with angiotensin-converting enzyme 2 (ACE2) expressed on the surface of target cells, and the S2 subunit allows further viral fusion. Nasal epithelial cells, tracheal and bronchial epithelial cells, and type II pneumocytes highly express ACE2 and are locations for initial viral infection and spread (7). The S protein of the virus is the primary target for developing vaccines (1). However, there are also vaccines developed for the full inactivation of SARS-CoV-2 (8).
Complex interactions are occurring in cells of the immune system upon encountering SARS-CoV-2, a summary of which is presented in Figure 1.
Figure 1 Interactions among cells of the immune system in SARS-CoV-2-induced disease. SARS-CoV-2 antagonizes STAT1 phosphorylation, which is linked to the reduced immune activation in monocyte-derived dendritic cells (DCs). The impact of SARS-CoV-2 on DCs results in the production of autoantibodies against interferon (IFN)-1. Activation of CD8+ T cells is essential for virus clearance, and the activity of CD4+ T cells is important for the activation of CD8+ T cells and optimal antibody responses. IL-3 released from CD4+ and CD8+ T cells stimulates IFN-α, which is further linked to the higher fraction of plasmacytoid DCs (pDCs). The effector activity of T cells is suppressed by SARS-CoV-2 inducible effect on myeloid-derived suppressor cells (MDSCs) and further release of ILs 6 and 10 from the cells. IL-6 stimulatory effect on Notch4 expression from regulatory T cells (Tregs) contributed to severe lung inflammation via hampering release of amphiregulin. Granulocytic (G)-MDSCs also suppress the proliferation of T cells through the release of arginase-1 (ARG-1), which contributed to lyphocytopenia. ARG-1 activity and the resultant low L-arginine level contributed to platelet activation and thrombosis. In addition, MDSCs block IFN-γ production from T cells through releasing transforming growth factor (TGF)-β.
DCs are a heterogeneous population and the most potent antigen-presenting cells (APCs) of the immune system, and their activity is important for supporting innate immunity and activation of adaptive responses from CD4+ and CD8+ T cells (7, 9). DCs present two functional subtypes: conventional (or myeloid) (cDCs) and plasmacytoid (pDCs). cDCs further include cDC1 (CD141+) and cDC2 (CD1c+) subsets (9). CD141+ DCs are localized in the vascular wall and mucosa and are involved in the activation of responses from T-helper 1 (Th1) cells (5). CD1c+ DCs support responses from CD4+ T cells. CD1c+ DCs stimulate the activity of follicular helper T (Tfh) cells, which are known as the key promoter of humoral adaptive immunity against viral infections (9). Another subset is also considered for cDCs, which is the monocyte-derived DC (mDC), also called DC3. mDCs express CD1c, CD11c, CD14, and CD163 (7). Plasmatic level of soluble CD14 and CD163 is higher in patients with SARS-CoV-2-induced disease compared with healthy donors, and their assessment is indicative of the severity of the disease (10).
pDCs are a specialized subset of DCs that display a morphology of plasma cells. The cells express human leukocyte antigen class DR (HLA-DR), CD4, CD123, and toll-like receptors (TLRs) 7 and 9 (11). pDCs are presented in lung parenchyma and airways and are involved mainly in the production of type I interferon (IFN1) (5), namely, IFN-α (12). pDCs are, in fact, considered as IFN factory in the immune system in which the production of IFN by the cells exceeds the amount generated from other cells (13), thereby displaying high anti-viral functionality (7). Three effector subsets of pDCs are P1, P2, and P3. P1 is contributed to the production of IFN-I and acts in innate immunity, P2 displays a mixed (innate and adaptive) function, and P3 stimulates T cells (14).
DCs show reduced fraction in the blood of patients with severe SARS-CoV-2-induced disease. CD123 is a marker for pDCs. In patients with severe disease, CD123high pDCs are almost lost in the lung and depleted in the blood, whereas CD1c+ DCs migrate preferentially from the blood into the lung (15). Reduced frequencies of cDCs and pDCs in the peripheral blood of severe/critical cases and the increased fraction of these cells in convalescent individuals is reported by Chen and colleagues (16). Reduced DC fraction is thus linked to a more severe disease. Pérez-Gómez and colleagues reported that the reduced fraction of pDCs and CD1c+ DCs persists for 7 months after infection with SARS-CoV-2 (12).
Defective defense from APCs is occurring in severe disease. Zhou and colleagues in a study evaluated responses from T cells and DCs during the acute phase of disease. Both cell types showed functional impairment, and there was a delayed response from antigen-specific T cells (nucleocapsid protein- and RBD-specific T cells) despite the rapid generation of neutralizing antibodies (17). pDC activation into effector subsets (P1–3) is induced by SARS-CoV-2 in an initial phase compared to the late-stage severe disease (14). Saichi and colleagues in a single-cell RNA sequencing assay announced multi-process defects in blood APCs in patients with severe disease. The defects were augmented pDC apoptosis, reduced innate sensors and major histocompatibility complex (MHC)-II representation on cDC1c+ DCs, and downregulated genes related to the stimulation of anti-viral IFN pathway (18). SARS-CoV-2 also impairs maturation of DCs (7) and monocytes (19), thereby hampering effective activation of adaptive immunity against the virus.
IFN-I deficiency within the blood is a hallmark of the severity of SARS-CoV-2-induced disease (20). The production of IFNs is reduced in pDCs (13). A link between impaired IFN-1, delineated by lack of IFN-β and low IFN-α formation, with a severe/critical disease is identified. Deficient IFN-I activity is positively related with exacerbation of inflammation and persistent viral load (20). Impaired generation of IFN-α and the resultant low plasma level of this cytokine in patients with SARS-CoV-2-induced disease is also reported by Arunachalam and colleagues (20). In patients with a critically ill disease, systemic changes are occurring in the immune system as a result of DC-derived autoantibodies against IFN-I (21). TNF and nuclear factor kappa B (NF-kB) are mediators of hypoxia and pro-inflammatory signaling that are upregulated in pDCs (13). The activity of IL-6, TNF-α, and NF-kB is partly related to the SARS-CoV-2-related inflammation (20). IL-3 is another cytokine, its concentration of which is linked with disease severity. Patients with high viral load show low IL-3 level. IL-3 level is positively linked with increased proportion of circulating pDCs. IL-3 has been found to be related possibly with enhanced IFN-α activity, suggesting a protective role mediated by IL-3 against SARS-CoV-2-induced disease (22).
CD8+ T cells or cytotoxic T lymphocytes (CTLs) are powerful cells for eliminating intracellular pathogens and tumor cells (23). CD8+ T cells belong to the adaptive immunity, and they are the frontline defensive cells against cancer (24). Based on a study, responses from CD8+ T cells were found in more than 75% of convalescent patients (25), which is indicative of their important anti-viral activities. An effective defense against SARS-COV-2 is the need for immunity from both T cells and antibodies. Activation of CD8+ T cells is effective for virus clearance, and the activity of CD4+ T cells is essential for the activation of CD8+ T cells and optimal antibody responses. S-protein specific CD8+ and CD4+ T cells were observed in the blood of 70% and 100% of convalescent individuals, respectively (26). Early response from CD8+ T cells against SARS-COV-2 is protective, whereas late responses are seemingly destructive, possibly due to amplifying inflammatory responses associated with cytokine storm and lung damage (27). SARS-CoV-2-specific responses from CD4+ and CD8+ T cells soon after the onset of symptoms is linked with the fast clearance of the virus and a mild disease. A delayed response is associated with poor clinical outcomes (28). Wang and colleagues evaluated the immune state in survived and deceased patients. They described early, middle, late, and end stages for survived and deceased patients based on the time from the start of symptoms, representing ≤10, 11–20, 21–30, and >30 days, respectively. In deceased patients at early, middle, and late stages, there were hypofunctional, hyperfunctional, and anergic immune states, respectively. The proportion of CD8+ T cells was lower for all stages in deceased vs. survived patients (29).
SARS-CoV-2 induces a terminal differentiation state in T cells. T cells display a lower proliferation rate, an outcome of which is the reduced fraction of such important anti-viral cells (30). The fraction of CD8+ T cells along with T-helper memory cells is reduced in the peripheral blood of patients with severe disease, but CD4+-naive T cells are increased (27). During the acute phase of the disease, CD4+ T cells have higher fraction compared with CD8+ T cells, with a decrease in the latter an indicative of weak responses. Results of the study by Zhou and colleagues indicate the importance of both antibody surge and CD8+ T cell functionality for reducing the severity of condition in patients with SARS-CoV-2-induced disease (17). Besides their role for viral clearance, CD8+ T cells play an important role for generating immunological memory and thereby promoting a long-lasting immunity against viral infection (31). Patients with a history of SARS-CoV-2-induced disease will develop memory T cells for reinvigorating responses against re-entry of the virus (32). In patients recently vaccinated for SARS-CoV-2, there is a possibility of higher responses from effector memory T cells when they have had prior Tetanus–Diphtheria–pertussis (Tdap) or Measles–Mumps–Rubella (MMR) vaccination, thereby experiencing reduced disease severity (28).
NK cells are defined as the two main subtypes: CD56dim CD16bright and CD56brightCD16dim/neg (33). CD56bright cells present low cytotoxic capacity. CD56dim cells, by contrast, show high cytotoxic potential (34). In patients with SARS-CoV-2-induced disease, there is a severe reduction in the NK cell number (15). It was found that NK cells are reduced only in patients with severe (not mild) disease (35). NK cells are low in number but are hyper-active. The cells present a characteristic of NKdim phenotype through evolving cytotoxic and pro-inflammatory roles (15).
The number of perforin-expressing NK cells was compared in intensive care unit (ICU) patients (vs. non-ICU) cases, and it was found that there was a low NK cell proportion in patients admitted at the ICU (36). Inflammatory and immunological profile of NK and T cells was also checked by Chen and colleagues in patients with SARS-CoV-2-induced disease and in convalescent individuals. In patients with severe or critically ill disease, there were high levels of inflammatory cytokines, hyperactive CD8+ T cells, and NK cells with reduced cytotoxic capacity (16). Such NK cells showed reduced cytotoxic activity possibly due to their hyper-active state. Elevated expression of programmed death-1 receptor (PD-1) on the surface of T cells and NK cells in patients with SARS-CoV-2-induced disease is indicative of this hyper-active state and their further exhaustion and dysfunctionality (37). PD-1 is a checkpoint receptor, in which its increased expression on the surface of T cells and NK cells in an environment with high presence of its ligand programmed death ligand 1 (PD-L1) is considered as a marker of cellular dysfunctionality (38).
Monocytes are cells of innate immunity that represent 10%–15% of peripheral blood mononuclear cells (PBMCs) (39). Monocytes are classified into classical, inflammatory transitional, and non-classical subsets. Classical monocytes are immature and are designated as CD14++CD16− cell subset, whereas the other two subtypes are inflammatory and more differentiated cells. Transitional cells are CD14+CD16+, while non-classical cells are CD14− CD16++ (9). Macrophages and DCs are acting as APCs for priming naive T cells and promoting their activation. Macrophages are plastic cells that have two subtypes: M1 macrophage is a classical and pro-inflammatory subtype, whereas M2 macrophage is an alternatively activated and anti-inflammatory subset (40–42). M1-like macrophages are more active in the early stages of anti-viral immune responses, while M2-like macrophages are active during late stages and act in initiating the resolution of inflammation. In fact, after induction of an inflammatory response, a shift from M1- to M2-like phenotype is effective for initiating such resolution and for recovering tissue homeostasis. Imbalanced activity of M1- to M2-like macrophages exacerbates the pathogenesis of SARS-CoV-2-induced disease (43). The dysregulation of mononuclear phagocytes is linked with disease severity (44). Monocyte-derived macrophages are known as the key promoter of inflammation in SARS-CoV-2-induced disease. By contrast, anti-inflammatory M2 macrophages contributed to the resolution of inflammation. Such cells regulate the duration and magnitude of acute inflammation through releasing protectins, resolvins, and maresins (45).
The fraction of non-classical monocytes is increased in the peripheral blood and within the lungs of patients with SARS-CoV-2-induced disease, particularly in cases with more severe disease. To explain, Tincati and colleagues evaluated the immune state in two categories of patients: mild SARS-CoV-2-induced disease with PaO2/FiO2 > 300 and severe disease with PaO2/FiO2 < 200. They noticed the higher fraction of circulatory non-classical monocytes along with increased polarization toward Th1 cells with pro-cytolytic activity in the severe vs. mild disease (46). Sánchez-Cerrillo and colleagues found an enrichment of lungs with inflammatory transitional and non-classical cells in patients who were developing ARDS. It was found that there was more preferential infiltration of inflammatory monocytes compared with DCs during the progressive stage of SARS-CoV-2-induced disease (9). A work by another group showed a link between CD169 expression on monocytes with their enhanced capacity to stimulate CD8+ T cells. CD169+ monocytes were detected in CD14+ cells (classical and intermediate [transitional] monocytes) and observed in bronchoalveolar fluid and blood of patients with SARS-CoV-2-induced disease. These CD14+ CD169+ cells were, thus, considered as a promising source in vaccination therapy (39).
Monocyte-derived macrophages are permissive to SARS-CoV-2 and promote host cell death. To explain, Yang and colleagues evaluated the interplay between SARS-CoV-2 with mDCs and macrophages. Both cell types were permissive to the virus, but did not allow viral replication. Reduced IFN response occurred in both cells, but the expression of pro-inflammatory cytokines/chemokines was triggered only in monocyte-derived macrophages (47). Zheng and colleagues announced that SARS-CoV-2, although showing abortive infection and no efficient replication in monocyte-derived macrophages, induced profound death of host cells (48). A report by another group showed no possible link between macrophages and mDCs with first wave of cytokine storm in patients with SARS-CoV-2 in which none of the infected cells generated pro-inflammatory cytokines. In vitro assays showed the sensitivity of such cells to the SARS-CoV-2 infection (49). SARS-CoV-2 inhibitory effect on STAT1 phosphorylation contributed to the reduced immune activation in mDCs (47). A study by Lv and colleagues delineated diverse responses from M1/M2 alveolar macrophages upon exposure to SARS-CoV-2. M1 macrophages were more prone to the viral fusion and replication due to having lower endosomal pH. By contrast, M2 macrophages were able to deliver the virus toward lysosomes for further degradation due to their lower lysosomal but higher endosomal pH (50) (Figure 2).
Figure 2 M1/M2 cellular states in SARS-CoV-2-induced disease. M1 macrophages are pro-inflammatory and are active during the early stage of viral entry, whereas M2 macrophages are anti-inflammatory that are active during late stages. Viral replication and fusion are high in M1 macrophages, whereas the rate of replication is low in M2 macrophages. Lower lysosomal pH favors more viral degradation in lysosomes of M2 macrophages. Therefore, M1 macrophages are more prone to viral load and lung infection. The expression of angiotensin-converting enzyme 2 (ACE2) on M2 macrophages is reduced by IL-13 released from T helper 2 (Th2) cells, which is indicative of a level of resistance among M2 macrophages. Th1 cells are induced by M1 macrophages. Th1 polarity with pro-cytolytic activity is also induced by severe SARS-CoV-2. A shift from M1- to M2-like phenotype is required for promoting resolution of inflammation.
M2 macrophages expressing PD-L1 are protected against severe SARS-CoV-2-induced disease. Trombetta and colleagues evaluated a link between myeloid cell immune activation and phenotype with recovery from SARS-CoV-2-induced disease. PD-L1+ M2-like monocytes had the highest proportion among ICU cases at discharge. The cells with such high fraction represented a phenotype of classical monocytes (CD14++CD16−). The percentage of PD-L1+ M2-like monocytes was inversely linked with levels of chemokines and inflammatory cytokines related to the IFN pathway and was linked directly to the anti-SARS-CoV-2 Ig (IgG and IgM) titer. A marked decrease in the proportions of CD141+ mDCs and pDCs was also identified in all cases, which were partially recovered in non-ICU patients at discharge (51).
Neutrophils comprise over 50% of human leukocytes in the peripheral blood and are known as the most frequent effector cells of innate immunity (52). High fraction of neutrophils and increased neutrophil-to-lymphocyte ratio are considered as initial sign of SARS-CoV-2-induced disease (53). SARS-CoV-2 induces neutrophil infiltration and stimulates a hyperactive state in such cells for promoting hyperinflammation. Hammoudeh and colleagues evaluated multi-organ involvement in SARS-CoV-2-related cytokine storm. Results showed dysregulation in cytokine generation by inflamed pulmonary and extra-pulmonary organs, such as the kidney, liver, and heart. It was also found that there was upregulation of genes related to the neutrophil-associated immune responses mainly in the lung tissue. By contrast, B cells, T cells, DCs, and monocytes showed almost similar dysregulated responses in all types of tissues (2). Vanderbeke and colleagues investigated the immune cell basis of SARS-CoV-2-related hyperinflammation and severity. A fundamental role was identified for classical monocytes and hyperactivated neutrophils in hypercytokinemia. Neutrophils showed higher fraction in the lungs of patients with severe disease. Hyperactive neutrophils were marked by the upregulation of C-X-C motif chemokine ligand 8 (CXCL8), IL-1β, and S100A12 (54). SARS-CoV-2-infected cells release CXCL2 and CXCL8 for attracting infiltration of neutrophil-inducing immune cells (6). Neutrophils are activated in response to IL-17 release from CD4+ and CD8+ T cells in SARS-CoV-2-related pneumonia. The activity of this cytokine is linked positively with strengthened inflammatory events, so it can be a target for therapy (30). The hyperactive neutrophils seemingly have an immature phenotype. In fact, hyperinflammation related to SARS-CoV-2 promotes a shift in the neutrophil phenotype toward an immature state (19).
Tregs are the key immunosuppressive cells (55) that migrate into areas of inflammation and contribute to the suppression of inflammatory responses (56). Tregs act for the stimulation of immune tolerance, and their presence is important for preventing inflammatory and autoimmune diseases (57). Thus, CD4+FoxP3+ Tregs play important roles for maintaining immune homeostasis (3). The reduction or defective Treg functionality is partly contributed to the excessive systemic inflammation and to the impaired lung repair in SARS-CoV-2-induced disease (56).
Tregs possibly contributed to the amelioration of anti-viral defense at early stage of SARS-CoV-2-induced disease, whereas the activity of these cells will help attenuate inflammation-induced organ damage at late stage of the disease (3). The number of Tregs is reduced in patients with a severe disease compared with mild disease cases (58), which may be a reason for immune hyperactivation and lung damage (57). A point, however, is that Treg activity can promote immunosuppression in favor of disease progression despite the counter-effect of these cells on SARS-CoV-2-related inflammatory responses. Based on a report, viral particles can be observed at 70 days after the initial diagnosis of SARS-CoV-2-induced disease, causing long duration of infection with the virus (59). Yang and colleagues in a study evaluated the immune state in patients with long vs. short duration of SARS-CoV-2 infection. In patients with long duration of disease, there was an increase in the number of Tregs, but NK cell population was decreased. NK cells were less activated in long duration compared with the short duration of disease. Therefore, immunosuppression can be a reason for promoting SARS-CoV-2 persistence (60), thereby causing chronic viral infection and high loads of antigens within the body (61).
Wei et al. compared the immune state in two groups of patients with SARS-CoV-2-induced disease: good (n = 3) vs. poor prognosis group (n = 3). In patients with poor prognosis at the time of exacerbation, Th2 cells and Tregs were relatively higher compared with the good prognosis group (62). However, injection of allogenic Tregs for two critically ill patients admitted at Johns Hopkins University hospital presented clinical benefits and attenuated inflammatory markers. Both patients receiving such therapy survived and were discharged from the hospital (63). To support this, Meckiff and colleagues analyzed the frequency of cytotoxic and regulatory CD4+ T cells in hospitalized and non-hospitalized patients. Hospitalized patients had low fraction of Tregs but high proportion of CD4+ cytotoxic T-follicular and T-helper cells (64). From the results, it can be postulated that there is a positive link between low Treg fraction with more severe disease. Thus, recovering the functionality and fraction of Tregs can be an effective strategy for reducing disease burden. Two points, however, require attention from what was discussed above: (1) Treg activation at early-stage of SARS-CoV-2-induced disease dampens anti-viral defense systems, and (2) Treg activation at late stage of the disease promotes immunosuppression and may cause persistence of viral infection and delayed viral clearance. Figure 3 shows diverse functionality among CD8+ T cells and Tregs in patients with early- or late-stage SARS-CoV-2-induced disease.
Figure 3 Diverse functionality of CD8+ T cells and regulatory T cells (Tregs) in patients with SARS-CoV-2-induced disease. During the early-stage of the disease, the activity of CD8+ T cells is protective, whereas the cells are hyper-active and amplify inflammatory responses associated with cytokine storm and lung damage at late stage of the disease. Tregs have their own pros and cons during each disease stage. At early stage, Tregs dampen systemic inflammation, but they can hamper anti-viral defense systems. At late stage of the disease, Tregs protect body organs from inflammatory-related damages, but they may promote immunosuppression and further persistence and delayed clearance of the virus.
Myeloid-derived suppressor cells (MDSCs) are a heterogeneous population of immature cells that belong to the innate immunity and take immunosuppressive activities (65, 66). MDSCs are mainly derived from granulocytes (G-MDSCs) and monocytes (M-MDSCs). M-MDSCs are CD14+, while G-MDSCs are CD15+, both of which are either negative for HLA-DR or show low expression of this factor (65). Severe SARS-CoV-2-induced disease coincides with the presence of MDSCs (44). In fact, the expansion of myeloid cell compartment is considered as a hallmark of a severe disease (67). A study by Reizine and colleagues showed that in patients with a severe disease (developing ARDS), both G-MDSCs and M-MDSCs were increased compared to the cases with moderate pneumonia, in which the fraction of both MDSC subtypes was high at day 7 after ICU admission only in patients developing ARDS (68). In another study, a huge expansion of MDSCs (90% of circulatory mononuclear cells) was identified in severe (vs. mild) disease (69). Xue and colleagues found a positive link between CD14+HLA-DRlow/neg MDSCs (M-MDSCs) with viral load in the oropharyngeal area and with the length of patient stay in the hospital (70). A high fraction of MDSCs was found within the blood but not in endotracheal or nasopharyngeal aspirates of patients with severe disease (71).
High MDSC fraction is linked negatively with T-cell functionality. The higher expansion of M-MDSCs contributed to the dysfunctional T cells in patients with severe SARS-CoV-2-induced disease. M-MDSCs release IL-6 and IL-10 to augment the rate of inflammation and accumulating Tregs and regulatory B cell (Bregs) (72). Tregs accumulated within the lung display high IL-6-induced Notch4 expression, which hampers the release of amphiregulin, a cytokine related to tissue repair, thereby promoting severe lung inflammation and a more severe SARS-CoV-2-induced disease (73). A positive relation between MDSC expansion with increased serum level of transforming growth factor (TGF)-β is reported. At the convalescent phase of SARS-CoV-2-induced disease, a reduced fraction of MDSCs is related with lower TGF-β levels (69). TGF-β is a multi-tasking and a potent pro-fibrotic cytokine with immunosuppressive activities (74–76). In patients with severe disease, TGF-β released from G-MDSCs contributed to the suppression of IFN-γ generation from T cells, which further hampers the effective elimination of the virus (77). From what was discussed above, MDSC expansion, involving both M-MDSC and G-MDSC subsets, occurs in severe SARS-CoV-2-induced disease, and the high fraction of these cells contributed to T-cell dysfunctionality. A contrast to these findings is a study carried out by Takano and colleagues evaluating MDSC subsets in Japanese patients. Here, they noticed a transient (rather than an immense) expansion of only G-MDSCs (no other subsets) in cases with severe SARS-CoV-2-induced disease. They showed that this delay or transient aggregation is beneficial in improving clinical outcomes, delineated by attenuated SARS-CoV-2-related severe lung inflammation. Such transient expansion was not seen in patients who died from the disease (67). From this study, it could be postulated that a rate of MDSCs is beneficial for patients with SARS-CoV-2-induced disease, but a high fraction of such cells will hamper T-cell functionality against this disease. To explain, lectin-type oxidized LDL receptor 1 (LOX-1) expression by MDSCs is indicative of their potent immunosuppressive activity. Coudereau and colleagues noticed an elevation of LOX-1+ MDSCs in patients developing ARDS, which hampers effective resolution of infection (78).
Mast cells are cells of innate immunity that take pathogenic roles in several inflammatory diseases. The activation of mast cells is associated with the SARS-CoV-2-related inflammatory events. Mast cells are among the fast-responder cells at the time of pathogen invasion. In the serum and lungs of patients with SARS-CoV-2-induced disease, there is a rise in the mast-cell-derived proteases (79). Mast cells are induced by chemokines synthesized during SARS-CoV-2 infection (80). Mast cell activation by the virus increases the risk of lung inflammation and fibrosis (81). Protease production by mast cells is a feature of hyperinflammation. Selective inhibition of mast cells using anti-Siglec-8 antibody is reported to suppress airway infiltration and disease severity in a model of respiratory viral infection, which is indicative of the importance of anti-Siglec-8 antibody in the attenuation of excess inflammatory events occurring during viral infection (79). However, a study by Valent and colleagues showed that in patients with mast cell activation disease, there was no elevation in the overall risk of developing toward SARS-CoV-2-induced disease, but they recommended to manage mastocytosis when complications, such as acute anaphylaxis, occur (82).
Basophil count is reduced in patients with acute disease and is correlated with poor prognosis (83). Based on a report by Rodriguez and colleagues, the proportion of the granulocyte subsets eosinophils and basophils is increased in patients passing from the acute to recovery phase, both of which were among the cells with the most dynamic changes during the severe disease. The number of basophils was also associated considerably with the production of IgG antibody from B cells against SARS-CoV-2. This is indicative of the key contribution taken by basophils to the immunopathology of SARS-CoV-2-induced disease and anti-viral defenses (84).
Eosinophils are a part of host immune defense against respiratory viruses (85). There is a report of a link between eosinopenia with acute respiratory deterioration upon SARS-CoV-2 infection and that eosinopenia may serve as a poor prognostic indicator and a marker of a more severe disease (86). Vieyra and coworkers announced that the presence of eosinophils is seemingly effective for controlling neutrophil-induced exacerbation of inflammation. They found a negative correlation between eosinophil count with neutrophil count and neutrophil-to-lymphocyte ratio. Another finding of this study was a decrease in the number of eosinophils in deceased (vs. recovered) patients (eosinophil level < 0.01 × 109/L: eosinopenia), while a rapid increase in their number was identified in recovered individuals (87). Another study demonstrated the positive link between higher eosinophil count with protection against poor outcomes from SARS-CoV-2-induced disease in patients receiving inhaled corticosteroid therapy (but not in cases without corticosteroid prescription), which is suggestive of the protective role of corticosteroids against SARS-CoV-2 infection (88). A study by Pala and colleagues showed the advantage of eosinophil involvement during the initial phase of SARS-CoV-2 infection, but eosinophilic activity in patients with severe SARS-CoV-2-induced disease is contributed to the mal-adaptive immune responses and is responsible for the immunopathology of the disease. The authors suggested the inhibition of eosinophile activation in severe SARS-CoV-2 patients as a possible strategy for contrasting harmful immunity (85). Lung eosinophilic immunopathology occurring secondary to the SARS-CoV-2 vaccination is called vaccine-associated enhanced respiratory disease. In order to reduce the risk of such immunopathology related to the eosinophilic activity, Hemmi and colleagues recommended intranasal vaccination therapy against S protein of the virus along with the application of the TLR9 agonist ODN2006 (89).
G-MDSCs contributed to the impaired proliferation of T cells in SARS-CoV-2-induced disease (90). A study by Reizine and colleagues attested a positive relation between high MDSC fraction with reduced T-cell count (lymphopenia). High arginase-1 activity of MDSCs is responsible for reduced T-cell proliferation (68). Patients with SARS-CoV-2-induced disease have high fraction of arginase-1+ neutrophils with an immature phenotypical state (91). This is presumably indicative of the implication G-MDSCs for hampering T-cell proliferation. Xiang and colleagues found a relation between inflammation and lymphopenia with decimation of secondary lymphoid organs including spleen and lymph nodes. Here, the high release of IL-1β and IL-6 from infected DCs was found as a possible reason for the decimation of such lymphoid organs and the subsequent lymphopenia (92). High IL-6 is also released from MHC-IIlow monocytes (13). T cells in the acute phase of disease display a unique metabolic profile. The cells present mitochondrial apoptosis that, in turn, promotes lymphopenia in this phase of SARS-CoV-2-induced disease (93).
ACE2, which serves as the receptor for S protein of SARS-CoV-2, is expressed by DCs and macrophages. This may indicate that the cells, although contributed to the anti-viral defensive system, may act to enable viral anchoring inside the lung parenchyma (94). IL-13 reduces expression of ACE2 by M2 macrophages, which may be indicative of viral resistance of this macrophage subtype (45). In addition, as mentioned above, due to having lower lysosomal pH, the cells tend to deliver SARS-CoV-2 virus to lysosomes for further degradation, thereby hampering further replication of the virus.
Patients with active SARS-CoV-2 infection show reduced ACE2 levels due to its cellular internalization. Engagement between SARS-CoV-2 with ACE2 will lead to the internalization of ACE2 and inhibition of its activity. Thus, activation of ACE2 receptor can be a possible approach for blocking the development of the condition toward cytokine storm in patients with SARS-CoV-2-induced disease. In a study, macrophages were treated with the ACE2 receptor activator diminazene aceturate, and there was an inhibition of SARS-CoV-2-related pro-inflammatory profile by this agent. Therefore, ACE2 receptor activator can be suggested as a treatment schedule for SARS-CoV-2-induced disease (95). Diminazene aceturate can also induce vascular repair and improve alveolar remodeling. ACE2 activators can, thus, be used as an approach for controlling the escalation of the disease (96).
Neutrophils are strongly associated with platelets in patients with severe SARS-CoV-2-induced disease (97). Acute viral infection is linked positively with dysregulated formation of neutrophil extracellular traps (NETs) (52). Excessive generation (52) and release (54) of NETs from neutrophils is related positively with severe disease. NETs restrict viral spreading, but they can promote thrombi formation through engaging platelets (6). Platelet activation is also increased when L-arginine concentration is low (98). L-Arginine is degraded by arginase-1, and G-MDSC is a cell type displaying arginase-1 activity (65). High levels of arginase is produced from G-MDSCs in severe SARS-CoV-2-induced disease (99). Therefore, G-MDSCs are linked positively with the development of thromboembolic events through their high arginase activity.
Severe SARS-CoV-2 infection reduces O2 levels within the blood and affected tissues (100). Hypoxia-inducible factor (HIF)-1α is a key mediator of severe or acute hypoxia (101). SARS-CoV-2 activates HIF-1α, the activity of which exacerbates generation of pro-inflammatory signaling and augments the rate of cytokine storm (102). Monocytes are more recruited toward hypoxic tissues and differentiated into macrophages (100). SARS-CoV-2 infection promotes hypoxemia, which further causes expression of HIFs by immune cells (103). The activity of HIFs on macrophages promotes their local aggregation and activation (103). The promoter of FoxP3 gene contains an HIF-responsive element (104). FoxP3 shows repressed expression in lungs of patients with severe SARS-CoV-2-induced disease. HIF-1α promotes aerobic glycolysis, which is further contributed to degradation of FoxP3, thereby blocking differentiation of Tregs (105).
Designing a strong vaccination system that is effective in generating sufficient levels of neutralizing antibodies after incubation with one dose is a preferred approach concerning the shortage of vaccine for SARS-CoV-2 virus. A strategy that can boost the rate and binding activity of antibody titers is to use O2 as a co-adjuvant with SARS-CoV-2 vaccination. O2 has strong immunological activity, and its co-adjuvant is an effective approach for potentiating vaccine potency. O2-generating COVID-19 cryogel-based vaccine (O2-CryogelVAX) is an example in this context. The application of O2-CryogelVAX in mice has found to induce high titers of antibodies and stimulate their potent neutralizing activity. Sustained generation of neutralizing antibodies along with promotion of local recruitment of immune cells is a virtue for the use of O2-CryogelVAX (106).
Diverse susceptibility among children and elderly individuals to SARS-CoV-2 infection is explained in the context of the immune system. The immunological profile in response to SARS-CoV-2 is different in children compared with adults. In children, immunophenotype is less inflammatory, and the humoral immunity is as strong as what is seen in adults (107). Based on the results of one study, there is increased proportion of CD63+ neutrophils in children but no response from these cells in adults during the acute phase of SARS-CoV-2-induced disease, and the impact of CD63 on neutrophil activation and secretion of pro-inflammatory mediators are all indicative of diverse responses from innate immunity between children and adults (108). Neeland and coworkers evaluated the immunological mechanism behind milder symptoms in children compared to adults. Children during acute phase of the disease had decreased proportions of all subsets of circulating monocytes including classical, transitional, and non-classical subtypes, whereas only the non-classical subtype of monocytes showed reduced fraction in adults (108). Elderly individuals, by contrast, have increased number of inflammatory transitional monocytes, which is a reason for more vulnerability to SARS-CoV-2-induced disease among aged individuals (109). Aged individuals present loss of Treg functionality, which results in the difficulty in controlling immune responses, thereby enhancing the inflammation rate and inflammatory storm upon encountering SARS-CoV-2. Thymus is an organ that acts in mediating adaptive immune responses and immunomodulation. Tregs are active during the early life period and take function as immunomodulatory cells. T cells destroyed by virus are replaced in the thymus, so shrinkage of this organ during adolescence period may be one possible reason for more severe SARS-CoV-2-induced disease in adults compared with children (110).
Induction of antigen-specific CD8+ T cell immunity provides appropriate protection against SARS-CoV-2. A strategy to achieve this aim is to use DNA vectors expressing different proteins of SARS-CoV-2. This approach is effective through eliciting CD8+ T-cell responses against various antigens in a single injection (111). N361-369-specific T-cell receptors (TCRs) can be obtained from individuals recovered from SARS-CoV-2-induced disease and can be exploited as a useful strategy for promoting CD8+ T-cell cytotoxicity and effector cytokine release from CD4+ T cells. Activation of CD8+ T cells and their cytolytic activity by the T-cell epitope N361–369 can cause lysis of SARS-CoV-2-infected cells and promote viral clearance in patients with SARS-CoV-2-induced disease (25).
Lipid-based nanoparticle vaccine platform (NVP) can be developed to promote long-lasting immunity against SARS-CoV-2. NVPs are loaded with SARS-CoV-2 antigens for inducing antigen-specific antibodies. Such NVPs are taken up by DCs and contribute to the maturation of DCs and strengthening of their antigen presentation capacity (112).
Extracellular vesicles (EVs) including exosomes can be used as a tool for developing vaccines against viral antigens. Exosomes loaded with therapeutic cargo can be used for such purpose. Exosomes interact with different cells of the immune system including NK cells, T cells, DCs, and macrophages to allow cell–cell communications and modulate anti-viral immune responses against SARS-CoV-2 (113). The reaction from CD8+ T cells can be induced using EVs engineered for representing viral antigens. In this way, EVs are acting as an APC. A virtue of EV-based therapy is the safety of this approach for designing virus-free vaccines due to evolving low baseline immunological profile (114).
Patients with severe SARS-CoV-2-induced disease show a dysregulated orchestration and functionality in cells of the immune system, which results in aggravation of the condition and promotion of systemic inflammation and multi-organ injury. MDSCs, neutrophils, and monocytes are highly present, whereas CD8+ T cells and NK cells are reduced in severe diseases (Figure 4). This is indicative of an immunosuppressive profile in the immune system, as depicted in the Figure 5. Strategies to bring back the normalization in the cellular immune state will be a valuable tool for powering the activity of the immune system against viral entry and strengthening the efficacy of vaccination therapy. Such strategy can reduce the involvement of other organs and the time of hospital stay in cases with a severe disease.
Figure 4 Different orchestration of cells of the immune system within the blood or lung of patients with severe SARS-CoV-2-induced disease. Monocyte-derived DCs (mDCs), non-classical monocytes, T helper 1 (Th1) cells, and myeloid-derived suppressor cells (MDSCs) are high in the peripheral blood of patients with severe disease. By contrast, CD8+ T cells, plasmacytoid DCs (pDCs), CD1c+ DCs, natural killer (NK) cells, macrophage type 2 (M2) cells, and regulatory T cells (Tregs) show low fraction within the circulation. M1 macrophages are high, whereas M2 macrophages are low within the lung of severe cases.
Figure 5 The impact of severe SARS-Cov2 induced disease on cellular immunity. In patients with a severe disease the activity of regulatory T cell (Tregs) and M2 macrophage is hampered. Whereas M1 macrophage, myeloid-derived suppressor cells (MDSCs), monocytes, neutrophils and Th1 cells show increased expansion. Other consequences of a severe infection are plasmacytoid dendritic cell (pDC) apoptosis, and CD8+ T cell and natural killer (NK) cell exhaustion.
Collection and revision of information, KM and JM. Conceptualization, KM. Writing, original draft preparation, review, and editing, JM and KM. Two authors have read and agreed to publish of the manuscript.
The authors declare that the research was conducted in the absence of any commercial or financial relationships that could be construed as a potential conflict of interest.
All claims expressed in this article are solely those of the authors and do not necessarily represent those of their affiliated organizations, or those of the publisher, the editors and the reviewers. Any product that may be evaluated in this article, or claim that may be made by its manufacturer, is not guaranteed or endorsed by the publisher.
1. Parker R, Partridge T, Wormald C, Kawahara R, Stalls V, Aggelakopoulou M, et al. Mapping the SARS-CoV-2 spike glycoprotein-derived peptidome presented by HLA class II on dendritic cells. Cell Rep (2021) 35(8):109179. doi: 10.1016/j.celrep.2021.109179
2. Hammoudeh SM, Hammoudeh AM, Bhamidimarri PM, Al Safar H, Mahboub B, Künstner A, et al. Systems immunology analysis reveals the contribution of pulmonary and extrapulmonary tissues to the immunopathogenesis of severe COVID-19 patients. Front Immunol (2021) 12. doi: 10.3389/fimmu.2021.595150
3. Wang Y, Zheng J, Islam MS, Yang Y, Hu Y, Chen X. The role of CD4+ FoxP3+ regulatory T cells in the immunopathogenesis of COVID-19: implications for treatment. Int J Biol Sci (2021) 17(6):1507. doi: 10.7150/ijbs.59534
4. Jouan Y, Guillon A, Gonzalez L, Perez Y, Boisseau C, Ehrmann S, et al. Phenotypical and functional alteration of unconventional T cells in severe COVID-19 patients. J Exp Med (2020) 217(12):e20200872. doi: 10.1084/jem.20200872
5. Campana P, Parisi V, Leosco D, Bencivenga D, Della Ragione F, Borriello A. Dendritic cells and SARS-CoV-2 infection: still an unclarified connection. Cells (2020) 9(9):2046. doi: 10.3390/cells9092046
6. Amor S, Fernández Blanco L, Baker D. Innate immunity during SARS-CoV-2: evasion strategies and activation trigger hypoxia and vascular damage. Clin Exp Immunol (2020) 202(2):193–209. doi: 10.1111/cei.13523
7. Galati D, Zanotta S, Capitelli L, Bocchino M. A bird's eye view on the role of dendritic cells in SARS-CoV-2 infection: perspectives for immune-based vaccines. Allergy 77:100–10. (2021). doi: 10.1111/all.15004
8. Costanzo M, De Giglio MAR, Roviello GN. Anti-coronavirus vaccines: Past investigations on SARS-CoV-1 and MERS-CoV, the approved vaccines from BioNTech/Pfizer, moderna, Oxford/AstraZeneca and others under development against SARSCoV-2 infection. Curr Medicinal Chem (2021). 29:4–18. doi: 10.2174/0929867328666210521164809
9. Sánchez-Cerrillo I, Landete P, Aldave B, Sánchez-Alonso S, Sánchez-Azofra A, Marcos-Jiménez A, et al. COVID-19 severity associates with pulmonary redistribution of CD1c+ DCs and inflammatory transitional and nonclassical monocytes. J Clin Invest (2020) 130(12):6290–300. doi: 10.1172/JCI140335
10. Zingaropoli MA, Nijhawan P, Carraro A, Pasculli P, Zuccalà P, Perri V, et al. Increased sCD163 and sCD14 plasmatic levels and depletion of peripheral blood pro-inflammatory monocytes, myeloid and plasmacytoid dendritic cells in patients with severe COVID-19 pneumonia. Front Immunol (2021) 12:147. doi: 10.3389/fimmu.2021.627548
11. Saadeh D, Kurban M, Abbas O. Plasmacytoid dendritic cell and type I interferons as possible explanation for clearance of longstanding warts during COVID-19 in a transplant patient, reply to erkayman. Transplant Infect Dis (2021) 23:e13585. doi: 10.1111/tid.13585
12. Perez-Gomez A, Vitalle J, del Carmen Gasca-Capote M, Gutierrez-Valencia A, Trujillo-Rodriguez M, Serna-Gallego A, et al. Dendritic cell deficiencies persist seven months after SARS-CoV-2 infection. bioRxiv (2021) 8:2128–39. doi: 10.1038/s41423-021-00728-2
13. Wack A. Monocyte and dendritic cell defects in COVID-19. Nat Cell Biol (2021) 23(5):445–7. doi: 10.1038/s41556-021-00685-y
14. Onodi F, Bonnet-Madin L, Meertens L, Karpf L, Poirot J, Zhang S-Y, et al. SARS-CoV-2 induces human plasmacytoid predendritic cell diversification via UNC93B and IRAK4. J Exp Med (2021) 218(4):e20201387. doi: 10.1084/jem.20201387
15. AbdelMassih AF, Menshawey R, Hozaien R, Kamel A, Mishriky F, Husseiny RJ, et al. The potential use of lactate blockers for the prevention of COVID-19 worst outcome, insights from exercise immunology. Med Hypotheses (2021) p:110520. doi: 10.1016/j.mehy.2021.110520
16. Chen Q, Yu B, Yang Y, Huang J, Liang Y, Zhou J, et al. Immunological and inflammatory profiles during acute and convalescent phases of severe/critically ill COVID-19 patients. Int Immunopharmacol (2021) 97:107685. doi: 10.1016/j.intimp.2021.107685
17. Zhou R, To KK-W, Wong Y-C, Liu L, Zhou B, Li X, et al. Acute SARS-CoV-2 infection impairs dendritic cell and T cell responses. Immunity (2020) 53(4):864–877.e5. doi: 10.1016/j.immuni.2020.07.026
18. Saichi M, Ladjemi MZ, Korniotis S, Rousseau C, Ait Hamou Z, Massenet-Regad L, et al. Single-cell RNA sequencing of blood antigen-presenting cells in severe COVID-19 reveals multi-process defects in antiviral immunity. Nat Cell Biol (2021) 23(5):538–51. doi: 10.1038/s41556-021-00681-2
19. Parackova Z, Zentsova I, Bloomfield M, Vrabcova P, Smetanova J, Klocperk A, et al. Disharmonic inflammatory signatures in COVID-19: augmented neutrophils’ but impaired monocytes’ and dendritic cells’ responsiveness. Cells (2020) 9(10):2206. doi: 10.3390/cells9102206
20. Hadjadj J, Yatim N, Barnabei L, corneau A, Boussier J, Smith N, et al. Impaired type I interferon activity and exacerbated inflammatory responses in severe cOVId-19 patients. Science (2020) 369:718–24. doi: 10.1126/science.abc6027
21. van der Wijst MG, Vazquez SE, Hartoularos GC, Bastard P, Grant T, Bueno R, et al. Type I interferon autoantibodies are associated with systemic immune alterations in patients with COVID-19. Sci Trans Med (2021) 13:eabh2624. doi: 10.1126/scitranslmed.abh2624
22. Bénard A, Jacobsen A, Brunner M, Krautz C, Klösch B, Swierzy I, et al. Interleukin-3 is a predictive marker for severity and outcome during SARS-CoV-2 infections. Nat Commun (2021) 12(1):1–8. doi: 10.1038/s41467-021-21310-4
23. Doedens AL, Phan AT, Stradner MH, Fujimoto JK, Nguyen JV, Yang E, et al. Hypoxia-inducible factors enhance the effector responses of CD8+ T cells to persistent antigen. Nat Immunol (2013) 14(11):1173–82doi: 10.1038/ni.2714.
24. Majidpoor J, Mortezaee K. Interleukin-2 therapy of cancer-clinical perspectives. Int Immunopharmacol (2021) 98:107836. doi: 10.1016/j.intimp.2021.107836
25. Hu C, Shen M, Han X, Chen Q, Li L, Chen S, et al. Identification of cross-reactive CD8+ T cell receptors with high functional avidity to a SARS-CoV-2 immunodominant epitope and its natural mutant variants. Genes Dis (2021) 9:216–29. doi: 10.1016/j.gendis.2021.05.006
26. Jeyanathan M, Afkhami S, Smaill F, Miller MS, Lichty BD, Xing Z. Immunological considerations for COVID-19 vaccine strategies. Nat Rev Immunol (2020) 20(10):615–32. doi: 10.1038/s41577-020-00434-6
27. Melenotte C, Silvin A, Goubet A-G, Lahmar I, Dubuisson A, Zumla A, et al. Immune responses during COVID-19 infection. Oncoimmunology (2020) 9(1):1807836. doi: 10.1080/2162402X.2020.1807836
28. Mysore V, Cullere X, Settles M, Ji X, Kattan MW, Desjardins M, et al. Protective heterologous T cell immunity in COVID-19 induced by MMR and tdap vaccine antigens. (2021) Med (N Y), 2:1050–71. doi: 10.1016/j.medj.2021.08.004
29. Wang F, Hou H, Yao Y, Wu S, Huang M, Ran X, et al. Systemically comparing host immunity between survived and deceased COVID-19 patients. Cell Mol Immunol (2020) 17(8):875–7. doi: 10.1038/s41423-020-0483-y
30. De Biasi S, Meschiari M, Gibellini L, Bellinazzi C, Borella R, Fidanza L, et al. Marked T cell activation, senescence, exhaustion and skewing towards TH17 in patients with COVID-19 pneumonia. Nat Commun (2020) 11(1):1–17. doi: 10.1038/s41467-020-17292-4
31. Buttenschön J, Mattner J. The interplay between dendritic cells and CD8 T lymphocytes is a crucial component of SARS-CoV-2 immunity. Cell Mol Immunol (2021) 18(2):247–9. doi: 10.1038/s41423-020-00624-1
32. Tavukcuoglu E, Horzum U, Inkaya AC, Unal S, Esendagli G. Functional responsiveness of memory T cells from COVID-19 patients. Cell Immunol (2021) 365:104363. doi: 10.1016/j.cellimm.2021.104363
33. Bähr I, Spielmann J, Quandt D, Kielstein H. Obesity-associated alterations of natural killer cells and immunosurveillance of cancer. Front Immunol (2020) 11:245. doi: 10.3389/fimmu.2020.00245
34. Bähr I, Jahn J, Zipprich A, Pahlow I, Spielmann J, Kielstein H. Impaired natural killer cell subset phenotypes in human obesity. Immunologic Res (2018) 66(2):234–44. doi: 10.1007/s12026-018-8989-4
35. Chen X, Huang J, Huang Y, Chen J, Huang Y, Jiang X, et al. Characteristics of immune cells and cytokines in patients with coronavirus disease 2019 in guangzhou, China. Hum Immunol (2020) 81(12):702–8. doi: 10.1016/j.humimm.2020.08.006
36. Bordoni V, Sacchi A, Cimini E, Notari S, Grassi G, Tartaglia E, et al. An inflammatory profile correlates with decreased frequency of cytotoxic cells in coronavirus disease 2019. Clin Infect Dis (2020) 71(16):2272–5. doi: 10.1093/cid/ciaa577
37. Li M, Guo W, Dong Y, Wang X, Dai D, Liu X, et al. Elevated exhaustion levels of NK and CD8+ T cells as indicators for progression and prognosis of COVID-19 disease. Front Immunol (2020) 11. doi: 10.3389/fimmu.2020.580237
38. Majidpoor J, Mortezaee K. The efficacy of PD-1/PD-L1 blockade in cold cancers and future perspectives. Clin Immunol (2021) 226:108707. doi: 10.1016/j.clim.2021.108707
39. Affandi AJ, Olesek K, Grabowska J, Nijen Twilhaar MK, Rodríguez E, Saris A, et al. CD169 defines activated CD14+ monocytes with enhanced CD8+ T cell activation capacity. Front Immunol (2021) 12:3044. doi: 10.3389/fimmu.2021.697840
40. Mortezaee K. Normalization in tumor ecosystem: Opportunities and challenges. Cell Biol Int (2021) 45:2017–030. doi: 10.1002/cbin.11655
41. Mortezaee K, Majidpoor J. Dysregulated metabolism: A friend-to-foe skewer of macrophages. Int Rev Immunol (2022), 1–17. doi: 10.1080/08830185.2022.2095374
42. Mortezaee K, Majidpoor J. Roles for macrophage-polarizing interleukins in cancer immunity and immunotherapy. Cell Oncol (2022) 45:1–21. doi: 10.1007/s13402-022-00667-8
43. Gracia-Hernandez M, Sotomayor EM, Villagra A. Targeting macrophages as a therapeutic option in COVID-19. Front Pharmacol (2020) 11:1659. doi: 10.3389/fphar.2020.577571
44. Kvedaraite E, Hertwig L, Sinha I, Ponzetta A, Hed Myrberg I, Lourda M, et al. Major alterations in the mononuclear phagocyte landscape associated with COVID-19 severity. Proc Natl Acad Sci (2021) 118(6):b5e2018587118. doi: 10.1101/2020.08.25.20181404
45. Martinez FO, Combes TW, Orsenigo F, Gordon S. Monocyte activation in systemic covid-19 infection: Assay and rationale. EBioMedicine (2020) 59:102964. doi: 10.1016/j.ebiom.2020.102964
46. Tincati C, Cannizzo ES, Giacomelli M, Badolato R, d'Arminio Monforte A, Marchetti G. Heightened circulating interferon-inducible chemokines, and activated pro-cytolytic Th1-cell phenotype features COVID-19 aggravation in the second week of illness. Front Immunol (2020) 11. doi: 10.3389/fimmu.2020.580987
47. Yang D, Chu H, Hou Y, et al. Attenuated interferon and proinflammatory response in SARS-CoV-2–infected human dendritic cells is associated with viral antagonism of STAT1 phosphorylation. J Infect Dis (2020) 222(5):734–45. doi: 10.1093/infdis/jiaa356
48. Zheng J, Wang Y, Li K, Meyerholz KD, Allamargot C, Perlman S, et al. Severe acute respiratory syndrome coronavirus 2–induced immune activation and death of monocyte-derived human macrophages and dendritic cells. J Infect Dis (2021) 223(5):785–95. doi: 10.1093/infdis/jiaa753
49. Niles MA, Gogesch P, Kronhart S, Iannazzo SO, Kochs G, Waibler Z, et al. Macrophages and dendritic cells are not the major source of pro-inflammatory cytokines upon SARS-CoV-2 infection. Front Immunol (2021) 12:1984. doi: 10.3389/fimmu.2021.647824
50. Lv J, Wang Z, Qu Y, Zhu H, Zhu Q, Tong W, et al. Distinct uptake, amplification, and release of SARS-CoV-2 by M1 and M2 alveolar macrophages. Cell Discovery (2021) 7(1):1–12. doi: 10.1038/s41421-021-00258-1
51. Trombetta AC, Farias GB, Gomes A, et al. Severe COVID-19 recovery is associated with timely acquisition of a myeloid cell immune-regulatory phenotype. Front Immunol (2021) 12:2346. doi: 10.3389/fimmu.2021.691725
52. Sung P-S, Hsieh S-L. C-type lectins and extracellular vesicles in virus-induced NETosis. J Biomed Sci (2021) 28(1):1–12. doi: 10.1186/s12929-021-00741-7
53. Singh Y, Fuloria NK, Fuloria S, et al. Disruption of the biological activity of protease-activated receptors2/4 in adults rather than children in SARS CoV-2 virus-mediated mortality in COVID-19 infection. Drug Dev Res. doi: 10.1002/ddr.21874
54. Vanderbeke L, Van Mol P, Van Herck Y, et al. Monocyte-driven atypical cytokine storm and aberrant neutrophil activation as key mediators of COVID-19 disease severity. Nat Commun (2021) 12(1):1–15. doi: 10.1038/s41467-021-24360-w
55. Farhood B, Najafi M, Salehi E, Goradel NH, Nashtaei MS, Khanlarkhani N, et al. Disruption of the redox balance with either oxidative or anti-oxidative overloading as a promising target for cancer therapy. J Cell Biochem (2019) 120(1):71–6. doi: 10.1002/jcb.27594
56. Gladstone DE, Kim BS, Mooney K, Karaba AH, D'Alessio FR. Regulatory T cells for treating patients with COVID-19 and acute respiratory distress syndrome: two case reports. Ann Internal Med (2020) 173(10):852–3. doi: 10.7326/L20-0681
57. Stephen-Victor E, Das M, Karnam A, Pitard B, Gautier J-F, Bayry J, et al. Potential of regulatory T-cell-based therapies in the management of severe COVID-19. Eur Respir J (2020) 56(3):2002182. doi: 10.1183/13993003.02182-2020
58. Rahimzadeh M, Naderi N. Toward an understanding of regulatory T cells in COVID-19: A systematic review. J Med Virol (2021) 93(7):4167–81. doi: 10.1002/jmv.26891
59. Avanzato VA, Matson MJ, Seifert SN, Pryce R, Williamson BN, Anzick SL, et al. Case Study: Prolonged Infectious SARS-CoV-2 Shedding from an Asymptomatic Immunocompromised Individual with Cancer. Cell (2020) 183:1901–12. doi: 10.1016/j.cell.2020.10.049
60. Yang B, Fan J, Huang J, Guo E, Fu Y, Liu S, et al. Clinical and molecular characteristics of COVID-19 patients with persistent SARS-CoV-2 infection. Nat Commun (2021) 12(1):1–13. doi: 10.1038/s41467-021-23621-y
61. Liu Y-N, Yang J-F, Huang D-J, Ni H-H, Zhang C-X, Zhang L, et al. Hypoxia induces mitochondrial defect that promotes t cell exhaustion in tumor microenvironment through myc-regulated pathways. Front Immunol (2020) 11:1906. doi: 10.3389/fimmu.2020.01906
62. Wei L.l., Wang W.j., Chen D.x., Xu B. Dysregulation of the immune response affects the outcome of critical COVID-19 patients. J Med Virol (2020) 92(11):2768–76. doi: 10.1002/jmv.26181
63. Abbasi J. Regulatory T cells tested in patients with COVID-19 ARDS. Jama (2020) 324(6):539–9. doi: 10.1001/jama.2020.13765
64. Meckiff BJ, Ramírez-Suástegui C, Fajardo V, Chee SJ, Kusnadi A, Simon H, et al. Imbalance of regulatory and cytotoxic SARS-CoV-2-reactive CD4+ T cells in COVID-19. Cell (2020) 183(5):1340–1353.e16. doi: 10.1016/j.cell.2020.10.001
65. Mortezaee K. Myeloid-derived suppressor cells in cancer immunotherapy-clinical perspectives. Life Sci (2021) 277:119627. doi: 10.1016/j.lfs.2021.119627
66. Najafi M, Majidpoor J, Toolee H, Mortezaee K. The current knowledge concerning solid cancer and therapy. J Biochem Mol Toxicol (2021) 35:e22900. doi: 10.1002/jbt.22900
67. Takano T, Matsumura T, Adachi Y, Terahara K, Moriyama S, Onodera T, et al. Myeloid cell dynamics correlating with clinical outcomes of severe COVID-19 in Japan. Int Immunol (2021) 33(4):241–7. doi: 10.1093/intimm/dxab005
68. Reizine F, Lesouhaitier M, Gregoire M, Pinceaux K, Gacouin A, Maamar A, et al. SARS-CoV-2-induced ARDS associates with MDSC expansion, lymphocyte dysfunction, and arginine shortage. J Clin Immunol (2021) 41(3):515–25. doi: 10.1007/s10875-020-00920-5
69. Agrati C, Sacchi A, Bordoni V, Cimini E, Notari S, Grassi G, et al. Expansion of myeloid-derived suppressor cells in patients with severe coronavirus disease (COVID-19). Cell Death Differentiation (2020) 27(11):3196–207. doi: 10.1038/s41418-020-0572-6
70. Xue G, Jiang M, Zhao R, Le A, Li J. Elevated frequencies of CD14+ HLA-DRlo/neg MDSCs in COVID-19 patients. Aging (Albany NY) (2021) 13(5):6236. doi: 10.18632/aging.202571
71. Falck-Jones S, Vangeti S, Yu M, Falck-Jones R, Cagigi A, Badolati I, et al. Functional monocytic myeloid-derived suppressor cells increase in blood but not airways and predict COVID-19 severity. J Clin Invest (2021) 131(6):e144734. doi: 10.1172/JCI144734
72. Tomić S, Đokić J, Stevanović D, Ilić N, Gruden-Movsesijan A, Dinić M, et al. Reduced expression of autophagy markers and expansion of myeloid-derived suppressor cells correlate with poor T cell response in severe COVID-19 patients. Front Immunol (2021) 12:208. doi: 10.3389/fimmu.2021.614599
73. Harb H, Benamar M, Lai PS, Contini P, Griffith JW, Crestani E, et al. Notch4 signaling limits regulatory T-cell-mediated tissue repair and promotes severe lung inflammation in viral infections. Immunity (2021) 54:1186–199.e7. doi: 10.1016/j.immuni.2021.04.002
74. Mortezaee K. Organ tropism in solid tumor metastasis: an updated review. Future Oncol (2021) 17(15):1943–61. doi: 10.2217/fon-2020-1103
75. Najafi M, Mortezaee K, Majidpoor J. Stromal reprogramming: a target for tumor therapy. Life Sci (2019) 239:117049. doi: 10.1016/j.lfs.2019.117049
76. Mortezaee K. Nicotinamide adenine dinucleotide phosphate (NADPH) oxidase (NOX) and liver fibrosis: a review. Cell Biochem Funct (2018) 36(6):292–302. doi: 10.1002/cbf.3351
77. Sacchi A, Grassi G, Bordoni V, Lorenzini P, Cimini E, Casetti R, et al. Early expansion of myeloid-derived suppressor cells inhibits SARS-CoV-2 specific T-cell response and may predict fatal COVID-19 outcome. Cell Death Dis (2020) 11(10):1–9. doi: 10.1038/s41419-020-03125-1
78. Coudereau R, Waeckel L, Cour M, Rimmele T, Pescarmona R, Fabri A, et al. Emergence of immunosuppressive LOX-1+ PMN-MDSC in septic shock and severe COVID-19 patients with acute respiratory distress syndrome. J leukocyte Biol (2021) 111:489–96. doi: 10.1002/JLB.4COVBCR0321-129R
79. Gebremeskel S, Schanin J, Coyle KM, Butuci M, Luu T, Brock EC, et al. Mast cell and eosinophil activation are associated with COVID-19 and TLR-mediated viral inflammation: implications for an anti-Siglec-8 antibody. Front Immunol (2021) 12:650331. doi: 10.3389/fimmu.2021.650331
80. Criado PR, Pagliari C, Criado RFJ, Marques GF, Belda W Jr. What the physicians should know about mast cells, dendritic cells, urticaria, and omalizumab during COVID-19 or asymptomatic infections due to SARS-CoV-2? Dermatologic Ther (2020) 33(6):e14068. doi: 10.1111/dth.14068
81. Gigante A, Aquili A, Farinelli L, Caraffa A, Ronconi R, Gallenga CE, et al. Sodium chromo-glycate and palmitoylethanolamide: a possible strategy to treat mast cell-induced lung inflammation in COVID-19. Med Hypotheses (2020) 143:109856. doi: 10.1016/j.mehy.2020.109856
82. Valent P, Akin C, Bonadonna P, Brockow K, Niedoszytko M, Nedoszytko B, et al. Risk and management of patients with mastocytosis and MCAS in the SARS-CoV-2 (COVID-19) pandemic: Expert opinions. J Allergy Clin Immunol (2020) 146(2):300–6. doi: 10.1016/j.jaci.2020.06.009
83. Murdaca G, Di Gioacchino M, Greco M, Borro M, Paladin F, Petrarca C, et al. Basophils and mast cells in COVID-19 pathogenesis. Cells (2021) 10(10):2754. doi: 10.3390/cells10102754
84. Rodriguez L, Pekkarinen PT, Lakshmikanth T, Tan Z, Consiglio CR, Pou C, et al. Systems-level immunomonitoring from acute to recovery phase of severe COVID-19. Cell Rep Med (2020) 1(5):100078. doi: 10.1016/j.xcrm.2020.100078
85. Pala D, Pistis M. Anti-IL5 drugs in COVID-19 patients: role of eosinophils in SARS-CoV-2-induced immunopathology. Front Pharmacol (2021) 12:622554. doi: 10.3389/fphar.2021.622554
86. Lindsley AW, Schwartz JT, Rothenberg ME. Eosinophil responses during COVID-19 infections and coronavirus vaccination. J Allergy Clin Immunol (2020) 146(1):1–7. doi: 10.1016/j.jaci.2020.04.021
87. Cortés-Vieyra R, Gutiérrez-Castellanos S, Álvarez-Aguilar C, Baizabal-Aguirre VM, Nuñez-Anita RE, Rocha-López AG, et al. Behavior of eosinophil counts in recovered and deceased COVID-19 patients over the course of the disease. Viruses (2021) 13(9):1675. doi: 10.3390/v13091675
88. Zein JG, Strauss R, Attaway AH, Hu B, Milinovich A, Jawhari N, et al. Eosinophilia is associated with improved COVID-19 outcomes in inhaled corticosteroid-treated patients. J Allergy Clin Immunology: In Pract (2022) 10(3):742–750.e14. doi: 10.1016/j.jaip.2021.12.034
89. Hemmi T, Ainai A, Hashiguchi T, Tobiume M, Kanno T, Iwata-Yoshikawa N, et al. Intranasal vaccination induced cross-protective secretory IgA antibodies against SARS-CoV-2 variants with reducing the potential risk of lung eosinophilic immunopathology. bioRxiv (2022) 40:5892–903. doi: 10.1101/2022.05.24.493348
90. Peñaloza HF, Lee JS, Ray P. Neutrophils and lymphopenia, an unknown axis in severe COVID-19 disease. PloS Pathog (2021) 17(9):e1009850. doi: 10.1371/journal.ppat.1009850
91. Rawat S, Vrati S, Banerjee A. Neutrophils at the crossroads of acute viral infections and severity. Mol Aspects Med (2021) 81:100996. doi: 10.1016/j.mam.2021.100996
92. Xiang Q, Feng Z, Diao B, Diao B, Tu C, Qiao Q, Yang H, et al. SARS-CoV-2 induces lymphocytopenia by promoting inflammation and decimates secondary lymphoid organs. Front Immunol (2021) 12:1292. doi: 10.3389/fimmu.2021.661052
93. Thompson EA, Cascino K, Ordonez AA, Zhou W, Vaghasia A, Hamacher-Brady A, et al. Metabolic programs define dysfunctional immune responses in severe COVID-19 patients. Cell Rep (2021) 34(11):108863. doi: 10.1016/j.celrep.2021.108863
94. Abassi Z, Knaney Y, Karram T, Heyman SN. The lung macrophage in SARS-CoV-2 infection: a friend or a foe? Front Immunol (2020) 11:1312. doi: 10.3389/fimmu.2020.01312
95. Pantazi I, Al-Qahtani AA, Alhamlan FS, Alothaid H, Matou-Nasri S, Sourvinos G, et al. SARS-CoV-2/ACE2 interaction suppresses IRAK-m expression and promotes pro-inflammatory cytokine production in macrophages. Front Immunol (2021) 12. doi: 10.3389/fimmu.2021.683800
96. Rodríguez-Puertas R. ACE2 activators for the treatment of COVID 19 patients. J Med Virol (2020) 92:1701–2 doi: 10.1002/jmv.25992.
97. Mazzitelli I, Bleichmar L, Ludueña MG, Pisarevsky A, Labato M, Chiaradia V, et al. IgG immune complexes may contribute to neutrophil activation in the course of severe COVID-19. J Infect Dis (2021) 224:575–85. doi: 10.1093/infdis/jiab174
98. Sacchi A, Grassi G, Notari S, Gili S, Bordoni V, Tartaglia E, et al. Expansion of myeloid derived suppressor cells contributes to platelet activation by l-arginine deprivation during SARS-CoV-2 infection. Cells (2021) 10(8):2111. doi: 10.3390/cells10082111
99. Dean MJ, Ochoa JB, Sanchez-Pino MD, Zabaleta J, Garai J, Del Valle L, et al. Severe COVID-19 is characterized by an impaired type I interferon response and elevated levels of arginase producing granulocytic myeloid derived suppressor cells. Front Immunol (2021) 12:2726. doi: 10.3389/fimmu.2021.695972
100. Peng T, Du S-Y, Son M, Diamond B. HIF-1α is a negative regulator of interferon regulatory factors: Implications for interferon production by hypoxic monocytes. Proc Natl Acad Sci (2021) 118(26):e2106017118. doi: 10.1073/pnas.2106017118
101. Mortezaee K. Hypoxia induces core-to-edge transition of progressive tumoral cells: A critical review on differential yet corroborative roles for HIF-1α and HIF-2α. Life Sci (2020) 242:117145. doi: 10.1016/j.lfs.2019.117145
102. AbdelMassih A, Yacoub E, Husseiny RJ, Kamel A, Hozaien R, El Shershaby M, et al. Hypoxia-inducible factor (HIF): The link between obesity and COVID-19. Obes Med (2020) 22:100317. doi: 10.1016/j.obmed.2020.100317
103. Loo J, Spittle DA, Newnham M. COVID-19, immunothrombosis and venous thromboembolism: biological mechanisms. Thorax (2021) 76(4):412–20. doi: 10.1136/thoraxjnl-2020-216243
104. Hsu T-S, Lin Y-L, Wang Y-A, Mo S-T, Chi P-Y, Chuan-Ying Lai A, et al. HIF-2α is indispensable for regulatory T cell function. Nat Commun (2020) 11(1):1–16. doi: 10.1038/s41467-020-18731-y
105. Kalfaoglu B, Almeida-Santos J, Tye CA, Satou Y, Ono M. T-Cell hyperactivation and paralysis in severe COVID-19 infection revealed by single-cell analysis. Front Immunol (2020) 11:2605. doi: 10.3389/fimmu.2020.589380
106. Colombani T, Eggermont LJ, Rogers ZJ, McKay L, Avena LE, Johnson RI, et al. Biomaterials and oxygen join forces to shape the immune response and boost COVID-19 vaccines. Advanced Sci (2021) 8:2100316. doi: 10.1002/advs.202100316
107. Jia R, Wang X, Liu P, Le W, Xie L, Li H, et al. Mild cytokine elevation, moderate CD4+ T cell response and abundant antibody production in children with COVID-19. Virologica Sin (2020) 35(6):734–43. doi: 10.1007/s12250-020-00265-8
108. Neeland MR, Bannister S, Clifford V, et al. Innate cell profiles during the acute and convalescent phase of SARS-CoV-2 infection in children. Nat Commun (2021) 12(1):1–5. doi: 10.1038/s41467-021-21414-x
109. Zheng Y, Liu X, Le W, Xie L, Li H, Wen W, et al. A human circulating immune cell landscape in aging and COVID-19. Protein Cell (2020) 11(10):740–70. doi: 10.1007/s13238-020-00762-2
110. Günes H, Dincer S, Acipayam C, Yurttutan S, Özkars MY. What chances do children have against COVID-19? is the answer hidden within the thymus? Eur J Pediatr (2021) 180:983–6. doi: 10.1007/s00431-020-03841-y
111. Ferrantelli F, Chiozzini C, Manfredi F, Giovannelli A, Leone P, Federico M, et al. Simultaneous CD8+ T-cell immune response against SARS-Cov-2 s, m, and n induced by endogenously engineered extracellular vesicles in both spleen and lungs. Vaccines (2021) 9(3):240. doi: 10.3390/vaccines9030240
112. Park KS, Bazzill JD, Son S, Nam J, Shin SW, Ochyl LJ, et al. Lipid-based vaccine nanoparticles for induction of humoral immune responses against HIV-1 and SARS-CoV-2. J Controlled Release (2021) 330:529–39. doi: 10.1016/j.jconrel.2020.12.031
113. Machhi J, Shahjin F, Das S, Patel M, Abdelmoaty MM, Cohen JD, et al. A role for extracellular vesicles in SARS-CoV-2 therapeutics and prevention. J Neuroimmune Pharmacol (2021) p:1–19. doi: 10.1007/s11481-020-09981-0
Keywords: SARS-CoV-2, CD8+ T cell, dendritic cell (DC), regulatory T cell (Treg), myeloid-derived suppressor cell (MDSC), neutrophil, monocyte, immunity
Citation: Mortezaee K and Majidpoor J (2022) Cellular immune states in SARS-CoV-2-induced disease. Front. Immunol. 13:1016304. doi: 10.3389/fimmu.2022.1016304
Received: 10 August 2022; Accepted: 31 October 2022;
Published: 23 November 2022.
Edited by:
Vijayakumar Velu, Emory University, United StatesReviewed by:
Daniel Smrz, Charles University, CzechiapCopyright © 2022 Mortezaee and Majidpoor. This is an open-access article distributed under the terms of the Creative Commons Attribution License (CC BY). The use, distribution or reproduction in other forums is permitted, provided the original author(s) and the copyright owner(s) are credited and that the original publication in this journal is cited, in accordance with accepted academic practice. No use, distribution or reproduction is permitted which does not comply with these terms.
*Correspondence: Keywan Mortezaee, a2V5d2FuOTg3QHlhaG9vLmNvbQ==; bW9ydGV6YWVlLmtAbXVrLmFjLmly
Disclaimer: All claims expressed in this article are solely those of the authors and do not necessarily represent those of their affiliated organizations, or those of the publisher, the editors and the reviewers. Any product that may be evaluated in this article or claim that may be made by its manufacturer is not guaranteed or endorsed by the publisher.
Research integrity at Frontiers
Learn more about the work of our research integrity team to safeguard the quality of each article we publish.