- 1Graduate Program in Epidemiology and Health Surveillance (PPGEVS), Bacteriology and Mycology Section (SABMI), Evandro Chagas Institute (IEC), Ananindeua, Brazil
- 2Bacteriology and Mycology Section (SABMI), Evandro Chagas Institute (IEC), Ananindeua, Brazil
Background: There is evidence that the adaptive or acquired immune system is one of the crucial variables in differentiating the course of coronavirus disease 2019 (COVID-19), caused by severe acute respiratory syndrome coronavirus 2 (SARS-CoV-2). This work aimed to analyze the immunopathological aspects of adaptive immunity that are involved in the progression of this disease.
Methods: This is a systematic review based on articles that included experimental evidence from in vitro assays, cohort studies, reviews, cross-sectional and case-control studies from PubMed, SciELO, MEDLINE, and Lilacs databases in English, Portuguese, or Spanish between January 2020 and July 2022.
Results: Fifty-six articles were finalized for this review. CD4+ T cells were the most resolutive in the health-disease process compared with B cells and CD8+ T lymphocytes. The predominant subpopulations of T helper lymphocytes (Th) in critically ill patients are Th1, Th2, Th17 (without their main characteristics) and regulatory T cells (Treg), while in mild cases there is an influx of Th1, Th2, Th17 and follicular T helper cells (Tfh). These cells are responsible for the secretion of cytokines, including interleukin (IL) - 6, IL-4, IL-10, IL-7, IL-22, IL-21, IL-15, IL-1α, IL-23, IL-5, IL-13, IL-2, IL-17, tumor necrosis factor alpha (TNF-α), CXC motivating ligand (CXCL) 8, CXCL9 and tumor growth factor beta (TGF-β), with the abovementioned first 8 inflammatory mediators related to clinical benefits, while the others to a poor prognosis. Some CD8+ T lymphocyte markers are associated with the severity of the disease, such as human leukocyte antigen (HLA-DR) and programmed cell death protein 1 (PD-1). Among the antibodies produced by SARS-CoV-2, Immunoglobulin (Ig) A stood out due to its potent release associated with a more severe clinical form.
Conclusions: It is concluded that through this study it is possible to have a brief overview of the main immunological biomarkers and their function during SARS-CoV-2 infection in particular cell types. In critically ill individuals, adaptive immunity is varied, aberrantly compromised, and late. In particular, the T-cell response is also an essential and necessary component in immunological memory and therefore should be addressed in vaccine formulation strategies.
1 Introduction
The 2019 coronavirus disease pandemic (COVID-19) began its occurrence around March 2020 according to the World Health Organization (WHO) through a novel coronavirus (CoV) that causes severe acute respiratory syndrome (SARS) (1). COVID-19, which arises from a Betacoronavirus of zoonotic origin that was first reported in Wuhan, China, has resulted in high rates of morbidity and mortality worldwide (2).
Currently, the main antigen of the virus is the SARS-CoV-2 S (Spike) protein, which can bind the virus to the human angiotensin converting enzyme 2 receptor (ACE2) through the receptor binding domain (RBD) and thus allow its entry into the cell (3–5). Clinically, patients with COVID-19 can be classified as mild (no lung involvement), moderate (with respiratory symptoms), severe (greater lung involvement, with uncontrolled systemic inflammation) and critical (requirement for mechanical ventilation and signs of septic shock) (6).
The human immune system is divided into innate and adaptive. Although innate and adaptive immune responses are interconnected and play a joint role, each of them has specialized cells with distinct functions. The adaptive system is made up of antibody-producing B cells, CD4+ T cells with various adjuvant and effector mechanisms, and CD8+ T cells with cytotoxic potential. The adaptive response is capable of producing immune memory and control of viral infection in a way that its understanding in COVID-19 is essential. However, it is proposed that the exacerbated inflammatory immune response against SARS-CoV-2 is related to the disease severity (7).
Each SARS-CoV-2 protein can initiate an immunological response that results in the generation of antibodies, and it has been shown that protein S and protein N are antigens that result in the development of neutralizing antibodies when present in high quantities (8). However, in addition to being more specific, compared to antibodies against nucleocapsid proteins, antibodies against RBD emerge sooner during the infection (9).
In patients with COVID-19, there is an incubation time of 4 to 7 days before the onset of symptoms and an additional 7 to 10 days before individual progress to severe illness. Therefore, seroconversion of the disease by IgM, although it can start on the fifth day of symptom onset, due to individual variability of the immune response, is best measured on the 10th day of symptom and IgG on the 14th day (10).
Both protective immunity and protection against reinfection are provided by natural SARS-CoV-2 infection. Most infected people produce IgM, IgG, and IgA antibodies against the Spike protein and its receptor-binding domain (RBD) in response to SARS-CoV-2. Although neutralizing antibodies from exposure to CoV cannot prevent infection by a variant or SARS-CoV-2, the disease can have a milder course (9, 11). Furthermore, many peptides derived from SARS-CoV-2, such as those from the M protein, induced specific memory T cell responses, as well as IFN-gamma responses, in recovered patients (12, 13).
There are signs that the immunological dysregulation caused by SARS-CoV-2 contributes more to the intensity of the disease than the virus itself. Acute respiratory distress syndrome (ARDS) and multiple organ failure are its hallmarks, while lymphocyte depletion, cytokine storm, and delayed and defective interferon (IFN) response are all present. All these factors can cause widespread lung tissue damage and subsequent thrombotic events (14, 15). It is crucial to conduct research on the virus’s defense mechanisms, especially how certain T cells interact with SARS-CoV-2, in addition to research on antibodies in the disease, is essential to fill the current knowledge gap to improve vaccine design (16).
In this situation, the question of “What immunopathological features of the virus in adaptive immunity contribute to the development of COVID-19” emerges. This study addresses this issue and develops a didactic plan for adaptive immunity from COVID-19.
2 Materials and methods
2.1 Study design
This is a systematic review of the subject with the aim of collecting concise, comprehensive, and recent data. This proposal provides an overview and visualization of the topic and can be used as a reference for future clinical trials. The research carried out the following actions: 1) Development of the central question; 2) Outline inclusion and exclusion standards; 3) Article selection; 4) Examination of articles; 5) Review interpretation, debate, and elaboration (17). The PICO technique was used for different headings to formulate the main question: Population; Intervention; Comparation; Outcome (18).
2.2 Search strategy and selection criteria
The search phrases used for Health Sciences Descriptors and Medical Subject Headings (DeCS/MeSH) used were “SARS-CoV-2,” “COVID-19,” “Adaptive Immunity,” “Immunity,” and “Humoral Immunity”. The descriptors and the Boolean operator “AND” were combined to search articles. The following data sources were used in the literature search: Medical Literature Analysis and Retrieval System Online (MEDLINE), Latin American and Caribbean Literature in Health Sciences (LILACS), National Library of Medicine National Institutes of Health (PUBMED), and Scientific Electronic Library Online (SCIELO).
What immunopathogenic characteristics of SARS-CoV-2 in adaptive immunity are involved during COVID-19? was the overarching topic that the studies were chosen to address. As a result, the PICOS technique generated the question, which can be understood as follows: patient population with COVID-19; the intervention to assess immunopathogenic features in SARS-CoV2 infected patients; the comparison links the virus’s immunopathogenic properties to adaptive immunity; the disease’s progression determines the outcome. Articles of the original, systematic review, clinical trial, quasi-experiment, ecological, comparative, multicenter, case series, case-control, cohort studies (prospective and retrospective), and meta-analyses types are included in study designs. Studies published between January 2020 and July 2022 that were fully available in English, Portuguese, or Spanish met the inclusion requirements. Publications published before 2020, those only accessible in the abstract, letters to the editor, and articles or materials with subjects unrelated to the study issue were excluded.
2.3 Study selection and data extraction
Two researchers (MJAS and LRR) separately read the titles and abstracts of the indicated papers, proceeding to full-text review of any publication judged possibly relevant by either researcher. If there was a difference of opinion, it was resolved by dialogue with another author (KVBL). The PRISMA flowchart was used and is a component of the PRISMA protocol used to illustrate the investigations in the database, document all stages, inclusions and exclusions, and demonstrate how the final sample was collected. The following information was extracted: author, title, publication year, type of study, goals, and results. Then, using Microsoft Office Excel 365, they were arranged in pairs and independently.
3 Results
3.1 Literature search
When the inclusion criteria were applied, 101 articles were found, although some of them were letters to the editor, incomplete, or contained material unrelated to the study subject (Figure 1). A total of 56 articles formed the final structure of the articles.
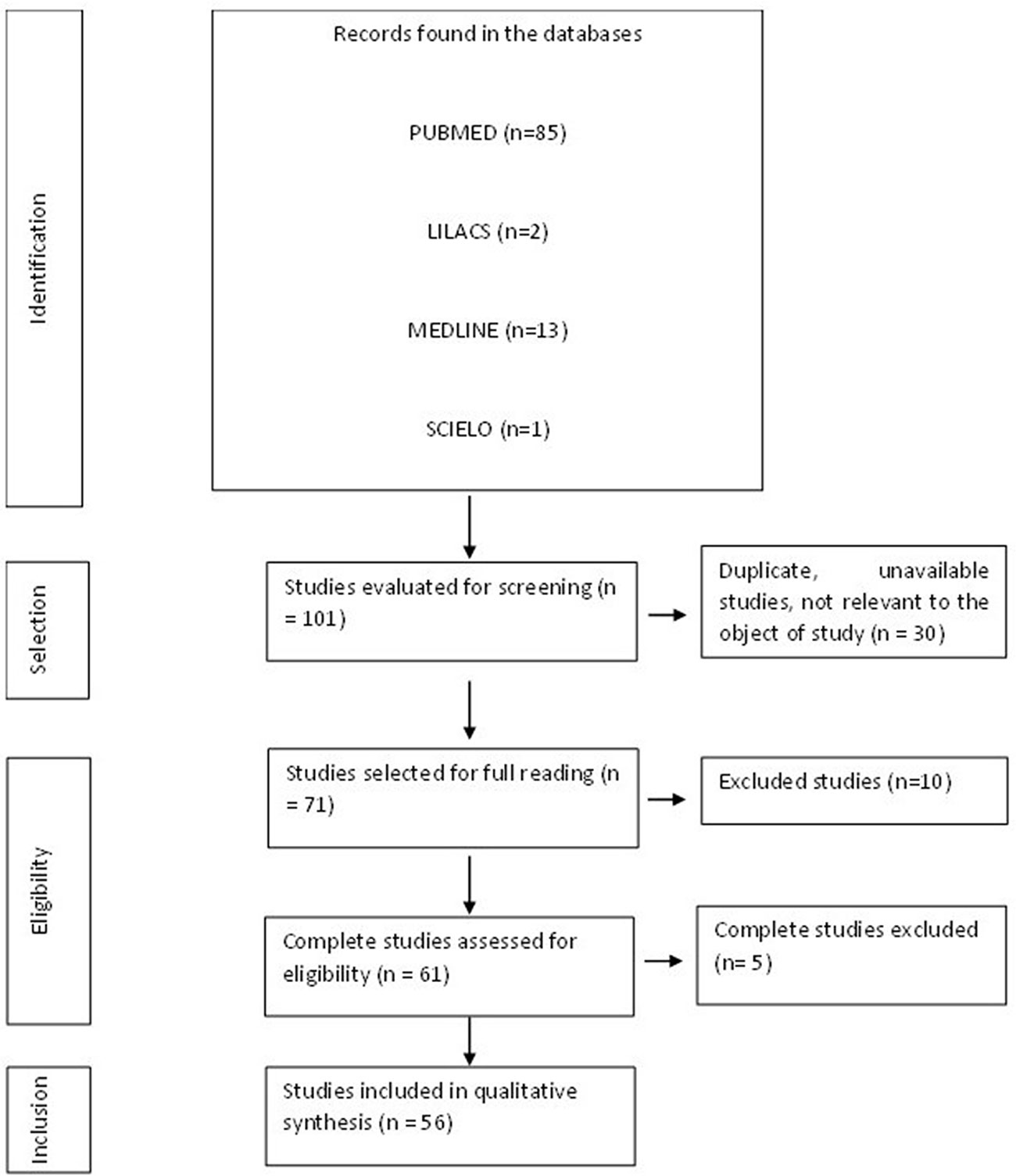
Figure 1 Flowchart of procedures for identification, selection, eligibility, and inclusion of studies for analysis. Belém, PA, Brazil, 2021.
3.2 Adaptive immune response to SARS-CoV-2 infection
As CD4+ T cells are related to the first response to infection, their percentage is higher than that of CD8+ T cells discovered after infection (7). The integrated adaptive immune response has been linked to a milder form of the disease, indicating a function of CD4+CD4 + and CD8+ T cells in the protective immunity of COVID-19 (19). A higher percentage of SARS-CoV-2-specific CD8+ T cells was observed in moderate patients (16). SARS-CoV-2-specific T cells in the acute-phase display a highly activated cytotoxic characteristic that is directly related to several clinical markers of disease intensity (20). The lungs of these seriously sick individuals also saw a large inflow of circulating T cells, overwhelming the local T cell compartment and suggesting vascular leakage. Patients in hospitals were distinguished by powerful stimulation of particularly potent or deficient CD4 + T cells and CD8 + T cells, reduced robust CD4 + T cells and CD8 + T cells comparable to highly functioning effectors, and absence of lymphocyte reaction (11).
The severity of the illness is correlated with the low IFN-γ and tumor necrosis factor – alpha (TNF-α) expression in CD4 + T cells and the elevated levels of granzyme B and perforin in CD8 + T lymphocytes. Furthermore, compared to moderate cases and healthy individuals, severe patients with COVID-19 had a significantly higher frequency of the depleted fraction (programmed cell death protein 1 [PD-1], CTLA-4, TIGIT) of CD8 + T cells. In severe COVID-19 infection, it showed reduced frequencies of CD4+ T cells producing IFN-γ. These data suggest that the poor outcome of the COVID-19 is related to functional abnormalities with respect to functional and loss of loss of CD8 + T cells (21).
A greatly increased population of CD8 + T cells was discovered in instances with moderate symptoms, indicating a link between a strong adaptive cellular immune response and improved disease management. Compared with healthy controls, CD8+ T and Natural Killer (NK cells) of COVID-19 patients expressed more inhibitory receptors of NKG2A (14). Although CD4 + T cells were not affected by SARS-CoV-2 infection, CD8 + T cells were. This response was characterized by the concurrent production of granzymes A and B, as well as perforin, in several subsets of CD8 + effector T cells. During primary infection, CD8+ T cells that expressed PD-1 also generated cytotoxic molecules, demonstrating that they were not physiologically exhausted (22). Despite a decrease in CD8+ T lymphocyte count, individuals with severe COVID-19 had a rate of HLA-DR expression in these cells that was higher than that of patients with mild disease (15).
Qualitative CD4+ T cell responses from critically ill individuals were compromised. In severe cases, Th cells begin to differentiate and develop atypically after Th0, resembling Th1, Th2 and Th17 but lacking primary functional immune features and regulatory T cells (Treg cells) (23). The different T and B cell responses shown might be a sign of an unbalanced immune response in people with COVID-19 who are seriously ill (24). Additionally, in severe cases of COVID-19, there are considerably fewer CD4+ memory T and Treg cells. Furthermore, these findings were related to a reduction in CD4+ and CD8+ T lymphocytes in the lymph nodes. Patients with COVID-19 atrophy of the spleen and lymph nodes, demonstrating the contribution of SARS-CoV-2 to the promotion of cell death. These results show that the number of CD4+ and CD8+ T lymphocytes is considerably reduced in patients with severe COVID-19 (15).
Patients with severe cases showed more robust T cell immune responses than patients with mild instances, which may indicate that patients with severe cases had better T cell memory against SARS-CoV-2. Evaluation of changes in the peripheral T and B cells of COVID-19 patients showed a negative connection between the humoral immune response and the immunological memory of T cells and the severity of the disease (13).
Follicular helper T cells (Tfh), a subset of CD4+ T cells that offer the support needed for B cell differentiation and antibody affinity maturation, promote the aging process of B cells in the restoration of COVID-19 (25). The specific IgG antibody response was unusually potent in these individuals. The tight relationship between the raised IgG response and the severity of the illness suggests a straightforward supplementary measure to distinguish between severe and nonsevere patients. In patients with COVID-19, a higher total antibody titer was independently linked to a poorer clinical outcome and increased much faster than IgM and IgG (26). In recovered patients, there was an inverse correlation between the frequency of memory B cell subsets and the severity of symptoms. However, they discovered that circulating megakaryocytes, elevated erythropoiesis, and proliferative and metabolically active plasmablasts are all increased in severe illness (27).
Early and transient rises of IgA, IgM, and, to a minor extent, IgG are related to asymptomatic SARS-CoV-2 infection. Severe illness is characterized by persistently high levels of IgA and IgG that are produced very late in the process of infection (28). IgA antibodies predominate the initial humoral responses unique to SARS-CoV-2. Shortly after the appearance of symptoms, peripheral growth of IgA plasmablasts with the ability to return to the mucosa was observed, culminating during the third week of sickness. IgG, IgM, and IgA were all viral-specific antibody responses, but IgA was more effective than IgG in neutralizing the virus (29).
The severity of the disease correlates with the increase in neutralizing titers (30). Additionally, it has been shown that the IgM or IgG nonresponder group had a considerably lower neutrophil-to-lymphocyte ratio than the IgM or IgG responder group. Patients with an IgM antibody response had substantially fewer CD20+ lymphocytes, while patients with an IgG antibody response had significantly more NK cells and CD4+ T cells than patients without an IgG antibody response. Furthermore, compared to the group, patients who developed IgM or IgG antibodies had a markedly higher proportion of total T lymphocytes and NK cells (31). Hypercoagulation and fibrinolysis can result from the deficiency or loss of hereditary angioneurotic edema function (C1-INH) (32).
In extreme instances, a reduction in RM, resting memory T cell frequency, and an increase of tissue-like memory, TLM subgroup of B cells were seen. Based on the expression of CD21 and CD27 in T cells, variations were found between subsets of B lymphocytes. S and M-specific CD4+ may impact the composition of the composition of the S1-specific B cell memory fraction (12).
The characteristics of the articles included in the formation of this review are described in the table below (Table 1). The articles were mostly international (n=55) and some national (n=1) derived from PUBMED, LILACS, MEDLINE, and SCIELO. The review resulted in 55 articles in English and one in Portuguese.
3.3 Immunological didactic model of COVID-19
The didactic model for COVID-19 was based on the results of this review for mild and moderate cases and for severe and critical cases in separate figures based on immune variability in host defense against infection in different clinical forms, which was described in the topics below. They were represented in chronological order of events by letters (A-Z). The corresponding references are marked within the image for each biological process in numbers within parentheses.
3.3.1 Adaptive immune response to SARS-CoV-2 infection in mild and moderate cases
Figure 2 illustrates adaptive immunity in SARS-CoV-2 infection in mild and moderate cases.
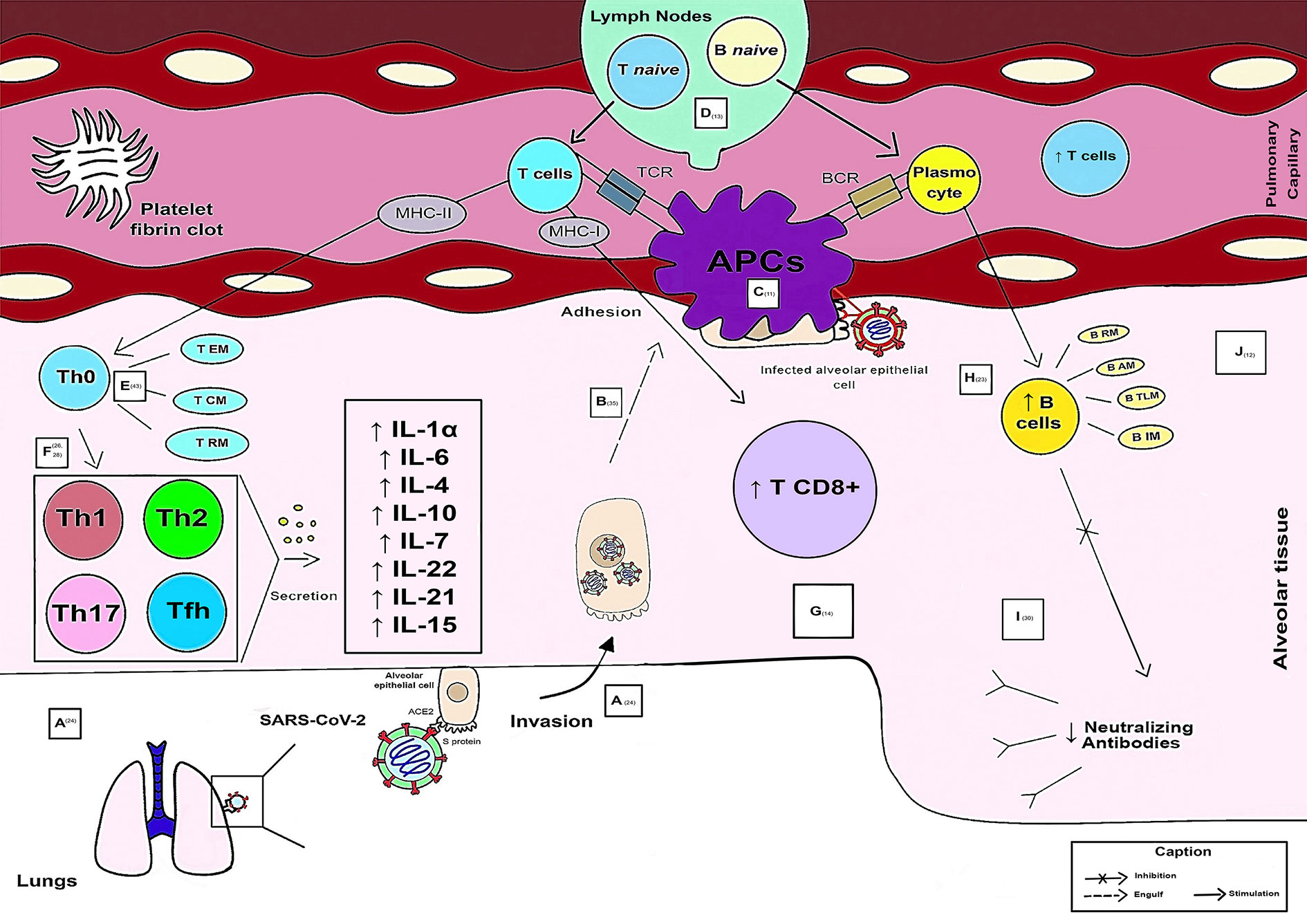
Figure 2 Schematic model of the evolution of the Adaptive Immune Response in mild and moderate cases of COVID-19.
Viral entry into the host occurs through binding of the Spike protein to the human ACE2 receptor in lung cells, mainly alveolar cells rich in this receptor. After activation and activity of the innate response, there was an evolution of the adaptive response (A). The APC is responsible for engulfing the alveolar epithelial cells infected by the virus (during the adhesion process) (B). Viral antigens (Ag) are recognized, processed, and presented through APCs to Ag recognition receptors (T cell receptor - TCR, through MHC-I and MCH-II, and B cell receptor - BCR) of adaptive immune cells (C). When antigens are presented by APCs, lymph nodes activate and secrete naive T and B cells into pulmonary capillaries that travel to the affected alveolar region. Thus, there is a greater release of functional T cells. Coagulopathy generated through platelet fibrin clots may be present in some cases (D). In pulmonary alveolar tissue, CD4+ memory T cells are found (T RM resting memory, T CM central memory and T EM memory effector) (E). The magnitude of T cell responses is maintained in less severe cases. Therefore, from Th0 onward, the responses of the helper T cells are consolidated into subpopulations of Th1, Th2, Th17 and Tfh. The cytokines secreted by these subpopulations of immune cells increase in mild cases and reflect an anti-inflammatory role, such as IL-1α, IL-6, IL-4, IL-10, IL-7, IL-22, IL-21, and IL-15 (F). A larger population of CD8+ T cells specific for SARS-CoV-2 is observed in these patients (G). B cells have a higher count and are subdivided into the following: B RM (resting memory), B AM (activated memory), B IM (intermediate memory), B TLM (tissue-like memory) (H). SARS-CoV-2 neutralizing antibodies levels are decreased in mild and moderate patients are decreased (I). Histopathological conditions caused by the virus, such as edema, fibrosis, hyaline membrane formation, and lymphocytic interstitial infiltration into the alveoli are rarely recorded because of clinical improvement (recovery) and seroconversion (J).
3.3.2 Adaptive immune response to SARS-CoV-2 infection in severe and critical cases
Figure 3 illustrates adaptive immunity in SARS-CoV-2 infection in severe and critical cases.
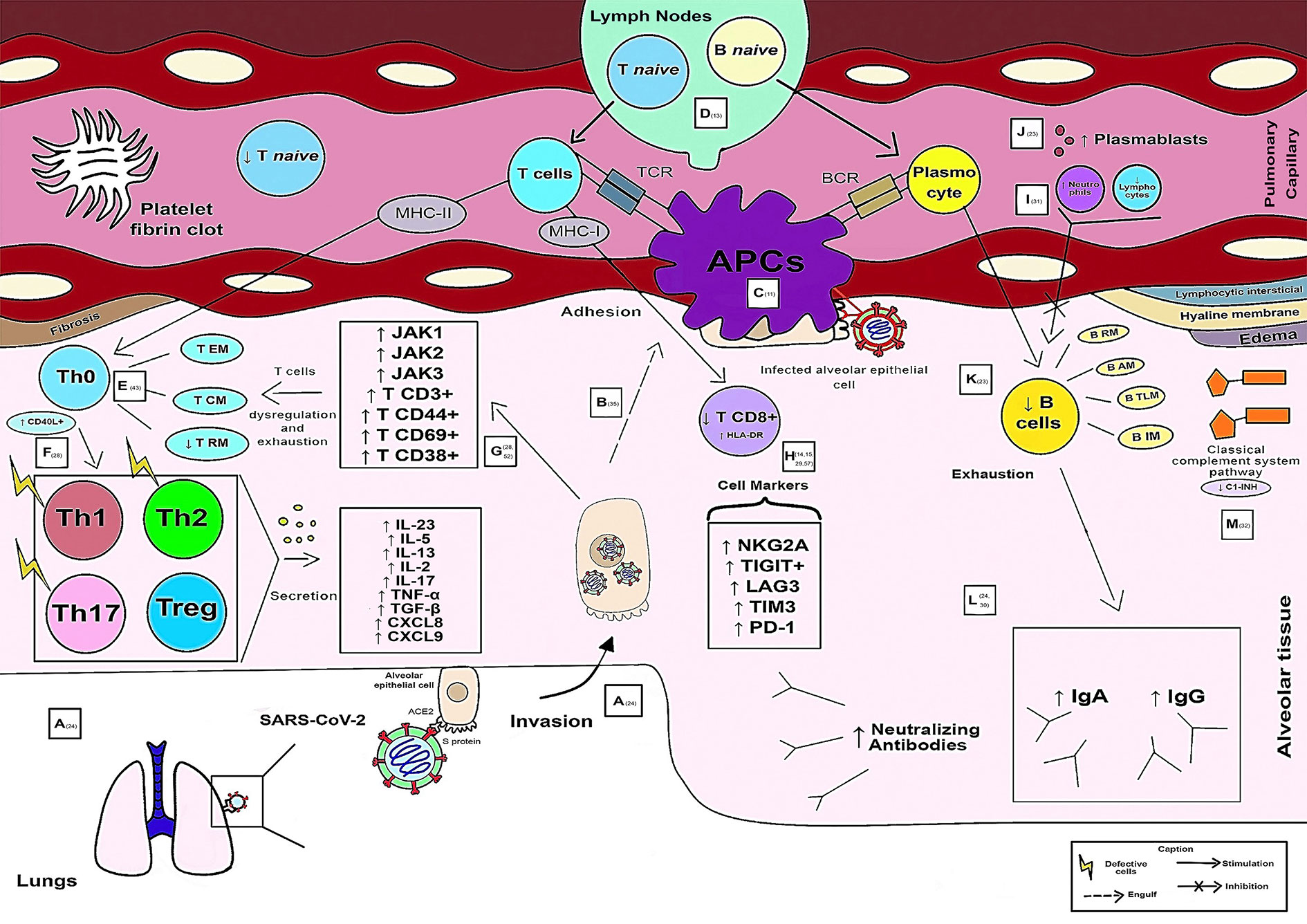
Figure 3 Schematic model of the evolution of the Adaptive Immune Response in severe and critical cases of COVID-19.
Viral entry into the host occurs by binding of the Spike protein to the human ACE2 receptor in lung cells, mainly alveolar cells rich in this receptor. After activation and activity of the innate response, there was an evolution of the adaptive response (A). The APC is responsible for engulfing the alveolar epithelial cells infected by the virus (during the adhesion process) (B). Viral antigens (Ag) are recognized, processed, and presented through APCs to Ag recognition receptors (T cell receptor – TCR, through MHC-I and MCH-II, and B cell receptor – BCR) of adaptive immune cells (C). When antigens are presented by APCs, lymph nodes activate and secrete naive T and B cells into pulmonary capillaries that travel to the affected alveolar region. Naive T cells were found at low levels in critically ill patients (D). In pulmonary alveolar tissue, in which CD4+ memory T cells are found (T RM resting memory, T CM central memory and T EM memory effector), there is a functional dysregulation of CD4+ T cells caused by the immunological imbalance of the virus in the individual. Increased expression of CD40L+ in CD4+ T cells (responsible for transmitting signals for cell activation, differentiation, and proliferation) and the low frequency of RM T cells were associated with the severity of the disease (E). From Th0 onward, differentiation and atypical formation of Th cells occur, similar to Th1, Th2, and Th17, but with the absence of main effector immune characteristics and Treg cells. The presence of a subpopulation of Treg cells was associated with the severity of the disease. The cytokines secreted by these subpopulations of immune cells are increased in severe cases and reflect inflammatory conditions, such as IL-23, CXCL8, CXCL9, IL-5, IL-13, IL-17, IL-2, TNF-α, and TGF-β (F). Co-stimulatory molecules such as Janus Kinases (JAK) 1, JAK2, and JAK3 and different types of T cells such as T CD3+, T CD44+, T CD69 +, and T CD38+, stimulated by viral immune dysregulation, are included in severe cases (G). However, CD8+ T lymphocytes are present in fewer numbers, with a high expression rate of human leukocyte antigen (HLA-DR, causing overactivation of these cells) in severe cases of COVID-19. Some markers in CD8+ T lymphocytes were associated with disease severity, such as NKG2A, TIGIT+, TIM3+, lymphocyte activation gene 3 (LAG3+) and programmed cell death protein 1 (PD-1) (H). The ratio of neutrophils to lymphocytes may be a predictor of decreased production of IgM or IgG antibodies and activation and depletion of memory B cells observed during COVID-19, which are positively correlated with the functions of CD4+ T cells (I). Proliferative and metabolically active plasmablasts (plasma cell precursor cells, differentiated B cells capable of producing antibodies) have increased in severe disease (J). B cells are smaller in number and are subdivided into the following: B RM (resting memory), B AM (activated memory), B IM (intermediate memory), B TLM (tissue-like memory) (K). The levels of SARS-CoV-2 neutralizing antibodies in critically ill patients are increased, with persistent and increased levels of IgA and IgG antibodies that have been associated with severe COVID-19 (L). Deficiency or loss of C1 esterase inhibitor function has been observed in hereditary angioneurotic edema (C1-INH) in severe cases that can lead to hypercoagulation (with fibrin clot formation) and fibrinolysis. Additionally, histopathologically, the virus is capable of causing edema, fibrosis, formation of hyaline membranes and lymphocytic interstitial infiltration into the alveoli (M).
4 Discussion
Delayed or debilitating type I IFN responses caused by prior dysregulation of the innate immune response disrupt adaptive immune activation. Long-term viral persistence worsens inflammatory reactions, which can fatigue the body and depress the immune system as a regulatory feedback loop (52). In this sense, effective adaptive immunity during viral infections is essential for removing viral particles from the host’s body, preventing the disease from developing to severe stages, and finally recovering afflicted people. Helper T lymphocytes, sometimes referred to as CD4+ T, are, in general, the key players in the adaptive immune response brought about by viral infections. Following virus detection, APCs (antigen-presenting cells) process and deliver viral antigens to CD4+ and CD8+ T lymphocytes. By controlling the course of T cell responses, APCs create a microenvironment of cytokines (15).
In several studies on adaptive immunity and virus, CD4+ and CD8+ T cells were associated with protective response, with greater resolution, despite the uncoordinated responses of these cells and the scarcity of naive T cells, related, among other factors, to aging, seems to make the case more aggravating (19, 35). Compared to antibodies and CD8+ T cells, SARS-CoV-2-specific CD4+ T cells showed a better correlation with lowering the severity of COVID-19 illness. Absolute neutrophil counts and neutrophil-to-lymphocyte ratio may be useful indicators of IgM or IgG antibody response, according to the Rezaei et al., 2021 (31). In this case, the reduction in the number of T cells in patients with severe conditions is negatively related to the production of IL-6 and IL-10 cytokines (11, 25).
The ability of CD4+ T cells to attract additional effector cells to a viral antigen site is one of their main functions. Expression of the CCL3/4/5 gene (MIP-1s) and the CXCL1 chemokine, which is exclusive to CD4+ T cells, may aid in effector cell recruitment. Increased apoptosis and decreased T cell proliferation rates may be the causes of the decrease in T cells in COVID-19, or they may both be contributing factors (25, 40, 63).
Double positive peripheral CD4+ and CD8+ T lymphocytes decreased in cases of illness with severe symptoms (51). A substantial fraction of cTfh is CCR6+, which could indicate accommodation of the mucosal airways. Tfh cells help fight SARS-CoV-2 infection by inducing the generation of neutralizing antibodies (67). The frequencies of cTfh cells (follicular helper T cells or CD4+ T cells) were associated with a reduced severity of the disease. It is complicated and still needs to be better understood how neutralizing antibodies, Tfh cells, and severity, the severity of the disease are related (35).
Specific to circulating S1, CM T cells and T EM (memory effector T cells) have improved effector characteristics, such as activation, proliferation, and production of immune mediators (50). EM T cells are ‘receptionists’ of entry because they are often located in tissues that are easily invaded by pathogenic microorganisms, whereas CM T cells are recruited to secondary lymphoid organs to accelerate the immune response triggered by antigen-presenting dendritic cells. EM T cells and CM T cells with antigen-specific S1 protein activity from most patients have a decreased functional and effector response to at least one parameter, namely activation, proliferation, and secretion of immune mediators (43). Low frequencies of memory T cells (T RM) were found, indicating an impairment of vascular integrity and epithelial barrier function and, therefore, alveolar leakage in critically ill and critically ill patients. Polyclonal T-cell activation is also a risk factor that causes a decrease in the production of certain cytokines together with terminal differentiation in patients (27). SARS-CoV-2 positive B and T cells from working memory were found 12 months after the first natural infection (63).
The presence of virus-specific CD8+ T lymphocytes was associated with improved COVID-19 outcomes in SARS-CoV-2 infection. In addition to that, due to their capacity to eradicate infected cells, CD8+ T cells are essential for recovery from many viral infections. Immune cells (CD8+ T) have programmed cell death protein 1 (PD-1) or differentiation group 279 (CD279) on their surface, which reduce immune system responses, inhibit the activity of inflammatory T cells, and increase self-tolerance. Continuous expression of PD-1 depletes T lymphocytes and reduces their ability to eradicate infectious cells. Although its modes of action are still unclear and may include numerous overlapping processes, CTLA-4 has been shown to be one of the inhibitory immunological checkpoint molecules. By attaching to the CD86 and CD80 ligands, which are similarly engaged in the early excitatory stages of immature and memory T cells, this molecule effectively reduces the excitatory activity of CD28 (15, 68).
COVID-19 is characterized by increased inflammation, which can be generated by regulatory T cells (Tregs). Additionally, FoxP3 induction (Treg cell generator) was considered an important viral pathogenic factor, which is potent in the lungs of patients with severe COVID-19 (25). To discover whether PD-1+ (as observed in patients) in CD8+ T cells in COVID-19 is helpful or leads to the onset of severe illness, more research is required. The Treg-specific transcription factor FoxP3 is well recognized, and T cells that express FoxP3 can inhibit T cell responses. Convalescent patients with COVID-19 had higher levels of FOXP3 expression in circulating CD4+ T cells than healthy subjects. However, in severely ill individuals, the frequency of Treg in the circulation decreases. Critically ill patients in the Intensive Care Unit (ICU) have persistent reductions in all lymphocyte subtypes, especially T cells, while having elevated expression of CD25, CTLA-4 and PD-1in CD4+ T cells. However, FOXP3 expression was not examined (25).
In COVID-19 patients, T cells are expected to exhibit a mixture of Th1, Th2 and Th17 responses, and it is not yet known which Th response is the most protective (25). Regarding the cytokines produced during the adaptive immune response phase in patients with COVID-19, the cytokines IL-2, IL-6, IL-10, IL-4, IL-7, IL-17, and TNF-α were the most cited in disease studies.
IL-2 plays a fundamental role in the dissemination of T cells and in the generation of effector and memory T cells through glucose metabolism, which promotes the proliferation of T, BB and NK cells (69). Thus, this cytokine is crucial for developing of effective immune responses and the preservation of self- tolerance (70). In normal circumstances, IL-2 signaling can activate FOXP3 (Treg cells), which acts as a negative feedback mechanism to stop the IL-2 response. However, in severe COVID-19, this activation is reduced (25). The severity of COVID-19 has a positive correlation with elevated blood levels of IL-2 (27).
IL-6 is a mediator that can inhibit the activation of normal T cells and is therefore the possible cause of lymphopenia in COVID-19 (71). This cytokine is responsible for controlling hematopoiesis, immune response, and inflammation. It may provide regenerative and anti-inflammatory effects by conventional signaling (72), which also suggests these functions during the adaptive phase of the response against infection. IL-6 was the only cytokine among those studied in a study by Lu et al., 2021, which increased in healthy people compared with patients with COVID-19 at this stage of the response to infection (73).
SARS-CoV-2 infection is capable of generating high secretion and activation of cytokines from Th2 cells, among which IL-10 and IL-4 can stimulate an anti-inflammatory role (74). IL-10 is a type 2 cytokine that inhibits the generation of pro-inflammatory cytokines (such as IL-6) in several cell types and acts in immunomodulation. Regarding its role in COVID-19, it has been described to have antagonistic roles, pro-inflammatory and anti-inflammatory, but with positive anti-inflammatory functions in the adaptive phase of the immune response (75). However, IL-4 functions primarily in the activation, multiplication and specialization of B lymphocytes and the production of IgE immunoglobulin, making it a highly sensitive cytokine for the Th2 subset that controls the humoral response (76). Furthermore, IL-4 has been proposed to be related to the metabolic plasticity of different tissues through its anti-inflammatory roles (77). In this sense, IL-4 may be associated with a beneficial role in delaying COVID-19 by inhibiting the inflammatory process (74).
During the disease, patients with severe COVID-19 developed a pattern of increased IL-5, IL-13, eotaxin-2, IgE, and eosinophils (78). IL-5 is a Th2-type cytokine that acts as a specific factor in the maturation and differentiation of eosinophils (79). In patients with COVID-19, it has been associated with a poor prognosis (80). Activated Th2 cells produce IL-13, which acts as a counterregulator of the Th1 immune response, while stimulating the synthesis of TGF-β, which has been correlated with increased severity and greater viral load (80, 81).
Numerous immune cells, including monocytes, macrophages, T cells, and others, can produce TNF-α. This mediator has been associated with pro-inflammatory reactions that are mediated by IL-1 and IL-6 (82). TNF-α, a crucial pleiotropic mediator of acute and chronic systemic inflammatory reactions, has the capacity to control cell proliferation and death, while also enhancing the synthesis of other chemokines and cytokines related, for example, to tissue homeostasis (83). One of the most significant pro-inflammatory cytokines of the innate immune response, TNF-α, can cause Cytokine Release Syndrome (CRS) when its signaling is out of balance. Crosstalk between T cells and other cell types is mediated through connections between responses of TNF and its receptors (TNFR) and regulate positively T cells (84). This cytokine has a positive association with the severity of COVID-19 at this stage of infection associated with hyperinflammatory reactions (cytokine storm) in individuals (15).
IL-7 has a profound impact on lymphocytic differentiation, be it in the development of T cells or plasma homeostasis (necessary for the formation of immature and memory CD4+ T cells, in addition to Th17 cells). It acts on T cell activation, increases the release of other pro-inflammatory cytokines, and correlates negative with TGF-β production (85). These actions depend on their interaction with IL-6 (86). In some life-threatening viral infections, IL-7 treatment restores cell number and functional activity, resulting in lower viral load and clinical improvement (87). In the case of COVID-19, this biomolecule is associated with improved patients outcome with the disease and its inverse relationship with severity, as it allows greater recruitment of T cells (25).
Additionally, through STAT3 activation and the expression of Th17 transcription factors, including ROR-t and AHR, IL-6 signaling promotes Th17 development. Th17 cells produce, for example, the cytokine IL-17, which is elevated in people with autoimmune disorders and inflammatory conditions. It is also generated by CD8+ cells and many subtypes of immature lymphocytes, including gamma delta T cells, NK cells, and group 3 innate lymphoid cells. Therefore, IL-17 is a pro-inflammatory cytokine involved in tissue damage repair, physiological stress reduction, and removal of infection. These roles differ depending on the tissue in which IL-17 is expressed, with the gastrointestinal system and skin being particularly significant. The recruitment of neutrophils by elevated levels of IL-17 in COVID-19 ARDS has been associated with parenchymal damage and lung edema (88).
IL-22 is closely related to tissue repair, especially lung and intestinal epithelial cells, and, during COVID-19, CD4+ T cell activity may effectively participate in lung tissue regeneration. SARS-CoV-2 appears to retain the ability of CD4+ memory T cells to produce IL-22 (27).
The expression of IL-21 has as its main function a robust memory of B cells in COVID-19 (23). Memory B cell activation and exhaustion during COVID-19 are correlated with CD4+ T cell activities. When people have recovered, CD4+ T cells that release IL-21+ and CD4+ T cells that express CD40L+ are better correlated with the affinity formation and maturation of B lymphocytes that are related to protective function (12).
Th17 is important for infections such as COVID-19 and autoimmune. Therefore, in individuals with a dominant Th17 response, medication directed against the Th17 phenotype may be advantageous. Through the inhibition of STAT3, which appears essential for generating the cytokine IL-17A, biological medicines can interfere with cell development toward the Th17 phenotype. Using the Janus kinase (JAK) 2 signaling route, IL-6, IL-23, and IL-21, as well as IL-21 through the JAK1 and JAK3 signaling pathways, activate STAT3. Because IL-23 causes Th17 cells to become pathogenic, inhibiting JAK signaling pathways can stop the growth of pathogenic Th17 cells (23).
IL-15 is a critical mediator for the immunomodulation of inflammation during viral infections. Myeloid cells produce IL-15 to help body T cells react and activate natural killer cells (NK). In mice, IL-15 deficiency promotes airway resistance, as its presence modulates allergen-induced airway obstruction, lowers goblet cell hyperplasia and controls pro-inflammatory cytokines, activating regulatory CD4 T cells, +CD25+Foxp3+ generate IL-10 and IFN-γ. In COVID-19 patients, IL-15 plays a protective role, being more present in mild and moderate cases, in addition to being a possible therapeutic target for the disease (25, 27, 53, 89).
The classical pathway of the complement system is one of the main mechanisms of adaptive humoral immunity. Regarding its activation, there may be an interaction between it and the elevated levels of IL-6 seen in patients with SARS-CoV-2 pneumonia. IL-6 is a potent inducer of C-reactive protein (CRP), capable of initiating complement activation. The initiation of the classical pathway through C1 in patients with severe COVID-19 appears to be impaired, as C1-esterase is low and is related to the thrombotic condition. This is corroborated by individuals with hereditary angioedema (HAE) caused by C1-INH deficiency having higher blood D-dimer levels (90).
Plasmablasts reflect the interactions of adaptive cells with the environment. After seroconversion by the SARS-CoV-2 S protein, plasmablasts lose the type 1 interferon signaling pattern and express gene signatures induced by IL-21 and TGF-β, producing mainly IgG1 and IgA1. In the immune reaction of critically ill patients, there is a change in the programming for inducing IgA2, so that this immunoglobulin does not bind to the dominant antigen of SARS-CoV-2, thus affecting the expression of TGF-β. The cytokine TGF-β is a mediator that regulates angiogenesis, inhibits cell proliferation, and is produced by lymphocytes, macrophages, fibroblasts, smooth muscle cells, chondrocytes, astrocytes, epithelial cells, platelets, and even tumor cells (91).
Notably, the quality of the B-cell response has changed specifically in severely sick ICU patients with COVID-19. These individuals will develop fucosylated IgG antibodies against protein S compared with those with minor symptoms. Fucosylated antibodies improve antibody-dependent cell cytotoxicity and have a higher binding affinity to Fc receptors (ADCC). Future research may help determine whether fucosylated IgG variants activate T cells like their fucosylated counterparts and help decrease the frequency of specific CD4+ T cell antigens seen in ICU patients until the crosslinking of Fc receptors is required for proper cross-presentation of antigen and T-cell initiation (92).
The magnitude and significant elevation of the SARS-CoV-2 peptide-specific neutralizing titers was associated in 2 studies with the severity of the disease, which can serve as a measure of epidemiological control and the evaluation of post-vaccine protective immunity (30, 45). They suggested that a worse result is associated with B-cell activation and proliferation in COVID-19 cases, particularly in severe instances. However, B cells also contribute to antigen presentation and activation of CD4+ T cells (26).
The durability of immunity appears to last 3 to 5 months for SARS-CoV-2 (11, 35, 39). IgA and IgG were shown to be present immediately after the beginning of symptoms and IgA has a stronger effect on viral neutralization than IgG. One month after the beginning of symptoms, serum specific IgA levels decline dramatically, while neutralizing IgA is still measurable in saliva for a longer period (even if it is still very short) (29). IgA levels between hospitalized patients and recovering plasma donors can vary greatly and are not always correlated with standard tests (47).
Regarding gender differences, 4 studies in the review addressed this topic (49, 54–56). The increased severity of COVID-19 in men is related to increased plasma levels of cytokines from the innate immune system (including IL-8 and IL-18). Because T-cell activation depends on the release of sex steroid hormones, the worsening of the male clinical status was associated with a considerably lower percentage of activated T cells (CD38+HLA-DR+), terminally differentiated T cells (PD- +, TIM-3+) and trends toward less IFN-γ+ and CD8+ T cells. In this sense, low testosterone levels in men were associated with a worse clinical outcome (93). Although similar relationships were not observed in female patients, low activation of CD8 + T cells and poor IFN-γ production by CD8 + T cells were strongly related to the age of the patient (55).
There are distinctions between the downstream effects that T cells have on monocytes, depending on the sex. Gender-specific studies revealed that healthy males and male nonventilated patients had higher levels of T-cell-induced up-regulation of CD123 in monocytes than the corresponding groups of female patients. CD123, the α chain of receptors of the interleukin 3, is a surface cell marker present in APCs, such as plasmacytoid dendritic cells (pDCs) and monocytes, critical for activation of adaptive CD4+ and CD8+ T cell responses. Increased binding of T-monocyte cells in males may explain the reported higher plasma concentrations of IL-8 and IL-18 in COVID-19 patients, as IL-3 leads to monocyte mobilization and since high serum levels of unconventional monocytes have been recorded in men, such as CD14+/CD16+, which activate inflammation through the release of these cytokines (94). By encouraging the recruitment of circulating pDCs into the airways and inducing the release of CXCL12 from pulmonary CD123+ epithelial cells, IL-3 boosts innate antiviral immunity (49, 95).
Regarding pregnancy, only 2 studies addressed this and concluded that there is only a small amount of information on the immunological response to COVID-19 throughout this period, postpartum, nursing and in newborns (11, 58). T cell activation is slightly different during pregnancy. After exposure to SARS-CoV-2 particles and the pools of its proteins/peptides, it is also linked to changes in peripheral B cell frequencies and phenotypes (58).
The limitations of this review in terms of its elaboration are: a) the methodology applied (since the search strategy was conducted based on the choice of keywords to answer the main question, so some relevant results may have been missed); b) the results focus on experiments for infection in humans (excluding data on animals with the virus); c) the degree of evidence in which the primary data included were obtained (since the selection of patients and controls came from different criteria and with different sampling and confounding factors); d) differentiation of clinical case definition, as well as severity of the disease in the studies of this review; e) different test methods and assays to investigate the characteristics of adaptive immune cells in the clinical forms evaluated; f) the reviews analyzed in this article present a summarized overview of information (which compromises a more in-depth description of the topic). The limitations also come up against questions not yet answered by the literature, mainly: a) few data found in the literature on the adaptive immune response in pregnant women; b) uncertainty about the durability of humoral responses and their practical significance in relation to sterilizing immunity; c) the durability and protection offered by virus-specific T-cell responses and their relative importance in defending against reinfection compared to antibodies; d) the imprecision of which of the T helper cell responses is/are more resolute.
Despite this, the articles included the present validation and standardization in addition to supporting major contributions to address the guiding question, ensuring an efficient and critical qualitative synthesis of the data. As a result, these factors do not imply significant methodological heterogeneity. These restrictions also highlight the need for immediate research, focusing primarily on postvaccination-scale T cell assays in people to improve the population’s immune surveillance systems and disseminate findings on the interdependence and relative importance of cellular and humoral responses in a balance between protective and pathological responses to make inferences with implications, especially for immunosuppressed and advanced age subgroups.
5 Conclusions
Adaptive immunity is diverse, dysregulated, impaired, and delayed in critically ill patients, making it essential to elucidate its immune factors and what makes the clinical outcome different between men and women.
To date, the developed vaccines correspond to great progress in the fight against disease for the formation of herd immunity. Moreover, it was shown here that vaccine production strategies must consider the severity of the clinical case and understanding of protective immunity through ongoing adaptive immunity studies in COVID-19 cases, so that vaccines can induce a long-lasting immunological response against reinfection or possible variants. Due to the limited duration of the antibody response and the emergence of virus variants, it is recommended that vaccines also induce CD4+ and CD8+ T cell responses. The T-cell response is also an essential and necessary component of immunological memory.
Data availability statement
The original contributions presented in the study are included in the article/supplementary material. Further inquiries can be directed to the corresponding author.
Author contributions
This document was written by MS and LR. This paper was corrected by KL and LL. All authors contributed to the article and approved the submitted version.
Conflict of interest
The authors declare that the research was conducted in the absence of any commercial or financial relationships that could be construed as a potential conflict of interest.
Publisher’s note
All claims expressed in this article are solely those of the authors and do not necessarily represent those of their affiliated organizations, or those of the publisher, the editors and the reviewers. Any product that may be evaluated in this article, or claim that may be made by its manufacturer, is not guaranteed or endorsed by the publisher.
References
1. World Health Organization. WHO director-general’s opening remarks at the media briefing on COVID-19 - 11 march 2020 (2020). Available at: https://www.who.int/director-general/speeches/detail/who-director-general-s-opening-remarks-at-the-media-briefing-on-covid-19—11-march-2020 (Accessed November 4, 2021).
2. Hu B, Guo H, Zhou P, Shi Z-L. Characteristics of SARS-CoV-2 and COVID-19. Nat Rev Microbiol (2021) 19:141–54. doi: 10.1038/s41579-020-00459-7
3. Amanat F, Krammer F. SARS-CoV-2 vaccines: Status report. Immunity (2020) 52:583–9. doi: 10.1016/j.immuni.2020.03.007
4. Lan J, Ge J, Yu J, Shan S, Zhou H, Fan S, et al. Structure of the SARS-CoV-2 spike receptor-binding domain bound to the ACE2 receptor. Nature (2020) 581:215–20. doi: 10.1038/s41586-020-2180-5
5. Thanh Le T, Andreadakis Z, Kumar A, Gómez Román R, Tollefsen S, Saville M, et al. The COVID-19 vaccine development landscape. Nat Rev Drug Discovery (2020) 19:305–6. doi: 10.1038/d41573-020-00073-5
6. Guan W, Ni Z, Hu Y, Liang W, Ou C, He J, et al. Clinical characteristics of coronavirus disease 2019 in China. New Engl J Med (2020) 382:1708–20. doi: 10.1056/NEJMoa2002032
7. Grifoni A, Weiskopf D, Ramirez SI, Mateus J, Dan JM, Moderbacher CR, et al. Targets of T cell responses to SARS-CoV-2 coronavirus in humans with COVID-19 disease and unexposed individuals. Cell (2020) 181:1489–501.e15. doi: 10.1016/j.cell.2020.05.015
8. Petherick A. Developing antibody tests for SARS-CoV-2. Lancet (2020) 395:1101–2. doi: 10.1016/S0140-6736(20)30788-1
9. Carrillo J, Izquierdo-Useros N, Ávila-Nieto C, Pradenas E, Clotet B, Blanco J. Humoral immune responses and neutralizing antibodies against SARS-CoV-2; implications in pathogenesis and protective immunity. Biochem Biophys Res Commun (2021) 538:187–91. doi: 10.1016/j.bbrc.2020.10.108
10. Huang C, Wang Y, Li X, Ren L, Zhao J, Hu Y, et al. Clinical features of patients infected with 2019 novel coronavirus in wuhan, China. Lancet (2020) 395:497–506. doi: 10.1016/S0140-6736(20)30183-5
11. Fergie J, Srivastava A. Immunity to SARS-CoV-2: Lessons learned. Front Immunol (2021) 12:654165. doi: 10.3389/fimmu.2021.654165
12. Pušnik J, Richter E, Schulte B, Dolscheid-Pommerich R, Bode C, Putensen C, et al. Memory b cells targeting SARS-CoV-2 spike protein and their dependence on CD4(+) T cell help. Cell Rep (2021) 35:109320–0. doi: 10.1016/j.celrep.2021.109320
13. Zhang F, Gan R, Zhen Z, Hu X, Li X, Zhou F, et al. Adaptive immune responses to SARS-CoV-2 infection in severe versus mild individuals. Signal Transduction Targeted Ther (2020) 5:156. doi: 10.1038/s41392-020-00263-y
14. Bordallo B, Bellas M, Cortez AF, Vieira M, Pinheiro M. Severe COVID-19: what have we learned with the immunopathogenesis? Adv Rheumatol (2020) 60:50–0. doi: 10.1186/s42358-020-00151-7
15. Lotfi R, Kalmarzi RN, Roghani SA. A review on the immune responses against novel emerging coronavirus (SARS-CoV-2). Immunol Res (2021) 69:213–24. doi: 10.1007/s12026-021-09198-0
16. Peng Y, Mentzer AJ, Liu G, Yao X, Yin Z, Dong D, et al. Broad and strong memory CD4(+) and CD8(+) T cells induced by SARS-CoV-2 in UK convalescent individuals following COVID-19. Nat Immunol (2020) 21:1336–45. doi: 10.1038/s41590-020-0782-6
17. Rother ET. Revisão sistemática X revisão narrativa. Acta Paulista Enfermagem (2007) 20:v–vi. doi: 10.1590/S0103-21002007000200001
18. Santos CM da C, Pimenta CA de M, Nobre MRC. The PICO strategy for the research question construction and evidence search. Rev Latino-Am Enfermagem (2007) 15:508–11. doi: 10.1590/S0104-11692007000300023
19. Rydyznski Moderbacher C, Ramirez SI, Dan JM, Grifoni A, Hastie KM, Weiskopf D, et al. Antigen-specific adaptive immunity to SARS-CoV-2 in acute COVID-19 and associations with age and disease severity. Cell (2020) 183:996–1012.e19. doi: 10.1016/j.cell.2020.09.038
20. Sekine T, Perez-Potti A, Rivera-Ballesteros O, Strålin K, Gorin J-B, Olsson A, et al. Robust T cell immunity in convalescent individuals with asymptomatic or mild COVID-19. Cell (2020) 183:158–68.e14. doi: 10.1016/j.cell.2020.08.017
21. Zheng H-Y, Zhang M, Yang C-X, Zhang N, Wang X-C, Yang X-P, et al. Elevated exhaustion levels and reduced functional diversity of T cells in peripheral blood may predict severe progression in COVID-19 patients. Cell Mol Immunol (2020) 17:541–3. doi: 10.1038/s41423-020-0401-3
22. Westmeier J, Paniskaki K, Karaköse Z, Werner T, Sutter K, Dolff S, et al. Impaired cytotoxic CD8(+) T cell response in elderly COVID-19 patients. mBio (2020) 11:e02243–20. doi: 10.1128/mBio.02243-20
23. Martonik D, Parfieniuk-Kowerda A, Rogalska M, Flisiak R. The role of Th17 response in COVID-19. Cells (2021) 10:1550. doi: 10.3390/cells10061550
24. Oja AE, Saris A, Ghandour CA, Kragten NAM, Hogema BM, Nossent EJ, et al. Divergent SARS-CoV-2-specific T- and b-cell responses in severe but not mild COVID-19 patients. Eur J Immunol (2020) 50:1998–2012. doi: 10.1002/eji.202048908
25. Kalfaoglu B, Almeida-Santos J, Tye CA, Satou Y, Ono M. T-Cell dysregulation in COVID-19. Biochem Biophys Res Commun (2021) 538:204–10. doi: 10.1016/j.bbrc.2020.10.079
26. Yang L, Liu S, Liu J, Zhang Z, Wan X, Huang B, et al. COVID-19: immunopathogenesis and immunotherapeutics. Signal Transduct Target Ther (2020) 5:128–8. doi: 10.1038/s41392-020-00243-2
27. Maecker HT. Immune profiling of COVID-19: Preliminary findings and implications for the pandemic. J Immunother Cancer (2021) 9:e002550. doi: 10.1136/jitc-2021-002550
28. Carsetti R, Zaffina S, Piano Mortari E, Terreri S, Corrente F, Capponi C, et al. Different innate and adaptive immune responses to SARS-CoV-2 infection of asymptomatic, mild, and severe cases. Front Immunol (2020) 11:610300. doi: 10.3389/fimmu.2020.610300
29. Sterlin D, Mathian A, Miyara M, Mohr A, Anna F, Claër L, et al. IgA dominates the early neutralizing antibody response to SARS-CoV-2. Sci Transl Med (2021) 13:eabd2223. doi: 10.1126/scitranslmed.abd2223
30. Trinité B, Tarrés-Freixas F, Rodon J, Pradenas E, Urrea V, Marfil S, et al. SARS-CoV-2 infection elicits a rapid neutralizing antibody response that correlates with disease severity. Sci Rep (2021) 11:2608. doi: 10.1038/s41598-021-81862-9
31. Rezaei M, Mahmoudi S, Mortaz E, Marjani M. Immune cell profiling and antibody responses in patients with COVID-19. BMC Infect Dis (2021) 21:646–6. doi: 10.1186/s12879-021-06278-2
32. Gao T, Hu M, Zhang X, Li H, Zhu L, Liu H, et al. Highly pathogenic coronavirus n protein aggravates lung injury by MASP-2-mediated complement over-activation. medRxiv (2020) 2020:3.29.20041962. doi: 10.1101/2020.03.29.20041962
33. Mateus J, Grifoni A, Tarke A, Sidney J, Ramirez SI, Dan JM, et al. Selective and cross-reactive SARS-CoV-2 T cell epitopes in unexposed humans. Science (2020) 370:89–94. doi: 10.1126/science.abd3871
34. Tan AT, Linster M, Tan CW, Le Bert N, Chia WN, Kunasegaran K, et al. Early induction of functional SARS-CoV-2-specific T cells associates with rapid viral clearance and mild disease in COVID-19 patients. Cell Rep (2021) 34:108728–8. doi: 10.1016/j.celrep.2021.108728
35. Sette A, Crotty S. Adaptive immunity to SARS-CoV-2 and COVID-19. Cell (2021) 184:861–80. doi: 10.1016/j.cell.2021.01.007
36. Bezemer GFG, Garssen J. TLR9 and COVID-19: A multidisciplinary theory of a multifaceted therapeutic target. Front Pharmacol (2021) 11:601685. doi: 10.3389/fphar.2020.601685
37. Wang Z, Yang X, Zhong J, Zhou Y, Tang Z, Zhou H, et al. Exposure to SARS-CoV-2 generates T-cell memory in the absence of a detectable viral infection. Nat Commun (2021) 12:1724. doi: 10.1038/s41467-021-22036-z
38. Yang HS, Costa V, Racine-Brzostek SE, Acker KP, Yee J, Chen Z, et al. Association of age with SARS-CoV-2 antibody response. JAMA Netw Open (2021) 4:e214302–e214302. doi: 10.1001/jamanetworkopen.2021.4302
39. Lagunas-Rangel FA, Chávez-Valencia V. What do we know about the antibody responses to SARS-CoV-2? Immunobiology (2021) 226:152054–4. doi: 10.1016/j.imbio.2021.152054
40. Quast I, Tarlinton D. B cell memory: Understanding COVID-19. Immunity (2021) 54:205–10. doi: 10.1016/j.immuni.2021.01.014
41. Shomuradova AS, Vagida MS, Sheetikov SA, Zornikova KV, Kiryukhin D, Titov A, et al. SARS-CoV-2 epitopes are recognized by a public and diverse repertoire of human T cell receptors. Immunity (2020) 53:1245–57.e5. doi: 10.1016/j.immuni.2020.11.004
42. Wei J, Zhao J, Han M, Meng F, Zhou J. SARS-CoV-2 infection in immunocompromised patients: Humoral versus cell-mediated immunity. J Immunother Cancer (2020) 8:e000862. doi: 10.1136/jitc-2020-000862
43. Tavukcuoglu E, Horzum U, Cagkan Inkaya A, Unal S, Esendagli G. Functional responsiveness of memory T cells from COVID-19 patients. Cell Immunol (2021) 365:104363–3. doi: 10.1016/j.cellimm.2021.104363
44. Schöllhorn A, Schuhmacher J, Besedovsky L, Fendel R, Jensen ATR, Stevanović S, et al. Integrin activation enables sensitive detection of functional CD4(+) and CD8(+) T cells: Application to characterize SARS-CoV-2 immunity. Front Immunol (2021) 12:626308. doi: 10.3389/fimmu.2021.626308
45. Rockstroh A, Wolf J, Fertey J, Kalbitz S, Schroth S, Lübbert C, et al. Correlation of humoral immune responses to different SARS-CoV-2 antigens with virus neutralizing antibodies and symptomatic severity in a German COVID-19 cohort. Emerg Microbes Infect (2021) 10:774–81. doi: 10.1080/22221751.2021.1913973
46. Lee E, Oh JE. Humoral immunity against SARS-CoV-2 and the impact on COVID-19 pathogenesis. Mol Cells (2021) 44:392–400. doi: 10.14348/molcells.2021.0075
47. Verkerke H, Saeedi BJ, Boyer D, Allen JW, Owens J, Shin S, et al. Are we forgetting about IgA? a re-examination of coronavirus disease 2019 convalescent plasma. Transfusion (2021) 61:1740–8. doi: 10.1111/trf.16435
48. Cao Y, Yisimayi A, Bai Y, Huang W, Li X, Zhang Z, et al. Humoral immune response to circulating SARS-CoV-2 variants elicited by inactivated and RBD-subunit vaccines. Cell Res (2021) 31:732–41. doi: 10.1038/s41422-021-00514-9
49. Renner K, Schwittay T, Chaabane S, Gottschling J, Müller C, Tiefenböck C, et al. Severe T cell hyporeactivity in ventilated COVID-19 patients correlates with prolonged virus persistence and poor outcomes. Nat Commun (2021) 12:3006–6. doi: 10.1038/s41467-021-23334-2
50. Grifoni A, Sidney J, Vita R, Peters B, Crotty S, Weiskopf D, et al. SARS-CoV-2 human T cell epitopes: Adaptive immune response against COVID-19. Cell Host Microbe (2021) 29:1076–92. doi: 10.1016/j.chom.2021.05.010
51. Kalpakci Y, Hacibekiroglu T, Trak G, Karacaer C, Demirci T, Kocayigit H, et al. Comparative evaluation of memory T cells in COVID-19 patients and the predictive role of CD4+CD8+ double positive T lymphocytes as a new marker. Rev Assoc Med Bras (1992) (2020) 66:1666–72. doi: 10.1590/1806-9282.66.12.1666
52. Prompetchara E, Ketloy C, Palaga T. Immune responses in COVID-19 and potential vaccines: Lessons learned from SARS and MERS epidemic. Asian Pac J Allergy Immunol (2020) 38:1–9. doi: 10.12932/AP-200220-0772
53. Abers MS, Delmonte OM, Ricotta EE, Fintzi J, Fink DL, de Jesus AAA, et al. An immune-based biomarker signature is associated with mortality in COVID-19 patients. JCI Insight (2021) 6:e144455. doi: 10.1172/jci.insight.144455
54. Bunders MJ, Altfeld M. Implications of sex differences in immunity for SARS-CoV-2 pathogenesis and design of therapeutic interventions. Immunity (2020) 53:487–95. doi: 10.1016/j.immuni.2020.08.003
55. Takahashi T, Ellingson MK, Wong P, Israelow B, Lucas C, Klein J, et al. Sex differences in immune responses that underlie COVID-19 disease outcomes. Nature (2020) 588:315–20. doi: 10.1038/s41586-020-2700-3
56. Viveiros A, Rasmuson J, Vu J, Mulvagh SL, Yip CYY, Norris CM, et al. Sex differences in COVID-19: candidate pathways, genetics of ACE2, and sex hormones. Am J Physiol Heart Circ Physiol (2021) 320:H296–304. doi: 10.1152/ajpheart.00755.2020
57. Zheng M, Gao Y, Wang G, Song G, Liu S, Sun D, et al. Functional exhaustion of antiviral lymphocytes in COVID-19 patients. Cell Mol Immunol (2020) 17:533–5. doi: 10.1038/s41423-020-0402-2
58. Gomez-Lopez N, Romero R, Tao L, Gershater M, Leng Y, Zou C, et al. Distinct cellular immune responses to SARS-CoV-2 in pregnant women. JI (2022) 208:1857–72. doi: 10.4049/jimmunol.2101123
59. Laurén I, Havervall S, Ng H, Lord M, Pettke A, Greilert-Norin N, et al. Long-term SARS-CoV-2-specific and cross-reactive cellular immune responses correlate with humoral responses, disease severity, and symptomatology. Immun Inflam Dis (2022) 10:e595. doi: 10.1002/iid3.595
60. Havervall S, Jernbom Falk A, Klingström J, Ng H, Greilert-Norin N, Gabrielsson L, et al. SARS-CoV-2 induces a durable and antigen specific humoral immunity after asymptomatic to mild COVID-19 infection. PloS One (2022) 17:e0262169. doi: 10.1371/journal.pone.0262169
61. Viurcos-Sanabria R, Manjarrez-Reyna AN, Solleiro-Villavicencio H, Rizo-Téllez SA, Méndez-García LA, Viurcos-Sanabria V, et al. In vitro exposure of primary human T cells and monocytes to polyclonal stimuli reveals a basal susceptibility to display an impaired cellular immune response and develop severe COVID-19. Front Immunol (2022) 13:897995. doi: 10.3389/fimmu.2022.897995
62. Moore T, Hossain R, Doores KJ, Shankar-Hari M, Fear DJ. SARS-CoV-2-Specific memory b cell responses are maintained after recovery from natural infection and postvaccination. Viral Immunol (2022) 35:425–36. doi: 10.1089/vim.2022.0013
63. Moga E, Lynton-Pons E, Domingo P. The robustness of cellular immunity determines the fate of SARS-CoV-2 infection. Front Immunol (2022) 13:904686. doi: 10.3389/fimmu.2022.904686
64. Rovito R, Augello M, Ben-Haim A, Bono V, d’Arminio Monforte A, Marchetti G. Hallmarks of severe COVID-19 pathogenesis: A pas de deux between viral and host factors. Front Immunol (2022) 13:912336. doi: 10.3389/fimmu.2022.912336
65. Gurevich M, Zilkha-Falb R, Sonis P, Magalashvili D, Menascu S, Flechter S, et al. SARS-CoV-2 memory b and T cell profiles in mild COVID-19 convalescent patients. Int J Infect Dis (2022) 115:208–14. doi: 10.1016/j.ijid.2021.12.309
66. Björkander S, Du L, Zuo F, Ekström S, Wang Y, Wan H, et al. SARS-CoV-2–specific b- and T-cell immunity in a population-based study of young Swedish adults. J Allergy Clin Immunol (2022) 149:65–75.e8. doi: 10.1016/j.jaci.2021.10.014
67. Cui D, Tang Y, Jiang Q, Jiang D, Zhang Y, Lv Y, et al. Follicular helper T cells in the immunopathogenesis of SARS-CoV-2 infection. Front Immunol (2021) 12:731100. doi: 10.3389/fimmu.2021.731100
68. Aghbash PS, Eslami N, Shamekh A, Entezari-Maleki T, Baghi HB. SARS-CoV-2 infection: The role of PD-1/PD-L1 and CTLA-4 axis. Life Sci (2021) 270:119124–4. doi: 10.1016/j.lfs.2021.119124
69. Rosenberg SA. IL-2: The first effective immunotherapy for human cancer. JI (2014) 192:5451–8. doi: 10.4049/jimmunol.1490019
70. Abbas AK, Trotta E R, Simeonov D, Marson A, Bluestone JA. Revisiting IL-2: Biology and therapeutic prospects. Sci Immunol (2018) 3:eaat1482. doi: 10.1126/sciimmunol.aat1482
71. Rose-John S. IL-6 trans-signaling via the soluble IL-6 receptor: Importance for the pro-inflammatory activities of IL-6. Int J Biol Sci (2012) 8:1237–47. doi: 10.7150/ijbs.4989
72. Rincon M, Irvin CG. Role of IL-6 in asthma and other inflammatory pulmonary diseases. Int J Biol Sci (2012) 8:1281–90. doi: 10.7150/ijbs.4874
73. Lu Q, Zhu Z, Tan C, Zhou H, Hu Y, Shen G, et al. Changes of serum IL-10, IL-1β, IL-6, MCP-1, TNF-α, IP-10 and IL-4 in COVID-19 patients. Int J Clin Pract (2021) 75:e14462. doi: 10.1111/ijcp.14462
74. Akbari H, Tabrizi R, Lankarani KB, Aria H, Vakili S, Asadian F, et al. The role of cytokine profile and lymphocyte subsets in the severity of coronavirus disease 2019 (COVID-19): A systematic review and meta-analysis. Life Sci (2020) 258:118167. doi: 10.1016/j.lfs.2020.118167
75. Saghazadeh A, Rezaei N. Immune-epidemiological parameters of the novel coronavirus – a perspective. Expert Rev Clin Immunol (2020) 16:465–70. doi: 10.1080/1744666X.2020.1750954
76. Turner MD, Nedjai B, Hurst T, Pennington DJ. Cytokines and chemokines: At the crossroads of cell signalling and inflammatory disease. Biochim Biophys Acta (BBA) - Mol Cell Res (2014) 1843:2563–82. doi: 10.1016/j.bbamcr.2014.05.014
77. Tsao C-H, Shiau M-Y, Chuang P-H, Chang Y-H, Hwang J. Interleukin-4 regulates lipid metabolism by inhibiting adipogenesis and promoting lipolysis. J Lipid Res (2014) 55:385–97. doi: 10.1194/jlr.M041392
78. Lucas C, Wong P, Klein J, Castro TBR, Silva J, Sundaram M, et al. Longitudinal analyses reveal immunological misfiring in severe COVID-19. Nature (2020) 584:463–9. doi: 10.1038/s41586-020-2588-y
79. Kay AB, Ying S, Varney V, Gaga M, Durham SR, Moqbel R, et al. Messenger RNA expression of the cytokine gene cluster, interleukin 3 (IL-3), IL-4, IL-5, and granulocyte/macrophage colony-stimulating factor, in allergen-induced late-phase cutaneous reactions in atopic subjects. J Exp Med (1991) 173:775–8. doi: 10.1084/jem.173.3.775
80. Pala D, Pistis M. Anti-IL5 drugs in COVID-19 patients: role of eosinophils in SARS-CoV-2-induced immunopathology. Front Pharmacol (2021) 12:309. doi: 10.3389/fphar.2021.622554
81. Costela-Ruiz VJ, Illescas-Montes R, Puerta-Puerta JM, Ruiz C, Melguizo-Rodríguez L. SARS-CoV-2 infection: The role of cytokines in COVID-19 disease. Cytokine Growth Factor Rev (2020) 54:62–75. doi: 10.1016/j.cytogfr.2020.06.001
82. Pasquereau S, Kumar A, Herbein G. Targeting TNF and TNF receptor pathway in HIV-1 infection: from immune activation to viral reservoirs. Viruses (2017) 9:64. doi: 10.3390/v9040064
83. Guo Y, Hu K, Li Y, Lu C, Ling K, Cai C, et al. Targeting TNF-α for COVID-19: Recent advanced and controversies. Front Public Health (2022) 10:833967. doi: 10.3389/fpubh.2022.833967
84. Croft M. The role of TNF superfamily members in T-cell function and diseases. Nat Rev Immunol (2009) 9:271–85. doi: 10.1038/nri2526
85. Nodland SE, Berkowska MA, Bajer AA, Shah N, de Ridder D, van Dongen JJM, et al. IL-7R expression and IL-7 signaling confer a distinct phenotype on developing human b-lineage cells. Blood (2011) 118:2116–27. doi: 10.1182/blood-2010-08-302513
86. Pellegrini M, Calzascia T, Toe JG, Preston SP, Lin AE, Elford AR, et al. IL-7 engages multiple mechanisms to overcome chronic viral infection and limit organ pathology. Cell (2011) 144:601–13. doi: 10.1016/j.cell.2011.01.011
87. Mackall CL, Fry TJ, Gress RE. Harnessing the biology of IL-7 for therapeutic application. Nat Rev Immunol (2011) 11:330–42. doi: 10.1038/nri2970
88. Pourgholaminejad A, Pahlavanneshan S, Basiri M. COVID-19 immunopathology with emphasis on Th17 response and cell-based immunomodulation therapy: Potential targets and challenges. Scandinavian J Immunol (2022) 95:e13131. doi: 10.1111/sji.13131
89. Kandikattu HK, Venkateshaiah SU, Kumar S, Mishra A. IL-15 immunotherapy is a viable strategy for COVID-19. Cytokine Growth Factor Rev (2020) 54:24–31. doi: 10.1016/j.cytogfr.2020.06.008
90. Jarlhelt I, Nielsen SK, Jahn CXH, Hansen CB, Pérez-Alós L, Rosbjerg A, et al. SARS-CoV-2 antibodies mediate complement and cellular driven inflammation. Front Immunol (2021) 12:767981. doi: 10.3389/fimmu.2021.767981
91. Ferreira-Gomes M, Kruglov A, Durek P, Heinrich F, Tizian C, Heinz GA, et al. SARS-CoV-2 in severe COVID-19 induces a TGF-β-dominated chronic immune response that does not target itself. Nat Commun (2021) 12:1961. doi: 10.1038/s41467-021-22210-3
92. Larsen MD, de Graaf EL, Sonneveld ME, Plomp HR, Nouta J, Hoepel W, et al. Afucosylated IgG characterizes enveloped viral responses and correlates with COVID-19 severity. Science (2021) 371:eabc8378. doi: 10.1126/science.abc8378
93. Ciarambino T, Para O, Giordano M. Immune system and COVID-19 by sex differences and age. Womens Health (Lond Engl) (2021) 17:17455065211022262. doi: 10.1177/17455065211022262
94. Silva MJA, Rodrigues YC, Lima KVB, Lima LNGC. Innate immunity to SARS-CoV-2 infection: a review. Epidemiol Infect (2022) 150:e142. doi: 10.1017/S095026882200125X
Keywords: immunity, adaptive immunity, SARS-CoV-2, COVID-19, humoral immunity
Citation: Silva MJA, Ribeiro LR, Lima KVB and Lima LNGC (2022) Adaptive immunity to SARS-CoV-2 infection: A systematic review. Front. Immunol. 13:1001198. doi: 10.3389/fimmu.2022.1001198
Received: 23 July 2022; Accepted: 26 September 2022;
Published: 10 October 2022.
Edited by:
Gerardo Guillen, Center for Genetic Engineering and Biotechnology (CIGB), CubaReviewed by:
Hongjing Gu, Beijing Institute of Microbiology and Epidemiology, ChinaWenxiao Gong, Center for Disease Control and Prevention of Guangming District, China
Arjun Sharma, Boston University, United States
Copyright © 2022 Silva, Ribeiro, Lima and Lima. This is an open-access article distributed under the terms of the Creative Commons Attribution License (CC BY). The use, distribution or reproduction in other forums is permitted, provided the original author(s) and the copyright owner(s) are credited and that the original publication in this journal is cited, in accordance with accepted academic practice. No use, distribution or reproduction is permitted which does not comply with these terms.
*Correspondence: Marcos Jessé Abrahão Silva, amVzc2VhYnJhaGFvMTBAZ21haWwuY29t