- 1Norwich Medical School, University of East Anglia, Norwich Research Park, Norwich, United Kingdom
- 2Department of Haematology, Norfolk and Norwich University Hospitals NHS Trust, Norwich, United Kingdom
- 3Earlham Institute, Norwich Research Park, Norwich, United Kingdom
Normal bone marrow (BM) homeostasis ensures consistent production of progenitor cells and mature blood cells. This requires a reliable supply of nutrients in particular free fatty acids, carbohydrates and protein. Furthermore, rapid changes can occur in response to stress such as infection which can alter the demand for each of these metabolites. In response to infection the haematopoietic stem cells (HSCs) must respond and expand rapidly to facilitate the process of emergency granulopoiesis required for the immediate immune response. This involves a shift from the use of glycolysis to oxidative phosphorylation for energy production and therefore an increased demand for metabolites. Thus, the right balance of each dietary component helps to maintain not only normal homeostasis but also the ability to quickly respond to systemic stress. In addition, some dietary components can drive chronic inflammatory changes in the absence of infection or immune stress, which in turn can impact on overall immune function. The optimal nutrition for the best immunological outcomes would therefore be a diet that supports the functions of immune cells allowing them to initiate effective responses against pathogens but also to resolve the response rapidly when necessary and to avoid any underlying chronic inflammation. In this review we discuss how these key dietary components can alter immune function, what is their impact on bone marrow metabolism and how changes in dietary intake of each of these can improve the outcomes of infections.
Introduction
Haematopoiesis is a tightly controlled process that occurs in the specialized bone marrow (BM) niche, consisting of both haematopoietic cells and numerous support cells, including BM stromal cells, adipocytes, endothelial cells, osteocytes and osteoblasts (1). Not only does the environment support the maintenance of self-renewing haematopoietic stem cells (HSCs) and the production of mature haematopoietic cells during normal haemostasis, but it is also required to rapidly adapt and respond to local and systemic stressors. This includes the rapid expansion of HSCs and progenitor cells and increased production and mobilization of mature immune cells in response to infection (2, 3). Bacterial infections, for example, trigger the release of granulocyte stimulating factor (G-CSF) from endothelial cells to drive emergency granulopoiesis in order to meet the increased demand for neutrophils (4, 5). In addition some bacterial infections drive production of monocyte chemoattractant protein 1 (MCP-1), a chemokine that activates the CCR2 receptor on monocytes and has been shown to be essential for the mobilization of monocytes out of the BM (6). Other chemokines and cytokines such as Interleukin 6 (IL-6) and interferon gamma (IFN-γ) have also been implicated in driving the myeloid expansion in response to infections (7, 8). Together, emergency myelopoiesis results in a neutrophilia, with release of immature (left shifted) neutrophils and a monocytosis in the peripheral blood (9). This forms part of the immediate immune response to an acute infection and helps to compensate for the increased consumption of myeloid cells at the site of infection. The BM response to viral infections is less well understood and may in some ways be more complex (10). Lymphocytes, particularly T cells and natural killer cells are often key to the clearance of viral infections and for many an adaptive immune response is required for complete clearance of the virus (11). Furthermore, viral infections can have more long-term consequences for BM cell populations and HSC health (12) and have been implicated in causing cytopenias, aplastic anaemia as well as being associated with lymphoid BM malignancies (13–15).
In order to facilitate this rapid and efficient expansion of mature immune cells, HSC and progenitor cells require adequate metabolic supplies and are able to utilise different pathways to maximise their energy production. The HSC niche is a hypoxic environment and in the steady state HSCs primarily rely on glycolysis (16). However, in response to stress they are able to rapidly switch to oxidative phosphorylation (2, 17). This change in energy production is partially driven by their individual requirements but also affected by the energy sources available to the cells. Here we will discuss how alterations in diet influence the metabolic processes involved in the BM response to stress. Here we will focus on the macronutrients including carbohydrates, fatty acids and protein as these have been extensively studied.
Dietary components and their impact on immune function
Fatty acids
Fatty acids can be classified by their structure (saturated or unsaturated) or length (short-chain, medium-chain and long-chain). The different groups of fatty acids vary in their roles and functions in different organs and consequently can influence the immune system in different ways. For example imbalance between saturated and unsaturated fatty acids has been shown to contribute to the aetiology of allergic, autoimmune and metabolic diseases (18–20). Western diets are rich in sugar, trans and saturated fats, but low in complex carbohydrates, fiber, micronutrients, polyphenols and omega 3 polyunsaturated fatty acids (PUFA’s). This diet has been linked to an increased uptake of LPS (lipopolysaccharide) from microbes in the gut due to increased gut leakiness (21). LPS stimulates innate immune cells and activates them via Toll-like receptor (TLR) 4) contributing to chronic inflammation manifested by elevated serum levels of proinflammatory cytokines (e.g. IL-1, IL-6, IL-8, IL-12, and TNFα), chemokines (e.g. MCP-1, RANTES, and MIP-1) and acute phase reactants (e.g. C-reactive protein, serum amyloid A, and ferritin) (22, 23). On the contrary, omega 3 and PUFAs can interfere with TLR4 activation through inhibition of COX-2 mediated prostaglandin release and thus ameliorate this inflammatory signal (24, 25).
Furthermore, it has been shown that n=3 PUFA’s can increase the phagocytic activity and inhibit apoptosis in alveolar macrophages (26, 27).
PUFA’s also have an inhibitory effect on the pro-inflammatory phenotype of dendritic cells (DC) and DC-mediated T cell responses (28, 29). In contrast, saturated fatty acids increase DC maturation, activation and T-cell stimulation properties (30). Thus, whilst common fat components of the typical western diet can drive an unwanted chronic inflammatory process, other fatty acids play a key role in regulating the immune response and reducing tissue inflammation (Figure 1).
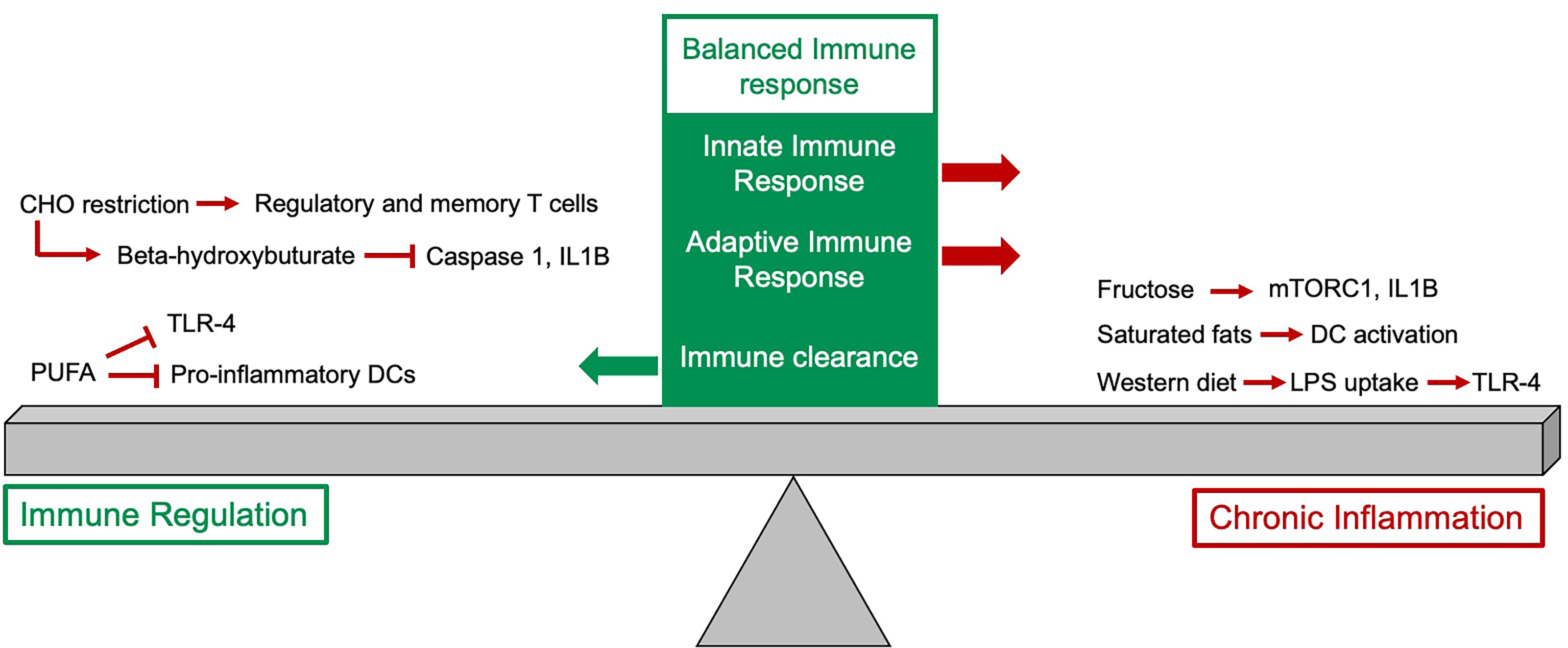
Figure 1 The impact of diet on the immune response. The balanced immune response is dependent on expansion of innate and adaptive immune cells as well as an appropriate downregulation of the response. Some dietary components, particularly fructose, saturated fats and the typical western diet have pro-inflammatory effects and if these persist this can drive chronic inflammation. Other dietary changes, such as reduction in carbohydrate (CHO) intake and increased intake of polyunsaturated fatty acids can help to downregulate the immune response.
Carbohydrates
Activation of the innate immune system requires metabolic rewiring primarily to favor oxidative glucose metabolism to activate macrophages and propagate their adhesion (31–34). In this scenario glucose is the major source of carbon, however increased blood levels of fructose present in Western diets (35) metabolically rewire innate immunity. In the recent study monocytes and macrophages exposed to fructose (main component of broadly used corn syrup) have shown increased activity of mTORC1 and expression of IL1B in response to LPS. Furthermore, these cells have reduced metabolic flexibility in response to glycolytic and mitochondrial inhibition, underpinning the pro-inflammatory role of dietary fructose (36).
B and T cells constitute major pillars of adaptive immunity. It has been shown that glucose is a major driver of B cell development and function.Increasing glucose levels drive expansion of splenic and mesenteric lymph node and Peyer’s patch B cells, which was also linked with enhanced B-lymphopoiesis in the bone marrow. Importantly, these B cells had increased immunoglobulin-G production after immunization and reduced apoptosis levels in early development via mTOR activation (37). Interestingly, human breast milk contains 7% carbohydrates (87% water, 1% protein, 4% lipid), which therefore forms the biggest nutrient resource for breast milk fed infants (38). As high carbohydrate intake has been shown to drive B cell development it is possible that this is the driver for B cell HSC skewing in infants (39). On the other hand, restriction of carbohydrate intake leads to production of ketone bodies such as beta-hydroxybutyrate (BHB), which can be utilized via mitochondrial oxidative phosphorylation (40). In animal models, BHB has been shown to attenuate caspase-1 activation and IL1B production by inhibiting NLRP3 inflammasome in phagocytes thus ameliorating low-level inflammation and associated autoinflammatory diseases (41–43). Interestingly, in human volunteers on a very-low-carbohydrate diet CD4+, CD8+ and regulatory T-cell capacity was markedly enhanced and T memory cell formation was increased. Molecular analysis of these cell subsets revealed an immunometabolic reprogramming in response to ketones favoring oxidative metabolism and conferring superior cellular energy supply and reactive oxygen species (ROS) signaling (44). The intake and availability of carbohydrates can therefore not only alter the inflammatory profile associated with both the innate and adaptive immune response but also the balance between different leukocyte populations produced and how they function (Figure 1).
Protein/amino acids
Activation of immune cells relies on specific amino acids, which are signaling molecules or provide metabolites (for TCA) and are not only involved in protein synthesis.Activated T cells upregulate amino acid transporter expression (45–47) required for rapid proliferation, translation of critical cytokines and adhesion molecules. CD4 and CD8+ T cells have similar repertoire of nutrient transporters, however differ in their expression level, where CD4+ have less copies of them which can underlie lower proliferative capacity compared to CD8+ T cells (47, 48). In addition innate immune cells, particularly activated macrophages, heavily depend on arginine uptake via CAT2 (49), while resting macrophages likely rely on CAT1 (50) and glutamine (51). LPS increases Slc7a5 transporter expression and leucine uptake to support proinflammatory macrophage cytokine production (52). NK cell activation depends on glutamine acquisition (53), while glutamine metabolism supports antibody production by activated B cells (54).
Glutathione (GSH) is a small molecule composed of glycine, glutamate and cysteine and is detrimental for quenching ROS upon T cell activation (55) or IL1B mRNA synthesis in macrophages stimulated with LPS (56). Branched amino acids (BCAAs)-leucine, isoleucine and valine provide coenzyme A (CoA) derivatives, which support metabolic reprogramming of immune cells (57), activate mTORC1 pathway (58) and epigenetic modification (acetylation) in macrophages or CD8+ T cells (58–60). BCAA by stimulating glucose uptake can promote glycolysis (61, 62).
Dietary components and their impact on BM metabolism
Fatty acids
The BM has vast stores of adipocytes and the proximity of these to the HSC niche is important as adipocytes store and provide free fatty acids (FFA), a crucial metabolite for HSCs and progenitor cells. Fatty acid oxidation has been shown to be essential for healthy HSC maintenance and implicated in self-renewal divisions (63). In response to stress fatty acid oxidation provides further substrates, in the form of acetyl coenzyme A, for the TCA cycle to help facilitate the rapid switch of HSCs from glycolysis to oxidative phosphorylation in response to infection (17). Thus, an adequate and reliable supply of FFA and therefore adipose tissue is required within the BM niche to drive healthy haematopoiesis and support the BM response to infection (Figure 2A). However, the adipocyte rich yellow bone marrow expands significantly both in obesity and with increased age (64). This accumulation of adipocytes can disrupt the normal haematopoietic processes and therefore directly impact on the production of mature blood cells in the steady state and the response to infection (65, 66). Increased adipocyte frequency in the BM metabolically reprograms megakaryocytes by active transfer of fatty acids through CD36 and shifts their ploidy towards 32 and 64N (67) and potentially has an impact on platelet activation status (68). Furthermore, obesity does not only drive local changes within the BM microenvironment but the abundance of adipose tissue in other organs have systemic implications, which in turn can alter immune function and BM health. Obesity has been associated with systemic chronic low-level inflammation (69), at least partially mediated by chronic activation of toll TLR 4 (20, 70). This is driven by the secretion of pro-inflammatory cytokines, adipokines and leptins and is known to contribute to many obesity-associated diseases (65, 71). The consequences of this on the BM microenvironment and immune function include increases in haematopoiesis affecting both myeloid and lymphoid lineages (65, 69), and changes in the innate and adaptive immune responses, with disrupted immediate responses observed in the BM (70) as well as impaired memory T-cells responses (72). Together these changes contribute to the increased morbidity and mortality known to be associated with infections in obese people (73, 74). The mechanisms behind these changes are likely multifactorial with both local and systemic factors manipulating the HSC niche, and therefore influencing HSC maintenance, output, and immune overall function. It is possible that the increase in BM cellularity and haematopoiesis reflects an attempt to compensate for the reduced function of immune cells in the obesity-associated environment. A clearer understanding of how the obesity associated pro-inflammatory environment disrupts normal haematopoiesis and the immune response to infection will help inform future treatments of obese patients to promote a better and regulated response to infection.
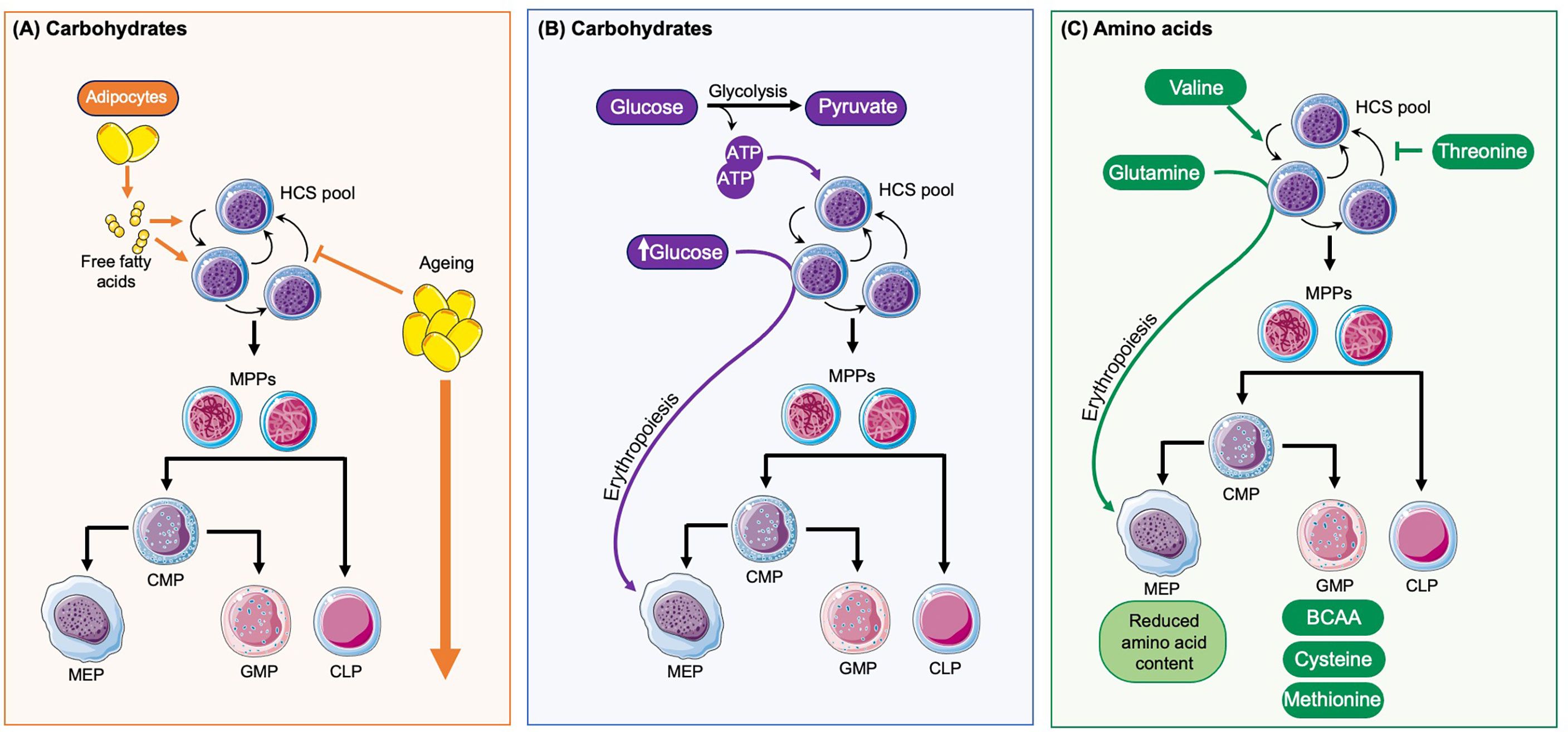
Figure 2 How diet influences haematopoesis. (A) Adipocytes are found in close proximity to HSCs and provide free fatty acids, which play a role in HSC maintenance and self-renewal and are an important energy source for HSC expansion. With ageing there is an accumulation of adipocytes which disrupt the normal regulation of haematopoiesis and overall drives the expansion of HSCs and progenitor cells. (B) In the steady state HSCs primarily rely on glycolysis for the production of ATP and normal haematopoietic maintenance. High levels of glucose also enhance HSC commitment towards the erythroid lineage. (C) The amino acid valine has been shown to be vital for HSC maintenance and self-renewal whilst threonine can disrupt this. Glutamine promotes erythropoiesis. The amino acids cell content varies between different progenitors with MEPs having reduced levels and GMPs the highest levels, particularly of branched amino acids (BRAA), cysteine and methionine.
Carbohydrates
Dormant HSCs are thought to have low energy consumption when compared to actively dividing cells (75, 76). They reside in the hypoxic BM niche, which is thought to limit oxidative respiration and favor glycolysis (Figure 2B) (77). This helps to reduce ROS levels in quiescent HSCs and promotes long-term HSC health. High availability of glucose has been shown to stimulate nucleotide biosynthesis and enhances HSC commitment to erythroid lineage (78). Fasting can exhibit a positive effect on regenerative potential of HSC via inhibition of insulin growth factor 1 (IGF1) signaling and decreased their mobilization potential in patients with diabetes type 2 (79).
Amino acids
Although HSCs have low rates of protein translation suggesting lower amino acid requirement (80), a recent study found that in vivo HSC maintenance depends on valine (Figure 2C). Dietary depletion of this amino acid in recipient mice for 2 weeks permitted BM transplantation and donor HSC engraftment without irradiation conditioning (81). For ex vivo HSC maintenance depletion of threonine (or downstream metabolites) had a positive effect. So far the metabolic function of valine and threonine for HSC maintenance are yet to be determined. Furthermore, HSC commitment to erythroid lineage has been attributed to increased glutamine levels (78). mTor complex 1 (mTORC1) is a central node in metabolic sensing and signaling (82) including essential amino acid leucine, which in turn regulates its activity through GTPase RagA (83). In a recent study RagA was found to be dispensable for HSC function (84), suggesting HSC are resilient to deprivation of certain nutrients.
A comprehensive proteomics study of BM progenitor - lineage- cKit+Sca1+ stem and progenitor cells (LSK), common myeloid progenitors (CMP), granulocytic and monocytic progenitors (GMP) and myeloerythroid progenitors (MEP) - showed differential content of amino acids, with lowest levels detected in MEPs and highest in GMP (in particular BRAA, cysteine, methionine), suggesting their highly proliferative nature compared to other analyzed cell types. On the contrary the global arginine bioavailability ratio (GABR), defined as the ratio between arginine and its major metabolites (ornithine and citrulline), was higher in the LSK and GMP populations. Ex vivo cultures of LKS supplemented with arginine and citrulline were able to expand the proportion of TMRM low cells (85). These metabolites may have a potential to improve in vivo HSC function (86, 87). Thus, different amino acids are key to the normal function, maintenance and homeostasis of HSCs and all progenitor cell populations.
Modulating diet to improve outcome of infection
Both steady state and stress hematopoiesis are dependent on a reliable and easily scalable energy supply. In order to support their varying energy demands, HSCs and progenitor cells are able to adapt and draw upon multiple different metabolites to generate ATP, thus not relying on one sole source of energy within the BM niche.This also means that the source of energy and therefore individual macronutrient dietary components may not necessarily have a direct impact on immune function. For example, it has long been believed that high fat diet content drives not only obesity but also all its associated diseases such as diabetes and heart disease, however more recently this concept has been contested. Studies have demonstrated that high fat diets can be associated with health benefits, these include anti-inflammatory and immune-supportive changes (88, 89). Debates may continue over which macronutrient is superior and drives better health outcomes, however, it is becoming increasingly apparent that a balance of each dietary component is required and that it is not the relative proportions of fat and carbohydrates that drive obesity, disease and immune dysregulation but any tendency to over-eating and excessive calorie intake (90).
In addition to carbohydrates and fat content, there are of course other essential dietary components required for a healthy immune response. These include amino acids, which play a vital role in HSC maintenance and differentiation (78, 85) as well as presenting a further metabolite for oxidative phosphorylation both during emergency haematopoiesis (91) and normal immune cell maintenance (92). For example, glutamine, the most abundant and versatile amino acid, can be used as a substrate for numerous pathways including nucleotide and NADPH synthesis (93) and has also been shown to regulate HSC differentiation (32). During infection or times of stress demand for amino acids increases and inadequate amino acid stores or complete depletion of existing stores, due to longer periods of increased consumption, have been associated with adverse outcomes (36). In contrast to this however, it is becoming increasingly apparent that a low protein diet can have a beneficial impact on the immune response (94) and it is therefore clear that a fine balance is needed to ensure sufficient stores are present to be used when required but any excess is limited to promote long term BM health.
Another factor to consider in the context of diet and the immune response is the effect of the microbiome on haematopoeisis and immune cell production, Previous work has suggested that the absence of commensal microbes can result in defective immune function. This can drive both failure of appropriate immune responses and immune suppression as well as autoimmune conditions (95, 96). Furthermore, there are numerous micronutrients which are key to a healthy immune response and appropriate immune regulation by providing building blocks, modulating cytokine expression and regulating immune cell activity. These include vitamins, some key micronutrients such as vitamins (97, 98), polyphenols, which have been shown to impact on immune cells function and alter expression of pro-inflammatory cytokines (99), and minerals, in particular zinc, where even minor deficiencies result in impaired immune responses which has been shown to be vital for both innate and adaptive immune cell function (100, 101).
An adequate supply of each of the major dietary components, including fat, carbohydrate, and protein, as well as the numerous micronutrients, is required to achieve a sufficient immediate immune response, mount an adaptive immune response with memory components and downregulate or resolve the immune response at the appropriate time to prevent chronic inflammation. In the absence of this the finely regulated BM microenvironment and its ability to mount an effective immune response is disrupted and this will only impact on how the body can respond to an acute infection but will also have long term consequences, with impaired long-term adaptive immunity as well as potentially prolonged periods of inflammation. Deficiencies in the required nutrients can result from any cause of malnutrition, however, when considering the effect of diet on the immune system it is particularly important to consider the aging population. Not only do dietary deficiencies increase with advanced age (102) but it is also known that immune function declines with age (103). It is therefore important to ensure adequate intake of all dietary components for older patients and to consider supplementation of some vital vitamins or minerals, as these have been shown to reduce infections, support the immune response and improve outcomes (104).
Conclusion
Any immune response is delicately orchestrated to drive the immediate innate reaction, the long-term adaptive response but eventually also to downregulate and control the inflammatory process. This fine balance is controlled by the BM, the BM niche and the circulating mature immune cells. Any disruption of this process will either result in an ineffective immune response, impaired future immune surveillance or state of chronic inflammation which in turn can lead to a number of pathologies. In this review we have demonstrated how different dietary components can impact on each step involved in the immune process. Dietary components are not only important metabolites required for an effective immune response but they also have a number of regulatory functions and therefore can have a much broader systemic impact, which in turn can affect immune function. Nutrition has the potential to effectively treat immune deficiencies related to poor intake, there is a great interest in whether specific dietary interventions can further enhance immune function in sub-clinical situations, and thus prevent the onset of infections or chronic inflammatory diseases.
Authors contributions
CH and EW conceptualized and wrote the paper. All authors contributed to the article and approved the submitted version.
Funding
CH is funded by Wellcome Trust Clinical Research Fellowship (220534/Z/20/Z) and was supported by the NNUH charitable fund. EW is funded by a Sir Henry Welcome Postdoctoral Fellowship (213731/Z/18/Z).
Acknowledgments
The Figures were partly generated using Servier Medical Art, provided by Servier, licensed under a Creative Commons Attribution 3.0 unported license.
Conflict of interest
The authors declare that the research was conducted in the absence of any commercial or financial relationships that could be construed as a potential conflict of interest.
Publisher’s note
All claims expressed in this article are solely those of the authors and do not necessarily represent those of their affiliated organizations, or those of the publisher, the editors and the reviewers. Any product that may be evaluated in this article, or claim that may be made by its manufacturer, is not guaranteed or endorsed by the publisher.
References
1. Scadden DT. Nice neighborhood: emerging concepts of the stem cell niche. Cell (2014) 157:41–50. doi: 10.1016/j.cell.2014.02.013
2. Mistry JJ, Marlein CR, Moore JA, Hellmich C, Wojtowicz EE, Smith JGW, et al. ROS-mediated PI3K activation drives mitochondrial transfer from stromal cells to hematopoietic stem cells in response to infection. Proc Natl Acad Sci U.S.A. (2019) 116:24610–9. doi: 10.1073/pnas.1913278116
3. Zaretsky AG, Engiles JB, Hunter CA. Infection-induced changes in hematopoiesis. J Immunol (2014) 192:27–33. doi: 10.4049/jimmunol.1302061
4. Lieschke, Grail, Hodgson, Metcalf, Stanley. Mice lacking G-CSF have chronic neutropenia, granulocyte and macrophage progenitor cell deficiency and impaired neutrophil mobilization. Blood (1994) 84(6):1737–46.
5. Boettcher S, Gerosa RC, Radpour R, Bauer J, Ampenberger F, Heikenwalder M, et al. Endothelial cells translate pathogen signals into G-CSF-driven emergency granulopoiesis. Blood (2014) 124:1393–403. doi: 10.1182/blood-2014-04-570762
6. Serbina NV, Pamer EG. Monocyte emigration from bone marrow during bacterial infection requires signals mediated by chemokine receptor CCR2. Nat Immunol (2006) 7:311–7. doi: 10.1038/ni1309
7. MacNamara KC, Jones M, Martin O, Winslow GM. Transient activation of hematopoietic stem and progenitor cells by IFNγ during acute bacterial infection. PloS One (2011) 6:e28669. doi: 10.1371/journal.pone.0028669
8. Chou DB, Sworder B, Bouladoux N, Roy CN, Uchida AM, Grigg M, et al. Stromal-derived IL-6 alters the balance of myeloerythroid progenitors during toxoplasma gondii infection. J Leukoc Biol (2012) 92:123–31. doi: 10.1189/jlb.1011527
9. Takizawa H, Boettcher S, Manz MG. Demand-adapted regulation of early hematopoiesis in infection and inflammation. Blood (2012) 119:2991–3002. doi: 10.1182/blood-2011-12-380113
10. Pascutti MF, Erkelens MN, Nolte MA. Impact of viral infections on hematopoiesis: From beneficial to detrimental effects on bone marrow output. Front Immunol (2016) 7:364. doi: 10.3389/fimmu.2016.00364
11. Braciale TJ, Sun J, Kim TS. Regulating the adaptive immune response to respiratory virus infection. Nat Rev Immunol (2012) 12:295–305. doi: 10.1038/nri3166
12. Isringhausen S, Mun Y, Kovtonyuk L, Kräutler NJ, Suessbier U, Gomariz A, et al. Chronic viral infections persistently alter marrow stroma and impair hematopoietic stem cell fitness. J Exp Med (2021) 218. doi: 10.1084/jem.20192070
13. Rouse BT, Sehrawat S. Immunity and immunopathology to viruses: what decides the outcome? Nat Rev Immunol (2010) 10:514–26. doi: 10.1038/nri2802
14. Ok CY, Li L, Young KH. EBV-driven b-cell lymphoproliferative disorders: from biology, classification and differential diagnosis to clinical management. Exp Mol Med (2015) 47:e132. doi: 10.1038/emm.2014.82
15. Klco JM, Geng B, Brunt EM, Hassan A, Nguyen T-D, Kreisel FH, et al. Bone marrow biopsy in patients with hepatitis c virus infection: spectrum of findings and diagnostic utility. Am J Hematol (2010) 85:106–10. doi: 10.1002/ajh.21600
16. Simsek T, Kocabas F, Zheng J, Deberardinis RJ, Mahmoud AI, Olson EN, et al. The distinct metabolic profile of hematopoietic stem cells reflects their location in a hypoxic niche. Cell Stem Cell (2010) 7:380–90. doi: 10.1016/j.stem.2010.07.011
17. Mistry JJ, Hellmich C, Moore JA, Jibril A, Macaulay I, Moreno-Gonzalez M, et al. Free fatty-acid transport via CD36 drives β-oxidation-mediated hematopoietic stem cell response to infection. Nat Commun (2021) 12:7130. doi: 10.1038/s41467-021-27460-9
18. Zhao M, Chiriboga D, Olendzki B, Xie B, Li Y, McGonigal LJ, et al. Substantial increase in compliance with saturated fatty acid intake recommendations after one year following the American heart association diet. Nutrients (2018) 10. doi: 10.3390/nu10101486
19. Venter C, Meyer RW, Nwaru BI, Roduit C, Untersmayr E, Adel-Patient K, et al. EAACI position paper: Influence of dietary fatty acids on asthma, food allergy, and atopic dermatitis. Allergy (2019) 74:1429–444. doi: 10.1111/all.13764
20. Rogero MM, Calder PC. Obesity, inflammation, toll-like receptor 4 and fatty acids. Nutrients (2018) 10. doi: 10.3390/nu10040432
21. Hietbrink F, Besselink MGH, Renooij W, de Smet MBM, Draisma A, van der Hoeven H, et al. Systemic inflammation increases intestinal permeability during experimental human endotoxemia. Shock (2009) 32:374–8. doi: 10.1097/shk.0b013e3181a2bcd6
22. Olefsky JM, Glass CK. Macrophages, inflammation, and insulin resistance. Annu Rev Physiol (2010) 72:219–46. doi: 10.1146/annurev-physiol-021909-135846
23. Shoelson SE, Lee J, Goldfine AB. Inflammation and insulin resistance. J Clin Invest (2006) 116:1793–801. doi: 10.1172/JCI29069
24. Lee JY, Plakidas A, Lee WH, Heikkinen A, Chanmugam P, Bray G, et al. Differential modulation of toll-like receptors by fatty acids: preferential inhibition by n-3 polyunsaturated fatty acids. J Lipid Res (2003) 44:479–86. doi: 10.1194/jlr.M200361-JLR200
25. Honda KL, Lamon-Fava S, Matthan NR, Wu D, Lichtenstein AH. EPA And DHA exposure alters the inflammatory response but not the surface expression of toll-like receptor 4 in macrophages. Lipids (2015) 50:121–9. doi: 10.1007/s11745-014-3971-y
26. Adolph S, Fuhrmann H, Schumann J. Unsaturated fatty acids promote the phagocytosis of P. aeruginosa and R. equi by RAW264.7 macrophages. Curr Microbiol (2012) 65:649–55. doi: 10.1007/s00284-012-0207-3
27. Saini A, Harjai K, Chhibber S. Inhibitory effect of polyunsaturated fatty acids on apoptosis induced by streptococcus pneumoniae in alveolar macrophages. Indian J Med Res (2013) 137:1193–8.
28. Uchi S-H, Yanai R, Kobayashi M, Hatano M, Kobayashi Y, Yamashiro C, et al. Dendritic cells mediate the anti-inflammatory action of omega-3 long-chain polyunsaturated fatty acids in experimental autoimmune uveitis. PloS One (2019) 14:e0219405. doi: 10.1371/journal.pone.0219405
29. Teague H, Rockett BD, Harris M, Brown DA, Shaikh SR. Dendritic cell activation, phagocytosis and CD69 expression on cognate T cells are suppressed by n-3 long-chain polyunsaturated fatty acids. Immunology (2013) 139:386–94. doi: 10.1111/imm.12088
30. Nicholas DA, Zhang K, Hung C, Glasgow S, Aruni AW, Unternaehrer J, et al. Palmitic acid is a toll-like receptor 4 ligand that induces human dendritic cell secretion of IL-1β. PloS One (2017) 12:e0176793. doi: 10.1371/journal.pone.0176793
31. Diskin C, Pålsson-McDermott EM. Metabolic modulation in macrophage effector function. Front Immunol (2018) 9:270. doi: 10.3389/fimmu.2018.00270
32. Izquierdo E, Cuevas VD, Fernández-Arroyo S, Riera-Borrull M, Orta-Zavalza E, Joven J, et al. Reshaping of human macrophage polarization through modulation of glucose catabolic pathways. J Immunol (2015) 195:2442–51. doi: 10.4049/jimmunol.1403045
33. Raulien N, Friedrich K, Strobel S, Rubner S, Baumann S, von Bergen M, et al. Fatty acid oxidation compensates for lipopolysaccharide-induced warburg effect in glucose-deprived monocytes. Front Immunol (2017) 8:609. doi: 10.3389/fimmu.2017.00609
34. Lee MKS, Al-Sharea A, Shihata WA, Bertuzzo Veiga C, Cooney OD, Fleetwood AJ, et al. Glycolysis is required for LPS-induced activation and adhesion of human CD14+CD16- monocytes. Front Immunol (2019) 10:2054. doi: 10.3389/fimmu.2019.02054
35. Herman MA, Samuel VT. The sweet path to metabolic demise: Fructose and lipid synthesis. Trends Endocrinol Metab (2016) 27:719–30. doi: 10.1016/j.tem.2016.06.005
36. Jones N, Blagih J, Zani F, Rees A, Hill DG, Jenkins BJ, et al. Fructose reprogrammes glutamine-dependent oxidative metabolism to support LPS-induced inflammation. Nat Commun (2021) 12:1209. doi: 10.1038/s41467-021-21461-4
37. Tan J, Ni D, Wali JA, Cox DA, Pinget GV, Taitz J, et al. Dietary carbohydrate, particularly glucose, drives b cell lymphopoiesis and function. iScience (2021) 24:102835. doi: 10.1016/j.isci.2021.102835
38. Boquien C-Y. Human milk: An ideal food for nutrition of preterm newborn. Front Pediatr (2018) 6:295. doi: 10.3389/fped.2018.00295
39. Jackson TR, Ling RE, Roy A. The origin of b-cells: Human fetal b cell development and implications for the pathogenesis of childhood acute lymphoblastic leukemia. Front Immunol (2021) 12:637975. doi: 10.3389/fimmu.2021.637975
40. Puchalska P, Crawford PA. Multi-dimensional roles of ketone bodies in fuel metabolism, signaling, and therapeutics. Cell Metab (2017) 25:262–84. doi: 10.1016/j.cmet.2016.12.022
41. Youm Y-H, Nguyen KY, Grant RW, Goldberg EL, Bodogai M, Kim D, et al. The ketone metabolite β-hydroxybutyrate blocks NLRP3 inflammasome–mediated inflammatory disease. Nat Med (2015) 21:263–9. doi: 10.1038/nm.3804
42. Goldberg EL, Asher JL, Molony RD, Shaw AC, Zeiss CJ, Wang C, et al. β-hydroxybutyrate deactivates neutrophil NLRP3 inflammasome to relieve gout flares. Cell Rep (2017) 18:2077–87. doi: 10.1016/j.celrep.2017.02.004
43. Newman JC, Covarrubias AJ, Zhao M, Yu X, Gut P, Ng C-P, et al. Ketogenic diet reduces midlife mortality and improves memory in aging mice. Cell Metab (2017) 26:547–557.e8. doi: 10.1016/j.cmet.2017.08.004
44. Hirschberger S, Strauß G, Effinger D, Marstaller X, Ferstl A, Müller MB, et al. Very-low-carbohydrate diet enhances human T-cell immunity through immunometabolic reprogramming. EMBO Mol Med (2021) 13:e14323. doi: 10.15252/emmm.202114323
45. Geiger R, Rieckmann JC, Wolf T, Basso C, Feng Y, Fuhrer T, et al. L-arginine modulates T cell metabolism and enhances survival and anti-tumor activity. Cell (2016) 167:829–842.e13. doi: 10.1016/j.cell.2016.09.031
46. Ron-Harel N, Ghergurovich JM, Notarangelo G, LaFleur MW, Tsubosaka Y, Sharpe AH, et al. Haigis MC. T Cell Activation Depends Extracellular Alanine Cell Rep (2019) 28:3011–3021.e4. doi: 10.1016/j.celrep.2019.08.034
47. Sinclair LV, Howden AJM, Brenes A, Spinelli L, Hukelmann JL, Macintyre AN, et al. Antigen receptor control of methionine metabolism in T cells. eLife (2019) 8. doi: 10.7554/elife.44210
48. Swamy M, Pathak S, Grzes KM, Damerow S, Sinclair LV, van Aalten DMF, et al. Glucose and glutamine fuel protein O-GlcNAcylation to control T cell self-renewal and malignancy. Nat Immunol (2016) 17:712–20. doi: 10.1038/ni.3439
49. Yeramian A, Martin L, Serrat N, Arpa L, Soler C, Bertran J, et al. Arginine transport via cationic amino acid transporter 2 plays a critical regulatory role in classical or alternative activation of macrophages. J Immunol (2006) 176:5918–24. doi: 10.4049/jimmunol.176.10.5918
50. Yeramian A, Martin L, Arpa L, Bertran J, Soler C, McLeod C, et al. Macrophages require distinct arginine catabolism and transport systems for proliferation and for activation. Eur J Immunol (2006) 36:1516–26. doi: 10.1002/eji.200535694
51. Carr EL, Kelman A, Wu GS, Gopaul R, Senkevitch E, Aghvanyan A, et al. Glutamine uptake and metabolism are coordinately regulated by ERK/MAPK during T lymphocyte activation. J Immunol (2010) 185:1037–44. doi: 10.4049/jimmunol.0903586
52. Yoon BR, Oh Y-J, Kang SW, Lee EB, Lee W-W. Role of SLC7A5 in metabolic reprogramming of human Monocyte/Macrophage immune responses. Front Immunol (2018) 9:53. doi: 10.3389/fimmu.2018.00053
53. Loftus RM, Assmann N, Kedia-Mehta N, O’Brien KL, Garcia A, Gillespie C, et al. Amino acid-dependent cMyc expression is essential for NK cell metabolic and functional responses in mice. Nat Commun (2018) 9:2341. doi: 10.1038/s41467-018-04719-2
54. Jiang S, Yan W, Wang SE, Baltimore D. Let-7 suppresses b cell activation through restricting the availability of necessary nutrients. Cell Metab (2018) 27:393–403.e4. doi: 10.1016/j.cmet.2017.12.007
55. Mak TW, Grusdat M, Duncan GS, Dostert C, Nonnenmacher Y, Cox M, et al. Glutathione primes T cell metabolism for inflammation. Immunity (2017) 46:1089–90. doi: 10.1016/j.immuni.2017.06.009
56. Rodriguez AE, Ducker GS, Billingham LK, Martinez CA, Mainolfi N, Suri V, et al. Serine metabolism supports macrophage IL-1β production. Cell Metab (2019) 29:1003–1011.e4. doi: 10.1016/j.cmet.2019.01.014
57. Sinclair LV, Rolf J, Emslie E, Shi Y-B, Taylor PM, Cantrell DA. Control of amino-acid transport by antigen receptors coordinates the metabolic reprogramming essential for T cell differentiation. Nat Immunol (2013) 14:500–8. doi: 10.1038/ni.2556
58. Son SM, Park SJ, Lee H, Siddiqi F, Lee JE, Menzies FM, et al. Leucine signals to mTORC1 via its metabolite acetyl-coenzyme a. Cell Metab (2019) 29:192–201.e7. doi: 10.1016/j.cmet.2018.08.013
59. Lugrin J, Le Roy D, Goy G, Mombelli M. Histone deacetylase inhibitors impair innate immune responses to toll-like receptor agonists and to infection. Blood J (2011) 117(4):1205–17. doi: 10.1182/blood-2010-05-284711
60. Balmer ML, Ma EH, Bantug GR, Grählert J, Pfister S, Glatter T, et al. Memory CD8+ T cells require increased concentrations of acetate induced by stress for optimal function. Immunity (2016) 44:1312–24. doi: 10.1016/j.immuni.2016.03.016
61. Doi M, Yamaoka I, Nakayama M, Mochizuki S, Sugahara K, Yoshizawa F. Isoleucine, a blood glucose-lowering amino acid, increases glucose uptake in rat skeletal muscle in the absence of increases in AMP-activated protein kinase activity. J Nutr (2005) 135:2103–8. doi: 10.1093/jn/135.9.2103
62. Nishitani S, Takehana K, Fujitani S, Sonaka I. Branched-chain amino acids improve glucose metabolism in rats with liver cirrhosis. Am J Physiol Gastrointest Liver Physiol (2005) 288:G1292–300. doi: 10.1152/ajpgi.00510.2003
63. Ito K, Carracedo A, Arai F, Ala U, Avigan D, Schafer Z, et al. A PML–PPAR-δ pathway for fatty acid oxidation regulates hematopoietic stem cell maintenance through the control of asymmetric division. Blood (2012) 120:2327–7. doi: 10.1182/blood.v120.21.2327.2327
64. Ambrosi TH, Scialdone A, Graja A, Gohlke S, Jank A-M, Bocian C, et al. Adipocyte accumulation in the bone marrow during obesity and aging impairs stem cell-based hematopoietic and bone regeneration. Cell Stem Cell (2017) 20:771–784.e6. doi: 10.1016/j.stem.2017.02.009
65. Trottier MD, Naaz A, Li Y, Fraker PJ. Enhancement of hematopoiesis and lymphopoiesis in diet-induced obese mice. Proc Natl Acad Sci U.S.A. (2012) 109:7622–9. doi: 10.1073/pnas.1205129109
66. Benova A, Tencerova M. Obesity-induced changes in bone marrow homeostasis. Front Endocrinol (2020) 11:294. doi: 10.3389/fendo.2020.00294
67. Valet C, Batut A, Vauclard A, Dortignac A, Bellio M, Payrastre B, et al. Adipocyte fatty acid transfer supports megakaryocyte maturation. Cell Rep (2020) 32:107875. doi: 10.1016/j.celrep.2020.107875
68. Gaspar RS, Unsworth AJ, Al-Dibouni A, Bye AP, Sage T, Stewart M, et al. Maternal and offspring high-fat diet leads to platelet hyperactivation in male mice offspring. Sci Rep (2021) 11. doi: 10.1038/s41598-020-80373-3
69. Singer K, DelProposto J, Morris DL, Zamarron B, Mergian T, Maley N, et al. Diet-induced obesity promotes myelopoiesis in hematopoietic stem cells. Mol Metab (2014) 3:664–75. doi: 10.1016/j.molmet.2014.06.005
70. Liu A, Chen M, Kumar R, Stefanovic-Racic M, O’Doherty RM, Ding Y, et al. Bone marrow lympho-myeloid malfunction in obesity requires precursor cell-autonomous TLR4. Nat Commun (2018) 9:708. doi: 10.1038/s41467-018-03145-8
71. Hotamisligil GS, Shargill NS, Spiegelman BM. Adipose expression of tumor necrosis factor-alpha: direct role in obesity-linked insulin resistance. Science (1993) 259:87–91. doi: 10.1126/science.7678183
72. Karlsson EA, Sheridan PA, Beck MA. Diet-induced obesity impairs the T cell memory response to influenza virus infection. J Immunol (2010) 184:3127–33. doi: 10.4049/jimmunol.0903220
73. Pugliese G, Liccardi A, Graziadio C, Barrea L, Muscogiuri G, Colao A. Obesity and infectious diseases: pathophysiology and epidemiology of a double pandemic condition. Int J Obes (2022) 46:449–65. doi: 10.1038/s41366-021-01035-6
74. Smith AG, Sheridan PA, Harp JB, Beck MA. Diet-induced obese mice have increased mortality and altered immune responses when infected with influenza virus. J Nutr (2007) 137:1236–43. doi: 10.1093/jn/137.5.1236
75. Karigane D, Takubo K. Metabolic regulation of hematopoietic and leukemic stem/progenitor cells under homeostatic and stress conditions. Int J Hematol (2017) 106:18–26. doi: 10.1007/s12185-017-2261-x
76. Suda T, Takubo K, Semenza GL. Metabolic regulation of hematopoietic stem cells in the hypoxic niche. Cell Stem Cell (2011) 9:298–310. doi: 10.1016/j.stem.2011.09.010
77. Takubo K, Goda N, Yamada W, Iriuchishima H, Ikeda E, Kubota Y, et al. Regulation of the HIF-1α level is essential for hematopoietic stem cells. Cell Stem Cell (2010) 7:391–402. doi: 10.1016/j.stem.2010.06.020
78. Oburoglu L, Tardito S, Fritz V, de Barros SC, Merida P, Craveiro M, et al. Glucose and glutamine metabolism regulate human hematopoietic stem cell lineage specification. Cell Stem Cell (2014) 15:169–84. doi: 10.1016/j.stem.2014.06.002
79. Ferraro F, Lymperi S, Méndez-Ferrer S, Saez B, Spencer JA, Yeap BY, et al. Diabetes impairs hematopoietic stem cell mobilization by altering niche function. Sci Transl Med (2011) 3:104ra101. doi: 10.1126/scitranslmed.3002191
80. Signer RAJ, Magee JA, Salic A, Morrison SJ. Haematopoietic stem cells require a highly regulated protein synthesis rate. Nature (2014) 509:49–54. doi: 10.1038/nature13035
81. Taya Y, Ota Y, Wilkinson AC, Kanazawa A, Watarai H, Kasai M, et al. Depleting dietary valine permits nonmyeloablative mouse hematopoietic stem cell transplantation. Science (2016) 354:1152–5. doi: 10.1126/science.aag3145
82. Efeyan A, Comb WC, Sabatini DM. Nutrient-sensing mechanisms and pathways. Nature (2015) 517:302–10. doi: 10.1038/nature14190
83. Saxton RA, Sabatini DM. mTOR signaling in growth, metabolism, and disease. Cell (2017) 169:361–71. doi: 10.1016/j.cell.2017.03.035
84. Kalaitzidis D, Lee D, Efeyan A, Kfoury Y, Nayyar N, Sykes DB, et al. Amino acid–insensitive mTORC1 regulation enables nutritional stress resilience in hematopoietic stem cells. J Clin Invest (2017) 127(4):1405–13. doi: 10.1172/JCI89452
85. Girotra M, Monnard C, Konz T, Sizzano F, Goulet L, Godin J-P, et al. Mineral and amino acid profiling of different hematopoietic populations from the mouse bone marrow. Int J Mol Sci (2020) 21. doi: 10.3390/ijms21176444
86. Vannini N, Girotra M, Naveiras O, Nikitin G, Campos V, Giger S, et al. Specification of haematopoietic stem cell fate via modulation of mitochondrial activity. Nat Commun (2016) 7:13125. doi: 10.1038/ncomms13125
87. Vannini N, Campos V, Girotra M, Trachsel V, Rojas-Sutterlin S, Tratwal J, et al. The NAD-booster nicotinamide riboside potently stimulates hematopoiesis through increased mitochondrial clearance. Cell Stem Cell (2019) 24:405–418.e7. doi: 10.1016/j.stem.2019.02.012
88. Lundsgaard A-M, Holm JB, Sjøberg KA, Bojsen-Møller KN, Myrmel LS, Fjære E, et al. Mechanisms preserving insulin action during high dietary fat intake. Cell Metab (2019) 29:229. doi: 10.1016/j.cmet.2018.10.002
89. Dehghan M, Mente A, Zhang X, Swaminathan S, Li W, Mohan V, et al. Associations of fats and carbohydrate intake with cardiovascular disease and mortality in 18 countries from five continents (PURE): a prospective cohort study. Lancet (2017) 390:2050–62. doi: 10.1016/S0140-6736(17)32252-3
90. Wali JA, Jarzebska N, Raubenheimer D, Simpson SJ, Rodionov RN, O’Sullivan JF. Cardio-metabolic effects of high-fat diets and their underlying mechanisms-a narrative review. Nutrients (2020) 12. doi: 10.3390/nu12051505
91. Martínez-Reyes I, Chandel NS. Mitochondrial TCA cycle metabolites control physiology and disease. Nat Commun (2020) 11:102. doi: 10.1038/s41467-019-13668-3
92. Mills EL, Kelly B, O’Neill LAJ. Mitochondria are the powerhouses of immunity. Nat Immunol (2017) 18:488–98. doi: 10.1038/ni.3704
94. Buettner D, Skemp S. Blue zones: Lessons from the world’s longest lived. Am J Lifestyle Med (2016) 10:318–21. doi: 10.1177/1559827616637066
95. Zheng D, Liwinski T, Elinav E. Interaction between microbiota and immunity in health and disease. Cell Res (2020) 30:492–506. doi: 10.1038/s41422-020-0332-7
96. Belkaid Y, Hand TW. Role of the microbiota in immunity and inflammation. Cell (2014) 157:121–41. doi: 10.1016/j.cell.2014.03.011
98. Chaari A, Bendriss G, Zakaria D, McVeigh C. Importance of dietary changes during the coronavirus pandemic: How to upgrade your immune response. Front Public Health (2020) 8:476. doi: 10.3389/fpubh.2020.00476
99. Watson RR, Preedy VR, Zibadi S. Polyphenols in human health and disease. Academic Press (2013). 1488 p.
100. Erkekoglu P, Kocer-Gumusel B. Nutritional deficiency. BoD – Books on Demand. InTech (2016). 206 p.
101. Shankar AH, Prasad AS. Zinc and immune function: the biological basis of altered resistance to infection. Am J Clin Nutr (1998) 68:447S–63S. doi: 10.1093/ajcn/68.2.447S
102. Woods JL, Iuliano-Burns S, Walker KZ. Immunological and nutritional factors in elderly people in low-level care and their association with mortality. Immun Ageing (2013) 10:32. doi: 10.1186/1742-4933-10-32
103. Foreman KJ, Marquez N, Dolgert A, Fukutaki K, Fullman N, McGaughey M, et al. Forecasting life expectancy, years of life lost, and all-cause and cause-specific mortality for 250 causes of death: reference and alternative scenarios for 2016-40 for 195 countries and territories. Lancet (2018) 392:2052–90. doi: 10.1016/S0140-6736(18)31694-5
Keywords: bone marrow, hematopoiesis, diet, metabolism, infection
Citation: Hellmich C and Wojtowicz EE (2022) You are what you eat: How to best fuel your immune system. Front. Immunol. 13:1003006. doi: 10.3389/fimmu.2022.1003006
Received: 25 July 2022; Accepted: 31 August 2022;
Published: 20 September 2022.
Edited by:
Ian Marriott, University of North Carolina at Charlotte, United StatesReviewed by:
Abdulhadi Suwandi, Hannover Medical School, GermanyCopyright © 2022 Hellmich and Wojtowicz. This is an open-access article distributed under the terms of the Creative Commons Attribution License (CC BY). The use, distribution or reproduction in other forums is permitted, provided the original author(s) and the copyright owner(s) are credited and that the original publication in this journal is cited, in accordance with accepted academic practice. No use, distribution or reproduction is permitted which does not comply with these terms.
*Correspondence: Charlotte Hellmich, Yy5oZWxsbWljaEB1ZWEuYWMudWs=; Edyta E. Wojtowicz, ZWR5dGEud29qdG93aWN6QGVhcmxoYW0uYWMudWs=