- 1Department of Oncology, Microbiology and Immunology, Anatomy Unit, Faculty of Science and Medicine, University of Fribourg, Fribourg, Switzerland
- 2Division Infectious Disease and International Medicine, Department of Medicine, Center for Immunology, Minneapolis, MN, United States
- 3Department of Biomedical Sciences, Venetian Institute of Molecular Medicine, Padova, Italy
- 4Department of Biomedical Sciences, University of Padua, Padova, Italy
Cell-mediated cytotoxicity is an essential immune defense mechanism to fight against viral, bacterial or parasitic infections. Upon recognition of an infected target cell, killer lymphocytes form an immunological synapse to release the content of their cytotoxic granules. Cytotoxic granules of humans contain two membrane-disrupting proteins, perforin and granulysin, as well as a homologous family of five death-inducing serine proteases, the granzymes. The granzymes, after delivery into infected host cells by the membrane disrupting proteins, may contribute to the clearance of microbial pathogens through different mechanisms. The granzymes can induce host cell apoptosis, which deprives intracellular pathogens of their protective niche, therefore limiting their replication. However, many obligate intracellular pathogens have evolved mechanisms to inhibit programed cells death. To overcome these limitations, the granzymes can exert non-cytolytic antimicrobial activities by directly degrading microbial substrates or hijacked host proteins crucial for the replication or survival of the pathogens. The granzymes may also attack factors that mediate microbial virulence, therefore directly affecting their pathogenicity. Many mechanisms applied by the granzymes to eliminate infected cells and microbial pathogens rely on the induction of reactive oxygen species. These reactive oxygen species may be directly cytotoxic or enhance death programs triggered by the granzymes. Here, in the light of the latest advances, we review the antimicrobial activities of the granzymes in regards to their cytolytic and non-cytolytic activities to inhibit pathogen replication and invasion. We also discuss how reactive oxygen species contribute to the various antimicrobial mechanisms exerted by the granzymes.
Introduction
A key mechanism against intracellular pathogens, such as viruses, bacteria and parasites, is cell-mediated cytotoxicity exerted by killer lymphocytes of the innate and adaptive immune systems (1, 2). When these cytotoxic immune cells recognize cells infected with intracellular pathogens, they release their cytotoxic granule contents to eliminate the target cells and the intracellular pathogen. Cytotoxicity is mediated by a group of highly homologous serine proteases, the granzymes (Gzms), that are localized in specialized lysosomes of the killer cells (3). The best-established biological role of the Gzms is the induction of programed cell death when these cytotoxic proteases are delivered into the target cells by perforin (PFN) (4–6). PNF is a pore-forming protein (7) that facilitates the uptake of other cytolytic granule components by enhancing endocytic uptake (8–10) and promoting endosomolysis to allow cytosolic release (11) (Figure 1). Lymphocytic cytotoxic granules of humans and some other mammals, but not rodents, contain another membrane-disrupting protein, granulysin (GNLY) (14). GNLY belongs to the saposin-like protein family (SAPLIP) that is characterized by a particular polypeptide motif and its affinity to a variety of lipids (15). GNLY was found to disrupt prokaryotic (but not eukaryotic (16, 17)) membranes and to kill bacteria, parasites and fungi in vitro (18).
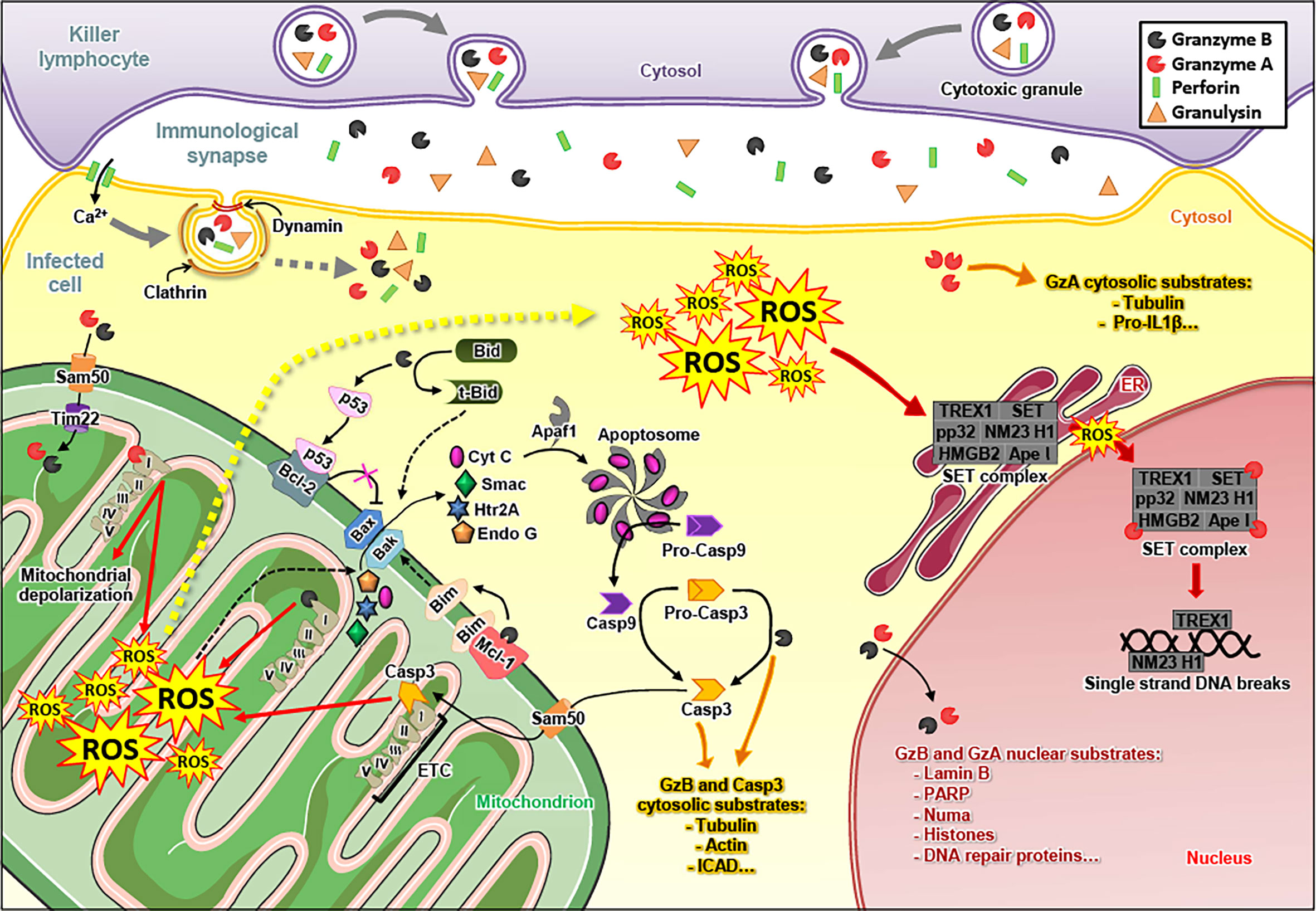
Figure 1 Granzyme A and Granzyme B induce apoptosis of infected cells. The killer lymphocyte releases the content of its granules in the immunological synapse, i.e. the immune effectors granzyme A (GzmA), granzyme B (GzmB), perforin (PFN) and granulysin (GNLY). The endocytosis of GzmA, GzmB, PFN and GNLY in the infected cell is mediated by a PFN-dependent calcium (Ca2+) influx and relies on clathrin and dynamin. Once in the cytosol, GzmA and GzmB cleave various substrates. GzmB triggers the formation of Bax/Bak pores in the outer mitochondrial membrane by cleaving Bid (in truncated-Bid, t-Bid), Mcl-1 (which releases Bim) (12) and p53 (which inhibits Bcl2) (13). GzmB also directly cleaves and matures pro-caspase 3 (Pro-Casp3) into active caspase 3 (Casp3). GzmB and caspase 3 target similar substrates that induce apoptosis of the infected cell. GzmA and GzmB enter the mitochondria – via Sam50 and Tim22 – where they target subunits of the electron transport chain (ETC) complex I, leading to the production of reactive oxygen species (ROS). The ROS favor the release of apoptogenic factors through the Bax/Bak pores, such as cytochrome C (Cyt C), Smac, Htr2A and endonuclease G (Endo G) in the cytosol. Cyt C binds Apaf1 to form the apoptosome, which matures the pro-caspase 9 (pro-Casp9) into active caspase 9 (Casp9). It is noteworthy that caspase 3, either activated by GzmB or caspase 9, also reaches the mitochondria – via Sam50 – where it cleaves a subunit of ETC complex I, leading to the production of ROS. Following these events, ROS concentration increases in the cytosol of the infected cell. The cytosolic ROS are involved in the translocation of the SET complex from the endoplasmic reticulum (ER) to the nucleus, where it is cleaved by GzmA and turns into a DNA degrading complex. GzmA and GzmB also reach the nucleus where they cleave nuclear substrates, such as lamin B, histones and PARP-1.
While the Gzms-mediated induction of host cell apoptosis is well established, the mechanisms of intracellular pathogen elimination is far less clear constituting an emerging field in recent years. The most obvious mechanism is that host cell death deprives obligate intracellular pathogens of their protective niche. Host cell death plays a major role in the elimination of many viruses (19) and obligate intracellular bacteria, such as Chlamydia spp (20, 21). These kind of pathogens counteract the host cell death machinery with a variety of inhibitory mechanisms, such as prevention of cytochrome C release (22) or the upregulation and/or mimicry of host anti-apoptotic proteins (23–25).
In addition to the induction of cell death, a direct mechanism of antimicrobial activities by the Gzms was discovered in numerous studies, which is mediated by the proteolytic degradation of microbial proteins to activate microbial death pathways, hence, limiting their growth inside a host, independently of host cell death. In this review, we aim to dissect these mechanisms with a particular focus on oxidative versus non-oxidative killing pathways.
The Granzymes
The Gzms are a family of serine proteases firstly described by the team of Jürg Tschopp in 1986, who identified “granular enzymes” in the secretory granules of cytolytic lymphocytes (26). There are 5 human Gzms (A, B, H, K and M) and 10 mouse orthologues (A, B, C, D, E, F, G, K, M and N). The human Gzms are encoded from three different chromosomal loci: the chymase locus on chromosome 14 encodes for GzmB and GzmH, the met-ase locus on chromosome 19 for GzmM, and the tryptase locus on chromosome 5 harbors GzmA and GzmK (27). Although the human Gzms are highly homologous and share the catalytic triad (His57, Asp102 and Ser195), there are remarkable differences in their primary substrate specificities (28). The tryptases GzmA and GzmK cleave substrates after Arg or Lys (29, 30), GzmB cleaves after Asp (31), GzmH cleaves after Tyr or Phe (32), and GzmM cleaves after Leu or Met (33).
It is noteworthy that the Gzms, in particular GzmB, are not only expressed and secreted by killer lymphocytes. They were also detected in various non-cytotoxic immune cells (34), non-immune cells (35) and cancer cells (36–38). Interestingly, the non-cytolytic activities of granzyme B also modulate the differentiation of lymphoid cells via the interference with NOTCH1 signaling (39) or with production of IL-17 (40). As PFN is exclusively expressed in activated killer lymphocytes (41), the Gzms released from the above listed cells will exhibit predominantly extracellular effects. Potential activities include remodeling of extracellular matrix (42), modulating inflammation (43–45), detachment-mediated cell death, anoikis (46), and – as reviewed below – exerting antimicrobial activity against invading pathogens in synergy with antimicrobial peptides (AMPs) or by targeting secreted microbial proteins.
The Granzymes in Cell Death
Due to this diversity in the cleavage site specificity, all Gzms have their unique degradomes (47), resulting in the activation of widely differing death pathways in target cells after cytosolic delivery by PFN. The best characterized death pathways are those of GzmA (48) and GzmB (49). GzmB executes death in a caspase-dependent manner (50) (Figure 1). Once released into the cytosol of a target cell, GzmB can directly cleave several caspases (51, 52), including caspase 3 (53). This executioner caspase trigger the release of an active DNase (CAD), responsible for DNA fragmentation upon various apoptotic stimuli (54). Human GzmB also efficiently cleaves the pro-apoptotic protein Bid (55). Truncated Bid induces Bad/Bax-dependent mitochondrial outer membrane permeabilization, the release of cytochrome C, SMAC/DIABLO, and other proteins, such as Htra2/omi, ultimately leading to apoptosome formation and activation of caspase 9 (56–61).
GzmA induces a cell death harboring morphological features similar to apoptosis: chromatin condensation, nuclear fragmentation, membrane blebbing, mitochondrial swelling and loss of cristae. However, GzmA does not activate executioner caspases to kill the cell. Cytosolic delivery of GzmA triggers a complex cascade of events that includes the translocation of a protein complex known as SET from the ER to the nucleus, ultimately leading to the nuclear transfer of two nucleases (NM23-H1 and Trex1) and lethal DNA damage (62–64). These pro-apoptotic features and mechanisms of GzmA were essentially established in the laboratory of Judy Lieberman (65).
An important common death mechanism of GzmA and GzmB is nuclear uptake to attack several nuclear proteins, involved in structural integrity, DNA repair and RNA splicing (66–72).
For the residual, “orphan” Gzms, caspase-dependency to induce death or even if the induction of apoptosis is their major function is still not clear and needs further study (73, 74). However, there are multiple lines of evidence suggesting that GzmM, GzmH and GzmK, as well as the non-orphan GzmA, have well defined proinflammatory and antimicrobial roles as further discussed below (75–78).
Oxidative Cell Death Pathways Driven by the Granzymes
A critical common feature of GzmA and GzmB death pathways is the mitochondrial uptake of these enzymes (Figure 1). Once in the mitochondria, the Gzms cleave four subunits of the respiratory chain complex 1 (NDUFS3, NDUFV1, NDUFS1 and NDUFS2). The disruption of the electron transport chain dramatically increases premature electron leakage, leading to the formation of reactive oxygen species (ROS), a decrease in mitochondrial respiration and the loss of cristae (79–81). Strikingly, caspase 3 also degrades a complex 1 subunit (NDUFS1) to induce ROS-dependent cell death (82–84). In challenge of the orthodox mitochondrial import biology, GzmA and GzmB (and potentially caspase 3), without containing a canonical mitochondrial import sequence, cross the outer mitochondrial membrane through SAM50 channels and the inner membrane through TIM22 in a mtHSP70-dependent manner (85). The resulting increased ROS generation facilitates the release of apoptogenic factors through Bax/Bak pores and drives the nuclear translocation of the SET complex to enhance GzmB and GzmA death pathways, respectively. At least one study suggests that there might be additional, extra-mitochondrial sources of ROS induced by GzmB, in particular via the activation of NADPH-oxidase (86).
Cytolytic Antimicrobial Functions of the Granzymes
The induction of host cell death via the granule exocytosis pathway is an obvious effector mechanism used by killer lymphocytes to eliminate the host cells and obligate intracellular pathogens, such as viruses (87) and certain unicellular parasites (88) or bacteria (89). Suicidal death is an approved defense mechanism of cells infected with pathogens independently of a lymphocyte attack (90–92). Programed host cell death deprives the pathogens of their protective niche, minimizes the risk of dissemination as membrane integrity is initially preserved, and recruits and activates phagocytes to digest the remains (93). Therefore, it is not surprising that obligate intracellular pathogens evolved multiple mechanisms to counteract the death machinery, as already documented in a vast body of comprehensive reviewing literature (87, 94–97). More interesting in this particular context, the Gzms are capable to digest vital microbial substrates independently of host cell death that can directly affect pathogen survival as discussed below in the main focus of this reviewing article.
Non-Cytolytic Antimicrobial Functions of the Granzymes
Non-cytolytic, direct antimicrobial activities by the Gzms were primarily demonstrated in virus-infected cells (2). When the Gzms enter a virus-infected cell in a PFN-dependent manner, they will induce apoptosis to deprive the virus of its protective niche as described above. The induction of programed cell death is often inefficient as many viruses evolved multiple pathways to inhibit the death machinery by means of caspase or Gzms inhibition, as well as by mimicking anti-apoptotic proteins, such as Bcl-2 (98–101). Nevertheless, the Gzms can effectively overcome this inhibition by targeting viral proteins or host proteins hijacked by the virus involved in viral replication. The laboratory of Markus Simon previously demonstrated that mouse GzmA cleaved and therefore inactivated the enzymatic activity of reverse transcriptase from Moloney murine leukemia virus. As reverse transcriptase activity is critical for the retroviral life cycle, GzmA might potentially interfere with retroviral replication (102).
In a report concerning adenovirus, GzmH was shown to proteolytically degrade adenovirus DNA-binding protein (DBP), a crucial viral component DNA replication (103). Interestingly, GzmH additionally directly inactivated L4-100K assembly protein crucial for viral assembly and also a potent inhibitor of GzmB (104), suggesting complex interaction of these serine proteases in virus-infected cells.
Also for the family of Herpesviridae, such as human cytomegalovirus (HCMV) or herpes simplex virus-1 (HSV-1), multiple viral substrates were identified that are targeted by the Gzms. GzmM interferes with HCMV replication independently of cell death by the proteolytic degradation of phosphoprotein 71, a HCMV protein critical for immediate-early protein expression (105). In more recent works, the same laboratory demonstrated that virus-specific T cells control HCMV replication in a non-cytolytic manner by the Gzms-mediated degradation of the HCMV immediate early proteins IE1 and IE2 (106), as well as of host cell hnRNP K, essential for HCMV replication (107).
GzmA deficiency in mice was associated with impaired control of HSV-1 in infected neurons (108). In addition, human GzmB cleaves the HSV-1 immediate-early protein ICP4, therefore potentially contributing to the non-cytolytic inhibition of viral reactivation in latently infected ganglion cells, mediated by HSV-1 specific T cells (109). In a more recent study, several novel HSV-1 GzmB substrates were identified, suggesting an even broader non-cytolytic role of GzmB in the control of Herpesviridae (110).
In conclusion, non-cytolytic, direct antimicrobial activities of the Gzms against viruses are well established. Viral substrates or, for viral replication, essential host factors that are targeted by the Gzms were identified for many additional viruses, such as vaccinia (111), hepatitis C (112) and hepatitis B (113), as well as influenza A virus (114).
Less is known for other intracellular pathogens, such as bacteria or parasites. In earlier work, we found that activating human naïve T cells with bacterial antigens not only triggered the expression of known antibacterial effectors, such as GNLY, interferon-γ or tumor necrosis factor-α, but also of the Gzms in remarkable high levels, in particular GzmB (115). These findings corroborated a previous study indicating elevated plasma levels of GzmA and GzmB in patients with bacterial infections or after endotoxin administration (116). By following up these works, we realized through functional antibacterial assays that GzmA, GzmB and GzmM (the other human Gzms were not tested) are potent antibacterial effectors when these enzymes are delivered into bacteria by GNLY (117, 118) (Figure 2). Building on these findings, a recent study demonstrated that the high levels of secreted GzmB and GNLY by activated mucosa-associated invariant T cells (MAIT) not only directly damage bacteria but also increase the susceptibility to carbapenems in multidrug resistant E. coli (119, 120). In addition, it was revealed that the three major effector molecules of cytotoxic lymphocytes (Gzms, GNLY and PFN) collaborate in a highly coordinated manner to kill intracellular bacteria, such as Listeria monocytogenes (121). The proteolytic activity of the Gzms is necessary to achieve this function, as mutations of their catalytic site impaired the killing of intracellular pathogens. These mutations were introduced by using a mammalian expression system, allowing the generation of comparable purifications of catalytically active and non-active Gzms (122). An unbiased proteomics search for GzmB substrates in several bacterial strains revealed a well-defined list of bacterial proteins, involved in multiple critical metabolic pathways, including protein synthesis and virulence (123). Indeed, extracellular Gzms degraded secreted bacterial virulence factors in absence of GNLY, overall decreasing virulence of the affected bacteria, therefore enabling bystander immune and non-immune cells to more efficiently eliminate the invading pathogens (124).
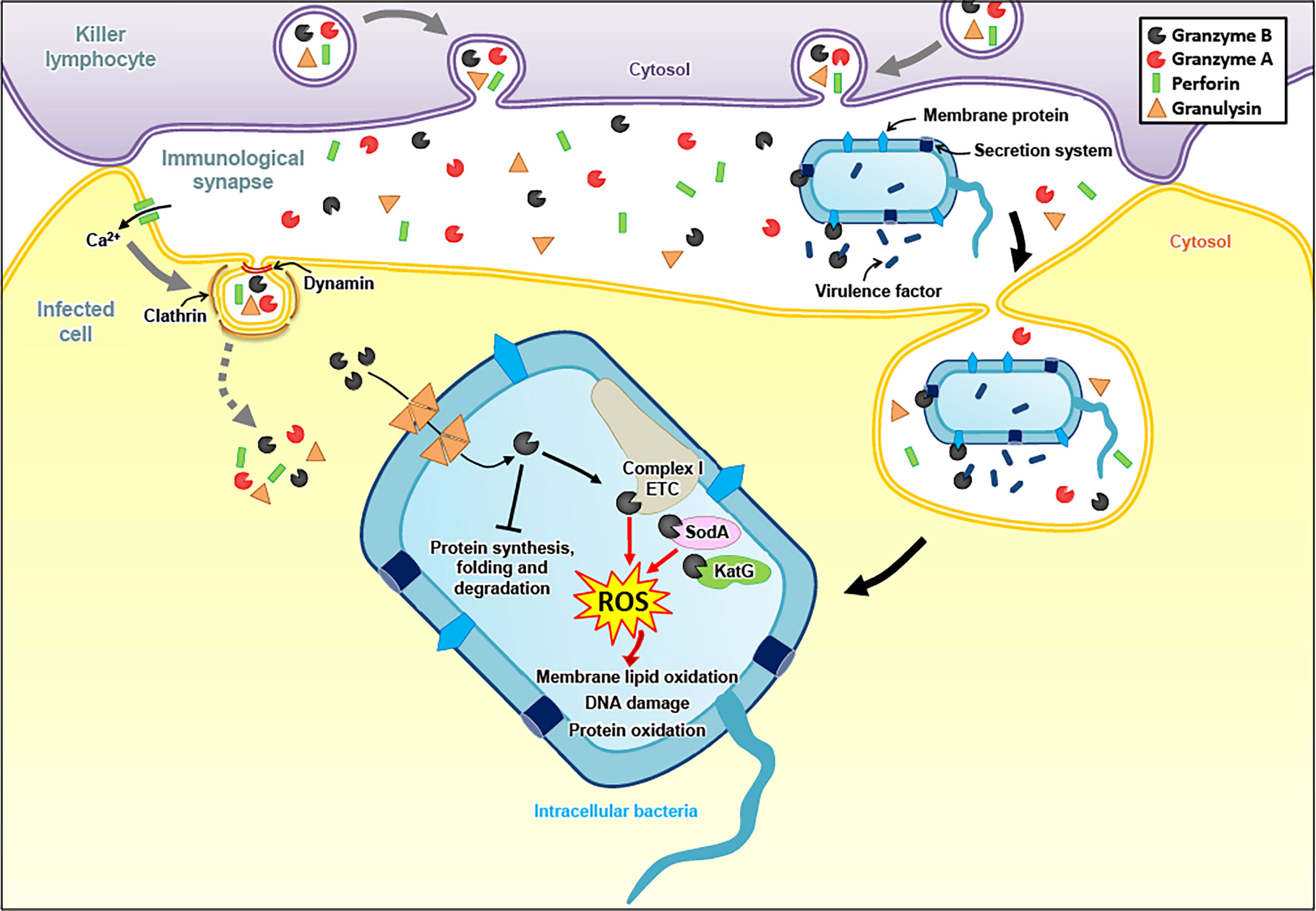
Figure 2 Granzyme-mediated death pathways in bacteria. To fight intracellular bacteria, Gzms and GNLY are delivered into infected cells in a PFN dependent manner. GNLY then forms pores in bacterial membranes, allowing the entry of the Gzms into the bacterial cytosol. GzmB cleaves the catalytic subunits of electron transport chain (ETC) complex I, as well as bacterial proteins involved in antioxidant defense, generating ROS that induce membrane lipid oxidation, DNA damage and protein oxidation. GzmB also target various bacterial proteins involved in protein synthesis, folding and degradation. Independently of GNLY, extracellular GzmB directly targets external bacterial components, such as secretion systems, membrane proteins and secreted virulence factors to attenuate virulence and, consequently, to facilitate bacterial elimination in bystander cells.
Interestingly, Gzms-mediated killing mechanisms after delivery by PFN and GNLY were also found against certain unicellular parasites, such as Plasmodium falciparum (125, 126). For the Plasmodium parasite, we found that the mechanism of Gzms delivery changed upon maturation of the intracellular pathogen in red blood cells (RBCs). While early stage infected RBCs (rings and trophozoites) are susceptible to PFN and resistant to GNLY, late stages (schizonts) display the opposite behavior due to membrane cholesterol depletion and increased phosphatidylserine exposure upon parasite maturation (Figure 3) (127). Also for Toxoplasma gondii and Trypanosoma cruzi, a PFN- and GNLY-dependent delivery mechanism of the Gzms was revealed that induced a death pathway in the parasites, displaying morphological features highly similar to mammalian apoptosis (Figure 4) (128).
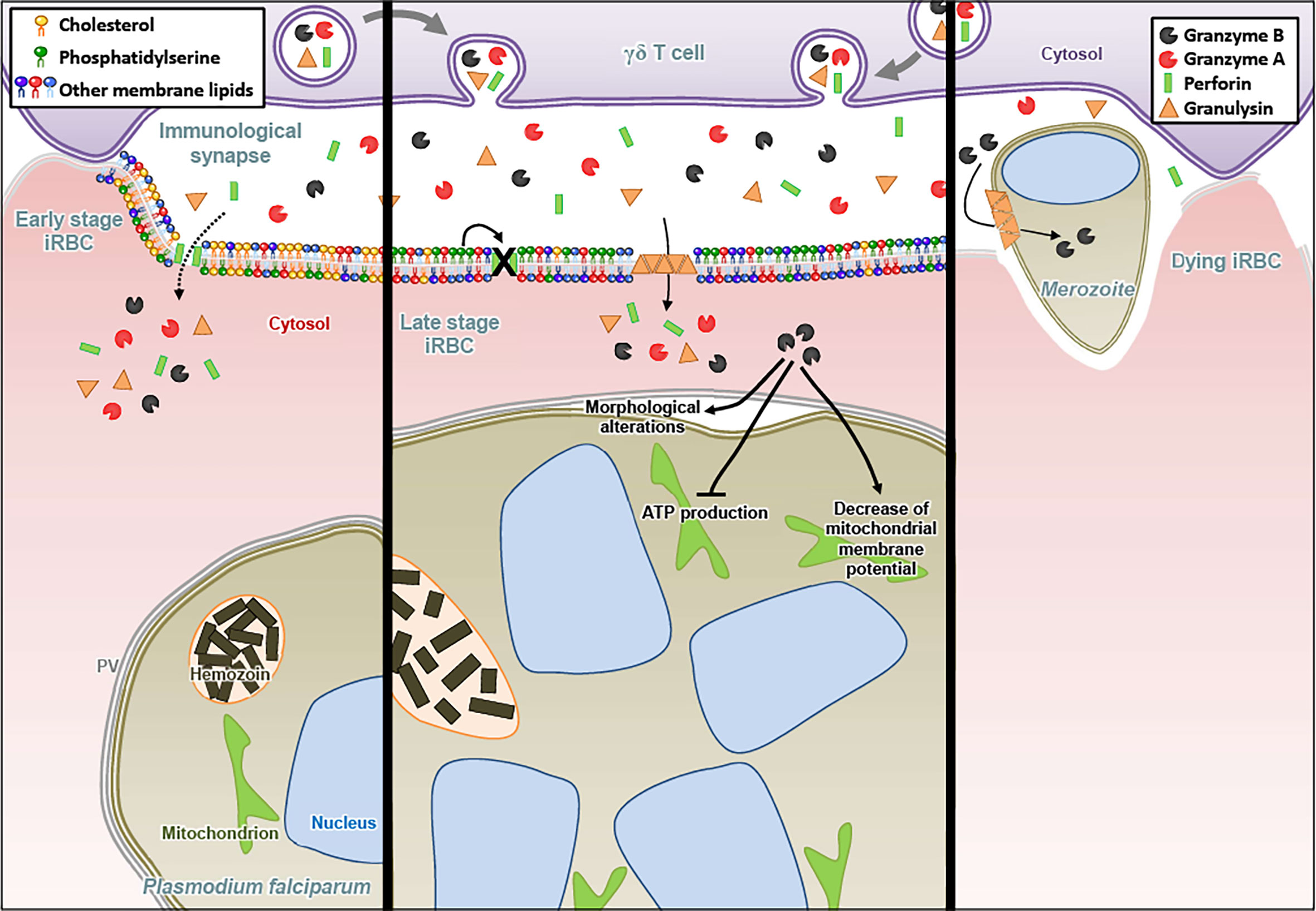
Figure 3 Granzyme B released by γδ T cells contributes to anti-malaria defense. The particular killer lymphocyte subset bearing the γδ T receptor forms an immunological synapse with Plasmodium falciparum-infected red blood cells (iRBC). In early stage iRBC, the plasma membrane contains cholesterol-enriched lipid rafts and the negatively charged phosphatidylserine (PS) is predominantly present in the inner leaflet. At that early stage, PFN can form membrane pores allowing the entry of Gzms, while being resistant to GNLY lysis. For late stage iRBC, cholesterol depletion allows the GNLY to disrupt the membrane while the surface exposure of PS inhibits the formation of PFN pores. Once in the iRBC, GzmB induces dramatic morphological alterations of late stage parasites (schizonts), notably the detachment of the parasitophorous vacuole (PV). Moreover, GzmB inhibits ATP production and decreases the mitochondrial membrane potential of the parasite. At the end of the parasite growth cycle, the rupture of iRBC plasma membrane leads to merozoites egress. GNLY also disrupts the membrane of the merozoite, allowing the entry of GzmB in the parasite.
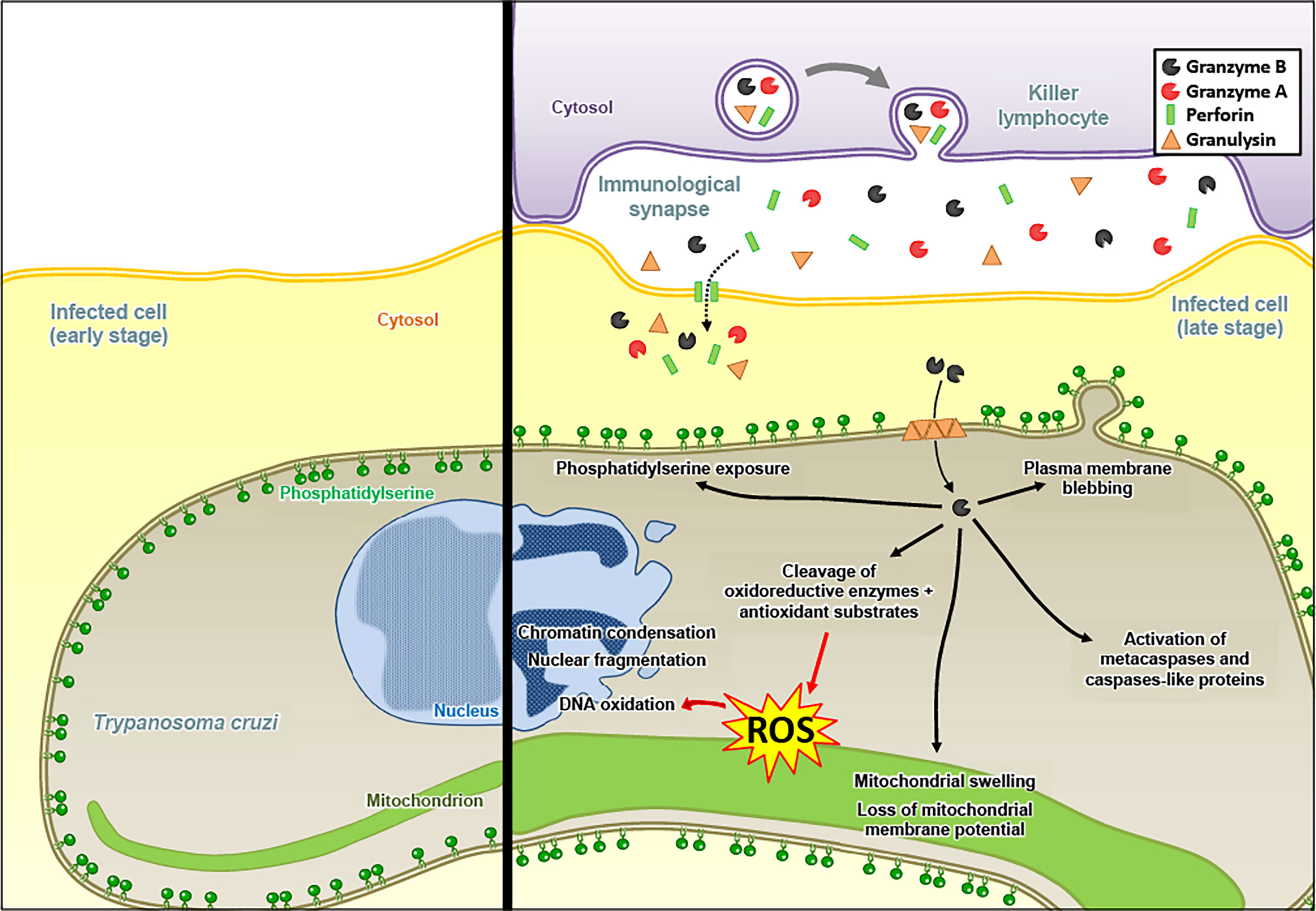
Figure 4 Granzyme B induces an apoptosis-like death pathway in Trypanosoma cruzi. The Gzms of killer lymphocyte granules reach the cytosol of T. cruzi in an infected host cell via a PFN- and GNLY-dependent delivery mechanism. After entry into the parasites, GzmB targets antioxidant defense substrates, which leads to the accumulation of ROS, responsible for DNA oxidation. GzmB triggers classical mammalian apoptosis features: (1) chromatin condensation and nuclear fragmentation, (2) mitochondrial swelling and loss of membrane potential, (3) plasma membrane blebbing, and (4) phosphatidylserine exposure. GzmB also induces the activation of metacaspases and caspase-like proteins, although it might not be required for GzmB-mediated parasite death.
Oxidative Antimicrobial Functions of the Granzymes
As for the cytolytic activities of the Gzms, the induction of ROS seems to be a critical merging point in the antimicrobial mechanisms against various pathogens. In E. coli, the Gzms attacked homologue subunits of the respiratory chain complex-1, as in mammalian mitochondria, suggesting an evolutionary well-conserved killing mechanism. The premature electron leakage from the disrupted respiratory machinery in combination with the GzmB proteolysis of important antioxidant defense enzymes, such as superoxide dismutase and catalase, triggered lethal ROS levels in the affected bacteria (118). GzmB also extensively targeted the ROS defense machinery in the proteome of Mycobacteria tuberculosis, Listeria monocytogenes, as well as Salmonella typhimurium, suggesting that oxidative mechanisms also play a central role in the GzmB-mediated death pathways in these bacterial pathogens (123, 124). Major antioxidant enzymes were also degraded by GzmB in the unicellular parasites, Plasmodium falciparum, Toxoplasma gondii and Trypanosoma cruzi (125, 128). For the latter parasites, a dominant role of ROS in the killing mechanism was indicated by several lines of evidence: 1. ROS were produced in response to GzmB after delivery with pore forming proteins, 2. ROS scavengers efficiently inhibited the killing, and 3. GzmB uncleavable point mutations in major antioxidant defense enzymes slowed down the death pathway (128). GzmB delivery into unicellular parasites also clearly affect the mitochondria as indicated in morphological alterations, loss of mitochondrial membrane potential and decreased ATP production (125, 128).
Conclusions
Though this particular field of research is only developing and further study is necessary, we think it is fair to state that the Gzms exert potent antimicrobial activities by direct proteolysis of vital microbial substrates that are crucial for their replication. Best studied so far in virus-infected cells; however, numerous studies indicate that the Gzms can also lethally affect intracellular bacteria and unicellular parasites by means that are independent of host cell cytolysis. Mitochondria and increased ROS generation seem to be on center stage in Gzms-mediated death pathways in mammalian cells and unicellular parasites. As mitochondria originated from endosymbiotic alpha-proteobacteria, it was not surprising to find respiratory chain disruption by the Gzms in modern living bacteria. To what exact extent these ROS contribute to the killing pathways of the different microbes and mammalian cells is still a matter of debate and needs further study. Nonetheless, there is little doubt that ROS pathways seem to be a highly conserved target in Gzms-mediated death pathways in various species that are evolutionarily far apart.
Author Contributions
MW conceived the concept. ML, MH-C, P-YM, DM and MW researched the literature, wrote, edited and revised the manuscript. ML designed and illustrated the figures. All authors contributed to the article and approved the submitted version.
Funding
This work was supported by the Swiss National Science Foundation (SNSF grant # 310030_169928), the Novartis Foundation for Medical-Biological Research (Novartis grant # 20B136), and the Vontobel-Foundation (all to MW). The funders were not involved in the study design, collection, analysis, interpretation of data, the writing of this article or the decision to submit it for publication.
Conflict of Interest
The authors declare that the research was conducted in the absence of any commercial or financial relationships that could be construed as a potential conflict of interest.
Publisher’s Note
All claims expressed in this article are solely those of the authors and do not necessarily represent those of their affiliated organizations, or those of the publisher, the editors and the reviewers. Any product that may be evaluated in this article, or claim that may be made by its manufacturer, is not guaranteed or endorsed by the publisher.
Acknowledgments
Figures were illustrated using SMART (Servier Medical Art), licensed under a Creative Common Attribution 3.0 Generic License. http://smart.servier.com/.
References
1. Barry M, Bleackley RC. Cytotoxic T Lymphocytes: All Roads Lead to Death. Nat Rev Immunol (2002) 2(6):401–9. doi: 10.1038/nri819
2. Russell JH, Ley TJ. Lymphocyte-Mediated Cytotoxicity. Annu Rev Immunol (2002) 20:323–70. doi: 10.1146/annurev.immunol.20.100201.131730
3. Trapani JA. Granzymes: A Family of Lymphocyte Granule Serine Proteases. Genome Biol (2001) 2(12):REVIEWS3014. doi: 10.1186/gb-2001-2-12-reviews3014
4. Lowin B, Peitsch MC, Tschopp J. Perforin and Granzymes: Crucial Effector Molecules in Cytolytic T Lymphocyte and Natural Killer Cell-Mediated Cytotoxicity. Curr Top Microbiol Immunol (1995) 198:1–24. doi: 10.1007/978-3-642-79414-8_1
5. Froelich CJ, Dixit VM, Yang X. Lymphocyte Granule-Mediated Apoptosis: Matters of Viral Mimicry and Deadly Proteases. Immunol Today (1998) 19(1):30–6. doi: 10.1016/S0167-5699(97)01184-5
6. Podack ER, Lowrey DM, Lichtenheld M, Hameed A. Function of Granule Perforin and Esterases in T Cell-Mediated Reactions. Components Required for Delivery of Molecules to Target Cells. Ann N Y Acad Sci (1988) 532:292–302. doi: 10.1111/j.1749-6632.1988.tb36347.x
7. Lichtenheld MG, Olsen KJ, Lu P, Lowrey DM, Hameed A, Hengartner H, et al. Structure and Function of Human Perforin. Nature (1988) 335(6189):448–51. doi: 10.1038/335448a0
8. Froelich CJ, Orth K, Turbov J, Seth P, Gottlieb R, Babior B, et al. New Paradigm for Lymphocyte Granule-Mediated Cytotoxicity. Target Cells Bind and Internalize Granzyme B, But an Endosomolytic Agent Is Necessary for Cytosolic Delivery and Subsequent Apoptosis. J Biol Chem (1996) 271(46):29073–9. doi: 10.1074/jbc.271.46.29073
9. Thiery J, Keefe D, Saffarian S, Martinvalet D, Walch M, Boucrot E, et al. Perforin Activates Clathrin- and Dynamin-Dependent Endocytosis, Which Is Required for Plasma Membrane Repair and Delivery of Granzyme B for Granzyme-Mediated Apoptosis. Blood (2010) 115(8):1582–93. doi: 10.1182/blood-2009-10-246116
10. Walch M, Latinovic-Golic S, Velic A, Sundstrom H, Dumrese C, Wagner CA, et al. Perforin Enhances the Granulysin-Induced Lysis of Listeria Innocua in Human Dendritic Cells. BMC Immunol (2007) 8:14. doi: 10.1186/1471-2172-8-14
11. Thiery J, Keefe D, Boulant S, Boucrot E, Walch M, Martinvalet D, et al. Perforin Pores in the Endosomal Membrane Trigger the Release of Endocytosed Granzyme B Into the Cytosol of Target Cells. Nat Immunol (2011) 12(8):770–7. doi: 10.1038/ni.2050
12. Han J, Goldstein LA, Gastman BR, Froelich CJ, Yin XM, Rabinowich H. Degradation of Mcl-1 by Granzyme B: Implications for Bim-Mediated Mitochondrial Apoptotic Events. J Biol Chem (2004) 279(21):22020–9. doi: 10.1074/jbc.M313234200
13. Ben Safta T, Ziani L, Favre L, Lamendour L, Gros G, Mami-Chouaib F, et al. Granzyme B-Activated P53 Interacts With Bcl-2 To Promote Cytotoxic Lymphocyte-Mediated Apoptosis. J Immunol (2015) 194(1):418–28. doi: 10.4049/jimmunol.1401978
14. Krensky AM, Clayberger C. Biology and Clinical Relevance of Granulysin. Tissue Antigens (2009) 73(3):193–8. doi: 10.1111/j.1399-0039.2008.01218.x
15. Vaccaro AM, Salvioli R, Tatti M, Ciaffoni F. Saposins and Their Interaction With Lipids. Neurochem Res (1999) 24(2):307–14. doi: 10.1023/A:1022530508763
16. Barman H, Walch M, Latinovic-Golic S, Dumrese C, Dolder M, Groscurth P, et al. Cholesterol in Negatively Charged Lipid Bilayers Modulates the Effect of the Antimicrobial Protein Granulysin. J Membr Biol (2006) 212(1):29–39. doi: 10.1007/s00232-006-0040-3
17. Walch M, Eppler E, Dumrese C, Barman H, Groscurth P, Ziegler U. Uptake of Granulysin via Lipid Rafts Leads to Lysis of Intracellular Listeria Innocua. J Immunol (2005) 174(7):4220–7. doi: 10.4049/jimmunol.174.7.4220
18. Stenger S, Hanson DA, Teitelbaum R, Dewan P, Niazi KR, Froelich CJ, et al. An Antimicrobial Activity of Cytolytic T Cells Mediated by Granulysin. Science (1998) 282(5386):121–5. doi: 10.1126/science.282.5386.121
19. Roulston A, Marcellus RC, Branton PE. Viruses and Apoptosis. Annu Rev Microbiol (1999) 53:577–628. doi: 10.1146/annurev.micro.53.1.577
20. Marino J, Stoeckli I, Walch M, Latinovic-Golic S, Sundstroem H, Groscurth P, et al. Chlamydophila Pneumoniae Derived From Inclusions Late in the Infectious Cycle Induce Aponecrosis in Human Aortic Endothelial Cells. BMC Microbiol (2008) 8:32. doi: 10.1186/1471-2180-8-32
21. Byrne GI, Ojcius DM. Chlamydia and Apoptosis: Life and Death Decisions of an Intracellular Pathogen. Nat Rev Microbiol (2004) 2(10):802–8. doi: 10.1038/nrmicro1007
22. Fan T, Lu H, Hu H, Shi L, McClarty GA, Nance DM, et al. Inhibition of Apoptosis in Chlamydia-Infected Cells: Blockade of Mitochondrial Cytochrome C Release and Caspase Activation. J Exp Med (1998) 187(4):487–96. doi: 10.1084/jem.187.4.487
23. Rajalingam K, Sharma M, Lohmann C, Oswald M, Thieck O, Froelich CJ, et al. Mcl-1 Is a Key Regulator of Apoptosis Resistance in Chlamydia Trachomatis-Infected Cells. PloS One (2008) 3(9):e3102. doi: 10.1371/journal.pone.0003102
24. Kvansakul M, Hinds MG. Structural Biology of the Bcl-2 Family and Its Mimicry by Viral Proteins. Cell Death Disease (2013) 4(11):e909. doi: 10.1038/cddis.2013.436
25. Zamaraev AV, Zhivotovsky B, Kopeina GS. Viral Infections: Negative Regulators of Apoptosis and Oncogenic Factors. Biochemistry-Moscow (2020) 85(10):1191–201. doi: 10.1134/S0006297920100077
26. Masson D, Zamai M, Tschopp J. Identification of Granzyme A Isolated From Cytotoxic T-Lymphocyte-Granules as One of the Proteases Encoded by CTL-Specific Genes. FEBS Lett (1986) 208(1):84–8. doi: 10.1016/0014-5793(86)81537-X
27. Akula S, Thorpe M, Boinapally V, Hellman L. Granule Associated Serine Proteases of Hematopoietic Cells - An Analysis of Their Appearance and Diversification During Vertebrate Evolution. PloS One (2015) 10(11):e0143091. doi: 10.1371/journal.pone.0143091
28. Odake S, Kam CM, Narasimhan L, Poe M, Blake JT, Krahenbuhl O, et al. Human and Murine Cytotoxic T Lymphocyte Serine Proteases: Subsite Mapping With Peptide Thioester Substrates and Inhibition of Enzyme Activity and Cytolysis by Isocoumarins. Biochemistry (1991) 30(8):2217–27. doi: 10.1021/bi00222a027
29. Van Damme P, Maurer-Stroh S, Hao H, Colaert N, Timmerman E, Eisenhaber F, et al. The Substrate Specificity Profile of Human Granzyme a. Biol Chem (2010) 391(8):983–97. doi: 10.1515/BC.2010.096
30. Plasman K, Demol H, Bird PI, Gevaert K, Van Damme P. Substrate Specificities of the Granzyme Tryptases A and K. J Proteome Res (2014) 13(12):6067–77. doi: 10.1021/pr500968d
31. Rugles SW, Fletterick RJ, Craik CS. Characterization of Structural Determinants of Granzyme B Reveals Potent Mediators of Extended Substrate Specificity. J Biol Chem (2004) 279(29):30751–9. doi: 10.1074/jbc.M400949200
32. Wang L, Zhang K, Wu L, Liu S, Zhang H, Zhou Q, et al. Structural Insights Into the Substrate Specificity of Human Granzyme H: The Functional Roles of a Novel RKR Motif. J Immunol (2012) 188(2):765–73. doi: 10.4049/jimmunol.1101381
33. de Poot SA, Westgeest M, Hostetter DR, Van Damme P, Plasman K, Demeyer K, et al. Human and Mouse Granzyme M Display Divergent and Species-Specific Substrate Specificities. Biochem J (2011) 437(3):431–42. doi: 10.1042/BJ20110210
34. Elavazhagan S, Fatehchand K, Santhanam V, Fang H, Ren L, Gautam S, et al. Granzyme B Expression Is Enhanced in Human Monocytes by TLR8 Agonists and Contributes to Antibody-Dependent Cellular Cytotoxicity. J Immunol (2015) 194(6):2786–95. doi: 10.4049/jimmunol.1402316
35. Boivin WA, Cooper DM, Hiebert PR, Granville DJ. Intracellular Versus Extracellular Granzyme B in Immunity and Disease: Challenging the Dogma. Lab Invest (2009) 89(11):1195–220. doi: 10.1038/labinvest.2009.91
36. D’Eliseo D, Pisu P, Romano C, Tubaro A, De Nunzio C, Morrone S, et al. Granzyme B Is Expressed in Urothelial Carcinoma and Promotes Cancer Cell Invasion. Int J Cancer (2010) 127(6):1283–94. doi: 10.1002/ijc.25135
37. Pearson JD, Zhang J, Wu Z, Thew KD, Rowe KJ, Bacani JT, et al. Expression of Granzyme B Sensitizes ALK+ ALCL Tumour Cells to Apoptosis-Inducing Drugs. Mol Cancer (2014) 13:199. doi: 10.1186/1476-4598-13-199
38. Wang H, Sun Q, Wu Y, Wang L, Zhou C, Ma W, et al. Granzyme M Expressed by Tumor Cells Promotes Chemoresistance and EMT In Vitro and Metastasis In Vivo Associated With STAT3 Activation. Oncotarget (2015) 6(8):5818–31. doi: 10.18632/oncotarget.3461
39. Ettersperger J, Montcuquet N, Malamut G, Guegan N, Lopez-Lastra S, Gayraud S, et al. Interleukin-15-Dependent T-Cell-Like Innate Intraepithelial Lymphocytes Develop in the Intestine and Transform Into Lymphomas in Celiac Disease. Immunity (2016) 45(3):610–25. doi: 10.1016/j.immuni.2016.07.018
40. Hoek KL, Greer MJ, McClanahan KG, Nazmi A, Piazuelo MB, Singh K, et al. Granzyme B Prevents Aberrant IL-17 Production and Intestinal Pathogenicity in CD4(+) T Cells. Mucosal Immunol (2021) 14(5):1088–99. doi: 10.1038/s41385-021-00427-1
41. Pipkin ME, Rao A, Lichtenheld MG. The Transcriptional Control of the Perforin Locus. Immunol Rev (2010) 235(1):55–72. doi: 10.1111/j.0105-2896.2010.00905.x
42. Hiebert PR, Wu D, Granville DJ. Granzyme B Degrades Extracellular Matrix and Contributes to Delayed Wound Closure in Apolipoprotein E Knockout Mice. Cell Death Differ (2013) 20(10):1404-14. doi: 10.1038/cdd.2013.96
43. Hiebert PR, Granville DJ. Granzyme B in Injury, Inflammation, and Repair. Trends Mol Med (2012) 18(12):732–41. doi: 10.1016/j.molmed.2012.09.009
44. Omoto Y, Yamanaka K, Tokime K, Kitano S, Kakeda M, Akeda T, et al. Granzyme B Is a Novel Interleukin-18 Converting Enzyme. J Dermatol Sci (2010) 59(2):129–35. doi: 10.1016/j.jdermsci.2010.05.004
45. Boivin WA, Shackleford M, Vanden Hoek A, Zhao H, Hackett TL, Knight DA, et al. Granzyme B Cleaves Decorin, Biglycan and Soluble Betaglycan, Releasing Active Transforming Growth Factor-Beta1. PloS One (2012) 7(3):e33163. doi: 10.1371/journal.pone.0033163
46. Buzza MS, Zamurs L, Sun J, Bird CH, Smith AI, Trapani JA, et al. Extracellular Matrix Remodeling by Human Granzyme B via Cleavage of Vitronectin, Fibronectin, and Laminin. J Biol Chem (2005) 280(25):23549–58. doi: 10.1074/jbc.M412001200
47. van Domselaar R, de Poot SA, Bovenschen N. Proteomic Profiling of Proteases: Tools for Granzyme Degradomics. Expert Rev Proteomics (2010) 7(3):347–59. doi: 10.1586/epr.10.24
48. Martinvalet D, Thiery J, Chowdhury D. Granzymes and Cell Death. Methods Enzymol (2008) 442:213–30. doi: 10.1016/S0076-6879(08)01411-0
49. Shresta S, Heusel JW, Macivor DM, Wesselschmidt RL, Russell JH, Ley TJ. Granzyme B Plays a Critical Role in Cytotoxic Lymphocyte-Induced Apoptosis. Immunol Rev (1995) 146:211–21. doi: 10.1111/j.1600-065X.1995.tb00690.x
50. Greenberg AH. Activation of Apoptosis Pathways by Granzyme B. Cell Death Differ (1996) 3(3):269–74.
51. Medema JP, Toes RE, Scaffidi C, Zheng TS, Flavell RA, Melief CJ, et al. Cleavage of FLICE (Caspase-8) by Granzyme B During Cytotoxic T Lymphocyte-Induced Apoptosis. Eur J Immunol (1997) 27(12):3492–8. doi: 10.1002/eji.1830271250
52. Andrade F, Roy S, Nicholson D, Thornberry N, Rosen A, Casciola-Rosen L. Granzyme B Directly and Efficiently Cleaves Several Downstream Caspase Substrates: Implications for CTL-Induced Apoptosis. Immunity (1998) 8(4):451–60. doi: 10.1016/S1074-7613(00)80550-6
53. Martin SJ, Amarante-Mendes GP, Shi L, Chuang TH, Casiano CA, O’Brien GA, et al. The Cytotoxic Cell Protease Granzyme B Initiates Apoptosis in a Cell-Free System by Proteolytic Processing and Activation of the ICE/CED-3 Family Protease, CPP32, via a Novel Two-Step Mechanism. EMBO J (1996) 15(10):2407–16. doi: 10.1002/j.1460-2075.1996.tb00598.x
54. McIlroy D, Sakahira H, Talanian RV, Nagata S. Involvement of Caspase 3-Activated DNase in Internucleosomal DNA Cleavage Induced by Diverse Apoptotic Stimuli. Oncogene (1999) 18(31):4401–8. doi: 10.1038/sj.onc.1202868
55. Sutton VR, Davis JE, Cancilla M, Johnstone RW, Ruefli AA, Sedelies K, et al. Initiation of Apoptosis by Granzyme B Requires Direct Cleavage of Bid, But Not Direct Granzyme B-Mediated Caspase Activation. J Exp Med (2000) 192(10):1403–14. doi: 10.1084/jem.192.10.1403
56. Casciola-Rosen L, Garcia-Calvo M, Bull HG, Becker JW, Hines T, Thornberry NA, et al. Mouse and Human Granzyme B Have Distinct Tetrapeptide Specificities and Abilities to Recruit the Bid Pathway. J Biol Chem (2007) 282(7):4545–52. doi: 10.1074/jbc.M606564200
57. Waterhouse NJ, Sedelies KA, Browne KA, Wowk ME, Newbold A, Sutton VR, et al. A Central Role for Bid in Granzyme B-Induced Apoptosis. J Biol Chem (2005) 280(6):4476–82. doi: 10.1074/jbc.M410985200
58. Goping IS, Barry M, Liston P, Sawchuk T, Constantinescu G, Michalak KM, et al. Granzyme B-Induced Apoptosis Requires Both Direct Caspase Activation and Relief of Caspase Inhibition. Immunity (2003) 18(3):355–65. doi: 10.1016/S1074-7613(03)00032-3
59. Sutton VR, Wowk ME, Cancilla M, Trapani JA. Caspase Activation by Granzyme B Is Indirect, and Caspase Autoprocessing Requires the Release of Proapoptotic Mitochondrial Factors. Immunity (2003) 18(3):319–29. doi: 10.1016/S1074-7613(03)00050-5
60. Waterhouse NJ, Sedelies KA, Sutton VR, Pinkoski MJ, Thia KY, Johnstone R, et al. Functional Dissociation of DeltaPsim and Cytochrome C Release Defines the Contribution of Mitochondria Upstream of Caspase Activation During Granzyme B-Induced Apoptosis. Cell Death Differ (2006) 13(4):607–18. doi: 10.1038/sj.cdd.4401772
61. Ekert PG, Silke J, Hawkins CJ, Verhagen AM, Vaux DL. DIABLO Promotes Apoptosis by Removing MIHA/XIAP From Processed Caspase 9. J Cell Biol (2001) 152(3):483–90. doi: 10.1083/jcb.152.3.483
62. Fan Z, Beresford PJ, Oh DY, Zhang D, Lieberman J. Tumor Suppressor NM23-H1 Is a Granzyme A-Activated DNase During CTL-Mediated Apoptosis, and the Nucleosome Assembly Protein SET Is Its Inhibitor. Cell (2003) 112(5):659–72. doi: 10.1016/S0092-8674(03)00150-8
63. Chowdhury D, Beresford PJ, Zhu P, Zhang D, Sung JS, Demple B, et al. The Exonuclease TREX1 Is in the SET Complex and Acts in Concert With NM23-H1 to Degrade DNA During Granzyme A-Mediated Cell Death. Mol Cell (2006) 23(1):133–42. doi: 10.1016/j.molcel.2006.06.005
64. Beresford PJ, Xia Z, Greenberg AH, Lieberman J. Granzyme A Loading Induces Rapid Cytolysis and a Novel Form of DNA Damage Independently of Caspase Activation. Immunity (1999) 10(5):585–94. doi: 10.1016/S1074-7613(00)80058-8
65. Chowdhury D, Lieberman J. Death by a Thousand Cuts: Granzyme Pathways of Programmed Cell Death. Annu Rev Immunol (2008) 26:389–420. doi: 10.1146/annurev.immunol.26.021607.090404
66. Zhu P, Zhang D, Chowdhury D, Martinvalet D, Keefe D, Shi L, et al. Which Causes Single-Stranded DNA Damage, Targets the Double-Strand Break Repair Protein Ku70. EMBO Rep (2006) 7(4):431–7. doi: 10.1038/sj.embor.7400622
67. Zhang D, Beresford PJ, Greenberg AH, Lieberman J. Granzymes A and B Directly Cleave Lamins and Disrupt the Nuclear Lamina During Granule-Mediated Cytolysis. Proc Natl Acad Sci USA (2001) 98(10):5746–51. doi: 10.1073/pnas.101329598
68. Rajani DK, Walch M, Martinvalet D, Thomas MP, Lieberman J. Alterations in RNA Processing During Immune-Mediated Programmed Cell Death. Proc Natl Acad Sci USA (2012) 109(22):8688–93. doi: 10.1073/pnas.1201327109
69. Thomas MP, Whangbo J, McCrossan G, Deutsch AJ, Martinod K, Walch M, et al. Leukocyte Protease Binding to Nucleic Acids Promotes Nuclear Localization and Cleavage of Nucleic Acid Binding Proteins. J Immunol (2014) 192(11):5390–7. doi: 10.4049/jimmunol.1303296
70. Froelich CJ, Hanna WL, Poirier GG, Duriez PJ, D’Amours D, Salvesen GS, et al. Granzyme B/perforin-Mediated Apoptosis of Jurkat Cells Results in Cleavage of Poly(ADP-Ribose) Polymerase to the 89-kDa Apoptotic Fragment and Less Abundant 64-kDa Fragment. Biochem Biophys Res Commun (1996) 227(3):658–65. doi: 10.1006/bbrc.1996.1565
71. Jans DA, Jans P, Briggs LJ, Sutton V, Trapani JA. Nuclear Transport of Granzyme B (Fragmentin-2). Dependence of Perforin In Vivo and Cytosolic Factors In Vitro. J Biol Chem (1996) 271(48):30781–9. doi: 10.1074/jbc.271.48.30781
72. Zhang D, Pasternack MS, Beresford PJ, Wagner L, Greenberg AH, Lieberman J. Induction of Rapid Histone Degradation by the Cytotoxic T Lymphocyte Protease Granzyme a. J Biol Chem (2001) 276(5):3683–90. doi: 10.1074/jbc.M005390200
73. Bovenschen N, Kummer JA. Orphan Granzymes Find a Home. Immunol Rev (2010) 235(1):117–27. doi: 10.1111/j.0105-2896.2010.00889.x
74. Bouwman AC, van Daalen KR, Crnko S, ten Broeke T, Bovenschen N. Intracellular and Extracellular Roles of Granzyme K. Front Immunol (2021) 12. doi: 10.3389/fimmu.2021.677707
75. Anthony DA, Andrews DM, Chow M, Watt SV, House C, Akira S, et al. A Role for Granzyme M in TLR4-Driven Inflammation and Endotoxicosis. J Immunol (2010) 185(3):1794–803. doi: 10.4049/jimmunol.1000430
76. Joeckel LT, Wallich R, Martin P, Sanchez-Martinez D, Weber FC, Martin SF, et al. Mouse Granzyme K Has Pro-Inflammatory Potential. Cell Death Differ (2011) 18(7):1112–9. doi: 10.1038/cdd.2011.5
77. Spencer CT, Abate G, Sakala IG, Xia M, Truscott SM, Eickhoff CS, et al. Granzyme A Produced by Gamma(9)Delta(2) T Cells Induces Human Macrophages to Inhibit Growth of an Intracellular Pathogen. PloS Pathog (2013) 9(1):e1003119. doi: 10.1371/journal.ppat.1003119
78. Rasi V, Wood D, Eickhoff C, Xia M, Edwards R, Walch M, et al. Granzyme A Produced by γ9δ2 T Cells Activates ER Stress Responses and ATP Production, and Protects Against Intracellular Mycobacterial Replication Independent of Enzymatic Activity. Front Immunol (2021) 12:712678. doi: 10.3389/fimmu.2021.712678
79. Martinvalet D, Dykxhoorn DM, Ferrini R, Lieberman J. Granzyme A Cleaves a Mitochondrial Complex I Protein to Initiate Caspase-Independent Cell Death. Cell (2008) 133(4):681–92. doi: 10.1016/j.cell.2008.03.032
80. Jacquemin G, Margiotta D, Kasahara A, Bassoy EY, Walch M, Thiery J, et al. Granzyme B-Induced Mitochondrial ROS Are Required for Apoptosis. Cell Death Differ (2015) 22(5):862–74. doi: 10.1038/cdd.2014.180
81. Lionello S, Marzaro G, Martinvalet D. SAM50, a Side Door to the Mitochondria: The Case of Cytotoxic Proteases. Pharmacol Res (2020) 160:105196. doi: 10.1016/j.phrs.2020.105196
82. Ricci JE, Munoz-Pinedo C, Fitzgerald P, Bailly-Maitre B, Perkins GA, Yadava N, et al. Disruption of Mitochondrial Function During Apoptosis Is Mediated by Caspase Cleavage of the P75 Subunit of Complex I of the Electron Transport Chain. Cell (2004) 117(6):773–86. doi: 10.1016/j.cell.2004.05.008
83. Ricci JE, Waterhouse N, Green DR. Mitochondrial Functions During Cell Death, a Complex (I-V) Dilemma. Cell Death Differ (2003) 10(5):488–92. doi: 10.1038/sj.cdd.4401225
84. Ricci JE, Gottlieb RA, Green DR. Caspase-Mediated Loss of Mitochondrial Function and Generation of Reactive Oxygen Species During Apoptosis. J Cell Biol (2003) 160(1):65–75. doi: 10.1083/jcb.200208089
85. Chiusolo V, Jacquemin G, Yonca Bassoy E, Vinet L, Liguori L, Walch M, et al. Granzyme B Enters the Mitochondria in a Sam50-, Tim22- and Mthsp70-Dependent Manner to Induce Apoptosis. Cell Death Differ (2017) 24(4):747–58. doi: 10.1038/cdd.2017.3
86. Aguilo JI, Anel A, Catalan E, Sebastian A, Acin-Perez R, Naval J, et al. Granzyme B of Cytotoxic T Cells Induces Extramitochondrial Reactive Oxygen Species Production via Caspase-Dependent NADPH Oxidase Activation. Immunol Cell Biol (2010) 88(5):545–54. doi: 10.1038/icb.2010.5
87. Barber GN. Host Defense, Viruses and Apoptosis. Cell Death Differ (2001) 8(2):113–26. doi: 10.1038/sj.cdd.4400823
88. Nishikawa Y, Makala L, Otsuka H, Mikami T, Nagasawa H. Mechanisms of Apoptosis in Murine Fibroblasts by Two Intracellular Protozoan Parasites, Toxoplasma Gondii and Neospora Caninum. Parasite Immunol (2002) 24(7):347–54. doi: 10.1046/j.1365-3024.2002.00476.x
89. Labbe K, Saleh M. Cell Death in the Host Response to Infection. Cell Death Different (2008) 15(9):1339–49. doi: 10.1038/cdd.2008.91
90. Orzalli MH, Kagan JC. Apoptosis and Necroptosis as Host Defense Strategies to Prevent Viral Infection. Trends Cell Biol (2017) 27(11):810–9. doi: 10.1016/j.tcb.2017.05.007
91. Zychlinsky A, Sansonetti P. Apoptosis in Bacterial Pathogenesis. J Clin Invest (1997) 100(12):S63–S5. doi: 10.1172/JCI119557
92. DosReis GA, Barcinski MA. Apoptosis and Parasitism: From the Parasite to the Host Immune Response. Adv Parasitol Vol 49 (2001) 49:133–61. doi: 10.1016/S0065-308X(01)49039-7
93. Thakur A, Mikkelsen H, Jungersen G. Intracellular Pathogens: Host Immunity and Microbial Persistence Strategies. J Immunol Res (2019) 2019:1356540. doi: 10.1155/2019/1356540
94. Heussler VT, Kuenzi P, Rottenberg S. Inhibition of Apoptosis by Intracellular Protozoan Parasites. Int J Parasitol (2001) 31(11):1166–76. doi: 10.1016/S0020-7519(01)00271-5
95. Faherty CS, Maurelli AT. Staying Alive: Bacterial Inhibition of Apoptosis During Infection. Trends Microbiol (2008) 16(4):173–80. doi: 10.1016/j.tim.2008.02.001
96. Behar SM, Briken V. Apoptosis Inhibition by Intracellular Bacteria and Its Consequence on Host Immunity. Curr Opin Immunol (2019) 60:103–10. doi: 10.1016/j.coi.2019.05.007
97. Kaminskyy V, Zhivotovsky B. To Kill or Be Killed: How Viruses Interact With the Cell Death Machinery. J Internal Med (2010) 267(5):473–82. doi: 10.1111/j.1365-2796.2010.02222.x
98. Turner PC, Sancho MC, Thoennes SR, Caputo A, Bleackley RC, Moyer RW. Myxoma Virus Serp2 Is a Weak Inhibitor of Granzyme B and Interleukin-1beta-Converting Enzyme In Vitro and Unlike CrmA Cannot Block Apoptosis in Cowpox Virus-Infected Cells. J Virol (1999) 73(8):6394–404. doi: 10.1128/JVI.73.8.6394-6404.1999
99. Boyd JM. Adenovirus-E1b 19-Kda and Bcl-2 Proteins Interact With a Common Set of Cellular Proteins. Cell (1994) 79(2):341–51. doi: 10.1016/0092-8674(94)90202-X
100. Zhou Q, Snipas S, Orth K, Muzio M, Dixit VM, Salvesen GS. Target Protease Specificity of the Viral Serpin CrmA - Analysis of Five Caspases. J Biol Chem (1997) 272(12):7797–800. doi: 10.1074/jbc.272.12.7797
101. Skaletskaya A, Bartle LM, Chittenden T, McCormick AL, Mocarski ES, Goldmacher VS. A Cytomegalovirus-Encoded Inhibitor of Apoptosis That Suppresses Caspase-8 Activation. Proc Natl Acad Sci USA (2001) 98(14):7829–34. doi: 10.1073/pnas.141108798
102. Simon HG, Fruth U, Kramer MD, Simon MM. A Secretable Serine Proteinase With Highly Restricted Specificity From Cytolytic Lymphocytes-T Inactivates Retrovirus-Associated Reverse-Transcriptase. FEBS Lett (1987) 223(2):352–60. doi: 10.1016/0014-5793(87)80318-6
103. Andrade F, Fellows E, Jenne DE, Rosen A, Young CS. Granzyme H Destroys the Function of Critical Adenoviral Proteins Required for Viral DNA Replication and Granzyme B Inhibition. EMBO J (2007) 26(8):2148–57. doi: 10.1038/sj.emboj.7601650
104. Andrade F, Bull HG, Thornberry NA, Ketner GW, Casciola-Rosen LA, Rosen A. Adenovirus L4-100K Assembly Protein Is a Granzyme B Substrate That Potently Inhibits Granzyme B-Mediated Cell Death. Immunity (2001) 14(6):751–61. doi: 10.1016/S1074-7613(01)00149-2
105. van Domselaar R, Philippen LE, Quadir R, Wiertz EJ, Kummer JA, Bovenschen N. Noncytotoxic Inhibition of Cytomegalovirus Replication Through NK Cell Protease Granzyme M-Mediated Cleavage of Viral Phosphoprotein 71. J Immunol (2010) 185(12):7605–13. doi: 10.4049/jimmunol.1001503
106. Shan LL, Li S, Meeldijk J, Blijenberg B, Hendriks A, van Boxtel KJWM, et al. Killer Cell Proteases can Target Viral Immediate-Early Proteins to Control Human Cytomegalovirus Infection in a Noncytotoxic Manner. PloS Pathog (2020) 16(4):e1008426. doi: 10.1371/journal.ppat.1008426
107. van Domselaar R, de Poot SA, Remmerswaal EB, Lai KW, ten Berge IJ, Bovenschen N. Granzyme M Targets Host Cell hnRNP K That Is Essential for Human Cytomegalovirus Replication. Cell Death Differ (2013) 20(3):419–29. doi: 10.1038/cdd.2012.132
108. Pereira RA, Simon MM, Simmons A. Granzyme A, a Noncytolytic Component of CD8(+) Cell Granules, Restricts the Spread of Herpes Simplex Virus in the Peripheral Nervous Systems of Experimentally Infected Mice. J Virol (2000) 74(2):1029–32. doi: 10.1128/JVI.74.2.1029-1032.2000
109. Knickelbein JE, Khanna KM, Yee MB, Baty CJ, Kinchington PR, Hendricks RL. Noncytotoxic Lytic Granule-Mediated CD8+ T Cell Inhibition of HSV-1 Reactivation From Neuronal Latency. Science (2008) 322(5899):268–71. doi: 10.1126/science.1164164
110. Gerada C, Steain M, Campbell TM, McSharry B, Slobedman B, Abendroth A. Granzyme B Cleaves Multiple Herpes Simplex Virus 1 and Varicella-Zoster Virus (VZV) Gene Products, and VZV ORF4 Inhibits Natural Killer Cell Cytotoxicity. J Virol (2019) 93(22):e01140-19. doi: 10.1128/JVI.01140-19
111. Marcet-Palacios M, Duggan BL, Shostak I, Barry M, Geskes T, Wilkins JA, et al. Granzyme B Inhibits Vaccinia Virus Production Through Proteolytic Cleavage of Eukaryotic Initiation Factor 4 Gamma 3. PloS Pathog (2011) 7(12):e1002447. doi: 10.1371/journal.ppat.1002447
112. Romero V, Fellows E, Jenne DE, Andrade F. Cleavage of La Protein by Granzyme H Induces Cytoplasmic Translocation and Interferes With La-Mediated HCV-IRES Translational Activity. Cell Death Differ (2009) 16(2):340–8. doi: 10.1038/cdd.2008.165
113. Tang H, Li C, Wang L, Zhang H, Fan Z. Granzyme H of Cytotoxic Lymphocytes Is Required for Clearance of the Hepatitis B Virus Through Cleavage of the Hepatitis B Virus X Protein. J Immunol (2012) 188(2):824–31. doi: 10.4049/jimmunol.1102205
114. Zhong C, Li C, Wang X, Toyoda T, Gao G, Fan Z. Granzyme K Inhibits Replication of Influenza Virus Through Cleaving the Nuclear Transport Complex Importin Alpha1/Beta Dimer of Infected Host Cells. Cell Death Differ (2012) 19(5):882–90. doi: 10.1038/cdd.2011.178
115. Walch M, Rampini SK, Stoeckli I, Latinovic-Golic S, Dumrese C, Sundstrom H, et al. Involvement of CD252 (CD134L) and IL-2 in the Expression of Cytotoxic Proteins in Bacterial- or Viral-Activated Human T Cells. J Immunol (2009) 182(12):7569–79. doi: 10.4049/jimmunol.0800296
116. Lauw FN, Simpson AJ, Hack CE, Prins JM, Wolbink AM, van Deventer SJ, et al. Soluble Granzymes Are Released During Human Endotoxemia and in Patients With Severe Infection Due to Gram-Negative Bacteria. J Infect Dis (2000) 182(1):206–13. doi: 10.1086/315642
117. Leon DL, Fellay I, Mantel PY, Walch M. Killing Bacteria With Cytotoxic Effector Proteins of Human Killer Immune Cells: Granzymes, Granulysin, and Perforin. Methods Mol Biol (2017) 1535:275–84. doi: 10.1007/978-1-4939-6673-8_18
118. Walch M, Dotiwala F, Mulik S, Thiery J, Kirchhausen T, Clayberger C, et al. Cytotoxic Cells Kill Intracellular Bacteria Through Granulysin-Mediated Delivery of Granzymes. Cell (2014) 157(6):1309–23. doi: 10.1016/j.cell.2014.03.062
119. Boulouis C, Sia WR, Gulam MY, Teo JQM, Png YT, Phan TK, et al. Human MAIT Cell Cytolytic Effector Proteins Synergize to Overcome Carbapenem Resistance in Escherichia Coli. PloS Biol (2020) 18(6):e3000644. doi: 10.1371/journal.pbio.3000644
120. Leeansyah E, Boulouis C, Kwa ALH, Sandberg JK. Emerging Role for MAIT Cells in Control of Antimicrobial Resistance. Trends Microbiol (2021) 29(6):504–16. doi: 10.1016/j.tim.2020.11.008
121. Narni-Mancinelli E, Vivier E. Delivering Three Punches to Knockout Intracellular Bacteria. Cell (2014) 157(6):1251–2. doi: 10.1016/j.cell.2014.05.023
122. Dotiwala F, Fellay I, Filgueira L, Martinvalet D, Lieberman J, Walch M. A High Yield and Cost-Efficient Expression System of Human Granzymes in Mammalian Cells. JoVE (2015) 100:e52911. doi: 10.3791/52911
123. Dotiwala F, Sen Santara S, Binker-Cosen AA, Li B, Chandrasekaran S, Lieberman J. Granzyme B Disrupts Central Metabolism and Protein Synthesis in Bacteria to Promote an Immune Cell Death Program. Cell (2017) 171(5):1125–37.e11. doi: 10.1016/j.cell.2017.10.004
124. Leon DL, Matthey P, Fellay I, Blanchard M, Martinvalet D, Mantel PY, et al. Granzyme B Attenuates Bacterial Virulence by Targeting Secreted Factors. iScience (2020) 23(3):100932. doi: 10.1016/j.isci.2020.100932
125. Hernández-Castañeda MA, Happ K, Cattalani F, Wallimann A, Blanchard M, Fellay I, et al. γδ T Cells Kill Plasmodium Falciparum in a Granzyme- and Granulysin-Dependent Mechanism During the Late Blood Stage. J Immunol (2020) 204(7):1798–809. doi: 10.4049/jimmunol.1900725
126. Junqueira C, Polidoro RB, Castro G, Absalon S, Liang ZT, Sen Santara S, et al. Gamma Delta T Cells Suppress Plasmodium Falciparum Blood-Stage Infection by Direct Killing and Phagocytosis. Nat Immunol (2021) 22(3):347–57. doi: 10.1038/s41590-020-00847-4
127. Hernandez-Castaneda MA, Lavergne M, Casanova P, Nydegger B, Merten C, Subramanian BY, et al. A Profound Membrane Reorganization Defines Susceptibility of Plasmodium Falciparum Infected Red Blood Cells to Lysis by Granulysin and Perforin. Front Immunol (2021) 12:643746. doi: 10.3389/fimmu.2021.643746
Keywords: granzymes, ROS - reactive oxygen species, caspases, antimicrobial defense, apoptosis, mitochondria
Citation: Lavergne M, Hernández-Castañeda MA, Mantel P-Y, Martinvalet D and Walch M (2021) Oxidative and Non-Oxidative Antimicrobial Activities of the Granzymes. Front. Immunol. 12:750512. doi: 10.3389/fimmu.2021.750512
Received: 30 July 2021; Accepted: 23 September 2021;
Published: 11 October 2021.
Edited by:
Johan Van Der Vlag, Radboud University Nijmegen, NetherlandsReviewed by:
Danyvid Olivares-Villagómez, Vanderbilt University Medical Center, United StatesJohan K. Sandberg, Karolinska Institutet (KI), Sweden
Copyright © 2021 Lavergne, Hernández-Castañeda, Mantel, Martinvalet and Walch. This is an open-access article distributed under the terms of the Creative Commons Attribution License (CC BY). The use, distribution or reproduction in other forums is permitted, provided the original author(s) and the copyright owner(s) are credited and that the original publication in this journal is cited, in accordance with accepted academic practice. No use, distribution or reproduction is permitted which does not comply with these terms.
*Correspondence: Michael Walch, bWljaGFlbC53YWxjaEB1bmlmci5jaA==