- Center for Neuroimmunology & Neuroinfectious Diseases, Departments of Medicine, Neuroscience, and Pathology & Immunology, Washington University School of Medicine, St. Louis, MO, United States
Many mosquito-borne viruses (arboviruses) are endemic in Africa, contributing to systemic and neurological infections in various geographical locations on the continent. While most arboviral infections do not lead to neuroinvasive diseases of the central nervous system, neurologic diseases caused by arboviruses include flaccid paralysis, meningitis, encephalitis, myelitis, encephalomyelitis, neuritis, and post-infectious autoimmune or memory disorders. Here we review endemic members of the Flaviviridae and Togaviridae families that cause neurologic infections, their neuropathogenesis and host neuroimmunological responses in Africa. We also discuss the potential for neuroimmune responses to aide in the development of new diagnostics and therapeutics, and current knowledge gaps to be addressed by arbovirus research.
Introduction
Recent studies indicate that climate changes in Africa may lead to a shift in vector-borne diseases from malaria to arboviruses due to differential effects of warming temperatures on the mosquito species that transmit these pathogens to humans [summarized in (1)]. Thus, neurotropic arboviruses that are transmitted by Aedes aegypti, and cycle between wildlife and livestock or humans in west sub-Saharan Africa, are likely to emerge in other areas of Africa where the current climates supports Anopheles gambiae transmission of malaria (2, 3). Recent epidemics of yellow fever (YFV) and Rift Valley fever (RVFV) viruses in Nigeria and Uganda (4, 5), respectively, and emergence of West Nile virus (WNV) in the Darfur region (6) are consistent with these predictions. In addition, the United Nations estimates suggest an increase in global population of 37% by 2050 (7), which facilitates transmission of vector-borne diseases through higher population densities and international travel. While the majority of infections with neurotropic arboviruses are asymptomatic, many persons develop flu-like symptoms that progress to neuroinvasive diseases in approximately half of symptomatic patients. In addition, 50-70% of survivors of CNS arboviral infection go on to develop neurocognitive and neuropsychiatric disorders that worsen over time (8). In this subsection, we will review the epidemiology, pathophysiology, and value of neuroimmune changes in diagnostics and therapeutics of medically important, African mosquito-borne neurotropic arboviruses. We will also provide current knowledge gaps and perspectives regarding future research in neurotropic arboviruses.
Overview of African Mosquito-Borne Arboviruses That Induce Neuroinvasive Diseases in Humans
The etiologic agents of arboviral neuroinvasive diseases occur within three virologic genera: Flaviviridae, Togaviridae, and Bunyaviridae. The Phlebovirus RVFV (Phenuiviridae family) has been recently and extensively reviewed (9–14). Categorization of these RNA viruses, their key attributes, types of neuroinvasive diseases they cause, in addition to geographic epidemiology, and pathophysiology for medically relevant Flaviviridae and Togaviridae family members are summarized below (see Table 1).
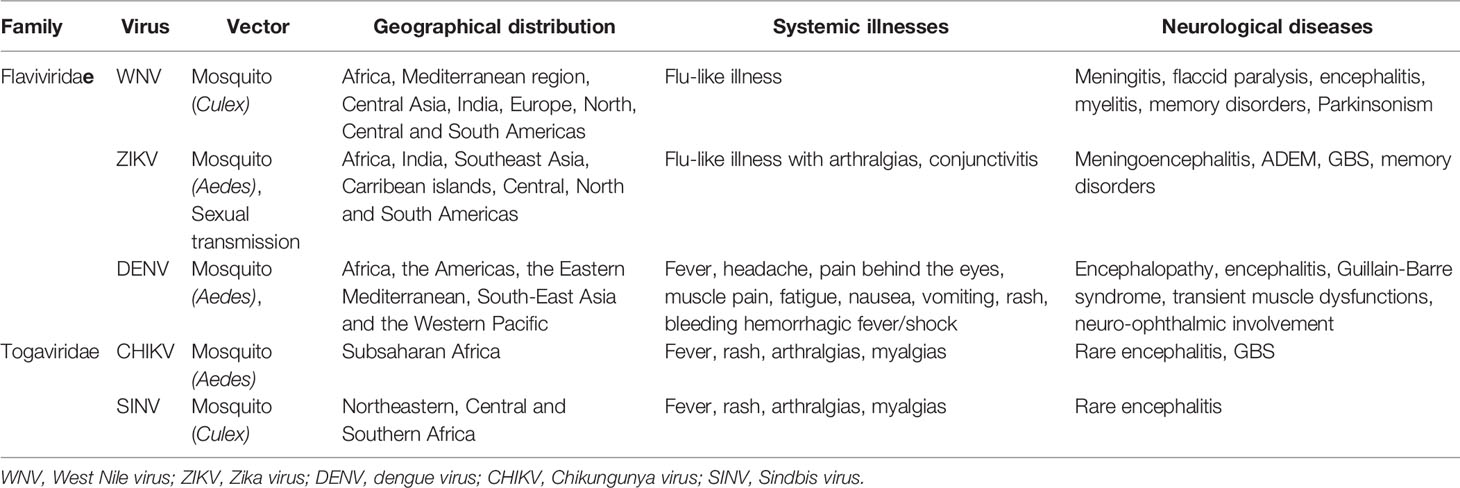
Table 1 African arboviruses: vectors, geographical distribution, and the illnesses they cause in adults.
Flaviviridae
Members of the Flaviviridae family of viruses are enveloped, with a positive single-strand RNA genome of 9-13 Kb with that replicates as a single open reading frame (ORF) with genes for three structural and seven nonstructural (NS) proteins (15). Structural proteins, which comprise the virion, consist of the viral capsid and the envelope glycoproteins. NS proteins are essential for replication of the viral genome, transcription and translation of viral genes, viral assembly, and may modulate immune function to promote infection and dissemination within humans. Phylogenetic trees indicate that all vector-borne flavirviruses originated in Africa, likely from non-vectored mammalian viruses (16). Medically important, neurotropic flaviviruses that cause CNS disease in Africa are transmitted by Culex (West Nile encephalitis viruses; WNV), and Aedes (Zika virus; ZIKV, Dengue virus; DENV) mosquito species (17). WNV was first isolated from a febrile patient in the West Nile district of Uganda in 1937, while ZIKV was first identified in a rhesus monkey from the African regions in Kampala, Uganda, in the Zika forest in 1947 (18, 19). A DENV epidemic was first reported in 1823 in the Zanzibar Islands (20). WNV human cases occur in most African countries throughout the continent with the exception of the western Sahara desert, Mauritania, Mali, Burkina Faso, Niger, northern Chad, Libya, and Angola (17) (Figure 1). ZIKV outbreaks in humans have occurred in only nine countries: Senegal, Cote D’Ivoire, Burkina Faso, Nigeria, Cameroon, Gabon, Central African Republic, Ethiopia, and Angola (Figure 1). DENV, which exists as four closely related but distinct serotypes, is endemic in almost all African countries, with the exception of Morocco, Algeria, Tunesia, Western Sahara, Niger, Chad, Sudan, Gambia, Guinea-Bissa, Guinea, Sierra Leone, Liberya, Ivory Coast, Central African Republic, South Sudan, Congo, Burundi, Botswana, Zimbabwe, Swaziland, and Lesotho (Figure 1). Both WNV and ZIKV may also be transmitted via transfusion of human blood products, and ZIKV can also be transmitted via sexual contact, primarily with males.
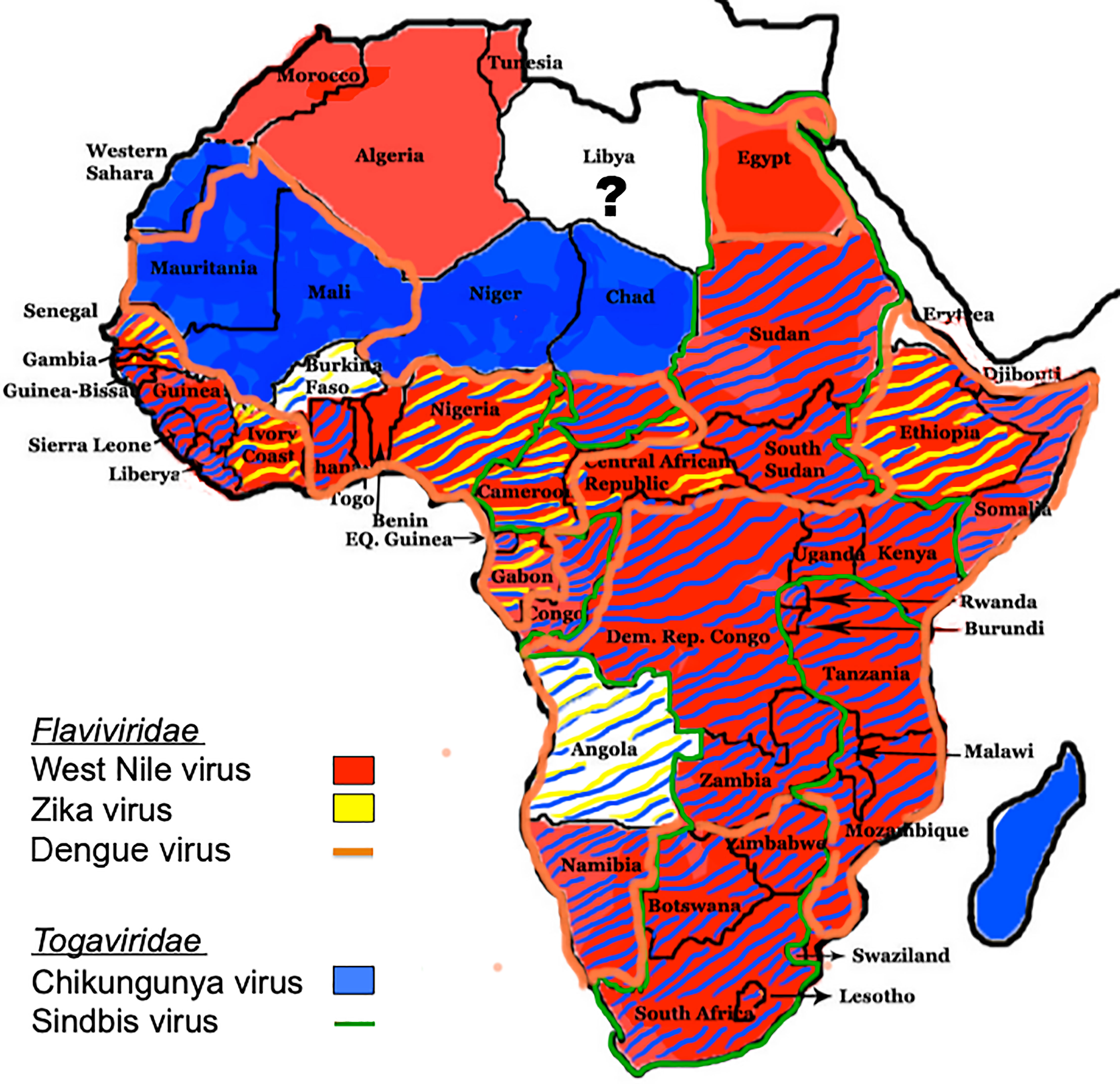
Figure 1 Distribution of flaviviruses and alphaviruses in Africa. The distribution of Culex- and Aedes-transmitted flaviviruses WNV, ZIKV, and DENV, and Aedes- and Culex-transmitted alphaviruses CHIKV and SINV, respectively, throughout Africa are shown (17).
WNV and ZIKV are neurotropic viruses that can cause acute flu-like illnesses with fever, headache, rash, pharyngitis, diarrhea, arthralgias, conjunctivitis, and myalgias (21). Most humans infected with WNV or ZIKV are asymptomatic, however 20-25% of cases become symptomatic, and in those infected with WNV, approximately half of these patients will develop neuroinvasive diseases including meningitis, encephalitis, myelitis, and flaccid paralysis. Vertical transmission leading to teratogenic effects of ZIKV during pregnancy is also well documented with approximately 20% of affected fetuses exhibiting morphological abnormalities by ultrasound (e.g., microcephaly or brain calcifications), whereas the vast majority exhibit no overt clinical manifestations at birth (22–24) Diagnostic tests include assessment of serum or CSF virus-specific IgM or PCR detection of viral RNA (21). Reported neuroinvasive diseases in the setting of ZIKV infection include cases of meningitis, encephalitis, and encephalomyelitis. Patients with a concurrent or past history of ZIKV systemic infection may also present with Guillain-Barré syndrome (GBS) and myeloradiculitis, which may respond to intravenous IVIG (25, 26). Neurologic and functional disability associated with these flaviviruses can also continue to cause morbidity in patients after recovery from acute illness. Studies of WNV survivors report that in 50-70% of survivors exhibit symptoms that persist and worsen over time including confusion, muscle weakness, concentration difficulties, parkinsonism, and memory impairments, especially in the realm of visuospatial memory (27). Severe cases of ZIKV-induced systemic disease may also lead to neurocognitive deficits, daily headaches, and chronic inflammatory demyelinating polyneuropathies that may persist for years (28–32).
Neurological diseases associated with DENV infection were first reported in 1976 as atypical symptoms of dengue infection, and their incidence rates have varied from 0.5% to 20% (33). Neurological symptoms associated with DENV infection have increasingly been reported in both children and adults, and include encephalopathy due to hepatic failure or metabolic disorders, encephalitis due to direct viral invasion, Guillain-Barre syndrome or transient muscle dysfunctions, and neuro-ophthalmic involvement (34).
Dengue serotypes 2 and 3 are most commonly associated with neurological symptoms (35, 36). Although DENV is not primarily neurotropic, a recent study utilizing genome analysis and characterization of DENV type 2 (DENV-2) strains isolated from cerebrospinal fluid (CSF) and/or serum of patients with dengue encephalitis revealed that the DENV-2 isolates belonged to a new clade of cosmopolitan genotype that are genetically close to strains identified in China, South Korea, Singapore, Malaysia, Thailand, and the Philippines (37). As DENV does not invade the CNS when inoculated peripherally in mice, few studies have determined its route of neuroinvasion or CNS immune responses that exert virologic control.
The pathogenesis of WNV and ZIKV CNS infections in humans is incompletely defined, although excellent mouse models have illuminated mechanisms of immune control in the periphery and central nervous system (CNS) (38), routes of viral neuroinvasion (39–45), features of virus-induced encephalitis (46, 47), and processes that induce post-infectious neurocognitive sequelae (48–52). Neuroinvasion can occur hematogenously as free virions or within CNS infiltrating immune cells, and via retrograde transport along sensory axons from sites of mosquito inoculation in the periphery (53) (Figure 2). The brain vasculature exhibits specializations that prevent paracellular and transcellular entry of cells, pathogens, and metabolites. These occur at the post-venular and capillary levels and include tight and adherens junctions (TJ and AJ), low levels of leukocyte adhesion molecules, and low rates of transcellular vesicle trafficking (transcytosis). Rho GTPase signaling pathways that control the assembly and disassembly of endothelial cytoskeletal proteins regulate TJ integrity, which affects BBB permeability. During acute infection with flaviviruses, local expression of BBB destabilizing cytokines activate the RhoA/ROCK/pMLC signaling pathway, which induces stress fiber formation that disrupts TJ and increases paracellular permeability. Increased blood-brain barrier (BBB) permeability during acute infection has also been linked to rising levels of NS1 within the blood, which correlate with severity of disease. NS1 is secreted from virally infected cells and may up-regulate the expression of cathepsin L and endoglycosidase heparanase in brain endothelial cells, leading to the degradation of glycocalyx-like layer (EGL) components with consequent damage to BBB integrity (54, 55). Flavivirus traversal across the BBB is believed to occur via paracellular and transcellular routes, the latter of which includes delivery by leukocytes (56). Neuroimaging during the acute setting may be normal or reveal BBB disruption, which is associated with more severe outcome (57).
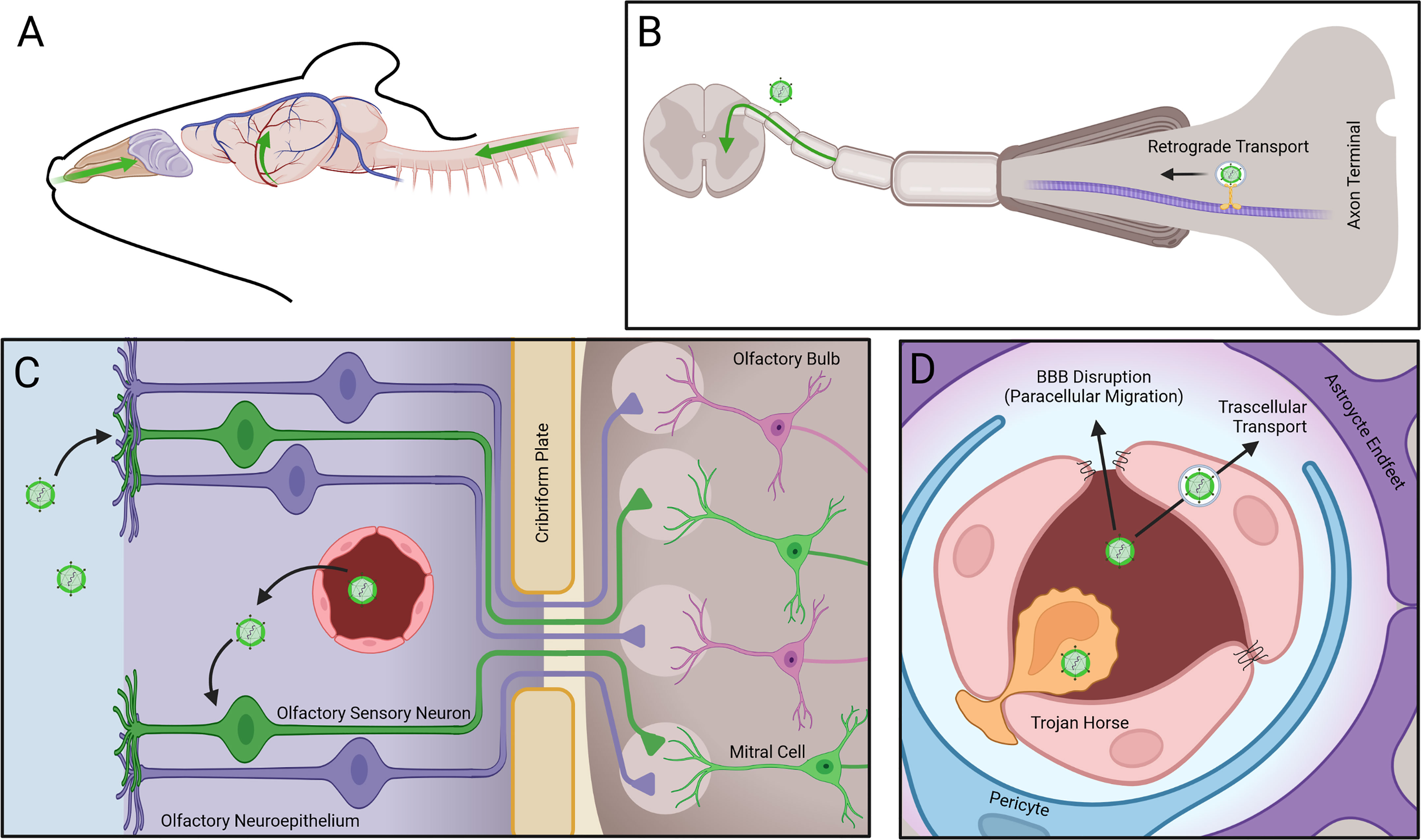
Figure 2 Mechanisms of arbovirus entry into the CNS. Arboviruses enter the CNS via three routes: (A, B) Retrograde transport of virus along axon microtubules (MT) of peripheral neurons allows entry into the spinal cord. (C) Infection of olfactory sensory neurons (OSNs) in the olfactory neuroepithelium (ONE) following infection from fenestrated capillaries (FC) allows viral intra-axonal migration through the cribiform plate (CP), followed by transynaptic infection of mitral cells (MC) at the glomeruli (G) of the olfactory bulb (OB). (D) Virus entry through the blood brain barrier (BBB) occurs via transcellular transport of virions, paracellular migration of virions following disruption of tight junctions (TJs), or via transmigration of infected leukocytes.
Once WNV or ZIKV enter the CNS, they infect and injure neurons (or neuroprogenitor cells in the case of ZIKV) through direct (virus infection-induced) and indirect (immune-mediated) mechanisms (58, 59). Microscopic examination of the post-mortem CNS specimens may reveal neuronal cell death, microglial activation, infiltrating macrophages, and accumulation of CD4+ and CD8+ T cells (60, 61). Depending on the flavivirus, these lesions can occur in the brainstem, cerebral cortex, hippocampus, thalamus, cerebellum or spinal cord. While it is well established that both humoral and cell-mediated immune responses critically control viral replication in peripheral tissues, virologic control within the CNS predominantly requires the infiltration of antiviral mononuclear cells (62–64). Viral replication within neurons is detected by the cytoplasmic RNA helicases RIG-I and MDA5, which signal through the adaptor protein mitochondrial antiviral signaling protein (MAVS) to promote antiviral gene expression and proinflammatory proteins, including T cell chemoattractants in both neurons and activated astrocytes and microglia (38). Antiviral, CD8 T cells recruited to the acutely infected CNS can eliminate virus from neurons via non-cytolytic effects of interferon(IFN)γ (65). Subpopulations of effector CD8 T cells persist as resident memory T cells (Trm) that continue to express IFNγ, which maintains microglia activation (49). During acute infection, infected neurons and activated microglia exhibit upregulation of complement proteins (52), which have been implicated in the maintenance or disruption of neural networks (66). Studies in WNV- and ZIKV-infected mice show complement- and microglia-mediated elimination of synapses within the trisynaptic circuit of the hippocampus (52) is associated with defects in spatial and other forms of learning and memory. Studies in humans that succumbed to WNV show similar loss of synapses. Macrophage delivery of interleukin(IL)-1 has been shown to maintain a proinflammatory state via direct effects on neural stem cells within the neurogenic niche of the hippocampus, promoting decreased neurogenesis in favor of production of neurotoxic, reactive astrocytes that prevent synapse repair, and persist long-term (67). Future studies are needed to determine whether these processes occur and may be targeted in humans to prevent or treat neurocognitive sequelae after recovery from neurotropic flavivirus infection.
Togaviridae
Members of the Togaviridae family of viruses are small, enveloped viruses with single-stranded positive-sense RNA genomes of 10–12 kb that encode five structural and four NS proteins (68). Two thirds of the genome of alphaviruses encodes the non-structural polyprotein(s) in a single ORF immediately after a 5′-non-coding region. Overlapping with the 3′-end of the non-structural ORF, there is a promoter for transcription of a subgenomic mRNA from which the structural polyprotein is translated (69). The genus Alphavirus comprises a large group of medically important mosquito-borne viruses that are transmitted by Aedes (Chikungunya virus; CHIKV), and Culex (Sindbis virus; SINV) (17). Phylogenetic tree analyses suggest that alphaviruses likely originated from an aquatic habitat, from ancestral strains such as the Southern elephant seal virus and other fish viruses, followed by spread to New and Old World (70). The first reported CHIKV and SINV outbreaks occurred in Tanzania and Egypt, respectively, in 1952 (71). Seroprevalence for CHIKV is found throughout sub-Saharan Africa (Figure 1), while SINV occurs in a geographical area that spans from South Africa to Egypt and from Cameroon to Kenya (16) (Figure 1).
CHIKV and SINV generally cause febrile syndromes with rashes and joint pain, and are only occasionally associated with neurologic diseases. CHIV infection is asymptomatic in up to 25% of human infections, with symptomatic cases presenting with fever, headache, myalgia, arthritis, conjunctivitis, nausea/vomiting, maculopapular rash and incapacitating bilateral and symmetric polyarthralgia, which may relapse or persist for months to years (72). Rare neurologic complications include seizures, acute flaccid paralysis, Guillain-Barré syndrome, cranial nerve palsies, myelitis, encephalopathy, and meningoencephalitis (73). Persons at risk for CNS disease include neonates exposed intrapartum, older adults (e.g., > 65 years), and persons undergoing immunosuppression for solid organ transplant (74). Case fatality rate for CHIKV encephalitis ranges from 4-28%, with higher rates mostly in older adults. Electroencephalogram in patients with neurologic signs may exhibit slow background activity and generalized epileptiform discharges, while brain MRI may show bilateral white matter hyperintensities and/or focal encephalitis. Postmortem brain examination of a patient who succumbed to CHIKV encephalitis revealed lymphocytic infiltrates with focal necrosis in the hippocampus, frontal lobes and medulla oblongata (75). While many SINV infections are asymptomatic, cases usually present with a maculopapular, pruritic exanthema over the trunk and limbs, mild fever, and arthralgia, particularly in wrists, hips, knees, and ankles, sometimes accompanied by nausea, general malaise, headache, and myalgia (76). Patients can experience persistent joint manifestations that continue for months or years, and in rare cases as a chronic arthritis. SINV is known to cause neurologic disease in horses (77), but human cases are extremely rare.
The mechanisms of CHIKV and SINV neuroinvasion in humans are unknown; however, animal models suggest entry may occur via invasion of brain endothelial cells and retrograde axonal transport, respectively (78) (Figure 2). Studies examining CHIKV and SINV infection of the CNS in murine models report multiple sites of neuronal and astrocyte infection progressing to cell death via caspase-mediated pathways, with microgliosis and perivascular cuffs (75, 79–82). Similar to reports in human cases of CHIKV encephalitis (75), neuronal degeneration in the hippocampus and lymphocytic meningitis is also observed in animals. As with flavivirus encephalitis, CHIKV RNA is detected in the brain by pattern recognition receptors, such as toll-like receptor(TLR)-3, that upregulate innate immune antiviral molecules that can reduce viral replication (83). While increased expression of the T cell cytokine IFNγ has been observed in animal models, mechanisms of T cell trafficking and virologic control within the brain have not been investigated. Likewise, there have been no reports of long-term follow-up in survivors of CHIKV neurologic diseases.
Can Neuroimmune Responses Aide in Diagnostics and/or Therapeutics?
The diagnosis of arboviral neuroinvasive diseases requires virus-specific assays so that novel therapies, such as antibody-based therapeutics, and patient prognoses can be accurately administered. Studies attempting to identify virus-specific innate or adaptive immune pathways via genomic approaches in animal models have been instrumental in identifying the critical antiviral pathways that control and clear virus (84, 85), but have failed to support the use of pathway analysis for diagnostic purposes. Knowledge regarding the status of BBB permeability may also be critical for treating acute neuroinvasive diseases. For example, animal studies examining patterns of BBB function throughout the course of flavivirus encephalitis indicate that induction of interferon responses may promote BBB closure via Rac1-mediated effects on TJ integrity (40, 45). Thus, use of anti-viral antibodies for CNS infection may have a limited window of penetration. While there are currently no treatments that limit the replication of specific arboviruses in the CNS, anti-inflammatory treatments, including corticosteroids, have been used in patients with chorioretintis, encephalitis or myelitis (86–88). New anti-inflammatory compounds are also under development (89).
Knowledge Gaps for Future Research
One of the challenges for limiting arboviral neuroinvasion and dissemination within the CNS is the incomplete knowledge regarding virus-specific entry receptors expressed at the BBB and by neural cells, including those involved in trans-synaptic spread between CNS regions. Entry receptors postulated to be involved in flavivirus entry include αvβ3 integrins, C-type lectin receptors (CLR), phosphatidylserine receptors TIM (T-cell immunoglobulin and mucin domain) and TYRO3, AXL and MER (TAM) family of receptor tyrosine kinases (90, 91). Attachment and entry receptors for CHIKV include glycosaminoglycans (GAGs), T-cell immunoglobulin and mucin 1 (TIM-1), and the cell adhesion molecule Mxra8 (92). While many of these receptors are expressed at CNS barriers and within the parenchyma, the demonstration these receptors are required for brain endothelial and neural cell entry is currently lacking. There is also a dire need to identify biomarkers that identify survivors of arboviral neuroinvasive diseases at risk for neurological sequelae, including neurocognitive impairments. Post-infectious neurocognitive sequelae modeled in murine models show benefit from administration of anakinra, a USFDA approved medication that targets the IL-1R for the treatment of rheumatoid arthritis, during acute encephalitis (93). Given the essential role of the IL-1R, in CNS virologic control, it is unclear whether the risk-benefit ratio supports use of this drug in humans with arboviral encephalitis. Future studies are needed to better identify and define safe therapeutic targets to limit the entry and dissemination of neurotropic arboviruses, and to prevent the development of neuroimmune processes that contribute long-term sequelae.
Author Contributions
The author confirms being the sole contributor of this work and has approved it for publication.
Funding
This work was supported by NIH grants R35NS122310.
Conflict of Interest
The author declares that the research was conducted in the absence of any commercial or financial relationships that could be construed as a potential conflict of interest.
Publisher’s Note
All claims expressed in this article are solely those of the authors and do not necessarily represent those of their affiliated organizations, or those of the publisher, the editors and the reviewers. Any product that may be evaluated in this article, or claim that may be made by its manufacturer, is not guaranteed or endorsed by the publisher.
Acknowledgments
The author wishes to thank Dr. Matthew Cain for Biorender illustration (Figure 2).
References
1. Mordecai EA, Ryan SJ, Caldwell JM, Shah MM, LaBeaud AD. Climate Change Could Shift Disease Burden From Malaria to Arboviruses in Africa. Lancet Planet Health (2020) 4:e416–23. doi: 10.1016/S2542-5196(20)30178-9
2. Buchwald AG, Hayden MH, Dadzie SK, Paull SH, Carlton EJ. Aedes-Borne Disease Outbreaks in West Africa: A Call for Enhanced Surveillance. Acta Trop (2020) 209:105468. doi: 10.1016/j.actatropica.2020.105468
3. Weetman D, Kamgang B, Badolo A, Moyes CL, Shearer FM, Coulibaly M, et al. Aedes Mosquitoes and Aedes-Borne Arboviruses in Africa: Current and Future Threats. Int J Environ Res Public Health (2018) 15:220–40. doi: 10.3390/ijerph15020220
4. Shoemaker TR, Nyakarahuka L, Balinandi S, Ojwang J, Tumusiime A, Mulei S, et al. First Laboratory-Confirmed Outbreak of Human and Animal Rift Valley Fever Virus in Uganda in 48 Years. Am J Trop Med Hyg (2019) 100:659–71. doi: 10.4269/ajtmh.18-0732
5. Nwachukwu WE, Yusuff H, Nwangwu U, Okon A, Ogunniyi A, Imuetinyan-Clement J, et al. The Response to Re-Emergence of Yellow Fever in Nigeria, 2017. Int J Infect Dis (2020) 92:189–96. doi: 10.1016/j.ijid.2019.12.034
6. Ahmed A, Elduma A, Magboul B, Higazi T, Ali Y. The First Outbreak of Dengue Fever in Greater Darfur, Western Sudan. Trop Med Infect Dis (2019) 4:43–52. doi: 10.3390/tropicalmed4010043
7. Raftery AE, Alkema L, Gerland P. Bayesian Population Projections for the United Nations. Stat Sci (2014) 29:58–68. doi: 10.1214/13-STS419
8. Salimi H, Cain MD, Klein RS. Encephalitic Arboviruses: Emergence, Clinical Presentation, and Neuropathogenesis. Neurotherapeutics (2016) 13:514–34. doi: 10.1007/s13311-016-0443-5
9. Agboli E, Zahouli JBZ, Badolo A, Jost H. Mosquito-Associated Viruses and Their Related Mosquitoes in West Africa. Viruses (2021) 13:891–919. doi: 10.3390/v13050891
10. Calkins CM, Scasta JD. Transboundary Animal Diseases (TADs) Affecting Domestic and Wild African Ungulates: African Swine Fever, Foot and Mouth Disease, Rift Valley Fever (1996-2018). Res Vet Sci (2020) 131:69–77. doi: 10.1016/j.rvsc.2020.04.001
11. Grossi-Soyster EN, LaBeaud AD. Rift Valley Fever: Important Considerations for Risk Mitigation and Future Outbreaks. Trop Med Infect Dis (2020) 5:89–102. doi: 10.3390/tropicalmed5020089
12. Ikegami T. Candidate Vaccines for Human Rift Valley Fever. Expert Opin Biol Ther (2019) 19:1333–42. doi: 10.1080/14712598.2019.1662784
13. Javelle E, Lesueur A, Pommier de Santi V, de Laval F, Lefebvre T, Holweck G, et al. The Challenging Management of Rift Valley Fever in Humans: Literature Review of the Clinical Disease and Algorithm Proposal. Ann Clin Microbiol Antimicrob (2020) 19:4. doi: 10.1186/s12941-020-0346-5
14. Kading RC, Abworo EO, Hamer GL. Rift Valley Fever Virus, Japanese Encephalitis Virus, and African Swine Fever Virus: Three Transboundary, Vector-Borne, Veterinary Biothreats With Diverse Surveillance, and Response Capacity Needs. Front Vet Sci (2019) 6:458. doi: 10.3389/fvets.2019.00458
15. Ramos-Lorente S, Romero-Lopez C, Berzal-Herranz A. Information Encoded by the Flavivirus Genomes Beyond the Nucleotide Sequence. Int J Mol Sci (2021) 22:3738–56. doi: 10.3390/ijms22073738
16. Braack L, Gouveia de Almeida AP, Cornel AJ, Swanepoel R, de Jager C. Mosquito-Borne Arboviruses of African Origin: Review of Key Viruses and Vectors. Parasit Vectors (2018) 11:29. doi: 10.1186/s13071-017-2559-9
17. Pierson TC, Diamond MS. The Continued Threat of Emerging Flaviviruses. Nat Microbiol (2020) 5:796–812. doi: 10.1038/s41564-020-0714-0
18. Chancey C, Grinev A, Volkova E, Rios M. The Global Ecology and Epidemiology of West Nile Virus. BioMed Res Int (2015) 2015:376230. doi: 10.1155/2015/376230
19. Sikka V, Chattu VK, Popli RK, Galwankar SC, Kelkar D, Sawicki SG, et al. The Emergence of Zika Virus as a Global Health Security Threat: A Review and a Consensus Statement of the INDUSEM Joint Working Group (JWG). J Glob Infect Dis (2016) 8:3–15. doi: 10.4103/0974-777X.176140
20. Amarasinghe A, Kuritsk JN, Letson GW, Margolis HS. Dengue Virus Infection in Africa. Emerg Infect Dis (2011) 17:1349–54. doi: 10.3201/eid1708.101515
21. Starolis MW, Perez O, Powell EA. Clinical Features and Laboratory Diagnosis of Emerging Arthropod-Transmitted Viruses: A Report From the Pan American Society for Clinical Virology Clinical Practice Committee. J Clin Virol (2020) 132:104651. doi: 10.1016/j.jcv.2020.104651
22. Brasil P, Pereira JP Jr, Moreira ME, Ribeiro Nogueira RM, Damasceno L, Wakimoto M, et al. Zika Virus Infection in Pregnant Women in Rio De Janeiro. N Engl J Med (2016) 375:2321–34. doi: 10.1056/NEJMoa1602412
23. Charlier C, Beaudoin MC, Couderc T, Lortholary O, Lecuit M. Arboviruses and Pregnancy: Maternal, Fetal, and Neonatal Effects. Lancet Child Adolesc Health (2017) 1:134–46. doi: 10.1016/S2352-4642(17)30021-4
24. Ades AE, Soriano-Arandes A, Alarcon A, Bonfante F, Thorne C, Peckham CS, et al. Vertical Transmission of Zika Virus and Its Outcomes: A Bayesian Synthesis of Prospective Studies. Lancet Infect Dis (2021) 21:537–45. doi: 10.1016/S1473-3099(20)30432-1
25. Siu R, Bukhari W, Todd A, Gunn W, Huang QS, Timmings P. Acute Zika Infection With Concurrent Onset of Guillain-Barre Syndrome. Neurology (2016) 87:1623–4. doi: 10.1212/WNL.0000000000003038
26. Uncini A, Gonzalez-Bravo DC, Acosta-Ampudia YY, Ojeda EC, Rodriguez Y, Monsalve DM, et al. Clinical and Nerve Conduction Features in Guillain-Barre Syndrome Associated With Zika Virus Infection in Cucuta, Colombia. Eur J Neurol (2018) 25:644–50. doi: 10.1111/ene.13552
27. Murray KO, Nolan MS, Ronca SE, Datta S, Govindarajan K, Narayana PA, et al. The Neurocognitive and MRI Outcomes of West Nile Virus Infection: Preliminary Analysis Using an External Control Group. Front Neurol (2018) 9:111. doi: 10.3389/fneur.2018.00111
28. da Silva IRF, Frontera JA, Bispo de Filippis AM, Nascimento O, Group R-G-ZR. Neurologic Complications Associated With the Zika Virus in Brazilian Adults. JAMA Neurol (2017) 74:1190–8. doi: 10.1001/jamaneurol.2017.1703
29. de Almeida Oliveira Evangelista G, Carvalho RH, Menezes GS, Abreu YC, Sardi SI, Campos GS. Meningoencephalitis Associated With Zika Virus and Chikungunya Virus Infection. Jpn J Infect Dis (2021) 74(6):584–6. doi: 10.7883/yoken.JJID.2020.1000
30. Lannuzel A, Ferge JL, Lobjois Q, Signate A, Roze B, Tressieres B, et al. Long-Term Outcome in Neurozika: When Biological Diagnosis Matters. Neurology (2019) 92:e2406–20. doi: 10.1212/WNL.0000000000007536
31. Souza INO, Barros-Aragao FGQ, Frost PS, Figueiredo CP, Clarke JR. Late Neurological Consequences of Zika Virus Infection: Risk Factors and Pharmaceutical Approaches. Pharmaceuticals (Basel) (2019) 12:60–81. doi: 10.3390/ph12020060
32. Zucker J, Neu N, Chiriboga CA, Hinton VJ, Leonardo M, Sheikh A, et al. Zika Virus-Associated Cognitive Impairment in Adolescent, 2016. Emerg Infect Dis (2017) 23:1047–8. doi: 10.3201/eid2306.162029
33. Saini L, Chakrabarty B, Pastel H, Israni A, Kumar A, Gulati S. Dengue Fever Triggering Hemiconvulsion Hemiplegia Epilepsy in a Child. Neurol India (2017) 65:636–8. doi: 10.4103/neuroindia.NI_1367_15
34. Carod-Artal FJ, Wichmann O, Farrar J, Gascon J. Neurological Complications of Dengue Virus Infection. Lancet Neurol (2013) 12:906–19. doi: 10.1016/S1474-4422(13)70150-9
35. Lum LC, Lam SK, Choy YS, George R, Harun F. Dengue Encephalitis: A True Entity? Am J Trop Med Hyg (1996) 54:256–9. doi: 10.4269/ajtmh.1996.54.256
36. Soares CN, Cabral-Castro MJ, Peralta JM, Freitas MR, Puccioni-Sohler M. Meningitis Determined by Oligosymptomatic Dengue Virus Type 3 Infection: Report of a Case. Int J Infect Dis (2010) 14:e150–2. doi: 10.1016/j.ijid.2009.03.016
37. Ngwe Tun MM, Muthugala R, Nabeshima T, Soe AM, Dumre SP, Rajamanthri L, et al. Complete Genome Analysis and Characterization of Neurotropic Dengue Virus 2 Cosmopolitan Genotype Isolated From the Cerebrospinal Fluid of Encephalitis Patients. PloS One (2020) 15:e0234508. doi: 10.1371/journal.pone.0234508
38. Suthar MS, Diamond MS, Gale M Jr. West Nile Virus Infection and Immunity. Nat Rev Microbiol (2013) 11:115–28. doi: 10.1038/nrmicro2950
39. Dai J, Wang P, Bai F, Town T, Fikrig E. Icam-1 Participates in the Entry of West Nile Virus Into the Central Nervous System. J Virol (2008) 82:4164–8. doi: 10.1128/JVI.02621-07
40. Lazear HM, Daniels BP, Pinto AK, Huang AC, Vick SC, Doyle SE, et al. Interferon-Lambda Restricts West Nile Virus Neuroinvasion by Tightening the Blood-Brain Barrier. Sci Transl Med (2015) 7:284ra59.
41. Maximova OA, Bernbaum JG, Pletnev AG. West Nile Virus Spreads Transsynaptically Within the Pathways of Motor Control: Anatomical and Ultrastructural Mapping of Neuronal Virus Infection in the Primate Central Nervous System. PloS Negl Trop Dis (2016) 10:e0004980. doi: 10.1371/journal.pntd.0004980
42. Paul AM, Acharya D, Duty L, Thompson EA, Le L, Stokic DS, et al. Osteopontin Facilitates West Nile Virus Neuroinvasion via Neutrophil “Trojan Horse” Transport. Sci Rep (2017) 7:4722. doi: 10.1038/s41598-017-04839-7
43. Roe K, Kumar M, Lum S, Orillo B, Nerurkar VR, Verma S. West Nile Virus-Induced Disruption of the Blood-Brain Barrier in Mice Is Characterized by the Degradation of the Junctional Complex Proteins and Increase in Multiple Matrix Metalloproteinases. J Gen Virol (2012) 93:1193–203. doi: 10.1099/vir.0.040899-0
44. Zhou W, Woodson M, Neupane B, Bai F, Sherman MB, Choi KH, et al. Exosomes Serve as Novel Modes of Tick-Borne Flavivirus Transmission From Arthropod to Human Cells and Facilitates Dissemination of Viral RNA and Proteins to the Vertebrate Neuronal Cells. PloS Pathog (2018) 14:e1006764. doi: 10.1371/journal.ppat.1006764
45. Daniels BP, Holman DW, Cruz-Orengo L, Jujjavarapu H, Durrant DM, Klein RS. Viral Pathogen-Associated Molecular Patterns Regulate Blood-Brain Barrier Integrity via Competing Innate Cytokine Signals. mBio (2014) 5:e01476–14. doi: 10.1128/mBio.01476-14
46. Davis LE, DeBiasi R, Goade DE, Haaland KY, Harrington JA, Harnar JB, et al. West Nile Virus Neuroinvasive Disease. Ann Neurol (2006) 60:286–300. doi: 10.1002/ana.20959
47. Debiasi RL, Tyler KL. West Nile Virus Meningoencephalitis. Nat Clin Pract Neurol (2006) 2:264–75. doi: 10.1038/ncpneuro0176
48. Figueiredo CP, Barros-Aragao FGQ, Neris RLS, Frost PS, Soares C, Souza INO, et al. Zika Virus Replicates in Adult Human Brain Tissue and Impairs Synapses and Memory in Mice. Nat Commun (2019) 10:3890. doi: 10.1038/s41467-019-11866-7
49. Garber C, Soung A, Vollmer LL, Kanmogne M, Last A, Brown J, et al. T Cells Promote Microglia-Mediated Synaptic Elimination and Cognitive Dysfunction During Recovery From Neuropathogenic Flaviviruses. Nat Neurosci (2019) 22:1276–88. doi: 10.1038/s41593-019-0427-y
50. Li H, Saucedo-Cuevas L, Shresta S, Gleeson JG. The Neurobiology of Zika Virus. Neuron (2016) 92:949–58. doi: 10.1016/j.neuron.2016.11.031
51. Tisoncik-Go J, Gale M Jr. Microglia in Memory Decline From Zika Virus and West Nile Virus Infection. Trends Neurosci (2019) 42:757–9. doi: 10.1016/j.tins.2019.08.009
52. Vasek MJ, Garber C, Dorsey D, Durrant DM, Bollman B, Soung A, et al. A Complement-Microglial Axis Drives Synapse Loss During Virus-Induced Memory Impairment. Nature (2016) 534:538–43. doi: 10.1038/nature18283
53. Cain MD, Salimi H, Diamond MS, Klein RS. Mechanisms of Pathogen Invasion Into the Central Nervous System. Neuron (2019) 103:771–83. doi: 10.1016/j.neuron.2019.07.015
54. Puerta-Guardo H, Glasner DR, Espinosa DA, Biering SB, Patana M, Ratnasiri K, et al. Flavivirus NS1 Triggers Tissue-Specific Vascular Endothelial Dysfunction Reflecting Disease Tropism. Cell Rep (2019) 26:1598–1613 e8. doi: 10.1016/j.celrep.2019.01.036
55. Rastogi M, Singh SK. Zika Virus NS1 Affects the Junctional Integrity of Human Brain Microvascular Endothelial Cells. Biochimie (2020) 176:52–61. doi: 10.1016/j.biochi.2020.06.011
56. Mustafa YM, Meuren LM, Coelho SVA, de Arruda LB. Pathways Exploited by Flaviviruses to Counteract the Blood-Brain Barrier and Invade the Central Nervous System. Front Microbiol (2019) 10:525. doi: 10.3389/fmicb.2019.00525
57. Ali M, Safriel Y, Sohi J, Llave A, Weathers S. West Nile Virus Infection: MR Imaging Findings in the Nervous System. AJNR Am J Neuroradiol (2005) 26:289–97.
58. Samuel MA, Morrey JD, Diamond MS. Caspase 3-Dependent Cell Death of Neurons Contributes to the Pathogenesis of West Nile Virus Encephalitis. J Virol (2007) 81:2614–23. doi: 10.1128/JVI.02311-06
59. Shrestha B, Gottlieb D, Diamond MS. Infection and Injury of Neurons by West Nile Encephalitis Virus. J Virol (2003) 77:13203–13. doi: 10.1128/JVI.77.24.13203-13213.2003
60. Bouffard JP, Riudavets MA, Holman R, Rushing EJ. Neuropathology of the Brain and Spinal Cord in Human West Nile Virus Infection. Clin Neuropathol (2004) 23:59–61.
61. Schwartzmann PV, Ramalho LN, Neder L, Vilar FC, Ayub-Ferreira SM, Romeiro MF, et al. Zika Virus Meningoencephalitis in an Immunocompromised Patient. Mayo Clin Proc (2017) 92:460–6. doi: 10.1016/j.mayocp.2016.12.019
62. Aguilar-Valenzuela R, Netland J, Seo YJ, Bevan MJ, Grakoui A, Suthar MS. Dynamics of Tissue-Specific CD8(+) T Cell Responses During West Nile Virus Infection. J Virol (2018) 92:e00014–18. doi: 10.1128/JVI.00014-18
63. Durrant DM, Daniels BP, Klein RS. IL-1R1 Signaling Regulates CXCL12-Mediated T Cell Localization and Fate Within the Central Nervous System During West Nile Virus Encephalitis. J Immunol (2014) 193:4095–106. doi: 10.4049/jimmunol.1401192
64. Brien JD, Daffis S, Lazear HM, Cho H, Suthar MS, Gale M Jr, et al. Interferon Regulatory Factor-1 (IRF-1) Shapes Both Innate and CD8(+) T Cell Immune Responses Against West Nile Virus Infection. PloS Pathog (2011) 7:e1002230. doi: 10.1371/journal.ppat.1002230
65. Stubblefield Park SR, Widness M, Levine AD, Patterson CE. T Cell-, Interleukin-12-, and Gamma Interferon-Driven Viral Clearance in Measles Virus-Infected Brain Tissue. J Virol (2011) 85:3664–76. doi: 10.1128/JVI.01496-10
66. Kanmogne M, Klein RS. Neuroprotective Versus Neuroinflammatory Roles of Complement: From Development to Disease. Trends Neurosci (2021) 44:97–109. doi: 10.1016/j.tins.2020.10.003
67. Garber C, Vasek MJ, Vollmer LL, Sun T, Jiang X, Klein RS. Astrocytes Decrease Adult Neurogenesis During Virus-Induced Memory Dysfunction via Interleukin-1. Nat Immunol (2018) 19:151–61. doi: 10.1038/s41590-017-0021-y
68. Pietila MK, Hellstrom K, Ahola T. Alphavirus Polymerase and RNA Replication. Virus Res (2017) 234:44–57. doi: 10.1016/j.virusres.2017.01.007
69. Kumar R, Mehta D, Mishra N, Nayak D, Sunil S. Role of Host-Mediated Post-Translational Modifications (PTMs) in RNA Virus Pathogenesis. Int J Mol Sci (2020) 22:323–50. doi: 10.3390/ijms22010323
70. Powers AM, Brault AC, Shirako Y, Strauss EG, Kang W, Strauss JH, et al. Evolutionary Relationships and Systematics of the Alphaviruses. J Virol (2001) 75:10118–31. doi: 10.1128/JVI.75.21.10118-10131.2001
71. Robinson MC. An Epidemic of Virus Disease in Southern Province, Tanganyika Territory, in 1952-53. I. Clinical Features. Trans R Soc Trop Med Hyg (1955) 49:28–32. doi: 10.1016/0035-9203(55)90080-8
72. Russo G, Subissi L, Rezza G. Chikungunya Fever in Africa: A Systematic Review. Pathog Glob Health (2020) 114:136–44. doi: 10.1080/20477724.2020.1748965
73. Ortiz-Quezada J, Rodriguez EE, Hesse H, Molina L, Duran C, Lorenzana I, et al. Chikungunya Encephalitis, a Case Series From an Endemic Country. J Neurol Sci (2021) 420:117279. doi: 10.1016/j.jns.2020.117279
74. Mrzljak A, Novak R, Pandak N, Tabain I, Franusic L, Barbic L, et al. Emerging and Neglected Zoonoses in Transplant Population. World J Transplant (2020) 10:47–63. doi: 10.5500/wjt.v10.i3.47
75. Das T, Jaffar-Bandjee MC, Hoarau JJ, Krejbich Trotot P, Denizot M, Lee-Pat-Yuen G, et al. Chikungunya Fever: CNS Infection and Pathologies of a Re-Emerging Arbovirus. Prog Neurobiol (2010) 91:121–9. doi: 10.1016/j.pneurobio.2009.12.006
76. Adouchief S, Smura T, Sane J, Vapalahti O, Kurkela S. Sindbis Virus as a Human Pathogen-Epidemiology, Clinical Picture and Pathogenesis. Rev Med Virol (2016) 26:221–41. doi: 10.1002/rmv.1876
77. Hubalek Z, Rudolf I, Nowotny N. Arboviruses Pathogenic for Domestic and Wild Animals. Adv Virus Res (2014) 89:201–75. doi: 10.1016/B978-0-12-800172-1.00005-7
78. Passoni G, Langevin C, Palha N, Mounce BC, Briolat V, Affaticati P, et al. Imaging of Viral Neuroinvasion in the Zebrafish Reveals That Sindbis and Chikungunya Viruses Favour Different Entry Routes. Dis Model Mech (2017) 10:847–57. doi: 10.1242/dmm.029231
79. Griffin DE. Neuronal Cell Death in Alphavirus Encephalomyelitis. Curr Top Microbiol Immunol (2005) 289:57–77. doi: 10.1007/3-540-27320-4_3
80. Melamed S, Avraham R, Rothbard DE, Erez N, Israely T, Klausner Z, et al. Innate Immune Response in Neuronopathic Forms of Gaucher Disease Confers Resistance Against Viral-Induced Encephalitis. Acta Neuropathol Commun (2020) 8:144. doi: 10.1186/s40478-020-01020-6
81. Das T, Hoarau JJ, Bandjee MCJ, Maquart M, Gasque P. Multifaceted Innate Immune Responses Engaged by Astrocytes, Microglia and Resident Dendritic Cells Against Chikungunya Neuroinfection. J Gen Virol (2015) 96:294–310. doi: 10.1099/vir.0.071175-0
82. Taylor A, Sheng KC, Herrero LJ, Chen W, Rulli NE, Mahalingam S. Methotrexate Treatment Causes Early Onset of Disease in a Mouse Model of Ross River Virus-Induced Inflammatory Disease Through Increased Monocyte Production. PloS One (2013) 8:e71146. doi: 10.1371/journal.pone.0071146
83. Priya R, Patro IK, Parida MM. TLR3 Mediated Innate Immune Response in Mice Brain Following Infection With Chikungunya Virus. Virus Res (2014) 189:194–205. doi: 10.1016/j.virusres.2014.05.010
84. Grifoni A, Tian Y, Sette A, Weiskopf D. Transcriptomic Immune Profiles of Human Flavivirus-Specific T-Cell Responses. Immunology (2020) 160:3–9. doi: 10.1111/imm.13161
85. Kumar M, Roe K, O’Connell M, Nerurkar VR. Induction of Virus-Specific Effector Immune Cell Response Limits Virus Replication and Severe Disease in Mice Infected With non-Lethal West Nile Virus Eg101 Strain. J Neuroinflamm (2015) 12:178. doi: 10.1186/s12974-015-0400-y
86. Kramer AH. Viral Encephalitis in the ICU. Crit Care Clin (2013) 29:621–49. doi: 10.1016/j.ccc.2013.03.011
87. Pyrgos V, Younus F. High-Dose Steroids in the Management of Acute Flaccid Paralysis Due to West Nile Virus Infection. Scand J Infect Dis (2004) 36:509–12. doi: 10.1080/00365540410020659
88. Zito R, Micelli Ferrari T, Di Pilato L, Lorusso M, Ferretta A, Micelli Ferrari L, et al. Clinical Course of Choroidal Neovascular Membrane in West Nile Virus Chorioretinitis: A Case Report. J Med Case Rep (2021) 15:206. doi: 10.1186/s13256-021-02700-0
89. Chuang FK, Huang SM, Liao CL, Lee AR, Lien SP, Chiu YL, et al. Anti-Inflammatory Compound Shows Therapeutic Safety and Efficacy Against Flavivirus Infection. Antimicrob Agents Chemother (2019) 64:e00941–19. doi: 10.1128/AAC.00941-19
90. Laureti M, Narayanan D, Rodriguez-Andres J, Fazakerley JK, Kedzierski L. Flavivirus Receptors: Diversity, Identity, and Cell Entry. Front Immunol (2018) 9:2180. doi: 10.3389/fimmu.2018.02180
91. Oliveira LG, Peron JPS. Viral Receptors for Flaviviruses: Not Only Gatekeepers. J Leukoc Biol (2019) 106:695–701. doi: 10.1002/JLB.MR1118-460R
92. Schnierle BS. Cellular Attachment and Entry Factors for Chikungunya Virus. Viruses (2019) 11:1078–87. doi: 10.3390/v11111078
Keywords: alphavirus biology, neuroimmunology, Flavivirus, Africa, CNS
Citation: Klein RS (2021) Encephalitic Arboviruses of Africa: Emergence, Clinical Presentation and Neuropathogenesis. Front. Immunol. 12:769942. doi: 10.3389/fimmu.2021.769942
Received: 02 September 2021; Accepted: 17 November 2021;
Published: 23 December 2021.
Edited by:
Willias Masocha, Kuwait University, KuwaitReviewed by:
Hikoaki Fukaura, Saitama Medical University, JapanMaria Reyes-Mantilla, Johns Hopkins Medicine, United States
Copyright © 2021 Klein. This is an open-access article distributed under the terms of the Creative Commons Attribution License (CC BY). The use, distribution or reproduction in other forums is permitted, provided the original author(s) and the copyright owner(s) are credited and that the original publication in this journal is cited, in accordance with accepted academic practice. No use, distribution or reproduction is permitted which does not comply with these terms.
*Correspondence: Robyn S. Klein, cmtsZWluQHd1c3RsLmVkdQ==