- 1Department of Molecular and Medical Pharmacology, University of California, Los Angeles, CA, United States
- 2High Containment Program, University of California, Los Angeles, CA, United States
- 3Department of Molecular Microbiology and Immunology, Keck School of Medicine of the University of Southern California, Los Angeles, CA, United States
Gamma-aminobutyric acid (GABA) and GABA-receptors (GABA-Rs) form a major neurotransmitter system in the brain. GABA-Rs are also expressed by 1) cells of the innate and adaptive immune system and act to inhibit their inflammatory activities, and 2) lung epithelial cells and GABA-R agonists/potentiators have been observed to limit acute lung injuries. These biological properties suggest that GABA-R agonists may have potential for treating COVID-19. We previously reported that GABA-R agonist treatments protected mice from severe disease induced by infection with a lethal mouse coronavirus (MHV-1). Because MHV-1 targets different cellular receptors and is biologically distinct from SARS-CoV-2, we sought to test GABA therapy in K18-hACE2 mice which develop severe pneumonitis with high lethality following SARS-CoV-2 infection. We observed that GABA treatment initiated immediately after SARS-CoV-2 infection, or 2 days later near the peak of lung viral load, reduced pneumonitis severity and death rates in K18-hACE2 mice. GABA-treated mice had reduced lung viral loads and displayed shifts in their serum cytokine/chemokine levels that are associated with better outcomes in COVID-19 patients. Thus, GABA-R activation had multiple effects that are also desirable for the treatment of COVID-19. The protective effects of GABA against two very different beta coronaviruses (SARS-CoV-2 and MHV-1) suggest that it may provide a generalizable off-the-shelf therapy to help treat diseases induced by new SARS-CoV-2 variants and novel coronaviruses that evade immune responses and antiviral medications. GABA is inexpensive, safe for human use, and stable at room temperature, making it an attractive candidate for testing in clinical trials. We also discuss the potential of GABA-R agonists for limiting COVID-19-associated neuroinflammation.
Introduction
Despite the great success of vaccines to reduce serious illness due to COVID-19, this approach has limitations due to break-through infections, vaccine hesitancy, viral variants, and novel coronaviruses. Antiviral drugs can greatly help reduce the risk for severe disease and mortality due to COVID-19, however, these drugs may not become readily available in developing countries and they may be less effective against coronaviruses that emerge in the future. The identification of additional therapeutics that have established safety records, are inexpensive, and do not have special storage requirements could be especially helpful for reducing COVID-19-associated morbidity and mortality worldwide.
Gamma-aminobutyric acid type A receptors (GABAA-Rs) are a family of ligand-gated chloride channels which play key roles in neurodevelopment and neurotransmission in the central nervous system (CNS) (1–3). GABAA-Rs are also expressed by cells of the human and murine immune systems [e.g (4–9)]. The activation of GABAA-Rs on innate immune system cells such as macrophages, dendritic, and natural killer (NK) cells reduces their inflammatory activities and shifts them toward anti-inflammatory phenotypes (7, 8, 10–17). Moreover, GABAA-R agonists inhibit the inflammatory activities of human and rodent Th17 and Th1 CD4+ T cells and cytotoxic CD8+ T cells (7, 18–22) while also promoting CD4+ and CD8+ Treg responses (18, 20, 23, 24). These properties have enabled treatments with GABA-R agonists to inhibit the progression of a diverse array of autoimmune diseases that occur in mice with different genetic backgrounds, including models of type 1 diabetes (T1D), multiple sclerosis (MS), rheumatoid arthritis, Sjogren’s syndrome, as well as inflammation in type 2 diabetes (11, 12, 15, 19, 20, 23–26). Relevant to the treatment of COVID-19, GABA inhibits human immune cell secretion of many pro-inflammatory cytokines and chemokines associated with cytokine storms in individuals with COVID-19 (5, 7, 27–31).
Airway epithelial cells, including type II alveolar epithelial cells, also express GABAA-Rs which modulate the intracellular ionic milieu and help maintain alveolar fluid homeostasis (32–35). GABA and GABAA-R positive allosteric modulators (PAMs) reduce inflammation and improve alveolar fluid clearance and lung functional recovery in different rodent models of acute lung injury (36–43), and limit pulmonary inflammatory responses and improve clinical outcomes in ventilated human patients (44–46). GABA decreases the secretion of inflammatory factors by human bronchial epithelial cells in vitro (37) and GABAA-R PAMs reduce macrophage infiltration and inflammatory cytokine levels in bronchoalveolar lavage fluid and reduce rodent and human macrophage inflammatory responses (10, 13, 33, 42, 47–49). Moreover, GABAA-R ligands inhibit human neutrophil activation (50) and platelet aggregation (51), which are potentially important because pulmonary thrombosis often occurs in critically ill COVID-19 patients. Finally, lower levels of plasma GABA are detected in hospitalized COVID-19 patients and associated with the pathogenesis of COVID-19 (52, 53), although the basis for this observation is not understood. Together, the multiple actions of GABAA-R modulators on various immune cells and lung epithelial cells, along with their safety for clinical use, make them candidates for limiting dysregulated immune responses, severe pneumonia, and lung damage due to coronavirus infection.
In a previous study, we began to assess whether GABA-R agonists had therapeutic potential for treating coronavirus infections by studying A/J mice that were inoculated with mouse hepatitis virus (MHV-1) (54). MHV-1 is a pneumotropic beta-coronavirus that induces a highly lethal pneumonitis in A/J mice (55–60). We observed that oral administration of GABA or the GABAA-R-specific agonist homotaurine, but not a GABAB-R-specific agonist, effectively inhibited MHV-1-induced pneumonitis, and reduced disease severity, and death rates when given before or after the onset of symptoms (54). GABA treatment also modestly reduced MHV-1 viral load in their lungs.
While MHV-1 and SARS-CoV-2 are both beta coronaviruses, they bind different cellular receptors (CEACAM1 vs. ACE2, respectively), differ antigenically, and are quite biologically distinct. Moreover, the host’s response to respiratory infections varies greatly between different virus strains and mouse strains (60). To more stringently test the therapeutic potential of GABA as a therapy for COVID-19, we assessed the ability of GABA treatment to limit the disease process in SARS-CoV-2-infected human ACE2 transgenic K18-hACE2 mice, which provide a widely used acute and lethal model of COVID-19 (61–66). We found that GABA-Rs are a new druggable target for limiting disease severity, lung viral load, pneumonitis, and death in SARS-CoV-2 infected mice. We also discuss the potential of GABAA-R agonists for limiting COVID-19-associated neuroinflammation.
Results
GABA treatment protects SARS-CoV-2-infected K18-hACE2 transgenic mice from severe illness and death. K18-ACE2 mice were inoculated with SARS-CoV-2 intranasally and placed in cages with water bottles containing 0, 0.2, or 2.0 mg/mL GABA, as described in Methods. The mice were maintained on these treatments for the entire study. Their body weights, behavior, and activity were monitored and scored for the onset and severity of the disease twice daily. There was no statistically significant difference in longitudinal body weights or the amounts of water consumed among the different groups of mice (Supplementary Figure 1). The SARS-CoV-2 infected control mice that received plain water developed overt signs of illness beginning around day 5 post-infection, which increased in severity and often necessitated humane euthanasia prior to the end of the study (Figures 1A, B), similar to previous observations in this model (64). In contrast, the mice receiving GABA displayed significantly reduced illness scores compared to the control mice (Figure 1A). Only 20% of control mice survived until the pre-determined end of the studies 7-8 days post-infection. In contrast, 80% of the mice that received GABA at 0.2 or 2 mg/mL were surviving on days 7-8 post-infection (Figure 1B, p=0.004 and p=0.018, respectively vs. the control).
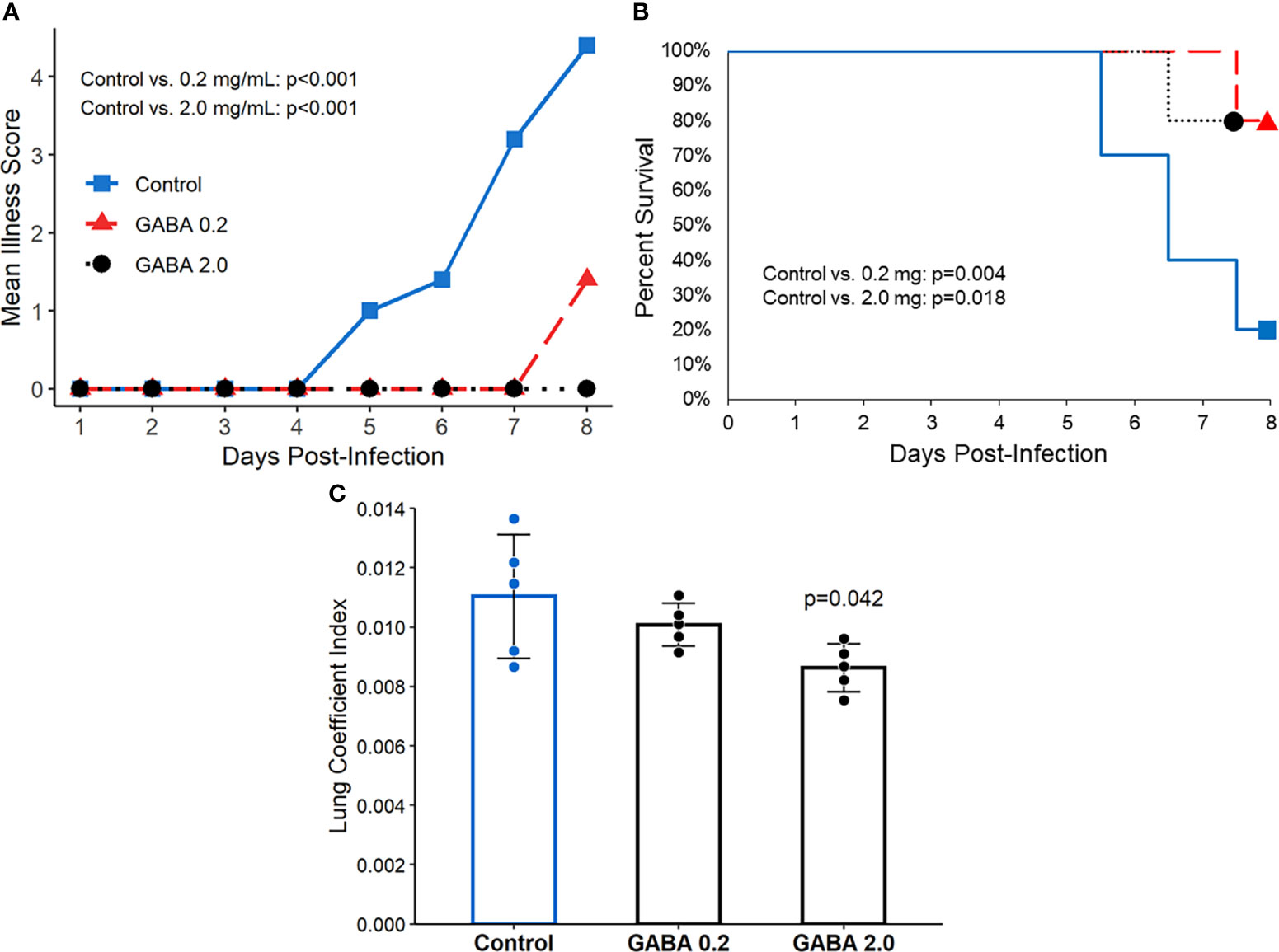
Figure 1 GABA treatment limits disease severity and mortality in SARS-CoV-2 infected K18-hACE2 mice. Following SARS-CoV-2 inoculation the mice were placed on plain water (blue line with squares), 0.2 mg/mL GABA (red line with triangles), or 2.0 mg/mL GABA (black dotted line with circles). (A) Longitudinal mean illness scores. Disease severity was scored in one of the studies and compared between groups by fitting mixed-effect linear regression models with group and time as fixed effects (to compare means), and with group, time and group by time interaction as fixed effects (to compare slopes). Mouse ID was used as a random effect. N=5 mice/group. (B) Combined percent surviving mice from two independent studies with 5 mice/group which followed the mice for 7 or 8 days post-infection (n=10 mice/group total). Survival curves were estimated using the Kaplan-Meier method and statistically analyzed by the log-rank test. (C) Mice that reached an illness score of 5 or survived to the end of the observation period were euthanized. Their lungs were dissected and weighed to calculate the lung coefficient index (the ratio of lung weight to body weight). The data shown are the mean lung coefficient index ± SEM/N=5 mice/group. The p-value was determined by Student’s t-test.
At the time of euthanasia, each animal’s lungs were excised and weighed. The ratio of lung weight to total body weight (i.e., the lung coefficient index) reflects the extent of inflammation in the lung. We observed a significant reduction in the lung coefficient index scores in the group treated with 2.0 mg/mL GABA (Figure 1C), indicating reduced lung inflammation. Together, these studies demonstrate that GABA treatment reduces disease severity, inflammation, and death rates in SARS-CoV-2 infected mice.
GABA treatment reduces SARS-CoV-2 titers in the lungs of infected K18-hACE2 mice
We next asked whether GABA’s protective mechanism could be due in part to reducing viral replication in their lungs. K18-hACE2 mice were infected with SARS-CoV-2 (2,000 TCID50 intranasally) and placed on plain water (control) or water containing GABA (2 mg/mL) for the rest of the study. Three days post-infection, which should be near to the peak of viral load in the lungs (64), we harvested their lung tissues to determine viral load by TCID50 assay using Vero E6 cells. We found that SARS-CoV-2 titers in the lungs of GABA-treated mice were on average a 1.36 log10 (23-fold) lower than that in the lungs of mice that received plain water (p<0.0001, Figure 2). Thus, GABA treatment inhibited the replication of SARS-CoV-2 in the lungs of K18-hACE2 mice.
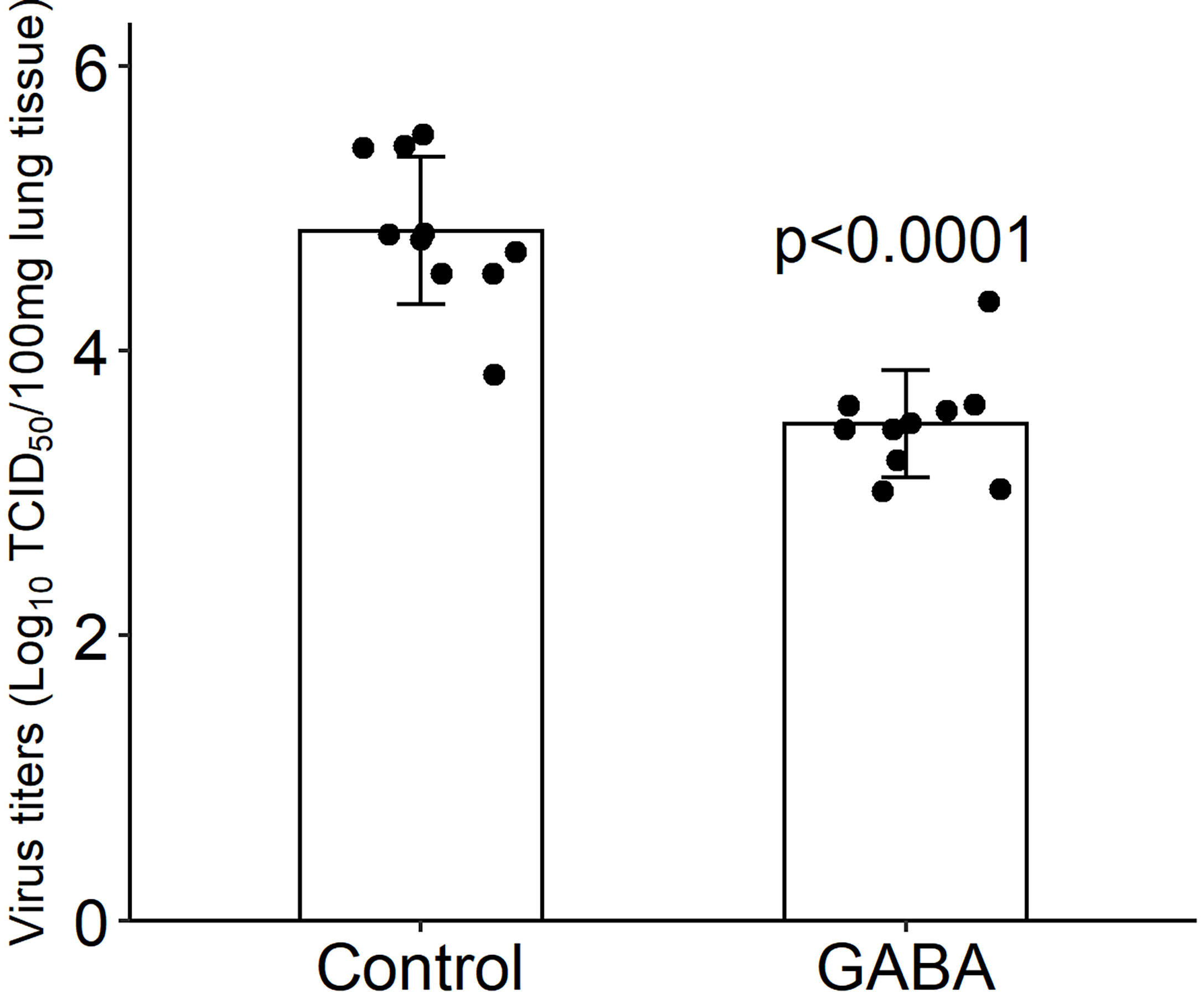
Figure 2 GABA treatment reduces SARS-CoV-2 titers in the lungs. Three days post-infection the right lung was harvested from each mouse and homogenized. The viral titer in lung tissue (100 mg) were determined by TCID50 assay using Vero E6 cells. Black dots show viral titers for individual mice (determined in quadruplicate). The data shown are the mean virus titer (Log10 TCID50/100 mg lung tissue) ± SD in mice given plain water (control) or GABA. N=10 mice/group. The p-value was determined by Student’s t-test.
GABA treatment modulates early cytokine and chemokine responses to SARS-CoV-2 infection
We next asked whether GABA treatment could modulate serum cytokine and chemokine levels in SARS-CoV-2 infected mice. Serum samples were collected three days post-infection from the same mice that were used to study lung viral load and were analyzed using a multiplex bead kit designed to detect 13 cytokines and chemokines. We did not detect any statistically significant difference in the levels of serum IFNα, IFNβ, IFNγ, IL-12, or GM-CSF between SARS-CoV-2 infected mice that did or did not receive GABA treatment (Figure 3). There was, however, some suggestion that GABA treatment elevated type I interferons in some mice because only 1/10 mice in the healthy control (HC) and in the SARS-CoV-2-infected control (SC) group had a detectable level of IFNß, while 3/10 of the infected mice which received GABA (G) had detectable IFNß levels and these were greater in magnitude than that found in the other groups (Figure 3). The median and the mean levels of IFNα were also slightly elevated in the GABA treated vs. untreated SARS-CoV-2 infected group (Figure 3). Conversely, 3/10 mice in the SC group displayed high levels of IFNγ vs 1/10 mice in the G group and the median and mean IFNγ levels were reduced in the GABA-treated vs. untreated SARS-CoV-2 infected mice (Figure 3, p=0.25)
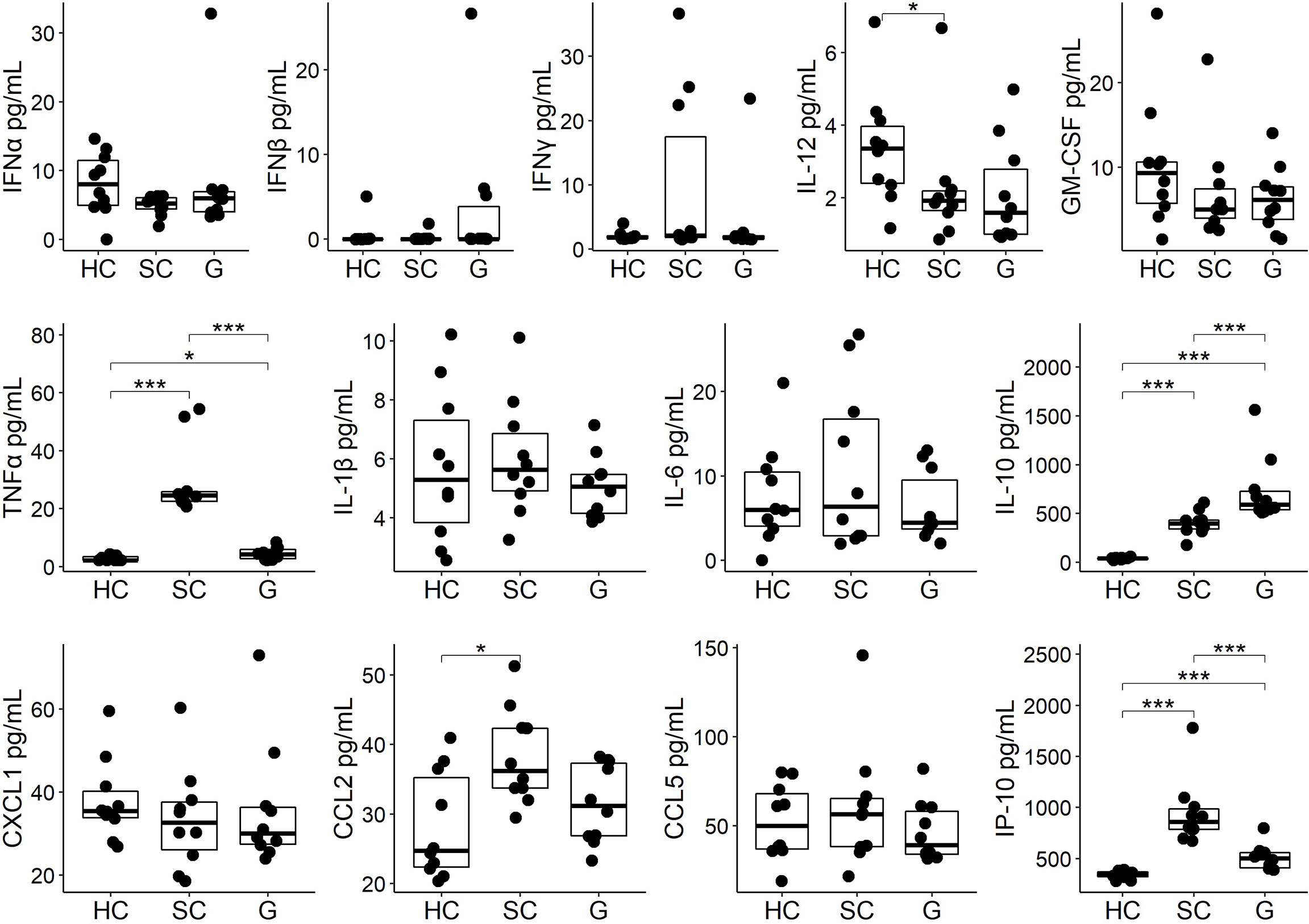
Figure 3 GABA treatment modulates circulating cytokines and chemokines in SARS-CoV-2-infected K18-hAC2 mice. Three days post-infection sera from individual SARS-CoV-2 infected control (SC) mice that were untreated, or were GABA treated (G), were collected and frozen at -80°C until analysis by a multiplex assay as described in Methods. In addition, sera from age-matched healthy control (HC) B6 mice were studied. The non-normally distributed data are shown in boxplots with the borders of the box indicating 1st and 3rd quartile of each group (n=10), the bolded line indicating the median. The data were analyzed by Wilcoxon rank-sum tests. *p<0.05, ***p<0.001.
Analysis of pro-inflammatory and anti-inflammatory cytokines revealed that SARS-CoV-2 infection significantly increased the levels of serum TNFα in the SC group (p<0.001, Figure 3) as in previous studies of SARS-CoV-2-infected K18-hACE2 mice (64, 66–68). GABA treatment significantly mitigated TNFα production following infection (p<0.001). There was no statistically significant difference in the levels of serum IL-1β and IL-6 although the levels of serum IL-1β and IL-6 in the GABA-treated group were slightly reduced compared with the SC group (Figure 3). SARS-CoV-2 infection significantly increased the levels of serum IL-10, as in past studies of K-18-hACE2 mice (64, 66, 68). GABA treatment further significantly elevated the serum IL-10 levels above that in untreated SARS-CoV-2 infected mice (p<0.001).
Analysis of serum chemokines revealed that untreated SARS-CoV-2 infected mice, but not the GABA-treated SARS-CoV-2 infected mice, displayed significantly higher levels of CCL2 relative to healthy controls (p<0.05), with the levels of CCL2 tending to be lower in GABA-treated vs. untreated-infected mice (p=0.07). Moreover, GABA treatment led to significantly reduced levels of IP-10 (CXCL10) compared to that in untreated SARS-CoV-2 infected mice (p<0.001, Figure 3). We observed no statistically significant difference in the levels of serum CXCL1 and CCL5 among these groups of mice. Thus, GABA treatment shifted systemic cytokine and chemokine responses towards those associated with less risk for developing severe COVID-19 by increasing early type I IFN responses in some mice, significantly reducing the levels of TNFα and IP-10, tending to reduce CCL2 levels, but enhancing IL-10 production in SARS-CoV-2 infected mice.
GABA treatment protects SARS-CoV-2-infected mice from severe illness and pneumonitis when treatment is initiated near the peak of viral load in the lungs
We next examined whether GABA treatment could be also beneficial when treatment was initiated at a later stage of the disease process. K18-hACE2 mice were infected with SARS-CoV-2 as above and GABA treatment (2 mg/mL) was initiated at 2 days post-infection, near the peak of viral load in the lungs. Compared to untreated control mice, GABA-treated mice displayed reduced longitudinal mean illness scores (overall p=0.002, Figure 4A). At the end of the study on day 7, only 22% of control mice survived, while 88% of GABA-treated mice had survived (p=0.004, Figure 4B). GABA-treated mice also had a reduced lung coefficient index (p<0.001, Figure 4C).
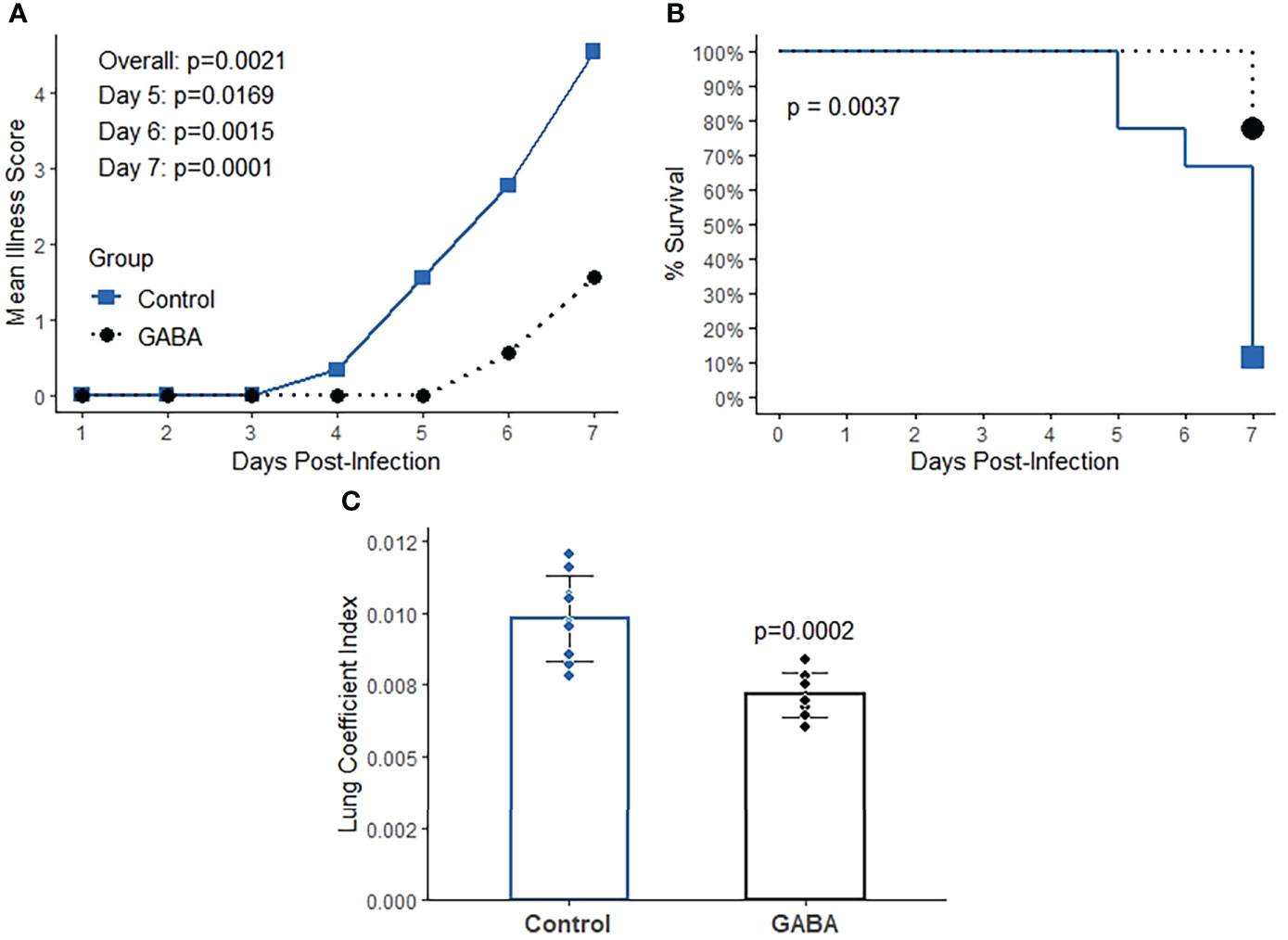
Figure 4 GABA treatment initiated near the peak of lung viral load has protective effects in SARS-CoV-2 infected K18-hACE2 mice. Mice were infected with SARS-CoV-2 and two days later mice received GABA through their drinking water (2 mg/mL), or were maintained on plain water for the remainder of the study. (A) Longitudinal mean illness scores. The data were analyzed by fitting mixed-effect linear regression models with group and time as fixed effects (to compare means), and with group, time and group by time interaction as fixed effects (to compare slopes). Mouse ID was used as a random effect. (B) Percent surviving mice. The data were analyzed by Kaplan-Meier survival curves and the log-rank test. (C) Lung coefficient index. The data were analyzed by Student t-test. N=9 mice/group.
Consistent with the reduced lung coefficient index, histological evaluation of lung sections from these mice revealed that while many inflammatory infiltrates, several hyaline-like membranes, severe diffused hemorrhage, and large areas of inflammatory consolidation were observed in the lungs of GABA-untreated mice, these pathological changes were obviously mitigated in the lungs of GABA-treated mice (Figures 5A, B). Quantitative analysis revealed that the pneumonitis scores in the mice which received GABA at 2 days post-infection were significantly less than that in the control mice (Figure 5C, p=0.027). Thus, GABA treatment limited SARS-CoV-2-induced pneumonitis and lung damages in mice.
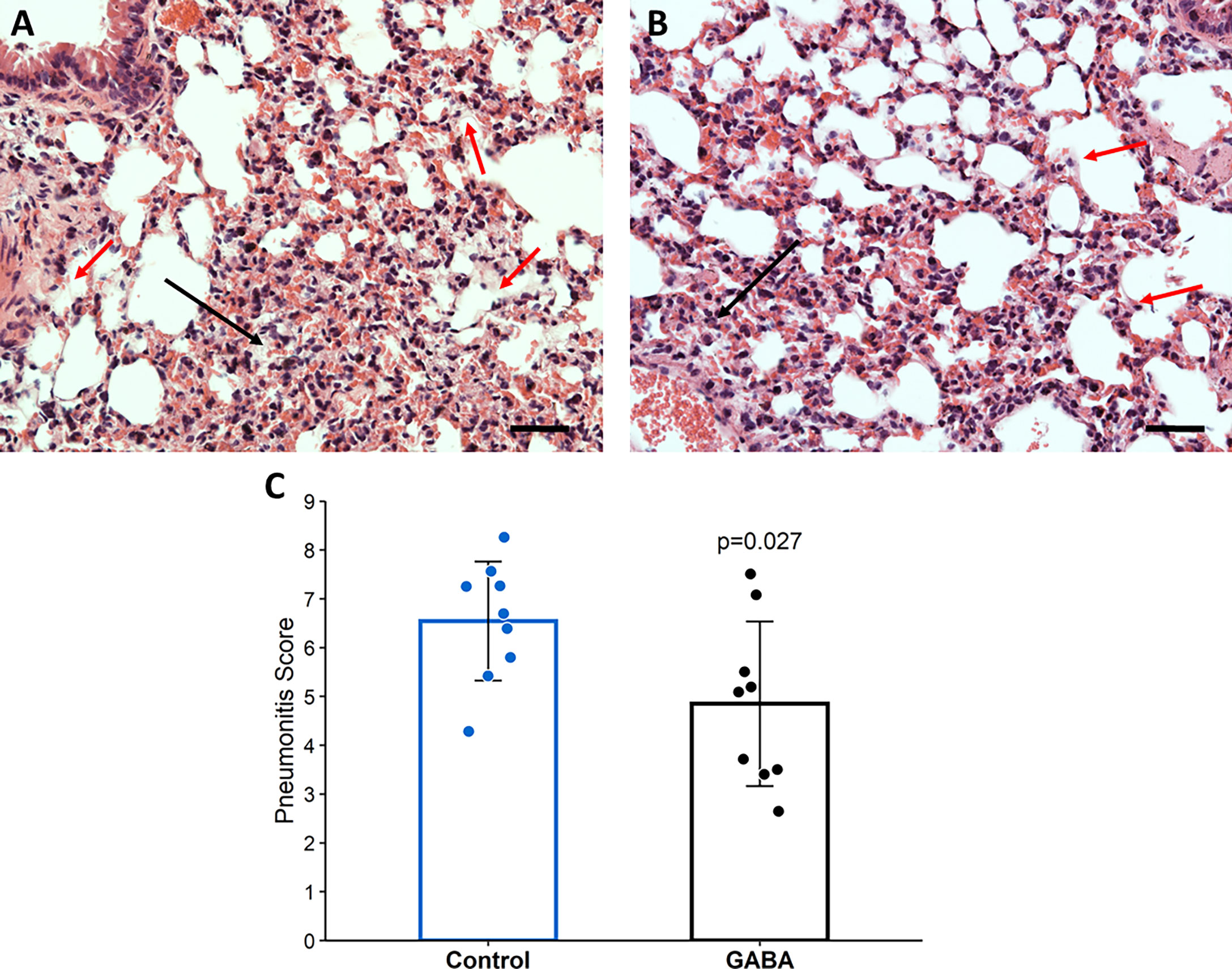
Figure 5 GABA treatment initiated 2-days post-infection reduces pneumonitis in SARS-CoV-2-infected mice. At the time of euthanasia, the lungs of mice studied in Figure 4 were removed and processed for histological examination as described in Methods. Representative images of H&E-stained lung sections (magnification × 200) from (A) untreated mice and (B) GABA-treated mice. Red arrows point to hyaline-like membranes and black arrows indicate local consolidation. The scale bar is 50 µm; (C) Quantitative analysis of the degrees of pneumonitis in the lungs. Data are expressed as the mean pneumonitis score ± SD of each group. The data were analyzed by Student t-test. N=9 mice per group.
Discussion
Our studies demonstrated that GABA administration initiated immediately, or two-day post-SARS-CoV-2 infection, reduced the lung coefficient index, lung viral load, pneumonitis, and death rate in SARS-CoV-2-infected K18-hACE2 mice. These results, along with our previous findings in the MHV-1 infected A/J mice, are the first reports of GABA administration modulating the outcome of viral infections. The ability of GABA treatment to limit the disease severity following infection with two biologically distinct and lethal coronaviruses in different mouse strains suggests that GABA-R activation may be a generalizable therapeutic strategy to help reduce the severity of coronavirus infections, at least in mice.
Our observations are surprising in a number of ways. First, based on GABA’s anti-inflammatory actions in models of autoimmune disease, cancer, and parasitic infection, it was highly possible that early GABA treatment could have exacerbated the disease in SARS-CoV-2 infected mice by limiting or delaying the innate immune responses to the viral infection. However, SARS-CoV-2-infected mice that received GABA treatment at the time of infection, or 2-days post-infection, fared much better than their untreated SARS-CoV-2-infected counterparts.
Second, GABA’s ability to modestly reduce viral load in the lungs was surprising. This indicates that GABA-R mediated changes in the intracellular ionic milieu modulate processes involved in SARS-Cov-2 entry, replication, and/or egress. We know from large-scale screens of drug libraries that GABA-R agonists do not interfere with SARS-CoV-2 binding to ACE2 or its internalization into cultured Vero E6 cells (69). Notably, GABAA-Rs are expressed by lung bronchial and alveolar cells (32, 33, 70) and it is possible that GABAA-R activation led to changes in intracellular ion levels that made the environment less favorable for viral replication. While the activation of neuronal GABAA-Rs leads to Cl- influx and hyperpolarization, the activation of GABAA-Rs on other types of cells, such as alveolar ATII cells, causes Cl- efflux and depolarization (32, 33). Many viruses, including some coronaviruses, can promote Ca2+ influx into their host cell to enhance their replication (71, 72). The activation of GABAA-Rs on infected cells could promote Cl- efflux which would oppose Ca2+ influx and reduce Ca2+ contents, limiting SARS-CoV-2 replication. Indeed, calcium blockers have been shown to reduce SARS-CoV-2 replication in vitro, but whether they confer beneficial effects to COVID-19 patients has been controversial (73–75). The activation of GABAA-Rs on alveolar and large airway epithelial cells may also have altered 1) the secretion of inflammatory signaling molecules from infected cells, 2) alveolar surfactant production/absorption, and/or 3) altered inflammatory responses and autophagy processes (17) in ways that limited virus infection and replication.
Third, while there has been some characterization of GABA’s effects on immune cell cytokine and chemokine secretion in models of autoimmune disease and cancer, little is known about GABA’s effects on anti-viral responses. We observed that GABA treatment shifted some cytokine and chemokine levels in directions that are expected to be beneficial if extended to COVID-19 treatment. Early GABA treatment elevated type 1 interferons in some mice. Since delayed or reduced type I interferon responses are a risk factor for developing severe COVID-19 (76), such tendencies could be beneficial. GABA treatment significantly reduced circulating TNFα levels in infected mice, extending previous observations that GABA inhibits the NF-κB activation in mouse and human immune cells (7, 21). As a result, GABA treatment slightly decreased mean serum IL-6 from 10.7 to 6.3 pg/mL. Since TNFα and IL-6 are important pro-inflammatory mediators, the decreased levels of circulating TNFα and IL-6 indicated that GABA treatment suppressed innate immune responses, which is likely to have contributed to its protective effects.
GABA-treated mice also had reduced levels of serum IP-10, a pro-inflammatory chemokine that attracts the migration of CXCR3+ macrophages/monocytes, T cells, and NK cells (77). Elevated levels of IP-10 are consistently detected in severely ill COVID-19 patients and may provide a predictive marker of patient outcome (78–81). IP-10 production is induced by IFNγ, NF-κB activation and other stimulators in several types of cells (82). Consistent with the reduced IP-10 levels, we also found that the serum mean IFNγ level in the GABA-treated mice was about half that in the untreated SARS-CoV-2 infected mice (4.0 vs. 9.8 pg/mL). These data suggest that early GABA treatment reduced IFNγ production and together with its inhibition of NF-κB activation, led to decreased IP-10 secretion. Given that IP-10 functions to recruit inflammatory cell infiltration into lesions and modulates cell survival, the lower levels of circulating IP-10 in GABA-treated mice are likely to have limited the migration of macrophages, monocytes, and NK cells into the pulmonary lesions and helped to protect the mice from death. Similarly, we also observed that GABA treatment slightly reduced the levels of serum CCL2 which may have contributed to protecting mice from death since high levels of CCL2 are associated with high mortality in COVID-19 patients (83).
GABA treatment also enhanced IL-10 levels in SARS-CoV-2 infected mice. IL-10 is generally regarded as an anti-inflammatory cytokine, however, it can be immunostimulatory in certain contexts and elevated levels of IL-10 are associated with the development of severe COVID-19 (84, 85). If the elevation of IL-10 levels in GABA-treated SARS-CoV-2 infected mice had counter-therapeutic effects, it is evident that GABA’s beneficial actions were functionally dominant leading to improved outcomes. Conceivably, the enhanced levels of IL-10 levels due to GABA treatment may have been therapeutic by 1) its classical anti-inflammatory actions, 2) exhausting immune cells, 3) reducing tissue damage in the lungs, or 4) other yet to be identified actions.
Initiating GABA treatment 2-days after SARS-CoV-2 infection, near the peak of viral loads in the lungs, was essentially just as effective as initiating the treatment immediately post-infection in terms of limiting disease severity and death rates during the observation period. Coinciding with those observations, the lungs of mice treated with GABA 2 days-post infection displayed reduced histopathological damage relative to untreated controls. It will be of interest to further test the efficacy of GABA when initiated at even later time points post-infection–however, the current findings clearly indicate GABA to be an excellent candidate therapeutic for COVD-19 and due to the inherent imperfections of any animal model, the ultimate test of this treatment will require human clinical trials.
Besides expressing the hACE2 transgene in lung cell epithelial cells, K18-hACE2 mice express hACE2 ectopically in their CNS leading to the spreading of SARS-CoV-2 infection to their CNS at late stages of the disease process (64, 66, 67). Because GABA does not pass through the blood-brain barrier (BBB), it is unlikely that GABA treatment directly exerted beneficial effects in the CNS. However, the decreased levels of circulating proinflammatory cytokines and chemokines in GABA-treated mice may have also reduced their entry into the CNS. While SARS-CoV-2 is thought not to efficiently replicate in the human CNS (86), some COVID-19 patients experience cognitive impairments (or “brain fog”). Histological studies of the brains from COVID-19 patients have observed immune cell infiltrates and increased frequencies of glial cells with inflammatory phenotypes which are indicative of neuroinflammatory responses (86–93). In previous studies, we have shown that treatment with homotaurine, a GABAA-R-specific agonist that can pass through the BBB, reduced the spreading of inflammatory T cell responses within the CNS, limited the pro-inflammatory activity of antigen-presenting cells, and ameliorated disease in mouse models of multiple sclerosis (15, 20). Since microglia and astrocytes express GABAA-Rs which act to down-regulate their inflammatory activities (94), homotaurine treatment may have also limited glial inflammation. Homotaurine was as effective as GABA in protecting MHV-1-infected A/J mice from severe illness, pointing to GABAA-Rs as the major mediators of GABA’s beneficial effects in this model (54). These observations suggest that homotaurine treatment may provide a new strategy to both reduce the deleterious effects of coronavirus infection in the periphery and limit inflammation in the CNS. Homotaurine (also known as Tramiprosate) was found to physically interfere with amyloid aggregation in vitro, leading to its testing as a treatment for Alzheimer’s disease in a large long-term phase III clinical study (95–97). While this treatment failed to meet primary endpoints, the treatment appeared to be very safe and follow-up studies suggested some disease-modifying effect (98, 99).
Finally, it is worth noting that circulating GABA levels are significantly reduced in hospitalized patients with COVID-19 (52, 53). This clinical finding, independent of our results presented here, raises the question of whether GABA therapy could be beneficial for COVID-19 patients.
It is likely that SARS-CoV-2 variants and novel coronaviruses will constantly arise that will be insufficiently controlled by available vaccines and antiviral medications. Developing new vaccines against these new viruses will be much slower than the spread of these new viruses among the world population. Our findings suggest that GABA-R agonists may provide inexpensive off-the-shelf agents to help lessen the severity of disease caused by these new viruses. Because GABA’s mechanisms of action are unlike that of other coronavirus treatments, combination treatments could have enhanced benefits.
GABA is regarded as safe for human use and is available as a dietary supplement in the USA, China, Japan, and much of Europe (100). In other countries, because GABA is a non-protein amino acid, it is regulated as a medicinal agent or drug (e.g., in the UK, Canada, and Australia). In our studies, GABA at 2.0 and 0.2 mg/mL were equally effective at protecting SARS-CoV-2 infected mice from death (Figure 1B). The human equivalent dose of GABA at 0.2 mg/mL [assuming consumption of 3.5 mL/day water, see Supplemental Figure 1 and calculated as per (101)] is 0.68 g/day for a 70 kg person, which is well within the level known to be safe (100). While our preclinical observations indicate that GABA-R agonists are promising candidates to help treat coronavirus infections, information on their dosing and the time window during which their effects might be beneficial or deleterious during a coronavirus infection in humans are lacking and clinical trials are needed to assess their therapeutic potential.
Limitations of this study
There are a number of major limitations of this study. First, the K18-hACE2 mouse model imperfectly models SARS-CoV-2 infection and immune responses in humans. Second, GABA’s impact on immune cells and lung cells may differ in important ways between mice and humans. Third, GABA treatment may only be beneficial during a specific time window of the disease process and at other times may be deleterious. Accordingly, careful clinical trials are needed to determine the time window and dosage, if any, that GABA-R agonist treatment has a beneficial effect in COVID-19 patients.
Materials and methods
Virus
SARS-CoV-2 (USA-WA1/2020) was obtained from the Biodefense and Emerging Infections Resources of the National Institute of Allergy and Infectious Diseases. All in vivo studies of SARS-CoV-2 infection were conducted within a Biosafety Level 3 facility at UCLA or USC. SARS-CoV-2 stocks were generated by infection of Vero-E6 cells (American Type Culture Collection (ATCC CRL1586)) cultured in DMEM growth media containing 10% fetal bovine serum, 2 mM L-glutamine, penicillin (100 units/ml), streptomycin (100 units/ml), and 10 mM HEPES. The cells were incubated at 37°C with 5% CO2 and virus titers were determined as described below for tittering virus in lung homogenates.
Mice and GABA treatments
Female K18-hACE2 mice (8 weeks in age) were purchased from the Jackson Laboratory. The first survival study was conducted within USC’s BSL3 Core facility and all following studies were conducted in the UCLA ABSL3 Core Facility. One week after arrival, they were inoculated with SARS-Cov-2 (2,000 PFU or 2,000 TCID50 (as per (64) at USC and UCLA, respectively) in 20 μl Dulbecco’s modified Eagle’s medium. The mice were randomized into groups of 5-9 mice and were placed on water bottles containing 0, 0.2, or 2.0 mg/mL GABA (Millipore-Sigma (stock #A2129), St. Louis, MO, USA) immediately, or 2 days post-infection (as indicated) and maintained on those treatments for the remainder of each study. These studies were carried out in accordance with the recommendations of the Guide for the Care and Use of Laboratory Animals of the National Institutes of Health. The protocols for all experiments using vertebrate animals were approved by the Animal Research Committee at UCLA (Protocol ID: ARC #2020-122; 8/25/20-8/24/2023) or USC (IACUC protocol # 21258; 1/28/2021-1/27/2024) and were carried out in compliance with the ARRIVE guidelines.
Illness scoring and lung index score
Individual mice were monitored by two observers twice daily for their illness development and progression which were scored on the following scale: 0) no symptoms, 1) slightly ruffled fur and altered hind limb posture; 2) ruffled fur and mildly labored breathing; 3) ruffled fur, inactive, moderately labored breathing; 4) ruffled fur, inactive, obviously labored breathing, hunched posture; 5) moribund or dead.
The percent survival of each group of mice was determined longitudinally. Mice with a disease score of 5 were weighed, euthanized, and their lungs removed and weighed for calculation of lung coefficient index (the ratio of lung weight to total body weight, which reflects the extent of edema and inflammation in the lungs). On day 7 or 8 post-infection, the surviving animals were weighed, euthanized, and their lungs were removed and weighed for determination of the lung coefficient index.
Histology
Individual mice were infected intranasally with SARS-CoV-2 virus and two days later, they were randomized to receive plain water, or water containing GABA (2 mg/mL) for the remainder of the study. On days 5-7 post infection, those mice which met euthanasia criteria or survived until the end of the study on day 7, were euthanized and their lungs were dissected. The left lung from each mouse was fixed in 10% neutral buffered formalin and embedded in paraffin. The lung tissue sections (4 µm) were routine-stained with hematoxylin and eosin. Five images from each mouse were captured under a light microscope at 200 × magnification. The degrees of pathological changes were scored, as in our previous report (54). Briefly, the degrees of pathological changes were scored, based on the number of hyaline-like membranes, % of pulmonary areas with obvious inflammatory infiltrates in lung parenchyma, and the % of area with inflammatory consolidation within the total area of the section. The total numbers of hyaline-like membranes with, or without, cell debris or hyaline-like deposition in alveoli of the lung tissue section were scored as 0: none detectable; 1: 1–5; 2: 6–10; 3: >10. The areas of lung inflammation and hemorrhage in one lung section were estimated and the severity of inflammation and hemorrhage in the section was scored as 1: mild; 2: moderate; 3: marked; 4: severe. Accordingly, an inflammatory score in each mouse was obtained by % of lung areas × severity score. The areas of lung inflammatory consolidation were estimated in the lung section and scored as 1: ≤10%; 2: 11–25%; 3: 26–50%; 4; >50%. Finally, the pneumonitis score of individual mice = inflammation score + lung consolidation score + hyaline membrane score with a maximum score of 11.
Viral titers in lungs
Female K18-ACE2 mice (9 weeks in age) were intranasally inoculated with 2,000 TCID50 SARS-Cov-2 and placed on plain water or water containing GABA (2 mg/mL) for the rest of the study. Three days post-infection, the mice were euthanized and individual blood samples were collected for preparing serum samples. A portion of their lower right lung was weighed and about 100 mg of wet lung tissue from each mouse was homogenized into 1 mL of ice-cold DMEM with 10% fetal calf serum (FBS) with 1 mm glass beads using a Minilys homogenizer at 50 Hz for 90 seconds, followed by centrifugation. Their supernatants were collected for virus tittering. Vero E6 cells (1x104 cells/well) were cultured in DMEM medium supplemented with 10% FBS in 96-well plates overnight to reach 80% of confluency and infected in quintuplicate with a series of diluted mouse lung homogenates in 100 µl of FBS-free DMEM medium at 37° C for four days. The percentages of viral cytopathic effect areas were determined. The SARS-CoV-2 titers were calculated by the Reed and Muench method (102).
Simultaneous detection of multiple cytokines and chemokinesby flow cytometry
Blood samples were collected from the same mice that were used to study viral loads in the lungs at three days post-infection. Sera from individual mice were prepared and stored at -80°C. The levels of serum cytokines and chemokines were determined by a bead-based multiplex assay using the LEGENDplex mouse anti-virus response panel (13-plex) kit (#740622, Biolegend, San Diego, USA), according to the manufacturer’s instructions. Briefly, the control and experimental groups of serum samples were diluted at 1:2 and tested in duplicate simultaneously. After being washed, the fluorescent signals in each well were analyzed by flow cytometry in an ATTUNE NxT flow cytometer (Thermofisher). The data were analyzed using the LEGENDplex™ Data Analysis Software Suite (Biolegend) and the levels of each cytokine or chemokine in serum samples were calculated, according to the standard curves established using the standards provided.
Statistics
Statistical methods are described in each figure legend. A p-value of <0.05 was considered statistically significant.
Data availability statement
The raw data supporting the conclusions of this article will be made available by the authors, without undue reservation.
Ethics statement
The animal study was reviewed and approved by Animal Research Committee at UCLA (Protocol ID: ARC #2020-122; Date 8/25/20-8/24/2023 and BUA-2021-071-001) and USC (IACUC protocol # 21258 (1/28/2021-1/27/2024), IBC: BUA 20-0030/20-0033).
Author contributions
Conceived and designed the experiments: JT and DK; Performed the experiments: JT, BD, and JH; Supervised work and assessed data quality in the ABSL3 labs BD, LC. Analyzed the data: JT, DLK. Wrote the paper: JT, DLK. DLK and JT are guarantors of this work and, as such, had full access to all the data in the study and take responsibility for the integrity of the data and the accuracy of the data analysis. All authors contributed to the article and approved the submitted version.
Funding
This work was supported by a grant to DK from the UCLA DGSOM-Broad Stem Cell Research Center COVID-19 Research Award (ORC #21-93), DK’s unrestricted funds, JT’s unrestricted funds, funds from the Department of Molecular and Medical Pharmacology, and the Immunotherapeutics Research Fund. Work in the Hasting Foundation and the Wright Foundation BSL3 lab at the Keck School of Medicine of USC was supported by a grant from the COVID-19 Keck Research Fund to LC. Statistical analysis was supported by a grant from the NCATS, UCLA CTSI Grant Number UL1TR001881.
Acknowledgments
We would like to thank Dr. Vaithilingaraja Arumugaswami for his kind provision of virus stock and Drs. Jennifer Hahn, Orian Shirihai, Anton Petcherski, Theodoros Kelesidis, and Joanne L. Zahorsky-Reeves, as well as Madhav Sharma, Melanie Ciampaglia, Tomoko Yamada, and Lenore Kaufman for their help. We thank Lucia Chen, Dr. Jeffery Gornbein, and Dr. Alexandra Klomhaus for statistical analysis and figure preparation, and Miss. Salem Haile in the Janice Gorgi Flow Cytometry Center for FACS analysis. We thank Jeremy Marshall and Philip Sell for their support in the ABSL3 lab at USC.
Conflict of interest
DLK and JT are inventors of GABA-related patents. DLK serves on the Scientific Advisory Board of Diamyd Medical. DLK gifted the Immunotherapeutics Research Fund.
The remaining authors declare that the research was conducted in the absence of any commercial or financial relationships that could be construed as a potential conflict of interest.
Publisher’s note
All claims expressed in this article are solely those of the authors and do not necessarily represent those of their affiliated organizations, or those of the publisher, the editors and the reviewers. Any product that may be evaluated in this article, or claim that may be made by its manufacturer, is not guaranteed or endorsed by the publisher.
Supplementary material
The Supplementary Material for this article can be found online at: https://www.frontiersin.org/articles/10.3389/fimmu.2022.1007955/full#supplementary-material
References
1. Olsen RW, Sieghart W. GABA a receptors: subtypes provide diversity of function and pharmacology. Neuropharmacology (2009) 56(1):141–8. doi: 10.1016/j.neuropharm.2008.07.045
2. Ben-Ari Y. Excitatory actions of gaba during development: the nature of the nurture. Nat Rev Neurosci (2002) 3(9):728–39. doi: 10.1038/nrn920
3. Deidda G, Bozarth IF, Cancedda L. Modulation of GABAergic transmission in development and neurodevelopmental disorders: investigating physiology and pathology to gain therapeutic perspectives. Front Cell Neurosci (2014) 8:119. doi: 10.3389/fncel.2014.00119
4. Tian J, Chau C, Hales TG, Kaufman DL. GABA(A) receptors mediate inhibition of T cell responses. J Neuroimmunol (1999) 96(1):21–8. doi: 10.1016/s0165-5728(98)00264-1
5. Bhandage AK, Jin Z, Korol SV, Shen Q, Pei Y, Deng Q, et al. GABA regulates release of inflammatory cytokines from peripheral blood mononuclear cells and CD4(+) T cells and is immunosuppressive in type 1 diabetes. EBioMedicine (2018) 30:283–94. doi: 10.1016/j.ebiom.2018.03.019
6. Tian J, Middleton B, Lee VS, Park HW, Zhang Z, Kim B, et al. GABAB-receptor agonist-based immunotherapy for type 1 diabetes in NOD mice. Biomedicines (2021) 9(1). doi: 10.3390/biomedicines9010043
7. Zhang B, Vogelzang A, Miyajima M, Sugiura Y, Wu Y, Chamoto K, et al. B cell-derived GABA elicits IL-10(+) macrophages to limit anti-tumour immunity. Nature (2021) 599(7885):471–6. doi: 10.1038/s41586-021-04082-1
8. Bhandage AK, Barragan A. GABAergic signaling by cells of the immune system: more the rule than the exception. Cell Mol Life Sci (2021) 78(15):5667–79. doi: 10.1007/s00018-021-03881-z
9. Mendu SK, Bhandage A, Jin Z, Birnir B. Different subtypes of GABA-a receptors are expressed in human, mouse and rat T lymphocytes. PLoS One (2012) 7(8):e42959. doi: 10.1371/journal.pone.0042959
10. Januzi L, Poirier JW, Maksoud MJE, Xiang YY, Veldhuizen RAW, Gill SE, et al. Autocrine GABA signaling distinctively regulates phenotypic activation of mouse pulmonary macrophages. Cell Immunol (2018) 332:7–23. doi: 10.1016/j.cellimm.2018.07.001
11. Tian J, Yong J, Dang H, Kaufman DL. Oral GABA treatment downregulates inflammatory responses in a mouse model of rheumatoid arthritis. Autoimmunity (2011) 44:465–70. doi: 10.3109/08916934.2011.571223
12. Bhat R, Axtell R, Mitra A, Miranda M, Lock C, Tsien RW, et al. Inhibitory role for GABA in autoimmune inflammation. Proc Natl Acad Sci U S A (2010) 107(6):2580–5. doi: 10.1073/pnas.0915139107
13. Wheeler DW, Thompson AJ, Corletto F, Reckless J, Loke JC, Lapaque N, et al. Anaesthetic impairment of immune function is mediated via GABA(A) receptors. PLoS One (2011) 6(2):e17152. doi: 10.1371/journal.pone.0017152
14. Reyes-Garcia MG, Hernandez-Hernandez F, Hernandez-Tellez B, Garcia-Tamayo F. GABA (A) receptor subunits RNA expression in mice peritoneal macrophages modulate their IL-6/IL-12 production. J Neuroimmunol (2007) 188(1-2):64–8. doi: 10.1016/j.jneuroim.2007.05.013
15. Tian J, Song M, Kaufman DL. Homotaurine limits the spreading of T cell autoreactivity within the CNS and ameliorates disease in a model of multiple sclerosis. Sci Rep (2021) 11(1):5402. doi: 10.1038/s41598-021-84751-3
16. Bhandage AK, Friedrich LM, Kanatani S, Jakobsson-Bjorken S, Escrig-Larena JI, Wagner AK, et al. GABAergic signaling in human and murine NK cells upon challenge with toxoplasma gondii. J Leukoc Biol (2021) 10:617–28. doi: 10.1002/JLB.3HI0720-431R
17. Kim JK, Kim YS, Lee HM, Jin HS, Neupane C, Kim S, et al. GABAergic signaling linked to autophagy enhances host protection against intracellular bacterial infections. Nat Commun (2018) 9(1):4184. doi: 10.1038/s41467-018-06487-5
18. Tian J, Dang H, Nguyen AV, Chen Z, Kaufman DL. Combined therapy with GABA and proinsulin/alum acts synergistically to restore long-term normoglycemia by modulating T-cell autoimmunity and promoting beta-cell replication in newly diabetic NOD mice. Diabetes (2014) 63(9):3128–34. doi: 10.2337/db13-1385
19. Tian J, Dang H, O’Laco K, Song M, Tiu B-C, Gilles S, et al. Homotaurine treatment enhances CD4+ and CD8+ treg responses and synergizes with low-dose anti-CD3 to enhance diabetes remission in type 1 diabetic mice. ImmuoHorizons (2019) 3(10):498–510. doi: 10.4049/immunohorizons.1900019
20. Tian J, Dang H, Wallner M, Olsen R, Kaufman DL. Homotaurine, a safe blood-brain barrier permeable GABAA-r-specific agonist, ameliorates disease in mouse models of multiple sclerosis. Sci Rep (2018) 8(1):16555. doi: 10.1038/s41598-018-32733-3
21. Prud'homme GJ, Glinka Y, Hasilo C, Paraskevas S, Li X, Wang Q. GABA protects human islet cells against the deleterious effects of immunosuppressive drugs and exerts immunoinhibitory effects alone. Transplantation (2013) 96(7):616–23. doi: 10.1097/TP.0b013e31829c24be
22. Mendu SK, Akesson L, Jin Z, Edlund A, Cilio C, Lernmark A, et al. Increased GABA(A) channel subunits expression in CD8(+) but not in CD4(+) T cells in BB rats developing diabetes compared to their congenic littermates. Mol Immunol (2011) 48(4):399–407. doi: 10.1016/j.molimm.2010.08.005
23. Soltani N, Qiu H, Aleksic M, Glinka Y, Zhao F, Liu R, et al. GABA exerts protective and regenerative effects on islet beta cells and reverses diabetes. Proc Natl Acad Sci U S A (2011) 108(28):11692–7. doi: 10.1073/pnas.1102715108
24. Tian J, Dang HN, Yong J, Chui WS, Dizon MP, Yaw CK, et al. Oral treatment with gamma-aminobutyric acid improves glucose tolerance and insulin sensitivity by inhibiting inflammation in high fat diet-fed mice. PLoS One (2011) 6(9):e25338. doi: 10.1371/journal.pone.0025338
25. Tian J, Lu Y, Zhang H, Chau CH, Dang HN, Kaufman DL. Gamma-aminobutyric acid inhibits T cell autoimmunity and the development of inflammatory responses in a mouse type 1 diabetes model. J Immunol (2004) 173(8):5298–304. doi: 10.4049/jimmunol.173.8.5298
26. Song M, Tian J, Middleton B, Nguyen CQ, Kaufman DL. GABA administration ameliorates sjogren's syndrome in two different mouse models. Biomedicines (2022) 10(1). doi: 10.3390/biomedicines10010129
27. Vabret N, Britton GJ, Gruber C, Hegde S, Kim J, Kuksin M, et al. Immunology of COVID-19: Current state of the science. Immunity (2020) 52(6):910–41. doi: 10.1016/j.immuni.2020.05.002
28. Lucas C, Wong P, Klein J, Castro TBR, Silva J, Sundaram M, et al. Longitudinal analyses reveal immunological misfiring in severe COVID-19. Nature (2020) 584(7821):463–9. doi: 10.1038/s41586-020-2588-y
29. Channappanavar R, Perlman S. Pathogenic human coronavirus infections: causes and consequences of cytokine storm and immunopathology. Semin Immunopathol (2017) 39(5):529–39. doi: 10.1007/s00281-017-0629-x
30. Sariol A, Perlman S. Lessons for COVID-19 immunity from other coronavirus infections. Immunity (2020) 53(2):248–63. doi: 10.1016/j.immuni.2020.07.005
31. Grant RA, Morales-Nebreda L, Markov NS, Swaminathan S, Querrey M, Guzman ER, et al. Circuits between infected macrophages and T cells in SARS-CoV-2 pneumonia. Nature (2021) 590(7847):635–41. doi: 10.1038/s41586-020-03148-w
32. Jin N, Kolliputi N, Gou D, Weng T, Liu L. A novel function of ionotropic gamma-aminobutyric acid receptors involving alveolar fluid homeostasis. J Biol Chem (2006) 281(47):36012–20. doi: 10.1074/jbc.M606895200
33. Xiang YY, Chen X, Li J, Wang S, Faclier G, Macdonald JF, et al. Isoflurane regulates atypical type-a gamma-aminobutyric acid receptors in alveolar type II epithelial cells. Anesthesiology (2013) 118(5):1065–75. doi: 10.1097/ALN.0b013e31828e180e
34. Xiang YY, Wang S, Liu M, Hirota JA, Li J, Ju W, et al. A GABAergic system in airway epithelium is essential for mucus overproduction in asthma. Nat Med (2007) 13(7):862–7. doi: 10.1038/nm1604
35. Barrios J, Kho AT, Aven L, Mitchel JA, Park JA, Randell SH, et al. Pulmonary neuroendocrine cells secrete gamma-aminobutyric acid to induce goblet cell hyperplasia in primate models. Am J Respir Cell Mol Biol (2019) 60(6):687–94. doi: 10.1165/rcmb.2018-0179OC
36. Huang T, Zhang Y, Wang C, Gao J. Propofol reduces acute lung injury by up-regulating gamma-aminobutyric acid type a receptors. Exp Mol Pathol (2019) 110:104295. doi: 10.1016/j.yexmp.2019.104295
37. Fortis S, Spieth PM, Lu WY, Parotto M, Haitsma JJ, Slutsky AS, et al. Effects of anesthetic regimes on inflammatory responses in a rat model of acute lung injury. Intensive Care Med (2012) 38(9):1548–55. doi: 10.1007/s00134-012-2610-4
38. Chintagari NR, Liu L. GABA receptor ameliorates ventilator-induced lung injury in rats by improving alveolar fluid clearance. Crit Care (2012) 16(2):R55. doi: 10.1186/cc11298
39. Jin S, Merchant ML, Ritzenthaler JD, McLeish KR, Lederer ED, Torres-Gonzalez E, et al. Baclofen, a GABABR agonist, ameliorates immune-complex mediated acute lung injury by modulating pro-inflammatory mediators. PLoS One (2015) 10(4):e0121637. doi: 10.1371/journal.pone.0121637
40. Voigtsberger S, Lachmann RA, Leutert AC, Schlapfer M, Booy C, Reyes L, et al. Sevoflurane ameliorates gas exchange and attenuates lung damage in experimental lipopolysaccharide-induced lung injury. Anesthesiology (2009) 111(6):1238–48. doi: 10.1097/ALN.0b013e3181bdf857
41. Faller S, Strosing KM, Ryter SW, Buerkle H, Loop T, Schmidt R, et al. The volatile anesthetic isoflurane prevents ventilator-induced lung injury via phosphoinositide 3-kinase/Akt signaling in mice. Anesth Analg (2012) 114(4):747–56. doi: 10.1213/ANE.0b013e31824762f0
42. Taniguchi T, Yamamoto K, Ohmoto N, Ohta K, Kobayashi T. Effects of propofol on hemodynamic and inflammatory responses to endotoxemia in rats. Crit Care Med (2000) 28(4):1101–6. doi: 10.1097/00003246-200004000-00032
43. Lin X, Ju YN, Gao W, Li DM, Guo CC. Desflurane attenuates ventilator-induced lung injury in rats with acute respiratory distress syndrome. BioMed Res Int (2018) 2018:7507314. doi: 10.1155/2018/7507314
44. Mahmoud K, Ammar A. Immunomodulatory effects of anesthetics during thoracic surgery. Anesthesiol Res Pract (2011) 2011:317410. doi: 10.1155/2011/317410
45. De Conno E, Steurer MP, Wittlinger M, Zalunardo MP, Weder W, Schneiter D, et al. Anesthetic-induced improvement of the inflammatory response to one-lung ventilation. Anesthesiology (2009) 110(6):1316–26. doi: 10.1097/ALN.0b013e3181a10731
46. Schilling T, Kozian A, Kretzschmar M, Huth C, Welte T, Buhling F, et al. Effects of propofol and desflurane anaesthesia on the alveolar inflammatory response to one-lung ventilation. Br J Anaesth (2007) 99(3):368–75. doi: 10.1093/bja/aem184
47. Kochiyama T, Li X, Nakayama H, Kage M, Yamane Y, Takamori K, et al. Effect of propofol on the production of inflammatory cytokines by human polarized macrophages. Mediators Inflammation (2019) 2019:1919538. doi: 10.1155/2019/1919538
48. Forkuo GS, Nieman AN, Kodali R, Zahn NM, Li G, Rashid Roni MS, et al. A novel orally available asthma drug candidate that reduces smooth muscle constriction and inflammation by targeting GABAA receptors in the lung. Mol Pharm (2018) 15(5):1766–77. doi: 10.1021/acs.molpharmaceut.7b01013
49. Boost KA, Leipold T, Scheiermann P, Hoegl S, Sadik CD, Hofstetter C, et al. Sevoflurane and isoflurane decrease TNF-alpha-induced gene expression in human monocytic THP-1 cells: potential role of intracellular IkappaBalpha regulation. Int J Mol Med (2009) 23(5):665–71. doi: 10.3892/ijmm_00000178
50. Bredthauer A, Geiger A, Gruber M, Pfaehler SM, Petermichl W, Bitzinger D, et al. Propofol ameliorates exaggerated human neutrophil activation in a LPS sepsis model. J Inflammation Res (2021) 14:3849–62. doi: 10.2147/JIR.S314192
51. Lin KH, Lu WJ, Wang SH, Fong TH, Chou DS, Chang CC, et al. Characteristics of endogenous gamma-aminobutyric acid (GABA) in human platelets: functional studies of a novel collagen glycoprotein VI inhibitor. J Mol Med (Berl) (2014) 92(6):603–14. doi: 10.1007/s00109-014-1140-7
52. Masoodi M, Peschka M, Schmiedel S, Haddad M, Frye M, Maas C, et al. Disturbed lipid and amino acid metabolisms in COVID-19 patients. J Mol Med (Berl) (2022) 100(4):555–68. doi: 10.1007/s00109-022-02177-4
53. Karu N, Kindt A, van Gammeren AJ, Ermens AAM, Harms AC, Portengen L, et al. Severe COVID-19 is characterised by perturbations in plasma amines correlated with immune response markers, and linked to inflammation and oxidative stress. Metabolites (2022) 12(7). doi: 10.3390/metabo12070618
54. Tian J, Middleton B, Kaufman DL. GABAA-receptor agonists limit pneumonitis and death in murine coronavirus-infected mice. Viruses (2021) 13(6). doi: 10.3390/v13060966
55. De Albuquerque N, Baig E, Ma X, Zhang J, He W, Rowe A, et al. Murine hepatitis virus strain 1 produces a clinically relevant model of severe acute respiratory syndrome in A/J mice. J Virol (2006) 80(21):10382–94. doi: 10.1128/JVI.00747-06
56. Khanolkar A, Hartwig SM, Haag BA, Meyerholz DK, Epping LL, Haring JS, et al. Protective and pathologic roles of the immune response to mouse hepatitis virus type 1: implications for severe acute respiratory syndrome. J Virol (2009) 83(18):9258–72. doi: 10.1128/JVI.00355-09
57. Khanolkar A, Hartwig SM, Haag BA, Meyerholz DK, Harty JT, Varga SM. Toll-like receptor 4 deficiency increases disease and mortality after mouse hepatitis virus type 1 infection of susceptible C3H mice. J Virol (2009) 83(17):8946–56. doi: 10.1128/JVI.01857-08
58. Khanolkar A, Fulton RB, Epping LL, Pham NL, Tifrea D, Varga SM, et al. T Cell epitope specificity and pathogenesis of mouse hepatitis virus-1-induced disease in susceptible and resistant hosts. J Immunol (2010) 185(2):1132–41. doi: 10.4049/jimmunol.0902749
59. Caldera-Crespo LA, Paidas MJ, Roy S, Schulman CI, Kenyon NS, Daunert S, et al. Experimental models of COVID-19. Front Cell Infect Microbiol (2021) 11:792584. doi: 10.3389/fcimb.2021.792584
60. Korner RW, Majjouti M, Alcazar MAA, Mahabir E. Of mice and men: The coronavirus MHV and mouse models as a translational approach to understand SARS-CoV-2. Viruses (2020) 12(8). doi: 10.3390/v12080880
61. McCray PB Jr., Pewe L, Wohlford-Lenane C, Hickey M, Manzel L, Shi L, et al. Lethal infection of K18-hACE2 mice infected with severe acute respiratory syndrome coronavirus. J Virol (2007) 81(2):813–21. doi: 10.1128/JVI.02012-06
62. Moreau GB, Burgess SL, Sturek JM, Donlan AN, Petri WA, Mann BJ. Evaluation of K18-hACE2 mice as a model of SARS-CoV-2 infection. Am J Trop Med Hyg (2020) 103(3):1215–9. doi: 10.4269/ajtmh.20-0762
63. Rathnasinghe R, Strohmeier S, Amanat F, Gillespie VL, Krammer F, Garcia-Sastre A, et al. Comparison of transgenic and adenovirus hACE2 mouse models for SARS-CoV-2 infection. Emerg Microbes Infect (2020) 9(1):2433–45. doi: 10.1080/22221751.2020.1838955
64. Golden JW, Cline CR, Zeng X, Garrison AR, Carey BD, Mucker EM, et al. Human angiotensin-converting enzyme 2 transgenic mice infected with SARS-CoV-2 develop severe and fatal respiratory disease. JCI Insight (2020) 5(19). doi: 10.1172/jci.insight.142032
65. Winkler ES, Bailey AL, Kafai NM, Nair S, McCune BT, Yu J, et al. SARS-CoV-2 infection in the lungs of human ACE2 transgenic mice causes severe inflammation, immune cell infiltration, and compromised respiratory function. bioRxiv (2020). doi: 10.1101/2020.07.09.196188
66. Oladunni FS, Park JG, Pino PA, Gonzalez O, Akhter A, Allue-Guardia A, et al. Lethality of SARS-CoV-2 infection in K18 human angiotensin-converting enzyme 2 transgenic mice. Nat Commun (2020) 11(1):6122. doi: 10.1038/s41467-020-19891-7
67. Zheng J, Wong LR, Li K, Verma AK, Ortiz ME, Wohlford-Lenane C, et al. COVID-19 treatments and pathogenesis including anosmia in K18-hACE2 mice. Nature (2021) 589(7843):603–7. doi: 10.1038/s41586-020-2943-z
68. Winkler ES, Bailey AL, Kafai NM, Nair S, McCune BT, Yu J, et al. SARS-CoV-2 infection of human ACE2-transgenic mice causes severe lung inflammation and impaired function. Nat Immunol (2020) 21(11):1327–35. doi: 10.1038/s41590-020-0778-2
69. NCATS COVID-19 OpenData portal. Available at: https://opendata.ncats.nih.gov/covid19/databrowser.
70. Zhang X, Zhang R, Zheng Y, Shen J, Xiao D, Li J, et al. Expression of gamma-aminobutyric acid receptors on neoplastic growth and prediction of prognosis in non-small cell lung cancer. J Transl Med (2013) 11:102. doi: 10.1186/1479-5876-11-102
71. Bai D, Fang L, Xia S, Ke W, Wang J, Wu X, et al. Porcine deltacoronavirus (PDCoV) modulates calcium influx to favor viral replication. Virology (2020) 539:38–48. doi: 10.1016/j.virol.2019.10.011
72. Kraeft SK, Chen DS, Li HP, Chen LB, Lai MM. Mouse hepatitis virus infection induces an early, transient calcium influx in mouse astrocytoma cells. Exp Cell Res (1997) 237(1):55–62. doi: 10.1006/excr.1997.3768
73. Loas G, Van de Borne P, Darquennes G, Le Corre P. Association of amlodipine with the risk of in-hospital death in patients with COVID-19 and hypertension: A reanalysis on 184 COVID-19 patients with hypertension. Pharm (Basel) (2022) 15(3). doi: 10.3390/ph15030380
74. Sadeghpopur S, Ghasemnejad-Berenji H, Pashapour S, Ghasemnejad-Berenji M. Using of calcium channel blockers in patients with COVID-19: a magic bullet or a double-edged sword? J Basic Clin Physiol Pharmacol (2021) 33(1):117–9. doi: 10.1515/jbcpp-2021-0334
75. Zhang LK, Sun Y, Zeng H, Wang Q, Jiang X, Shang WJ, et al. Calcium channel blocker amlodipine besylate therapy is associated with reduced case fatality rate of COVID-19 patients with hypertension. Cell Discovery (2020) 6(1):96. doi: 10.1038/s41421-020-00235-0
76. Blanco-Melo D, Nilsson-Payant BE, Liu WC, Uhl S, Hoagland D, Moller R, et al. Imbalanced host response to SARS-CoV-2 drives development of COVID-19. Cell (2020) 181(5):1036–45.e9. doi: 10.1016/j.cell.2020.04.026
77. Karimabad MN, Kounis NG, Hassanshahi G, Hassanshahi F, Mplani V, Koniari I, et al. The involvement of CXC motif chemokine ligand 10 (CXCL10) and its related chemokines in the pathogenesis of coronary artery disease and in the COVID-19 vaccination: A narrative review. Vaccines (Basel) (2021) 9(11). doi: 10.3390/vaccines9111224
78. Blot M, Jacquier M, Aho Glele LS, Beltramo G, Nguyen M, Bonniaud P, et al. CXCL10 could drive longer duration of mechanical ventilation during COVID-19 ARDS. Crit Care (2020) 24(1):632. doi: 10.1186/s13054-020-03328-0
79. Ravindran R, McReynolds C, Yang J, Hammock BD, Ikram A, Ali A, et al. Immune response dynamics in COVID-19 patients to SARS-CoV-2 and other human coronaviruses. PloS One (2021) 16(7):e0254367. doi: 10.1371/journal.pone.0254367
80. Kwon JS, Kim JY, Kim MC, Park SY, Kim BN, Bae S, et al. Factors of severity in patients with COVID-19: Cytokine/Chemokine concentrations, viral load, and antibody responses. Am J Trop Med Hyg (2020) 103(6):2412–8. doi: 10.4269/ajtmh.20-1110
81. Tripathy AS, Vishwakarma S, Trimbake D, Gurav YK, Potdar VA, Mokashi ND, et al. Pro-inflammatory CXCL-10, TNF-alpha, IL-1beta, and IL-6: biomarkers of SARS-CoV-2 infection. Arch Virol (2021) 166(12):3301–10. doi: 10.1007/s00705-021-05247-z
82. Liu M, Guo S, Hibbert JM, Jain V, Singh N, Wilson NO, et al. CXCL10/IP-10 in infectious diseases pathogenesis and potential therapeutic implications. Cytokine Growth Factor Rev (2011) 22(3):121–30. doi: 10.1016/j.cytogfr.2011.06.001
83. Abers MS, Delmonte OM, Ricotta EE, Fintzi J, Fink DL, de Jesus AAA, et al. An immune-based biomarker signature is associated with mortality in COVID-19 patients. JCI Insight (2021) 6(1). doi: 10.1172/jci.insight.144455
84. Han H, Ma Q, Li C, Liu R, Zhao L, Wang W, et al. Profiling serum cytokines in COVID-19 patients reveals IL-6 and IL-10 are disease severity predictors. Emerg Microbes Infect (2020) 9(1):1123–30. doi: 10.1080/22221751.2020.1770129
85. Zhao Y, Qin L, Zhang P, Li K, Liang L, Sun J, et al. Longitudinal COVID-19 profiling associates IL-1RA and IL-10 with disease severity and RANTES with mild disease. JCI Insight (2020) 5(13). doi: 10.1172/jci.insight.139834
86. Yang AC, Kern F, Losada PM, Agam MR, Maat CA, Schmartz GP, et al. Dysregulation of brain and choroid plexus cell types in severe COVID-19. Nature (2021) 595(7868):565–71. doi: 10.1038/s41586-021-03710-0
87. Douaud G, Lee S, Alfaro-Almagro F, Arthofer C, Wang C, McCarthy P, et al. SARS-CoV-2 is associated with changes in brain structure in UK biobank. Nature (2022) 604(7907):697–707. doi: 10.1038/s41586-022-04569-5
88. Frere JJ, Serafini RA, Pryce KD, Zazhytska M, Oishi K, Golynker I, et al. SARS-CoV-2 infection results in lasting and systemic perturbations post recovery. Biorxiv (2022). doi: 10.1101/2022.01.18.476786
89. Crunfli F, Carregari CC, Veras FP, Henrique Vendramini P, Valença AGF, Antunes ASLM, et al. Morphological, cellular and molecular basis of brain infection in COVID-19 patients. MedRxiv (2020) 16. doi: 10.1101/2020.10.09.20207464
90. Virhammar J, Naas A, Fallmar D, Cunningham JL, Klang A, Ashton NJ, et al. Biomarkers for central nervous system injury in cerebrospinal fluid are elevated in COVID-19 and associated with neurological symptoms and disease severity. Eur J Neurol (2021) 28(10):3324–31. doi: 10.1111/ene.14703
91. Kanberg N, Ashton NJ, Andersson LM, Yilmaz A, Lindh M, Nilsson S, et al. Neurochemical evidence of astrocytic and neuronal injury commonly found in COVID-19. Neurology (2020) 95(12):e1754–e9. doi: 10.1212/WNL.0000000000010111
92. Lee MH, Perl DP, Nair G, Li W, Maric D, Murray H, et al. Microvascular injury in the brains of patients with covid-19. N Engl J Med (2021) 384(5):481–3. doi: 10.1056/NEJMc2033369
93. Johansson A, Mohamed MS, Moulin TC, Schioth HB. Neurological manifestations of COVID-19: A comprehensive literature review and discussion of mechanisms. J Neuroimmunol (2021) 358:577658. doi: 10.1016/j.jneuroim.2021.577658
94. Lee M, Schwab C, McGeer PL. Astrocytes are GABAergic cells that modulate microglial activity. Glia (2011) 59(1):152–65. doi: 10.1002/glia.21087
95. Manzano S, Aguera L, Aguilar M, Olazaran J. A review on tramiprosate (Homotaurine) in alzheimer's disease and other neurocognitive disorders. Front Neurol (2020) 11:614. doi: 10.3389/fneur.2020.00614
96. Aisen PS, Gauthier S, Ferris SH, Saumier D, Haine D, Garceau D, et al. Tramiprosate in mild-to-moderate alzheimer's disease - a randomized, double-blind, placebo-controlled, multi-centre study (the alphase study). Arch Med Sci (2010) 7(1):102–11. doi: 10.5114/aoms.2011.20612
97. Tsolaki M. Future strategies of management of alzheimer's disease. the role of homotaurine. Hell J Nucl Med (2019) 22 Suppl:82–94.
98. Gauthier S, Aisen PS, Ferris SH, Saumier D, Duong A, Haine D, et al. Effect of tramiprosate in patients with mild-to-moderate alzheimer's disease: exploratory analyses of the MRI sub-group of the alphase study. J Nutr Health Aging (2009) 13(6):550–7. doi: 10.1007/s12603-009-0106-x
99. Bossù P, Salani F, Ciaramella A, Sacchinelli E, Mosca A, Banaj N, et al. Anti-inflammatory effects of homotaurine in patients with amnestic mild cognitive impairment. Front Aging Neurosci (2018) 210:285. doi: 10.3389/fnagi.2018.00285
100. Oketch-Rabah HA, Madden EF, Roe AL, Betz JM. United states pharmacopeia (USP) safety review of gamma-aminobutyric acid (GABA). Nutrients (2021) 13(8). doi: 10.3390/nu13082742
101. Nair AB, Jacob S. A simple practice guide for dose conversion between animals and human. J Basic Clin Pharm (2016) 7(2):27–31. doi: 10.4103/0976-0105.177703
Keywords: gaba, GABA-receptors, COVID-19, SARS-CoV-2, antiviral, long-covid, neuroinflammation, homotaurine
Citation: Tian J, Dillion BJ, Henley J, Comai L and Kaufman DL (2022) A GABA-receptor agonist reduces pneumonitis severity, viral load, and death rate in SARS-CoV-2-infected mice. Front. Immunol. 13:1007955. doi: 10.3389/fimmu.2022.1007955
Received: 31 July 2022; Accepted: 07 October 2022;
Published: 25 October 2022.
Edited by:
Alfonso J. Rodriguez-Morales, Fundacion Universitaria Autónoma de las Américas, ColombiaReviewed by:
Stephen Rawlings, Maine Health, United StatesAndrea Gabriela Rodríguez Morales, CSR Salvador Allende, Chile
Copyright © 2022 Tian, Dillion, Henley, Comai and Kaufman. This is an open-access article distributed under the terms of the Creative Commons Attribution License (CC BY). The use, distribution or reproduction in other forums is permitted, provided the original author(s) and the copyright owner(s) are credited and that the original publication in this journal is cited, in accordance with accepted academic practice. No use, distribution or reproduction is permitted which does not comply with these terms.
*Correspondence: Daniel Kaufman, ZGthdWZtYW5AbWVkbmV0LnVjbGEuZWR1; Jide Tian, anRpYW5AbWVkbmV0LnVjbGEuZWR1