- Infection and Innate Immunity Laboratory, Department of Biological Sciences, The George Washington University, Washington, DC, United States
The multifaceted functions ranging from cellular and developmental mechanisms to inflammation and immunity have rendered TGF-ß signaling pathways as critical regulators of conserved biological processes. Recent studies have indicated that this evolutionary conserved signaling pathway among metazoans contributes to the Drosophila melanogaster anti-nematode immune response. However, functional characterization of the interaction between TGF-ß signaling activity and the mechanisms activated by the D. melanogaster immune response against parasitic nematode infection remains unexplored. Also, it is essential to evaluate the precise effect of entomopathogenic nematode parasites on the host immune system by separating them from their mutualistic bacteria. Here, we investigated the participation of the TGF-ß signaling branches, activin and bone morphogenetic protein (BMP), to host immune function against axenic or symbiotic Heterorhabditis bacteriophora nematodes (parasites lacking or containing their mutualistic bacteria, respectively). Using D. melanogaster larvae carrying mutations in the genes coding for the TGF-ß extracellular ligands Daw and Dpp, we analyzed the changes in survival ability, cellular immune response, and phenoloxidase (PO) activity during nematode infection. We show that infection with axenic H. bacteriophora decreases the mortality rate of dpp mutants, but not daw mutants. Following axenic or symbiotic H. bacteriophora infection, both daw and dpp mutants contain only plasmatocytes. We further detect higher levels of Dual oxidase gene expression in dpp mutants upon infection with axenic nematodes and Diptericin and Cecropin gene expression in daw mutants upon infection with symbiotic nematodes compared to controls. Finally, following symbiotic H. bacteriophora infection, daw mutants have higher PO activity relative to controls. Together, our findings reveal that while D. melanogaster Dpp/BMP signaling activity modulates the DUOX/ROS response to axenic H. bacteriophora infection, Daw/activin signaling activity modulates the antimicrobial peptide and melanization responses to axenic H. bacteriophora infection. Results from this study expand our current understanding of the molecular and mechanistic interplay between nematode parasites and the host immune system, and the involvement of TGF-ß signaling branches in this process. Such findings will provide valuable insight on the evolution of the immune role of TGF-ß signaling, which could lead to the development of novel strategies for the effective management of human parasitic nematodes.
Introduction
Transforming growth factor (TGF-ß) signaling is an evolutionary conserved signaling pathway among metazoans (1). Having components present in all animals studied to date, TGF-ß signaling has been regarded as a key pathway in metazoan evolution and in transition to multicellularity (1, 2). In addition, its role has been implicated in various biological processes ranging from development to metabolism (3). Compared to vertebrates, TGF-ß signaling in Drosophila melanogaster comprises fewer representatives of each signaling component, yet still regulates diverse functions including axis formation, body patterning, and morphogenesis (4–7). Activin and bone morphogenetic protein (BMP) are the two signaling branches of the D. melanogaster TGF-ß pathway, which are characterized by extracellular ligands, type I and type II receptors, and intracellular transducers (8). Signaling is initiated by the binding of ligands to transmembrane receptor complex of serine/threonine kinases, which in turn phosphorylates transcription factors that regulate the activation of downstream genes (9).
In addition to its contribution to wounding response and anti-bacterial immunity, the role of TGF-ß signaling has been implicated in anti-nematode immunity in D. melanogaster (10–14). In particular, gene transcript levels of the ligands Daw (activin branch) and Dpp (BMP branch) are upregulated following infection with the parasitic nematodes, Heterorhabditis gerrardi and Heterorhabditis bacteriophora in adult flies (12). Inactivation of dpp leads to increased survival ability and induction of humoral immunity against H. bacteriophora infection (11). Also, in response to H. gerrardi infection, daw mutants have decreased expression of Dual oxidase (Duox) compared to their background controls (14). However, detailed functional characterization of the interaction between TGF-ß signaling activity and cellular, humoral, and melanization responses in D. melanogaster against parasitic nematode infection remains incomplete.
The entomopathogenic nematode (EPN) H. bacteriophora and its mutualistic bacterium P. luminescens constitute an excellent model to elucidate the interaction between TGF-ß signaling and host anti-nematode immunity. The nematodes and their bacteria, together or separately, can infect and kill a variety of insect species (15). Infective juveniles (IJs), the only free-living stage of the nematodes, enter the insect hemocoel either through natural openings or by using their special appendage to penetrate through the cuticle (16). Upon entry, IJs regurgitate their Photorhabdus bacteria into the hemolymph to spread into the host and overcome the insect immune response (17, 18). Once the insect dies and resources in the cadaver are depleted, IJs emerge from the carcass to search for new prey (15) D. melanogaster is not a natural host of H. bacteriophora (19). However, previous studies have established methods for infecting D. melanogaster larvae and adult flies with Heterorhabditis nematodes, which can develop efficiently in both stages of the host (19, 20).
Although previous studies have shown that TGF-ß signaling interacts with the D. melanogaster larval and adult immune response during infection with the Heterorhabditis-Photorhabdus complex, it is crucial to elucidate the precise effect of the parasites on the host immune system by separating them from their mutualistic bacteria (11–14). To this end, we investigated the participation of the two TGF-ß signaling branches, activin and BMP, in host immune function against axenic (lacking P. luminescens) or symbiotic (containing P. luminescens) H. bacteriophora nematodes. Using D. melanogaster larvae carrying mutations in the genes coding for the TGF-ß extracellular ligands Daw and Dpp, we explored modifications in cellular, humoral, and melanization responses during infection with these pathogens.
In this study, we show that BMP signaling activity promotes survival to D. melanogaster larvae when infected by symbiotic H. bacteriophora but increases mortality to larvae infected by axenic nematodes. Also, following axenic H. bacteriophora infection, BMP signaling activity leads to reduced DUOX expression and increased Diptericin and Drosomycin antimicrobial gene expression. Similarly, activin signaling activity upregulates Drosomycin expression against axenic nematode infection but downregulates Diptericin and Cecropin expression against symbiotic nematode infection. Finally, we demonstrate that in response to symbiotic H. bacteriophora infection, activin signaling activity lowers PO activity in D. melanogaster larvae. Taken together our findings provide a detailed characterization of the D. melanogaster activin and BMP signaling activity regulation in relation to host immune function against parasitic nematodes deficient of their mutualistic bacteria. The information garnered from these results set the stage for elucidating the evolution of the immune role of TGF-ß signaling against parasitic nematode infection and contribute to the effective use of Heterorhabditis nematodes as models for human parasitic diseases.
Materials and Methods
Fly and Nematode Stocks
All flies were reared on Bloomington Drosophila Stock Center cornmeal food (Labexpress) supplemented with yeast (Carolina Biological Supply), maintained at 25°C, and a 12:12-h light:dark photoperiodic cycle. Larvae carrying a spontaneous dpps1 mutation (strain 397, Bloomington, IL, USA) and carrying P-bac insertion Pbac{XP}daw05680 (strain d05680, Exelixis, Boston, MA, USA) were used, as previously described (14). Line w1118 (strain 3605, Bloomington, IL, USA) was used as the background control. Infective juveniles (IJs) of H. bacteriophora strain TT01 were amplified in larvae of the wax moth Galleria mellonella using the water trap technique (21). To generate axenic H. bacteriophora nematodes lacking their P. luminescens bacteria, a previously established protocol was followed (22). Prior to experiments, axenic nematodes were surface sterilized in 5% bleach solution and rinsed five times with water to remove the bleach residue. Nematodes were used 1-4 weeks after collection.
Larval Infection and Survival Assay
Infections were carried out using 96-well plates containing 100 μL of 1.25% agarose in each well. The suspension of axenic or symbiotic H. bacteriophora IJs (100 nematodes in 10 μL of sterile distilled water) was added to a single larva (late second to third instar) in each well. Sterile distilled water (10 μL) served as uninfected control. The plate was covered with a sealing film (USA Scientific, Ocala, FL, USA) and air holes were poked for ventilation. Plates were kept in the dark at room temperature for 24 h. At the 24-h time point, larvae were collected and frozen at −80°C, or immediately used in experiments. The survival of larvae kept in nematode solution or in sterile distilled water was estimated at 12-h intervals and up to 60 h. The survival experiments were replicated three times.
Gene Expression Analysis
RNA was extracted from 4-5 D. melanogaster larvae (late second to third instar) using Trizol reagent (Ambion, Life Technologies) and reverse transcription was performed using High-Capacity cDNA Reverse Transcription Kit (Applied Biosystems) according to the manufacturers’ instructions. Real time PCR experiments were conducted in a CFX96 Real-Time System, C1000 Thermal Cycler (Bio-Rad) with gene-specific primers (Table 1) using GreenLink qPCR Mix (BioLink). Each experiment was run in technical triplicates and repeated three times.
Hemocyte Assays
Following axenic or symbiotic H. bacteriophora infection, 10 D. melanogaster larvae (late second to third instar) were bled into 30 μl of 2.5× protease inhibitor cocktail (Sigma). Hemolymph samples were loaded on a hemocytometer and total numbers and aggregates of cells were counted using 40× magnification on a compound microscope (Olympus CX21). Clumps of two or more hemocytes were considered as aggregates and each clump was counted as one aggregate. In each experiment five technical replicates were used, and each experiment was repeated three times.
Hemocyte Staining and Microscopy
To distinguish the different types of hemocytes following infection with axenic or symbiotic H. bacteriophora, six D. melanogaster larvae (late second to third instar) were washed in 1xPBS and bled into 30 μl of 1x PBS on a poly-lysine coated microscope slide which was air dried for 20 min. Hemocytes were fixed in 4% paraformaldehyde for 15 min, washed with 1xPBS, permeabilized in PBST (0.3% Triton in 1x PBS) for 20 min, and washed again in 1x PBS. Hemocytes were blocked in PBSTB (8% BSA in PBST) for 15 min, washed in 1x PBS, and then incubated with diluted phalloidin TRITC (1:100) for 20 min before a final wash in 1x PBS. Using ProLong® Gold Antifade reagent with DAPI, hemocytes were mounted and stored at 4°C until imaging. Hemocytes were visualized using Zeiss LSM 510 confocal microscope and their types were distinguished based on morphology, as previously described (23). The experiment was repeated three times.
Nitric Oxide and Aconitase Assays
To assess levels of Nitric Oxide (NO) and aconitase activity, six late second to third instar D. melanogaster larvae were collected 24-h post-infection with axenic or symbiotic H. bacteriophora. For NO estimation, larvae were homogenized in PBS and centrifuged at 10,000 x g for 10 min at 4°C. The resultant supernatant was used to quantify proteins as previously described and then mixed 1:1 with Griess reagent (Sigma) (1). Absorbance was measured at 595 nm using a plate reader (BioTek). To calculate NO levels, a silver nitrite standard curve was used. For the estimation of aconitase activity, larvae were homogenized in aconitase assay buffer and processed following the manufacturer’s instructions (MAK051-1KT; Sigma). Absorbance was measured at 450 nm and levels of aconitase activity were calculated from an isocitrate standard curve. Experiments were conducted in technical duplicates and repeated three times.
Phenoloxidase Assay
To collect hemolymph following infection with axenic or symbiotic H. bacteriophora, 10 larvae from each D. melanogaster line were bled into 30 μl of 2.5x protease inhibitor. Hemolymph samples were added to Pierce® Spin Columns and spun at 4°C and 13,000 rpm for 10 min. Protein concentrations were measured using the Pierce™ BCA Protein Assay Kit (Thermo Fisher Scientific). A mixture containing 15 μg of protein, 5 mM CaCl2, and 2.5× protease inhibitor was added to 160 μl of fresh L-DOPA solution (in phosphate buffer, pH 6.6) in a clear microplate well. Absorbance values were measured at 29°C and 492 nm for 60 min. Absorbance of the blank was subtracted from the absorbance of each sample. Each experiment was repeated three times.
Assessment of Melanization
To observe the ability of hemolymph to melanize following infection with axenic or symbiotic H. bacteriophora, hemolymph samples were collected from 10 larvae of each D. melanogaster line in 1xPBS, added to a Pierce® Spin Column and spun at 4°C and 13,000 rpm for 10 min. Hemolymph plasma samples were transferred to a 96 well microtiter plate. After 3 h incubation at room temperature, the presence of melanization in the wells was noted.
Statistical Analysis
GraphPad Prism 8 was used for data plotting and statistical analyses. Statistics for the results from the survival experiments were carried out using log-rank (Mantel-Cox) test. One-way analysis of variance (ANOVA) and Tukey post-hoc tests were used for analyzing the results from the rest of the experiments.
Results
BMP Branch Activity Promotes Survival of D. melanogaster Larvae Upon Symbiotic H. bacteriophora Infection
To understand whether activin and BMP TGF-ß signaling branches contribute to the survival ability of D. melanogaster larvae against infection with H. bacteriophora containing or lacking their mutualistic bacteria, we challenged daw and dpp mutants with either type of nematode to assess their time-course survival rate following infection. For this, we monitored larval survival every 12 h and up to 48 h post nematode infection. We did not observe any significant differences between daw mutants and their background controls (w1118) following axenic or symbiotic H. bacteriophora infection (Figure 1A). However, we found that while dpp mutants showed higher survival rates upon axenic H. bacteriophora infection, their survival ability to symbiotic nematode infection decreased compared to w1118 controls (Figure 1B). These results indicate that the BMP signaling activity in D. melanogaster larvae promotes survival against symbiotic H. bacteriophora infection but limits survival against axenic H. bacteriophora infection.
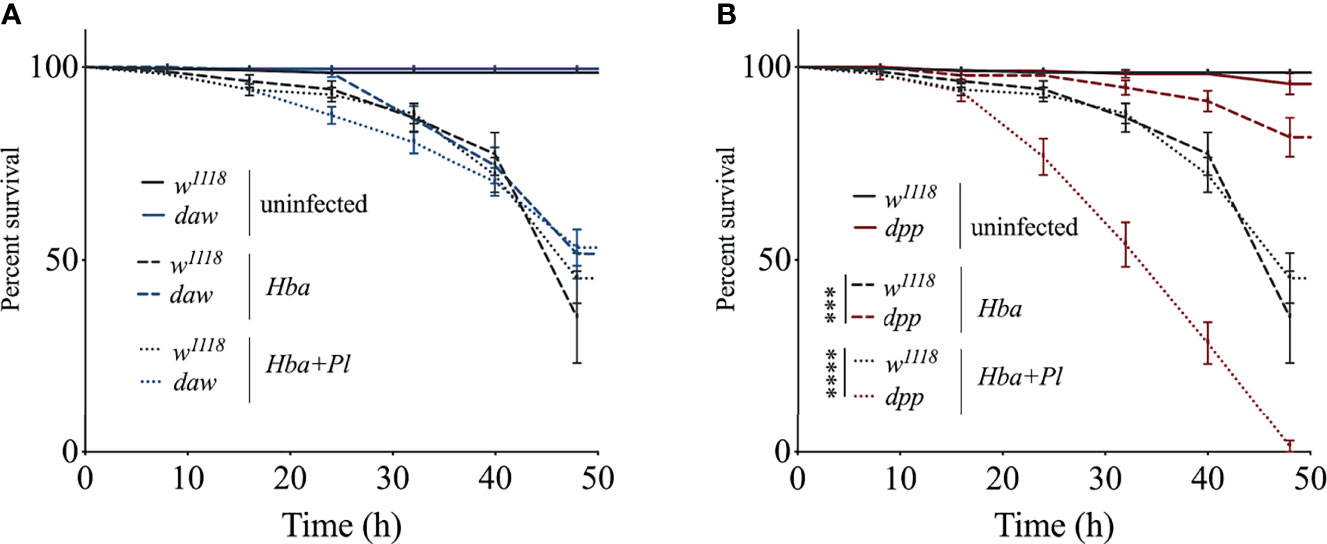
Figure 1 Survival analysis of Drosophila melanogaster TGF-ß mutant larvae upon infection with axenic (Hba) or symbiotic (Hba+Pl) Heterorhabditis bacteriophora. Daw and dpp mutant larvae and their background controls (w1118) were infected with either H. bacteriophora axenic or symbiotic nematodes. Treatment with water served as negative control (uninfected). Larval survival was estimated every 12 h and up to 48 h after infection. Significance levels were assessed using log-rank (Mantel-Cox) test. Survival ability of daw (A) and dpp (B) mutants following infection with axenic or symbiotic H. bacteriophora (***p = 0.0002, ****p < 0.0001).
Activin and BMP Signaling Activity Do Not Participate in the D. melanogaster Larval Cellular Immune Response to H. bacteriophora Infection
The cellular immune response is mediated by the hemocytes and forms an integral part of the D. melanogaster host defense because it acts in conjunction with humoral immune mechanisms to oppose infection (24, 25). Previous studies have linked the number of hemocytes to immune system capacity in D. melanogaster (26–29). To determine whether activin and BMP signaling activity modulate the number of hemocytes in D. melanogaster larvae responding to parasitic nematode infection, we counted the number of circulating hemocytes in daw and dpp mutants following infection with axenic or symbiotic H. bacteriophora. Of note, here we focused only on circulating hemocytes, but there is a possibility that some of the sessile hemocytes may have become circulating to act on the anti-nematode response. We found that the number of hemocytes was not significantly affected in TGF-ß mutants when infected with either type of nematode compared to their background controls. However, hemocyte numbers decreased in dpp mutants following infection with axenic H. bacteriophora relative to uninfected dpp individuals suggesting a link between hemocyte proliferation and BMP signaling activity (Figure 2A). Wasp parasitization in D. melanogaster larvae induces the differentiation of lamellocytes, which are specialized type of hemocytes (29–32). Previous evidence suggests that infection with the EPN Steinernema carpocapsae also leads to lamellocyte differentiation in D. melanogaster wild-type larvae (23). To elucidate whether activin and BMP signaling branches in D. melanogaster larvae are involved in the differentiation of lamellocytes during H. bacteriophora infection, we stained hemocytes in daw and dpp mutants and their w1118 background controls with phalloidin-TRITC and DAPI and visualized them using confocal microscopy (Figure 2B). We only detected plasmatocytes in either TGF-ß mutant following infection with axenic or symbiotic H. bacteriophora nematodes. Interestingly, we noticed the formation of a higher number of hemocyte aggregates in uninfected daw mutants compared to their background controls. To quantitively assess whether inactivating TGF-ß signaling branches leads to changes in hemocyte aggregation in the context of nematode infection, we counted the number of hemocyte aggregates in daw and dpp mutants following infection with axenic or symbiotic H. bacteriophora. Consistent with the initial observation, we found that in the absence of infection, there was a higher number of hemocyte aggregates in daw mutants compared to those detected in w1118 individuals. In addition, following axenic H. bacteriophora infection, the number of cell aggregates decreased in daw mutant larvae compared to their uninfected counterparts (Figure 2C). These findings suggest that activin and BMP signaling activity do not affect cellular immune processes in D. melanogaster larvae, such as total hemocyte numbers, differentiation of lamellocytes, and formation of hemocyte aggregates upon H. bacteriophora infection.
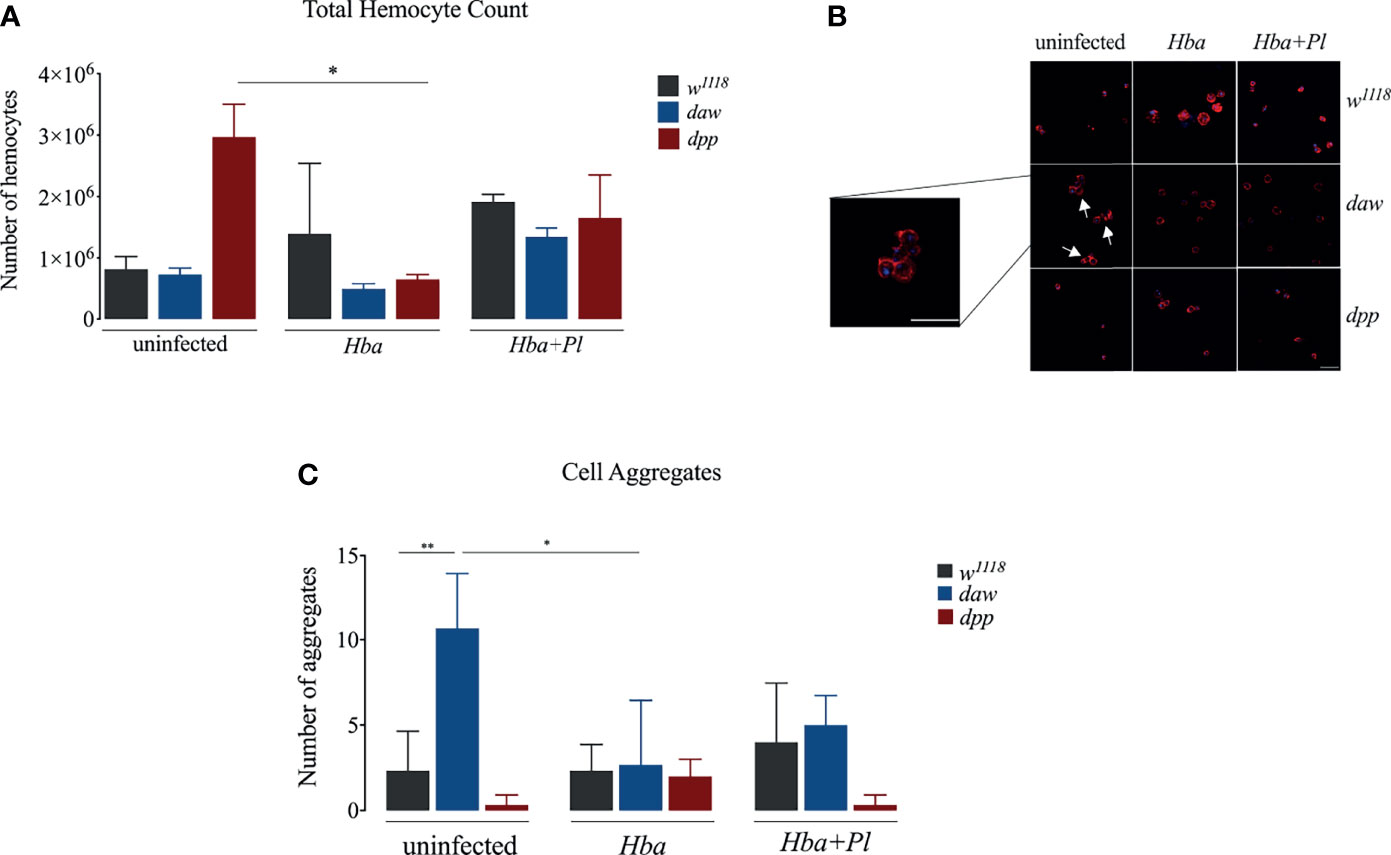
Figure 2 Cellular immune response of Drosophila melanogaster TGF-ß mutant larvae against axenic (Hba) or symbiotic (Hba+Pl) Heterorhabditis bacteriophora nematode infection. (A) Total number of circulating hemocytes in D. melanogaster daw and dpp mutant larvae and their background controls (w1118) upon infection with either axenic or symbiotic nematodes. Hemolymph samples were collected from 10 larvae at 24 h following infection and the number of circulating hemocytes was assessed using a hemocytometer under a light microscope (*p = 0.0343). (B) Confocal microscopy images of hemocytes stained with Phalloidin-TRITC (red) and DAPI (blue) in D. melanogaster daw and dpp mutants and their background controls (w1118) following axenic or symbiotic H. bacteriophora nematode infection. Scale bar is 20 μm. White arrows indicate hemocyte aggregates. (C) Assessment of the number of hemocyte aggregates in D. melanogaster daw and dpp mutant larvae and their background controls (w1118) following H. bacteriophora axenic or symbiotic nematode infection (*p = 0.0123, **p = 0.0086). Significance levels were assessed using one-way analysis of variance (ANOVA).
BMP Signaling Activity in D. melanogaster Larvae Lowers Expression of DUOX Upon Axenic H. bacteriophora Infection
Similar to vertebrates, intermediates of both oxygen (ROI) and nitrogen (RNO) in D. melanogaster constitute a prominent defense strategy against bacterial infections (33). D. melanogaster has a single NADPH, Dual oxidase (DUOX), which acts as a principal factor in the production of reactive oxygen species (ROS) (34, 35). Recently, it was shown that D. melanogaster daw mutant larvae express DUOX at higher levels upon infection with H. gerrardi nematodes (14). To elucidate whether activin and BMP branches are involved in the D. melanogaster DUOX mediated ROS response to H. bacteriophora infection, we estimated the gene expression level of DUOX in daw and dpp mutant larvae following infection with either axenic or symbiotic nematodes. We found no significant difference in DUOX expression between daw mutants and w1118 controls when infected with either type of nematode (Figure 3A). Interestingly, dpp mutants expressed DUOX at higher levels compared to w1118 individuals during axenic but not symbiotic H. bacteriophora infection. This finding indicates that the BMP branch mediates ROS response through reducing the expression of DUOX upon infection with P. luminescens-deficient H. bacteriophora nematodes. We have also assessed ROS levels by measuring the relative aconitase activity, yet we have not observed any significant differences between daw and dpp mutants and w1118 controls upon infection with axenic or symbiotic H. bacteriophora (Figure S1A). We then investigated the association between nitric oxide (NO) response, which is controlled by the enzyme NO synthase (NOS), and TGF-ß signaling activity in the D. melanogaster anti-nematode response. For this, we performed qRT-PCR to determine the expression level of NOS and spectrophotometrically quantified nitrite protein levels in daw and dpp mutant larvae infected by either axenic or symbiotic H. bacteriophora. There were no statistically significant differences in NOS expression or nitrite protein levels between nematode infected TGF-ß mutants and the w1118 controls (Figures 3B and S1B). This outcome suggests that TGF-ß signaling activity in D. melanogaster larvae is not involved in the NO response to parasitic nematode infection.
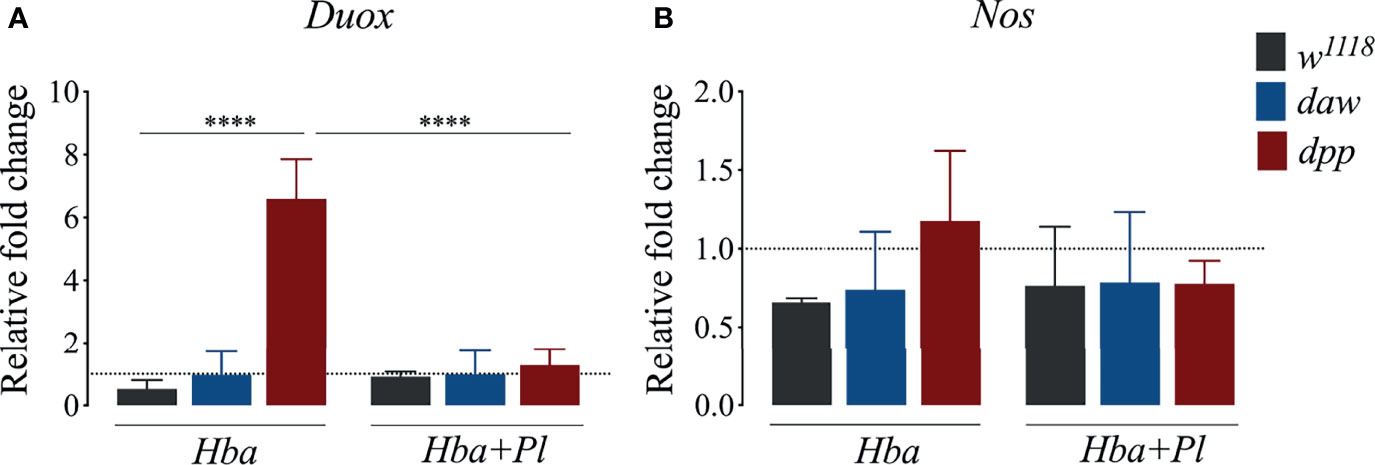
Figure 3 Quantitative PCR analysis of dual oxidase (DUOX) (A) and nitric oxide synthase (NOS) (B) expression in Drosophila melanogaster daw and dpp mutant larvae and their background controls (w1118) responding to infection by either axenic (Hba) or symbiotic (Hba + Pl) Heterorhabditis bacteriophora nematodes. Dotted line at 1.0 indicates normalization of fold change relative to uninfected controls. Significance levels were assessed using one-way analysis of variance (ANOVA, ****p < 0.0001).
Activin Signaling Activity Lowers Expression of Diptericin and Cecropin in D. melanogaster Larvae Following Infection With Symbiotic H. bacteriophora
The hallmark of the D. melanogaster host defense is the inducible synthesis and secretion of antimicrobial peptides (AMPs) through NF-κB signaling pathways (36). Also, using GFP reporter transgenes, infection of D. melanogaster larvae with symbiotic H. bacteriophora results in higher percentage of fly larvae expressing the AMP encoding genes Attacin, Diptericin, Drosomycin, and Metchnikowin (19). Previous studies have demonstrated a connection between TGF-ß signaling and synthesis of AMPs in the context of parasitic nematode infection (19, 37). In addition, NF-κB/Rel signaling interferes with the expression of Activinβ (activin signaling ligand), while NF-κB/Dif signaling interferes with the expression of glass bottom boat (BMP signaling ligand) in D. melanogaster larvae infected by H. gerrardi nematodes (37). To further study the link between TGF-ß signaling activity and expression of AMPs following infection with axenic or symbiotic H. bacteriophora nematodes, we used qRT-PCR and gene-specific primers to determine the expression levels of Diptericin, Cecropin, Drosomycin, and Metchnikowin in D. melanogaster daw and dpp mutants. We found that following symbiotic H. bacteriophora infection, expression of Diptericin and Cecropin were higher in daw mutant larvae compared to w1118 larvae (Figures 4A, B). Diptericin and Cecropin are mainly induced by Gram-negative bacterial infections (38). Therefore, our results indicate that these two AMPs were possibly induced by P. luminescens bacteria which are mutualistically associated with H. bacteriophora nematodes. Conversely, we found that Drosomycin expression significantly decreased in both daw and dpp mutants compared to w1118 controls upon infection with axenic H. bacteriophora (Figure 4C). We have not found any significant differences in Metchnikowin expression between TGF-ß mutants and their w1118 controls when infected with either axenic or symbiotic nematodes (Figure 4D). Taken together, these findings suggest that activin signaling activity interacts with the Imd pathway through decreasing the expression of Diptericin and Cecropin in D. melanogaster larvae during infection with H. bacteriophora nematodes harboring their mutualistic P. luminescens bacteria.
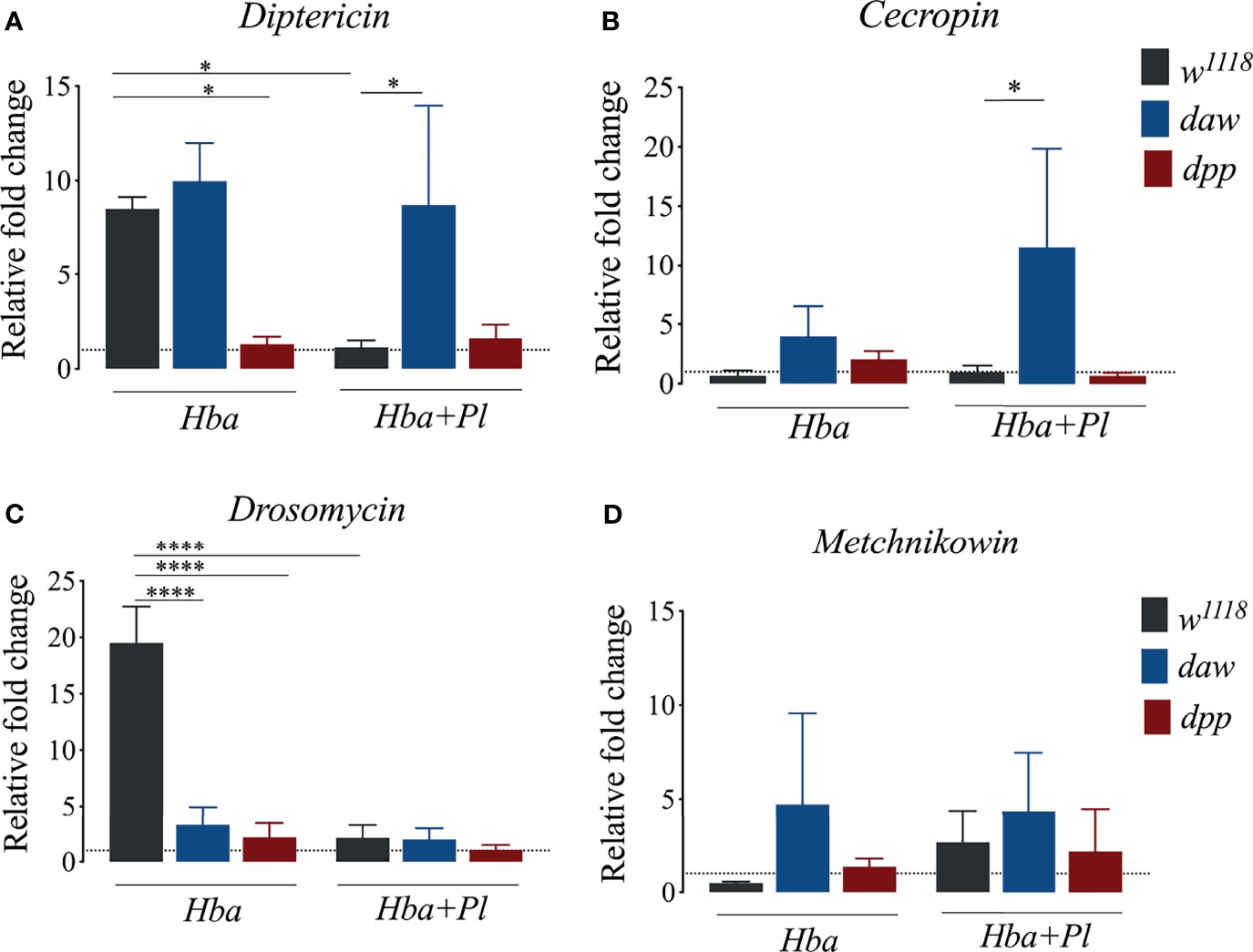
Figure 4 Expression of the antimicrobial peptide encoding genes Diptericin, Cecropin, Drosomycin, and Metchnikowin in Drosophila melanogaster daw and dpp mutant larvae and their background controls (w1118) following infection with either axenic (Hba) or symbiotic (Hba+Pl) Heterorhabditis bacteriophora. Dotted line at 1.0 indicates normalization of fold change relative to uninfected controls. Significance levels were assessed using one-way analysis of variance (ANOVA). (A) Diptericin (*p = 0.0180), (B) Cecropin (*p = 0.0318) (C) Drosomycin (****p < 0.0001), and (D) Metchnikowin expression via qPCR.
Activin Signaling Activity in D. melanogaster Larvae Reduces the Melanization Response Upon Symbiotic H. bacteriophora Infection
Melanization mediated by the enzyme phenoloxidase (PO) is an immediate and essential response to pathogen infection in D. melanogaster (39). When Daw/activin signaling is impaired in the absence of infection, melanotic tumors are observed in adult flies and PO enzyme activity increases in uninfected larvae (10, 14). Interestingly, daw mutant larvae have lower PO activity when challenged by H. gerrardi nematodes, suggesting an association between the activin branch and regulation of the melanization response against parasitic nematode infection (14). To examine the interaction between TGF-ß signaling activity in D. melanogaster and the melanization response to axenic or symbiotic H. bacteriophora, we estimated the expression levels of the inactive precursors of PO prophenoloxidase (PPO) genes PPO1, PPO2, and PPO3 in daw and dpp mutant larvae infected with either type of nematode (39). We did not observe any significant differences in PPO1 and PPO2 expression between the TGF-ß mutants and their w1118 controls following axenic or symbiotic H. bacteriophora infection (Figures S2A–C). However, expression of PPO3 increased significantly in dpp mutants compared to their background control following axenic nematode infection. The activity of PO enzyme in larval hemolymph can be measured spectrophotometrically through the detection of dopachrome which is converted by PO from L-DOPA (40). Using this method, we determined PO activity in the hemolymph plasma of daw and dpp mutants following infection with axenic or symbiotic H. bacteriophora. Daw mutants exhibited significantly increased PO activity compared to w1118 controls when infected with symbiotic H. bacteriophora, but not with axenic nematodes (Figure 5A). Extraction of hemolymph followed by incubation of the plasma at room temperature leads to blackening due to the production of melanin (41). We observed that hemolymph plasma from daw mutant larvae, which had previously been infected with symbiotic H. bacteriophora, melanized at room temperature (Figure 5B). However, we have not observed melanization in hemolymph plasma from dpp mutants or background controls following symbiotic H. bacteriophora infection. These findings collectively suggest that activin signaling activity regulates the melanization response in D. melanogaster larvae through reducing the levels of PO following symbiotic H. bacteriophora infection.
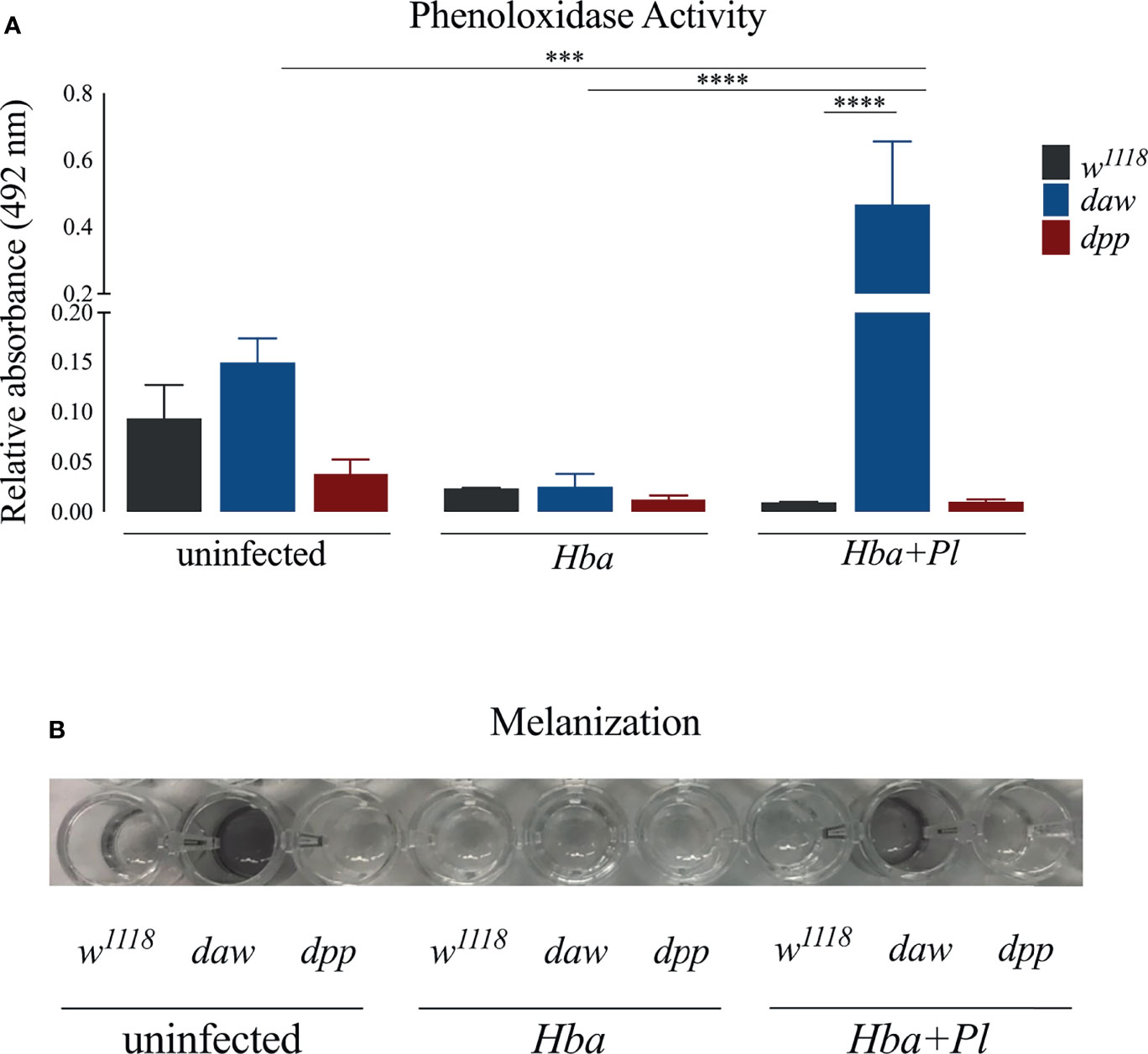
Figure 5 Assessment of phenoloxidase (PO) activity and melanization response in Drosophila melanogaster TGF-ß mutant larvae following infection with axenic (Hba) or symbiotic (Hba+Pl) Heterorhabditis bacteriophora. (A) PO activity in the hemolymph plasma of daw and dpp mutant larvae and their background controls (w1118) infected with either H. bacteriophora axenic or symbiotic nematodes. Significance levels were assessed using one-way analysis of variance (ANOVA), (***p = 0.0003; ****p < 0.0001). (B) Melanization of hemolymph plasma of daw and dpp mutant larvae and their background controls (w1118) infected with H. bacteriophora axenic or symbiotic nematodes one hour post incubation at room temperature.
Discussion
Previous studies have demonstrated that the conserved TGF-ß signaling pathway is induced in D. melanogaster larvae and adult flies during infection with EPNs (12, 13). Also, activin signaling activity decreases DUOX expression and increased PO activity in D. melanogaster larvae following infection with H. gerrardi symbiotic nematodes (14). Therefore, it is important to explore any additional roles that activin as well as BMP signaling may play in regulating cellular, humoral, and melanization immune responses in D. melanogaster during parasitic nematode infection. Identification of the precise molecular mechanisms underlying these processes, specifically against infection with the nematode parasites without any input from their mutualistic bacteria will contribute to a more comprehensive understanding of the host defense capability against parasitic diseases. Here, we used symbiotic together with axenic H. bacteriophora nematodes to examine the participation of the activin and the BMP signaling branches in the regulation of the D melanogaster anti-nematode immune response. We report that BMP signaling activity promotes survival against symbiotic H. bacteriophora infection but reduces survival against axenic nematode infection. Also, BMP signaling activity reduces DUOX expression but increases Diptericin and Drosomycin expression upon infection of D. melanogaster larvae with axenic H. bacteriophora. In addition, a functional activin pathway increases Drosomycin expression upon axenic nematode infection and decreases Diptericin and Cecropin expression upon symbiotic nematode infection. Finally, we report that activin signaling activity modulates the melanization response by reducing the PO enzyme levels upon symbiotic H. bacteriophora nematode parasitism (Figure 6).
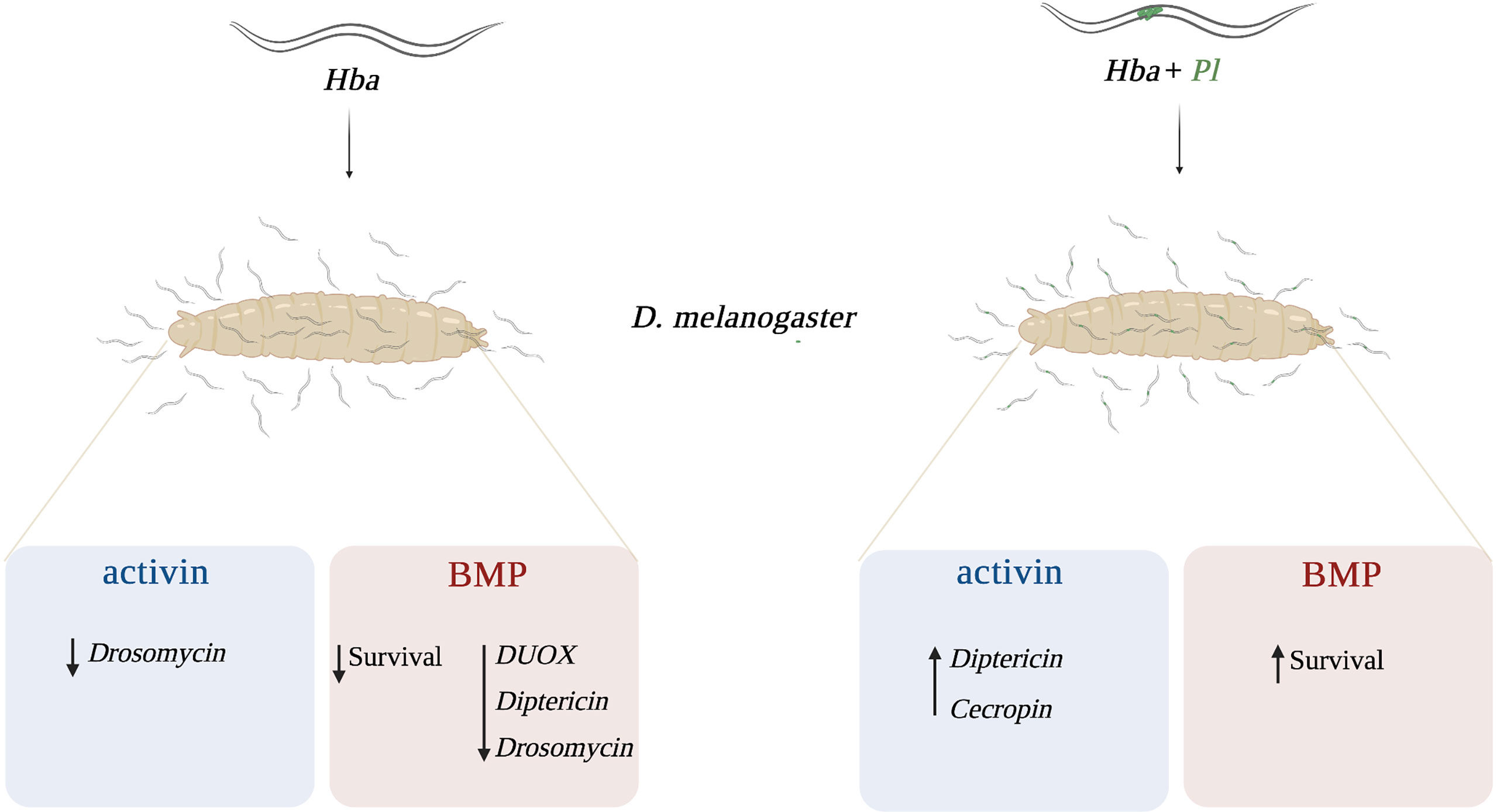
Figure 6 Proposed model of the interaction between activin and BMP signaling branches of the TGF-ß signaling pathway and Drosophila melanogaster immunity against Heterorhabditis bacteriophora parasitic nematodes. In response to axenic (Hba) H. bacteriophora infection, activin signaling activity reduces the expression of Drosomycin while BMP signaling activity reduces the survival ability and the expression of dual oxidase (DUOX), Diptericin, and Drosomycin. Following symbiotic (Hba + Pl) H. bacteriophora infection, activin signaling activity promotes the expression of Diptericin and Cecropin whereas BMP signaling activity promotes the survival of D. melanogaster larvae.
Heterorhabditis nematode invasion through the cuticle causes injury to the insect host and the wounding response, which is characterized by the loss of tissue integrity, is observed throughout the infection (42). Previous work has indicated that in D. melanogaster larvae, dpp is transcriptionally induced by injury and might have a role in attenuating immune responses following wounding and infection (10). In this study, we found that dpp mutant larvae survive better to axenic H. bacteriophora infection relative to their background controls. A possible explanation for this observation could be that the injury caused by IJs during entry into D. melanogaster larvae, which may lead to the activation of humoral and cellular immune reactions.
Humoral immunity in D. melanogaster is characterized by the synthesis and secretion of AMPs into the hemolymph through the activation of two NF-κB signaling pathways, Toll and Imd (36). While the Toll signaling is predominantly activated by Gram-positive bacteria and fungi, the Imd signaling is mainly activated by Gram-negative bacteria (43–46). The Imd pathway has also been previously implicated in the immune response of D. melanogaster to H. bacteriophora. More precisely, Diptericin, the readout AMP-encoding gene for the Imd pathway, is upregulated at 6 h post-injection of D. melanogaster adult flies and larvae with excreted secreted products (ESPs) isolated from H. bacteriophora axenic nematodes (22, 36). In addition, feeding of D. melanogaster larvae with P. luminescens bacteria also leads to upregulation of Diptericin (19). Interestingly, we have found that expression of Diptericin is lower in w1118 larvae (background control) following symbiotic H. bacteriophora infection compared to those infected with axenic nematodes. It is possible that at the 24 h time point, molecules produced by the nematode-bacteria pair during infection are able to suppress Diptericin expression. Another plausible explanation could be that Diptericin expression starts to decrease in larvae at 6 h post symbiotic H. bacteriophora infection, similar to Diptericin expression decrease in adult flies infected with Escherichia coli or Micrococcus luteus (47). We also demonstrate that Diptericin and Cecropin are upregulated following symbiotic H. bacteriophora infection in activin deficient larvae compared to the background control line. This implies that activin signaling activity interacts with the Imd pathway in D. melanogaster larvae during infection with the H. bacteriophora-P. luminescens complex to modify the AMP response. However, additional work is required to fully elucidate if Imd and activin signaling in D. melanogaster work synergistically against parasitic nematode infection. It would be interesting to distinguish the factors produced by the nematodes and their mutualistic bacteria to determine those that lead to Diptericin upregulation in daw mutants. The two virulence factors secreted by H. bacteriophora nematodes, Ecdysteroid glycosyltransferase Hba_07292 and Serine carboxypeptidase (Hba_11636) are able to suppress Diptericin expression in D. melanogaster larvae (40, 48). Investigating whether injection of these factors into daw mutant larvae is associated with any changes in Diptericin expression would provide insight about the impact of ESPs on activin-Imd interaction.
Previous work has shown that in the absence of infection, BMP signaling is regulated by Toll signaling activity via binding of Dorsal to Dpp and suppressing its expression in the ventral domain of the embryo (49). The interplay between these two signaling pathways is essential for D. melanogaster development (50). Here, we suggest that interaction between TGF-ß signaling branches and Toll signaling could be further extended to the immune response against parasitic nematode infection. This is particularly due to our observation of the reduced expression of Drosomycin in both daw and dpp mutants upon axenic H. bacteriophora infection. Our findings provide a basis for future investigations aimed at elucidating interactions between activin and BMP signaling activity with the Toll pathway during the D. melanogaster response to H. bacteriophora. Toll signaling receptors, GNBP1, PGRP-SA and PGRP-SD are upregulated following infection with symbiotic H. bacteriophora nematodes in D. melanogaster adult flies (36, 51). To identify potential interactions between TGF-ß and Toll signaling at the level of recognition, changes in the expression of daw and dpp following infection with H. bacteriophora nematodes or injection with ESPs can be studied in flies deficient of GNBP1, PGRP-SA or PGRP-SD. Moreover, to identify the interactions at downstream expression, flies deficient of the Toll pathway transcription factor Dif could be used to assess the expression of daw and dpp or other intracellular TGF-ß signaling components in the context of response to parasitic nematode infection (36). Lessons we learn through studying these interactions could be applicable to higher organisms, including humans, considering the similarities between innate immunity in D. melanogaster and mammals and the phylogenetic relationship between H. bacteriophora nematodes and vertebrate parasitic nematodes including Necator, Dictyocaulus, and Oslerus (52–54).
In our study we found that D. melanogaster activin deficient larvae have increased levels of PO as well as melanin formation in their hemolymph plasma compared to their background controls following infection with symbiotic H. bacteriophora. This finding suggests that activin signaling activity reduces the melanization response in D. melanogaster larvae upon symbiotic nematode infection. In contrast, we have not found any significant differences in daw mutants following axenic H. bacteriophora infection suggesting that activin signaling activity reduces the melanization response only when nematodes infect D. melanogaster larvae together with their mutualistic bacteria. Interestingly, a recent study has shown that following infection of daw mutant larvae with symbiotic H. gerrardi nematodes, PO activity remains at low levels (14). It is therefore possible that the property of functional activin signaling to decrease the melanization response in D. melanogaster larvae is restricted only to the response against the H. bacteriophora-P. luminescens complex. It would be interesting to further explore how other parasitic nematodes influence the interaction between activin signaling activity and the melanization response by testing the response of D. melanogaster to other EPNs such as S. carpocapsae. Infection of D. melanogaster larvae with S. carpocapsae nematodes leads to increased PO activity in the hemolymph (23). In contrast, inoculation of the S. carpocapsae mutualistic bacteria, Xenorhabdus nematophila, via pricking into D. suzukii larvae results in lower PO activity in the hemolymph (55). Based on this information, it would be interesting to test whether activin signaling activity has a role in regulating the D. melanogaster PO activity and melanization response against the S. carpocapsae-X. nematophila complex. In addition, considering the role of melanization in clot formation, another field of inquiry would be to study whether activin signaling interacts with the clotting response in D. melanogaster against parasitic nematode infection (56). Clotting factors such as Fondue, Eig71Ee, and transglutaminase protect D. melanogaster larvae against symbiotic H. bacteriophora infection (57, 58). Future studies could focus on understanding if this protection extends to the mutants of activin signaling suggesting an interaction between clotting and TGF-ß signaling activity during the D. melanogaster response to EPN infection. This is a particularly interesting avenue of research because not only it can provide important insight about the regulation of clotting response in mammals against infection with nematode parasites but also it can give clues about the evolutionary basis of anti-parasitic immune response.
Although we cannot exclude the possibility that the current observations may be due to the different genetic background of the mutants as compared to the control flies and rescue experiments may be needed to confirm the observed phenotypes, our findings demonstrate novel functions for activin and BMP signaling activity in regulating the D. melanogaster immune response to parasitic nematode infection. In addition to paving the way to a better understanding of host-parasite interactions and the evolution of the immune role of TGF-ß signaling, results obtained from this study could have broader impacts. Parasitic nematodes pose a major threat to human health, and thus identification of the key immune signaling components that oppose infection against parasitic nematodes will provide important clues for the development of novel treatment strategies against parasitic diseases.
Data Availability Statement
The original contributions presented in the study are included in the article/Supplementary Material. Further inquiries can be directed to the corresponding author.
Author Contributions
YO designed and conducted the experiments, analyzed the data, constructed the figures, interpreted the results, and wrote drafts of the manuscript. DR conducted parts of the experiments. IE designed the experiments, interpreted the results, and revised the manuscript. All authors contributed to the article and approved the submitted version.
Funding
This research was funded by the National Institute of Allergy and Infectious Diseases (grants 1R01AI110675 and 1R56AI110675) and the National Science Foundation (grant 2019869).
Conflict of Interest
The authors declare that the research was conducted in the absence of any commercial or financial relationships that could be construed as a potential conflict of interest.
Publisher’s Note
All claims expressed in this article are solely those of the authors and do not necessarily represent those of their affiliated organizations, or those of the publisher, the editors and the reviewers. Any product that may be evaluated in this article, or claim that may be made by its manufacturer, is not guaranteed or endorsed by the publisher.
Acknowledgments
We thank Zachary Stickelman for maintaining and amplifying the laboratory fly lines and members of the Department of Biological Sciences at George Washington University for providing feedback to the project.
Supplementary Material
The Supplementary Material for this article can be found online at: https://www.frontiersin.org/articles/10.3389/fimmu.2021.795331/full#supplementary-material
References
1. Huminiecki L, Goldovsky L, Freilich S, Moustakas A, Ouzounis C, Heldin CH. Emergence, Development and Diversification of the TGF-β Signalling Pathway Within the Animal Kingdom. BMC Evol Biol (2009) 9:28. doi: 10.1186/1471-2148-9-28
2. Pang K, Ryan JF, Baxevanis AD, Martindale MQ. Evolution of the TGF-β Signaling Pathway and its Potential Role in the Ctenophore, Mnemiopsis leidyi. PLoS One (2011) 6:e24152. doi: 10.1371/journal.pone.0024152
4. Masucci JD, Miltenberger RJ, Hoffmann FM. Pattern-Specific Expression of the Drosophila Decapentaplegic Gene in Imaginal Disks Is Regulated by 3’ Cis-Regulatory Elements. Genes Dev (1990) 4:2011–23. doi: 10.1101/gad.4.11.2011
5. Montanari MP, Tran NV, Shimmi O. Regulation of Spatial Distribution of BMP Ligands for Pattern Formation. Dev Dyn (2021). doi: 10.1002/dvdy.397
6. Dobens LL, Raftery LA. Drosophila Oogenesis: A Model System to Understand TGF-ß/Dpp Directed Cell Morphogenesis. Ann NY Acad Sci (1998) 857:245–7. doi: 10.1111/j.1749-6632.1998.tb10123.x
7. Campbell G. Distalization of the Drosophila Leg by Graded EGF-Receptor Activity. Nature (2002) 418:781–5. doi: 10.1038/nature00971
8. Upadhyay A, Moss-Taylor L, Kim M-J, Ghosh AC, O’Connor MB. TGF-β Family Signaling in Drosophila. Cold Spring Harb Perspect Biol (2017) 9:a022152. doi: 10.1101/cshperspect.a022152
9. Zi Z, Chapnick DA, Liu X. Dynamics of TGF-ß/Smad Signaling. FEBS Lett (2012) 586:1921–8. doi: 10.1016/j.febslet.2012.03.063
10. Clark RI, Woodcock KJ, Geissmann F, Trouillet C, Dionne MS. Multiple TGF-β Superfamily Signals Modulate the Adult Drosophila Immune Response. Curr Biol (2011) 21:1672–7. doi: 10.1016/j.cub.2011.08.048
11. Patrnogic J, Heryanto C, Eleftherianos I. Wounding-Induced Upregulation of the Bone Morphogenic Protein Signaling Pathway in Drosophila Promotes Survival Against Parasitic Nematode Infection. Gene (2018) 673:112–8. doi: 10.1016/j.gene.2018.06.052
12. Eleftherianos I, Castillo JC, Patrnogic J. TGF-β Signaling Regulates Resistance to Parasitic Nematode Infection in Drosophila melanogaster. Immunobiol (2016) 221:1362–8. doi: 10.1016/j.imbio.2016.07.011
13. Patrnogic J, Heryanto C, Eleftherianos I. Transcriptional Up-Regulation of the TGF-β Intracellular Signaling Transducer Mad of Drosophila Larvae in Response to Parasitic Nematode Infection. Innate Immun (2018) 24:349–56. doi: 10.1177/1753425918790663
14. Ozakman Y, Eleftherianos I. TGF-β Signaling Interferes With the Drosophila Innate Immune and Metabolic Response to Parasitic Nematode Infection. Front Physiol (2019) 10:716. doi: 10.3389/fphys.2019.00716
15. Ozakman Y, Eleftherianos I. Nematode Infection and Antinematode Immunity in Drosophila. Trends Parasitol (2021) 37:1002–13. doi: 10.1016/j.pt.2021.06.001
16. Ciche TA, Ensign JC. For the Insect Pathogen Photorhabdus luminescens, Which End of a Nematode Is Out? Appl Environ Microbiol (2003) 69:1890–7. doi: 10.1128/AEM.69.4.1890-1897.2003
17. Stock SP, Blair HG. Entomopathogenic Nematodes and Their Bacterial Symbionts: The Inside Out of a Mutualistic Association. Symbiosis (2008) 46:65–75.
18. Castillo JC, Reynolds SE, Eleftherianos I. Insect Immune Responses to Nematode Parasites. Trends Parasitol (2011) 27:537–47. doi: 10.1016/j.pt.2011.09.001
19. Hallem EA, Rengarajan M, Ciche TAA, Sternberg PW. Nematodes, Bacteria, and Flies: A Tripartite Model for Nematode Parasitism. Curr Biol (2007) 17:898–904. doi: 10.1016/j.cub.2007.04.027
20. Castillo JC, Shokal U, Eleftherianos I. A Novel Method for Infecting Drosophila Adult Flies With Insect Pathogenic Nematodes. Virulence (2012) 3:339–47. doi: 10.4161/viru.20244
21. White GF. A Method for Obtaining Infective Nematode Larvae From Cultures. Science (1927) 66:302–3. doi: 10.1126/science.66.1709.302-a
22. Kenney E, Hawdon JM, O’Halloran D, Eleftherianos I. Heterorhabditis bacteriophora Excreted-Secreted Products Enable Infection by Photorhabdus luminescens Through Suppression of the Imd Pathway. Front Immunol (2019) 10:2372. doi: 10.3389/fimmu.2019.02372
23. Cooper D, Wuebbolt C, Heryanto C, Eleftherianos I. The Prophenoloxidase System in Drosophila Participates in the Anti-Nematode Immune Response. Mol Immunol (2019) 109:88–98. doi: 10.1016/j.molimm.2019.03.008
24. Honti V, Csordás G, Kurucz É, Márkus R, Andó I. The Cell-Mediated Immunity of Drosophila melanogaster: Hemocyte Lineages, Immune Compartments, Microanatomy and Regulation. Dev Comp Immunol (2014) 42:47–56. doi: 10.1016/j.dci.2013.06.005
25. Gold KS, Brückner K. Macrophages and Cellular Immunity in Drosophila melanogaster. Semin Immunol (2015) 27:357–68. doi: 10.1016/j.smim.2016.03.010
26. Eleftherianos I, More K, Spivack S, Paulin E, Khojandi A, Shukla S. Nitric Oxide Levels Regulate the Immune Response of Drosophila melanogaster Reference Laboratory Strains to Bacterial Infections. Infect Immun (2014) 82:4169–81. doi: 10.1128/IAI.02318-14
27. Vlisidou I, Wood W. Drosophila Blood Cells and Their Role in Immune Responses. FEBS J (2015) 282:1368–82. doi: 10.1111/febs.13235
28. Shokal U, Kopydlowski H, Eleftherianos I. The Distinct Function of Tep2 and Tep6 in the Immune Defense of Drosophila melanogaster Against the Pathogen Photorhabdus. Virulence (2017) 8:1668–82. doi: 10.1080/21505594.2017.1330240
29. Lavine MD, Strand MR. Insect Hemocytes and Their Role in Immunity. Insect Biochem Mol Biol (2002) 32:1295–309. doi: 10.1016/S0965-1748(02)00092-9
30. Rizki TM, Rizki RM. Lamellocyte Differentiation in Drosophila Larvae Parasitized by Leptopilina. Dev Comp Immunol (1992) 16:103–10. doi: 10.1016/0145-305X(92)90011-Z
31. Williams MJ. Drosophila Hemopoiesis and Cellular Immunity. J Immunol (2007) 178:4711–6. doi: 10.4049/jimmunol.178.8.4711
32. Rizki RM, Rizki TM. Effects of Lamellolysin From a Parasitoid Wasp on Drosophila Blood Cells In Vitro. J Exp Zool (1991) 2:236–44. doi: 10.1002/jez.140257021
33. Myers AL, Harris CM, Choe K-M, Brennan CA. Inflammatory Production of Reactive Oxygen Species by Drosophila Hemocytes Activates Cellular Immune Defenses. Biochem Biophys Res Commun (2018) 505:726–32. doi: 10.1016/j.bbrc.2018.09.126
34. Kim S-H, Lee W-J. Role of DUOX in Gut Inflammation: Lessons From Drosophila Model of Gut-Microbiota Interactions. Front Cell Infect Microbiol (2014) 3:116. doi: 10.3389/fcimb.2013.00116
35. Lee K-A, Cho K-C, Kim B, Jang I-H, Nam K, Kwon YE, et al. Inflammation-Modulated Metabolic Reprogramming Is Required for DUOX-Dependent Gut Immunity in Drosophila. Cell Host Microbe (2018) 23:338–352.e5. doi: 10.1016/j.chom.2018.01.011
36. Ferrandon D, Imler J-L, Hetru C, Hoffmann JA. The Drosophila Systemic Immune Response: Sensing and Signalling During Bacterial and Fungal Infections. Nat Rev Immunol (2007) 7:862–74. doi: 10.1038/nri2194
37. Patrnogic J, Heryanto C, Ozakman Y, Eleftherianos I. Transcript Analysis Reveals the Involvement of NF-κb Transcription Factors for the Activation of TGF-β Signaling in Nematode-Infected Drosophila. Immunogenetics (2019) 71:501–10. doi: 10.1007/s00251-019-01119-8
38. Imler JL, Bulet P. Antimicrobial Peptides in Drosophila: Structures, Activities and Gene Regulation. Chem Immunol Allergy (2005) 86:1–21. doi: 10.1159/000086648
39. Binggeli O, Neyen C, Poidevin M, Lemaitre B. Prophenoloxidase Activation Is Required for Survival to Microbial Infections in Drosophila. PLoS Path (2014) 10:e1004067. doi: 10.1371/journal.ppat.1004067
40. Kenney E, Yaparla A, Hawdon JM, O’ Halloran DM, Grayfer L, Eleftherianos I. A Putative Lysozyme and Serine Carboxypeptidase From Heterorhabditis bacteriophora Show Differential Virulence Capacities in Drosophila melanogaster. Dev Comp Immunol (2021) 114:103820. doi: 10.1016/j.dci.2020.103820
41. Dudzic JP, Hanson MA, Iatsenko I, Kondo S, Lemaitre B. More Than Black or White: Melanization and Toll Share Regulatory Serine Proteases in Drosophila. Cell Rep (2019) 27:1050–61.e3. doi: 10.1016/j.celrep.2019.03.101
42. Dziedziech A, Shivankar S, Theopold U. High-Resolution Infection Kinetics of Entomopathogenic Nematodes Entering Drosophila melanogaster. Insects (2020) 1:60. doi: 10.3390/insects11010060
43. Michel T, Reichhart J-M, Hoffmann JA, Royet J. Drosophila Toll Is Activated by Gram-Positive Bacteria Through a Circulating Peptidoglycan Recognition Protein. Nature (2001) 414:756–9. doi: 10.1038/414756a
44. Gottar M, Gobert V, Matskevich AA, Reichhart J-M, Wang C, Butt TM, et al. Dual Detection of Fungal Infections in Drosophila via Recognition of Glucans and Sensing of Virulence Factors. Cell (2006) 127:1425–37. doi: 10.1016/j.cell.2006.10.046
45. Lemaitre B, Kromer-Metzger E, Michaut L, Nicolas E, Meister M, Georgel P, et al. A Recessive Mutation, Immune Deficiency (Imd), Defines Two Distinct Control Pathways in the Drosophila Host Defense. Proc Natl Acad Sci USA (1995) 92:9465–9. doi: 10.1073/pnas.92.21.9465
46. Kleino A, Silverman N. The Drosophila IMD Pathway in the Activation of the Humoral Immune Response. Dev Comp Immunol (2014) 42:25–35. doi: 10.1016/j.dci.2013.05.014
47. Lemaitre B, Reichhart J, Hoffmann JA. Drosophila Host Defense: Differential Induction of Antimicrobial Peptide Genes After Infection by Various Classes of Microorganisms. Proc Natl Acad Sci USA (1997) 26:14614–9. doi: 10.1073/pnas.94.26.14614
48. Kenney E, Yaparla A, Hawdon JM, O’Halloran DM, Grayfer L, Eleftherianos I. A Putative UDP-Glycosyltransferase From Heterorhabditis bacteriophora Suppresses Antimicrobial Peptide Gene Expression and Factors Related to Ecdysone Signaling. Sci Rep (2020) 10:12312. doi: 10.1038/s41598-020-69306-212
49. Xiao C, Shim J, Klüppel M, Zhang SS, Dong C, Flavell RA, et al. Ecsit Is Required for Bmp Signaling and Mesoderm Formation During Mouse Embryogenesis. Genes Dev (2003) 23:2933–49. doi: 10.1101/gad.1145603.lated
50. Pechmann M, Kenny NJ, Pott L, Heger P, Lynch J, Roth S, et al. Striking Parallels Between Dorsoventral Patterning in Drosophila and Gryllus Reveal a Complex Evolutionary History Behind a Model Gene Regulatory Network. eLife (2021) 10:e68287. doi: 10.7554/eLife.68287
51. Castillo JC, Creasy T, Kumari P, Shetty A, Shokal U, Tallon LJ, et al. Drosophila Anti-Nematode and Anti- Bacterial Immune Regulators Revealed by RNA-Seq. BMC Genomics (2015) 16:1–21. doi: 10.1186/s12864-015-1690-2
52. Plotkin S, Diemert D, Bethony JM, Hotez PJ. Hookworm Vaccines. Clin Infect Dis (2008) 46:282–8. doi: 10.1086/524070
53. Panuska C. Lungworms of Ruminants. Vet Clin North Am Food Anim Pract (2006) 22:583–93. doi: 10.1016/j.cvfa.2006.06.002
54. Vieson MD, Piñeyro P, LeRoith T. A Review of the Pathology and Treatment of Canine Respiratory Infections. Vet Med (2012) 3:25–39. doi: 10.2147/VMRR.S25021
55. Garriga A, Mastore M, Morton A, Del Pino FG, Brivio MF. Immune Response of Drosophila suzukii Larvae to Infection With the Nematobacterial Complex Steinernema carpocapsae–Xenorhabdus nematophila. Insects (2020) 11:210. doi: 10.3390/insects11040210
56. Bidla G, Lindgren M, Theopold U, Dushay MS. Hemolymph Coagulation and Phenoloxidase in Drosophila Larvae. Dev Comp Immunol (2005) 8:669–79. doi: 10.1016/j.dci.2004.11.007
57. Wang Z, Wilhelmsson C, Hyrsl P, Loof TG, Dobes P, Klupp M, et al. Pathogen Entrapment by Transglutaminase – A Conserved Early Innate Immune Mechanism. PLoS Pathog (2010) 2:e1000763. doi: 10.1371/journal.ppat.1000763
Keywords: Drosophila, innate immunity, Heterorhabditis, Photorhabdus, TGF-ß
Citation: Ozakman Y, Raval D and Eleftherianos I (2021) Activin and BMP Signaling Activity Affects Different Aspects of Host Anti-Nematode Immunity in Drosophila melanogaster. Front. Immunol. 12:795331. doi: 10.3389/fimmu.2021.795331
Received: 14 October 2021; Accepted: 06 December 2021;
Published: 22 December 2021.
Edited by:
Katherine Buckley, Auburn University, United StatesReviewed by:
Samuel Liegeois, University of Strasbourg, FranceLage Cerenius, Uppsala University, Sweden
Copyright © 2021 Ozakman, Raval and Eleftherianos. This is an open-access article distributed under the terms of the Creative Commons Attribution License (CC BY). The use, distribution or reproduction in other forums is permitted, provided the original author(s) and the copyright owner(s) are credited and that the original publication in this journal is cited, in accordance with accepted academic practice. No use, distribution or reproduction is permitted which does not comply with these terms.
*Correspondence: Ioannis Eleftherianos, aW9hbm5pc2VAZ3d1LmVkdQ==