- Department of Orthopedics, The First Hospital of Jilin University, Changchun, China
Cellular associations in the bone microenvironment are involved in modulating the balance between bone remodeling and resorption, which is necessary for maintaining a normal bone morphology. Macrophages and osteoclasts are both vital components of the bone marrow. Macrophages can interact with osteoclasts and regulate bone metabolism by secreting a variety of cytokines, which make a significant contribution to the associations. Although, recent studies have fully explored either macrophages or osteoclasts, indicating the significance of these two types of cells. However, it is of high importance to report the latest discoveries on the relationships between these two myeloid-derived cells in the field of osteoimmunology. Therefore, this paper reviews this topic from three novel aspects of the origin, polarization, and subgroups based on the previous work, to provide a reference for future research and treatment of bone-related diseases.
Introduction
The skeleton is a complex organ that facilitates locomotion, retains blood calcium concentration, provides stable support to soft tissues, and is a site for adult hematopoiesis. Continued bone remodeling is necessary to maintain these crucial functions by preventing the accumulation of bone injuries, and sustaining both calcium homeostasis and bone strength (1, 2). The remodeling of bone is a tightly-coupled process that involves osteoclasts and osteoblasts. Among them, osteoclasts are multinucleated cells that develop from the fusion of osteoclast precursors (OCPs) through the activation of macrophage colony‐stimulating factor (M‐CSF) and receptor activator of NF‐kB ligand (RANKL), which can secret H+, Cl-, cathepsin K (CtsK), and matrix metalloproteinases (MMPs) (3–5). Importantly, osteoclasts are the only bone-resorbing cells in the human body, and are crucial for remodeling of the skeletal system.
On the other hand, macrophages are phagocytes from the mononuclear myeloid lineage. They are known for their protective roles in eliminating pathogens and recruitment of other immune cells from the peripheral circulation to the sites of infection and inflammation. These cells have evolved; emphasizing their distinct and critical significance in almost all tissues. Moreover, most tissues and organs host a resident group of macrophages that have adapted to the local conditions and are able to perform crucial tissue-specific functions in order to maintain homeostasis (6). For instance, osteal macrophages, which are the resident tissue macrophages in bones, perform various functions in the bone microenvironment. Besides, macrophages can change their functional and phenotypic properties in response to signals released from the immediate environment (7). Based on their activation states, macrophages can be categorized into M1 and M2 subtypes (8). In fact, M1- and M2-like macrophages share several features with T helper (Th) 1/Th2 cells (9). It has been reported that M1 macrophages are antimicrobial and proinflammatory, whereas M2 macrophages are anti-inflammatory (10, 11). Various physiological processes, including skeletal homeostasis, depend on the balance between M1 and M2 macrophages.
In the field of osteoimmunity, immune and skeletal systems share numerous biological factors, such as chemokines, cytokines, hormones, etc. In addition, the interactions between osteoclasts and macrophages exert an indispensable influence in this area. Despite the well-established wealth of knowledge on macrophage genesis, activity, and polarization, the relationships between macrophages and osteoclasts in osteoimmunity still need further elucidation. In this article, we primarily focused on the effect of macrophage polarization on osteoclasts. We found that various cytokines, such as interleukins (ILs), chemokines, and tumor necrosis factors (TNFs) produced during the polarization process are intimately associate with he differentiation, activity, and survival of osteoclasts. Furthermore, we complementarily described the monocyte/macrophage origin of osteoclasts and the relationships between macrophage subpopulations and osteoclasts. In general, we summarized the current knowledge on macrophage-osteoclast interactions from diverse perspectives to provide references for future studies.
The Monocyte/Macrophage Origin of Osteoclasts
Various theories have been propounded to account for the origin of osteoclasts since they were discovered in 1873 (12). However, Walker’s pioneering experiments in the 1970s confirmed their hematopoietic origin, as transfusions of spleen and myeloid cells from wild-type mice reversed bone resorption in osteosclerotic and osteopetrotic mice lacking osteoclasts. This demonstrated that hematopoietic organs are capable of generating cells that cause hard tissues to resorb (12, 13). In 1986, Scheven and coworkers were the first to show that osteoclasts can be produced from a subgroup of cells that are rich in hemopoietic progenitors (14). In 1990, the monocyte/macrophage origin of osteoclast was later certified by Udagawa and partners after they proved that hemopoietic stem cells differentiate into the monocyte-macrophages through the activation of M-CSF (15). Subsequent studies further confirmed that osteoclasts can be formed from monocyte-macrophage precursor cells as well as from mature macrophages in tissues (15).
Furthermore, bones and bone marrow contain three unique macrophage populations, namely: osteoclasts and bone marrow macrophages (erythroid island macrophages), hematopoietic stem cell macrophages, and a newly-discovered group of macrophages called osteal macrophages or “osteomacs” (16). This also suggests that osteoclasts and macrophages may have a similar origin. Studies have shown that cells from the mononuclear/macrophage system, which contain hematopoietic marrow cells, blood monocytes, and peritoneal macrophages, possess the ability to develop into bone-resorbing osteoclasts; thus, squarely classifying osteoclastic groups within these series of cells (17–20). In fact, osteoclast and macrophage are the two differentiation products from myeloid precursors that compete with each other (21). In summary, osteoclasts are generated by a series of processes that begins with the commitment of hematopoietic stem cells (HSCs) to the mononuclear/phagocyte series (22), followed by the proliferation of pre-osteoclasts, and final maturation into osteoclasts, which possess bone-resorbing capacity (23).
In the diverse colony-forming units (CFUs), which generate various myeloid cells, only the CFU macrophages, when exposed to M-CSF, evolve into macrophage and dendritic cell (DC) progenitor (MDP). MDP is a bipotent progenitor (24) which undergoes osteoclastic differentiation (25) (Figure 1). In addition to the differentiation of myeloid progenitors into osteoclasts, macrophages also serve as an osteoclastic source. Osteoclasts can be formed from tissue-specific macrophages in inflammatory and immunological environments (26) (Figure 1). For example, osteoclasts can be differentiated from osteal macrophages and synovial macrophages in an inflammatory condition (26, 27). Further, premature DCs can develop into typical DCs, while they can also become osteoclasts when exposed to M-CSF and RANKL (25, 28) (Figure 1).
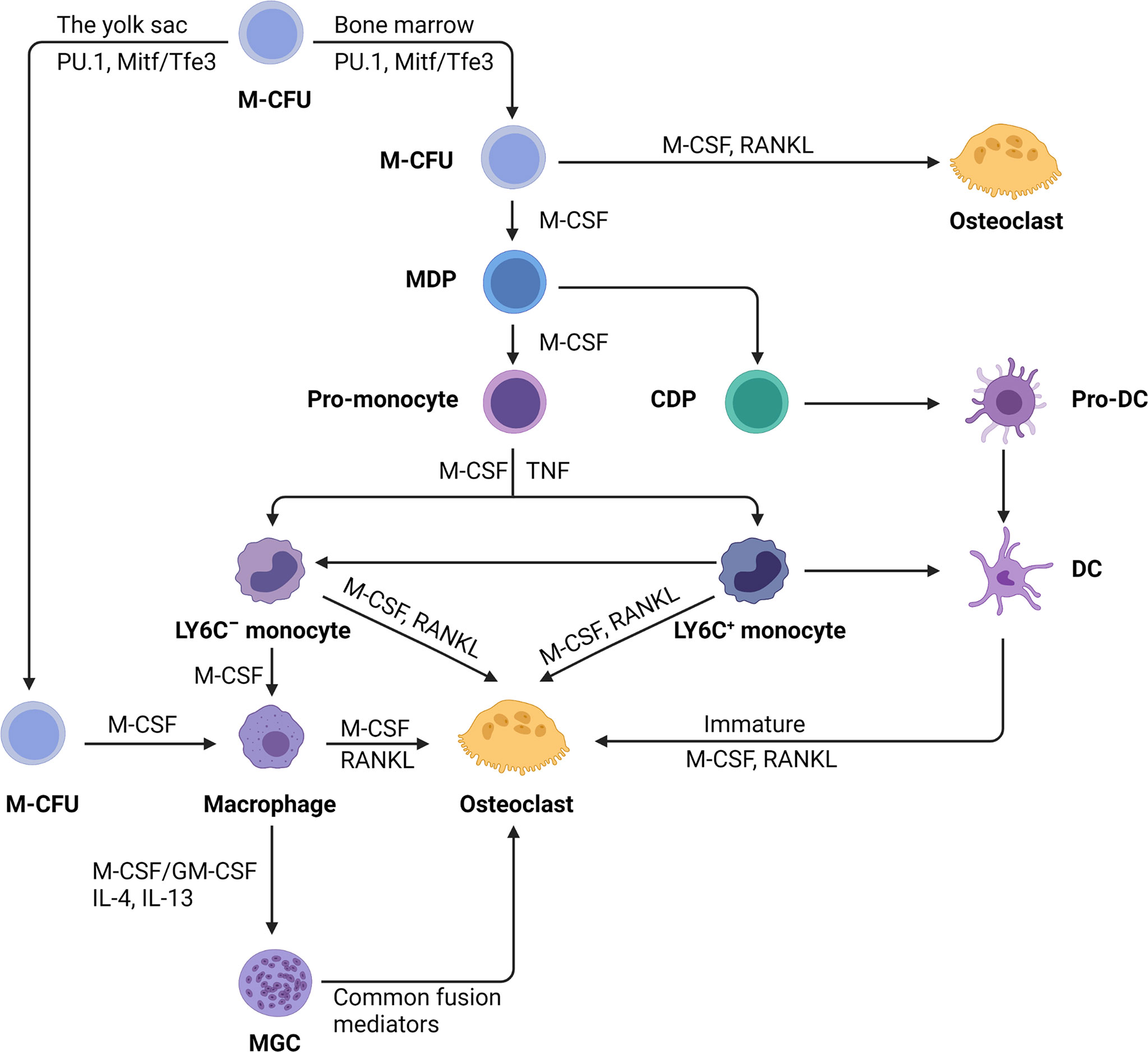
Figure 1 The monocyte/macrophage origin of osteoclasts. Hematopoietic stem cells myeloid colony-forming units (M-CFU) from bone marrow or yolk sac is the main site of myeloid cell production. Recent researches have shown that tissue-resident macrophages initially arise from myeloid stem cells (M-CFU) in the yolk sac of the developing embryo. In M-CFU, early expression of PU.1 and Mitf induces the emergence of M-CSFR (the receptor for M-CSF). Subsequently, in combination with macrophage colony‐stimulating factor (M-CSF) and receptor activator of NF‐κB ligand (RANKL), M-CFU can differentiate directly into osteoclasts. But in the separate action of M-CSF, M-CFU can differentiate into M-CSF-dependent macrophage and dendritic cell progenitor (MDP). MDP can differentiate into both dendritic cells and monocytes, the latter of which can differentiate into pro-monocytes under the sustained action of M-CSF. Pro-monocytes can differentiate to form specifically marked monocytes, namely: LY6C+ or LY6C- blood monocytes, under appropriate stimulation, such as M-CSF, TNF. Monocytes (Ly-6C−) induced by M-CSF develop into macrophages, but the addition of RANKL converts monocytes into osteoblasts commitment. Early-stage Ly-6C+ monocytes exhibit a high potential for osteoclast commitment in response to M-CSF and RANKL activation while still retaining the capacity to transform into Ly6C-monocytes. Completely differentiated macrophages induced by M-CSF and RANKL can fuse to become osteoclasts. Under pathological situations, macrophages can generate multinucleated giant cells (MGCs) when stimulated with M-CSF or stimulating factor (GM-CSF) and interleukins (IL-4, IL-13). MGCs continue to differentiate into osteoblasts in the presence of common fusion mediators. In addition, immature dendritic cells have the potential for osteolytic differentiation.
On the basis of CD14 and CD16 antigens expression, monocytes are divided into three groups: classical (CD14++CD16−), intermediate (CD14++CD16+), and nonclassical (CD14+CD16++) (29, 30). According to this hypothesis, classical monocytes are the major source of osteoclasts, while intermediate monocytes can transform into high-absorption-capacity osteoclasts during inflammation, with non-classical monocytes evolving into non-resorbable osteoclasts (31–34). The study has reported that the intermediate monocytes convert into M1-macrophages to perform crucial functions in inflammation of the synovium. However, findings have shown that the classical monocytes account for the vast majority of monocytes in rheumatoid arthritis (RA) (35). This suggests that in an inflammatory environment, the main cells that are recruited are still classical monocytes, while the main role is played by M1-type macrophages which differentiate from intermediate monocytes. A recent study has identified an erythromyeloid progenitor (EMP)-derived osteoclast precursor population (36). Yolk-sac macrophages of EMP origin produced neonatal osteoclasts that can create a space for postnatal bone marrow hematopoiesis (36). Furthermore, EMPs gave rise to long-lasting OCPs that contribute to postnatal bone remodeling in both physiological and pathological settings (36). Single-cell RNA-sequencing data showed that EMP-derived OCPs arose independently of the HSC lineage, and the data from fate-tracking of EMP and HSC lineages indicated the possibility of cell-cell fusion between these two lineages (36). Cx3cr1+ yolk-sac macrophage descendants reside in the adult spleen, and parabiosis experiments showed that these cells migrated through the bloodstream to the remodeled bone after injury (36). Another study also found that the postnatal maintenance of osteoclasts, bone mass, and bone marrow cavity involves iterative fusion of circulating blood monocytic cells with long-lived osteoclast syncytia (37).
Polarization of Macrophages: Influence on Osteoclasts
Since Elie Metchnikoff first defined these phagocytic cells over a century ago, the forms and roles of macrophages are becoming increasingly diverse (38). The ability of macrophages to modify their phenotypic features due to different external stimuli is known as activation (39, 40) (Figure 2). Alternatively activated macrophages (AAM; M2) were described in the early 1990s as possessing a distinct phenotype of macrophages from the traditionally activated or inflammatory macrophages (M1) (41). This categorization was developed as a result of the phenotypic changes observed in vitro after treatment with a variety of chemicals (42). Following the discovery of the M1/M2 macrophage paradigm, more evidence was found to support the idea that between these two seemingly opposed resultant phenotypes, there is a transitional form of intermediate phenotypes (39, 43, 44). Recently, a human macrophage with an open spectrum of activation, as identified by transcriptional clusters associated with diverse stimulations, was reported in a study. Under this condition, scholars have gradually coined the terminology ‘polarization’ to describe the disruption of macrophages by some stimuli, which induce various patterns of gene expression (40). However, the M1/M2 terminology was eventually accepted due to the discovery of different features of macrophages in cells generated from Th1 or Th2 dominant mouse lineages (42).
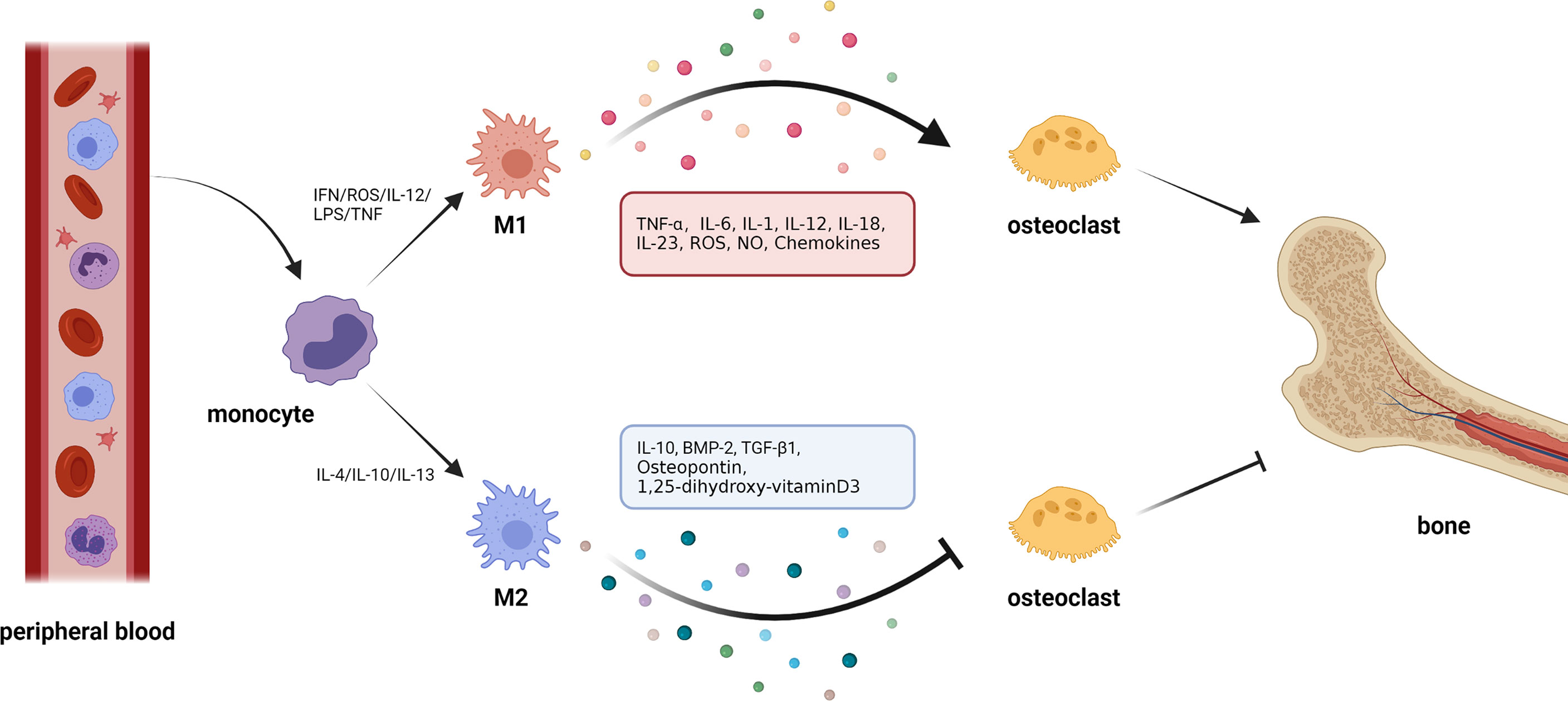
Figure 2 Macrophage polarization and osteoclasts. 1. Circulating macrophages change their phenotype according to the external environment. In the presence of cytokines, such as interferon (IFN), reactive oxygen species (ROS), interleukin-12 (IL-12), and tumor necrosis factor (TNF), macrophages polarize to M1 type. In response to interleukin-4 (IL-4), interleukin-10 (IL-10), interleukin-13 (IL-13), and other cytokines, macrophages can be polarized to M2 type. 2. Macrophages can produce cytokines with opposite effects in different polarization states. M1 macrophages can secrete cytokines, such as tumor necrosis factor-α (TNF-α), interleukin-6 (IL-6), interleukin-1 (IL-1), etc., which generally show the effect of activating osteoclasts and promoting bone resorption. M2 macrophages can secrete cytokines, such as IL-10, bone morphogenetic protein-2 (BMP-2), transforming growth factor-β1(TGF-β1), etc., most of which can inhibit osteoclastic bone resorption.
It is worth noting that the metabolic state of macrophages and osteoclasts is dependent on “activation” status, which itself is closely related to external stimuli or the disease environment. In pathological conditions, macrophages tend to be activated as M1-type macrophages that secrete proinflammatory cytokines against pathogens. Specifically, in inflammatory conditions, for example, following an infection, inflammatory stimuli, such as IL-12, lipopolysaccharide (LPS), interferon (IFN)-γ, and reactive oxygen species (ROS), that are generated by a damaged or necrotic tissue, promote polarization of macrophages into M1 phenotype (45–49). This is mediated by signal transducer and activator of transcription (STAT) 1, and interferon-regulatory factor (IRF) 5. As an important inflammatory response, polarized M1 can produce high levels of ROS, nitric oxide (NO), and proinflammatory cytokines, such as IL-1, IL-2, IL-6, IL-12, TNF-α, and IFN-γ, which are involved in enhancing the host’s defense response (50, 51). Under non-inflammatory conditions, macrophages largely exhibit the M2 phenotype which promotes tissue homeostasis and repair. Specifically, M2 macrophages are mainly present in the subsiding phase of inflammation, and they are responsible for the production of anti-inflammatory cytokines and the clearance of apoptotic cells. Exposure to anti-inflammatory cytokines (IL-4, IL-10, and IL-13), IL-1 receptor ligands, or immune complexes and Toll-like receptors (TLRs) can lead to M2 macrophage polarization via STAT6 and IRF4 (9, 52, 53). M2 macrophages can produce anti-inflammatory cytokines, such as chemokine (C-C motif) ligand 18 (CCL-18), CCL-22, IL-10, and a small amount of IL-12 family members (39, 54). In addition, M2 macrophages can produce a large number of osteogenic growth factors, such as bone morphogenetic protein-2 (BMP-2), a subclass of the TGF-β family and a potent promoter to osteogenic differentiation of MSCs (55, 56), TGF-β (57), osteopontin (58), and 1, 25-dihydroxyvitamin D3 (59). M1 macrophage-related cytokines, such as TNF-α, IL-6, and IL-1β, can induce osteoclastogenesis, while the M2 macrophage-related cytokines, such as IL-4 and IL-10, can inhibit osteoclastogenesis through the downregulation of NFATc1 (60, 61). Thus, the polarization of macrophages (M1/M2) itself is important for the determination of osteoclastogenesis, which makes the interaction between macrophages and osteoclasts even more complex.
Similar to macrophages, the metabolic state of osteoclasts is also closely related to the disease conditions. For instance, the seminal study of Trouillet-Assant et al. discovered that infection of bone marrow-derived OCPs with live S. aureus promoted their differentiation into activated macrophages rather than osteoclasts (62). However, the cultivation of OCPs with only the supernatants of the infected macrophages promoted the differentiation into bone-resorbing cells, due to the secreted proinflammatory cytokines they contained. Infection of mature osteoclasts with S. aureus promoted their bone-resorbing capacity in a hydroxyapatite matrix degradation assay which proceeded with enhanced cellular fusion events, and a higher number of nuclei per osteoclast with a significantly increased size (62). Similarly, when fractures occur, osteoclasts can be activated via the RANKL pathway (63). Subsequently, immature HSC precursors are recruited via CXCR4 and MMP-9 pathways to promote HSC migration (63).
M1 Polarization and Osteoclasts
In inflammatory conditions, for instance, after an infection, inflammatory stimuli, such as IL-12, IFN-γ, and ROS, that are generated by damaged or necrotic tissue, promote the polarization of macrophages into M1 phenotype (45–49), resulting in the release of cytokines, such as TNF-α, IL-6, IL-1, and others to affect osteoclast differentiation and formation (Table 1).
Osteoclastogenesis is promoted via TNF-α both directly by increasing OCP population and/or differentiation, and indirectly by enhancing RANKL secretion in osteoblasts and other cells (64, 65, 113). Moreover, TNF-α has been reported to be able to change CD11b+F4/80+ cells (bone marrow cells) from Ly6C−Gr1−M2 macrophages to Ly6C−Gr1−CD11c+ and Ly6C+Gr1−CD11c+ M1 macrophages. Pretreatment of M-CSF-stimulated mouse bone marrow with TNF-α increased osteoclast progenitor population, resulting in an increased number of osteoclasts generated by Ly6C-Gr1 and Ly6C+Gr1- monocyte series (66). This may indicate that the function of TNF-α is to boost osteoclastic precursors by changing the M-CSF-activated M2 differentiation to M1 macrophages, with elevated osteoclastogenesis potentials (66). IL-1 can also promote osteoclastogenesis by downregulating osteoprotegerin (OPG) levels and upregulating RANKL levels (113).
Lipocytes, muscle cells, and lymphocytes all generate IL-6, which is part of the cytokine family that also includes IL-11, IL-27, oncostatin M (OSM), and others (114). In response to injuries, IL-6 is generated locally and released into the bloodstream, where it triggers a rapid immune response (115). Osteocytes and osteoblasts have been shown to be potently stimulated by IL-6 to produce RANKL (73, 74). The interaction of IL-6/IL-6 receptor and glycoprotein 130 (gp130) receptor can ignite Janus-activated kinase (JAK) and cause the phosphorylation of STAT3, allowing RANKL to enhance osteoclastic development (74). Treatment with STAT3 blocker, CP690,550, decreased expression of the IL-6 family members, and also the generation of osteoclast both in vivo and in vitro (116). Importantly, the potential of IL-6 to stimulate osteoclastogenesis is determined by the presence of IL-6 receptors on osteoblasts, but not on OCPs (117). The membrane-bound IL-6 receptors (IL-6Rs), and the soluble IL-6 receptor (sIL-6R) are the two major transmitters that transduce IL-6 signals significantly. Interaction of IL-6 with IL-6R results in the dimerization with common signaling receptor subunit gp130, and subsequent downstream signaling transduction through phosphorylation of the JAK/STAT pathway (118). In cells without membrane-bound IL-6R, the interaction of IL-6 with sIL-6R results in the formation of a molecule that can combine with membrane-bound gp130 and activate downstream signaling (118). Soluble IL-6R signaling is hypothesized to be involved in the proinflammatory effects of IL-6 on several tissues. In addition, the effects of TNF-α and IL-1 on human osteoclastogenesis can also be mediated by IL-6 (75).
As a result of its significant bone-resorbing property, IL-1 was first classified as an osteoclast-activating factor (119). The adaptor molecule, MyD88, is activated by IL-1 to initiate signaling pathways that result in the initiation of NF-κB and MAPKs, as well as downstream transcription factors that promote gene expression and osteoclast formation (120). The IL-1 gene family contains a number of different members, including: IL-1α, IL-1β, and the IL-1R antagonist (IL-1Ra) (69). IL-1α and IL-1β are agonists, while IL-1Ra is a specialized receptor antagonist. Among these members, IL-1β is one of the key mediators of bone resorption in inflammatory conditions. IL-1β promotes TNF-α-stimulated osteoclast formation by improving the synthesis of RANKL in mesenchymal cells (70). In the presence of adequate RANKL concentrations, IL-1β directly promotes the development of OCPs under the regulation of p38 MAPK (70). Moreover, since IL-1R is localized on the osteoclast surface (71), IL-1β and other IL-1s can suppress the apoptosis of osteoclasts by activating the NF-κB pathway (121). However, IL-1β is a multifaceted cytokine with mostly pro-osteoclastogenesis features, but can also employ negative feedback mechanisms that attenuate osteoclast formation (69). IL-1β is greatly time-dependent in its impacts on osteoclastogenesis. As shown in earlier projects with mouse cells and as demonstrated in multiple experiments employing human OCPs (67, 72), exposure to IL-1β before or simultaneously with RANKL inhibits osteoclast formation. Conversely, exposure to IL-1β post-RANKL treatment has the reverse result, i.e., enhancing osteoclast development and resorptive activity. The reason for this phenomenon is that IL-1Rs share a cytosolic Toll-IL-1R domain and common intracellular signaling molecules with TLR, so IL-1β can directly block the initial phases of human osteoclast maturation by dropping of the M-CSF receptor, c-Fms, that is essential for RANK synthesis after binding with TLRs (72). In addition to IL-β, IL-1α is also known to be a potent osteoclastogenic cytokine. IL-1α also requires adequate RANKL concentrations to trigger the expression of OC biomarkers, such as tartrate-resistant acid phosphatase (TRAP), CtsK, MMP-9, and nuclear factor of activated T-cells cytoplasmic 1 (NFATc1) (68). Furthermore, regardless of RANKL, IL-1 may promote OC differentiation in bone marrow macrophages by activating the microphthalmia transcription factor (MITF) (67).
The cytokines, IL-12 and IL-23, which are reported to perform a key part in inflammatory responses, participate in the inflammation-induced bone abnormalities. IL-12, composed of p35 and p40, is one of the key factors influencing osteoclasts. IL-12 is mainly produced by macrophages and DCs, and is essential for Th cell formation and activity (76–78). IFN-γ is the main product of activated Th cells (79). This factor prevents osteogenic differentiation of bone marrow mesenchymal stem cells and causes damage to the cells (80). Notably, IFN-γ is also a powerful blocker of osteoclast differentiation. Briefly, it can inhibit osteoclast differentiation by decreasing CtsK levels (81) or increasing the decomposition of tumor necrosis factor receptor (TNFR)-associated factor 6 (TRAF6) (82, 83). In addition, IL-12 itself can also inhibit osteoclast activity (84–86). Mechanistically, IL-12 can downregulate NFATc1 expression, and thus inhibit RANKL-induced osteoclastogenic potentials (87). Also, IL-12 can promote TNF-α-induced osteoclast apoptosis via the FAS/FASL pathway (88). IL-23, composed of p40 and p19 subunits, is also a member of the IL-12 family. The overexpression of IL-23 has been reported to increase Th1 production and induce significant bone loss in mice (122). Further studies demonstrated that bone marrow macrophages isolated from IL-23 knockout mice possessed diminished osteoclast capacity and resorption efficiency (122). The two main mechanisms through which IL-23 positively regulates osteoclasts involve either directly increasing RANK expression in OCPs (97) or indirectly promoting RANKL production in CD4+ T cells (98). Furthermore, IL-23 can affect osteoclasts through its regulation of IL-17 secretion. In the presence of IL-17, the transformation of OCPs into osteoclasts is inhibited and the secretion of MMP-9 and CtsK by mature osteoclasts is reduced (99). Follow-up studies have shown that activation of NF-κB, p38, and ERK signaling pathways is the main mechanism by which IL-17 acts.
IL-18, like IL-12, also inhibits the formation of osteoclasts, and both have a synergistic effect (84). In combination with IL-12, IL-18 prevents the formation of osteoclasts more effectively (84). In addition, IL-18, similar to IL-12, was originally recognized as an IFN-inducing agent, and studies have demonstrated that the two cytokines work in tandem, with combination therapy leading to considerably more IFN-γ production than either IL alone (89–92). IFN-γ can induce cell apoptosis and suppress osteoclast differentiation. Of note, IL-18 can also inhibit osteoclast bone resorption partially mediated by IL-6 (93). Mechanistically, by modulating the synthesis of M-CSF and granulocyte-macrophage colony‐stimulating factor (GM-CSF) through T-cells, IL-18 inhibits the differentiation of osteoclasts, and induces apoptosis of osteoclasts by the generation of NO (94, 95). However, some studies have reported that in RA synovitis, IL-18 can indirectly induce osteoclastogenesis by upregulating RANKL synthesis through T cells (96). Therefore, the effects of macrophages on osteoclasts still need to be discussed.
Most commonly referenced ROS are hydroxyl radicals (HO·), hydrogen peroxide (H2O2), and hyperoxide (superoxide) anions (O2-) (123). It is important to note that cell reproduction, viability, apoptosis, maturation, motility, and metabolic activity are all regulated by ROS. ROS are critical secondary intracellular messengers involved in numerous physiological activities, such as apoptosis, epigenetics, and the initiation of cell signal transductions (124). ROS are primarily generated by mitochondrial metabolism, particularly by ETC complexes I and II, where oxygen utilization results in ROS generation due to the reverse electron transfer between the complexes and molecular oxygen. Also, ROS could be produced in the cytosol by the oxidative process of NADPH oxidases (NOX). It has been known for many years that the activities and development of osteoclasts are regulated by ROS (107, 108, 125–127). Exposure of macrophages to exogenous ROS, such as H2O2, causes the RANK signaling cascade to become activated, resulting in the development of osteoclasts (107), whereas RANKL initiation causes the synthesis of endogenous ROS, which subsequently functions as a second messenger to induce the conversion of macrophages into osteoclasts (109). RANKL can enhance the levels of intracellular ROS throughout osteoclastogenesis by initiating signaling pathways that include: TRAF6 and NOX1 (108). There is growing evidence that the activities of important osteoclast transcription factors, such as NF-κB and NFATc1, may also be influenced by ROS (110). Furthermore, the production of ROS by RANKL-stimulated osteoclasts has been shown to suppress the synthesis of antioxidant proteins like catalase (CAT) and superoxide dismutase (SOD) (111). As a result, ROS can be regarded as a crucial signaling messenger during osteoclastogenesis. In osteoclast-mediated diabetic osteoporosis, the over-activation of ROS/MAPKs/NF-κB/NLRP3 pathway is also the main cause of osteoclast resorption (112).
NO is a free radical that regulates many physiological activities, including vascular relaxation (128), neurotransmission, platelet aggregation, and immunological control (129). In vivo, NO is generated by the enzyme NO synthase (NOS) (130, 131). It is known as NOS for its ability to catalyze the oxidation of guanidine nitrogen from L-arginine in the presence of calcium ions, NADH, and tetrahydrobiopterin as co-factors (130, 131). NO and NOS have been shown to have a significant influence on bone cell activity in recent years. The effects of NO on skeletal structures have been shown to be extremely concentration-dependent, according to recent research reports (130, 131). The impacts of NO on osteoclast development and survival are similar to those seen in osteoblasts: a low level of NO enhances, whereas an excess of NO suppresses (104–106). In vivo, NOS1 performs necessary functions for the development and survival of osteoclasts because NOS1-lacking mice exhibited significantly reduced osteoclast production, and in vitro, NOS1-lacking bone marrow monocytes formed dysfunctional osteoclasts (106, 132). Also, the synthesis of NOS2 in OCPs is induced by RANKL, and NOS2 produces high NO concentrations that suppress osteoclastogenesis in a cGMP-independent manner (133). A subsequent study in vitro has shown that OCPs from NOS2-lacking mice were prone to differentiate more easily and form bone pits more quickly (133). Additionally, the production of NOS2 by IL-1 and IFN-γ has also been shown to inhibit the activities of osteoclasts (134–136).
Chemokines are dynamic molecules that are released in response to inflammatory conditions (137). They serve as a key regulator in osteoclast formation (138). Inflammatory osteo-disorders cause C-X-C motif chemokine ligand (CXCL)8, CXCL9, CXCL10, and chemoattractant chemokine ligand (CCL)20 concentrations to rise (101, 139). According to recent research reports, CXCL8 and CCL20 seem to have a contribution to osteoclast generation via regulating IL-6 synthesis in primordial osteoblasts (101). Likewise, CXCL10 enhances the secretion of RANKL and TNF-α in stimulated CD4+ T cells, which subsequently stimulates osteoclast formation (102). Mutually, CXCL10 synthesis in OC precursors is also induced by RANKL (102). CXCL2, which is produced by RANKL activation, promotes the procreation of OC progenitors by activating ERK (100). Osteoblast-derived CX3C chemokine ligand (CX3CL)1 enhances osteoclastogenesis by causing OC precursors to adhere to the site of bone resorption (100). CCL4 is a key regulator of OC motility through the promotion of PI3K activity, according to recent findings (103). In addition, CCL2 (MIP-1), CCL5 (RANTES), CCL7 (MCP-3), and CXCL12 (SDF-1) can also promote motility, adherence, absorption, and survivability of OC (140, 141).
M2 Polarization and Osteoclasts
M2 macrophages are mostly found during the descending stages of infection and they secrete anti-inflammatory factors, e.g., IL-10 (39, 54). In addition, M2 macrophages can generate several osteogenic growth factors, such as BMP-2, TGF-β1 (57), OPN (58), and 1,25-dihydroxyvitamin D3 (1,25-(OH)2D3) (59). Through these factors, M2 macrophages further exert their influence on osteoblasts (Table 2).
As a founding member of the IL-10 cytokine family, IL-10 demonstrates immune suppressive properties in a wide spectrum. In addition to the IL-10 molecule, this cytokine community includes the IL-20 subfamily system (IL-19, IL-20, IL-22, IL-24) as well as the more genetically distinct IFN family (IL-28A, IL-28B, IL-29) (147, 148, 166). IL-10 is produced by a subpopulation of Th2 cells and possesses the ability to inhibit secretions by Th1 cells (149). Therefore, IL-10 was originally labeled as a secretory cytokine synthesis inhibitory factor (CSIF) (149). As research progresses, various immune cells, such as macrophages, DCs, mast cells, eosinophils, neutrophils, natural killer cells, CD4+ and CD8+ T cells, have been found to secrete IL-10. All of these cells are derived from the innate and acquired immune systems (150, 166). IL-10 has been demonstrated in some trials to prevent osteoclast formation (142, 143). Actually, through interference with NFATc1 activation and nuclear translocation, IL-10 is a robust osteoclastogenesis inhibitor (144). OPG synthesis is upregulated by IL-10, while RANKL and M-CSF expressions are downregulated (145). IL-10 suppresses osteoclastic activity by blocking the generation of pro-osteoclast factors, like IL-1, IL-6 and TNF-α (146). Therefore, IL-10 can be classified as an anti-osteoclastogenic cytokine.
BMP-2 is a member of the BMP family of proteins, and also belongs to the TGF cytokine superfamily (151). M2 macrophages are the main secretors of BMP-2 compared to M0- and M1-type macrophages. Previous studies have shown that BMP-2 becomes a potent inducer of bone remodeling by directly regulating osteoclast differentiation and osteoblast activity. Specifically, by regulating the synthesis of RANKL and M-CSF, BMP-2 organizes osteoclast differentiation and manages osteoclast survival, maturation, and activation (167–171). The significance of BMP-2-induced phosphatidylinositol 3-kinase and its downstream target AKT kinase has been identified in BMP-2-involved osteoblast maturation, and M-CSF secretion from osteoblasts to promote osteoclastogenesis (170, 172). In addition, through a self-regulatory circuit including Smad/Akt/Ca2+ signaling pathway, BMP-2 can also activate the NFATc1 transcription factor (173). NFATc1 promotes osteoclast differentiation through transcriptional activation of RANKL, which in turn stimulates the production of NFATc1 (174–176). It can be seen that BMP-2 performs a key function in the formation, development, and activation of osteoclasts.
TGF-β1, which is required for skeletal metabolism, can be synthesized by macrophages. The osteoclastogenic transcription factor, NF-κB, which is made up of proteins like p65, is part of the RANKL-RANK signaling pathway (177) and the main downstream controller of this pathway is NFATc1 (178). TGF-β1 has been demonstrated to limit the production and matrix degradation of osteoclast by directly inhibiting the expression of NFATc1 via obstruction p65 in the receptor activator (152).
OPN is a highly phosphorylated glycophosphoprotein with acidic properties and a high aspartic acid content (153). As a versatile factor, OPN is involved in the processes of inflammation, biomineralization, cell viability, and wound healing, as well as cardiovascular disease, cancer, diabetes, and renal calculus formation (153). OPN is secreted from osteoblasts and osteoclasts in osteoid (153). Research activities on mice with OPN genes knocked out show that the volume and length of the ruffled margins on the osteoclasts were several folds lower implying poorer resorptive potentials (154). In RA, OPN expressions can be aided by many factors in synovial tissues, and OPN is an active factor in the recruitment of osteoclasts (155) by acting as a coupling agent of osteoclasts to bones (156). Moreover, OPN affects organisms by modulating osteoclast activity and altering CD44 receptors, as well as through the secretion levels of cytokines, like IL-10, IL-12, IL–3, IFN-γ, integrin vB3, NF-κB (153). Furthermore, OPN, which is one of the most firmly coupled non-collagen proteins, is thought to aid in the adhesion of osteoclasts to the surface bones (156, 157). In summary, during the biomineralization stage, OPN has three key functions, namely: regulation of osseous cell attachment, regulation of osteoclastic activity, and regulation of matrix calcification.
The active form of vitamin D, i.e., 1,25-dihydroxyvitamin D3 (also called calcitriol), is a steroid molecule that balances calcium and bone metabolism, regulates cell proliferation and differentiation, and exerts immunoregulatory functions (179). Some research reports have shown that calcitriol possesses the ability to suppress osteoclastogenesis, while also promoting osteogenic differentiation (158, 159). In fact, 1,25-dihydroxyvitamin D3 can inhibit osteoclast differentiation by downregulating PAR2 mRNA expression which contributes to the determination of cells of osteoclast lineages (160). Furthermore, findings have uncovered the interactions between the BMP-Smad1 and IκB-NF-κB pathways in the process of osteoclast formation (161). Through the BMP-Smad1 and IκB-NF-κB pathways, 1,25-dihydroxyvitamin D3 can also influence osteoclast lineage commitment (161). Calcitriol suppresses osteoclastogenesis by altering the quantity and function of Th cell subgroups (Th2/Th17) under an inflammatory condition (162). However, there are also reports indicating the promoting role of 1,25-dihydroxyvitamin D3 in osteoclastogenesis. For example, applying high doses of calcitriol to congenital osteoporosis promotes the production of osteoclasts and generates certain effects (163). In addition, genetic effects of 1,25-dihydroxyvitamin D3 are exerted by its coupling of vitamin D receptor (VDR) to VDR response elements (VDREs) in the promoter sequences of target genes for vitamin D (164). More so, VDREs include genes of commonly-expressed proteins, such as RANKL, associated with adjustment of osteoclastogenesis (165). It can be seen that the role of 1,25-dihydroxyvitamin D3 in osteoclastogenesis needs to be further assessed.
The Relationship Between Subgroups of Macrophages and Osteoclasts
Macrophages are a type of innate immune cells that may be present in almost every tissue and exert effects on immunological responses, wound-healing, and homeostasis (180). Macrophages that are distributed in various tissues are called tissue-resident macrophages. Tissue-resident macrophages were formerly thought of as differentiated monocytes that seeded the tissues and performed immunological sentinel and homeostatic activities as well as other tasks (181). However, these macrophages are not all the same, but rather a collection of cells that share a common set of functions and characteristics (181). In the next section, we will discuss the relationship between osteoclasts and subgroups of macrophages, mainly tissue macrophages.
Osteal Macrophages and Osteoclasts
Osteal macrophages, a subgroup of bone-resident macrophages, are found right next to osteoblasts, where they govern bone production and play a variety of roles in the homeostasis of the skeleton (182). It is known that osteal macrophages can support the function of osteoblasts and promote bone anabolism, but the relationship between osteal macrophages and osteoclasts is still unclear. Myeloid progenitor cells give rise to osteoclasts and osteal macrophages (183), yet research findings have revealed that osteoclast formation is the more robust or preferable access for myeloid lineage differentiation (184). Although osteal macrophages can become osteoclast under the stimulation of RANKL and M-CSF, monocytes and other myeloid progenitors have been discovered to be more effective OCPs (185). In addition, osteoclasts and osteal macrophages possess unique membrane characteristics that distinguish them from one another. Osteal macrophages are independent of osteoclasts, having F4/80 positivity but TRAP negativity (185). As reported in recent studies, osteal macrophages were shown to exhibit both typical membrane antigens of macrophage-like CD68 and more specialized antigens, like Mac-3 and CD169 (182, 186, 187). These findings showed that osteal macrophages are a malleable, yet distinctive cell type with specialized roles in the microenvironment of the bone marrow. However, osteal macrophages can indeed influence osteoclasts. A recent study found that osteal macrophages perform a unique part in promoting osteoclast activity, as well as the first proof of their participation in the pathogenesis of osteoporosis (188). Additionally, osteal macrophages are involved in the effect of PTH on osteoclasts (184). When stimulated by local stimuli, osteal macrophages tend to release pro- and anti-osteoclastogenic factors, such as TNF-α (65, 189), IL-6 (190), IL-1 (189, 191), or IFN-β (192, 193), regulating osteoclast production and activity. In general, the relationship between osteal macrophages and osteoclasts needs further exploration.
Tumor-Associated Macrophages and Osteoclasts
The term “tumor-associated macrophages” (TAMs) refers to macrophages that have been attracted from circulating monocytes to tumors and have been impacted by the presence of cancer to facilitate tumor aggressiveness and development (194). TAMs are made up of M2 cells and a few M1 cells (195). Research has proven that the recruited monocytes in tumor sites are capable of being differentiated into TAMs and osteoclasts (196). Although TAMs and osteoclasts have a similar precursor, however, bisphosphonates have been demonstrated to precisely target TAMs (197, 198). Notably, TAMs are also a source of osteoclasts. According to previous studies, when exposed to cancer cells, 1,25-(OH)2D3, and M-CSF or to M-CSF and RANKL, TAMs have been reported to transform into functional bone-resorbing osteoclasts (18, 199). Moreover, under different polarization states, TAMs can release numerous cytokines, like IL-10 (200), IL-12 (201), which regulate osteoclast generation and function. In some tumor metastasis, TAMs and osteoclasts have similar roles (202, 203). Overall, the mechanisms regarding the interaction between TAMs and osteoclasts remain unclear and this area needs to be further explored. CD4- macrophages have a positive effect on osteoclast differentiation.
Synovial Macrophages and Osteoclasts
Specialized lining cells of the synovial intima, as well as macrophages found in the synovial subintima and synovial fluid, represent synovial macrophages (204). Similar to monocyte macrophages in other organs, they are produced from a common bone marrow progenitor and show some functional and biological properties (205). The most distinguishing characteristic of these cells is their proclivity for phagocytosis. However, they also perform a variety of other functions, including the initiation and modulation of hormones and cellular immunity, as well as the generation and discharge of a great number of secretory substances (205). A study has proven that synovial macrophages can develop into mature osteoclasts with lacunar resorption capabilities, but this process requires TNF-α/IL-1 or M-CSF along with RANKL (27). Because inflammatory synovial fluids have an increased number of macrophages and higher concentrations of RANKL, TNF-α, and IL-1, synovial macrophages in rheumatoid or crystal arthritis have a stronger potential to generate osteoclasts. Furthermore, a study has shown that OCPs in the synovium are monocytes/macrophages that express the CD14 antigen (206). In RA and inflammatory OA, CD14- macrophages have a positive effect on osteoclast differentiation of CD14+ macrophages (206). Overall, the exploration of the relationship between synovial macrophages and osteoclasts will help researchers better understand the therapy and pathogenesis of diseases, such as OA and RA.
Other Macrophage Subgroups and Osteoclasts
In addition to traditionally activated M1 macrophages, researchers recently found that human atherosclerotic plaques contain macrophages that express the mannose receptor (MR), an alternative macrophage identifier, implying that plaque macrophage populations are heterogeneous (207). This specific type of M2 macrophage, being positive for both CD68 and MRs, can develop into osteoclast-like cells (OLCs) and have a poor display of RANKL-stimulated osteoclastic bone resorption (208, 209). Osteoclasts and microglia are separate tissue-resident macrophages found in the bone and brain where they cause pathological alterations of osteoporosis and Alzheimer’s disease (AD), respectively (210). Both of them share critical signaling pathways, including three receptor signaling pathways, namely: TREM2/DAP12, M-CSF, and CCR5, which converge to control actin-microtubule dynamics and cytoskeleton architecture via the Pyk2 signaling pathway (210). Research has shown that AD patients are more prone to develop osteoporosis than the general population (210). Alveolar macrophages and osteoclasts have certain similarities, and therefore bisphosphonates can inhibit both cell types. A study has further shown that alendronate suppresses macrophage migratory and phagocytotic functions, as well as the inflammatory sensitivity of alveolar macrophages by blocking NF-κB signaling pathway (211). Albeit, alendronate inhalation ameliorates elastase-induced pulmonary emphysema by inducing apoptosis of alveolar macrophages (211). In addition, a case has been reported in which alveolar macrophages can be transformed into osteoclasts (212). In addition to the above cells, epithelioid and foam cells also exhibit certain associations with osteoclasts (213, 214).
Conclusion and Future Perspectives
Macrophages and osteoclasts have always been a hot and difficult area of research in osteoimmunity. Previous studies tended to focus on a certain type of cell and seldom summarize the latest updates on the interconnection between these two myeloid origin cells for the field of osteoimmunology. However, in this paper, we have outlined present knowledge on the relationships between macrophages and osteoclasts, including their common origin, regulation during polarization, and subgroups’ interactions. Be that as it may, the study of these two cellular associations still has many aspects that need to be further explored, and perplexing difficulties still remain. Notably, current studies tend to focus on the unidirectional effect of macrophages on osteoclasts during polarization at the expense of the effect of osteoclasts on macrophages. Actually, osteoclasts can be involved in the production of various cytokines, which act on macrophages. Here, we hypothesize that there are interactions among macrophages, osteoclasts, and osteoblasts to maintain bone homeostasis. Therefore, we believe that the imbalance between these cells in disease states and its specific mechanisms will be a new field for further exploration. Additionally, as a member of the mononuclear macrophage system (215), osteoclasts are considered as the resident macrophages in bone (182) and play a phagocytic role similar to that of macrophages. The similarities and differences between the two types of cells in performing phagocytosis are also an aspect worth investigating. A recent study reveals that besides apoptosis, RANKL-stimulated osteoclasts have an alternative cell fate in which they undergo fission into daughter cells called osteomorphs, once resorption is complete (216). Osteoclasts recycle via osteomorphs during RANKL-stimulated bone resorption (216). As an unexplored therapeutic target, the interaction of osteomorphs with macrophages will also be a new area of research.
The ultimate objective of practically all biomedical exploration is to improve patient outcomes and develop novel medicines, and renewed efforts in osteoimmunity will be important in accomplishing this translational goal. Currently, most therapies for bone-related diseases primarily focus on one specific cell type, but lack definite approaches aiming at the connections among cells in the bone microenvironment. Thus, the pharmaceutical targets in terminating the pathological association of macrophages with osteoclasts in disease states could become a noteworthy concern in the nearest future.
Author Contributions
YS drafted the manuscript. JL and XX assisted in reviewing literature. FG, ZS, and KZ modified the manuscript. YS and TY reviewed and edited the final manuscript. TY revised the manuscript. All authors contributed to the article and approved the submitted version.
Funding
This article was supported by the National Natural Science Foundation of China (Grant No. 31970090).
Conflict of Interest
The authors declare that the research was conducted in the absence of any commercial or financial relationships that could be construed as a potential conflict of interest.
Publisher’s Note
All claims expressed in this article are solely those of the authors and do not necessarily represent those of their affiliated organizations, or those of the publisher, the editors and the reviewers. Any product that may be evaluated in this article, or claim that may be made by its manufacturer, is not guaranteed or endorsed by the publisher.
References
1. Clarke B. Normal Bone Anatomy and Physiology. Clin J Am Soc Nephrol CJASN (2008) 3 Suppl 3(Suppl 3):S131–9. doi: 10.2215/cjn.04151206
2. Feng X, McDonald JM. Disorders of Bone Remodeling. Annu Rev Pathol (2011) 6:121–45. doi: 10.1146/annurev-pathol-011110-130203
3. Khosla S, Oursler MJ, Monroe DG. Estrogen and the Skeleton. Trends Endocrinol Metab (2012) 23(11):576–81. doi: 10.1016/j.tem.2012.03.008
4. Mirza F, Canalis E. Management of Endocrine Disease: Secondary Osteoporosis: Pathophysiology and Management. Eur J Endocrinol (2015) 173(3):R131–51. doi: 10.1530/eje-15-0118
5. Sobacchi C, Schulz A, Coxon FP, Villa A, Helfrich MH. Osteopetrosis: Genetics, Treatment and New Insights Into Osteoclast Function. Nat Rev Endocrinol (2013) 9(9):522–36. doi: 10.1038/nrendo.2013.137
6. Davies LC, Jenkins SJ, Allen JE, Taylor PR. Tissue-Resident Macrophages. Nat Immunol (2013) 14(10):986–95. doi: 10.1038/ni.2705
7. Horwood NJ. Macrophage Polarization and Bone Formation: A Review. Clin Rev Allergy Immunol (2016) 51(1):79–86. doi: 10.1007/s12016-015-8519-2
8. Yao Y, Xu XH, Jin L. Macrophage Polarization in Physiological and Pathological Pregnancy. Front Immunol (2019) 10:792. doi: 10.3389/fimmu.2019.00792
9. Mantovani A, Sica A, Sozzani S, Allavena P, Vecchi A, Locati M. The Chemokine System in Diverse Forms of Macrophage Activation and Polarization. Trends Immunol (2004) 25(12):677–86. doi: 10.1016/j.it.2004.09.015
10. Porta C, Riboldi E, Ippolito A, Sica A. Molecular and Epigenetic Basis of Macrophage Polarized Activation. Semin Immunol (2015) 27(4):237–48. doi: 10.1016/j.smim.2015.10.003
11. Weisser SB, McLarren KW, Kuroda E, Sly LM. Generation and Characterization of Murine Alternatively Activated Macrophages. Methods Mol Biol (Clifton NJ) (2013) 946:225–39. doi: 10.1007/978-1-62703-128-8_14
12. Walker DG. Spleen Cells Transmit Osteopetrosis in Mice. Science (1975) 190(4216):785–7. doi: 10.1126/science.1198094
13. Walker DG. Bone Resorption Restored in Osteopetrotic Mice by Transplants of Normal Bone Marrow and Spleen Cells. Science (1975) 190(4216):784–5. doi: 10.1126/science.1105786
14. Scheven BA, Visser JW, Nijweide PJ. In Vitro Osteoclast Generation From Different Bone Marrow Fractions, Including a Highly Enriched Haematopoietic Stem Cell Population. Nature (1986) 321(6065):79–81. doi: 10.1038/321079a0
15. Udagawa N, Takahashi N, Akatsu T, Tanaka H, Sasaki T, Nishihara T, et al. Origin of Osteoclasts: Mature Monocytes and Macrophages Are Capable of Differentiating Into Osteoclasts Under a Suitable Microenvironment Prepared by Bone Marrow-Derived Stromal Cells. Proc Natl Acad Sci (1990) 87(18):7260–4. doi: 10.1073/pnas.87.18.7260
16. Chang MK, Raggatt LJ, Alexander KA, Kuliwaba JS, Fazzalari NL, Schroder K, et al. Osteal Tissue Macrophages Are Intercalated Throughout Human and Mouse Bone Lining Tissues and Regulate Osteoblast Function In Vitro and In Vivo. J Immunol (2008) 181(2):1232–44. doi: 10.4049/jimmunol.181.2.1232
17. Bar-Shavit Z. The Osteoclast: A Multinucleated, Hematopoietic-Origin, Bone-Resorbing Osteoimmune Cell. J Cell Biochem (2007) 102(5):1130–9. doi: 10.1002/jcb.21553
18. Quinn JM, McGee JO, Athanasou NA. Human Tumour-Associated Macrophages Differentiate Into Osteoclastic Bone-Resorbing Cells. J Pathol (1998) 184(1):31–6. doi: 10.1002/(sici)1096-9896(199801)184:1<31::Aid-path962>3.0.Co;2-v
19. Quinn JM, Neale S, Fujikawa Y, McGee JO, Athanasou NA. Human Osteoclast Formation From Blood Monocytes, Peritoneal Macrophages, and Bone Marrow Cells. Calcified Tissue Int (1998) 62(6):527–31. doi: 10.1007/s002239900473
20. Wiktor-Jedrzejczak W, Bartocci A, Ferrante AW Jr, Ahmed-Ansari A, Sell KW, Pollard JW, et al. Total Absence of Colony-Stimulating Factor 1 in the Macrophage-Deficient Osteopetrotic (Op/Op) Mouse. Proc Natl Acad Sci USA (1990) 87(12):4828–32. doi: 10.1073/pnas.87.12.4828
21. Yang D, Wan Y. Molecular Determinants for the Polarization of Macrophage and Osteoclast. Semin Immunopathol (2019) 41(5):551–63. doi: 10.1007/s00281-019-00754-3
22. Theill LE, Boyle WJ, Penninger JM. RANK-L and RANK: T Cells, Bone Loss, and Mammalian Evolution. Annu Rev Immunol (2002) 20(1):795–823. doi: 10.1146/annurev.immunol.20.100301.064753
23. Roodman GD. Regulation of Osteoclast Differentiation. Ann NY Acad Sci (2006) 1068(1):100–9. doi: 10.1196/annals.1346.013
24. Xiao Y, Palomero J, Grabowska J, Wang L, de Rink I, van Helvert L, et al. Macrophages and Osteoclasts Stem From a Bipotent Progenitor Downstream of a Macrophage/Osteoclast/Dendritic Cell Progenitor. Blood Adv (2017) 1(23):1993–2006. doi: 10.1182/bloodadvances.2017008540
25. Miyamoto T, Ohneda O, Arai F, Iwamoto K, Okada S, Takagi K, et al. Bifurcation of Osteoclasts and Dendritic Cells From Common Progenitors. Blood J Am Soc Hematol (2001) 98(8):2544–54. doi: 10.1182/blood.V98.8.2544
26. Yao Y, Cai X, Ren F, Ye Y, Wang F, Zheng C, et al. The Macrophage-Osteoclast Axis in Osteoimmunity and Osteo-Related Diseases. Front Immunol (2021) 12:1066. doi: 10.3389/fimmu.2021.664871
27. Adamopoulos IE, Sabokbar A, Wordsworth BP, Carr A, Ferguson DJ, Athanasou NA. Synovial Fluid Macrophages Are Capable of Osteoclast Formation and Resorption. J Pathol (2006) 208(1):35–43. doi: 10.1002/path.1891
28. Speziani C, Rivollier A, Gallois A, Coury F, Mazzorana M, Azocar O, et al. Murine Dendritic Cell Transdifferentiation Into Osteoclasts Is Differentially Regulated by Innate and Adaptive Cytokines. Eur J Immunol (2007) 37(3):747–57. doi: 10.1002/eji.200636534
29. Liu W, Zhang Y, Zhu W, Ma C, Ruan J, Long H, et al. Sinomenine Inhibits the Progression of Rheumatoid Arthritis by Regulating the Secretion of Inflammatory Cytokines and Monocyte/Macrophage Subsets. Front Immunol (2018) 9:2228. doi: 10.3389/fimmu.2018.02228
30. Ziegler-Heitbrock L, Ancuta P, Crowe S, Dalod M, Grau V, Hart DN, et al. Nomenclature of Monocytes and Dendritic Cells in Blood. Blood J Am Soc Hematol (2010) 116(16):e74–80. doi: 10.1182/blood-2010-02-258558
31. Cren M, Nziza N, Carbasse A, Mahe P, Dufourcq-Lopez E, Delpont M, et al. Differential Accumulation and Activation of Monocyte and Dendritic Cell Subsets in Inflamed Synovial Fluid Discriminates Between Juvenile Idiopathic Arthritis and Septic Arthritis. Front Immunol (2020) 11:1716. doi: 10.3389/fimmu.2020.01716
32. Ravenhill BJ, Soday L, Houghton J, Antrobus R, Weekes MP. Comprehensive Cell Surface Proteomics Defines Markers of Classical, Intermediate and Non-Classical Monocytes. Sci Rep (2020) 10(1):1–11. doi: 10.1038/s41598-020-61356-w
33. Sprangers S, Schoenmaker T, Cao Y, Everts V, De Vries TJ. Different Blood-Borne Human Osteoclast Precursors Respond in Distinct Ways to IL-17a. J Cell Physiol (2016) 231(6):1249–60. doi: 10.1002/jcp.25220
34. Wypasek E, Padjas A, Szymańska M, Plens K, Siedlar M, Undas A. Non-Classical and Intermediate Monocytes in Patients Following Venous Thromboembolism: Links With Inflammation. Adv Clin Exp Med (2019) 28(1):51–8. doi: 10.17219/acem/76262
35. Rana AK, Li Y, Dang Q, Yang F. Monocytes in Rheumatoid Arthritis: Circulating Precursors of Macrophages and Osteoclasts and, Their Heterogeneity and Plasticity Role in RA Pathogenesis. Int Immunopharmacol (2018) 65:348–59. doi: 10.1016/j.intimp.2018.10.016
36. Yahara Y, Barrientos T, Tang YJ, Puviindran V, Nadesan P, Zhang H, et al. Erythromyeloid Progenitors Give Rise to a Population of Osteoclasts That Contribute to Bone Homeostasis and Repair. Nat Cell Biol (2020) 22(1):49–59. doi: 10.1038/s41556-019-0437-8
37. Jacome-Galarza CE, Percin GI, Muller JT, Mass E, Lazarov T, Eitler J, et al. Developmental Origin, Functional Maintenance and Genetic Rescue of Osteoclasts. Nature (2019) 568(7753):541–5. doi: 10.1038/s41586-019-1105-7
38. Gordon S. Elie Metchnikoff: Father of Natural Immunity. Eur J Immunol (2008) 38(12):3257–64. doi: 10.1002/eji.200838855
39. Mosser DM, Edwards JP. Exploring the Full Spectrum of Macrophage Activation. Nat Rev Immunol (2008) 8(12):958–69. doi: 10.1038/nri2448
40. Murray PJ, Allen JE, Biswas SK, Fisher EA, Gilroy DW, Goerdt S, et al. Macrophage Activation and Polarization: Nomenclature and Experimental Guidelines. Immunity (2014) 41(1):14–20. doi: 10.1016/j.immuni.2014.06.008
41. Stein M, Keshav S, Harris N, Gordon S. Interleukin 4 Potently Enhances Murine Macrophage Mannose Receptor Activity: A Marker of Alternative Immunologic Macrophage Activation. J Exp Med (1992) 176(1):287–92. doi: 10.1084/jem.176.1.287
42. Mills CD, Kincaid K, Alt JM, Heilman MJ, Hill AM. M-1/M-2 Macrophages and the Th1/Th2 Paradigm. J Immunol (2000) 164(12):6166–73. doi: 10.4049/jimmunol.164.12.6166
43. Mantovani A, Sica A, Locati M. Macrophage Polarization Comes of Age. Immunity (2005) 23(4):344–6. doi: 10.1016/j.immuni.2005.10.001
44. Martinez FO, Gordon S. The M1 and M2 Paradigm of Macrophage Activation: Time for Reassessment. F1000prime Rep (2014) 6:13. doi: 10.12703/p6-13
45. Liu YC, Zou XB, Chai YF, Yao YM. Macrophage Polarization in Inflammatory Diseases. Int J Biol Sci (2014) 10(5):520–9. doi: 10.7150/ijbs.8879
46. Sica A, Mantovani A. Macrophage Plasticity and Polarization: In Vivo Veritas. J Clin Invest (2012) 122(3):787–95. doi: 10.1172/jci59643
47. Tan HY, Wang N, Li S, Hong M, Wang X, Feng Y. The Reactive Oxygen Species in Macrophage Polarization: Reflecting Its Dual Role in Progression and Treatment of Human Diseases. Oxid Med Cell Longevity (2016) 2016:2795090. doi: 10.1155/2016/2795090
48. Wang N, Liang H, Zen K. Molecular Mechanisms That Influence the Macrophage M1-M2 Polarization Balance. Front Immunol (2014) 5:614. doi: 10.3389/fimmu.2014.00614
49. Zhou D, Huang C, Lin Z, Zhan S, Kong L, Fang C, et al. Macrophage Polarization and Function With Emphasis on the Evolving Roles of Coordinated Regulation of Cellular Signaling Pathways. Cell Signalling (2014) 26(2):192–7. doi: 10.1016/j.cellsig.2013.11.004
50. Genin M, Clement F, Fattaccioli A, Raes M, Michiels C. M1 and M2 Macrophages Derived From THP-1 Cells Differentially Modulate the Response of Cancer Cells to Etoposide. BMC Cancer (2015) 15:577. doi: 10.1186/s12885-015-1546-9
51. Mosser DM. The Many Faces of Macrophage Activation. J Leukocyte Biol (2003) 73(2):209–12. doi: 10.1189/jlb.0602325
52. Guihard P, Danger Y, Brounais B, David E, Brion R, Delecrin J, et al. Induction of Osteogenesis in Mesenchymal Stem Cells by Activated Monocytes/Macrophages Depends on Oncostatin M Signaling. Stem Cells (Dayton Ohio) (2012) 30(4):762–72. doi: 10.1002/stem.1040
53. Woo SR, Corrales L, Gajewski TF. Innate Immune Recognition of Cancer. Annu Rev Immunol (2015) 33:445–74. doi: 10.1146/annurev-immunol-032414-112043
54. Guo Y, Cao W, Zhu Y. Immunoregulatory Functions of the IL-12 Family of Cytokines in Antiviral Systems. Viruses (2019) 11(9):772. doi: 10.3390/v11090772
55. Champagne CM, Takebe J, Offenbacher S, Cooper LF. Macrophage Cell Lines Produce Osteoinductive Signals That Include Bone Morphogenetic Protein-2. Bone (2002) 30(1):26–31. doi: 10.1016/s8756-3282(01)00638-x
56. Li CJ, Xiao Y, Yang M, Su T, Sun X, Guo Q, et al. Long Noncoding RNA Bmncr Regulates Mesenchymal Stem Cell Fate During Skeletal Aging. J Clin Invest (2018) 128(12):5251–66. doi: 10.1172/jci99044
57. Assoian RK, Fleurdelys BE, Stevenson HC, Miller PJ, Madtes DK, Raines EW, et al. Expression and Secretion of Type Beta Transforming Growth Factor by Activated Human Macrophages. Proc Natl Acad Sci USA (1987) 84(17):6020–4. doi: 10.1073/pnas.84.17.6020
58. Takahashi F, Takahashi K, Shimizu K, Cui R, Tada N, Takahashi H, et al. Osteopontin Is Strongly Expressed by Alveolar Macrophages in the Lungs of Acute Respiratory Distress Syndrome. Lung (2004) 182(3):173–85. doi: 10.1007/s00408-004-0309-1
59. Kreutz M, Andreesen R, Krause SW, Szabo A, Ritz E, Reichel H. 1,25-Dihydroxyvitamin D3 Production and Vitamin D3 Receptor Expression Are Developmentally Regulated During Differentiation of Human Monocytes Into Macrophages. Blood (1993) 82(4):1300–7. doi: 10.1182/blood.V82.4.1300.bloodjournal8241300
60. Nakashima T, Hayashi M, Takayanagi H. New Insights Into Osteoclastogenic Signaling Mechanisms. Trends Endocrinol Metabol: TEM (2012) 23(11):582–90. doi: 10.1016/j.tem.2012.05.005
61. Takayanagi H. Osteoimmunology: Shared Mechanisms and Crosstalk Between the Immune and Bone Systems. Nat Rev Immunol (2007) 7(4):292–304. doi: 10.1038/nri2062
62. Trouillet-Assant S, Gallet M, Nauroy P, Rasigade JP, Flammier S, Parroche P, et al. Dual Impact of Live Staphylococcus Aureus on the Osteoclast Lineage, Leading to Increased Bone Resorption. J Infect Dis (2015) 211(4):571–81. doi: 10.1093/infdis/jiu386
63. Pape HC, Marcucio R, Humphrey C, Colnot C, Knobe M, Harvey EJ. Trauma-Induced Inflammation and Fracture Healing. J Orthopaedic Trauma (2010) 24(9):522–5. doi: 10.1097/BOT.0b013e3181ed1361
64. Horwood NJ, Elliott J, Martin TJ, Gillespie MT. Osteotropic Agents Regulate the Expression of Osteoclast Differentiation Factor and Osteoprotegerin in Osteoblastic Stromal Cells. Endocrinology (1998) 139(11):4743–6. doi: 10.1210/endo.139.11.6433
65. Kobayashi K, Takahashi N, Jimi E, Udagawa N, Takami M, Kotake S, et al. Tumor Necrosis Factor Alpha Stimulates Osteoclast Differentiation by a Mechanism Independent of the ODF/RANKL-RANK Interaction. J Exp Med (2000) 191(2):275–86. doi: 10.1084/jem.191.2.275
66. Zhao Z, Hou X, Yin X, Li Y, Duan R, Boyce BF, et al. TNF Induction of NF-κb RelB Enhances RANKL-Induced Osteoclastogenesis by Promoting Inflammatory Macrophage Differentiation But Also Limits It Through Suppression of NFATc1 Expression. PloS One (2015) 10(8):e0135728. doi: 10.1371/journal.pone.0135728
67. Kim JH, Jin HM, Kim K, Song I, Youn BU, Matsuo K, et al. The Mechanism of Osteoclast Differentiation Induced by IL-1. J Immunol (Baltimore Md 1950) (2009) 183(3):1862–70. doi: 10.4049/jimmunol.0803007
68. Jules J, Zhang P, Ashley JW, Wei S, Shi Z, Liu J, et al. Molecular Basis of Requirement of Receptor Activator of Nuclear Factor κb Signaling for Interleukin 1-Mediated Osteoclastogenesis. J Biol Chem (2012) 287(19):15728–38. doi: 10.1074/jbc.M111.296228
69. Dinarello CA. The Interleukin-1 Family: 10 Years of Discovery. FASEB J Off Publ Fed Am Soc Exp Biol (1994) 8(15):1314–25. doi: 10.1096/fasebj.8.15.8001745
70. Wei S, Kitaura H, Zhou P, Ross FP, Teitelbaum SL. IL-1 Mediates TNF-Induced Osteoclastogenesis. J Clin Invest (2005) 115(2):282–90. doi: 10.1172/jci23394
71. Chen Z, Su L, Xu Q, Katz J, Michalek SM, Fan M, et al. IL-1r/TLR2 Through MyD88 Divergently Modulates Osteoclastogenesis Through Regulation of Nuclear Factor of Activated T Cells C1 (NFATc1) and B Lymphocyte-Induced Maturation Protein-1 (Blimp1). J Biol Chem (2015) 290(50):30163–74. doi: 10.1074/jbc.M115.663518
72. Lee B, Kim TH, Jun JB, Yoo DH, Woo JH, Choi SJ, et al. Direct Inhibition of Human RANK+ Osteoclast Precursors Identifies a Homeostatic Function of IL-1beta. J Immunol (Baltimore Md 1950) (2010) 185(10):5926–34. doi: 10.4049/jimmunol.1001591
73. O’Brien CA, Nakashima T, Takayanagi H. Osteocyte Control of Osteoclastogenesis. Bone (2013) 54(2):258–63. doi: 10.1016/j.bone.2012.08.121
74. Wu Q, Zhou X, Huang D, Ji Y, Kang F. IL-6 Enhances Osteocyte-Mediated Osteoclastogenesis by Promoting JAK2 and RANKL Activity In Vitro. Cell Physiol Biochem Int J Exp Cell Physiol Biochem Pharmacol (2017) 41(4):1360–9. doi: 10.1159/000465455
75. Devlin RD, Reddy SV, Savino R, Ciliberto G, Roodman GD. IL-6 Mediates the Effects of IL-1 or TNF, But Not PTHrP or 1,25(OH)2D3, on Osteoclast-Like Cell Formation in Normal Human Bone Marrow Cultures. J Bone Mineral Res Off J Am Soc Bone Mineral Res (1998) 13(3):393–9. doi: 10.1359/jbmr.1998.13.3.393
76. Teng MW, Bowman EP, McElwee JJ, Smyth MJ, Casanova JL, Cooper AM, et al. IL-12 and IL-23 Cytokines: From Discovery to Targeted Therapies for Immune-Mediated Inflammatory Diseases. Nat Med (2015) 21(7):719–29. doi: 10.1038/nm.3895
77. Trinchieri G. Interleukin-12 and the Regulation of Innate Resistance and Adaptive Immunity. Nat Rev Immunol (2003) 3(2):133–46. doi: 10.1038/nri1001
78. Vignali DA, Kuchroo VK. IL-12 Family Cytokines: Immunological Playmakers. Nat Immunol (2012) 13(8):722–8. doi: 10.1038/ni.2366
79. Gao Y, Grassi F, Ryan MR, Terauchi M, Page K, Yang X, et al. IFN-Gamma Stimulates Osteoclast Formation and Bone Loss In Vivo via Antigen-Driven T Cell Activation. J Clin Invest (2007) 117(1):122–32. doi: 10.1172/jci30074
80. Xu J, Wang Y, Li J, Zhang X, Geng Y, Huang Y, et al. IL-12p40 Impairs Mesenchymal Stem Cell-Mediated Bone Regeneration via CD4(+) T Cells. Cell Death Differ (2016) 23(12):1941–51. doi: 10.1038/cdd.2016.72
81. Kamolmatyakul S, Chen W, Li YP. Interferon-Gamma Down-Regulates Gene Expression of Cathepsin K in Osteoclasts and Inhibits Osteoclast Formation. J Dental Res (2001) 80(1):351–5. doi: 10.1177/00220345010800011001
82. Takayanagi H, Kim S, Taniguchi T. Signaling Crosstalk Between RANKL and Interferons in Osteoclast Differentiation. Arthritis Res (2002) 4 Suppl 3(Suppl 3):S227–32. doi: 10.1186/ar581
83. Takayanagi H, Ogasawara K, Hida S, Chiba T, Murata S, Sato K, et al. T-Cell-Mediated Regulation of Osteoclastogenesis by Signalling Cross-Talk Between RANKL and IFN-Gamma. Nature (2000) 408(6812):600–5. doi: 10.1038/35046102
84. Horwood NJ, Elliott J, Martin TJ, Gillespie MT. IL-12 Alone and in Synergy With IL-18 Inhibits Osteoclast Formation In Vitro. J Immunol (Baltimore Md 1950) (2001) 166(8):4915–21. doi: 10.4049/jimmunol.166.8.4915
85. Nagata N, Kitaura H, Yoshida N, Nakayama K. Inhibition of RANKL-Induced Osteoclast Formation in Mouse Bone Marrow Cells by IL-12: Involvement of IFN-Gamma Possibly Induced From Non-T Cell Population. Bone (2003) 33(4):721–32. doi: 10.1016/s8756-3282(03)00213-8
86. Shanmugarajan S, Kawanabe N, Koide M, Tsuruga E, Arroyo JE, Key LL Jr, et al. IL-12 Stimulates the Osteoclast Inhibitory Peptide-1 (OIP-1/Hsca) Gene Expression in CD4+ T Cells. J Cell Biochem (2009) 107(1):104–11. doi: 10.1002/jcb.22104
87. Amcheslavsky A, Bar-Shavit Z. Interleukin (IL)-12 Mediates the Anti-Osteoclastogenic Activity of CpG-Oligodeoxynucleotides. J Cell Physiol (2006) 207(1):244–50. doi: 10.1002/jcp.20563
88. Kitaura H, Nagata N, Fujimura Y, Hotokezaka H, Yoshida N, Nakayama K. Effect of IL-12 on TNF-Alpha-Mediated Osteoclast Formation in Bone Marrow Cells: Apoptosis Mediated by Fas/Fas Ligand Interaction. J Immunol (Baltimore Md 1950) (2002) 169(9):4732–8. doi: 10.4049/jimmunol.169.9.4732
89. Ahn HJ, Maruo S, Tomura M, Mu J, Hamaoka T, Nakanishi K, et al. A Mechanism Underlying Synergy Between IL-12 and IFN-Gamma-Inducing Factor in Enhanced Production of IFN-Gamma. J Immunol (Baltimore Md 1950) (1997) 159(5):2125–31.
90. Micallef MJ, Ohtsuki T, Kohno K, Tanabe F, Ushio S, Namba M, et al. Interferon-Gamma-Inducing Factor Enhances T Helper 1 Cytokine Production by Stimulated Human T Cells: Synergism With Interleukin-12 for Interferon-Gamma Production. Eur J Immunol (1996) 26(7):1647–51. doi: 10.1002/eji.1830260736
91. Munder M, Mallo M, Eichmann K, Modolell M. Murine Macrophages Secrete Interferon Gamma Upon Combined Stimulation With Interleukin (IL)-12 and IL-18: A Novel Pathway of Autocrine Macrophage Activation. J Exp Med (1998) 187(12):2103–8. doi: 10.1084/jem.187.12.2103
92. Robinson D, Shibuya K, Mui A, Zonin F, Murphy E, Sana T, et al. IGIF Does Not Drive Th1 Development But Synergizes With IL-12 for Interferon-Gamma Production and Activates IRAK and NFkappaB. Immunity (1997) 7(4):571–81. doi: 10.1016/s1074-7613(00)80378-7
93. Iwasaki T, Yamashita K, Tsujimura T, Kashiwamura S, Tsutsui H, Kaisho T, et al. Interleukin-18 Inhibits Osteolytic Bone Metastasis by Human Lung Cancer Cells Possibly Through Suppression of Osteoclastic Bone-Resorption in Nude Mice. J Immunother (Hagerstown Md 1997) (2002) 25 Suppl 1:S52–60. doi: 10.1097/00002371-200203001-00008
94. Horwood NJ, Udagawa N, Elliott J, Grail D, Okamura H, Kurimoto M, et al. Interleukin 18 Inhibits Osteoclast Formation via T Cell Production of Granulocyte Macrophage Colony-Stimulating Factor. J Clin Invest (1998) 101(3):595–603. doi: 10.1172/jci1333
95. Kitaura H, Fujimura Y, Yoshimatsu M, Kohara H, Morita Y, Aonuma T, et al. IL-12- and IL-18-Mediated, Nitric Oxide-Induced Apoptosis in TNF-α-Mediated Osteoclastogenesis of Bone Marrow Cells. Calcified Tissue Int (2011) 89(1):65–73. doi: 10.1007/s00223-011-9494-0
96. Dai SM, Nishioka K, Yudoh K. Interleukin (IL) 18 Stimulates Osteoclast Formation Through Synovial T Cells in Rheumatoid Arthritis: Comparison With IL1 Beta and Tumour Necrosis Factor Alpha. Ann Rheum Dis (2004) 63(11):1379–86. doi: 10.1136/ard.2003.018481
97. Chen L, Wei XQ, Evans B, Jiang W, Aeschlimann D. IL-23 Promotes Osteoclast Formation by Up-Regulation of Receptor Activator of NF-kappaB (RANK) Expression in Myeloid Precursor Cells. Eur J Immunol (2008) 38(10):2845–54. doi: 10.1002/eji.200838192
98. Ju JH, Cho ML, Moon YM, Oh HJ, Park JS, Jhun JY, et al. IL-23 Induces Receptor Activator of NF-kappaB Ligand Expression on CD4+ T Cells and Promotes Osteoclastogenesis in an Autoimmune Arthritis Model. J Immunol (Baltimore Md 1950) (2008) 181(2):1507–18. doi: 10.4049/jimmunol.181.2.1507
99. Kitami S, Tanaka H, Kawato T, Tanabe N, Katono-Tani T, Zhang F, et al. IL-17A Suppresses the Expression of Bone Resorption-Related Proteinases and Osteoclast Differentiation via IL-17RA or IL-17RC Receptors in RAW264.7 Cells. Biochimie (2010) 92(4):398–404. doi: 10.1016/j.biochi.2009.12.011
100. Ha J, Choi HS, Lee Y, Kwon HJ, Song YW, Kim HH. CXC Chemokine Ligand 2 Induced by Receptor Activator of NF-Kappa B Ligand Enhances Osteoclastogenesis. J Immunol (Baltimore Md 1950) (2010) 184(9):4717–24. doi: 10.4049/jimmunol.0902444
101. Pathak JL, Bakker AD, Verschueren P, Lems WF, Luyten FP, Klein-Nulend J, et al. CXCL8 and CCL20 Enhance Osteoclastogenesis via Modulation of Cytokine Production by Human Primary Osteoblasts. PloS One (2015) 10(6):e0131041. doi: 10.1371/journal.pone.0131041
102. Kwak HB, Ha H, Kim HN, Lee JH, Kim HS, Lee S, et al. Reciprocal Cross-Talk Between RANKL and Interferon-Gamma-Inducible Protein 10 Is Responsible for Bone-Erosive Experimental Arthritis. Arthritis Rheum (2008) 58(5):1332–42. doi: 10.1002/art.23372
103. Xuan W, Feng X, Qian C, Peng L, Shi Y, Xu L, et al. Osteoclast Differentiation Gene Expression Profiling Reveals Chemokine CCL4 Mediates RANKL-Induced Osteoclast Migration and Invasion via PI3K Pathway. Cell Biochem Funct (2017) 35(3):171–7. doi: 10.1002/cbf.3260
104. Kalyanaraman H, Ramdani G, Joshua J, Schall N, Boss GR, Cory E, et al. A Novel, Direct NO Donor Regulates Osteoblast and Osteoclast Functions and Increases Bone Mass in Ovariectomized Mice. J Bone Mineral Res Off J Am Soc Bone Mineral Res (2017) 32(1):46–59. doi: 10.1002/jbmr.2909
105. Lee SK, Huang H, Lee SW, Kim KH, Kim KK, Kim HM, et al. Involvement of iNOS-Dependent NO Production in the Stimulation of Osteoclast Survival by TNF-Alpha. Exp Cell Res (2004) 298(2):359–68. doi: 10.1016/j.yexcr.2004.04.039
106. van’t Hof RJ, Macphee J, Libouban H, Helfrich MH, Ralston SH. Regulation of Bone Mass and Bone Turnover by Neuronal Nitric Oxide Synthase. Endocrinology (2004) 145(11):5068–74. doi: 10.1210/en.2004-0205
107. Bax BE, Alam AS, Banerji B, Bax CM, Bevis PJ, Stevens CR, et al. Stimulation of Osteoclastic Bone Resorption by Hydrogen Peroxide. Biochem Biophys Res Commun (1992) 183(3):1153–8. doi: 10.1016/s0006-291x(05)80311-0
108. Lee NK, Choi YG, Baik JY, Han SY, Jeong DW, Bae YS, et al. A Crucial Role for Reactive Oxygen Species in RANKL-Induced Osteoclast Differentiation. Blood (2005) 106(3):852–9. doi: 10.1182/blood-2004-09-3662
109. Callaway DA, Jiang JX. Reactive Oxygen Species and Oxidative Stress in Osteoclastogenesis, Skeletal Aging and Bone Diseases. J Bone Mineral Metab (2015) 33(4):359–70. doi: 10.1007/s00774-015-0656-4
110. Liu Y, Wang C, Wang G, Sun Y, Deng Z, Chen L, et al. Loureirin B Suppresses RANKL-Induced Osteoclastogenesis and Ovariectomized Osteoporosis via Attenuating NFATc1 and ROS Activities. Theranostics (2019) 9(16):4648–62. doi: 10.7150/thno.35414
111. Park CK, Lee Y, Kim KH, Lee ZH, Joo M, Kim HH. Nrf2 Is a Novel Regulator of Bone Acquisition. Bone (2014) 63:36–46. doi: 10.1016/j.bone.2014.01.025
112. An Y, Zhang H, Wang C, Jiao F, Xu H, Wang X, et al. Activation of ROS/MAPKs/NF-κb/NLRP3 and Inhibition of Efferocytosis in Osteoclast-Mediated Diabetic Osteoporosis. FASEB J Off Publ Fed Am Soc Exp Biol (2019) 33(11):12515–27. doi: 10.1096/fj.201802805RR
113. Hofbauer L, Lacey D, Dunstan C, Spelsberg T, Riggs B, Khosla S. Interleukin-1β and Tumor Necrosis Factor-α, But Not Interleukin-6, Stimulate Osteoprotegerin Ligand Gene Expression in Human Osteoblastic Cells. Bone (1999) 25(3):255–9. doi: 10.1016/S8756-3282(99)00162-3
114. Rose-John S. Interleukin-6 Family Cytokines. Cold Spring Harbor Perspect Biol (2018) 10(2):a028415. doi: 10.1101/cshperspect.a028415
115. Tanaka T, Narazaki M, Kishimoto T. IL-6 in Inflammation, Immunity, and Disease. Cold Spring Harbor Perspect Biol (2014) 6(10):a016295. doi: 10.1101/cshperspect.a016295
116. Deng Z, Zhang R, Li M, Wang S, Fu G, Jin J, et al. STAT3/IL-6 Dependent Induction of Inflammatory Response in Osteoblast and Osteoclast Formation in Nanoscale Wear Particle-Induced Aseptic Prosthesis Loosening. Biomater Sci (2021) 9(4):1291–300. doi: 10.1039/d0bm01256d
117. Udagawa N, Takahashi N, Katagiri T, Tamura T, Wada S, Findlay DM, et al. Interleukin (IL)-6 Induction of Osteoclast Differentiation Depends on IL-6 Receptors Expressed on Osteoblastic Cells But Not on Osteoclast Progenitors. J Exp Med (1995) 182(5):1461–8. doi: 10.1084/jem.182.5.1461
118. Akbari M, Hassan-Zadeh V. IL-6 Signalling Pathways and the Development of Type 2 Diabetes. Inflammopharmacology (2018) 26(3):685–98. doi: 10.1007/s10787-018-0458-0
119. Gowen M, Wood DD, Ihrie EJ, McGuire MK, Russell RG. An Interleukin 1 Like Factor Stimulates Bone Resorption In Vitro. Nature (1983) 306(5941):378–80. doi: 10.1038/306378a0
120. Dunne A, O’Neill LA. The Interleukin-1 Receptor/Toll-Like Receptor Superfamily: Signal Transduction During Inflammation and Host Defense. Sci STKE Signal Transduct Knowledge Environ (2003) 2003(171):re3. doi: 10.1126/stke.2003.171.re3
121. Jimi E, Nakamura I, Ikebe T, Akiyama S, Takahashi N, Suda T. Activation of NF-KappaB Is Involved in the Survival of Osteoclasts Promoted by Interleukin-1. J Biol Chem (1998) 273(15):8799–805. doi: 10.1074/jbc.273.15.8799
122. Adamopoulos IE, Tessmer M, Chao CC, Adda S, Gorman D, Petro M, et al. IL-23 Is Critical for Induction of Arthritis, Osteoclast Formation, and Maintenance of Bone Mass. J Immunol (2011) 187(2):951–9. doi: 10.4049/jimmunol.1003986
123. Bigarella CL, Liang R, Ghaffari S. Stem Cells and the Impact of ROS Signaling. Development (2014) 141(22):4206–18. doi: 10.1242/dev.107086
124. Stolina M, Dwyer D, Niu QT, Villasenor KS, Kurimoto P, Grisanti M, et al. Temporal Changes in Systemic and Local Expression of Bone Turnover Markers During Six Months of Sclerostin Antibody Administration to Ovariectomized Rats. Bone (2014) 67:305–13. doi: 10.1016/j.bone.2014.07.031
125. Darden AG, Ries WL, Wolf WC, Rodriguiz RM, Key LL Jr. Osteoclastic Superoxide Production and Bone Resorption: Stimulation and Inhibition by Modulators of NADPH Oxidase. J Bone Mineral Res Off J Am Soc Bone Mineral Res (1996) 11(5):671–5. doi: 10.1002/jbmr.5650110515
126. Garrett IR, Boyce BF, Oreffo RO, Bonewald L, Poser J, Mundy GR. Oxygen-Derived Free Radicals Stimulate Osteoclastic Bone Resorption in Rodent Bone In Vitro and In Vivo. J Clin Invest (1990) 85(3):632–9. doi: 10.1172/jci114485
127. Steinbeck MJ, Appel WH Jr, Verhoeven AJ, Karnovsky MJ. NADPH-Oxidase Expression and In Situ Production of Superoxide by Osteoclasts Actively Resorbing Bone. J Cell Biol (1994) 126(3):765–72. doi: 10.1083/jcb.126.3.765
128. Palmer RM, Ferrige AG, Moncada S. Nitric Oxide Release Accounts for the Biological Activity of Endothelium-Derived Relaxing Factor. Nature (1987) 327(6122):524–6. doi: 10.1038/327524a0
129. Moncada S, Higgs A. The L-Arginine-Nitric Oxide Pathway. N Engl J Med (1993) 329(27):2002–12. doi: 10.1056/nejm199312303292706
130. Ignarro LJ. Nitric Oxide: Biology and Pathobiology. Los Angeles, California: Academic press (2000).
131. Williams DLH. A Chemist’s View of the Nitric Oxide Story. Organic Biomol Chem (2003) 1(3):441–9. doi: 10.1039/b209748f
132. Jung JY, Lin AC, Ramos LM, Faddis BT, Chole RA. Nitric Oxide Synthase I Mediates Osteoclast Activity In Vitro and In Vivo. J Cell Biochem (2003) 89(3):613–21. doi: 10.1002/jcb.10527
133. Zheng H, Yu X, Collin-Osdoby P, Osdoby P. RANKL Stimulates Inducible Nitric-Oxide Synthase Expression and Nitric Oxide Production in Developing Osteoclasts. An Autocrine Negative Feedback Mechanism Triggered by RANKL-Induced Interferon-Beta via NF-KappaB That Restrains Osteoclastogenesis and Bone Resorption. J Biol Chem (2006) 281(23):15809–20. doi: 10.1074/jbc.M513225200
134. Brandi ML, Hukkanen M, Umeda T, Moradi-Bidhendi N, Bianchi S, Gross SS, et al. Bidirectional Regulation of Osteoclast Function by Nitric Oxide Synthase Isoforms. Proc Natl Acad Sci USA (1995) 92(7):2954–8. doi: 10.1073/pnas.92.7.2954
135. Löwik CW, Nibbering PH, van de Ruit M, Papapoulos SE. Inducible Production of Nitric Oxide in Osteoblast-Like Cells and in Fetal Mouse Bone Explants Is Associated With Suppression of Osteoclastic Bone Resorption. J Clin Invest (1994) 93(4):1465–72. doi: 10.1172/jci117124
136. Ralston SH, Ho LP, Helfrich MH, Grabowski PS, Johnston PW, Benjamin N. Nitric Oxide: A Cytokine-Induced Regulator of Bone Resorption. J Bone Mineral Res Off J Am Soc Bone Mineral Res (1995) 10(7):1040–9. doi: 10.1002/jbmr.5650100708
137. Mackay CR. Chemokines: Immunology’s High Impact Factors. Nat Immunol (2001) 2(2):95–101. doi: 10.1038/84298
138. Lee SH, Kim TS, Choi Y, Lorenzo J. Osteoimmunology: Cytokines and the Skeletal System. BMB Rep (2008) 41(7):495–510. doi: 10.5483/bmbrep.2008.41.7.495
139. Kuan WP, Tam LS, Wong CK, Ko FW, Li T, Zhu T, et al. CXCL 9 and CXCL 10 as Sensitive Markers of Disease Activity in Patients With Rheumatoid Arthritis. J Rheumatol (2010) 37(2):257–64. doi: 10.3899/jrheum.090769
140. Yu X, Huang Y, Collin-Osdoby P, Osdoby P. Stromal Cell-Derived Factor-1 (SDF-1) Recruits Osteoclast Precursors by Inducing Chemotaxis, Matrix Metalloproteinase-9 (MMP-9) Activity, and Collagen Transmigration. J Bone Mineral Res Off J Am Soc Bone Mineral Res (2003) 18(8):1404–18. doi: 10.1359/jbmr.2003.18.8.1404
141. Yu X, Huang Y, Collin-Osdoby P, Osdoby P. CCR1 Chemokines Promote the Chemotactic Recruitment, RANKL Development, and Motility of Osteoclasts and Are Induced by Inflammatory Cytokines in Osteoblasts. J Bone Mineral Res Off J Am Soc Bone Mineral Res (2004) 19(12):2065–77. doi: 10.1359/jbmr.040910
142. Tanaka K, Yamagata K, Kubo S, Nakayamada S, Sakata K, Matsui T, et al. Glycolaldehyde-Modified Advanced Glycation End-Products Inhibit Differentiation of Human Monocytes Into Osteoclasts via Upregulation of IL-10. Bone (2019) 128:115034. doi: 10.1016/j.bone.2019.115034
143. Xu H, Zhao H, Lu C, Qiu Q, Wang G, Huang J, et al. Triptolide Inhibits Osteoclast Differentiation and Bone Resorption In Vitro via Enhancing the Production of IL-10 and TGF-β1 by Regulatory T Cells. Mediators Inflamm (2016) 2016:8048170. doi: 10.1155/2016/8048170
144. Evans KE, Fox SW. Interleukin-10 Inhibits Osteoclastogenesis by Reducing NFATc1 Expression and Preventing Its Translocation to the Nucleus. BMC Cell Biol (2007) 8:4. doi: 10.1186/1471-2121-8-4
145. Liu D, Yao S, Wise GE. Effect of Interleukin-10 on Gene Expression of Osteoclastogenic Regulatory Molecules in the Rat Dental Follicle. Eur J Oral Sci (2006) 114(1):42–9. doi: 10.1111/j.1600-0722.2006.00283.x
146. Houri-Haddad Y, Soskolne WA, Halabi A, Shapira L. IL-10 Gene Transfer Attenuates P. Gingivalis-Induced Inflammation. J Dental Res (2007) 86(6):560–4. doi: 10.1177/154405910708600614
147. Pestka S, Krause CD, Sarkar D, Walter MR, Shi Y, Fisher PB. Interleukin-10 and Related Cytokines and Receptors. Annu Rev Immunol (2004) 22:929–79. doi: 10.1146/annurev.immunol.22.012703.104622
148. Rutz S, Wang X, Ouyang W. The IL-20 Subfamily of Cytokines–From Host Defence to Tissue Homeostasis. Nat Rev Immunol (2014) 14(12):783–95. doi: 10.1038/nri3766
149. Fiorentino DF, Bond MW, Mosmann TR. Two Types of Mouse T Helper Cell. IV. Th2 Clones Secrete a Factor That Inhibits Cytokine Production by Th1 Clones. J Exp Med (1989) 170(6):2081–95. doi: 10.1084/jem.170.6.2081
150. Moore KW, de Waal Malefyt R, Coffman RL, O’Garra A. Interleukin-10 and the Interleukin-10 Receptor. Annu Rev Immunol (2001) 19:683–765. doi: 10.1146/annurev.immunol.19.1.683
151. Muñoz J, Akhavan NS, Mullins AP, Arjmandi BH. Macrophage Polarization and Osteoporosis: A Review. Nutrients (2020) 12(10):2999. doi: 10.3390/nu12102999
152. Tokunaga T, Mokuda S, Kohno H, Yukawa K, Kuranobu T, Oi K, et al. Tgfβ1 Regulates Human RANKL-Induced Osteoclastogenesis via Suppression of NFATc1 Expression. Int J Mol Sci (2020) 21(3):800. doi: 10.3390/ijms21030800
153. Icer MA, Gezmen-Karadag M. The Multiple Functions and Mechanisms of Osteopontin. Clin Biochem (2018) 59:17–24. doi: 10.1016/j.clinbiochem.2018.07.003
154. Franzén A, Hultenby K, Reinholt FP, Onnerfjord P, Heinegård D. Altered Osteoclast Development and Function in Osteopontin Deficient Mice. J Orthopaedic Res Off Publ Orthopaedic Res Soc (2008) 26(5):721–8. doi: 10.1002/jor.20544
155. Qian J, Xu L, Sun X, Wang Y, Xuan W, Zhang Q, et al. Adiponectin Aggravates Bone Erosion by Promoting Osteopontin Production in Synovial Tissue of Rheumatoid Arthritis. Arthritis Res Ther (2018) 20(1):26. doi: 10.1186/s13075-018-1526-y
156. Reinholt FP, Hultenby K, Oldberg A, Heinegård D. Osteopontin–a Possible Anchor of Osteoclasts to Bone. Proc Natl Acad Sci USA (1990) 87(12):4473–5. doi: 10.1073/pnas.87.12.4473
157. Ross FP, Chappel J, Alvarez JI, Sander D, Butler WT, Farach-Carson MC, et al. Interactions Between the Bone Matrix Proteins Osteopontin and Bone Sialoprotein and the Osteoclast Integrin Alpha V Beta 3 Potentiate Bone Resorption. J Biol Chem (1993) 268(13):9901–7. doi: 10.1016/S0021-9258(18)98430-9
158. Liu H, Cui J, Feng W, Lv S, Du J, Sun J, et al. Local Administration of Calcitriol Positively Influences Bone Remodeling and Maturation During Restoration of Mandibular Bone Defects in Rats. Mater Sci Eng C Mater Biol Appl (2015) 49:14–24. doi: 10.1016/j.msec.2014.12.064
159. Lu CL, Shyu JF, Wu CC, Hung CF, Liao MT, Liu WC, et al. Association of Anabolic Effect of Calcitriol With Osteoclast-Derived Wnt 10b Secretion. Nutrients (2018) 10(9):1164. doi: 10.3390/nu10091164
160. Georgy SR, Pagel CN, Ghasem-Zadeh A, Zebaze RM, Pike RN, Sims NA, et al. Proteinase-Activated Receptor-2 Is Required for Normal Osteoblast and Osteoclast Differentiation During Skeletal Growth and Repair. Bone (2012) 50(3):704–12. doi: 10.1016/j.bone.2011.11.023
161. Li A, Cong Q, Xia X, Leong WF, Yeh J, Miao D, et al. Pharmacologic Calcitriol Inhibits Osteoclast Lineage Commitment via the BMP-Smad1 and Iκb-NF-κb Pathways. J Bone Mineral Res Off J Am Soc Bone Mineral Res (2017) 32(7):1406–20. doi: 10.1002/jbmr.3146
162. Bi CS, Li X, Qu HL, Sun LJ, An Y, Hong YL, et al. Calcitriol Inhibits Osteoclastogenesis in an Inflammatory Environment by Changing the Proportion and Function of T Helper Cell Subsets (Th2/Th17). Cell Proliferation (2020) 53(6):e12827. doi: 10.1111/cpr.12827
163. Key L, Carnes D, Cole S, Holtrop M, Bar-Shavit Z, Shapiro F, et al. Treatment of Congenital Osteopetrosis With High-Dose Calcitriol. N Engl J Med (1984) 310(7):409–15. doi: 10.1056/nejm198402163100701
164. Bouillon R, Carmeliet G, Verlinden L, van Etten E, Verstuyf A, Luderer HF, et al. Vitamin D and Human Health: Lessons From Vitamin D Receptor Null Mice. Endocr Rev (2008) 29(6):726–76. doi: 10.1210/er.2008-0004
165. Willems HME, van den Heuvel E, Schoemaker RJW, Klein-Nulend J, Bakker AD. Diet and Exercise: A Match Made in Bone. Curr Osteoporosis Rep (2017) 15(6):555–63. doi: 10.1007/s11914-017-0406-8
166. Ouyang W, Rutz S, Crellin NK, Valdez PA, Hymowitz SG. Regulation and Functions of the IL-10 Family of Cytokines in Inflammation and Disease. Annu Rev Immunol (2011) 29:71–109. doi: 10.1146/annurev-immunol-031210-101312
167. Abe E, Yamamoto M, Taguchi Y, Lecka-Czernik B, O’Brien CA, Economides AN, et al. Essential Requirement of BMPs-2/4 for Both Osteoblast and Osteoclast Formation in Murine Bone Marrow Cultures From Adult Mice: Antagonism by Noggin. J Bone Mineral Res Off J Am Soc Bone Mineral Res (2000) 15(4):663–73. doi: 10.1359/jbmr.2000.15.4.663
168. Ghosh-Choudhury N, Singha PK, Woodruff K, St Clair P, Bsoul S, Werner SL, et al. Concerted Action of Smad and CREB-Binding Protein Regulates Bone Morphogenetic Protein-2-Stimulated Osteoblastic Colony-Stimulating Factor-1 Expression. J Biol Chem (2006) 281(29):20160–70. doi: 10.1074/jbc.M511071200
169. Itoh K, Udagawa N, Katagiri T, Iemura S, Ueno N, Yasuda H, et al. Bone Morphogenetic Protein 2 Stimulates Osteoclast Differentiation and Survival Supported by Receptor Activator of Nuclear factor-kappaB Ligand. Endocrinology (2001) 142(8):3656–62. doi: 10.1210/endo.142.8.8300
170. Mandal CC, Ghosh Choudhury G, Ghosh-Choudhury N. Phosphatidylinositol 3 Kinase/Akt Signal Relay Cooperates With Smad in Bone Morphogenetic Protein-2-Induced Colony Stimulating Factor-1 (CSF-1) Expression and Osteoclast Differentiation. Endocrinology (2009) 150(11):4989–98. doi: 10.1210/en.2009-0026
171. Manolagas SC, Jilka RL. Bone Marrow, Cytokines, and Bone Remodeling. Emerging Insights Into the Pathophysiology of Osteoporosis. N Engl J Med (1995) 332(5):305–11. doi: 10.1056/nejm199502023320506
172. Ghosh-Choudhury N, Abboud SL, Nishimura R, Celeste A, Mahimainathan L, Choudhury GG. Requirement of BMP-2-Induced Phosphatidylinositol 3-Kinase and Akt Serine/Threonine Kinase in Osteoblast Differentiation and Smad-Dependent BMP-2 Gene Transcription. J Biol Chem (2002) 277(36):33361–8. doi: 10.1074/jbc.M205053200
173. Mandal CC, Das F, Ganapathy S, Harris SE, Choudhury GG, Ghosh-Choudhury N. Bone Morphogenetic Protein-2 (BMP-2) Activates NFATc1 Transcription Factor via an Autoregulatory Loop Involving Smad/Akt/Ca2+ Signaling. J Biol Chem (2016) 291(3):1148–61. doi: 10.1074/jbc.M115.668939
174. Lee HL, Bae OY, Baek KH, Kwon A, Hwang HR, Qadir AS, et al. High Extracellular Calcium-Induced NFATc3 Regulates the Expression of Receptor Activator of NF-κb Ligand in Osteoblasts. Bone (2011) 49(2):242–9. doi: 10.1016/j.bone.2011.04.006
175. Takayanagi H. The Role of NFAT in Osteoclast Formation. Ann NY Acad Sci (2007) 1116:227–37. doi: 10.1196/annals.1402.071
176. Takayanagi H, Kim S, Koga T, Nishina H, Isshiki M, Yoshida H, et al. Induction and Activation of the Transcription Factor NFATc1 (NFAT2) Integrate RANKL Signaling in Terminal Differentiation of Osteoclasts. Dev Cell (2002) 3(6):889–901. doi: 10.1016/s1534-5807(02)00369-6
177. Park JH, Lee NK, Lee SY. Current Understanding of RANK Signaling in Osteoclast Differentiation and Maturation. Mol Cells (2017) 40(10):706–13. doi: 10.14348/molcells.2017.0225
178. Okamoto K, Takayanagi H. Regulation of Bone by the Adaptive Immune System in Arthritis. Arthritis Res Ther (2011) 13(3):219. doi: 10.1186/ar3323
179. Mathieu C, Adorini L. The Coming of Age of 1,25-Dihydroxyvitamin D(3) Analogs as Immunomodulatory Agents. Trends Mol Med (2002) 8(4):174–9. doi: 10.1016/s1471-4914(02)02294-3
180. Gentek R, Molawi K, Sieweke MH. Tissue Macrophage Identity and Self-Renewal. Immunol Rev (2014) 262(1):56–73. doi: 10.1111/imr.12224
181. Davies LC, Taylor PR. Tissue-Resident Macrophages: Then and Now. Immunology (2015) 144(4):541–8. doi: 10.1111/imm.12451
182. Sinder BP, Pettit AR, McCauley LK. Macrophages: Their Emerging Roles in Bone. J Bone Mineral Res Off J Am Soc Bone Mineral Res (2015) 30(12):2140–9. doi: 10.1002/jbmr.2735
183. Chen K, Jiao Y, Liu L, Huang M, He C, He W, et al. Communications Between Bone Marrow Macrophages and Bone Cells in Bone Remodeling. Front Cell Dev Biol (2020) 8:598263. doi: 10.3389/fcell.2020.598263
184. Cho SW, Soki FN, Koh AJ, Eber MR, Entezami P, Park SI, et al. Osteal Macrophages Support Physiologic Skeletal Remodeling and Anabolic Actions of Parathyroid Hormone in Bone. Proc Natl Acad Sci USA (2014) 111(4):1545–50. doi: 10.1073/pnas.1315153111
185. Lo CH, Lynch CC. Multifaceted Roles for Macrophages in Prostate Cancer Skeletal Metastasis. Front Endocrinol (2018) 9:247. doi: 10.3389/fendo.2018.00247
186. Batoon L, Millard SM, Wullschleger ME, Preda C, Wu AC, Kaur S, et al. CD169(+) Macrophages Are Critical for Osteoblast Maintenance and Promote Intramembranous and Endochondral Ossification During Bone Repair. Biomaterials (2019) 196:51–66. doi: 10.1016/j.biomaterials.2017.10.033
187. Pettit AR, Chang MK, Hume DA, Raggatt LJ. Osteal Macrophages: A New Twist on Coupling During Bone Dynamics. Bone (2008) 43(6):976–82. doi: 10.1016/j.bone.2008.08.128
188. Batoon L, Millard SM, Raggatt LJ, Wu AC, Kaur S, Sun LWH, et al. Osteal Macrophages Support Osteoclast-Mediated Resorption and Contribute to Bone Pathology in a Postmenopausal Osteoporosis Mouse Model. J Bone Mineral Res Off J Am Soc Bone Mineral Res (2021) 36(11):2214–28. doi: 10.1002/jbmr.4413
189. Stacey KJ, Sweet MJ, Hume DA. Macrophages Ingest and Are Activated by Bacterial DNA. J Immunol (1996) 157(5):2116–22.
190. Itoh K, Udagawa N, Kobayashi K, Suda K, Li X, Takami M, et al. Lipopolysaccharide Promotes the Survival of Osteoclasts via Toll-Like Receptor 4, But Cytokine Production of Osteoclasts in Response to Lipopolysaccharide Is Different From That of Macrophages. J Immunol (2003) 170(7):3688–95. doi: 10.4049/jimmunol.170.7.3688
191. Jimi E, Nakamura I, Duong LT, Ikebe T, Takahashi N, Rodan GA, et al. Interleukin 1 Induces Multinucleation and Bone-Resorbing Activity of Osteoclasts in the Absence of Osteoblasts/Stromal Cells. Exp Cell Res (1999) 247(1):84–93. doi: 10.1006/excr.1998.4320
192. Takayanagi H, Kim S, Matsuo K, Suzuki H, Suzuki T, Sato K, et al. RANKL Maintains Bone Homeostasis Through C-Fos-Dependent Induction of Interferon-Beta. Nature (2002) 416(6882):744–9. doi: 10.1038/416744a
193. van Holten J, Smeets TJ, Blankert P, Tak PP. Expression of Interferon Beta in Synovial Tissue From Patients With Rheumatoid Arthritis: Comparison With Patients With Osteoarthritis and Reactive Arthritis. Ann Rheum Dis (2005) 64(12):1780–2. doi: 10.1136/ard.2005.040477
194. Cassetta L, Pollard JW. Tumor-Associated Macrophages. Curr Biol CB (2020) 30(6):R246–8. doi: 10.1016/j.cub.2020.01.031
195. Zhou J, Tang Z, Gao S, Li C, Feng Y, Zhou X. Tumor-Associated Macrophages: Recent Insights and Therapies. Front Oncol (2020) 10:188. doi: 10.3389/fonc.2020.00188
196. Mizutani K, Sud S, McGregor NA, Martinovski G, Rice BT, Craig MJ, et al. The Chemokine CCL2 Increases Prostate Tumor Growth and Bone Metastasis Through Macrophage and Osteoclast Recruitment. Neoplasia (New York NY) (2009) 11(11):1235–42. doi: 10.1593/neo.09988
197. Moriceau G, Ory B, Gobin B, Verrecchia F, Gouin F, Blanchard F, et al. Therapeutic Approach of Primary Bone Tumours by Bisphosphonates. Curr Pharm Design (2010) 16(27):2981–7. doi: 10.2174/138161210793563554
198. Roelofs AJ, Thompson K, Ebetino FH, Rogers MJ, Coxon FP. Bisphosphonates: Molecular Mechanisms of Action and Effects on Bone Cells, Monocytes and Macrophages. Curr Pharm Design (2010) 16(27):2950–60. doi: 10.2174/138161210793563635
199. Yang TT, Sabokbar A, Gibbons CL, Athanasou NA. Human Mesenchymal Tumour-Associated Macrophages Differentiate Into Osteoclastic Bone-Resorbing Cells. J Bone Joint Surg Br Vol (2002) 84(3):452–6. doi: 10.1302/0301-620x.84b3.11945
200. Pan Y, Yu Y, Wang X, Zhang T. Tumor-Associated Macrophages in Tumor Immunity. Front Immunol (2020) 11:583084. doi: 10.3389/fimmu.2020.583084
201. Perry CJ, Muñoz-Rojas AR, Meeth KM, Kellman LN, Amezquita RA, Thakral D, et al. Myeloid-Targeted Immunotherapies Act in Synergy to Induce Inflammation and Antitumor Immunity. J Exp Med (2018) 215(3):877–93. doi: 10.1084/jem.20171435
202. Endo-Munoz L, Evdokiou A, Saunders NA. The Role of Osteoclasts and Tumour-Associated Macrophages in Osteosarcoma Metastasis. Biochim Biophys Acta (2012) 1826(2):434–42. doi: 10.1016/j.bbcan.2012.07.003
203. Pang Y, Fu Y, Li C, Wu Z, Cao W, Hu X, et al. Metal-Organic Framework Nanoparticles for Ameliorating Breast Cancer-Associated Osteolysis. Nano Lett (2020) 20(2):829–40. doi: 10.1021/acs.nanolett.9b02916
204. Ghadially F. Overview Article: The Articular Territory of the Reticuloendothelial System. Ultrastructural Pathol (1980) 1(2):249–64. doi: 10.3109/01913128009141422
206. Danks L, Sabokbar A, Gundle R, Athanasou NA. Synovial Macrophage-Osteoclast Differentiation in Inflammatory Arthritis. Ann Rheum Dis (2002) 61(10):916–21. doi: 10.1136/ard.61.10.916
207. Bouhlel MA, Derudas B, Rigamonti E, Dièvart R, Brozek J, Haulon S, et al. PPARgamma Activation Primes Human Monocytes Into Alternative M2 Macrophages With Anti-Inflammatory Properties. Cell Metab (2007) 6(2):137–43. doi: 10.1016/j.cmet.2007.06.010
208. Chinetti-Gbaguidi G, Daoudi M, Rosa M, Vinod M, Louvet L, Copin C, et al. Human Alternative Macrophages Populate Calcified Areas of Atherosclerotic Lesions and Display Impaired RANKL-Induced Osteoclastic Bone Resorption Activity. Circ Res (2017) 121(1):19–30. doi: 10.1161/circresaha.116.310262
209. Zavaczki E, Gáll T, Zarjou A, Hendrik Z, Potor L, Tóth CZ, et al. Ferryl Hemoglobin Inhibits Osteoclastic Differentiation of Macrophages in Hemorrhaged Atherosclerotic Plaques. Oxid Med Cell Longevity (2020) 2020:3721383. doi: 10.1155/2020/3721383
210. Lee JW, Lee IH, Iimura T, Kong SW. Two Macrophages, Osteoclasts and Microglia: From Development to Pleiotropy. Bone Res (2021) 9(1):11. doi: 10.1038/s41413-020-00134-w
211. Ueno M, Maeno T, Nishimura S, Ogata F, Masubuchi H, Hara K, et al. Alendronate Inhalation Ameliorates Elastase-Induced Pulmonary Emphysema in Mice by Induction of Apoptosis of Alveolar Macrophages. Nat Commun (2015) 6:6332. doi: 10.1038/ncomms7332
212. Tsuji T, Nakamura S, Komuro I, Mikami M, Baba M, Tanaka M. A Living Case of Pulmonary Ossification Associated With Osteoclast Formation From Alveolar Macrophage in the Presence of T-Cell Cytokines. Internal Med (Tokyo Japan) (2003) 42(9):834–8. doi: 10.2169/internalmedicine.42.834
213. Barascuk N, Skjøt-Arkil H, Register TC, Larsen L, Byrjalsen I, Christiansen C, et al. Human Macrophage Foam Cells Degrade Atherosclerotic Plaques Through Cathepsin K Mediated Processes. BMC Cardiovasc Disord (2010) 10:19. doi: 10.1186/1471-2261-10-19
214. Bühling F, Reisenauer A, Gerber A, Krüger S, Weber E, Brömme D, et al. Cathepsin K–a Marker of Macrophage Differentiation? J Pathol (2001) 195(3):375–82. doi: 10.1002/path.959
215. Burger EH, van der Meer JW, van de Gevel JS, Gribnau JC, Thesingh GW, van Furth R. In Vitro Formation of Osteoclasts From Long-Term Cultures of Bone Marrow Mononuclear Phagocytes. J Exp Med (1982) 156(6):1604–14. doi: 10.1084/jem.156.6.1604
Keywords: macrophages, osteoclasts, associations, origin, polarization, cytokines, subgroups
Citation: Sun Y, Li J, Xie X, Gu F, Sui Z, Zhang K and Yu T (2021) Macrophage-Osteoclast Associations: Origin, Polarization, and Subgroups. Front. Immunol. 12:778078. doi: 10.3389/fimmu.2021.778078
Received: 16 September 2021; Accepted: 15 November 2021;
Published: 01 December 2021.
Edited by:
Elizabeth Bradley, University of Minnesota Twin Cities, United StatesReviewed by:
Kim Mansky, University of Minnesota Twin Cities, United StatesAline Bozec, University of Erlangen Nuremberg, Germany
Copyright © 2021 Sun, Li, Xie, Gu, Sui, Zhang and Yu. This is an open-access article distributed under the terms of the Creative Commons Attribution License (CC BY). The use, distribution or reproduction in other forums is permitted, provided the original author(s) and the copyright owner(s) are credited and that the original publication in this journal is cited, in accordance with accepted academic practice. No use, distribution or reproduction is permitted which does not comply with these terms.
*Correspondence: Tiecheng Yu, eXV0Y0BqbHUuZWR1LmNu