- 1Clinical and Experimental Sciences, Faculty of Medicine, University of Southampton, Southampton, United Kingdom
- 2National Institute for Health Research Southampton Biomedical Research Centre, Southampton Centre for Biomedical Research, Southampton General Hospital, Southampton, United Kingdom
- 3Birmingham Medical School, University of Birmingham, Birmingham, United Kingdom
Chronic obstructive pulmonary disease (COPD) is one of the leading causes of death worldwide. Individuals with COPD typically experience a progressive, debilitating decline in lung function as well as systemic manifestations of the disease. Multimorbidity, is common in COPD patients and increases the risk of hospitalisation and mortality. Central to the genesis of multimorbidity in COPD patients is a self-perpetuating, abnormal immune and inflammatory response driven by factors including ageing, pollutant inhalation (including smoking) and infection. As many patients with COPD have multiple concurrent chronic conditions, which require an integrative management approach, there is a need to greater understand the shared disease mechanisms contributing to multimorbidity. The intercellular transfer of extracellular vesicles (EVs) has recently been proposed as an important method of local and distal cell-to-cell communication mediating both homeostatic and pathological conditions. EVs have been identified in many biological fluids and provide a stable capsule for the transfer of cargo including proteins, lipids and nucleic acids. Of these cargo, microRNAs (miRNAs), which are short 17-24 nucleotide non-coding RNA molecules, have been amongst the most extensively studied. There is evidence to support that miRNA are selectively packaged into EVs and can regulate recipient cell gene expression including major pathways involved in inflammation, apoptosis and fibrosis. Furthermore changes in EV cargo including miRNA have been reported in many chronic diseases and in response to risk factors including respiratory infections, noxious stimuli and ageing. In this review, we discuss the potential of EVs and EV-associated miRNA to modulate shared pathological processes in chronic diseases. Further delineating these may lead to the identification of novel biomarkers and therapeutic targets for patients with COPD and multimorbidities.
Introduction
Chronic obstructive pulmonary disease (COPD) is the third leading cause of death worldwide due to its prevalence, severity and the absence of an effective treatment to reverse disease progression (1). Individuals with COPD experience progressive lung function decline with periods of acute exacerbation (AECOPD) that impact quality of life and present a high economic burden due to direct medical costs and loss of working days (2–5). The development of more sensitive diagnostics and personalised treatments for COPD remains challenging due to our limited understanding of the complex molecular mechanisms underlying the disease (6).
The main pathological driver of COPD is inhalation of noxious stimuli such as cigarette smoke (CS) and particulate matter (PM) air pollution. Repeated exposure to respiratory toxins compromises the function of the immune system, induces chronic inflammation and directly damages structural cells, leading to emphysema and vascular remodelling (7, 8). In addition, damage caused by noxious stimuli promotes features of accelerated ageing including a state of cell cycle arrest, known as cellular senescence, that increases the release of inflammatory factors and has been associated with COPD pathogenesis (9). Furthermore exposure to noxious stimuli and age-associated immune alterations contribute to a dysfunctional immune response and increased susceptibility to acute respiratory infections observed in COPD (10–12). Respiratory infections result in elevation of airway and systemic inflammation on top of the chronic inflammation present in stable COPD and are the predominant cause of AECOPD (13).
The occurrence of multiple chronic conditions termed “comorbidities” or “multimorbidities” are common in patients with COPD (14, 15) (Figure 1). Other conditions commonly observed in conjunction with COPD include cardiovascular disease (CVD), diabetes, osteoporosis and gastro-oesophageal reflux disease (15). While the term ‘‘comorbidity’’ refers to the combined effects of additional conditions in reference to an index chronic condition, “multimorbidity” indicates that no single condition holds priority over any of the co-occurring conditions (16). As many patients with COPD have multiple concurrent chronic conditions, which require an integrative management approach, there is a need to greater understand the shared disease mechanisms contributing to multimorbidity.
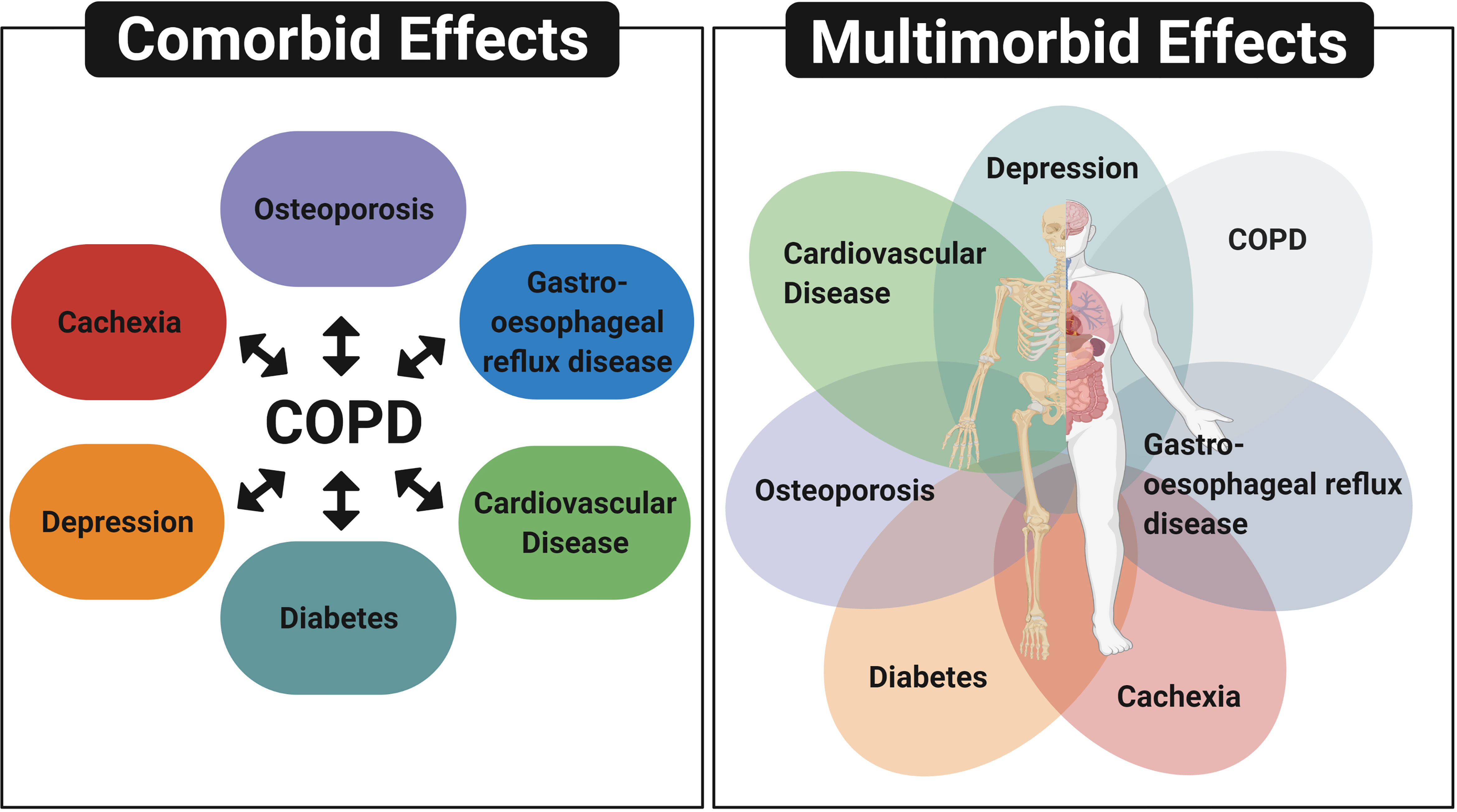
Figure 1 Comorbidity and multimorbidity in patients with COPD. The term ‘‘comorbidity’’ refers to the combined effects of additional conditions in reference to COPD, whereas “multimorbidity” indicates that no single condition holds priority over any of the co-occurring conditions. Diagram depicts other conditions commonly observed in patients with COPD, which include cardiovascular disease, depression, cachexia, diabetes, osteoporosis and gastro-oesophageal reflux disease. Created with BioRender.com.
Our current understanding of the mechanistic drivers for multimorbidity in COPD, including the role of shared risk factors were recently reviewed in detail by Burke and Wilkinson (15). These authors suggested that disease co-occurrence may not be by chance but as a result of shared genetic predisposition and responses to biological and environmental stressors (15). They also highlighted current and novel management strategies that target these underlying mechanisms. In support of this suggestion, network analyses have identified shared genes, proteins and biological pathways common to COPD and its most prevalent coexisting diseases (17). In addition, there is accumulating evidence that exposure to shared risk factors including inhalation of noxious stimuli and accelerated ageing may act as a central mechanism contributing to the development of multiple chronic diseases (18, 19). Furthermore, Burke and Wilkinson introduced the concept that extracellular vesicles (EVs) may contribute to dissemination of inflammation and therefore multimorbidity in COPD patients (15).
Recently EVs have been reported as local and systemic immune and inflammatory mediators that may act to spread or alleviate disease (15, 20–22). During acute and chronic inflammation, vascular permeability is dramatically increased and the alveolar-capillary barrier is reduced allowing lung inflammatory mediators such as proteins and EVs to reach the systemic circulation and potentially distant organs (21, 23). In this review we appraise the latest evidence around the potential role of EVs as circulating inflammatory mediators which could propagate systemic inflammation and multimorbidity in response to shared risk factors including ageing, inhaled noxious stimuli and respiratory infections.
Extracellular Vesicles
EVs are highly heterogeneous lipid bilayer particles that have been isolated from a variety of cell types and biological fluids including serum, plasma, urine and bronchoalveolar lavage fluid (BALF) (24–30). They are generated and released by cells via a range of mechanisms that have been used to categorise EVs into three distinct subgroups; exosomes, microvesicles and apoptotic bodies (Figure 2) (31). However, our understanding of the role of specific EV subgroups remains limited due to the technical challenges associated with isolating pure subgroups, including EV size overlap and the current lack of subgroup specific markers (32). Therefore, this review will consider the overall role of EVs rather than specific subgroups.
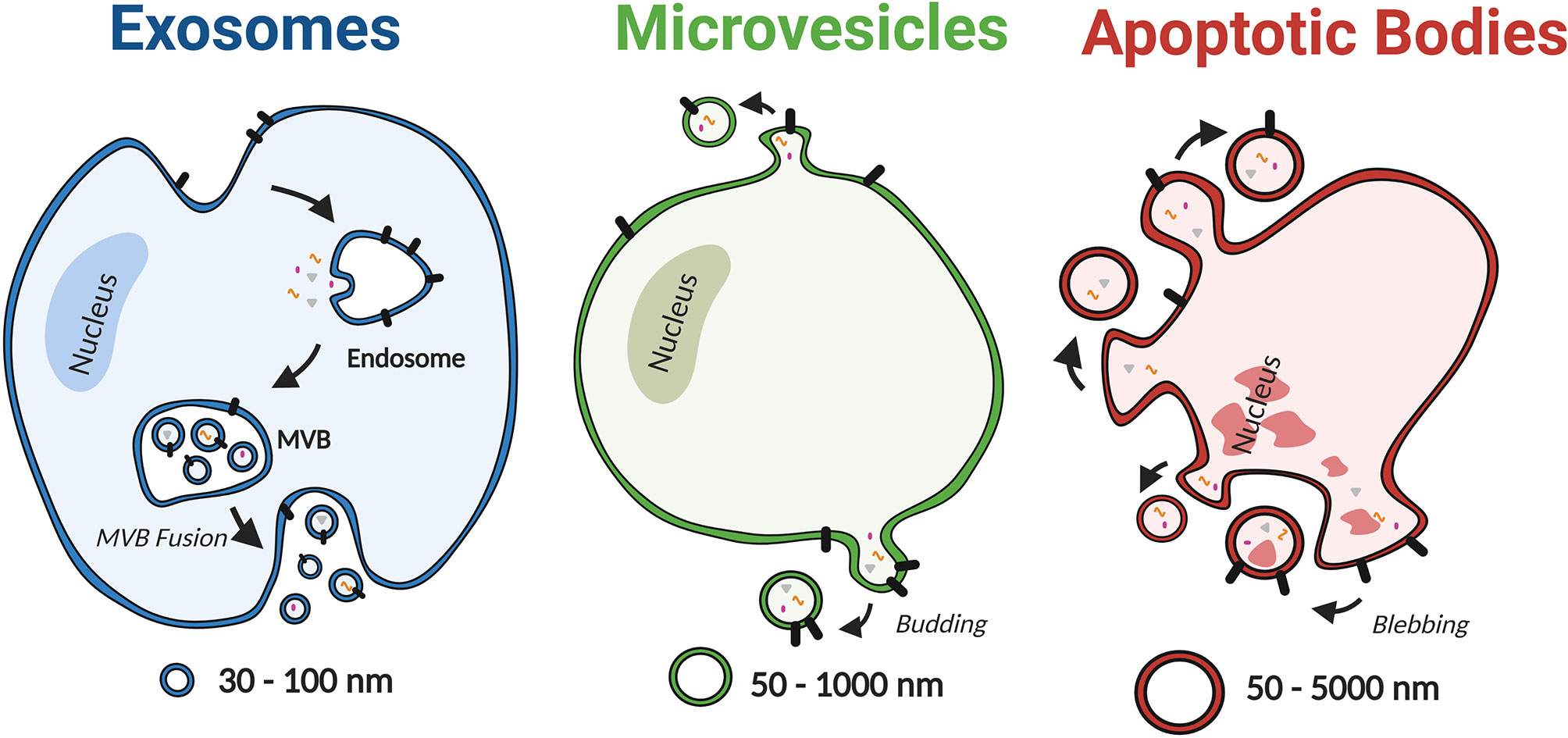
Figure 2 Schematic depiction of EV subtypes, including exosomes, microvesicles, and apoptotic bodies. Based on the mechanism of biogenesis, EVs can be categorised into three distinct subgroups; exosomes, microvesicles and apoptotic bodies. Exosomes are the smallest subclass of EVs with a diameter ranging from 30 nm to 100 nm. They are produced as intraluminal vesicles by inward budding of the endosomal membrane to form multivesicular bodies (MVB), which release vesicles upon fusion with the plasma membrane. In contrast, MVs are 50 nm to 1000 nm in diameter and are formed by direct budding from the plasma membrane. Lastly apoptotic bodies have the broadest range of diameters (50–5000 nm) and are produced by cells undergoing apoptosis. Created with BioRender.com.
EVs were originally considered to be cell debris but have since been shown to transfer lipids, proteins and nucleic acids locally and systemically as a form of intercellular communication mediating both homeostatic and pathological conditions (33, 34). Furthermore distinct EV-associated cargo have been reported depending on the origin and the physiological conditions including disease status (35, 36). EVs are enriched in surface proteins with immunoregulatory functions, such as the major histocompatibility molecules (MHC) class I and II (37). In addition, the transfer of immune and inflammatory mediators such as cytokines, chemokines and proteolytic enzymes via EVs has been shown to be altered in response to stimuli and to modulate recipient cell behaviour (38).
One of the most widely studied EV cargo are microRNAs (miRNAs). MiRNAs are short 17-24 nucleotide, non-coding RNA molecules that regulate gene expression by translational repression or degradation of mRNA (39). The miRNA content of EVs has been reported to be markedly different from that of the parent cell, suggesting that cells are capable of sorting miRNA into EVs (40). In support of this a number of sorting mechanisms have been reported and were recently discussed in detail in a review by Groot et al. (41). These mechanisms broadly include RNA-binding proteins such as hnRNPA2B1, membranous proteins involved in EV biogenesis such as Caveolin-1 and posttranscriptional RNA modifications such as 3’-end uridylation (42, 43). Furthermore there is evidence that the miRNA cargo of EVs can be taken up and alter gene expression and biological processes, such as immune response in the recipient cells (44, 45). The intercellular transfer of EV miRNA has therefore been implicated in mediating a range of pathophysiological processes including in the development of COPD (46).
Given the ability of EVs to transfer cargo it is possible they allow distal communication to contribute to or protect against pathological mechanisms underpinning the development of multiple chronic diseases. The following sections will discuss the current research around the potential role of EVs and EV-associated miRNA as a shared disease mechanism that could contribute to the development of COPD and multimorbidity.
Ageing, Inflammation and the Development of Chronic Diseases
The world's ageing population presents a major challenge for health care services globally, particularly as the prevalence of many chronic diseases increases with age (47, 48). There is accumulating evidence that ageing induces a state of chronic inflammation, that may simultaneously contribute to the development of multiple age-related chronic diseases such as COPD, CVD and osteoporosis (10, 49, 50). This process, known as inflammaging, is characterised by significantly higher levels of circulating pro-inflammatory markers, such as interleukin (IL)-1, IL-6, IL-8, IL-13, IL-18, C-reactive protein (CRP), transforming growth factor-β (TGFβ) and tumour necrosis factor (TNF) (49). Inflammaging is thought to be a consequence of the accumulation of cellular damage due to mitochondrial dysfunction, defective autophagy/mitophagy, an impaired ubiquitin/proteasome system and endoplasmic reticulum stress in combination with exhaustion of endogenous damage-associated molecular pattern (DAMP) clearance mechanism (51–54). Cellular senescence, defined as irreversible cell cycle arrest, has also been suggested to contribute to inflammaging. This process is driven by a variety of mechanisms including the DNA damage response and age-related telomere attrition that activate the senescence-associated secretory phenotype (SASP) wherein cells release high levels of pro-inflammatory factors (55). The number of senescent cells increases with age, generating low-grade inflammation which has widely been implicated in the pathogenesis of age-related diseases (56). Inflammaging is also associated with changes in immune cell function and subset composition with age, known as immunosenescence. The key changes occurring in immunosenescence were recently summarised by Feehan et al. and broadly include reduced phagocytosis, altered immune-modulatory cytokine expression, increased autoimmunity and diminished activation of the adaptive immune response (57). Furthermore there is increasing evidence that COPD and its coexistent conditions represent an acceleration of the ageing process (58, 59).
EVs as Immunomodulatory Factors in Ageing
Age related changes in the concentration of circulating EVs has been a topic of debate as it remains difficult to accurately measure the concentration of EVs due to the complexity of isolating pure EV populations, detecting EVs and enumerating them (60). Using nano-particle tracking analysis (NTA), Eitan et al. reported that the concentration of circulating EVs decreased with age in human plasma (61). These authors suggested this decrease was partially due to enhanced internalization by B cells, as determined by a FACS-based assay using fluorescently labelled EVs (61). It was also suggested that the reduction in EV concentration with age could be a consequence of an impaired clearance mechanism, given EVs may function to dispose of unwanted proteins and other molecules (61). Later studies using NTA have reported no significant difference in the concentration of plasma EVs from non-smokers, smokers and patients with COPD (62, 63). However using NTA to determine EV concentration has major limitations given it cannot differentiate other particles such as lipoproteins that are commonly co-isolated with EVs. Other studies have demonstrated changes in EV concentration based on common EV markers. For example, the concentration of CD9 positive EVs was shown to be significantly elevated in COPD patients and to correlate with physiological markers of multimorbidity including CRP and IL-6 (64, 65).
Inflammation is a major hall-mark of aging and age related diseases. A number of studies have suggested an age-related increase of EV miRNAs with an anti-inflammatory role, which may act as a compensatory mechanism to oppose the hyperinflammatory state that increases during normal aging and is accelerated in progression of aging-related diseases such as COPD. Plasma EVs from aged mice have been shown to be enriched in miR-192 that functions to suppress the inflammatory response in macrophages (66). This was demonstrated by a significant reduction of IL-6 and IL-1β expression in stimulated murine macrophages following treatment with EVs isolated from miR-192 transfected RAW264.7 cells. Furthermore, exogenous intravenous administration of EVs, isolated from miR-192 transfected RAW264.7 cells, were shown to significantly reduce macrophage recruitment and expression of IL-6, IL-12, TNF, interferon (IFN) and CCL2 in lung tissue of mice following inoculation with inactivated influenza whole virus particles (WVP). Increased levels of miR-192 have also been reported in plasma derived EVs from COPD patients suggesting that the EV miR-192 response to hyperinflammatory state is exaggerated in COPD patents (63). Furthermore, EVs containing miR-192 have been shown to delay diabetic retinopathy and inflammatory responses in rheumatoid arthritis, supporting the role of miR-192 in mediating multiple disease pathways (67, 68). Other miRNA including miR-21, miR-146a and miR-223 have been identified to be elevated in EVs isolated from the plasma of old mice (69). These miRNA were shown to contribute to an anti-inflammatory phenotype, as demonstrated by increased expression of Arg1, Il10 and Mrc1 in LPS-stimulated macrophages and reduced endothelial cell response to VEGF (69). Similarly to miR-192, studies have shown miR-21, miR-146a and miR-223 to be increased in EVs isolated from patients with COPD or other chronic diseases, such as osteoporosis, CVD and diabetes (70–77). Therefore, these EV miRNA may provide a common link and potential biomarker for accelerated ageing and age-related diseases. However, although these studies suggest an increase in anti-inflammatory EV miRNA as a mechanism to decrease inflammation, other studies have suggested that removal of miRNA, such as miR-223, from alveolar macrophages and T cells via EVs releases suppression of the NLRP3 inflammasome thereby promoting activation (78, 79). Further studies using clinically relevant samples are required to establish if the increase in anti-inflammatory EV miRNA observed in ageing and age-related chronic diseases is a mechanism to counteract chronic inflammation or is a mechanism to release suppression of cellular immune and inflammatory pathways.
Mesenchymal stem cells (MSC) have been of particular scientific interest because of their potent immunomodulatory and anti-inflammatory properties. However stem cell exhaustion has been implicated in ageing and several chronic diseases including COPD (80). Huang et al. demonstrated that human MSC EVs from a young donor suppressed the activation of IL-6, IL-1β and TNF in the lung following LPS insult when injected into mice (81). On the other hand, this anti-inflammatory response was not observed for MSC EVs isolated from an old donor. Significantly higher levels of anti-inflammatory miR-223 and lower levels of pro-inflammatory miR-127 and miR-125b were observed in MSC-EVs from young vs old mice which may explain this phenomenon (81). In a separate study, MSC-EVs that originated from older rats were demonstrated to have a lower content of miR-133b-3p and miR-294, two miRNAs that inhibit TGF-β1-mediated epithelial-to-mesenchymal transition, which contributes to fibrosis (82). Reduced expression of miR-133b has also been identified in COPD patients, coronary artery disease and osteoporosis with a range of suggested functions including regulating vascular smooth muscle cells and osteoblast differentiation (83–85). These studies suggest EVs from aged MSC may be less capable of protecting against chronic inflammation and tissue damage, which in turn may promote accelerated ageing and the development of chronic diseases such as COPD.
Endothelial cells that line the lumen of blood vessels not only act as a physical barrier but play a pivotal role in regulating blood flow and immune cell recruitment (86). Ageing and exposure to noxious stimuli induces senescence of endothelial cells which contributes to endothelial dysfunction in both COPD and CVD (87, 88). Epigenetic regulation of DNA damage and senescence has been reported as a pathogenic mechanism linked to endothelial dysfunction in COPD patients (89). Mensà et al. demonstrated that EVs isolated from an in vitro model of endothelial replicative senescence enhanced the senescent associated profile in recipient endothelial cells including increased expression of cell cycle inhibitor p16 and SASP factors including IL-6 and IL-8 (90). The mechanism by which EVs from senescent cells can spread premature senescence was suggested to be due to inhibition of the epigenetic regulators DNMT1 and SIRT1 via increased levels of EV miR-21 and miR-217 (90). Furthermore, EVs from the plasma of elderly humans and senescent cultured endothelial cells, have been shown to promote calcification in vascular smooth muscle cells, a risk factor for the development of CVD (91). In addition, EVs released from senescent endothelial cells have been shown to be enriched in miR-31 that inhibits osteogenic differentiation of MSCs, providing a possible link between endothelial dysfunction and osteoporosis (92).
Research over recent years into characterising the age-related changes in EVs reveals diverse functional changes in EVs with age and suggest it is unlikely to be as simple as concluding they either promote or inhibit “inflammaging” and chronic disease. More likely, there are different subtypes of EVs with different functions depending on their origin and the mechanism by which they are released. Further research is required to characterise these distinct EV populations across the human lifespan. Increased susceptibility to infection and the multimorbidity observed in COPD may be partially attributed to the reduced immune function observed with ageing as discussed in the following section.
Respiratory Infections, Inflammation and the Development of Chronic Disease
Respiratory infections are the primary driver of COPD exacerbations, which lead to worsening of symptoms and increased risk of hospitalisation and mortality (93–96). Respiratory viruses, including rhinovirus, respiratory syncytial virus (RSV) and influenza, are commonly associated with COPD exacerbations whilst colonisation of bacteria such as non-typeable Haemophilus influenzae (NTHi), Streptococcus pneumoniae and Moraxella catarrhalis in the airways of COPD patients is common during both stable disease and exacerbations (12, 13, 97–100). In particular, acquisition of new bacterial strains appears to be associated with an increased risk of COPD exacerbations (101, 102). Additionally, acute childhood infections are thought to play a role in initiating pathological mechanisms which could predispose individuals to chronic diseases in later life (103, 104). The potential long-term impact of respiratory infections has been exemplified by the recent SARS-CoV-2 pandemic with severe COVID-19 leading to multiple organ damage and a range of long-term systemic effects (105, 106). In addition, greater morbidity and increased mortality has been observed following infection with SARS-CoV-2 in individuals with existing chronic diseases such as COPD, CVD and diabetes (107–111). However, the long-term impact of COVID-19 disease on the body and resultant pathological mechanisms that could drive susceptibility to lung diseases, such as COPD and other systemic diseases, is still unravelling.
Immune and Inflammatory Role of EVs in Response to Respiratory Infection
Respiratory infections have been reported to trigger increased levels of lung-derived EVs released from alveolar macrophages and epithelial cells (112, 113). EVs released in response to respiratory infections, that are frequently detected in AECOPD, contribute to the production of immune and inflammatory mediators. A recent study reported significant differences in small RNA EV cargo released by the human alveolar epithelial A549 cell line when infected with RSV (114). These EVs were shown to activate the innate immune response as demonstrated by increased production of cytokines and chemokines including CXCL10, CCL5 and TNF in other A549 cells and human monocytes (114). On the other hand, proteomic characterisation of EVs released from human macrophages upon influenza infection revealed EVs may directly transfer pro-inflammatory cytokines (115). EVs have also been shown to upregulate type I IFNs, a key mediator of anti-viral responses. Liu et al. demonstrated that EVs produced by influenza infected A549 cells induced IFN production to inhibit viral replication through upregulation of miR-1975 (116). Similarly, BALF EVs from a murine model of highly pathogenic avian influenza have been reported to have an increased level of miR-483-3p and to potentiate IFN immune response (117). Furthermore, pattern-associated molecular patterns (PAMPs)-containing EVs have also been shown to stimulate a proinflammatory response in macrophages through TLR and enhance T cell activation (118, 119). In addition SARS-CoV-2 viral RNA within EVs derived from A549 epithelial cells has been shown to be internalised by cardiomyocytes and up-regulate inflammatory genes (120). The systemic dissemination of inflammation by macrophage-derived EVs has also been demonstrated using a LPS challenged murine model (121). This effect was proposed to be due to the interaction of histones on the outer surface of vesicles with TLR4 (121).
Endothelial dysfunction driven by respiratory infections has been associated with higher incidence of acute cardiovascular events following COPD exacerbations (122, 123). The concentration of endothelial EVs has been reported to be elevated in patients with COPD who have frequent exacerbations and may contribute to systemic effects by mediating coagulation, vascular tone and angiogenesis (124). Indeed coagulant proteins have been reported to be enriched in EVs in the plasma of COPD and cardiovascular patients (62, 125). EVs released in response to respiratory infections may be a contributing mechanism. For example, SARS-CoV-2 infection induces the release of tissue factor (TF) positive EVs into the circulation that was suggested to contribute to thrombosis and mortality in patients with COVID-19 (126–129).
Although there are a growing number of studies that report that EVs released in response to infection are pro-inflammatory and can induce systemic inflammation, there is currently no direct research into whether host EVs released in response to infection contribute to development and exacerbation of chronic diseases including COPD. However plasma EVs from COPD patients have been shown to contain higher levels of miR-125b that has previously been suggested to directly reduce antiviral signaling and cause exaggerated inflammation and impaired antiviral responses to IAV (63, 130). Therefore, further research is required to determine if dissemination and uptake of EVs contribute to the exacerbated or persistent inflammation observed in chronic disease in response to infection. In addition, given that EVs have been shown to modulate the IFN response, they may have useful anti-viral therapeutic applications. The potential of IFN treatment in the prevention of virally induced exacerbations in COPD is currently being investigated (131, 132).
Gram-negative bacteria associated with COPD, such as NTHi and M. catarrhalis, have also been shown to release EVs known as outer membrane vesicles (OMVs). OMVs are a similar size to host EVs, approximately 20 to 350 nm and can also export a range of cargo including proteins and small RNAs (133). Despite a wide number of studies demonstrating the effects of OMVs in host-microbe interactions, including their ability to enter the systemic circulation and induce a variety of immunological and metabolic responses, the exact mechanisms of bacterial vesicles and their content are still largely unknown (134, 135). Bacterial OMVs can deliver their cargo to a range of host cells including epithelial cells, neutrophils and macrophages and subsequently stimulate an inflammatory response (133, 136, 137). For example, OMVs of M. catarrhalis can bind to TLR2 on epithelial cells and are subsequently internalized, causing a pro-inflammatory response and increased levels of IL-8 (138). Furthermore, OMVs have been implicated in the formation of biofilms that increase tolerance to antimicrobial treatments and the immune system (99, 139). While the presence of NTHi and M. catarrhalis have been associated with a heightened risk of COPD exacerbation, the mechanisms for this remains unclear. Given that NTHi and M. catarrhalis have been shown to release OMVs, that can activate host immune responses as well as potentially support bacterial colonization, they may provide a novel mechanism contributing to the nature of chronic and recurrent bacterial infections in AECOPD and systemic disease. In support of this, a recent review highlighted the role of Porphyromonas gingivalis in promoting the development of related systemic diseases including diabetes and cardiovascular disease through long distance transmission of OMVs (140, 141). Further research is, therefore, required to determine role of OMVs in COPD and other systemic diseases.
Our understanding of the role of EVs in activating the host immune response against respiratory pathogens, or facilitating infection, remains sparse. The release of pro-inflammatory EVs in response to infection may have the ability to induce inflammation, both locally in the lung and distally, contributing to the pathology of COPD and allied chronic diseases. Further studies are required to elucidate the mechanisms by which EV cargoes modulate the immune response of recipient cells over the course of infection and whether this is dysregulated in chronic diseases and could contribute to an impaired immune response. In contrast, other environmental risk factors such as CS have been shown to increase the incidence of multiple chronic diseases.
Respiratory Toxins, Inflammation and the Development of Chronic Disease
Exposure to respiratory toxins, such as CS and air pollution, has been shown to be the primary risk factor for COPD as well as significantly increasing the risk of developing CVD and diabetes complications. Sustained exposure to harmful stimuli results in profound functional and structural changes to the airway epithelium including changes in mucous production, impairment of epithelium regeneration and reduction in cilia development (142). In addition, smoking contributes to immune dysregulation including an increase in pro-inflammatory effects such as increased immune cell recruitment as well as immunosuppressive effects including suppression of immune cell effector functions (143). Furthermore, the damage caused by respiratory toxins has been associated with accelerated ageing and increased susceptibility to infections that contribute to chronic disease pathology, as discussed previously. Studies in smokers with mild-moderate COPD have shown that the relationship between COPD and CVD is mediated through established cardiovascular risk factors such as tobacco smoking rather than through COPD itself (18). As with respiratory infections, a link between CS exposure in early life and the development of chronic disease during adulthood has also been demonstrated (144). However, while smoking cessation slows the rate of decline of pulmonary function in COPD patients it does not halt disease progression and systemic inflammation persists. Therefore, EVs may provide a mechanism contributing to the self-perpetuating spread of systemic inflammation.
Immune Modulating EVs Released in Response to Respiratory Toxins
Oxidative stress induced by the imbalance between oxidants and antioxidants from exposure to CS has been shown to increase the levels of EVs released by the airway epithelium. Benedikter et al. proposed that exposure of the BEAS-2B human bronchial epithelial cell line to cigarette smoke extract (CSE) increases the release of EVs due to oxidative depletion of surface thiols (145). In support of this proposal, antioxidants such as N-acetyl-L-cysteine (NAC) were shown to prevent CSE-associated increases in EVs (145). However, it is possible that the increased levels of EVs detected were due to reduced EV uptake, as another study reported reduced EV uptake following exposure to CSE that was reversed by the presence of NAC (146). While there is overwhelming evidence that oxidative stress and oxidative damage play a pivotal role in the pathogenesis of COPD and other systemic diseases, further research is required to determine if redox-dependent thiol modification is a potential mechanism contributing to the modulation of EVs in multimorbid patients’ (147).
Exposure to respiratory toxins upregulates inflammatory pathways that result in increased immune cell recruitment and release of pro-inflammatory mediators commonly upregulated in COPD and other chronic diseases. EVs released in response to respiratory toxins have been shown to promote cytokine release in epithelial cells. Heliot et al. reported that EVs isolated from the BAL of smokers increased secretion of IL-6 by BEAS-2B cells (148). Similarly, Martin et al. reported that EVs released by THP-1 macrophages which were exposed to PM2.5 promoted the release of IL-6 and TNF from BEAS-2B cells (149). The release of EVs from airway epithelial cells in response to respiratory toxins has been reported to induce recruitment of monocytes and activation of macrophages (30, 150). One study reported that EVs released from BEAS-2B cells under oxidative stress activate macrophages and promote expression of TNF, IL-1β, and IL-6 though increased EV levels of miR-320a and miR-221 (151). Increased levels of miR-221 have been reported to enhance smoking-induced inflammation in COPD (152). Furthermore, increased EV miR-320a has been reported in the plasma of osteoporosis patients and was suggested to impair osteoblast function and induces oxidative stress (77, 153). The manifestation of systemic disease has been suggested to be potentially caused by elevated levels of pro-inflammatory Wnt5a in circulating EVs in response to smoking and in COPD patients (154). Elevated levels of Wnt5a have also been demonstrated in other chronic diseases including heart failure (155). In addition, the production of circulating procoagulant EVs in response to CS has been demonstrated, further supporting the role of EVs as a mechanism contributing to the systemic effects of inhalation of noxious stimuli (156). Overall these studies suggest that EVs released in response to noxious stimuli contain cargo that have been associated with chronic disease and promote inflammation.
In COPD, airway epithelial cells and immune cells secrete an increased level of proteolytic enzymes resulting in chronic inflammation and destruction of lung parenchyma (7, 157, 158). Li et al. reported a 3-fold increase in EV matrix metalloproteinase (MMP)-14 released from macrophages following exposure to CSE (159). In addition, EVs have been found to be associated with neutrophil elastase that was suggested to contribute to the ability of EVs to degrade extracellular matrix and promote alveolar destruction (160). These studies provide a mechanism by which EVs, released in response to noxious stimuli, may contribute towards tissue damage that promotes airway remodelling. EVs have also been reported to play a role in airway remodelling by mediating fibrosis through epithelial-mesenchymal transition (EMT) and myofibroblast differentiation. CSE has been shown to increase miR-210 and miR-21 in human bronchial epithelial cell EVs, leading to suppression of autophagy and an increase in myofibroblast differentiation (161, 162). On the other hand, He et al. reported a reduction in EV miR-21 released from CSE-treated BEAS-2B cells, which indirectly modulated EMT by alleviating the polarization of M2 macrophages (73). Furthermore, Coresello et al. reported no significant changes in the level of EV miR-21 released from human small airway epithelial cells in response to CSE (163). Variations in the type of airway epithelial cell models, CS sources and EV isolation methods could account for the differences in EV miR-21 levels reported between the studies (164). As mentioned previously, miR-21 has been identified in elevated levels in EVs in response to risk factors such as ageing, as well as from patients with chronic disease including COPD, CVD, diabetes and oseteoporosis. Furthermore, miR-21 has been widely reported as an inflammatory mediator and suggested as a key switch in the inflammatory response (165). Therefore it will be vital to complete further research with standardised EV isolation and characterisation techniques to fully understand the biologically relevant effects of EV miR-21 and its contribution to multimorbidity.
Exposure to respiratory toxins damages the epithelial and endothelial barrier, This contributes to the “overspill” of inflammatory mediators, including EVs produced in the lung, to the circulation. An increase in circulating EVs and alteration of their cargo in response to noxious stimuli may contribute to systemic inflammation and the development of chronic diseases such as COPD. Further studies are required to understand if a distinct EV population can be used to discern the smokers who will go on to develop multiple chronic diseases.
Future Perspectives
The field of EV research is still relatively new and our understanding of the role of EVs is rapidly evolving. Given the proposed function of EVs in mediating pathways that are central to multiple chronic diseases they may pose as novel biomarkers or useful therapeutic targets (Figure 3). However, no definitive link has yet been established between circulating EVs and the development or exacerbation of chronic diseases. A critical question is whether EVs directly contribute to associated pathology or are simply a consequence of the disease. The impact of exposure to different risk factors in modulating disease relevant EVs needs to be determined, alongside their relative contribution and combinatory effects. Furthermore, while a number of studies have alluded to EVs as systemic immune modulators able to reach distant organs, further studies are required to validate this theory.
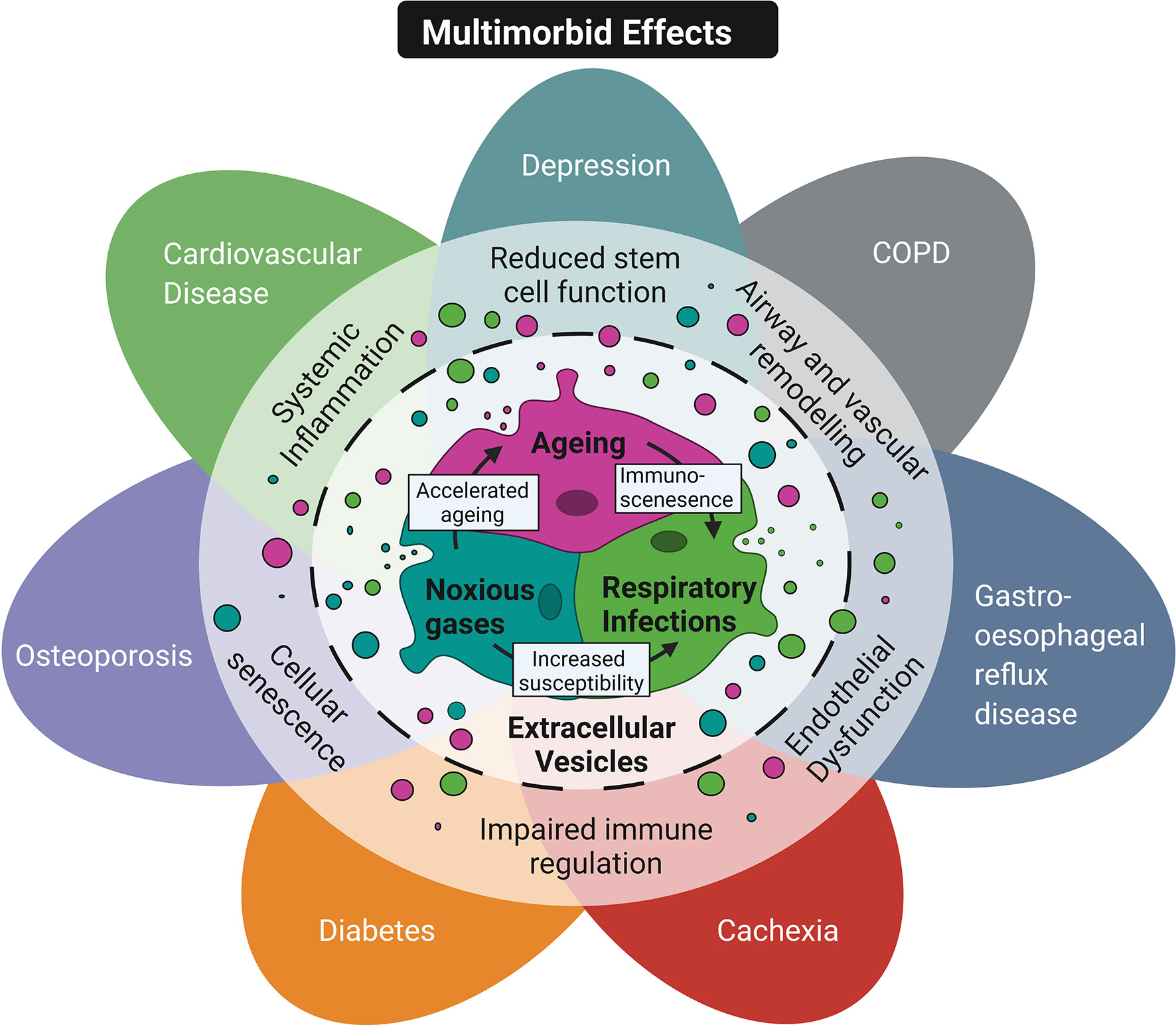
Figure 3 Conceptual diagram of EVs as a common mechanism in multimorbidity. Shared risk factors including ageing, inhalation of noxious stimuli and respiratory infections are thought to contribute to multimorbidity in COPD patients. These risk factors can exacerbate each other. For example ageing promotes immunosenescence and reduces the ability of the immune response to neutralise infection. In addition, smoking increases susceptibility to infection and accelerates features of ageing. These risk factors have also been shown to promote the release of EVs that mediate key pathological features in multimorbid COPD patients' such as reduced stem cell function, airway and vascular remodeling, endothelial dysfunction, impaired immune regulation, cellular senescence and systemic inflammation. Created with BioRender.com.
Current studies investigating the role of EVs in chronic diseases have been limited by the lack of a gold standard for EV isolation and functional characterisation. EV profile characterisation has been demonstrated to be dependent on the experimental models and techniques applied, with variable particle yield, genomic and proteomic EV profiles reported between different methodologies (25). Application of recently emerging technologies will allow better isolation and characterisation of distinct EV populations. For example, microfluidic technologies, such as asymmetric flow field-flow fractionation (AF4), have recently been demonstrated as an improved technique for isolating distinct nanoparticle subpopulations and therefore allow better characterisation of heterogeneous EV populations (166). Furthermore, there is growing interest in techniques that allow single-EV analysis and therefore can tease apart the distinct biophysical and molecular properties of individual EVs in a heterogeneous population. One recent example is single-particle interferometric reflectance imaging (SP-IRI), recently developed and sold as an automated platform called the ExoView system (NanoView Biosciences) (167, 168). This system can be used for multiplexed analysis and allows simultaneous sizing and protein profiling. Once techniques for EV isolation and characterisation are standardised, the use of multi-omics approaches, including transcriptomic, proteomic, metabolomic, and lipidomic would be beneficial to better understand the function and relationship of EV biomolecules (169). However, this will require more transparent reporting of methodological details and increased data availability in future studies.
Another issue is that our current understanding of the immunomodulatory role of EVs in response to biological and environmental risk factors is based largely on in vitro cell culture models that may oversimplify the in vivo functions of EVs due to the limited intercellular interactions. Moreover, many in vivo studies into the function of EVs have been based on murine studies that have previously shown to have limitations regarding recapitulation in the human biological system. Therefore, further work using relevant human ex vivo and co-culture models are required to obtain clinically relevant data. Additionally, while clinical samples provide the biological complexity necessary, they should include a well characterised patient cohort which is large enough to reduce bias due to patient heterogeneity. In clinical studies, analysing EVs across a range of chronic diseases using a standardised isolation protocol and with stratification of multimorbid patients will also be necessary to compare disease related changes in EV cargo.
Conclusion
On review of recent EV literature it is apparent that there is overlap in EV cargo shown to be altered across a range of chronic diseases and in response to disease risk factors such as ageing, infection and smoking. Furthermore many of these changes in EV cargo mediate pathological features, such as chronic inflammation, that are central to multimorbidity in COPD patients and therefore may provide novel diagnostics and therapeutics. However, further studies investigating the function of EVs in multimorbidity using physiologically relevant, disease specific, ex vivo models are required.
Author Contributions
LR: conceptualization, investigation, literature searching, analysis, project administration, writing original draft, reviewing and editing. CS: supervision, conceptualization, reviewing & editing. AW: supervision, reviewing & editing. KS: supervision, conceptualization, reviewing & editing. TW: supervision, conceptualization, reviewing & editing. All authors contributed to the article and approved the submitted version.
Funding
This work was funded by an MRC Integrated PhD studentship awarded for LR’s doctoral studies.
Conflict of Interest
KS reports grants from AstraZeneca, outside the conduct of the study. TW reports grants and personal fees from AstraZeneca, outside the conduct of the study; personal fees and other from MMH, grants and personal fees from GSK, grants and personal fees from AZ, personal fees from BI, grants and personal fees from Synairgen, outside the submitted work.
The remaining authors declare that the research was conducted in the absence of any commercial or financial relationships that could be construed as a potential conflict of interest.
Publisher’s Note
All claims expressed in this article are solely those of the authors and do not necessarily represent those of their affiliated organizations, or those of the publisher, the editors and the reviewers. Any product that may be evaluated in this article, or claim that may be made by its manufacturer, is not guaranteed or endorsed by the publisher.
Acknowledgments
We gratefully acknowledge the help of Jake Weeks for his assistance in creating the figures.
References
1. Statista. Percentage of Leading 10 Causes of Death Worldwide in 2019 (2020). Available at: https://www.statista.com/statistics/311925/top-ten-causes-of-death-worldwide/.
2. European Respiratory Society. The Burden of Lung Disease. European Lung White Book. Available at: https://www.erswhitebook.org/chapters/the-burden-of-lung-disease/.
3. Public Health England. The 2nd Atlas of Variation in Risk Factors and Healthcare for Respiratory Disease in England (2019). Available at: http://fingertips.phe.org.uk/profile/atlas-of-variation.
4. Wedzicha JA, Wilkinson T. Impact of Chronic Obstructive Pulmonary Disease Exacerbations on Patients and Payers. Proc Am Thorac Soc (2006) 3(3):218–21. doi: 10.1513/pats.200510-114SF
5. Singh D, Agusti A, Anzueto A, Barnes PJ, Bourbeau J, Celli BR, et al. Global Strategy for the Diagnosis, Management, and Prevention of Chronic Obstructive Lung Disease: The GOLD Science Committee Report 2019. Eur Respir J (2019) 53:1900164. doi: 10.1183/13993003.00164-2019
6. Wilkinson TMA, Donaldson GC, Hurst JR, Seemungal TAR, Wedzicha JA. Early Therapy Improves Outcomes of Exacerbations of Chronic Obstructive Pulmonary Disease. Am J Respir Crit Care Med (2004) 169(12):1298–303. doi: 10.1164/rccm.200310-1443OC
7. Gao W, Li L, Wang Y, Zhang S, Adcock IM, Barnes PJ, et al. Bronchial Epithelial Cells: The Key Effector Cells in the Pathogenesis of Chronic Obstructive Pulmonary Disease? Respirology (2015) 20(5):722–9. doi: 10.1111/resp.12542
8. Wang YL, Bai C, Li K, Adler KB, Wang X. Role of Airway Epithelial Cells in Development of Asthma and Allergic Rhinitis. Respir Med (2008) 102(7):949–55. doi: 10.1016/j.rmed.2008.01.017
9. Nyunoya T, Mebratu Y, Contreras A, Delgado M, Chand HS, Tesfaigzi Y. Molecular Processes That Drive Cigarette Smoke-Induced Epithelial Cell Fate of the Lung. Am J Respir Cell Mol Biol (2014) 50(3):471–82. doi: 10.1165/rcmb.2013-0348TR
10. John-Schuster G, Günter S, Hager K, Conlon TM, Eickelberg O, Yildirim AÖ. Inflammaging Increases Susceptibility to Cigarette Smokeinduced COPD. Oncotarget (2016) 7(21):30068–83. doi: 10.18632/oncotarget.4027
11. Colarusso C, Terlizzi M, Molino A, Pinto A, Sorrentino R. Role of the Inflammasome in Chronic Obstructive Pulmonary Disease (COPD). Oncotarget (2017) 8(47):81813–24. doi: 10.18632/oncotarget.17850
12. Santos S, Marin A, Serra-Batlles J, de la Rosa D, Solanes I, Pomares X, et al. Treatment of Patients With COPD and Recurrent Exacerbations: The Role of Infection and Inflammation. Int J COPD (2016) 11(1):515–25. doi: 10.2147/COPD.S98333
13. Viniol C, Vogelmeier CF. Exacerbations of COPD. Eur Respir Rev (2018) 27(147):170103. doi: 10.1183/16000617.0103-2017
14. Sapey E, Bafadhel M, Bolton CE, Wilkinson T, Hurst JR, Quint JK. Building Toolkits for COPD Exacerbations: Lessons From the Past and Present. Thorax (2019) 74(9):898–905. doi: 10.1136/thoraxjnl-2018-213035
15. Burke H, Wilkinson TMA. Unravelling the Mechanisms Driving Multimorbidity in COPD to Develop Holistic Approaches to Patient-Centred Care. Eur Respir Rev (2021) 30(160):210041. doi: 10.1183/16000617.0041-2021
16. Tugwell P, Knottnerus JA. Multimorbidity and Comorbidity Are Now Separate MESH Headings. J Clin Epidemiol (2019) 105:VI–VIII. doi: 10.1016/j.jclinepi.2018.11.019
17. Grosdidier S, Ferrer A, Faner R, Piñero J, Roca J, Cosío B, et al. Network Medicine Analysis of COPD Multimorbidities. Respir Res (2014) 15(1):111. doi: 10.1186/s12931-014-0111-4
18. Soumagne T, Guillien A, Roche N, Annesi-Maesano I, Andujar P, Laurent L, et al. In Patients With Mild-to-Moderate Copd, Tobacco Smoking, and Not COPD, Is Associated With a Higher Risk of Cardiovascular Comorbidity. Int J COPD (2020) 15:1545–55. doi: 10.2147/COPD.S253417
19. Kubben N, Misteli T. Shared Molecular and Cellular Mechanisms of Premature Ageing and Ageing-Associated Diseases. Nat Rev Mol Cell Biol (2017) 18(10):595–609. doi: 10.1038/nrm.2017.68
20. Panagiotou N, Neytchev O, Selman C, Shiels P. Extracellular Vesicles, Ageing, and Therapeutic Interventions. Cells (2018) 7(8):110. doi: 10.3390/cells7080110
21. Wahlund CJE, Eklund A, Grunewald J, Gabrielsson S. Pulmonary Extracellular Vesicles as Mediators of Local and Systemic Inflammation. Front Cell Dev Biol (2017) 5:39. doi: 10.3389/fcell.2017.00039
22. Kadota T, Fujita Y, Yoshioka Y, Araya J, Kuwano K, Ochiya T. Emerging Role of Extracellular Vesicles as a Senescence-Associated Secretory Phenotype: Insights Into the Pathophysiology of Lung Diseases. Mol Aspects Med (2018) 60:92–103. doi: 10.1016/j.mam.2017.11.005
23. Watson A, Madsen J, Clark HW, Marshall JS. SP-A and SP-D : Dual Functioning Immune Molecules With Antiviral and Immunomodulatory Properties. Front Immunol (2021) 11:622598. doi: 10.3389/fimmu.2020.622598.
24. Brennan K, Martin K, FitzGerald SP, O’Sullivan J, Wu Y, Blanco A, et al. A Comparison of Methods for the Isolation and Separation of Extracellular Vesicles From Protein and Lipid Particles in Human Serum. Sci Rep (2020) 10(1):1039. doi: 10.1038/s41598-020-57497-7
25. Takov K, Yellon DM, Davidson SM. Comparison of Small Extracellular Vesicles Isolated From Plasma by Ultracentrifugation or Size-Exclusion Chromatography: Yield, Purity and Functional Potential. J Extracell Vesicles (2019) 8:1560809. doi: 10.1080/20013078.2018.1560809
26. Lee H, Groot M, Pinilla-Vera M, Fredenburgh LE, Jin Y. Identification of miRNA-Rich Vesicles in Bronchoalveolar Lavage Fluid: Insights Into the Function and Heterogeneity of Extracellular Vesicles. J Control Release (2019) 294:43–52. doi: 10.1016/j.jconrel.2018.12.008
27. Merchant ML, Rood IM, Deegens JKJ, Klein JB. Isolation and Characterization of Urinary Extracellular Vesicles: Implications for Biomarker Discovery. Nat Rev Nephrol (2017) 13(12):731–49. doi: 10.1038/nrneph.2017.148
28. Li Y, He X, Li Q, Lai H, Zhang H, Hu Z, et al. EV-Origin: Enumerating the Tissue-Cellular Origin of Circulating Extracellular Vesicles Using exLR Profile. Comput Struct Biotechnol J (2020) 18:2851–9. doi: 10.1016/j.csbj.2020.10.002
29. Serban KA, Rezania S, Petrusca DN, Poirier C, Cao D, Justice MJ, et al. Structural and Functional Characterization of Endothelial Microparticles Released by Cigarette Smoke. Sci Rep (2016) 6:31596. doi: 10.1038/srep31596
30. Moon HG, Cao Y, Yang J, Lee JH, Choi HS, Jin Y. Lung Epithelial Cell-Derived Extracellular Vesicles Activate Macrophage-Mediated Inflammatory Responses via ROCK1 Pathway. Cell Death Dis (2015) 6:e2016. doi: 10.1038/cddis.2015.282
31. Théry C, Witwer KW, Aikawa E, Alcaraz MJ, Anderson JD, Andriantsitohaina R, et al. Minimal Information for Studies of Extracellular Vesicles 2018 (MISEV2018): A Position Statement of the International Society for Extracellular Vesicles and Update of the MISEV2014 Guidelines. J Extracell Vesicles (2018) 7:1535750. doi: 10.1080/20013078.2018.1535750.
32. Gandham S, Su X, Wood J, Nocera AL, Alli SC, Milane L, et al. Technologies and Standardization in Research on Extracellular Vesicles. Trends Biotechnol (2020) 38:1066–98. doi: 10.1016/j.tibtech.2020.05.012
33. Simons M, Raposo G. Exosomes - Vesicular Carriers for Intercellular Communication. Curr Opin Cell Biol (2009) 21(4):575–81. doi: 10.1016/j.ceb.2009.03.007
34. Raposo G, Stoorvogel W. Extracellular Vesicles: Exosomes, Microvesicles, and Friends. J Cell Biol (2013) 200(4):373–83. doi: 10.1083/jcb.201211138
35. Gibbings DJ, Ciaudo C, Erhardt M, Voinnet O. Multivesicular Bodies Associate With Components of miRNA Effector Complexes and Modulate miRNA Activity. Nat Cell Biol (2009) 11(9):1143–9. doi: 10.1038/ncb1929
36. Mir B, Goettsch C. Extracellular Vesicles as Delivery Vehicles of Specific Cellular Cargo. Cells (2020) 9(7):1601. doi: 10.3390/cells9071601
37. Admyre C, Grunewald J, Thyberg J, Bripenäck S, Tornling G, Eklund A, et al. Exosomes With Major Histocompatibility Complex Class II and Co-Stimulatory Molecules Are Present in Human BAL Fluid. Eur Respir J (2003) 22(4):578–83. doi: 10.1183/09031936.03.00041703
38. Aiello A, Giannessi F, Percario ZA, Affabris E. An Emerging Interplay Between Extracellular Vesicles and Cytokines. Cytokine Growth Factor Rev (2020) 51:49–60. doi: 10.1016/j.cytogfr.2019.12.003
39. Bartel DP. MicroRNAs: Genomics, Biogenesis, Mechanism, and Function. Cell (2004) 116(2):281–97. doi: 10.1016/S0092-8674(04)00045-5
40. Nolte’T Hoen ENM, Buermans HPJ, Waasdorp M, Stoorvogel W, Wauben MHM. ‘T Hoen PAC. Deep Sequencing of RNA From Immune Cell-Derived Vesicles Uncovers the Selective Incorporation of Small Non-Coding RNA Biotypes With Potential Regulatory Functions. Nucleic Acids Res (2012) 40(18):9272–85. doi: 10.1093/nar/gks658
41. Groot M, Lee H. Sorting Mechanisms for MicroRNAs Into Extracellular Vesicles and Their Associated Diseases. Cells (2020) 9(4):1044. doi: 10.3390/cells9041044
42. Koppers-Lalic D, Hackenberg M, Bijnsdorp IV, van Eijndhoven MAJ, Sadek P, Sie D, et al. Nontemplated Nucleotide Additions Distinguish the Small RNA Composition in Cells From Exosomes. Cell Rep (2014) 8(6):1649–58. doi: 10.1016/j.celrep.2014.08.027
43. Villarroya-Beltri C, Gutiérrez-Vázquez C, Sánchez-Cabo F, Pérez-Hernández D, Vázquez J, Martin-Cofreces N, et al. Sumoylated Hnrnpa2b1 Controls the Sorting of miRNAs Into Exosomes Through Binding to Specific Motifs. Nat Commun (2013) 4:2980. doi: 10.1038/ncomms3980
44. Valadi H, Ekström K, Bossios A, Sjöstrand M, Lee JJ, Lötvall JO. Exosome-Mediated Transfer of mRNAs and microRNAs Is a Novel Mechanism of Genetic Exchange Between Cells. Nat Cell Biol (2007) 9(6):654–9. doi: 10.1038/ncb1596
45. O’Brien K, Breyne K, Ughetto S, Laurent LC, Breakefield XO. RNA Delivery by Extracellular Vesicles in Mammalian Cells and Its Applications. Nat Rev Mol Cell Biol (2020) 21(10):585–606. doi: 10.1038/s41580-020-0251-y
46. Guiot J, Struman I, Louis E, Louis R, Malaise M, Njock M-S. Exosomal miRNAs in Lung Diseases: From Biologic Function to Therapeutic Targets. J Clin Med (2019) 8(9):1345. doi: 10.3390/jcm8091345
47. Watson A, Wilkinson TMA. Respiratory Viral Infections in the Elderly. Ther Adv Respir Dis (2021) 15:1753466621995050. doi: 10.1177/1753466621995050
48. Espeland MA, Crimmins EM, Grossardt BR, Crandall JP, Gelfond JAL, Harris TB, et al. Clinical Trials Targeting Aging and Age-Related Multimorbidity. J Gerontol - Ser A Biol Sci Med Sci (2017) 72(3):355–61. doi: 10.1093/gerona/glw220
49. Singh T, Newman AB. Inflammatory Markers in Population Studies of Aging. Ageing Res Rev (2011) 10(3):319–29. doi: 10.1016/j.arr.2010.11.002
50. Ferrucci L, Fabbri E. Inflammageing: Chronic Inflammation in Ageing, Cardiovascular Disease, and Frailty. Nat Rev Cardiol (2018) 15(9):505–22. doi: 10.1038/s41569-018-0064-2
51. Picca A, Guerra F, Calvani R, Bucci C, Monaco MR Lo, Bentivoglio AR, et al. Mitochondrial Dysfunction and Aging: Insights From the Analysis of Extracellular Vesicles. Int J Mol Sci (2019) 20(4):805. doi: 10.3390/ijms20040805
52. Kaushik S, Cuervo AM. The Coming of Age of Chaperone-Mediated Autophagy. Nat Rev Mol Cell Biol (2018) 19(6):365–81. doi: 10.1038/s41580-018-0001-6
53. Carrard G, Bulteau AL, Petropoulos I, Friguet B. Impairment of Proteasome Structure and Function in Aging. Int J Biochem Cell Biol (2002) 34(11):1461–74. doi: 10.1016/S1357-2725(02)00085-7
54. Brown MK, Naidoo N. The Endoplasmic Reticulum Stress Response in Aging and Age-Related Diseases. Front Physiol (2012) p. 263. doi: 10.3389/fphys.2012.00263
55. Fulop T, Larbi A, Dupuis G, Page A, Frost EH, Cohen AA, et al. Immunosenescence and Inflamm-Aging as Two Sides of the Same Coin: Friends or Foes? Front Immunol (2018) 8:1960. doi: 10.3389/fimmu.2017.01960
56. Song S, Lam EW, Tchkonia T, Kirkland JL, Sun Y. Senescent Cells : Emerging Targets for Human Aging and Age-Related Diseases. Trends Biochem Sci (2020) 45(7):578–92. doi: 10.1016/j.tibs.2020.03.008
57. Feehan J, Tripodi N, Apostolopoulos V. The Twilight of the Immune System: The Impact of Immunosenescence in Aging. Maturitas (2021) 147(January):7–13. doi: 10.1016/j.maturitas.2021.02.006
58. Mckendry RT, Spalluto CM, Burke H, Nicholas B, Cellura D, Al-shamkhani A, et al. Dysregulation of Antiviral Function of CD8 1 T Cells in the Chronic Obstructive Pulmonary Disease Lung Role of the PD-1 – PD-L1 Axis. Am J Respir Crit Care Med (2016) 193:642–51. doi: 10.1164/rccm.201504-0782OC
59. MacNee W, Rabinovich RA, Choudhury G. Ageing and the Border Between Health and Disease. Eur Respir J (2014) 44(5):1332–52. doi: 10.1183/09031936.00134014
60. Kurian TK, Banik S, Gopal D, Chakrabarti S, Mazumder N. Elucidating Methods for Isolation and Quantification of Exosomes: A Review. Mol Biotechnol (2021) 63(4):249–66. doi: 10.1007/s12033-021-00300-3
61. Eitan E, Green J, Bodogai M, Mode NA, Bæk R, Jørgensen MM, et al. Age-Related Changes in Plasma Extracellular Vesicle Characteristics and Internalization by Leukocytes. Sci Rep (2017) 7(1):1342. doi: 10.1038/s41598-017-01386-z
62. Sundar IK, Li D, Rahman I. Proteomic Analysis of Plasma-Derived Extracellular Vesicles in Smokers and Patients With Chronic Obstructive Pulmonary Disease. ACS Omega (2019) 4(6):10649–61. doi: 10.1021/acsomega.9b00966
63. Sundar IK, Li D, Rahman I. Small RNA-Sequence Analysis of Plasma-Derived Extracellular Vesicle miRNAs in Smokers and Patients With Chronic Obstructive Pulmonary Disease as Circulating Biomarkers. J Extracell Vesicles (2019) 8(1):1684816. doi: 10.1080/20013078.2019.1684816
64. Tan DBA, Armitage J, Teo T-H, Ong NE, Shin H, Moodley YP. Elevated Levels of Circulating Exosome in COPD Patients are Associated With Systemic Inflammation. Respir Med (2017) 132:261–4. doi: 10.1016/j.rmed.2017.04.014
65. Ferreira GD, Simões JA, Senaratna C, Pati S, Timm PF, Batista SR, et al. Physiological Markers and Multimorbidity. J Comorbidity (2018) 8(1):2235042X1880698. doi: 10.1177/2235042X18806986
66. Tsukamoto H, Kouwaki T, Oshiumi H. Aging-Associated Extracellular Vesicles Contain Immune Regulatory microRNAs Alleviating Hyperinflammatory State and Immune Dysfunction in the Elderly. iScience (2020) 23(9):101520. doi: 10.1016/j.isci.2020.101520
67. Zheng J, Zhu L, Iok In I, Chen Y, Jia N, Zhu W. Bone Marrow-Derived Mesenchymal Stem Cells-Secreted Exosomal microRNA-192-5p Delays Inflammatory Response in Rheumatoid Arthritis. Int Immunopharmacol (2020) 78:105985. doi: 10.1016/j.intimp.2019.105985
68. Gu C, Zhang H, Gao Y. Adipose Mesenchymal Stem Cells-Secreted Extracellular Vesicles Containing microRNA-192 Delays Diabetic Retinopathy by Targeting ITGA1. J Cell Physiol (2021) 236(7):5036–51. doi: 10.1002/jcp.30213
69. Alibhai FJ, Lim F, Yeganeh A, DiStefano PV, Binesh-Marvasti T, Belfiore A, et al. Cellular Senescence Contributes to Age-Dependent Changes in Circulating Extracellular Vesicle Cargo and Function. Aging Cell (2020) 19(3):1–14. doi: 10.1111/acel.13103
70. Jaeger A, Zollinger L, Saely CH, Muendlein A, Evangelakos I, Nasias D, et al. Circulating microRNAs -192 and -194 Are Associated With the Presence and Incidence of Diabetes Mellitus. Sci Rep (2018) 8(1):14274. doi: 10.1038/s41598-018-32274-9
71. Matsumoto S, Sakata Y, Suna S, Nakatani D, Usami M, Hara M, et al. Circulating P53-Responsive MicroRNAs Are Predictive Indicators of Heart Failure After Acute Myocardial Infarction. Circ Res (2013) 113(3):322–6. doi: 10.1161/CIRCRESAHA.113.301209
72. Burke H, Heinson A, Freeman A, Ostridge K, Watson A, Staples K, et al. Late Breaking Abstract-Differentially Expressed Exosomal miRNAs Target Key Inflammatory Pathways in COPD. Eur Respir J (2018) 52:OA4922. doi: 10.1183/13993003.congress-2018.OA4922
73. He S, Chen D, Hu M, Zhang L, Liu C, Traini D, et al. Bronchial Epithelial Cell Extracellular Vesicles Ameliorate Epithelial–Mesenchymal Transition in COPD Pathogenesis by Alleviating M2 Macrophage Polarization. Nanomed Nanotechnol Biol Med (2019) 18:259–71. doi: 10.1016/j.nano.2019.03.010
74. Zhang M-W, Shen Y-J, Shi J, Yu J-G. MiR-223-3p in Cardiovascular Diseases: A Biomarker and Potential Therapeutic Target. Front Cardiovasc Med (2021) 7:610561. doi: 10.3389/fcvm.2020.610561
75. Saferding V, Hofmann M, Brunner JS, Niederreiter B, Timmen M, Magilnick N, et al. microRNA-146a Controls Age-Related Bone Loss. Aging Cell (2020) 19(11):e13244. doi: 10.1111/acel.13244
76. Zhang J, Xing Q, Zhou X, Li J, Li Y, Zhang L, et al. Circulating miRNA-21 Is a Promising Biomarker for Heart Failure. Mol Med Rep (2017) 16(5):7766–74. doi: 10.3892/mmr.2017.7575
77. Xu J, Chen Y, Yu D, Zhang L, Dou X, Wu G, et al. Evaluation of the Cargo Contents and Potential Role of Extracellular Vesicles in Osteoporosis. Aging (Albany NY) (2021) 13(15):19282–92. doi: 10.18632/aging.203264
78. Zhang D, Lee H, Wang X, Groot M, Sharma L, Dela Cruz CS, et al. A Potential Role of Microvesicle-Containing miR-223/142 in Lung Inflammation. Thorax (2019) 74(9):865–74. doi: 10.1136/thoraxjnl-2018-212994
79. Chiou NT, Kageyama R, Ansel KM. Selective Export Into Extracellular Vesicles and Function of tRNA Fragments During T Cell Activation. Cell Rep (2018) 25(12):3356–70. doi: 10.1016/j.celrep.2018.11.073
80. Mercado N, Ito K, Barnes PJ. Accelerated Ageing of the Lung in COPD: New Concepts. Thorax (2015) 70(5):482–9. doi: 10.1136/thoraxjnl-2014-206084
81. Huang R, Qin C, Wang J, Hu Y, Zheng G, Qiu G, et al. Differential Effects of Extracellular Vesicles From Aging and Young Mesenchymal Stem Cells in Acute Lung Injury. Aging (Albany NY) (2019) 11(18):7996–8014. doi: 10.18632/aging.102314
82. Wang Y, Fu B, Sun X, Li D, Huang Q, Zhao W, et al. Differentially Expressed microRNAs in Bone Marrow Mesenchymal Stem Cell-Derived Microvesicles in Young and Older Rats and Their Effect on Tumor Growth Factor-β1-Mediated Epithelial-Mesenchymal Transition in HK2 Cells. Stem Cell Res Ther (2015) 6:185. doi: 10.1186/s13287-015-0179-x
83. Soeda S, Ohyashiki JH, Ohtsuki K, Umezu T, Setoguchi Y, Ohyashiki K. Clinical Relevance of Plasma miR-106b Levels in Patients With Chronic Obstructive Pulmonary Disease. Int J Mol Med (2013) 31(3):533–9. doi: 10.3892/ijmm.2013.1251
84. Wang J, Gao Z, Gao P. MiR-133b Modulates the Osteoblast Differentiation to Prevent Osteoporosis Via Targeting Gnb4. Biochem Genet (2021) 59(5):1146–57. doi: 10.1007/s10528-021-10048-9
85. Kumar D, Narang R, Sreenivas V, Rastogi V, Bhatia J, Saluja D, et al. Circulatory miR-133b and miR-21 as Novel Biomarkers in Early Prediction and Diagnosis of Coronary Artery Disease. Genes (Basel) (2020) 11(2):164. doi: 10.3390/genes11020164
86. Rajendran P, Rengarajan T, Thangavel J, Nishigaki Y, Sakthisekaran D, Sethi G, et al. The Vascular Endothelium and Human Diseases. Int J Biol Sci (2013) 9(10):1057–69. doi: 10.7150/ijbs.7502
87. Polverino F, Celli BR, Owen CA. COPD as an Endothelial Disorder: Endothelial Injury Linking Lesions in the Lungs and Other Organs? (2017 Grover Conference Series). Pulm Circ (2018) 8(1):1–18. doi: 10.1177/2045894018758528
88. Katsuumi G, Shimizu I, Yoshida Y, Minamino T. Vascular Senescence in Cardiovascular and Metabolic Diseases. Front Cardiovasc Med (2018) 5:18. doi: 10.3389/fcvm.2018.00018
89. Paschalaki KE, Starke RD, Hu Y, Mercado N, Margariti A, Gorgoulis VG, et al. Dysfunction of Endothelial Progenitor Cells From Smokers and Chronic Obstructive Pulmonary Disease Patients Due to Increased Dna Damage and Senescence. Stem Cells (2013) 31(12):2813–26. doi: 10.1002/stem.1488
90. Mensà E, Guescini M, Giuliani A, Bacalini MG, Ramini D, Corleone G, et al. Small Extracellular Vesicles Deliver miR-21 and miR-217 as Pro-Senescence Effectors to Endothelial Cells. J Extracell Vesicles (2020) 9:1725285. doi: 10.1080/20013078.2020.1725285
91. Alique M, Ruíz-Torres MP, Bodega G, Noci MV, Troyano N, Bohórquez L, et al. Microvesicles From the Plasma of Elderly Subjects and From Senescent Endothelial Cells Promote Vascular Calcification. Aging (Albany NY) (2017) 9(3):778–89. doi: 10.18632/aging.101191
92. Weilner S, Schraml E, Wieser M, Messner P, Schneider K, Wassermann K, et al. Secreted Microvesicular miR-31 Inhibits Osteogenic Differentiation of Mesenchymal Stem Cells. Aging Cell (2016) 15(4):744–54. doi: 10.1111/acel.12484
93. Perera WR, Hurst JR, Wilkinson TMA, Sapsford RJ, Mu H. Inflammatory Changes, Recovery and Recurrence at COPD Exacerbation. Eur Respir J (2007) 29(3):527–34. doi: 10.1183/09031936.00092506
94. Wilkinson TMA, Aris E, Bourne S, Clarke SC, Peeters M, Pascal TG, et al. A Prospective, Observational Cohort Study of the Seasonal Dynamics of Airway Pathogens in the Aetiology of Exacerbations in COPD. Thorax (2017) 72:919–27. doi: 10.1136/thoraxjnl-2016-209023
95. Ivey KS, Edwards KM, Talbot HK. Respiratory Syncytial Virus and Associations With Cardiovascular Disease in Adults. J Am Coll Cardiol (2018) 71(14):1574–83. doi: 10.1016/j.jacc.2018.02.013
96. Allard R, Leclerc P, Tremblay C, Tannenbaum TN. Diabetes and the Severity of Pandemic Influenza A (H1N1) Infection. Diabetes Care (2010) 33(7):1491–3. doi: 10.2337/dc09-2215
97. Yin T, Zhu Z, Mei Z, Feng J, Zhang W, He Y, et al. Analysis of Viral Infection and Biomarkers in Patients With Acute Exacerbation of Chronic Obstructive Pulmonary Disease. Clin Respir J (2018) 12(3):1228–39. doi: 10.1111/crj.12656
98. Mulpuru S, Li L, Ye L, Hatchette T, Andrew MK, Ambrose A, et al. Effectiveness of Influenza Vaccination on Hospitalizations and Risk Factors for Severe Outcomes in Hospitalized Patients With COPD. Chest (2019) 155(1):69–78. doi: 10.1016/j.chest.2018.10.044
99. Weeks JR, Staples KJ, Spalluto CM, Watson A, Wilkinson TMA. The Role of Non-Typeable Haemophilus Influenzae Biofilms in Chronic Obstructive Pulmonary Disease. Front Cell Infect Microbiol (2021) 11:720742. doi: 10.3389/fcimb.2021.720742
100. Staples KJ, Taylor S, Thomas S, Leung S, Cox K, Pascal TG, et al. Relationships Between Mucosal Antibodies, Non-Typeable Haemophilus Influenzae (NTHi) Infection and Airway Inflammation in COPD. PLoS ONE (2016) 11(11):e0167250. doi: 10.1371/journal.pone.0167250
101. Osman KL, Jeffer JMC, Woelk CH, Devos N, Pascal TG, Mortier M, et al. Patients With Chronic Obstructive Pulmonary Disease Harbour a Variation of Haemophilus Species. Sci Rep (2018) 8:14734. doi: 10.1038/s41598-018-32973-3
102. Sethi S, Murphy TF. Infection in the Pathogenesis and Course of Chronic Obstructive Pulmonary Disease. N Engl J Med (2008) 359(22):2355. doi: 10.1056/NEJMra0800353
103. Hayden LP, Hobbs BD, Cohen RT, Wise RA, Checkley W, Crapo JD, et al. Childhood Pneumonia Increases Risk for Chronic Obstructive Pulmonary Disease: The COPDGene Study. Respir Res (2015) 16:115. doi: 10.1186/s12931-015-0273-8
104. Burgner DP, Cooper MN, Moore HC, Stanley FJ, Thompson PL, De Klerk NH, et al. Childhood Hospitalisation With Infection and Cardiovascular Disease in Early-Mid Adulthood: A Longitudinal Population-Based Study. PloS One (2015) 10(5):e0125342. doi: 10.1371/journal.pone.0125342
105. Burke H, Freeman A, Cellura DC, Stuart BL, Brendish NJ, Poole S, et al. Inflammatory Phenotyping Predicts Clinical Outcome in COVID-19. Respir Res (2020) 21:245. doi: 10.1186/s12931-020-01511-z
106. Becker RC. Anticipating the Long-Term Cardiovascular Effects of COVID-19. J Thromb Thromb (2020) 50(3):512–24. doi: 10.1007/s11239-020-02266-6
107. Watson A, Öberg L, Angermann B, Spalluto CM, Hühn M, Burke H, et al. Dysregulation of COVID − 19 Related Gene Expression in the COPD Lung. Respir Res (2021) 22:164. doi: 10.1186/s12931-021-01755-3
108. Leung JM, Niikura M, Yang CWT, Sin DD. COVID-19 and COPD. Eur Respir J (2020) 56(2):2002108. doi: 10.1183/13993003.02108-2020
109. Rabbani G, Shariful Islam SM, Rahman MA, Amin N, Marzan B, Robin RC, et al. Pre-Existing COPD Is Associated With an Increased Risk of Mortality and Severity in COVID-19: A Rapid Systematic Review and Meta-Analysis. Expert Rev Respir Med (2021) 15(5):705–16. doi: 10.1080/17476348.2021.1866547
110. Zheng YY, Ma YT, Zhang JY, Xie X. COVID-19 and the Cardiovascular System. Nat Rev Cardiol (2020) 17(5):259–60. doi: 10.1038/s41569-020-0360-5
111. Wu J, Zhang J, Sun X, Wang L, Xu Y, Zhang Y, et al. Influence of Diabetes Mellitus on the Severity and Fatality of SARS-CoV-2 (COVID-19) Infection. Diabetes Obes Metab (2020) 22(10):1907–14. doi: 10.1111/dom.14105
112. Eltom S, Dale N, Raemdonck KRG, Stevenson CS, Snelgrove RJ, Sacitharan PK, et al. Respiratory Infections Cause the Release of Extracellular Vesicles: Implications in Exacerbation of Asthma/COPD. PloS One (2014) 9(6):e101087. doi: 10.1371/journal.pone.0101087
113. Soni S, Wilson MR, O’Dea KP, Yoshida M, Katbeh U, Woods SJ, et al. Alveolar Macrophage-Derived Microvesicles Mediate Acute Lung Injury. Thorax (2016) 71(11):1020–9. doi: 10.1136/thoraxjnl-2015-208032
114. Chahar HS, Corsello T, Kudlicki AS, Komaravelli N, Casola A. Respiratory Syncytial Virus Infection Changes Cargo Composition of Exosome Released From Airway Epithelial Cells. Sci Rep (2018) 8(1):387. doi: 10.1038/s41598-017-18672-5
115. Cypryk W, Lorey M, Puustinen A, Nyman TA, Matikainen S. Proteomic and Bioinformatic Characterization of Extracellular Vesicles Released From Human Macrophages Upon Influenza A Virus Infection. J Proteome Res (2017) 16(1):217–27. doi: 10.1021/acs.jproteome.6b00596
116. Liu YM, Tseng CH, Chen YC, Yu WY, Ho MY, Ho CY, et al. Exosome-Delivered and Y RNA-Derived Small RNA Suppresses Influenza Virus Replication. J BioMed Sci (2019) 26(1):58. doi: 10.1186/s12929-019-0553-6
117. Maemura T, Fukuyama S, Kawaoka Y. High Levels of miR-483-3p Are Present in Serum Exosomes Upon Infection of Mice With Highly Pathogenic Avian Influenza Virus. Front Microbiol (2020) 11:144. doi: 10.3389/fmicb.2020.00144
118. Smith VL, Cheng Y, Bryant BR, Schorey JS. Exosomes Function in Antigen Presentation During an In Vivo Mycobacterium Tuberculosis Infection. Sci Rep (2017) 7:43578. doi: 10.1038/srep43578
119. Bhatnagar S, Schorey JS. Exosomes Released From Infected Macrophages Contain Mycobacterium Avium Glycopeptidolipids and Are Proinflammatory. J Biol Chem (2007) 282(35):25779–89. doi: 10.1074/jbc.M702277200
120. Kwon Y, Nukala SB, Srivastava S, Miyamoto H, Ismail NI, Jousma J, et al. Detection of Viral RNA Fragments in Human iPSC Cardiomyocytes Following Treatment With Extracellular Vesicles From SARS-CoV-2 Coding Sequence Overexpressing Lung Epithelial Cells. Stem Cell Res Ther (2020) 11:514. doi: 10.1186/s13287-020-02033-7
121. Nair RR, Mazza D, Brambilla F, Gorzanelli A, Agresti A, Bianchi ME. LPS-Challenged Macrophages Release Microvesicles Coated With Histones. Front Immunol (2018) 9:1463 . doi: 10.3389/fimmu.2018.01463
122. Goto T, Shimada YJ, Faridi MK, Camargo CA, Hasegawa K. Incidence of Acute Cardiovascular Event After Acute Exacerbation of COPD. J Gen Intern Med (2018) 33(9):1461–8. doi: 10.1007/s11606-018-4518-3
123. Smeeth L, Thomas SL, Hall AJ, Hubbard R, Farrington P, Vallance P. Risk of Myocardial Infarction and Stroke After Acute Infection or Vaccination. N Engl J Med (2004) 351(25):2611–8. doi: 10.1056/NEJMoa041747
124. Takahashi T, Kobayashi S, Fujino N, Suzuki T, Ota C, He M, et al. Increased Circulating Endothelial Microparticles in COPD Patients: A Potential Biomarker for COPD Exacerbation Susceptibility. Thorax (2012) 67(12):1067–74. doi: 10.1136/thoraxjnl-2011-201395
125. Amabile N, Cheng S, Renard JM, Larson MG, Ghorbani A, McCabe E, et al. Association of Circulating Endothelial Microparticles With Cardiometabolic Risk Factors in the Framingham Heart Study. Eur Heart J (2014) 35(42):2972–9. doi: 10.1093/eurheartj/ehu153
126. Inal J. COVID-19 Comorbidities, Associated Procoagulant Extracellular Vesicles and Venous Thromboembolisms: A Possible Link With Ethnicity? Br J Haematol (2020) 190(4):e218–20. doi: 10.1111/bjh.17011
127. Rosell A, Havervall S, von Meijenfeldt F, Hisada Y, Aguilera K, Grover SP, et al. Patients With COVID-19 Have Elevated Levels of Circulating Extracellular Vesicle Tissue Factor Activity That Is Associated With Severity and Mortality. Arterioscler Thromb Vasc Biol (2021) 41(2):878–82. doi: 10.1161/ATVBAHA.120.315547.
128. van Haren FMP, Page C, Laffey JG, Artigas A, Camprubi-Rimblas M, Nunes Q, et al. Nebulised Heparin as a Treatment for COVID-19: Scientific Rationale and a Call for Randomised Evidence. Critical Care (2020) 24(1):454.
129. Tree JA, Turnbull JE, Buttigieg KR, Elmore MJ, Coombes N, Hogwood J, et al. Unfractionated Heparin Inhibits Live Wild Type SARS-CoV-2 Cell Infectivity at Therapeutically Relevant Concentrations. British Journal of Pharmacology (2021) 178(3):626–35.
130. Hsu ACY, Dua K, Starkey MR, Haw TJ, Nair PM, Nichol K, et al. MicroRNA-125a and -B Inhibit A20 and MAVS to Promote Inflammation and Impair Antiviral Response in COPD. JCI Insight (2017) 2(7):e90443. doi: 10.1172/jci.insight.90443
131. Watson A, Spalluto CM, McCrae C, Cellura D, Burke H, Cunoosamy D, et al. Dynamics of IFN-β Responses During Respiratory Viral Infection. Insights for Therapeutic Strategies. Am J Respir Crit Care Med (2020) 201(1):83–94. doi: 10.1164/rccm.201901-0214OC
132. Reynolds S, Lunn K, Beegan R, Tear V, Monk PD. Antiviral Biomarkers Are Upregulated in Sputum Cells Following Administration of Inhaled Interferon Beta to COPD Patients. Eur Respir Soc (2019) 54:OA263. doi: 10.1183/13993003.congress-2019.OA263
133. Volgers C, Benedikter BJ, Grauls GE, Savelkoul PHM, Stassen FRM. Immunomodulatory Role for Membrane Vesicles Released by THP-1 Macrophages and Respiratory Pathogens During Macrophage Infection. BMC Microbiol (2017) 17:216. doi: 10.1186/s12866-017-1122-3
134. Ahmadi Badi S, Bruno SP, Moshiri A, Tarashi S. Siadat, Seyed Davar Masotti A. Small RNAs in Outer Membrane Vesicles and Their Function in Host-Microbe Interactions. Front Microbiol (2020) 11:1209. doi: 10.3389/fmicb.2020.01209e.
135. Yu YJ, Wang XH, Fan GC. Versatile Effects of Bacterium-Released Membrane Vesicles on Mammalian Cells and Infectious/Inflammatory Diseases. Acta Pharmacol Sin (2018) 39(4):514–33. doi: 10.1038/aps.2017.82
136. Kim HJ, Kim YS, Kim KH, Choi JP, Kim YK, Yun S, et al. The Microbiome of the Lung and Its Extracellular Vesicles in Nonsmokers, Healthy Smokers and COPD Patients. Exp Mol Med (2017) 49(4):e316–8. doi: 10.1038/emm.2017.7
137. Sharpe SW, Kuehn MJ, Mason KM. Elicitation of Epithelial Cell-Derived Immune Effectors by Outer Membrane Vesicles of Nontypeable Haemophilus Influenzae. Infect Immun (2011) 79(11):4361–9. doi: 10.1128/IAI.05332-11
138. Schaar V, Vries SPW De, Laura M, Vidakovics AP, Bootsma HJ, Larsson L, et al. Multicomponent Moraxella Catarrhalis Outer Membrane Vesicles Induce an Inflammatory Response and Are Internalized by Human Epithelial Cells. Cell Microbiol (2011) 13(3):432–49. doi: 10.1111/j.1462-5822.2010.01546.x
139. Gunn JS, Bakaletz LO, Wozniak DJ. What’s on the Outside Matters: The Role of the Extracellular Polymeric Substance of Gram-Negative Biofilms in Evading Host Immunity and as a Target for Therapeutic Intervention. J Biol Chem (2016) 291(24):12538–46. doi: 10.1074/jbc.R115.707547
140. Zhang Z, Liu D, Liu S, Zhang S, Pan Y. The Role of Porphyromonas Gingivalis Outer Membrane Vesicles in Periodontal Disease and Related Systemic Diseases. Front Cell Infect Microbiol (2021) 10:585917. doi: 10.3389/fcimb.2020.585917
141. Aguayo S, Schuh CMAP, Vicente B, Aguayo LG. Association Between Alzheimer’s Disease and Oral and Gut Microbiota: Are Pore Forming Proteins the Missing Link? J Alzheimers Dis (2018) 65(1):29–46. doi: 10.3233/JAD-180319
142. Hadzic S, Wu CY, Avdeev S, Weissmann N, Schermuly RT, Kosanovic D. Lung Epithelium Damage in COPD – An Unstoppable Pathological Event? Cell Signal (2020) 68:109540. doi: 10.1016/j.cellsig.2020.109540
143. Stämpfli MR, Anderson GP. How Cigarette Smoke Skews Immune Responses to Promote Infection, Lung Disease and Cancer. Nat Rev Immunol (2009) 9(5):377–84. doi: 10.1038/nri2530
144. Johannessen A, Bakke PS, Hardie JA, Eagan TML. Association of Exposure to Environmental Tobacco Smoke in Childhood With Chronic Obstructive Pulmonary Disease and Respiratory Symptoms in Adults. Respirol (2012) 17(3):499–505. doi: 10.1111/j.1440-1843.2012.02129.x
145. Benedikter BJ, Volgers C, van Eijck PH, Wouters EFM, Savelkoul PHM, Reynaert NL, et al. Cigarette Smoke Extract Induced Exosome Release is Mediated by Depletion of Exofacial Thiols and can be Inhibited by Thiol-Antioxidants. Free Radic Biol Med (2017) 108:334–44. doi: 10.1016/j.freeradbiomed.2017.03.026
146. Schneider DJ, Speth JM, Penke LR, Wettlaufer SH, Swanson JA, Peters-Golden M. Mechanisms and Modulation of Microvesicle Uptake in a Model of Alveolar Cell Communication. J Biol Chem (2017) 292(51):20897–910. doi: 10.1074/jbc.M117.792416
147. Van Eeden SF, Sin DD. Oxidative Stress in Chronic Obstructive Pulmonary Disease: A Lung and Systemic Process. Can Respir J (2013) 20(1):27–9. doi: 10.1155/2013/509130
148. Héliot A, Landkocz Y, Roy Saint-Georges F, Gosset P, Billet S, Shirali P, et al. Smoker Extracellular Vesicles Influence Status of Human Bronchial Epithelial Cells. Int J Hyg Environ Health (2017) 220(2):445–54. doi: 10.1016/j.ijheh.2016.12.010
149. Martin PJ, Héliot A, Trémolet G, Landkocz Y, Dewaele D, Cazier F, et al. Cellular Response and Extracellular Vesicles Characterization of Human Macrophages Exposed to Fine Atmospheric Particulate Matter. Environ Pollut (2019) 254:112933. doi: 10.1016/j.envpol.2019.07.101
150. Benedikter BJ, Bouwman FG, Vajen T, Heinzmann ACA, Grauls G, Mariman EC, et al. Ultrafiltration Combined With Size Exclusion Chromatography Efficiently Isolates Extracellular Vesicles From Cell Culture Media for Compositional and Functional Studies. Sci Rep (2017) 7:15297. doi: 10.1038/s41598-017-15717-7
151. Lee H, Zhang D, Zhu Z, Dela Cruz CS, Jin Y. Epithelial Cell-Derived Microvesicles Activate Macrophages and Promote Inflammation via Microvesicle-Containing microRNAs. Sci Rep (2016) 6:35250. doi: 10.1038/srep35250
152. Shen Y, Lu H, Song G. MiR-221-3p and miR-92a-3p Enhances Smoking-Induced Inflammation in COPD. J Clin Lab Anal (2021) 35(7):e23857. doi: 10.1002/jcla.23857
153. De-Ugarte L, Balcells S, Nogues X, Grinberg D, Diez-Perez A, Garcia-Giralt N. Pro-Osteoporotic miR-320a Impairs Osteoblast Function and Induces Oxidative Stress. PloS One (2018) 13(11):e0208131. doi: 10.1371/journal.pone.0208131
154. Feller D, Kun J, Ruzsics I, Rapp J, Sarosi V, Kvell K, et al. Cigarette Smoke-Induced Pulmonary Inflammation Becomes Systemic by Circulating Extracellular Vesicles Containing Wnt5a and Inflammatory Cytokines. Front Immunol (2018) 9:1724 . doi: 10.3389/fimmu.2018.01724
155. Abraityte A, Vinge LE, Askevold ET, Lekva T, Michelsen AE, Ranheim T, et al. Wnt5a Is Elevated in Heart Failure and Affects Cardiac Fibroblast Function. J Mol Med (Berl) (2017) 95:767–77. doi: 10.1007/s00109-017-1529-1
156. Benedikter BJ, Bouwman FG, Heinzmann ACA, Vajen T, Mariman EC, Wouters EFM, et al. Proteomic Analysis Reveals Procoagulant Properties of Cigarette Smoke-Induced Extracellular Vesicles. J Extracell Vesicles (2019) 8(1):1585163. doi: 10.1080/20013078.2019.1585163
157. Ostridge K, Williams N, Kim V, Bennett M, Harden S, Welch L, et al. Relationship Between Pulmonary Matrix Metalloproteinases and Quantitative CT Markers of Small Airways Disease and Emphysema in COPD. Thorax (2016) 71(2):126–32. doi: 10.1136/thoraxjnl-2015-207428
158. Ostridge K, Williams N, Kim V, Harden S, Bourne S, Coombs NA, et al. Distinct Emphysema Subtypes Defined by Quantitative CT Analysis Are Associated With Specific Pulmonary Matrix Metalloproteinases. Respir Res (2016) 17(1):92. doi: 10.1186/s12931-016-0402-z
159. Li CJ, Liu Y, Chen Y, Yu D, Williams KJ, Liu ML. Novel Proteolytic Microvesicles Released From Human Macrophages After Exposure to Tobacco Smoke. Am J Pathol (2013) 182(5):1552–62. doi: 10.1016/j.ajpath.2013.01.035
160. Genschmer KR, Russell DW, Lal C, Szul T, Bratcher PE, Noerager BD, et al. Activated PMN Exosomes: Pathogenic Entities Causing Matrix Destruction and Disease in the Lung. Cell (2019) 176(1–2):113–26.e15. doi: 10.1016/j.cell.2018.12.002
161. Fujita Y, Araya J, Ito S, Kobayashi K, Kosaka N, Yoshioka Y, et al. Suppression of Autophagy by Extracellular Vesicles Promotes Myofibroblast Differentiation in COPD Pathogenesis. J Extracell Vesicles (2015) 4(1):28388. doi: 10.3402/jev.v4.28388
162. Xu H, Ling M, Xue J, Dai X, Sun Q, Chen C, et al. Exosomal microRNA-21 Derived From Bronchial Epithelial Cells is Involved in Aberrant Epithelium-Fibroblast Cross-Talk in COPD Induced by Cigarette Smoking. Theranostics (2018) 8(19):5419–33. doi: 10.7150/thno.27876
163. Corsello T, Kudlicki AS, Garofalo RP, Casola A. Cigarette Smoke Condensate Exposure Changes RNA Content of Extracellular Vesicles Released From Small Airway Epithelial Cells. Cells (2019) 8(12):1652. doi: 10.3390/cells8121652
164. Gupta R, Radicioni G, Abdelwahab S, Dang H, Carpenter J, Chua M, et al. Intercellular Communication Between Airway Epithelial Cells Is Mediated by Exosome-Like Vesicles. Am J Respir Cell Mol Biol (2019) 60(2):209–20. doi: 10.1165/rcmb.2018-0156OC
165. Sheedy FJ. Turning 21: Induction of miR-21 as a Key Switch in the Inflammatory Response. Front Immunol (2015) 6:19. doi: 10.3389/fimmu.2015.00019
166. Zhang H, Freitas D, Kim HS, Fabijanic K, Li Z, Chen H, et al. Identification of Distinct Nanoparticles and Subsets of Extracellular Vesicles by Asymmetric Flow Field-Flow Fractionation. Nat Cell Biol (2018) 20(3):332–43. doi: 10.1038/s41556-018-0040-4
167. Daaboul GG, Gagni P, Benussi L, Bettotti P, Ciani M, Cretich M, et al. Digital Detection of Exosomes by Interferometric Imaging. Nat Publ Gr (2016) 6:37246. doi: 10.1038/srep37246
168. Crescitelli R, Lässer C, Lötvall J. Isolation and Characterization of Extracellular Vesicle Subpopulations From Tissues. Nat Protoc (2021) 16(3):1548–80. doi: 10.1038/s41596-020-00466-1
Keywords: EV - extracellular vesicle, miRNA - microRNA, COPD - chronic obstructive pulmonary disease, multimorbidity, inflammation
Citation: Reid LV, Spalluto CM, Watson A, Staples KJ and Wilkinson TMA (2021) The Role of Extracellular Vesicles as a Shared Disease Mechanism Contributing to Multimorbidity in Patients With COPD. Front. Immunol. 12:754004. doi: 10.3389/fimmu.2021.754004
Received: 05 August 2021; Accepted: 04 November 2021;
Published: 02 December 2021.
Edited by:
Antoine Louveau, Cleveland Clinic, United StatesReviewed by:
Cristina Tecchio, University of Verona, ItalyJun Araya, Jikei University School of Medicine, Japan
Kazuyoshi Kuwano, The Jikei University School of Medicine, Japan
Sabina Antonela Antoniu, Grigore T. Popa University of Medicine and Pharmacy, Romania
Copyright © 2021 Reid, Spalluto, Watson, Staples and Wilkinson. This is an open-access article distributed under the terms of the Creative Commons Attribution License (CC BY). The use, distribution or reproduction in other forums is permitted, provided the original author(s) and the copyright owner(s) are credited and that the original publication in this journal is cited, in accordance with accepted academic practice. No use, distribution or reproduction is permitted which does not comply with these terms.
*Correspondence: Laura V. Reid, TC5WLlJlaWRAc290b24uYWMudWs=