- 1Division of Biotherapy, Cancer Center, West China Hospital, Cancer Center, Sichuan University, Chengdu, China
- 2Head & Neck Oncology Ward, Cancer Center, West China Hospital, Cancer Center, Sichuan University, Chengdu, China
- 3West China School of Medicine, West China Hospital, Sichuan University, Chengdu, China
Ovarian cancer is currently one of the most common malignant tumors in females with poor survival rates around the world, killing about 200,000 women each year. Although great progress has been made in treatment, most patients receiving first-line therapy experience tumor recurrence. The tumor microenvironment plays an important role in regulating the progression and prognosis of ovarian cancer. Cancer-associated mesothelial cells are the main cell population in the tumor microenvironment, which affect the progression, prognosis and chemical resistance of ovarian cancer. Cancer-associated mesothelial cells can also interact with other microenvironmental components, such as exosomes, macrophages, and adipocytes. Some studies have developed drugs targeting cancer-associated mesothelial cells in ovarian cancer to evaluate the therapeutic efficiency. In this review we highlighted the key role of cancer-associated mesothelial cells in the progression and prognosis of ovarian cancer. We also described the progress of cancer-associated mesothelial cells targeted therapy for ovarian cancer. Continued insight into the role of cancer-associated mesothelial cells in ovarian cancer will potentially contribute to the development of new and effective therapeutic regiments.
Introduction
Ovarian cancer (OC) is a common malignant cancer among women in the world and has a poor prognosis (1). According to the latest world health organization data, 313959 patients are diagnosed with OC and 207252 die from it worldwide annually (2). Current frontline treatment for OC consists of initial debulking surgery and subsequent consolidation chemotherapy. 70-80% of patients experience recurrence after standard frontline treatment, making the five-year survival reach about 45% (3). The high mortality and recurrence rates in OC patients are mainly due to chemotherapy resistance and widespread abdominal metastasis. Recently, immune therapeutics have been introduced to the ovarian cancer treatment landscape, including but not limited to immune checkpoint inhibition (ICI), tumor antigen vaccines and engineered immune cells (4). However, some clinical trials testing ICI in OC have not delivered positive results (5). More effective treatment is urgently in need.
Tumor microenvironment (TME) plays a key role in tumor progression and response to standard chemotherapy. A ton of basic and preclinical studies suggests that co-treatment targeting TME improves therapeutic effect (6). Cancer-associated mesothelial cells (CAMs) are a major part of the OC microenvironment, contributing to cancer progression and chemoresistance. This review summarizes how CAMs obviously influence the progression and prognosis of OC and reviews several targeted therapies for CAMs.
The effects of CAMs in OC
The peritoneum, the omentum and the serous membranes of the small intestine and large intestine are covered by monolayer mesothelial cells. These cells are the primary barrier to prevent the dissemination of OC cells. Like other epithelial cell layers in the body, such as cervical epithelium, the mesothelial cells act as a protective barrier to protect the underlying tissue from OC cells and limit access to the retroperitoneum. A previous study found that primary human mesothelial cells inhibited the initial adhesion and invasion of at least two OC cell lines and three different early passage human OC cell cultures (7). However, in patients with OC, cancer cells can secrete a series of cancer-promoting factors to induce the mesothelial-mesenchymal transition (MMT) of normal mesothelial cells (8). We defined mesothelial cells differentiated by OC cells stimulation as cancer-associated mesothelial cells (CAMs). Compared with mesothelial cells, CAMs undergo obvious morphological changes, and the polarity of cytoskeleton becomes disordered. CAMs also showed significant epithelial-mesenchymal transition (EMT) characteristics, such as the increase of fibronectin, α-SMA and vimentin and reduction of E-cadherin. CAMs no longer have a protective effect, but secrete chemokines to promote the peritoneal metastasis and chemoresistance of ovarian cancer cells.
CAMs and peritoneal metastasis in OC
During the process of peritoneal metastasis of OC, CAMs promote the adhesion and invasion of OC cells to the peritoneum through regulating the expression of multiple chemokines. A recent study found that the expression of intelectin-1 (ITLN1) in CAMs and serum ITLN1 levels in OC patients were significantly lower than those in healthy women. ITLN1 fused with lactotransferrin (LTF) and dampened the binding of LTF to its receptor on the surface of OC cells, low-intensity lipoprotein-receptor-related protein 1(LRP1). ITLN1 attached to LRP1 and transcriptionally increased the expression of MMP1, which contributed to the invasion and metastasis of cancer cells. Simultaneously, ITLN1 inhibited the invasion ability of OC cells by suppressing LTF-induced calcium mobilization and stress fiber formation. In addition, ITLN1 increased recombinant glucose transporter-4 (GLUT4) production in adipocytes, which contributed to increased glucose uptake by adiposes and decreased glucose uptake by tumor cells, thereby the proliferation of OC cells was inhibited. In vivo experiments, treatment with recombinant ITLN1 inhibited OC growth (9). In ovarian cancer, hypoxic microenvironment induced CAMs and cancer cells to stabilize HIF-1 and HIF-2. HIF signaling upregulated collagen prolyl 4-hydroxylases (P4HA1, P4HA2 and P4HA3), lysyl hydroxylases (PLOD1 and PLOD2) and lysyl oxidase (LOX) to facilitate the crosslinking and deposition of extracellular collagen type I in mesothelial cells, finally contributed to OC cells metastasis (10). CAMs can also secret several cytokines to promote the metastasis of OC. CAMs were reported to increase the secretion of IL-8 (11) and CCL2 (12). IL-8 secreted by CAMs induced the overexpression of pyruvate dehydrogenase kinase-1 (PDK1) in OC cells via CXCR1. PDK1 upregulated the expression of α5 and β1 integrin to enhance the adhesion to fibronectin and mesothelial cells. PDK1 also activated JNK signaling to induce IL-8 production in OC cells (11). In addition, IL-8 bound to CXCR1 and CXCR2 on endothelial cells situated on subperitoneal tissue to promote tumor neovascularization (13). CCL2 facilitated the trans-mesothelial migration and invasion of OC cells via activating p38-MAPK pathway through CCR2 (12). Pericellular hyaluronic acid (HA) secreted by CAMs can bind to CD44v3-Vav2 complex on OC cells to activate RhoGTPase (Rac1) pathway signaling, in turn, promoted the activation of cytoskeleton, finally facilitating cancer cells invasion. Simultaneously, HA bound to CD44v3- p185HER2 complex to promote p185HER2 tyrosine kinase (TK) activation, and then the adaptor molecule Grb2 was recruited. Grb2 not only activated Ras pathway signaling to regulate cancer cells growth, but also interacted with Vav2 to activate Rac1 pathway signaling (14). High levels of Wnt5a deriving from CAMs in ascites fluid boosted the metastasis of OC cells via activating its downstream effector Src family kinase Fgr (15). A previous study found that CAMs generated lysophosphatidic acid (LPA) via cytosolic phospholipase A2 (cPLA2) and calcium-independent phospholipase A2 (iPLA2) activity to activate extracellular signal-regulated kinase (ERK) and Akt pathway in OC cells, in turn, boosted OC cells to adhere to collagen I, finally promoted the metastasis of OC (16).
The intricate crosstalk between CAMs and cancer cells facilitates the metastasis of OC. Transforming growth factor-β (TGF-β) derived from OC cells induced the phenotypic changes of mesothelial cells to CAMs (17). CAMs increased the secretion of vascular endothelial growth factor (VEGF) in a TGF-β-dependent manner. VEGF secreted by CAMs acted on endothelial cells situated in subperitoneal space and boosted their migratory potential and tube formation ability, thereby promoting tumor neovascularization (18). TGF-β also activated RAC1/SMAD3 pathway via TGF-βRII to induce CAMs to upregulate fibronectin expression. Fibronectin in extracellular matrix binds to α5 and β1 integrin on OC cells to support the metastasis (19). Moreover, extrinsic TGF-β derived from OC cells induced the extra secretion of TGF-β from CAMs, leading to a cumulative effect of TGF-β (20). A previous study showed that OC cells secreted hepatocyte growth factor (HGF) to induce MCs to differentiate into CAMs (21). Hepatocyte growth factor (HGF) derived from OC cells also promoted the premature senescence of normal mesothelial cells by inducing mitochondrial oxidative stress via activating several signaling pathways including p38-MAPK, AKT and NF-κB (22). Senescent mesothelial cells upregulated the expression of fibronectin (FN) (23) and downregulated the expression of junctional proteins, such as connexin 43, E-cadherin, occludin and desmoglein, leading to destruction of the integrity of the peritoneal mesothelium and makes it easier for the invasion of ovarian cancer (24). Senescent mesothelial cells also secreted angiogenic agents such as CXCL1, CXCL8 and VEGF to stimulate subperitoneal tumor neovascularization (25). In addition, OC cells overexpressed plasminogen activator inhibitor-1 (PAI-1) and transcription factor DLX4 to induce the expression of IL-8/CXCL5 and IL-1β/CD44 via activating NF-κB signaling, further enhancing tumor-mesothelial cell interactions and facilitating the metastasis (26, 27).
A schematic illustration of the interaction between CAMs and OC cells to promote metastasis is shown in Figure 1.
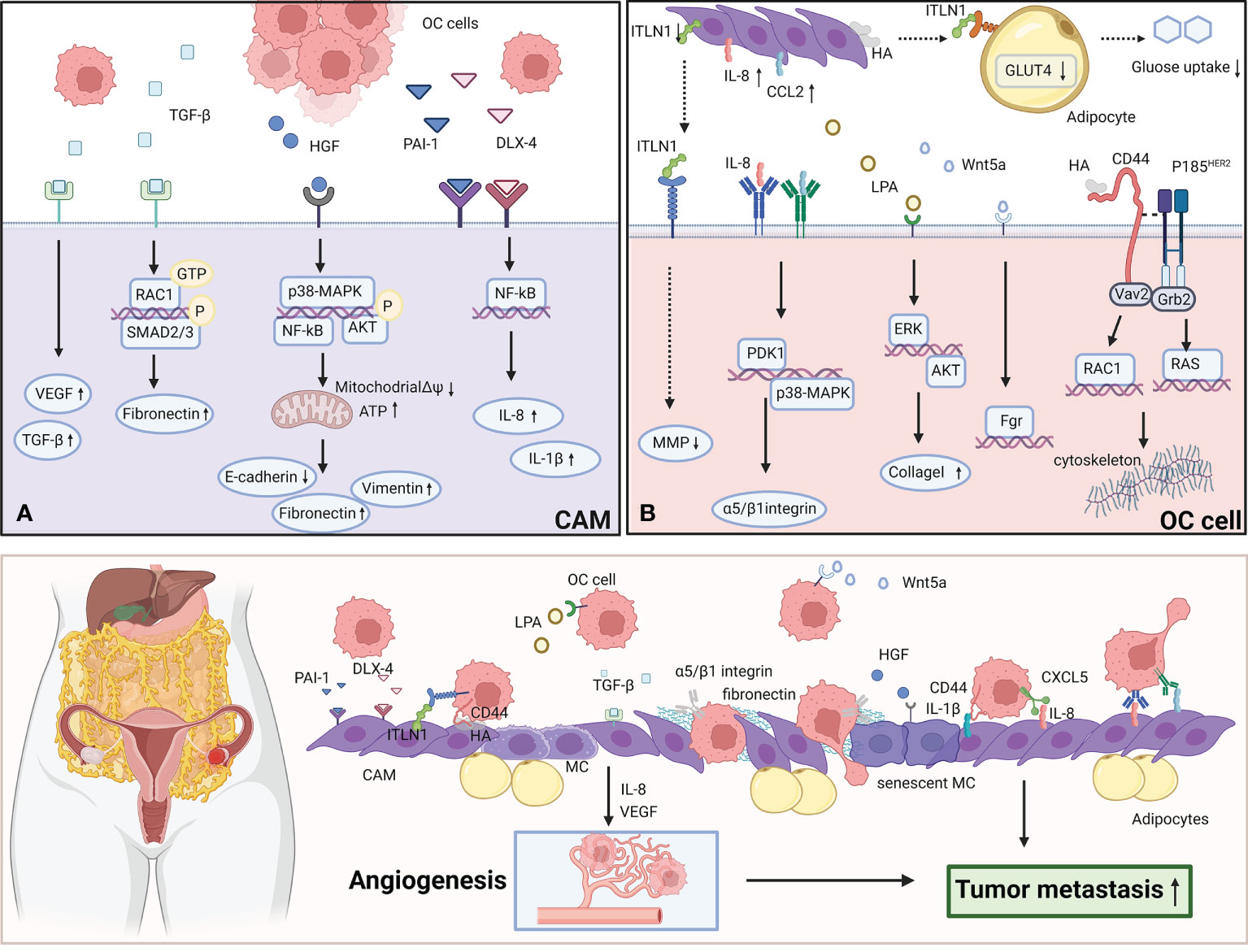
Figure 1 CAMs interact with OC cells to promote the metastasis. (A) OC cells secret TGF-β, HGF, PAI-1, DLX-4 to effect CAMs via various signaling pathways. TGF-β activates RAC1/SMAD3 pathway via TGF-βRII to induce CAMs to upregulate fibronectin expression. HGF promotes the premature senescence of normal mesothelial cells by inducing mitochondrial oxidative stress via activating several signaling pathways including p38-MAPK, AKT and NF-κB. PAI-1 and DLX4 induce the expression of IL-8/CXCL5 and IL-1β/CD44 via activating NF-κB signaling. (B) CAMs overexpress ITLN1, IL-8, CCL2, LPA, Wnt5a and HA to effect OC cells by activating several signaling pathways. IL-8 induces the overexpression of PDK1 in OC cells via CXCR1.PDK1 upregulates the expression of α5 and β1 integrin to enhance the adhesion to fibronectin and mesothelial cells. CCL2 facilitates the trans-mesothelial migration and invasion of OC cells via activating p38-MAPK pathway through CCR2. Wnt5a boosts the metastasis of OC cells via activating its downstream effector Src family kinase Fgr. LPA activates ERK and Akt pathway to boost OC cells to adhere to collagen I. HA can bind to CD44v3-Vav2 complex on OC cells to activate Rac1 and Ras pathway signaling. The figure was created with BioRender.com.
CAMs and chemoresistance in OC
Chemoresistance is a primary drawback in the treatment of OC. Multiple studies have demonstrated that HA-CD44 interaction facilitated chemoresistance in various cancers via several signaling, such as breast cancer and multiple myeloma (28, 29). In OC, the binding of HA to CD44-Nanog complex activated the expression of Nanog-special target genes Rex1 and Sox2. Nanog activation was determined to be closely related to maintaining the stem cell properties of cancer cells. Some activated Nanog interacted with STAT3 to upregulate the expression of multidrug resistance-1 (MDR1) gene, which contributed to the chemoresistance of cancer cells. In addition, HA facilitated the interaction of ankyrin-MDR1 (P-gp) with CD44 and the complex led to chemotherapeutic drugs efflux in OC cells (30). Similarly, another study found that HA induced the expression of membrane ATP binding cassette (ABC) transporter proteins in OC cells to increase chemo resistance (31).
Recently, a study found that CAMs can secret osteopontin (OPN) to media chemoresistance and stemness in OC. OC cells induced CAMs to upregulate the expression and secretion of OPN in a TGF-β dependent manner. OPN activated HA/CD44/PI3K-AKT signaling pathway to promote the expression of ABC transporter proteins and regulate BCL-2/BAX ratio, finally contributed to boosting chemoresistance (32). Another mechanistic study indicated that the overexpression of FN in CAMs also reduced platinum-sensitivity in OC cells by activating Akt signaling pathway (33). Moreover, the OC spheroids display enhanced resistance to anti-cancer drugs compared to monolayers, while CAMs promoted spheroid formation by OC cells and induced their motility (34, 35). Chemoresistance OC cells showed a higher ability to adhere and grow on mesothelium, which enhances the dissemination and invasion of cancer cells. A schematic illustration of CAMs promoting chemoresistance in OC is shown in Figure 2A.
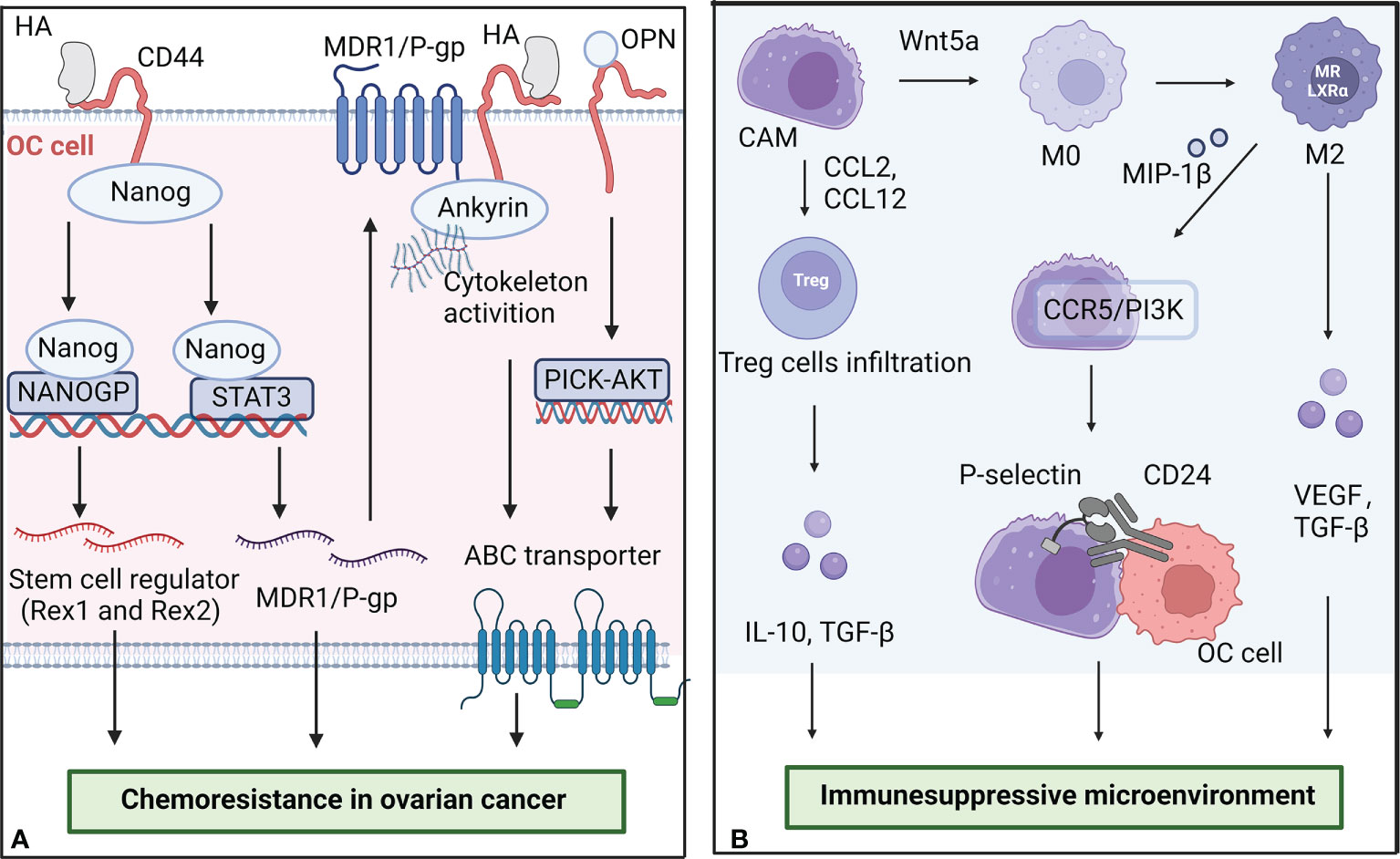
Figure 2 The role of CAMs in chemoresistance and the formation of immunesuppressive microenvironment. (A) CAMs secret HA and OPN to promote the chemoresistance of OC cells. The binding of HA to CD44-Nanog complex activated the expression of Nanog-special target genes Rex1 and Sox2. Some activated Nanog interacted with STAT3 to upregulate the expression of multidrug resistance-1 (MDR1) gene. OPN activated HA/CD44/PI3K-AKT signaling pathway to promote the expression of ABC transporter proteins. (B) CAMs interact with other cells in the microenvironment to promote the formation of immunesuppressive microenvironment in OC. CAMs can secrete Wnt5a to regulate macrophage polarization and increase T regulatory cell infiltration. M2 macrophages promote the adhesion of CAMs and OC cells by overexpressing MIP-1β. The figure was created with BioRender.com.
CAMs and prognosis of OC
Peritoneal metastasis and chemoresistance dramatically influence the prognosis of OC. Secretion of CAMs such as OPN and ITLN1 have been demonstrated to predict overall survival rates in mice (9, 32). A recent scRNA-seq study, which analyzed 18,403 cells gathered from seven untreated patients with high-grade serous tubo-ovarian cancer, identified 6 cellular phenotypes associated with prognosis (36). It was found that concentration of CAMs was correlated with poor outcome. A prospective observational cohort study found that the expression of vascular cell adhesion molecule-1 (VCAM-1) on the CAMs negatively correlated with progression-free and overall survival in OC (37). Moreover, patients with consistently high VCAM-1 expression were more likely to develop platinum resistance than patients expressing low VCAM-1.
CAMs and microenvironment in OC
The highly inhibitory immune microenvironment is considered to be one of the dominant reasons for tumor progression and treatment failure in OC patients. As a key part of the tumor microenvironment, CAMs interact with other cells in the microenvironment to regulate the progression of OC. A schematic illustration of CAMs interacting with other cells in the microenvironment in OC is shown in Figure 2B.
CAMs and exosomes
Exosomes are 30-100nm membrane vesicles of endocytosis origin, mediating cell-cell communication and antigen presentation via transferring proteins, mRNAs, and microRNAs (38). Recent reports displayed that tumor-derived extracellular exosomes played an important role in communication between CAMs and OC cells to induce immunosuppression, thereby promoting the direct adhesion and invasion of cancer cells to CAMs. For example, OC-derived exosomes carrying CD44 reprogramed mesothelial cells to a more EMT phenotype, which facilitated cancer adhesion and invasion (39). Similarly, via co-culturing exosomal annexin A2 (ANXA2) derived from OC cells with human peritoneal mesothelial cells, researchers found that ANXA2 activated PI3K/AKT/mTOR pathway to promote MMT and the degradation of the extracellular matrix of mesothelial cells, finally facilitating establishing pre-metastasis microenvironment of OC (40). In addition, some researchers proposed that OC-derived extracellular vesicles containing MMP1 mRNA induced the apoptosis of mesothelial cells, which exposed the underlying tissue and facilitated peritoneum colonization (41).
CAMs and macrophages
CAMs can secrete Wnt5a to regulate macrophage polarization. High levels of Wnt5a in ascites fluids activated the Src family kinase Fgr to enhance the immunosuppressive immune landscape of OC and promote peritoneal colonization. The knockout of Wnt5a contributed to an increase in M1 macrophages and a decrease in M2 macrophages in a mouse model of ovarian cancer (15). Generally, M1 macrophages secrete proinflammatory cytokines such as TNF-α, IL-1 and IL-6, which promotes anti-tumor immune response and enhances immune monitoring, while M2 macrophages mediate immunosuppressive response and promote chronic inflammation and tumor invasion mainly by secreting inhibitory cytokines such as TGF-β, VEGF and MMPs. Moreover, Wnt5a expression increased CCL2, CCL12, CXCL10, and CXCL12 production, which correlating with T regulatory cell and tumor-associated macrophage infiltration.
CAMs influence macrophage polarization, while macrophages can also regulate CAMs to promote the adhesion and invasion of ovarian cancer. M2 macrophages can secrete MIP-1β to activate CCR5/PI3K signaling and then increase P-selectin production by CAMs. P-selectin binds to CD24 on the OC cells surface, leading to increased adhesion of cancer cells (42). Anti-P-selectin antibody and small molecular inhibitor were demonstrated to inhibit OC cells adhesion in vivo and in vitro.
CAMs and cancer-associated fibroblasts (CAFs)
During peritoneal metastasis, tumor cells can induce the transformation of mesothelial cells into CAFs. A previous study found the presence of CAFs expressing mesothelial markers at the site of tumor implantation in patients with peritoneal dissemination (8). By single-cell RNA sequencing and spectral tracing assays, a recent study demonstrated that antigen-presenting CAFs were derived from mesothelial cells. Further study revealed that IL-1 and TGF-β can induce mesothelial cells to downregulate mesothelial features and acquire fibroblastic features during tumor progression. Antigen-presenting CAFs induced regulatory T cells formation and expansion in an antigen-specific manner, which contributes to tumor immune escape (43).
CAMs and adipocytes
OC cells are prone to metastasize to the omental fat pad (44). Omental adipocytes release and transport free fatty acids to maintain the high energy requirements of cancer cells (45). In diet-induced obesity mice, the density of microvilli on peritoneal mesothelial cells was significantly increased, which contributes to early cell-cell adhesive events in metastatic colonization in ovarian cancer (46). Moreover, the downregulation of ITLN1 in CAMs inhibited the insulin-dependent glucose uptake in mature adipocytes via suppressing the expression of GLUT4, which increased the glucose available to OC cells and promote the tumor growth (9).
Targeting CAMs in therapy of OC
CAMs interact with OC cells via expressing some specific markers, such as MSLN, FN and HA. Some studies have developed antibodies targeting these markers to block the interaction of CAMls with OC cells, thereby inhibiting the progression of OC and improving the prognosis of OC survivors.
MUC16 - Mesothelin
MUC16 is a glycoprotein that is overexpressed by OC cells. The shedding of MUC16 from the surface of OC cells to circulation is the basis of serum assay CA125 in clinical. Mesothelin (MSLN) is a differentiation antigen mainly expressed on CAMs, OC cells and mesothelioma cells. MUC16–MSLN interaction mediated the attachment and adhesion of OC cells to CAMs. Blocking the MUC16–MSLN interaction can effectively inhibit cancer cell adhesion and invasion.
Initially, antibodies against MUC16 were developed for the immunotherapy of OC, such as murine IgG1 oregovomab (mAb B43.13, OvaRex) (47). However, the treatment with oregovomab monotherapy failed to prolong the survival of patients with advanced OC in a phase III clinical trial (48). The combination therapy with oregovomab plus carboplatin/paclitaxel effectively improved overall and progression-free survival (49). Some clinical trials of oregovomab in combination with other drugs are also underway, such as bevacizumab and niraparib (ClinicalTrials.gov identifier: NCT04938583; NCT05335993). Later, Antibody–drug conjugates (ADCs) targeting the repetitive MUC16 epitopes were developed (50), which were revealed to have higher antitumor activity, such as 3A5-MMAE (monomethyl auristatin E) (51). Constructing chimeric antigen receptor (CAR) targeting to the retained extracellular domain of MUC16 (MUC-CD) has also been demonstrated to exhibit efficient antitumor activity in vitro and in vivo (52). Recently, a human bispecific T-cell engaging antibodies (REGN4018) bridging MUC16-expressing cells with CD3 T cells was developed (53). In preclinical studies and toxicology studies, REGN4018 displayed potent antitumor activity and good tolerability. Moreover, the combination of bispecific T-cell engaging antibodies and anti-VEGF enhanced the efficacy (54). Using an oncolytic adenovirus carrying a MUC16- bispecific T-cell engagers (BiTEs) can activate and retarget CTLs to enhance the anti-tumor effect (55). Currently, Phase 1/2 trails are recruiting patients (ClinicalTrials.gov identifier: NCT03564340; NCT04590326).
MORAb-009, a chimeric antibody targeting MSLN, is being investigated in multiple clinical studies. Patients with MORAb-009 treatment exhibited more stable disease and clear increase in serum MUC16, which suggesting MORAb-009 disturbs the MSLN–MUC16 interaction (56). Anti-MSLN antibody-drug conjugate anetumab ravtansine is composed of a human anti-MSLN IgG1 and a maytansine derivative tubulin inhibitor DM4, which shows selective and potent antitumor activity in xenograft tumor models (57). In a phase I multicenter trial, anetumab ravtansine displayed a manageable safety, favorable pharmacokinetics and preliminary antitumor activity in patients with mesothelin-expressing solid tumors (58). Additionally, A randomized phase II Study is underway (ClinicalTrials.gov identifier: NCT03587311). Anti-MSLN CAR-T cells are in progress with some clinical trials (ClinicalTrials.gov identifier: NCT04562298; NCT04503980). In orthotopic mouse models of OC, MSLN-directed CAR T cells provided antitumor immunity and significantly prolonged survival (59). Clinical trials of MUC16-MSLN targeted drugs are described in detail in Table 1.
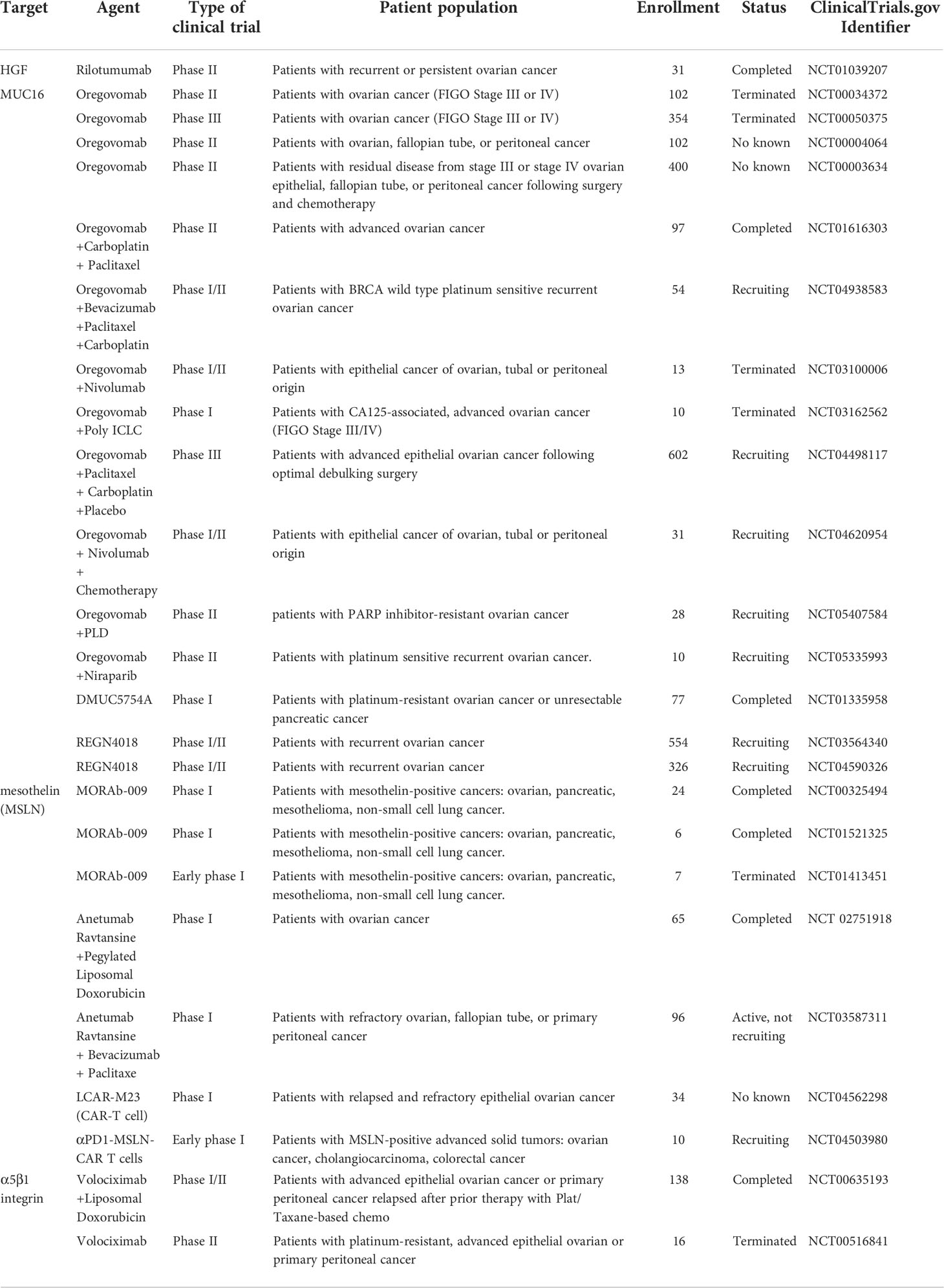
Table 1 Summary of clinical trials using MUC16-mesothelin (MSLN) and FN - α5β1 integrin targeted agents.
FN - α5β1 integrin
FN secreted by CAMs is one of the most abundant extra-cellular matrix proteins in the peritoneal microenvironment. OC cells can adhere to FN via α5β1 integrin and directly induce phosphorylation of focal adhesion kinase (FAK), further leading to activation of mitogenic pathways supporting tumor growth (60). Blocking antibodies against α5β1 integrin effectively inhibited OC cells adhesion to mesothelial cells. Volociximab is a high-affinity, chimeric antibody directed against human α5β1 integrin. However, in a phase II, single-arm study, volociximab treatment failed to achieve sufficient clinical activity in patients with recurrent, platinum-resistant ovarian cancer (61). The disappointed result may be related to the use of a single agent intervention in recurrent and advanced OC patients. Combination therapy with volociximab in low-volume residual disease after cytoreductive surgery or as maintenance therapy to prevent recurrence of ovarian cancer may be effective. Moreover, a previous study reported that resveratrol decreased cellular α5β1 integrin level to inhibit ovarian cancer cell adhesion to CAMs in vitro (62).
Tissue transglutaminase (TG2) is a transpeptidase that promotes the formation of FN - α5β1 integrin complexes by interacting with FN. A function-inhibiting antibody against the TG2 FN-binding domain suppressed complexes formation and blocked the proliferation of cancer stem cells (63). Compound ITP-79 inhibited the binding of TG2 peptide to the 42-KDA FN fragment in a dose-dependent manner, thereby disrupting FN - α5β1 integrin complexes and blocking the adhesion of cancer cells to mesothelial cells (64). FN - α5β1 integrin complexes targeting strategies need to be further optimized and tested for safety, tolerability and efficacy in clinical trials in the future. Clinical trials of FN - α5β1 integrin targeted drugs are described in detail in Table 1.
HA-CD44
The binding of HA derived from CAMs to CD44 expressed on OC plays a significant part in promoting tumor metastasis and chemoresistance. Formerly, HA-based drugs have been shown to have anticancer activity in human OC nude mouse xenograft models (65). A study showed that CD44 targeting HA nanoparticles successfully delivered MDR1 siRNA into OC cells, and the nanoparticles combined with paclitaxel improved the sensitivity of MDR cells to paclitaxel and overcome the chemoresistance of OC (66). Subsequently, various HA-conjugated nanomedicines were developed to delivery chemotherapeutic agents such as Granzyme B, paclitaxel and FAK siRNA (67, 68). Some clinical trials have demonstrated the safety and tolerance of HA-based nanoconstructs in colon cancer (69). In the future, clinical trials are needed to further explore the efficacy of CD44 targeting HA-conjugated nanomedicines in the treatment of OC.
HGF
HGF derived from OC promoted the premature senescence of normal mesothelial cells. Senescent mesothelial cells facilitated mesothelial clearance and tumor angiogenesis. A separate study showed HGF led to chemoresistance of OC by upregulating the MET/PI3K/Akt pathway (70). Rilotumumab (AMG 102) is an anti-HGF monoclonal antibody developed to neutralize the biological activity of HGF, thus blocking the HGF/MET pathway. However, in a phase II clinical trail, rilotumumab monotherapy showed limited benefit in patients suffering recurrent or persistent OC (71). This implies that HGF inhibitor combined with other therapeutic strategies may potentially improve efficacy and overcome chemoresistance.
Other drugs mechanism effect CAMs for OC treatment
Several studies have shown that drugs normally used to treat tumors can also modulate CAMs, such as vitamin D, metformin, tamoxifen and so on.
Vitamin D
Some epidemiological studies suggest that low circulating level of vitamin D is related to poor outcome in patients with various cancers (72, 73). In a meta-analysis of randomized controlled trials, vitamin D supplementation therapy can significantly reduce cancer-related mortality (74). A recent study found that vitamin D inhibited the EMT of mesothelial cells to suppress tumorigenesis in OC (75). Mechanistically, vitamin D inhibited thrombospondin-1 expression by suppressing Smad-dependent TGF-β signaling through VDR-SMad3 competition, which blocking the interaction between CAMs and cancer cells. In particular, the stabilized mesenchymal state of CAMs was restored to its normal epithelial state of preventing cancer cell adhesion and growth by adding vitamin D. Moreover, vitamin D was confirmed to reduce MMPs secretion in cancer-associated mesothelial cells. The inhibition of TGF-β signaling and MMPs secretion can enhance the efficacy of immune checkpoint inhibitors (76, 77). These results displayed that the combination of vitamin D and chemotherapy may be effective in advanced ovarian cancer.
β-Escin
β-Escin is the main active component in horse chestnut seed extract, and its anticancer activity has also been reported in various cancers. A study using a three-dimensional quantitative high-throughput screening platform (3D-qHTS) to screen 2420 naturally extracted compounds found thatβ-escin can effectively suppress migration and viability of OC cells in vitro. In further mechanistical study, β-escin treatment regulated HIF1α stability and reduced the expression of fibronectin, laminin-C1, tenascin, and collagen1-a2 in CAMs in mouse, which contributing to the decreased ability of OC cells to adhere and invade (78).
Metformin
Metformin is a common drug used to treat type 2 diabetes. Recent epidemiological studies have shown that metformin has antitumor effects. A prospective phase II clinical trial found that metformin treatment was well tolerated in nondiabetic OC patients and contributed to better median overall survival (OS) (79). Metformin may target multiple immune cells in OC, such as T cells, myeloid-derived suppressor cells (MDSCs), neutrophils and macrophage (80–83). Recently, metformin was reported to alter CAMs in the omental microenvironment (84). Metformin inhibited the expression of tricarboxylic acid (TCA) enzyme succinyl CoA ligase (SUCLG2), activated prolyl hydroxylases (PHDs), finally leading to the inhibition of TGF-β-driven metabolic upregulation of HIF1α in CAMs. The degradation of HIF1α contributed to reducing CCL2 and IL-8 production, thereby blocking the invasion of OC cells to mesothelial cells.
Acacetin
Acacetin is a natural flavonoid widely found in vegetables. Previous studies suggested that acacetin showed anti-cancer efficacy in various cancers. In a mouse model of gastric cancer, acacetin treatment delayed the development of peritoneal metastasis via inhibiting PI3K/Akt/Snail signal pathway (85). Recently, emerging evidence has confirmed that acacetin inhibits CAMs-evoked malignant characteristics and reduces PCNA and MMPs secretion, which suppressing the proliferation and invasion of OC cells (86). Mechanically, acacetin can suppress LPA secretion in CAMs and further block the activation of receptor for advanced glycation end-products (RAGE)-PI3K/AKT signaling in OC cells. Moreover, acacetin decreased the secretion of pro-inflammatory cytokine IL-6 and IL-8 production in CAMs.
L-carnosine
L-carnosine is a dipeptide widely distributed in human tissues, and it has anti-senescence and anti-cancer properties. Some studies showed that L-carnosine prolonged the replication life of somatic cells and inhibited the growth of cancer cells in vitro and in vivo (87). Interestingly, a previous report found that L-carnosine retarded senescence of human peritoneal mesothelial cells and suppressed progression of OC cells (88, 89). As mentioned earlier, mesothelial cells are peculiarlysusceptible to oxidative stress, which facilitates their senescence. L-carnosine can reduce mitochondrial oxidative stress by improving the cell’s ability to produce ATP, thereby leading to a compensatory reduction in mitochondrial biogenesis and superoxide production. Moreover, L-carnosine decreases various pro-cancerogenic factors secretion by CAMs, such as IL6, IL8, GRO1, PAI 1 and TGFβ1.
HSVTK-modified CAMs
CAMs can be also used as drug carriers to enhance antitumor effects. A previous study engineered CAMs with the herpes simplex virus thymidine kinase/ganciclovir (HSVTK/GCV) system (90). Engineered CAMs can deliver the HSVTK bystander effect to human OC cells and induce the apoptosis of cancer cells. Intraperitoneal administration of HSVTK-expressing CAMs resulted in reduced tumor growth and prolonged survival in mouse model of OC. Moreover, distribution studies showed that engineered CAMs were preferentially located in tumor sites.
Tamoxifen
Tamoxifen is an estrogen receptor modulator that has been shown to be used in the treatment of chronic peritoneal diseases. In mice peritoneal dialysis model, tamoxifen blocked TGF-β1-induced MMT of normal mesothelial cells, thereby inhibiting peritoneal fibrosis (91). Tamoxifen also inhibited GSK-3β/β-catenin signal pathway to attenuate peritoneal fibrosis (92). In the future, tamoxifen can be used as a prevention against mesothelial cells transformation in improving the treatment of OC peritoneal metastasis (93).
Eficiencies and prospects
OC is a fatal disease with a high recurrence rate and a low 5-year survival. Immunotherapy has a lower successful ratio in OC compared with other immunogenic tumors, such as non-small cell lung cancer (NSCLC) and melanoma. In the last two decades, improvements in surgical approaches and the development of chemotherapeutic agents have led to improved survival rates in patients with advanced ovarian cancer. However, cytotoxic drug therapy is non-selective and usually results in transient antitumor responses and significant toxicity. The vast majority of women with ovarian cancer develop drug resistance after receiving first-line chemotherapy (94). There is increasing evidence that TME plays an important role in shaping tumor heterogeneity and drug resistance (95). Some studies have analyzed the feasibility of modifying TME as a treatment for OC. Exploring immunotherapies targeting the components of TME, such as dysfunctional immune cells, exosomes, CAMs and metabolites, would help to develop immunotherapies in OC. Mesothelial cells are the major components of OC microenvironment. They are arranged in the viscera and wall of peritoneal cavity, and are widely present in malignant ascites. Some studies have found that CAMs are closely related to the intraperitoneal metastasis, chemical resistance and tumor recurrence of OC (32, 33). In addition, some therapies that attempt to target CAMs have proved effective. For example, in a preclinical study, therapeutic targeting of CAMs-derived OPN enhances cisplatin response by increasing drug concentrations and DNA damage in OC cells (32). Blocking the interaction of CAMs with OC cells by using neutralizing antibody or aptamers has also been shown to be effective in vivo and in vitro. The review highlighted the key role of CAMs in the progression and prognosis of OC. We also described the progress of CAMs targeted therapy for OC. As the understanding of the mechanisms by which the TME effects OC progression and metastasis continue to improve, new therapeutic targets will be identified and validated, potentially contributing to the development of new and effective therapeutic regiments.
Author contributions
AZ wrote original draft, drew the figure and made the summarizing table. YW, YZ and TZ corrected the draft and wrote the final version. All authors have read and agreed to the published version of the manuscript.
Acknowledgments
We acknowledge the editors and the reviewers for insightful suggestions on this work.
Conflict of interest
The authors declare that the research was conducted in the absence of any commercial or financial relationships that could be construed as a potential conflict of interest.
Publisher’s note
All claims expressed in this article are solely those of the authors and do not necessarily represent those of their affiliated organizations, or those of the publisher, the editors and the reviewers. Any product that may be evaluated in this article, or claim that may be made by its manufacturer, is not guaranteed or endorsed by the publisher.
References
1. Siegel RL, Miller KD, Fuchs HE, Jemal A. Cancer statistics, 2021. CA Cancer J Clin (2021) 71(1):7–33. doi: 10.3322/caac.21654
2. Sung H, Ferlay J, Siegel RL, Laversanne M, Soerjomataram I, Jemal A, et al. Global cancer statistics 2020: Globocan estimates of incidence and mortality worldwide for 36 cancers in 185 countries. CA Cancer J Clin (2021) 71(3):209–49. doi: 10.3322/caac.21660
3. Gogineni V, Morand S, Staats H, Royfman R, Devanaboyina M, Einloth K, et al. Current ovarian cancer maintenance strategies and promising new developments. J Cancer (2021) 12(1):38–53. doi: 10.7150/jca.49406
4. Morand S, Devanaboyina M, Staats H, Stanbery L, Nemunaitis J. Ovarian cancer immunotherapy and personalized medicine. Int J Mol Sci (2021) 22(12):6532. doi: 10.3390/ijms22126532
5. Barber E, Matei D. Immunotherapy in ovarian cancer: We are not there yet. Lancet Oncol (2021) 22(7):903–5. doi: 10.1016/s1470-2045(21)00303-x
6. Nwani NG, Sima LE, Nieves-Neira W, Matei D. Targeting the microenvironment in high grade serous ovarian cancer. Cancers (Basel) (2018) 10(8):266. doi: 10.3390/cancers10080266
7. Kenny HA, Krausz T, Yamada SD, Lengyel E. Use of a novel 3d culture model to elucidate the role of mesothelial cells, fibroblasts and extra-cellular matrices on adhesion and invasion of ovarian cancer cells to the omentum. Int J Cancer (2007) 121(7):1463–72. doi: 10.1002/ijc.22874
8. Sandoval P, Jiménez-Heffernan JA, Rynne-Vidal Á, Pérez-Lozano ML, Gilsanz Á, Ruiz-Carpio V, et al. Carcinoma-associated fibroblasts derive from mesothelial cells Via mesothelial-to-Mesenchymal transition in peritoneal metastasis. J Pathol (2013) 231(4):517–31. doi: 10.1002/path.4281
9. Au-Yeung CL, Yeung TL, Achreja A, Zhao H, Yip KP, Kwan SY, et al. Itln1 modulates invasive potential and metabolic reprogramming of ovarian cancer cells in omental microenvironment. Nat Commun (2020) 11(1):3546. doi: 10.1038/s41467-020-17383-2
10. Natarajan S, Foreman KM, Soriano MI, Rossen NS, Shehade H, Fregoso DR, et al. Collagen remodeling in the hypoxic tumor-mesothelial niche promotes ovarian cancer metastasis. Cancer Res (2019) 79(9):2271–84. doi: 10.1158/0008-5472.Can-18-2616
11. Siu MKY, Jiang YX, Wang JJ, Leung THY, Ngu SF, Cheung ANY, et al. Pdk1 promotes ovarian cancer metastasis by modulating tumor-mesothelial adhesion, invasion, and angiogenesis Via A5β1 integrin and Jnk/Il-8 signaling. Oncogenesis (2020) 9(2):24. doi: 10.1038/s41389-020-0209-0
12. Yasui H, Kajiyama H, Tamauchi S, Suzuki S, Peng Y, Yoshikawa N, et al. Ccl2 secreted from cancer-associated mesothelial cells promotes peritoneal metastasis of ovarian cancer cells through the P38-mapk pathway. Clin Exp Metastasis (2020) 37(1):145–58. doi: 10.1007/s10585-019-09993-y
13. Waugh DJ, Wilson C. The interleukin-8 pathway in cancer. Clin Cancer Res (2008) 14(21):6735–41. doi: 10.1158/1078-0432.Ccr-07-4843
14. Bourguignon LY, Zhu H, Zhou B, Diedrich F, Singleton PA, Hung MC. Hyaluronan promotes Cd44v3-Vav2 interaction with Grb2-P185(Her2) and induces Rac1 and ras signaling during ovarian tumor cell migration and growth. J Biol Chem (2001) 276(52):48679–92. doi: 10.1074/jbc.M106759200
15. Asem M, Young AM, Oyama C, Claure de la Zerda A, Liu Y, Yang J, et al. Host Wnt5a potentiates microenvironmental regulation of ovarian cancer metastasis. Cancer Res (2020) 80(5):1156–70. doi: 10.1158/0008-5472.Can-19-1601
16. Ren J, Xiao YJ, Singh LS, Zhao X, Zhao Z, Feng L, et al. Lysophosphatidic acid is constitutively produced by human peritoneal mesothelial cells and enhances adhesion, migration, and invasion of ovarian cancer cells. Cancer Res (2006) 66(6):3006–14. doi: 10.1158/0008-5472.Can-05-1292
17. Rynne-Vidal A, Au-Yeung CL, Jiménez-Heffernan JA, Pérez-Lozano ML, Cremades-Jimeno L, Bárcena C, et al. Mesothelial-to-Mesenchymal transition as a possible therapeutic target in peritoneal metastasis of ovarian cancer. J Pathol (2017) 242(2):140–51. doi: 10.1002/path.4889
18. Fujikake K, Kajiyama H, Yoshihara M, Nishino K, Yoshikawa N, Utsumi F, et al. A novel mechanism of neovascularization in peritoneal dissemination Via cancer-associated mesothelial cells affected by tgf-B derived from ovarian cancer. Oncol Rep (2018) 39(1):193–200. doi: 10.3892/or.2017.6104
19. Kenny HA, Chiang CY, White EA, Schryver EM, Habis M, Romero IL, et al. Mesothelial cells promote early ovarian cancer metastasis through fibronectin secretion. J Clin Invest (2014) 124(10):4614–28. doi: 10.1172/jci74778
20. Kajiyama H, Shibata K, Ino K, Nawa A, Mizutani S, Kikkawa F. Possible involvement of sdf-1alpha/Cxcr4-Dppiv axis in tgf-Beta1-Induced enhancement of migratory potential in human peritoneal mesothelial cells. Cell Tissue Res (2007) 330(2):221–9. doi: 10.1007/s00441-007-0455-x
21. Nakamura M, Ono YJ, Kanemura M, Tanaka T, Hayashi M, Terai Y, et al. Hepatocyte growth factor secreted by ovarian cancer cells stimulates peritoneal implantation Via the mesothelial-mesenchymal transition of the peritoneum. Gynecol Oncol (2015) 139(2):345–54. doi: 10.1016/j.ygyno.2015.08.010
22. Mikuła-Pietrasik J, Uruski P, Pakuła M, Maksin K, Szubert S, Woźniak A, et al. Oxidative stress contributes to hepatocyte growth factor-dependent pro-senescence activity of ovarian cancer cells. Free Radic Biol Med (2017) 110:270–9. doi: 10.1016/j.freeradbiomed.2017.06.015
23. Ksiazek K, Mikula-Pietrasik J, Korybalska K, Dworacki G, Jörres A, Witowski J. Senescent peritoneal mesothelial cells promote ovarian cancer cell adhesion: The role of oxidative stress-induced fibronectin. Am J Pathol (2009) 174(4):1230–40. doi: 10.2353/ajpath.2009.080613
24. Pakuła M, Witucka A, Uruski P, Radziemski A, Moszyński R, Szpurek D, et al. Senescence-related deterioration of intercellular junctions in the peritoneal mesothelium promotes the transmesothelial invasion of ovarian cancer cells. Sci Rep (2019) 9(1):7587. doi: 10.1038/s41598-019-44123-4
25. Mikuła-Pietrasik J, Sosińska P, Naumowicz E, Maksin K, Piotrowska H, Woźniak A, et al. Senescent peritoneal mesothelium induces a pro-angiogenic phenotype in ovarian cancer cells in vitro and in a mouse xenograft model in vivo. Clin Exp Metastasis (2016) 33(1):15–27. doi: 10.1007/s10585-015-9753-y
26. Haria D, Trinh BQ, Ko SY, Barengo N, Liu J, Naora H. The homeoprotein Dlx4 stimulates nf-Kb activation and Cd44-mediated tumor-mesothelial cell interactions in ovarian cancer. Am J Pathol (2015) 185(8):2298–308. doi: 10.1016/j.ajpath.2015.04.004
27. Peng Y, Kajiyama H, Yuan H, Nakamura K, Yoshihara M, Yokoi A, et al. Pai-1 secreted from metastatic ovarian cancer cells triggers the tumor-promoting role of the mesothelium in a feedback loop to accelerate peritoneal dissemination. Cancer Lett (2019) 442:181–92. doi: 10.1016/j.canlet.2018.10.027
28. Vincent T, Molina L, Espert L, Mechti N. Hyaluronan, a major non-protein glycosaminoglycan component of the extracellular matrix in human bone marrow, mediates dexamethasone resistance in multiple myeloma. Br J Haematol (2003) 121(2):259–69. doi: 10.1046/j.1365-2141.2003.04282.x
29. Misra S, Ghatak S, Toole BP. Regulation of Mdr1 expression and drug resistance by a positive feedback loop involving hyaluronan, phosphoinositide 3-kinase, and Erbb2. J Biol Chem (2005) 280(21):20310–5. doi: 10.1074/jbc.M500737200
30. Bourguignon LY, Peyrollier K, Xia W, Gilad E. Hyaluronan-Cd44 interaction activates stem cell marker nanog, stat-3-Mediated Mdr1 gene expression, and ankyrin-regulated multidrug efflux in breast and ovarian tumor cells. J Biol Chem (2008) 283(25):17635–51. doi: 10.1074/jbc.M800109200
31. Ricciardelli C, Ween MP, Lokman NA, Tan IA, Pyragius CE, Oehler MK. Chemotherapy-induced hyaluronan production: A novel chemoresistance mechanism in ovarian cancer. BMC Cancer (2013) 13:476. doi: 10.1186/1471-2407-13-476
32. Qian J, LeSavage BL, Hubka KM, Ma C, Natarajan S, Eggold JT, et al. Cancer-associated mesothelial cells promote ovarian cancer chemoresistance through paracrine osteopontin signaling. J Clin Invest (2021) 131(16):e146186. doi: 10.1172/jci146186
33. Yoshihara M, Kajiyama H, Yokoi A, Sugiyama M, Koya Y, Yamakita Y, et al. Ovarian cancer-associated mesothelial cells induce acquired platinum-resistance in peritoneal metastasis Via the Fn1/Akt signaling pathway. Int J Cancer (2020) 146(8):2268–80. doi: 10.1002/ijc.32854
34. Rieppi M, Vergani V, Gatto C, Zanetta G, Allavena P, Taraboletti G, et al. Mesothelial cells induce the motility of human ovarian carcinoma cells. Int J Cancer (1999) 80(2):303–7. doi: 10.1002/(sici)1097-0215(19990118)80:2<303::aid-ijc21>3.0.co;2-w
35. Shishido A, Mori S, Yokoyama Y, Hamada Y, Minami K, Qian Y, et al. Mesothelial cells facilitate cancer Stem−Like properties in spheroids of ovarian cancer cells. Oncol Rep (2018) 40(4):2105–14. doi: 10.3892/or.2018.6605
36. Olbrecht S, Busschaert P, Qian J, Vanderstichele A, Loverix L, Van Gorp T, et al. High-grade serous tubo-ovarian cancer refined with single-cell rna sequencing: Specific cell subtypes influence survival and determine molecular subtype classification. Genome Med (2021) 13(1):111. doi: 10.1186/s13073-021-00922-x
37. Scalici JM, Arapovic S, Saks EJ, Atkins KA, Petroni G, Duska LR, et al. Mesothelium expression of vascular cell adhesion molecule-1 (Vcam-1) is associated with an unfavorable prognosis in epithelial ovarian cancer (Eoc). Cancer (2017) 123(6):977–84. doi: 10.1002/cncr.30415
38. Lee JK, Park SR, Jung BK, Jeon YK, Lee YS, Kim MK, et al. Exosomes derived from mesenchymal stem cells suppress angiogenesis by down-regulating vegf expression in breast cancer cells. PloS One (2013) 8(12):e84256. doi: 10.1371/journal.pone.0084256
39. Nakamura K, Sawada K, Kinose Y, Yoshimura A, Toda A, Nakatsuka E, et al. Exosomes promote ovarian cancer cell invasion through transfer of Cd44 to peritoneal mesothelial cells. Mol Cancer Res (2017) 15(1):78–92. doi: 10.1158/1541-7786.Mcr-16-0191
40. Gao L, Nie X, Gou R, Hu Y, Dong H, Li X, et al. Exosomal Anxa2 derived from ovarian cancer cells regulates epithelial-mesenchymal plasticity of human peritoneal mesothelial cells. J Cell Mol Med (2021) 25(23):10916–29. doi: 10.1111/jcmm.16983
41. Carollo E, Paris B, Samuel P, Pantazi P, Bartelli TF, Dias-Neto E, et al. Detecting ovarian cancer using extracellular vesicles: Progress and possibilities. Biochem Soc Trans (2019) 47(1):295–304. doi: 10.1042/bst20180286
42. Carroll MJ, Fogg KC, Patel HA, Krause HB, Mancha AS, Patankar MS, et al. Alternatively-activated macrophages upregulate mesothelial expression of p-selectin to enhance adhesion of ovarian cancer cells. Cancer Res (2018) 78(13):3560–73. doi: 10.1158/0008-5472.Can-17-3341
43. Huang H, Wang Z, Zhang Y, Pradhan RN, Ganguly D, Chandra R, et al. Mesothelial cell-derived antigen-presenting cancer-associated fibroblasts induce expansion of regulatory T cells in pancreatic cancer. Cancer Cell (2022) 40(6):656–73.e7. doi: 10.1016/j.ccell.2022.04.011
44. Lengyel E. Ovarian cancer development and metastasis. Am J Pathol (2010) 177(3):1053–64. doi: 10.2353/ajpath.2010.100105
45. Nieman KM, Kenny HA, Penicka CV, Ladanyi A, Buell-Gutbrod R, Zillhardt MR, et al. Adipocytes promote ovarian cancer metastasis and provide energy for rapid tumor growth. Nat Med (2011) 17(11):1498–503. doi: 10.1038/nm.2492
46. Liu Y, Metzinger MN, Lewellen KA, Cripps SN, Carey KD, Harper EI, et al. Obesity contributes to ovarian cancer metastatic success through increased lipogenesis, enhanced vascularity, and decreased infiltration of M1 macrophages. Cancer Res (2015) 75(23):5046–57. doi: 10.1158/0008-5472.Can-15-0706
47. Noujaim AA, Schultes BC, Baum RP, Madiyalakan R. Induction of Ca125-specific b and T cell responses in patients injected with Mab-B43.13–evidence for antibody-mediated antigen-processing and presentation of Ca125 in vivo. Cancer Biother Radiopharm (2001) 16(3):187–203. doi: 10.1089/10849780152389384
48. Berek J, Taylor P, McGuire W, Smith LM, Schultes B, Nicodemus CF. Oregovomab maintenance monoimmunotherapy does not improve outcomes in advanced ovarian cancer. J Clin Oncol (2009) 27(3):418–25. doi: 10.1200/jco.2008.17.8400
49. Battaglia A, Buzzonetti A, Fossati M, Scambia G, Fattorossi A, Madiyalakan MR, et al. Translational immune correlates of indirect antibody immunization in a randomized phase ii study using scheduled combination therapy with Carboplatin/Paclitaxel plus oregovomab in ovarian cancer patients. Cancer Immunol Immunother (2020) 69(3):383–97. doi: 10.1007/s00262-019-02456-z
50. Chen Y, Clark S, Wong T, Chen Y, Chen Y, Dennis MS, et al. Armed antibodies targeting the mucin repeats of the ovarian cancer antigen, Muc16, are highly efficacious in animal tumor models. Cancer Res (2007) 67(10):4924–32. doi: 10.1158/0008-5472.Can-06-4512
51. Liu JF, Moore KN, Birrer MJ, Berlin S, Matulonis UA, Infante JR, et al. Phase I study of safety and pharmacokinetics of the anti-Muc16 antibody-drug conjugate Dmuc5754a in patients with platinum-resistant ovarian cancer or unresectable pancreatic cancer. Ann Oncol (2016) 27(11):2124–30. doi: 10.1093/annonc/mdw401
52. Chekmasova AA, Rao TD, Nikhamin Y, Park KJ, Levine DA, Spriggs DR, et al. Successful eradication of established peritoneal ovarian tumors in scid-beige mice following adoptive transfer of T cells genetically targeted to the Muc16 antigen. Clin Cancer Res (2010) 16(14):3594–606. doi: 10.1158/1078-0432.Ccr-10-0192
53. Crawford A, Haber L, Kelly MP, Vazzana K, Canova L, Ram P, et al. A mucin 16 bispecific T cell-engaging antibody for the treatment of ovarian cancer. Sci Transl Med (2019) 11(497):eaau7534. doi: 10.1126/scitranslmed.aau7534
54. Yeku OO, Rao TD, Laster I, Kononenko A, Purdon TJ, Wang P, et al. Bispecific T-cell engaging antibodies against Muc16 demonstrate efficacy against ovarian cancer in monotherapy and in combination with pd-1 and vegf inhibition. Front Immunol (2021) 12:663379. doi: 10.3389/fimmu.2021.663379
55. Wang Q, Ma X, Wu H, Zhao C, Chen J, Li R, et al. Oncolytic adenovirus with Muc16-bite shows enhanced antitumor immune response by reversing the tumor microenvironment in pdx model of ovarian cancer. Oncoimmunology (2022) 11(1):2096362. doi: 10.1080/2162402x.2022.2096362
56. Hassan R, Cohen SJ, Phillips M, Pastan I, Sharon E, Kelly RJ, et al. Phase I clinical trial of the chimeric anti-mesothelin monoclonal antibody morab-009 in patients with mesothelin-expressing cancers. Clin Cancer Res (2010) 16(24):6132–8. doi: 10.1158/1078-0432.Ccr-10-2275
57. Golfier S, Kopitz C, Kahnert A, Heisler I, Schatz CA, Stelte-Ludwig B, et al. Anetumab ravtansine: A novel mesothelin-targeting antibody-drug conjugate cures tumors with heterogeneous target expression favored by bystander effect. Mol Cancer Ther (2014) 13(6):1537–48. doi: 10.1158/1535-7163.Mct-13-0926
58. Hassan R, Blumenschein GR Jr., Moore KN, Santin AD, Kindler HL, Nemunaitis JJ, et al. First-in-Human, multicenter, phase I dose-escalation and expansion study of anti-mesothelin antibody-drug conjugate anetumab ravtansine in advanced or metastatic solid tumors. J Clin Oncol (2020) 38(16):1824–35. doi: 10.1200/jco.19.02085
59. Schoutrop E, El-Serafi I, Poiret T, Zhao Y, Gultekin O, He R, et al. Mesothelin-specific car T cells target ovarian cancer. Cancer Res (2021) 81(11):3022–35. doi: 10.1158/0008-5472.Can-20-2701
60. Schlaepfer DD, Jones KC, Hunter T. Multiple Grb2-mediated integrin-stimulated signaling pathways to Erk2/Mitogen-activated protein kinase: Summation of both c-src- and focal adhesion kinase-initiated tyrosine phosphorylation events. Mol Cell Biol (1998) 18(5):2571–85. doi: 10.1128/mcb.18.5.2571
61. Bell-McGuinn KM, Matthews CM, Ho SN, Barve M, Gilbert L, Penson RT, et al. A phase ii, single-arm study of the anti-A5β1 integrin antibody volociximab as monotherapy in patients with platinum-resistant advanced epithelial ovarian or primary peritoneal cancer. Gynecol Oncol (2011) 121(2):273–9. doi: 10.1016/j.ygyno.2010.12.362
62. Mikuła-Pietrasik J, Sosińska P, Książek K. Resveratrol inhibits ovarian cancer cell adhesion to peritoneal mesothelium in vitro by modulating the production of A5β1 integrins and hyaluronic acid. Gynecol Oncol (2014) 134(3):624–30. doi: 10.1016/j.ygyno.2014.06.022
63. Condello S, Sima L, Ivan C, Cardenas H, Schiltz G, Mishra RK, et al. Tissue tranglutaminase regulates interactions between ovarian cancer stem cells and the tumor niche. Cancer Res (2018) 78(11):2990–3001. doi: 10.1158/0008-5472.Can-17-2319
64. Khanna M, Chelladurai B, Gavini A, Li L, Shao M, Courtney D, et al. Targeting ovarian tumor cell adhesion mediated by tissue transglutaminase. Mol Cancer Ther (2011) 10(4):626–36. doi: 10.1158/1535-7163.Mct-10-0912
65. Auzenne E, Ghosh SC, Khodadadian M, Rivera B, Farquhar D, Price RE, et al. Hyaluronic acid-paclitaxel: Antitumor efficacy against Cd44(+) human ovarian carcinoma xenografts. Neoplasia (2007) 9(6):479–86. doi: 10.1593/neo.07229
66. Yang X, Iyer AK, Singh A, Choy E, Hornicek FJ, Amiji MM, et al. Mdr1 sirna loaded hyaluronic acid-based Cd44 targeted nanoparticle systems circumvent paclitaxel resistance in ovarian cancer. Sci Rep (2015) 5:8509. doi: 10.1038/srep08509
67. Byeon Y, Lee JW, Choi WS, Won JE, Kim GH, Kim MG, et al. Cd44-targeting plga nanoparticles incorporating paclitaxel and fak sirna overcome chemoresistance in epithelial ovarian cancer. Cancer Res (2018) 78(21):6247–56. doi: 10.1158/0008-5472.Can-17-3871
68. Chen J, Ouyang J, Chen Q, Deng C, Meng F, Zhang J, et al. Egfr and Cd44 dual-targeted multifunctional hyaluronic acid nanogels boost protein delivery to ovarian and breast cancers in vitro and in vivo. ACS Appl Mater Interfaces (2017) 9(28):24140–7. doi: 10.1021/acsami.7b06879
69. Choi KY, Jeon EJ, Yoon HY, Lee BS, Na JH, Min KH, et al. Theranostic nanoparticles based on pegylated hyaluronic acid for the diagnosis, therapy and monitoring of colon cancer. Biomaterials (2012) 33(26):6186–93. doi: 10.1016/j.biomaterials.2012.05.029
70. Deying W, Feng G, Shumei L, Hui Z, Ming L, Hongqing W. Caf-derived hgf promotes cell proliferation and drug resistance by up-regulating the c-Met/Pi3k/Akt and Grp78 signalling in ovarian cancer cells. Biosci Rep (2017) 37(2):BSR20160470. doi: 10.1042/bsr20160470
71. Martin LP, Sill M, Shahin MS, Powell M, DiSilvestro P, Landrum LM, et al. A phase ii evaluation of amg 102 (Rilotumumab) in the treatment of persistent or recurrent epithelial ovarian, fallopian tube or primary peritoneal carcinoma: A gynecologic oncology group study. Gynecol Oncol (2014) 132(3):526–30. doi: 10.1016/j.ygyno.2013.12.018
72. Maalmi H, Ordóñez-Mena JM, Schöttker B, Brenner H. Serum 25-hydroxyvitamin d levels and survival in colorectal and breast cancer patients: Systematic review and meta-analysis of prospective cohort studies. Eur J Cancer (2014) 50(8):1510–21. doi: 10.1016/j.ejca.2014.02.006
73. Wang B, Jing Z, Li C, Xu S, Wang Y. Blood 25-hydroxyvitamin d levels and overall mortality in patients with colorectal cancer: A dose-response meta-analysis. Eur J Cancer (2014) 50(12):2173–5. doi: 10.1016/j.ejca.2014.05.004
74. Keum N, Lee DH, Greenwood DC, Manson JE, Giovannucci E. Vitamin d supplementation and total cancer incidence and mortality: A meta-analysis of randomized controlled trials. Ann Oncol (2019) 30(5):733–43. doi: 10.1093/annonc/mdz059
75. Kitami K, Yoshihara M, Tamauchi S, Sugiyama M, Koya Y, Yamakita Y, et al. Peritoneal restoration by repurposing vitamin d inhibits ovarian cancer dissemination Via blockade of the tgf-B1/Thrombospondin-1 axis. Matrix Biol (2022) 109:70–90. doi: 10.1016/j.matbio.2022.03.003
76. Puré E. Seeking synergy of checkpoint blockade through tgfβ inhibition. Cancer Immunol Res (2018) 6(12):1444. doi: 10.1158/2326-6066.Cir-18-0784
77. Zhao F, Evans K, Xiao C, DeVito N, Theivanthiran B, Holtzhausen A, et al. Stromal fibroblasts mediate anti-Pd-1 resistance Via mmp-9 and dictate tgfβ inhibitor sequencing in melanoma. Cancer Immunol Res (2018) 6(12):1459–71. doi: 10.1158/2326-6066.Cir-18-0086
78. Kenny HA, Hart PC, Kordylewicz K, Lal M, Shen M, Kara B, et al. The natural product B-escin targets cancer and stromal cells of the tumor microenvironment to inhibit ovarian cancer metastasis. Cancers (Basel) (2021) 13(16):3931. doi: 10.3390/cancers13163931
79. Brown JR, Chan DK, Shank JJ, Griffith KA, Fan H, Szulawski R, et al. Phase ii clinical trial of metformin as a cancer stem cell-targeting agent in ovarian cancer. JCI Insight (2020) 5(11):e133247. doi: 10.1172/jci.insight.133247
80. Li L, Wang L, Li J, Fan Z, Yang L, Zhang Z, et al. Metformin-induced reduction of Cd39 and Cd73 blocks myeloid-derived suppressor cell activity in patients with ovarian cancer. Cancer Res (2018) 78(7):1779–91. doi: 10.1158/0008-5472.Can-17-2460
81. Menegazzo L, Scattolini V, Cappellari R, Bonora BM, Albiero M, Bortolozzi M, et al. The antidiabetic drug metformin blunts netosis in vitro and reduces circulating netosis biomarkers in vivo. Acta Diabetol (2018) 55(6):593–601. doi: 10.1007/s00592-018-1129-8
82. Chiang CF, Chao TT, Su YF, Hsu CC, Chien CY, Chiu KC, et al. Metformin-treated cancer cells modulate macrophage polarization through ampk-Nf-Kb signaling. Oncotarget (2017) 8(13):20706–18. doi: 10.18632/oncotarget.14982
83. Eikawa S, Nishida M, Mizukami S, Yamazaki C, Nakayama E, Udono H. Immune-mediated antitumor effect by type 2 diabetes drug, metformin. Proc Natl Acad Sci U.S.A. (2015) 112(6):1809–14. doi: 10.1073/pnas.1417636112
84. Hart PC, Kenny HA, Grassl N, Watters KM, Litchfield LM, Coscia F, et al. Mesothelial cell Hif1α expression is metabolically downregulated by metformin to prevent oncogenic tumor-stromal crosstalk. Cell Rep (2019) 29(12):4086–98.e6. doi: 10.1016/j.celrep.2019.11.079
85. Zhang G, Li Z, Dong J, Zhou W, Zhang Z, Que Z, et al. Acacetin inhibits invasion, migration and tgf-B1-Induced emt of gastric cancer cells through the Pi3k/Akt/Snail pathway. BMC Complement Med Ther (2022) 22(1):10. doi: 10.1186/s12906-021-03494-w
86. Tian M, Tang Y, Huang T, Liu Y, Pan Y. Amelioration of human peritoneal mesothelial cell Co-Culture-Evoked malignant potential of ovarian cancer cells by acacetin involves lpa release-activated rage-Pi3k/Akt signaling. Cell Mol Biol Lett (2021) 26(1):51. doi: 10.1186/s11658-021-00296-3
87. Ksiazek K, Mikula-Pietrasik J, Olijslagers S, Jörres A, von Zglinicki T, Witowski J. Vulnerability to oxidative stress and different patterns of senescence in human peritoneal mesothelial cell strains. Am J Physiol Regul Integr Comp Physiol (2009) 296(2):R374–82. doi: 10.1152/ajpregu.90451.2008
88. McFarland GA, Holliday R. Retardation of the senescence of cultured human diploid fibroblasts by carnosine. Exp Cell Res (1994) 212(2):167–75. doi: 10.1006/excr.1994.1132
89. Zhang Z, Miao L, Wu X, Liu G, Peng Y, Xin X, et al. Carnosine inhibits the proliferation of human gastric carcinoma cells by retarding Akt/Mtor/P70s6k signaling. J Cancer (2014) 5(5):382–9. doi: 10.7150/jca.8024
90. Rancourt C, Bergeron C, Lane D, Garon G, Piché A. Delivery of herpes simplex thymidine kinase bystander effect by engineered human mesothelial cells for the treatment of ovarian cancer. Cytotherapy (2003) 5(6):509–22. doi: 10.1080/14653240310003620
91. Loureiro J, Sandoval P, del Peso G, Gónzalez-Mateo G, Fernández-Millara V, Santamaria B, et al. Tamoxifen ameliorates peritoneal membrane damage by blocking mesothelial to mesenchymal transition in peritoneal dialysis. PloS One (2013) 8(4):e61165. doi: 10.1371/journal.pone.0061165
92. Yan P, Tang H, Chen X, Ji S, Jin W, Zhang J, et al. Tamoxifen attenuates dialysate-induced peritoneal fibrosis by inhibiting gsk-3β/B-Catenin axis activation. Biosci Rep (2018) 38(6):BSR20180240. doi: 10.1042/bsr20180240
93. Wilson RB, Archid R, Reymond MA. Reprogramming of mesothelial-mesenchymal transition in chronic peritoneal diseases by estrogen receptor modulation and tgf-B1 inhibition. Int J Mol Sci (2020) 21(11):4158. doi: 10.3390/ijms21114158
94. Grunewald T, Ledermann JA. Targeted therapies for ovarian cancer. Best Pract Res Clin Obstet Gynaecol (2017) 41:139–52. doi: 10.1016/j.bpobgyn.2016.12.001
Keywords: ovarian cancer, cancer-associated mesothelial cells, tumor progression, chemoresistance, tumor therapy
Citation: Zheng A, Wei Y, Zhao Y, Zhang T and Ma X (2022) The role of cancer-associated mesothelial cells in the progression and therapy of ovarian cancer. Front. Immunol. 13:1013506. doi: 10.3389/fimmu.2022.1013506
Received: 07 August 2022; Accepted: 15 September 2022;
Published: 04 October 2022.
Edited by:
Qi Zhao, University of Macau, ChinaReviewed by:
Masato Yoshihara, Nagoya University, JapanCopyright © 2022 Zheng, Wei, Zhao, Zhang and Ma. This is an open-access article distributed under the terms of the Creative Commons Attribution License (CC BY). The use, distribution or reproduction in other forums is permitted, provided the original author(s) and the copyright owner(s) are credited and that the original publication in this journal is cited, in accordance with accepted academic practice. No use, distribution or reproduction is permitted which does not comply with these terms.
*Correspondence: Xuelei Ma, ZHJtYXh1ZWxlaUBnbWFpbC5jb20=