- 1Departments of Pathology and Microbiology, Wexner Medical Center, The Ohio State University, Columbus, OH, United States
- 2Laboratory of Emerging Pathogens, Division of Emerging and Transfusion Transmitted Diseases, Center for Biologics Evaluation and Research, Food and Drug Administration, Silver Spring, MD, United States
Leishmaniasis is endemic to the tropical and subtropical regions of the world and is transmitted by the bite of an infected sand fly. The multifaceted interactions between Leishmania, the host innate immune cells, and the adaptive immunity determine the severity of pathogenesis and disease development. Leishmania parasites establish a chronic infection by subversion and attenuation of the microbicidal functions of phagocytic innate immune cells such as neutrophils, macrophages and dendritic cells (DCs). Other innate cells such as inflammatory monocytes, mast cells and NK cells, also contribute to resistance and/or susceptibility to Leishmania infection. In addition to the cytokine/chemokine signals from the innate immune cells, recent studies identified the subtle shifts in the metabolic pathways of the innate cells that activate distinct immune signal cascades. The nexus between metabolic pathways, epigenetic reprogramming and the immune signaling cascades that drive the divergent innate immune responses, remains to be fully understood in Leishmania pathogenesis. Further, development of safe and efficacious vaccines against Leishmaniasis requires a broader understanding of the early interactions between the parasites and innate immune cells. In this review we focus on the current understanding of the specific role of innate immune cells, the metabolomic and epigenetic reprogramming and immune regulation that occurs during visceral leishmaniasis, and the strategies used by the parasite to evade and modulate host immunity. We highlight how such pathways could be exploited in the development of safe and efficacious Leishmania vaccines.
Introduction
Protozoan parasites of the genus Leishmania are the causative agents of leishmaniasis, a spectrum of vector-borne neglected diseases affecting over 12 million people worldwide with growing geographical extension (1, 2). The clinical manifestations of leishmaniasis differ widely, depending principally on the causative species. An estimated 20 different Leishmania spp. cause the three main clinical disease manifestations: cutaneous, mucocutaneous, and visceral leishmaniasis (3). Among these, the visceral form is fatal if not treated. Most cutaneous leishmaniasis (CL) and visceral leishmaniasis (VL) patients develop long-term protective immunity after cure from the infection, indicating the feasibility of developing an effective prophylactic strategy against leishmaniasis (4, 5). However, no vaccine is currently available against human leishmaniasis.
Leishmania is a digenetic parasite, whose life cycle includes two hosts: the insect vector, and a vertebrate host. Following the bite of an infected sand fly, the obligate intracellular parasite Leishmania rapidly infects its host cells (2, 6). A better understanding of the spectrum of immune responses following infection with Leishmania parasites is required to develop more effective preventative approaches, as the host immune response is a key determinant of the outcome of leishmaniasis (7). Following inoculation, innate cells such as neutrophils, inflammatory monocytes, macrophages, dendritic cells (DCs), mast cells, and natural killer (NK) cells create either a permissive or hostile environment for the parasite through their effector functions, and subsequently modulate the adaptive immune responses towards host susceptibility or resistance. Although the adaptive branch of the immune response is crucial to control leishmaniasis, it has been extensively reviewed elsewhere and it is out of the scope of this review. Specifically, we focus on the innate immune responses in visceral leishmaniasis due to its significant mortality (7, 8).
Leishmania parasites have evolved highly successful strategies to evade the microbicidal activity of neutrophils, to prime infected macrophages towards an anti-inflammatory/alternative phenotype, and to undermine the Th1 polarizing functions of DCs, thereby attenuating the host protective adaptive immune responses (9–11). These altered immunological characteristics of the host cells upon infection are often induced by changes in the host metabolic pathways. Thus, there has been an emerging interest in profiling host metabolomics in the context of immune regulation, and especially to understand how metabolites and metabolic processes such as oxidative metabolism, glycolysis, and glutaminolysis can influence immune cell proliferation, differentiation and effector functions (12, 13). For instance, in pathogen-associated molecular pattern (PAMP)-activated neutrophils, mast cells, and DCs, glycolysis acts as a metabolic effector response that fuels reactive oxygen species (ROS) production, degranulation, and antigen presentation, respectively (13). Furthermore, changes in the metabolomic profile of infected immune cells can alter nutrient availability to the parasite, rendering the intracellular environment either permissive or hostile thus determining the anti-leishmanial activities (14). Leishmania parasites can also alter the metabolic pathways of the host cell to their advantage, for example by influencing nutrient uptake (15). It is hypothesized these metabolomic alterations can protect the parasite from elevated temperatures, low pH, and ROS in the parasitophorous vacuole (PV), leading to enhanced survival inside the host cell (15).
Increasing evidence points to a crucial role of innate immune cells in determining the outcome of the infection. This review particularly attempts to highlight the role of the innate immune cells, the metabolomic and epigenetic reprogramming, and immune regulation during VL, and discuss their implications with respect to the development of a vaccine against VL.
Innate Cells and Their Role in VL
Neutrophils
Role of Neutrophils in VL Pathogenesis
Neutrophils are the first innate immune cells recruited to the dermal site of Leishmania infection in response to several factors derived either from the host, the sand fly, or the parasite itself (16, 17) (Figure 1). Neutrophils’ anti-parasitic activities include phagocytosis, formation of neutrophil extracellular traps (NETs), as well as the release of reactive species (RNS and ROS) and granule-derived toxic compounds in the local environment or into the phagosome (18–23) (Figure 1). Additionally, neutrophils actively participate in modulating the adaptive immune responses. Specifically, several cytokines and granule proteins secreted by neutrophils such as interleukin (IL)-12, interferon (IFN)-γ, tumor necrosis factor (TNF)-α, and neutrophil elastase can induce T cell activation (24–27). Alternatively, IL-10, transforming growth factor (TGF)-β (Figure 1), and eicosanoids (thromboxane A2) from neutrophils suppress T cell activity (24–26, 28).
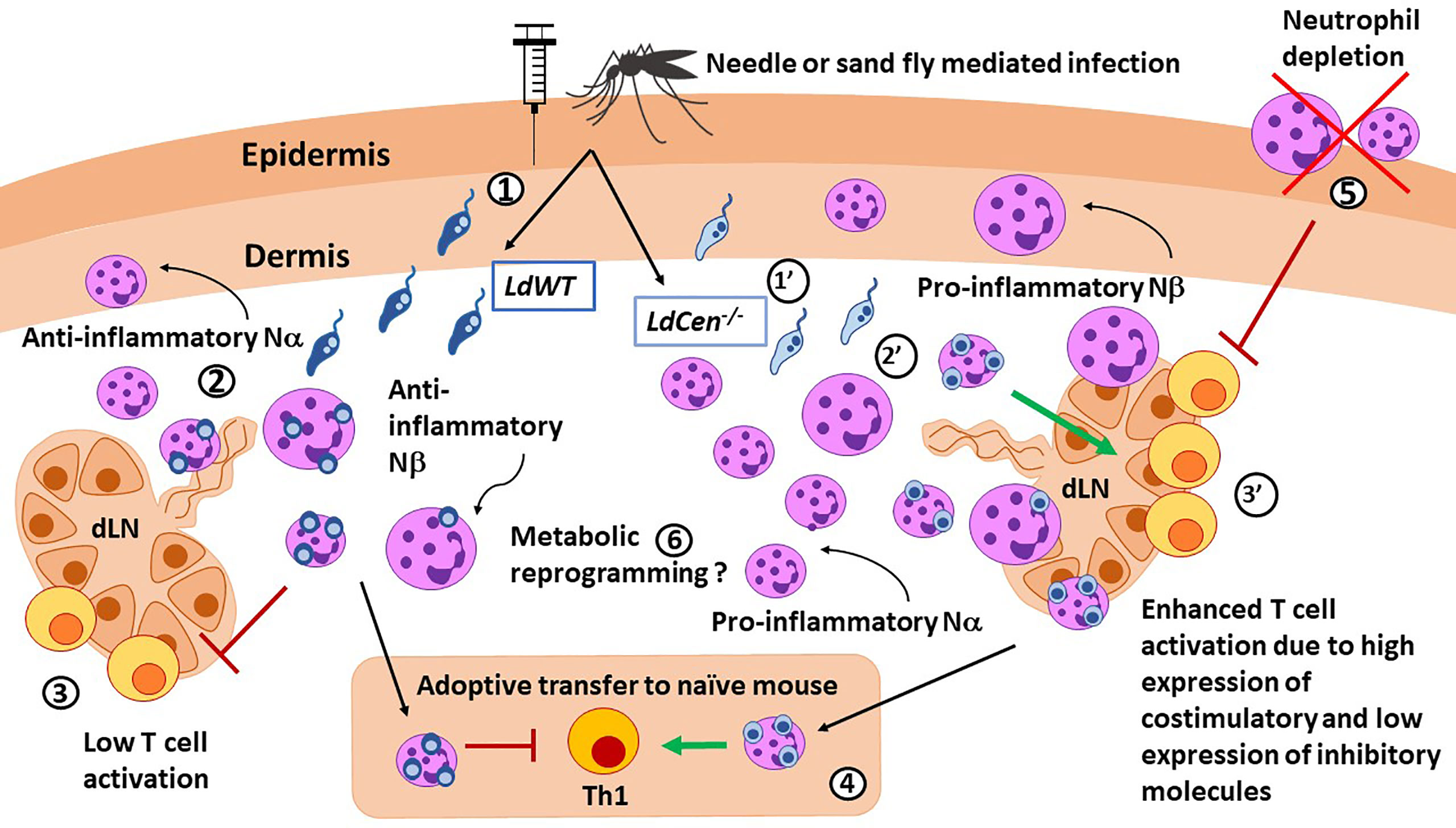
Figure 1 Role of neutrophils in immunity against Leishmania parasites. LdWT (1) or Ldcen-/- (1’) parasites are injected with a needle or by the sand fly vector. Compared with LdWT infection (2), LdCen−/− (2’) parasites induce higher recruitment of neutrophils, especially the smaller Nα subtype, to the ear dermis and ear draining lymph nodes (dLN), which were predominantly proinflammatory in nature. Similar Nα and Nβ subtypes were reported in live attenuated HSV vaccine studies. Neutrophils from ear dLN of LdCen−/−-immunized (3’) mice exhibited heightened expression of costimulatory molecules and attenuated expression of coinhibitory molecules necessary for higher T cell activation compared to LdWT (3). 4) Adoptive transfer of neutrophils bearing LdCen−/− parasites induced an increased Th1 response in naive mice compared to LdWT. 5) Neutrophil depletion significantly abrogated Ag-specific CD4+ T cell proliferation in LdCen−/−-immunized mice and impaired protection against virulent challenge. Conversely, replenishing of neutrophils significantly restored the LdCen−/−-induced host-protective response. 6) Neutrophils can undergo metabolic reprogramming to acquire trained immunity characteristics as shown in BCG vaccination studies.
The divergent immune responses observed in neutrophils may be induced by changes in the metabolism of Leishmania infected cells (27). For instance, L. donovani-infected neutrophils undergo a rapid upregulation of glycolytic enzymes at 6 hours post infection. Inhibition of glycolysis prior to infection led to significantly higher parasitic burdens in neutrophils, by inhibiting the generation of ROS production due to reduced NADPH oxidase activity (13, 27). Metabolic reprogramming in neutrophils may affect other functions in addition to microbicidal activities and remains an area of active investigations in Leishmania and other pathogenic agents.
Neutrophils have been shown to play a protective role during L. donovani infection (11). The rapid recruitment of neutrophils during L. donovani infection promotes resistance via the induction of an IFN-γ–dominant Th1 response in mice (29), and canine models of VL (30). Different visceralizing species of Leishmania can differentially affect neutrophil activities. For example, L. donovani infection can mediate recruitment, survival, and deactivation of neutrophils via the lipoxin A4 receptor (16, 31). Conversely, L. infantum interaction with the lipoxin A4 receptor promoted the activation of neutrophils characterized by an increase in NET formation (32). These divergent neutrophil roles due to different Leishmania species were identified by manipulating the neutrophils in animal models by treatment with neutrophil depleting antibodies that may have certain limitations (17, 18, 31). In addition to metabolite receptors such as lipoxin A4, vector saliva derived factors such as sialogenins and yellow salivary protein may also contribute to the diverse neutrophil-specific responses including FasL-mediated apoptosis in different experimental models (33, 34). Due to the important role played by neutrophils in early immunity, these populations have been studied in human VL as summarized in the following sections.
Role of Neutrophil Interactions With Other Immune Cells in Leishmania Immune Response
Neutrophils, in addition to their independent role in orchestrating the early cytokine/chemokine responses, also interact with other immune cell types including DCs, macrophages, NK cells, B cells, and T cells (35). Since macrophages are the principal host cells for Leishmania parasites, the crosstalk between neutrophils and infected macrophages has been studied in VL. The interaction between neutrophils and other immune cells has been shown to affect Leishmania infection and innate immune functions upon acquiring parasitized neutrophils (36–40). The species of Leishmania parasites, the host genetic background, and the apoptotic or necrotic nature of the neutrophils determine parasite transfer from neutrophils to macrophages, and the subsequent outcome of their interaction with macrophages (23, 37). The crosstalk between neutrophils and macrophages/DCs in VL remains to be studied (41), although it is known that when human macrophages are co-cultured with neutrophils previously infected with L. infantum in the presence of salivary gland sonicate (SGS), they acquired significantly more parasites from neutrophils. These macrophages also produced more TGF‐β and prostaglandin E2 (PGE2), suggesting the importance of neutrophil-macrophage crosstalk (42). Parasitized neutrophils may facilitate the uptake by DCs via the expression of apoptotic markers that lead to decreased DC activation and impaired cross-presentation for CD8+ T cell activation. Although CD11b on neutrophils may interact with DC‐SIGN of DCs and induce secretion of TNF‐α, such interaction remains to be demonstrated in VL (41). A predominant immunoregulatory role for neutrophils in regulating DC functions and subsequent T cell differentiation via DCs during Leishmania infection has also been reported (16, 41). The role of alarmins released during degranulation of neutrophils and their role in altering DC functions remains to be studied in VL (43).
Phenotypic Heterogeneity of Neutrophils
Heterogenous subsets of neutrophils identified based on size and granularity have been observed to perform either pathogenic or protective roles in autoimmune diseases, tumors, viral diseases and vaccines (44–46). Accordingly, Nα and Nβ neutrophil sub-populations have been described previously in the context of murine vaccination with attenuated New York vaccinia virus (NYVAC-C3). In this paper, Pilato, et al. reported higher expression of activation markers (CD11c, CD80, and CD86) in Nβ neutrophils. Compared to Nα cells, Nβ had greater capacity to induce antigen-specific CD8+ T-cell activation against the virus (46). Similar studies elucidating the functional roles of distinct neutrophil subsets revealed diverse roles of neutrophils in VL. Characterization of recruited dermal neutrophils in C57BL/6 mice during VL revealed the presence of heterogeneous Nα and Nβ neutrophil populations; Nα being the predominant population susceptible to infection (47). Further, studies in human VL identified the presence of a subset of HLA-DR+ low density circulating neutrophils that expressed elevated levels of arginase-1 and IL-10. These neutrophils failed to stimulate autologous T cell proliferation and promoted T cell exhaustion due to higher PD-L1 on their surface and elevated PD-1 expression by lymphocytes (48). The divergent functional specialization of neutrophil subsets observed in VL suggests that their role in other Leishmania infections warrants further studies.
Neutrophils and Their Role in Vaccine Induced Immunity
In addition to the innate immune functions either independently or through crosstalk between macrophages and DCs illustrated in the previous section in the context of VL, neutrophils have been shown to directly present antigens and promote T cell activation (49–51). Due to their capacity to perform antigen presentation to naïve T cells, similarly to other APCs, neutrophils may be particularly relevant in vaccine immunity. Indeed, a neutrophil-mediated T cell priming response was illustrated in numerous vaccines, including modified vaccinia Ankara virus, poxvirus, and live attenuated tuberculosis vaccine (46, 52, 53). The protective role of neutrophils following immunization against tuberculosis is demonstrated when depletion of neutrophils abrogated the induction of Th1-specific responses (52). Similarly, neutrophil depletion significantly abrogated Ag-specific CD4+ T cell proliferation in live attenuated centrin gene-deleted L. donovani (LdCen-/-)-immunized mice and impaired protective immunity against virulent L. donovani challenge (47). In contrast, depletion of neutrophils in animals immunized with killed L. major + CpG vaccine enhanced protection upon sandfly mediated challenge (19). In LdCen-/- immunization studies, distinct functional roles of neutrophil subsets were observed. Specifically, Nα subsets from LdCen−/− infected mice expressed significantly higher levels of costimulatory molecules along with attenuation of coinhibitory molecules, resulting in significantly higher CD4+ T cell activation compared with the Nα population from L. donovani wild type-infected mice (47). More studies are needed to elucidate the role of neutrophil subsets in other anti-leishmanial vaccination regimens.
In addition to the role of neutrophils in adaptive immune responses, recent vaccination studies have shown that neutrophils may also acquire non-specific memory due to epigenetic and metabolic changes. This process termed “trained immunity” is being intensely studied in neutrophils and other cell types. Metabolic reprogramming induced by immunological signals determines the epigenetic changes that orchestrate trained immunity (54). These epigenetic modifications are usually broad, not pathogen-specific, and include chromatin changes to a gene or locus (55). A recent report form Moorlag, et al. demonstrated that neutrophils acquire trained immunity after Bacillus Calmette-Guérin (BCG) vaccination. Furthermore, trained neutrophils showed long-term reprogramming and maintained their enhanced activation up to 3 months after vaccination (56). Similar data is not available in leishmaniasis models. The broader role of neutrophils in vaccine immunity in general and their capacity to acquire trained immunity characteristics in particular, are of significant interest although durability and thus relevance of trained immunity in neutrophils remain to be demonstrated in anti-Leishmania vaccines.
Monocytes
Monocytes, along with neutrophils, are the earliest immune cell populations to be recruited after infection of Leishmania (57) (Figure 2). Recruitment of monocytes is modulated by pro-inflammatory cytokines and chemokines after infection with different Leishmania species. Monocyte subpopulations exist within a phenotypical spectrum ranging from long-lived patrolling monocytes (non-classical) that mainly monitor the vasculature as part of normal physiology (58) to short-lived inflammatory (classical) monocytes (iMOs, CD11b+ CX3CR1lo Ly6Chi CCR2+ CD115+) (59, 60). During the bloodmeal, Leishmania is injected along with sand fly salivary components, which modulate inflammatory responses that lead to CCR2-mediated chemotaxis of iMOs to the infection site (61), reviewed in (62, 63). iMOs play contradictory roles in different infections: while they mediate host defense against toxoplasmosis (64) and malaria (65), a detrimental role in VL was demonstrated (51). Monocytes can recognize Leishmania parasites via Toll-like receptors (TLR)s and produce pro- and anti-inflammatory cytokines that can play paradoxical roles during leishmaniasis (66–69) (Figure 2). The expression of TLRs and the molecules on the parasite surface could also generate a diverse range of monocytic responses during infection with different Leishmania species. For instance, L. donovani infection of monocytes inhibits oxidative burst and antigen presentation and activates IL-10 expression via downregulation of TLR2 and TLR4 signaling (70, 71). In contrast, during L. tropica infection, monocytes showed higher expression of TLR9 in addition to TLR2/TLR4, and elevated TNF-α that resulted in a decrease of inducible nitric oxide synthase (72). Furthermore, L. braziliensis infection of human monocytes showed enhanced production of TNF-α and higher TNF-α/IL-10 ratios, and controlled parasite burdens more effectively compared to L. infantum infection (66). In addition to their role in pathogenesis, monocytes play an important role in granuloma formation, necessary for parasite clearance in murine models of L. donovani infection. However, an IL-17-/- mouse model of L. donovani infection showed diminished monocyte and neutrophil infiltration resulting in smaller isolated granulomas in the liver and spleen, yet controlled parasite burden due to elevated IFN-γ production (73).
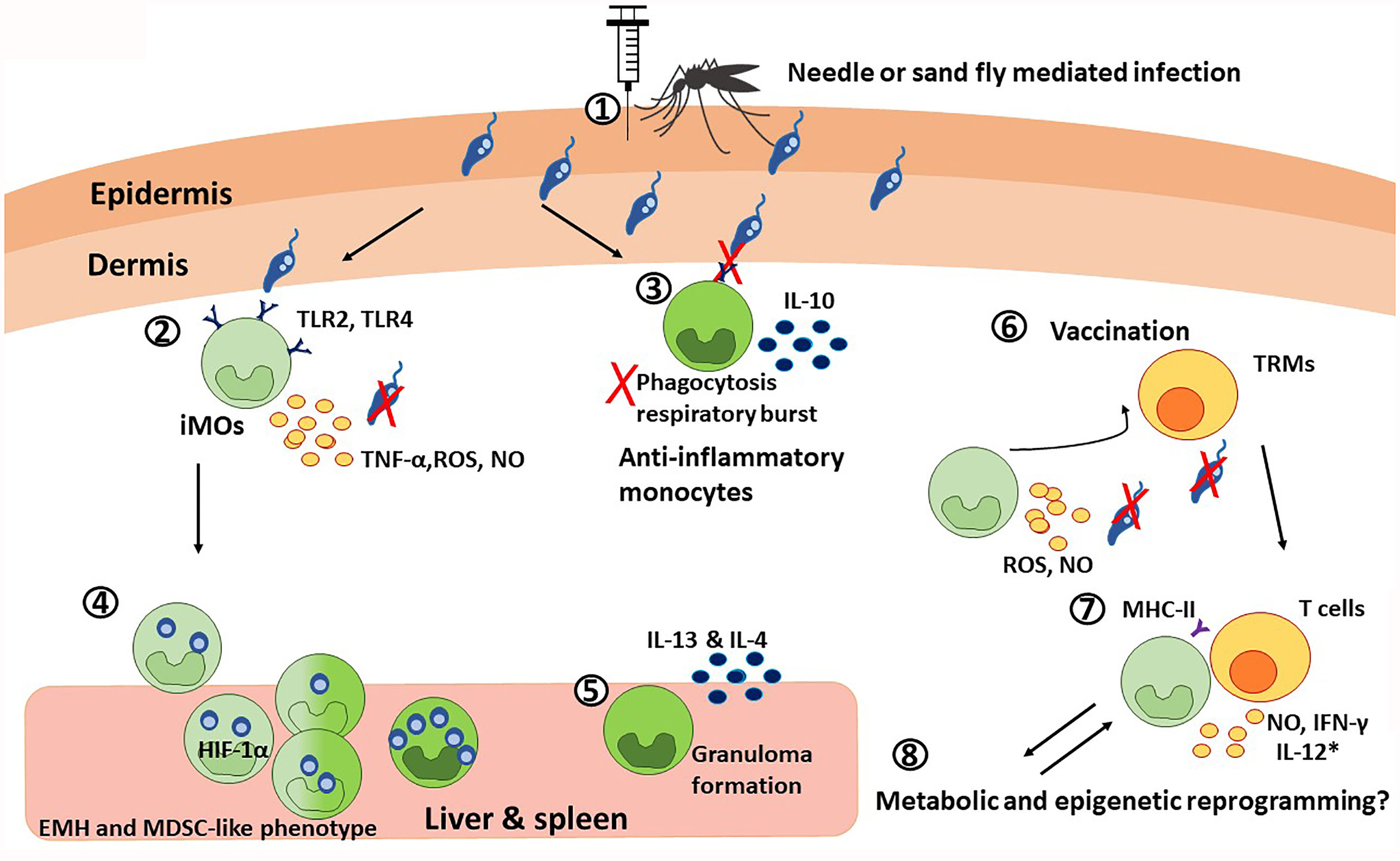
Figure 2 The paradoxical roles of monocytes during visceral leishmaniasis. 1) Monocyte chemotaxis to the infection site is mediated in a CCR2-dependent manner. During the blood meal, this process is additionally potentiated by sand fly salivary components. 2) The inflammatory monocytes, iMOs (light green), recognize Leishmania trough TLR2 and TLR4. TLR recognition, together with the IFN-γ released by neutrophils, induce the secretion of TNF-α, ROS, and NO that results in parasite killing. Although the specific cytokine regulation is species-specific. 3) Particularly during VL, Leishmania can downregulate TLR2 and TLR4 signaling, dampening phagocytosis and respiratory burst, and promoting IL-10 production. This way, the parasite promotes its own survival by favoring the anti-inflammatory monocyte profile (dark green). 4) Infected iMOs migrate rapidly to the liver and spleen, where they undergo extramedullary hematopoiesis (EMH) and acquire a HIF-1α-mediated MDSC-like phenotype, that is permissive for parasite survival, as observed in VL patients. 5) In the liver and spleen, anti-inflammatory monocytes are necessary for granuloma formation via the production of IL-13 and IL-4, which has a protective role during VL. 6) In the context of vaccination, iMOs and T cells interplay is critical for memory responses. On the one hand, skin resident CD4+ memory T cells (TRMs) recruit iMOs to the infection site, where they produce ROS and NO to control the infection. 7) At the same time, iMOs activate T cell responses by presenting antigens via MHC-II and producing NO and IFN-γ; and with the use of co-adjuvants targeting monocytic response, iMOs can also produce IL-12 (*). 8) Finally, it is possible that monocytes undergo metabolic and epigenetic reprograming during VL.
Studies of active VL patients in Brazil and India showed an increase of CD14+ monocytes, and elevated expression of IL-10, which affects macrophage polarization and the outcome of the disease (74, 75). Other studies with Indian VL patients reported an anti-inflammatory (non-classical) monocyte response in the peripheral blood characterized by reduced expression of TLR2 and TLR4, chemokine receptors and adhesion molecules, as well as impaired phagocytosis and oxidative burst, important for the elimination of Leishmania parasites (70, 76). These observations illustrate the critical role of monocytes in the early modulation of host immune responses and the clinical outcome of L. donovani infection. The divergent engagement of TLRs and associated immune responses following infection of monocytes with different Leishmania species suggests that targeting these interactions could be exploited in the development of effective anti-parasitic strategies.
Role of Monocyte Subsets in the Pathogenesis of VL
A growing body of research is focused on elucidating the role of sub-populations of monocytes such as iMOs, intermediate, and non-classical patrolling monocytes in Leishmania infection. Monocytic subsets (CD14+ CD16- and CD14+ CD16+) of human origin displayed significantly increased phagocytic capacity and intracellular NO production when infected with L. infantum, compared to L. braziliensis (77). iMOs contribute to parasite control at the lesion site in CL (78), but they play a detrimental role in VL (59). Similarly, different subsets of resistant (Ly6Clo/M1-like) or permissive (Ly6Chi/M2-like) monocytes have also been reported in VL (79). Our previous studies demonstrated that iMOs (CD11b+ Ly6Chi) are rapidly recruited into the spleen and liver within 24 hours of L. donovani infection in a CCR2-dependent manner, such that iMOs can make up to 15-25% of the myeloid cells in these organs (Figure 2) (59). The iMOs recruited to the spleen during L. donovani infection can acquire a hypoxia‐inducible factor (HIF)-1α mediated myeloid-derived suppressor cells (MDSC) like phenotype, which promotes a chronic infection (80). L. donovani infection of hamsters has been shown to induce extramedullary hematopoiesis of myeloid cells in the spleen resulting in the accumulation of monocytes that support parasite replication (81). Migration of Ly6Chi iMOs to the spleen and liver is facilitated by STAT-1 signaling (59, 82). Therefore, altering monocyte migration to spleen and liver by immunomodulatory agents could be a potent parasite control strategy (83). Indeed, therapeutic reduction of the iMOs influx to the visceral organs with Ibrutinib, is shown to reduce susceptibility to L. donovani infection in mice (84).
Role of iMOs in Vaccine Induced Immunity
Due to the pluripotent nature of monocytes to differentiate into macrophages and DCs, it is important to understand the early interaction between monocytes and Leishmania parasites. A recent study showed that iMOs are necessary to control L. major infection during challenge in a healed C57Bl/6 mouse model (85). iMOs are the main inducible nitric oxide synthase (iNOS) producers during secondary infections (86). Specifically, skin resident CD4+ memory T cells (TRMs) responsible for controlling the secondary infection recruit iMOs to the challenge site. These recruited iMOs reduced the parasite burden even in absence of CD44+ IFN-γ+ effector T cells by producing ROS and nitric oxide (NO) (85). iMOs also play a critical role in initiating differentiation of T cells to acquire a memory phenotype by secreting IL-18 and IL-15 in Listeria monocytogenes models, suggesting that iMOs could be an analogous source of these cytokines in Leishmania infections, though this needs to be formally demonstrated (87). Thus, due to their roles in parasite clearance at the challenge site and potentially in initiating a TRM response, targeting iMOs could improve efficacy of anti-Leishmania vaccines. For example, CpG oligonucleotides, when used as adjuvants, have been shown to induce IL-12 in monocytes (88). Studies of Leishmune®, a vaccine for canine VL, have elucidated the critical role of monocytes in relation to vaccine efficacy. Monocytes and neutrophils from vaccinated dogs showed increased phagocytic activity, NO and IFN-γ production, expression of TLRs, costimulatory molecules, and MHC-II (89, 90). The importance of monocytes relies not only on their early immune responses, but also in their capacity to activate an effective adaptive response, which is crucial in any vaccination design.
As described in the neutrophil section, there is an emerging interest in exploring the role of epigenetic and metabolic reprogramming in the context of trained immunity in monocytes (Figure 2). For example, Bacillus Calmette-Guérin (BCG) and β-glucan from Candida albicans can induce epigenetic changes in histone trimethylation at H3K4 in monocytes and macrophages to promote trained immunity (91). In particular, it seems that aerobic glycolysis induced by the activation of Akt, mammalian target of rapamycin complex 1 (mTORC1), and HIF-1 is responsible for cellular activation and trained immunity to BCG vaccination (92). Similarly, β-glucan from Candida albicans is shown to induce non-specific trained immunity in monocytes that conferred protection against L. braziliensis, by promoting IL-1β signaling and inducing epigenetic changes that result in upregulation of IL-32 (93). However similar trained immunity has not been demonstrated in other Leishmania species. These studies highlight how epigenetic and metabolic reprogramming can have profound effects on pathogen immunity. However, little is known about how such mechanisms are operating during Leishmania infections overall and how they can be exploited for the development of a pan-Leishmania vaccine including protection against visceral leishmaniasis. Since the life span of innate immune cells is limited, trained immunity has been shown to last up only up to one year (94). It is possible that in Leishmania endemic areas, where individuals are constantly exposed to Leishmania parasites, trained immunity can be repeatedly induced and could play a role in durable protection. Therefore, similar to the BCG vaccine, it may be desirable for efficacious vaccines against VL to target the induction of a robust trained immunity in addition to strong adaptive immune responses that are likely long-lasting.
Macrophages
Macrophages are considered the canonical hosts for Leishmania in the later stages of infection, following phagocytosis of Leishmania-infected apoptotic neutrophils (Figure 3) (95). Neutrophils provide the chemical cues (MCP-1/CCL2, MIP-1α/CCL3, MIP-1β/CCL4 and MIP-2/CXCL2) that help recruit macrophages to the infection site (96). In addition, salivary components induce a significant influx of macrophages in a CCL2/MCP-1 dependent mechanism in L. chagasi infection (97). Macrophage response to infection is dependent on the interplay of a) the surface molecules on the Leishmania parasites, such as glycoprotein-63 (gp63), lipophosphoglycan (LPG) and glycosylphosphatidylinositol (GPI); and b) the receptors which recognize them, such as Toll-like receptors (TLRs) (98) fibronectin, mannose, complement and Fc receptors (Figure 3) (99). Binding of Leishmania to complement and Fc receptors slows down phagosome maturation (100) and induces IL-10 production (99, 101, 102). TLRs recognize Leishmania surface gp63 and activate the NF-κB pathway in macrophages (Figure 3) (103). Interestingly, Leishmania uses gp63 to negatively regulate ROS production (104) and is shown to be critical for Leishmania-mediated inhibition of NLRP3 inflammasome and attenuating IL-1b secretion (105, 106). Macrophages play a dual role in the infection, as they not only serve as permissive hosts, but are also as anti-Leishmania effector cells (107). These contrasting roles depend on the microenvironmental signals in the host tissue (108) in response to different Leishmania species and on the differential recognition of the parasites. Depending on the stimulus, infected macrophages can polarize towards a functional phenotype within the spectrum ranging from inflammatory macrophages (also called classically activated or M1) and anti-inflammatory macrophages (also called alternatively activated of M2, Figure 3). Macrophage polarization can determine the outcome of the disease (37, 109–111). As discussed in the previous section, iMOs usually mature into M1 macrophages, while anti-inflammatory monocytes are more likely to differentiate into M2 macrophages (59, 112). Some of the parasite factors, such as L. infantum-derived lipid mediators, can also induce M2 phenotype by preventing IFN-γ-mediated M1 polarization (113). Functionally, macrophages classified within the M1 and M2 spectrum show distinct activities. The relationship between the macrophage phenotype and the immune milieu during Leishmania infection is discussed in more detail elsewhere (107). It is important to note that the phenotypic spectrum of macrophages is quite dynamic, and this plasticity confers a wide range of distinct phenotypic characteristics. However, for the purpose of this review we refer to the pro-inflammatory and anti-inflammatory ends of the spectrum as M1 and M2, respectively, as has been previously established in Leishmania immunology.
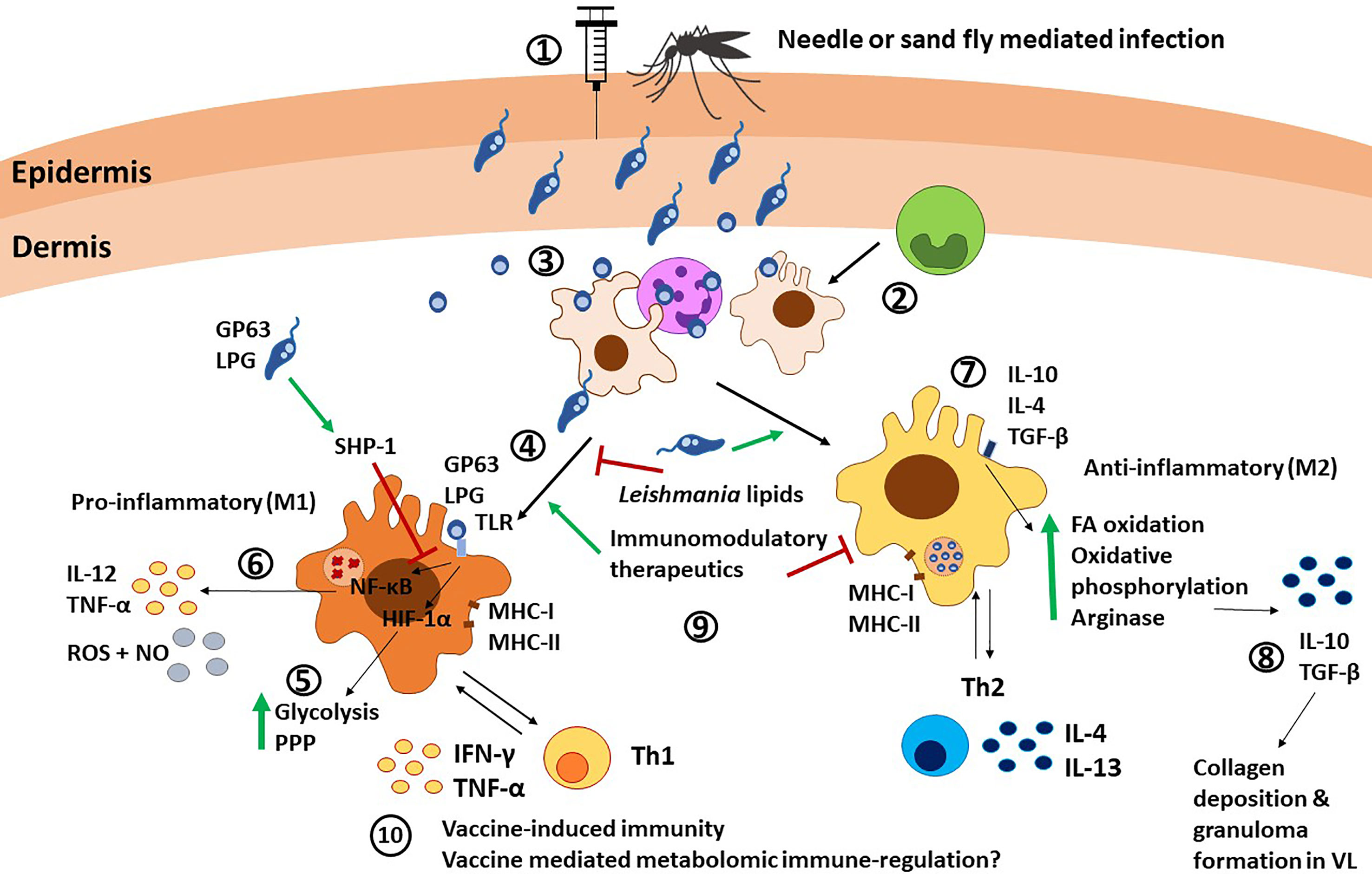
Figure 3 Macrophage immunity against Leishmania parasites. 1) Leishmania promastigotes are injected into the host via needle injection or sand fly injection. 2) Monocytes differentiate into macrophages at the infection site. 3) Macrophages are recruited by neutrophils and infected after neutrophils release Leishmania parasites, or as a consequence of phagocytosis of necrotic neutrophils. 4) Macrophages are the canonical host for Leishmania and can polarize towards M1 after TLR stimulation via Leishmania molecules such as LPG and GP63. 5) TLR signaling induces HIF-1α and subsequent upregulation of glycolysis and the pentose phosphate pathway (PPP). 6) TLR signaling also leads to NF-κB translocation to the nucleus and subsequent release of IL-12, TNF-α, and NO. SHP-1 upregulation by the parasite GP63 and LPS can interfere with this pathway. 7) On the other hand, stimuli such as IL-10, IL-4, and TGF-β can polarize macrophages towards an M2 anti-inflammatory phenotype, characterized by upregulation of fatty acid (FA) oxidation, oxidative phosphorylation and arginase production. 8) M2 macrophages produce IL-10 and TGF-β, which mediate collagen deposition and granuloma formation in VL. 9) Different factors such as Leishmania-derived lipids, immunomodulatory therapeutics and Th cells can induce and alter macrophage polarization. 10) The interplay between macrophages and Th1 cells via antigen presentation and cytokine release is involved in vaccine-induced immunity. Furthermore, metabolomic reprogramming could influence vaccine immunity, as shown in BCG vaccine, although this remains underexplored in Leishmania. PPP, pentose phosphate pathways; FA, fatty acid; NO, nitric oxide.
Types of Macrophages and Their Role in VL
Macrophages of M1/M2 phenotype are identified primarily based on their immunological characteristics. Recent studies have brought to focus distinct metabolic reprogramming that underlies the functional specialization of macrophages in addition to the vector/parasite derived factors that affect the macrophages. Leishmania parasite infection of macrophages is accompanied by metabolic changes that help the parasite adapt to the new environment. For example, L. infantum parasites optimize their metabolism to compensate for limited resources within macrophages by redistributing host metabolites towards the synthesis of biomass (114). Although the intracellular metabolism of Leishmania parasites is widely conserved, significant differences between L. infantum and L. mexicana infections have been reported (114–116). Tight junctions between the parasites and the parasitophorous vacuole (PV) allow for the bi-directional transport of lipids (14). Because of this, lipid bodies can accumulate in and around PVs and provide amastigotes with a carbon source of polyunsaturated fatty acids (PUFA) (14). Parasite metabolites themselves can affect the polarization of the macrophages. For instance, the infective metacyclic stages of L. infantum showed increased levels of docosahexaenoic acid, a PUFA, compared to the non-infective procyclic forms (113). Docosahexaenoic acid and its derivative resolvin D1 were shown to induce M2 polarization in macrophages (117). In this context, it is interesting to note high serum levels of resolvin D1 and other lipids, such as prostaglandin F2 and leukotriene B4, observed in VL patients (118).
Activation of pro-inflammatory macrophages (M1) is due to stimuli such as IFN-γ, lipopolysaccharide (LPS), and TLR signaling (111, 119). Metabolomic studies, however, identified such activation to be dependent on upregulated glycolysis and glutaminolysis. Conversely, activation of anti-inflammatory macrophages (M2) occurs through stimuli such as IL-13 and IL-4, independently of TLR. Anti-inflammatory macrophage-activation is also dependent on fatty acid oxidation (Figure 3) (13, 120). Additional metabolic changes have been identified that drive subsequent immunomodulation of macrophages. For example, the upregulation of the pentose phosphate pathway, crucial for pro-inflammatory (M1) effector functions (121), occurs several hours prior to the induction of pro-inflammatory cytokines (122).
In pro-inflammatory (M1) macrophages, HIF-1α has been identified as the main regulator of glycolytic metabolism, and it contributes to pathogen clearance (Figure 3) (13). M1 macrophages upregulate anaerobic or aerobic glycolysis and the pentose phosphate pathway to generate ATP and NADPH, used for ROS production, which aids parasite clearance. On the other hand, M2 macrophages shift towards a more efficient mitochondrial respiration, making the macrophage more permissive to amastigote growth (14, 123). Additionally, arginine transport is upregulated in activated M1 macrophages, where it is readily used to produce NO. On the other hand, low IFN-γ activation leads to decrease NO levels and increased arginine availability for the parasite (14, 124). Taken together, these studies show that metabolic reprogramming of the host cell has a profound effect on its effector functions of macrophages.
Pro-inflammatory (M1) macrophages upregulate IL-12 and TNF-α expression, as well as ROS and RNS production, crucial to control Leishmania infection, as demonstrated in both mice and human models (111, 125, 126). Within this inflammatory microenvironment, IL-12 can upregulate iNOS and promote the development of IFN-γ-producing Th1 cells (99). IFN-γ then induces IL-12 and iNOS expression (99), which synthesizes high amounts of NO with leishmanicidal activities. However, the signal transduction of both IL-12 and IFN-γ in macrophages is interrupted in Leishmania infections (L. donovani, L. major, and L. mexicana) by inhibition of STAT-1 and STAT-4 phosphorylation (127, 128). Also, Leishmania favors the activation of SHP-1, an inhibitor of the TLR cascade, which impairs production of pro-inflammatory cytokines (Figure 3) (129). The importance of SHIP-1 pathway in parasite survival was demonstrated in invitro studies by the inhibition of SHIP-1 pathway by using antileishmanial drug sodium stibogluconate (130). Similarly, surface molecules such as lipophosphoglycan (LPG) from L. donovani dampen the IL-12 and ROS production by preventing MAPK activation (127), although the protective effect of LPG seems to be species and strain dependent (131, 132). A more in-depth discussion of the signaling pathways involved in Leishmania infection has been covered in detail in other reviews (74, 80, 82, 99).
Anti-inflammatory (M2) macrophages show an IL-10 and IL-4 dominant response that can lead to decrease NO secretion (7, 109, 133). Furthermore, IL-10 can make macrophages refractory to IFN-γ-mediated activation (99). In human VL, high IL-10 levels in blood and lesion tissue correlated with high parasitic load (134–139). Thus, human L. donovani infections show a predominant activity of M2 macrophages (140, 141) however M1/M2 dichotomy in protection versus pathogenicity is not always as binary. M2 macrophages can also play a protective role during VL, as IL-4 and IL-13 are necessary for collagen deposition and granuloma formation (Figure 3) (142).
Role of Macrophages in Vaccine Induced Immunity
Due to the specialized role of macrophages in the induction of immune response against leishmaniasis, they are extensively studied in the context of assessing candidate vaccines. Macrophages due to their role of professional antigen presenting cells, are crucial for T cell activation after antigen presentation. The combined signals provided by the parasitized macrophages displaying Leishmania antigenic epitopes and their interaction with naïve CD4+ T cells bearing cognate T cell receptors lead to the development of an antigen-specific effector T-cell response. Accordingly, the role of macrophages in Leishmania clearance during challenge infection following activation of T effector cells has been reported in numerous experimental vaccines including protein-based, nano-particulate encapsulated, and DNA-based vaccines (143–147). The macrophage chemokine network is critical in producing tissue resident CD4+T memory cells (TRM) in viral vaccines (148). A similar role for macrophages in the generation of tissue resident memory T cells in Leishmania remains to be demonstrated.
The role of macrophages in the design and development of novel candidate vaccines has been reviewed previously (149, 150). Still, it is possible that vaccines or adjuvants targeting macrophages potentiate and complement the cellular immune response, at the same time allowing the macrophages to intensify the killing of internalized Leishmania. M1 macrophage-mediated induction of a polarized Th1 response was associated with resistance to intracellular pathogens. Such a polarization of T cells by pro-inflammatory (M1) macrophages was associated with protection in several vaccine studies, including studies of recombinant Mycobacterium bovis BCG, attenuated West Nile virus (WNV), and live attenuated measles virus. These studies show that efficacious candidate vaccines manipulate macrophages to enhance pro-inflammatory responses and yield improved protection (151–153). Exploitation of differential macrophage phenotype towards improving the efficacy of experimental vaccines warrants further studies.
The role of macrophages in potentiating a Th1 or Th2 response in vaccine immunity or pathogenesis has been shown in experimental L. donovani infections, with a special emphasis on the role of membrane cholesterol in enabling anti-leishmanial activities of macrophages (154). Specifically, L. donovani can alter the physiology of the macrophage membrane by depleting cholesterol, resulting in defective antigen presentation and impaired T cell responses (155–157). Interestingly, as opposed to virulent wild type L. donovani (LdWT) infection, immunization with the avirulent genetically modified LdCen-/- does not interfere with membrane fluidity and antigen presentation, allowing for macrophage activation and induction of Th1 immunity (120). Similarly, human macrophages infected with LdWT or LdCen-/- have shown differential expression of miR-21 and its target gene IL-12 illustrating the early immune modulatory role of the live attenuated vaccines (158). In summary, although macrophages have been studied extensively in VL pathogenesis and in experimental vaccines, emerging data in other vaccines exploring their role in trained immunity suggests that early metabolomic reprogramming in macrophages similarly could have profound effects in determining efficacy or Leishmania vaccines and therapeutics thus remain to be investigated.
Dendritic Cells
Dendritic cells (DCs) are professional antigen-presenting cells (APCs), are ubiquitous in the peripheral tissues, and perform sentinel functions (Figure 4). Several distinct subsets of DCs (plasmacytoid, conventional, monocyte derived and more) have been identified based on their functional specialization and expression of distinct markers (159). Similar to other phagocytic cells, DCs can take up antigens via Fc receptors, C-type lectin receptors (CLRs), and pattern recognition receptors such as TLRs (Figure 4) (160). Recognition of Leishmania parasites by DCs is accomplished via TLR-2, -4, and -9 (161). TLR-9 has been identified as being responsible for DC activation and production of neutrophil chemo-attractants and IL-12 secretion in L. infantum infection in C57BL/6 mice (162). Following infection, DCs undergo maturation and express MHCII, CD80, CD86, CD40 and migrate to lymphoid tissues where they present antigens to naïve T cells (Figure 4) (10). DCs are the main producers of IL-12, which is critical for polarization of naïve T cells into IFN-γ producing Th1 cells (163). L. donovani infected DCs start producing IL-12, IL-23 and IL-27 within 5 hours of infection, IL-12 being mostly produced in CD8α+ DC subsets (164). In the chronic stage of L. donovani infection, CD11c+ splenic DCs showed a functional impairment, correlated with reduced surface expression of MHC-II and impaired IL-12 production (165). Similarly, migration of splenic DC into marginal zones is regulated by the CCR7 ligands CCL19/CCL21, which also affect the ability of DCs to produce IL-12 (166).
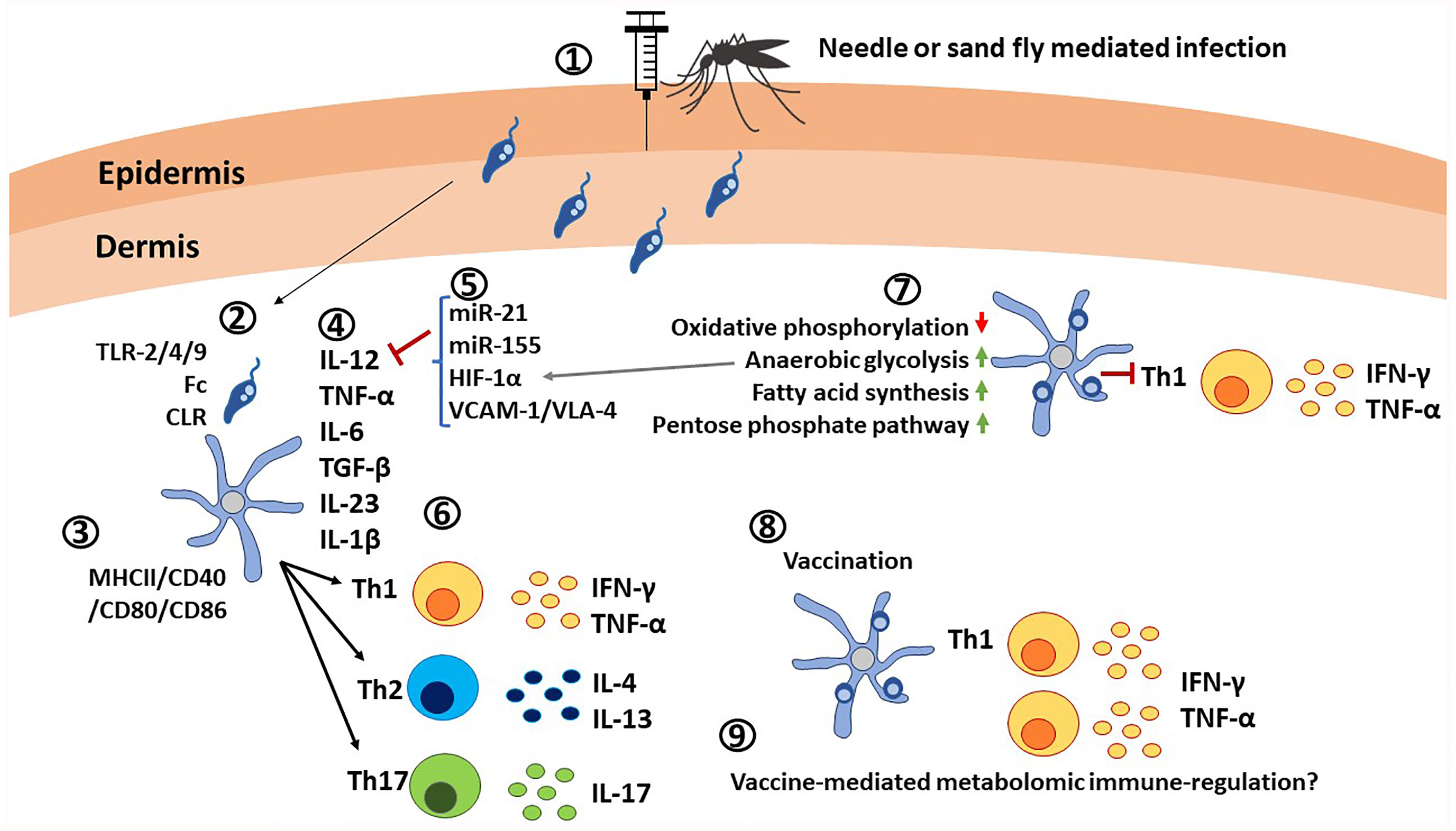
Figure 4 Critical roles of dendritic cells in immunity against Leishmania parasites. 1) Leishmania promastigotes are injected into the host via needle injection or sand fly injection. 2) DCs interact with Leishmania parasites through TLR-2/4/9, Fc receptors and C-type lectin receptors. 3) Activation of DCs is indicated by the elevated expression of MHC-II/CD40/CD80/CD86. Leishmania infections also causes expression of co-inhibitory molecules. 4) DCs are the main producers of IL-12 in addition to TNF-α, IL-6, TGF-β, IL-23, and IL-1β, which determine the differentiation of naïve T cells. 5) Expression of IL-12 is affected by microRNAs, transcription factors such as HIF-1α and ligands such as VCAM-1/VLA-4. 6) Upon interaction with DCs in lymphoid tissues in presence of cytokine signals, naive T cells differentiate into Th1, Th2 or Th17 cells and produce pro-inflammatory cytokines IFN-γ, TNF-α, or anti-inflammatory cytokines IL-4, IL-13 or IL-17. 7) Infected DCs are characterized by upregulation of anerobic glycolysis, fatty acid synthesis, pentose phosphate pathway, and by down regulation of oxidative phosphorylation, which results in poor expansion of Th1 cells. 8) Vaccination with live attenuated Leishmania parasites has shown elevated IL-12 production and expansion of Th1 cells. 9) Similar to other innate immune cells, metabolomic immune regulation in DCs upon vaccination remains an unexplored area.
Role of DCs in VL Pathogenesis
Expression of IL-12 in DCs is regulated by several factors including HIF-1α (167), interactions between VCAM-1/VLA-4 (Vascular cell adhesion molecule-1 and very late antigen-4) (168), miR-21 (169), and miR-155 (Figure 4) (170). DCs patrol for pathogens and participate in the immune response against Leishmania parasites, mainly by migrating to secondary lymphoid tissues and activating naïve T cells through antigen presentation. In addition, parasite factors such as L. infantum excreted/secreted proteins (LipESP) reduced the ability of human DCs to produce IL-12p70 (171). Similarly, exosomes from L. donovani failed to prime monocyte-derived human DCs to drive the differentiation of naive CD4 T cells into IFN-γ-producing Th1 cells in vitro. Vesicles from L. donovani parasites deficient in HSP100 showed a stronger pro-inflammatory phenotype in human DCs in vitro (172). Similarly, exosomes from L. donovani-infected bone marrow-derived DCs (BMDCs) showed a miR-21 dependent inhibition of IL-12 production indicating numerous mechanisms exist that regulate the function of DCs, particularly IL-12 production (158).
In addition to cytokine/chemokine-mediated immune regulation, metabolic changes in DCs have also been shown to affect their functions. For instance, inactivated DCs use oxidative phosphorylation and fatty acid oxidation for energy supply and biomolecule synthesis (173). However, infection leads to substantial metabolic changes as a result of PAMP-mediated activation. In particular, oxidative phosphorylation is reduced (174), while anaerobic glycolysis, fatty acid synthesis, and the pentose phosphate pathway are induced for ATP production and biosynthesis of lipids and nucleotides (Figure 4) (175, 176). These metabolic changes play important roles in inflammation and in the establishment of an immune response. Generally, the regulator of glycolytic metabolism HIF-1α plays a key role in DC maturation and activation during inflammation (177). However, HIF-1α was shown to promote L. donovani infection by reducing IL-12 production in splenic DCs, thereby limiting Th1 expansion (167), and impairing CD8+ expansion (178) in murine VL models. These findings are in contrast to what was shown in macrophages, where HIF-1α reduced susceptibility to L. donovani (179), and suggest that the effects of glycolysis are likely to be host cell specific.
Role of DCs in Vaccine Induced Immunity
DCs due to their potent APC activities have been used as potential Leishmania vaccines by loading selected vaccine antigens or DC-derived exosomes, although these vaccines did not progress beyond laboratory studies (180–182). More recent studies focus on ex-vivo pulsing of DCs, and then using these cells to vaccinate mice, which has shown promising results. As such, pulsing the DCs with the N-terminal of Leishmania elongation factor 2, an antigen overlapping MHC-I and MHC-II epitopes, triggered a T cell response when used with CpG oligodeoxynucleotides. Such vaccinated mice could control L. infantum infection, resulting in IL-2 and TNF-α, but not IL-10 production by CD4+ T cells, and in the production of IFN-γ by both CD4+ and CD8+ T cells (183). DCs have also been shown to play a critical role in priming Th17 response in L. donovani infections. Immunization with LdCen-/- parasites showed that LdCen-/–infected DCs produce IL-1β, IL-6, and TGF-β to promote the development of Th17 lineage, and upon virulent challenge with wild-type L. donovani (LdWT) infection both CD4 and CD8 T cells produced IL-17, resulting in protection (184). Similar results were found when priming DCs with the C-terminal domain of nucleoside hydrolase NH36 from L. donovani (185). In addition to the T cell response against the L. infantum chagasi challenge, the defect in DCs migration to the lymph node was reversed, allowing proper antigen presentation (185).
The multifarious roles played by DCs as a critical source of IL-12 and the redundant mechanisms that affect the expression of IL-12 in Leishmania infection highlight the pivotal role of DCs in pathogenesis and potentiating an efficacious vaccine response.
Mast Cells
Mast cells (MCs) have been shown to participate in the innate immune responses in CL and VL (8, 186). MCs participate in the initiation and orchestration of innate and adaptive defense to pathogens and in various inflammatory responses (187, 188). MCs are present in large numbers in the skin, predominantly in the superficial dermis, the site where Leishmania parasites are deposited after the bite of infected sand flies (189, 190).
Literature on MCs with respect to visceral infections of Leishmania is sparse. Much of our understanding of the role of MCs in Leishmania is derived from studies of CL. MC-derived TNF-α followed by neutrophil influx and MIP-1α/β release is required for the recruitment of macrophages during cutaneous granuloma formation, a hallmark of parasite-induced inflammatory responses (191). Indeed, MCs recruit effector cells of innate and adaptive immunity to sites of L. major infection and induce systemic protective dendritic cell (DC)-dependent adaptive immune responses that eventually control L. major infection. Differential MC infiltration patterns and responses have been reported depending on the mouse strains (8) and Leishmania species (186). For example, there was a significant uptake and killing of L. tropica by MCs compared to L. donovani. Interactions of MCs with both these Leishmania species ensues the release of MC extracellular traps (MCETs) analogous to NETs produced by neutrophils (Figure 1). Although both L. donovani and L. tropica seem susceptible to MCETs, relatively higher number of viable promastigotes of L. donovani in comparison to those of L. tropica are observed indicating the relative resistance of L. donovani parasites. Thus, MCs play a very important role in early innate immune response to L. tropica and L. donovani and thus may play an important role in the success of vaccines against Leishmaniasis (186). Indeed, it has been hypothesized that MC-induced control of L. major infections is not only restricted to the induction of local inflammation, but that MC recruitment of pro-inflammatory cells (i.e. DCs) to sites of L. major inoculation ensures the development of protective, long-lasting memory responses against L. major (192), suggesting the relevance of MCs in vaccine immunity. Interestingly, vaccination with LdCen-/- parasites against virulent L. mexicana challenge resulted in reduced infiltration or absence of degranulated MCs, which correlated with protection (193). MCs may have a role in Leishmania vaccination, similar to that observed in BCG vaccination, where MCs phagocytize BCG, produce ROS, and release MCETs (194). More studies in experimental models and in humans are required to understand the immune mechanisms that determine MCs involvement in Leishmania vaccine-induced immunity. MCs-derived molecules have shown potent adjuvant activity in certain vaccines [Reviewed in (195, 196)]. In Toxoplasma gondii infection, the treatment with two different MCs-derived molecules, C48/80 or cromoglycate, can either increase or decrease the parasite burden by differentially favoring Th1 or Th2 responses, respectively (197). Similar results have also been observed in a Trypanosoma cruzi murine model, where cromoglycate administration led to an increase of parasitic burden (198). Administration of other MCs-derived molecules like tryptase, resulted in the cleavage of many Th2 cytokines, and consequent Th1 polarization (199). Similar studies in Leishmania exploring the role of MC-derived molecules as potential immunomodulators remain to be undertaken.
Natural Killer and NKT Cells
Natural killer (NK) cells, identified as CD3−CD56+ in humans, are characterized by their cytotoxic activity and cytokine production (200), and have been shown to participate in the immune response against Leishmania (Figure 1). VL patients show three different NK subsets: CD56–CD161+, CD56+CD161–, and CD56+CD161+, with a loss of the CD56+CD161+ population in comparison to the healthy individuals (201). TLR-2 recognition of Leishmania LPG activates NK cells and induces the production of IFN-γ and TNF-α and the translocation of NF-κB to the nucleus. This activation seems to be more intense in response to metacyclic promastigotes, compared to procyclic promastigotes (202). IFN-γ production and NK cytotoxicity is also dependent on TLR-9 (203). Interestingly, the downregulation of STAT-1 related to the reduction in IFN-γ, TNF-α and TLR2 expression by NK cells allows the development of diffuse cutaneous leishmaniasis (DCL), a form of the disease characterized by the uncontrolled spread of the parasites (204). Moreover, lesion healing of DCL patients corelates with the increase of CD16+ CD56+ NK cells (205). Despite their important role, NK cells are not necessary for the establishment of an effective Th1 response against L. major (206), or L. tropica, whereas they are indispensable for the elimination of L. donovani amastigotes (207). A more detailed appraisal of the role of NK cells during leishmaniasis can be found in (208). Recent studies have identified NK cells to play a role in vaccine-mediated immunity. Laabs, et al. have shown that vaccination with live L. major combined with CpG DNA leads to DC and NK activation, as well as increased IFN-γ production by NK cells (209). Interestingly, NK cells have also been shown to acquire trained immunity, in the context of BCG vaccination. However, the exact epigenetic and metabolic reprogramming underlying this phenomenon remains to be fully elucidated (210, 211). Similar to other innate cell types, the role of trained immunity in NK cells needs to be further investigated in the context of Leishmania infection and vaccination.
There are few reports about the role of NK-T cells (NKTs) in leishmaniasis. NKTs are CD1d-restricted T cells with innate and adaptive properties, which react to a variety of stress proteins and glycolipids using either NK or T cell effector mechanisms (212). Glycoconjugates on the Leishmania surface are detected by CD1d+ NKT cells, which are protective against leishmaniasis in early stages of VL (213). CD8+ NKT cells are also protective, express IFN-γ and Killer cell immunoglobulin-like receptors (KIRs) and do not migrate towards the L. donovani infection site, whereas the CD4+ NKTs are found to be pathogenic as they migrate towards the infection site and express CD25, FoxP3 and IL-10 (214). In comparison to other immune cells, literature on the role of NK and NKT cells remains limited in VL. They may yet play important roles in protective immunity in anti-leishmania vaccines analogous to the limited studies in BCG vaccination that revealed potent trained immunity that contributed to protection.
Conclusion
The immune response to Leishmania infection is primarily initiated by innate immune cells which orchestrate the generation of protective innate and adaptive immunity against Leishmania parasites. Innate immune cells specialize in the clearance of invading pathogens. Leishmania parasites have evolved a range of evasion strategies to subvert normal innate cell function. While the role of the innate immune cells in host protection during Leishmania infection are being explored, important questions regarding their roles in shaping the protective immunity in a prophylactic vaccine setting remain to be investigated. Furthermore, chronic infections with Leishmania parasites have been shown to induce prominent changes in host metabolomic pathways that influence immune cell proliferation, differentiation, and effector functions. Current advances in metabolomics have made significant impact and present important implications in the management of VL. Metabolomic profiling during VL may reveal novel immune regulation networks that can be exploited for vaccine development. Additionally, while metabolic changes in different Leishmania species are well studied and could serve as biomarkers of virulence and disease progression, what would be a desirable metabolic profile for innate immune cells during vaccination remains to be investigated. In conclusion, innate immune cells are critical for the development protective immunity due to their profound role in shaping adaptive immunity. Furthermore, innate cells themselves may undergo epigenetic and metabolic changes that renders them to acquire trained immunity, an emerging concept that has been studied in various vaccines. Such studies of metabolomic and epigenetic changes of innate immune cells will benefit the development of efficacious vaccines against VL.
Author Contributions
GV, TPF, PB, TO, and SG drafted the article. ARS and HLN conceived the theme of the article. HLN and SG reviewed the draft. All authors contributed to the article and approved the submitted version.
Funding
This work is supported by NIH/NIAID grants R21 AI130485 02, RO3 AI144253 01 (ARS), Global Health Innovative Technology Fund grants G2018-201 and G2019-213 (ARS, HLN) and by intramural funding from FDA.
Author Disclaimer
Contributions by the FDA authors are an informal communication and represent their best judgment. These comments do not bind or obligate FDA.
Conflict of Interest
The authors declare that the research was conducted in the absence of any commercial or financial relationships that could be construed as a potential conflict of interest.
Publisher’s Note
All claims expressed in this article are solely those of the authors and do not necessarily represent those of their affiliated organizations, or those of the publisher, the editors and the reviewers. Any product that may be evaluated in this article, or claim that may be made by its manufacturer, is not guaranteed or endorsed by the publisher.
References
1. Alvar J, Velez ID, Bern C, Herrero M, Desjeux P, Cano J, et al. Leishmaniasis Worldwide and Global Estimates of Its Incidence. PloS One (2012) 7:e35671. doi: 10.1371/journal.pone.0035671
2. Kaye P, Scott P. Leishmaniasis: Complexity at the Host-Pathogen Interface. Nat Rev Microbiol (2011) 9:604–15. doi: 10.1038/nrmicro2608
3. Desjeux P. Leishmaniasis: Current Situation and New Perspectives. Comp Immunol Microbiol Infect Dis (2004) 27:305–18. doi: 10.1016/j.cimid.2004.03.004
4. Kumar R, Goto Y, Gidwani K, Cowgill KD, Sundar S, Reed SG. Evaluation of Ex Vivo Human Immune Response Against Candidate Antigens for a Visceral Leishmaniasis Vaccine. Am J Trop Med Hyg (2010) 82:808–13. doi: 10.4269/ajtmh.2010.09-0341
5. Selvapandiyan A, Dey R, Gannavaram S, Lakhal-Naouar I, Duncan R, Salotra P, et al. Immunity to Visceral Leishmaniasis Using Genetically Defined Live-Attenuated Parasites. J Trop Med (2012) 2012:631460. doi: 10.1155/2012/631460
6. Gurung P, Kanneganti TD. Innate Immunity Against Leishmania Infections. Cell Microbiol (2015) 17:1286–94. doi: 10.1111/cmi.12484
7. Rossi M, Fasel N. How to Master the Host Immune System? Leishmania Parasites Have the Solutions! Int Immunol (2018) 30:103–11. doi: 10.1093/intimm/dxx075
8. Saha B, Tonkal AM, Croft S, Roy S. Mast Cells at the Host-Pathogen Interface: Host-Protection Versus Immune Evasion in Leishmaniasis. Clin Exp Immunol (2004) 137:19–23. doi: 10.1111/j.1365-2249.2004.02505.x
9. Soong L. Modulation of Dendritic Cell Function by Leishmania Parasites. J Immunol (2008) 180:4355–60. doi: 10.4049/jimmunol.180.7.4355
10. Liu D, Uzonna JE. The Early Interaction of Leishmania With Macrophages and Dendritic Cells and Its Influence on the Host Immune Response. Front Cell Infect Microbiol (2012) 2:83. doi: 10.3389/fcimb.2012.00083
11. Martinez-Lopez M, Soto M, Iborra S, Sancho D. Leishmania Hijacks Myeloid Cells for Immune Escape. Front Microbiol (2018) 9:883. doi: 10.3389/fmicb.2018.00883
12. Everts B. Metabolomics in Immunology Research. Methods Mol Biol (2018) 1730:29–42. doi: 10.1007/978-1-4939-7592-1_2
13. Ganeshan K, Chawla A. Metabolic Regulation of Immune Responses. Annu Rev Immunol (2014) 32:609–34. doi: 10.1146/annurev-immunol-032713-120236
14. Saunders EC, McConville MJ. Immunometabolism of Leishmania Granulomas. Immunol Cell Biol (2020) 98:832–44. doi: 10.1111/imcb.12394
15. McConville MJ, Saunders EC, Kloehn J, Dagley MJ. Leishmania Carbon Metabolism in the Macrophage Phagolysosome- Feast or Famine? F1000Res (2015) 4:938. doi: 10.12688/f1000research.6724.1
16. Ribeiro-Gomes FL, Sacks D. The Influence of Early Neutrophil-Leishmania Interactions on the Host Immune Response to Infection. Front Cell Infect Microbiol (2012) 2:59. doi: 10.3389/fcimb.2012.00059
17. Carlsen ED, Liang Y, Shelite TR, Walker DH, Melby PC, Soong L. Permissive and Protective Roles for Neutrophils in Leishmaniasis. Clin Exp Immunol (2015) 182:109–18. doi: 10.1111/cei.12674
18. Mollinedo F, Janssen H, de la Iglesia-Vicente J, Villa-Pulgarin JA, Calafat J. Selective Fusion of Azurophilic Granules With Leishmania-Containing Phagosomes in Human Neutrophils. J Biol Chem (2010) 285:34528–36. doi: 10.1074/jbc.M110.125302
19. Peters NC, Kimblin N, Secundino N, Kamhawi S, Lawyer P, Sacks DL. Vector Transmission of Leishmania Abrogates Vaccine-Induced Protective Immunity. PloS Pathog (2009) 5:e1000484. doi: 10.1371/journal.ppat.1000484
20. Pillay J, den Braber I, Vrisekoop N, Kwast LM, de Boer RJ, Borghans JA, et al. In Vivo Labeling With 2H2O Reveals a Human Neutrophil Lifespan of 5.4 Days. Blood (2010) 116:625–7. doi: 10.1182/blood-2010-01-259028
21. Charmoy M, Megnekou R, Allenbach C, Zweifel C, Perez C, Monnat K, et al. Leishmania Major Induces Distinct Neutrophil Phenotypes in Mice That Are Resistant or Susceptible to Infection. J Leukoc Biol (2007) 82:288–99. doi: 10.1189/jlb.0706440
22. Guimaraes-Costa AB, Nascimento MT, Froment GS, Soares RP, Morgado FN, Conceicao-Silva F, et al. Leishmania Amazonensis Promastigotes Induce and Are Killed by Neutrophil Extracellular Traps. Proc Natl Acad Sci USA (2009) 106:6748–53. doi: 10.1073/pnas.0900226106
23. Hurrell BP, Regli IB, Tacchini-Cottier F. Different Leishmania Species Drive Distinct Neutrophil Functions. Trends Parasitol (2016) 32:392–401. doi: 10.1016/j.pt.2016.02.003
24. Kalyan S, Kabelitz D. When Neutrophils Meet T Cells: Beginnings of a Tumultuous Relationship With Underappreciated Potential. Eur J Immunol (2014) 44:627–33. doi: 10.1002/eji.201344195
25. Borregaard N, Sorensen OE, Theilgaard-Monch K. Neutrophil Granules: A Library of Innate Immunity Proteins. Trends Immunol (2007) 28:340–5. doi: 10.1016/j.it.2007.06.002
26. Yang CW, Unanue ER. Neutrophils Control the Magnitude and Spread of the Immune Response in a Thromboxane A2-Mediated Process. J Exp Med (2013) 210:375–87. doi: 10.1084/jem.20122183
27. Ohms M, Ferreira C, Busch H, Wohlers I, Guerra de Souza AC, Silvestre R, et al. Enhanced Glycolysis Is Required for Antileishmanial Functions of Neutrophils Upon Infection With. Front Immunol (2021) 12:632512. doi: 10.3389/fimmu.2021.632512
28. Mantovani A, Cassatella MA, Costantini C, Jaillon S. Neutrophils in the Activation and Regulation of Innate and Adaptive Immunity. Nat Rev Immunol (2011) 11:519–31. doi: 10.1038/nri3024
29. McFarlane E, Perez C, Charmoy M, Allenbach C, Carter KC, Alexander J, et al. Neutrophils Contribute to Development of a Protective Immune Response During Onset of Infection With Leishmania Donovani. Infect Immun (2008) 76:532–41. doi: 10.1128/IAI.01388-07
30. Almeida BF, Narciso LG, Bosco AM, Pereira PP, Braga ET, Avanco SV, et al. Neutrophil Dysfunction Varies With the Stage of Canine Visceral Leishmaniosis. Vet Parasitol (2013) 196:6–12. doi: 10.1016/j.vetpar.2013.02.016
31. van Zandbergen G, Hermann N, Laufs H, Solbach W, Laskay T. Leishmania Promastigotes Release a Granulocyte Chemotactic Factor and Induce Interleukin-8 Release But Inhibit Gamma Interferon-Inducible Protein 10 Production by Neutrophil Granulocytes. Infect Immun (2002) 70:4177–84. doi: 10.1128/IAI.70.8.4177-4184.2002
32. Wei F, Gong W, Wang J, Yang Y, Liu J, Wang Y, et al. Role of the Lipoxin A4 Receptor in the Development of Neutrophil Extracellular Traps in Leishmania Infantum Infection. Parasit Vectors (2019) 12:275. doi: 10.1186/s13071-019-3530-8
33. Guimaraes-Costa AB, Shannon JP, Waclawiak I, Oliveira J, Meneses C, de Castro W, et al. A Sand Fly Salivary Protein Acts as a Neutrophil Chemoattractant. Nat Commun (2021) 12:3213. doi: 10.1038/s41467-021-23002-5
34. Prates DB, Araújo-Santos T, Luz NF, Andrade BB, França-Costa J, Afonso L, et al. Lutzomyia Longipalpis Saliva Drives Apoptosis and Enhances Parasite Burden in Neutrophils. J Leukoc Biol (2011) 90:575–82. doi: 10.1189/jlb.0211105
35. Mocsai A. Diverse Novel Functions of Neutrophils in Immunity, Inflammation, and Beyond. J Exp Med (2013) 210:1283–99. doi: 10.1084/jem.20122220
36. Carlsen ED, Jie Z, Liang Y, Henard CA, Hay C, Sun J, et al. Interactions Between Neutrophils and Leishmania Braziliensis Amastigotes Facilitate Cell Activation and Parasite Clearance. J Innate Immun (2015) 7:354–63. doi: 10.1159/000373923
37. Ribeiro-Gomes FL, Otero AC, Gomes NA, Moniz-De-Souza MC, Cysne-Finkelstein L, Arnholdt AC, et al. Macrophage Interactions With Neutrophils Regulate Leishmania Major Infection. J Immunol (2004) 172:4454–62. doi: 10.4049/jimmunol.172.7.4454
38. de Souza Carmo EV, Katz S, Barbieri CL. Neutrophils Reduce the Parasite Burden in Leishmania (Leishmania) Amazonensis-Infected Macrophages. PloS One (2010) 5:e13815. doi: 10.1371/journal.pone.0013815
39. Novais FO, Santiago RC, Bafica A, Khouri R, Afonso L, Borges VM, et al. Neutrophils and Macrophages Cooperate in Host Resistance Against Leishmania Braziliensis Infection. J Immunol (2009) 183:8088–98. doi: 10.4049/jimmunol.0803720
40. Afonso L, Borges VM, Cruz H, Ribeiro-Gomes FL, DosReis GA, Dutra AN, et al. Interactions With Apoptotic But Not With Necrotic Neutrophils Increase Parasite Burden in Human Macrophages Infected With Leishmania Amazonensis. J Leukoc Biol (2008) 84:389–96. doi: 10.1189/jlb.0108018
41. Kupani M, Pandey RK, Mehrotra S. Neutrophils and Visceral Leishmaniasis: Impact on Innate Immune Response and Cross-Talks With Macrophages and Dendritic Cells. J Cell Physiol (2021) 236:2255–67. doi: 10.1002/jcp.30029
42. Teixeira CR, Santos CDS, Prates DB, Dos Santos RT, Araújo-Santos T, de Souza-Neto SM, et al. Saliva Drives Interleukin-17-Induced Neutrophil Recruitment Favoring. Front Microbiol (2018) 9:881. doi: 10.3389/fmicb.2018.00881
43. Yang D, de la Rosa G, Tewary P, Oppenheim JJ. Alarmins Link Neutrophils and Dendritic Cells. Trends Immunol (2009) 30:531–7. doi: 10.1016/j.it.2009.07.004
44. Piccard H, Muschel RJ, Opdenakker G. On the Dual Roles and Polarized Phenotypes of Neutrophils in Tumor Development and Progression. Crit Rev Oncol Hematol (2012) 82:296–309. doi: 10.1016/j.critrevonc.2011.06.004
45. Denny MF, Yalavarthi S, Zhao W, Thacker SG, Anderson M, Sandy AR, et al. A Distinct Subset of Proinflammatory Neutrophils Isolated From Patients With Systemic Lupus Erythematosus Induces Vascular Damage and Synthesizes Type I IFNs. J Immunol (2010) 184:3284–97. doi: 10.4049/jimmunol.0902199
46. Di Pilato M, Mejias-Perez E, Zonca M, Perdiguero B, Gomez CE, Trakala M, et al. NFkappaB Activation by Modified Vaccinia Virus as a Novel Strategy to Enhance Neutrophil Migration and HIV-Specific T-Cell Responses. Proc Natl Acad Sci USA (2015) 112:E1333–42. doi: 10.1073/pnas.1424341112
47. Bhattacharya P, Dey R, Saxena A, Karmakar S, Ismail N, Gannavaram S, et al. Essential Role of Neutrophils in the Protective Immune Response Induced by a Live Attenuated Leishmania Vaccine. J Immunol (2020) 205:3333–47. doi: 10.4049/jimmunol.2000829
48. Sharma S, Davis RE, Srivastva S, Nylen S, Sundar S, Wilson ME. A Subset of Neutrophils Expressing Markers of Antigen-Presenting Cells in Human Visceral Leishmaniasis. J Infect Dis (2016) 214:1531–8. doi: 10.1093/infdis/jiw394
49. Abi Abdallah DS, Egan CE, Butcher BA, Denkers EY. Mouse Neutrophils Are Professional Antigen-Presenting Cells Programmed to Instruct Th1 and Th17 T-Cell Differentiation. Int Immunol (2011) 23:317–26. doi: 10.1093/intimm/dxr007
50. Radsak M, Iking-Konert C, Stegmaier S, Andrassy K, Hansch GM. Polymorphonuclear Neutrophils as Accessory Cells for T-Cell Activation: Major Histocompatibility Complex Class II Restricted Antigen-Dependent Induction of T-Cell Proliferation. Immunology (2000) 101:521–30. doi: 10.1046/j.1365-2567.2000.00140.x
51. Vono M, Lin A, Norrby-Teglund A, Koup RA, Liang F, Lore K. Neutrophils Acquire the Capacity for Antigen Presentation to Memory CD4(+) T Cells In Vitro and Ex Vivo. Blood (2017) 129:1991–2001. doi: 10.1182/blood-2016-10-744441
52. Trentini MM, de Oliveira FM, Kipnis A, Junqueira-Kipnis AP. The Role of Neutrophils in the Induction of Specific Th1 and Th17 During Vaccination Against Tuberculosis. Front Microbiol (2016) 7:898. doi: 10.3389/fmicb.2016.00898
53. Duffy D, Perrin H, Abadie V, Benhabiles N, Boissonnas A, Liard C, et al. Neutrophils Transport Antigen From the Dermis to the Bone Marrow, Initiating a Source of Memory CD8+ T Cells. Immunity (2012) 37:917–29. doi: 10.1016/j.immuni.2012.07.015
54. Arts RJ, Joosten LA, Netea MG. Immunometabolic Circuits in Trained Immunity. Semin Immunol (2016) 28:425–30. doi: 10.1016/j.smim.2016.09.002
55. Netea MG, Joosten LA, Latz E, Mills KH, Natoli G, Stunnenberg HG, et al. Trained Immunity: A Program of Innate Immune Memory in Health and Disease. Science (2016) 352:aaf1098. doi: 10.1126/science.aaf1098
56. Moorlag SJCF, Rodriguez-Rosales YA, Gillard J, Fanucchi S, Theunissen K, Novakovic B, et al. BCG Vaccination Induces Long-Term Functional Reprogramming of Human Neutrophils. Cell Rep (2020) 33:108387. doi: 10.1016/j.celrep.2020.108387
57. Beil WJ, Meinardus-Hager G, Neugebauer DC, Sorg C. Differences in the Onset of the Inflammatory Response to Cutaneous Leishmaniasis in Resistant and Susceptible Mice. J Leukoc Biol (1992) 52:135–42. doi: 10.1002/jlb.52.2.135
58. Guilliams M, Mildner A, Yona S. Developmental and Functional Heterogeneity of Monocytes. Immunity (2018) 49:595–613. doi: 10.1016/j.immuni.2018.10.005
59. Terrazas C, Varikuti S, Oghumu S, Steinkamp HM, Ardic N, Kimble J, et al. Ly6C Hi Inflammatory Monocytes Promote Susceptibility to Leishmania Donovani Infection. Sci Rep (2017) 7:14693. doi: 10.1038/s41598-017-14935-3
60. Geissmann F, Jung S, Littman DR. Blood Monocytes Consist of Two Principal Subsets With Distinct Migratory Properties. Immunity (2003) 19:71–82. doi: 10.1016/S1074-7613(03)00174-2
61. Serbina NV, Pamer EG. Monocyte Emigration From Bone Marrow During Bacterial Infection Requires Signals Mediated by Chemokine Receptor CCR2. Nat Immunol (2006) 7:311–7. doi: 10.1038/ni1309
62. de Araújo FF, Costa-Silva MF, Pereira AAS, Rêgo FD, Pereira VHS, de Souza JP, et al. Chemokines in Leishmaniasis: Map of Cell Movements Highlights the Landscape of Infection and Pathogenesis. Cytokine (2021) 147:155339. doi: 10.1016/j.cyto.2020.155339
63. Lestinova T, Rohousova I, Sima M, de Oliveira CI, Volf P. Insights Into the Sand Fly Saliva: Blood-Feeding and Immune Interactions Between Sand Flies, Hosts, and Leishmania. PloS Negl Trop Dis (2017) 11:e0005600. doi: 10.1371/journal.pntd.0005600
64. Biswas A, Bruder D, Wolf SA, Jeron A, Mack M, Heimesaat MM, et al. Ly6C(high) Monocytes Control Cerebral Toxoplasmosis. J Immunol (2015) 194:3223–35. doi: 10.4049/jimmunol.1402037
65. Antonelli LR, Leoratti FM, Costa PA, Rocha BC, Diniz SQ, Tada MS, et al. The CD14+CD16+ Inflammatory Monocyte Subset Displays Increased Mitochondrial Activity and Effector Function During Acute Plasmodium Vivax Malaria. PloS Pathog (2014) 10:e1004393. doi: 10.1371/journal.ppat.1004393
66. Viana AG, Magalhaes LMD, Giunchetti RC, Dutra WO, Gollob KJ. Infection of Human Monocytes With Leishmania Infantum Strains Induces a Downmodulated Response When Compared With Infection With Leishmania Braziliensis. Front Immunol (2017) 8:1896. doi: 10.3389/fimmu.2017.01896
67. Campos TM, Passos ST, Novais FO, Beiting DP, Costa RS, Queiroz A, et al. Matrix Metalloproteinase 9 Production by Monocytes Is Enhanced by TNF and Participates in the Pathology of Human Cutaneous Leishmaniasis. PloS Negl Trop Dis (2014) 8:e3282. doi: 10.1371/journal.pntd.0003282
68. Fromm PD, Kling J, Mack M, Sedgwick JD, Korner H. Loss of TNF Signaling Facilitates the Development of a Novel Ly-6C(Low) Macrophage Population Permissive for Leishmania Major Infection. J Immunol (2012) 188:6258–66. doi: 10.4049/jimmunol.1100977
69. Steinbrink K, Schonlau F, Rescher U, Henseleit U, Vogel T, Sorg C, et al. Ineffective Elimination of Leishmania Major by Inflammatory (MRP14-Positive) Subtype of Monocytic Cells. Immunobiology (2000) 202:442–59. doi: 10.1016/S0171-2985(00)80103-5
70. Roy S, Mukhopadhyay D, Mukherjee S, Ghosh S, Kumar S, Sarkar K, et al. A Defective Oxidative Burst and Impaired Antigen Presentation Are Hallmarks of Human Visceral Leishmaniasis. J Clin Immunol (2015) 35:56–67. doi: 10.1007/s10875-014-0115-3
71. Chandra D, Naik S. Leishmania Donovani Infection Down-Regulates TLR2-Stimulated IL-12p40 and Activates IL-10 in Cells of Macrophage/Monocytic Lineage by Modulating MAPK Pathways Through a Contact-Dependent Mechanism. Clin Exp Immunol (2008) 154:224–34. doi: 10.1111/j.1365-2249.2008.03741.x
72. Oliaee RT, Sharifi I, Afgar A, Jafarzadeh A, Kareshk AT, Bamorovat M, et al. Differential Expression of TLRs 2, 4, 9, iNOS and TNF-α and Arginase Activity in Peripheral Blood Monocytes From Glucantime Unresponsive and Responsive Patients With Anthroponotic Cutaneous Leishmaniasis Caused by Leishmania Tropica. Microb Pathog (2019) 126:368–78. doi: 10.1016/j.micpath.2018.11.004
73. Terrazas C, Varikuti S, Kimble J, Moretti E, Boyaka PN, Satoskar AR. IL-17A Promotes Susceptibility During Experimental Visceral Leishmaniasis Caused by Leishmania Donovani. FASEB J (2016) 30:1135–43. doi: 10.1096/fj.15-277202
74. Roy S, Mukhopadhyay D, Mukherjee S, Moulik S, Chatterji S, Brahme N, et al. An IL-10 Dominant Polarization of Monocytes Is a Feature of Indian Visceral Leishmaniasis. Parasite Immunol (2018) 40:e12535. doi: 10.1111/pim.12535
75. Peruhype-Magalhães V, Martins-Filho OA, Prata A, Silva L, Rabello A, Teixeira-Carvalho A, et al. Immune Response in Human Visceral Leishmaniasis: Analysis of the Correlation Between Innate Immunity Cytokine Profile and Disease Outcome. Scand J Immunol (2005) 62:487–95. doi: 10.1111/j.1365-3083.2005.01686.x
76. Singh N, Kumar R, Chauhan SB, Engwerda C, Sundar S. Peripheral Blood Monocytes With an Antiinflammatory Phenotype Display Limited Phagocytosis and Oxidative Burst in Patients With Visceral Leishmaniasis. J Infect Dis (2018) 218:1130–41. doi: 10.1093/infdis/jiy228
77. Ribeiro CV, Rocha BFB, Oliveira E, Teixeira-Carvalho A, Martins-Filho OA, Murta SMF, et al. Leishmania Infantum Induces High Phagocytic Capacity and Intracellular Nitric Oxide Production by Human Proinflammatory Monocyte. Mem Inst Oswaldo Cruz (2020) 115:e190408. doi: 10.1590/0074-02760190408
78. Costa DL, Lima-Júnior DS, Nascimento MS, Sacramento LA, Almeida RP, Carregaro V, et al. CCR2 Signaling Contributes to the Differentiation of Protective Inflammatory Dendritic Cells in Leishmania Braziliensis Infection. J Leukoc Biol (2016) 100:423–32. doi: 10.1189/jlb.4A0715-288R
79. Abidin BM, Hammami A, Stäger S, Heinonen KM. Infection-Adapted Emergency Hematopoiesis Promotes Visceral Leishmaniasis. PloS Pathog (2017) 13:e1006422. doi: 10.1371/journal.ppat.1006422
80. Hammami A, Abidin BM, Charpentier T, Fabié A, Duguay AP, Heinonen KM, et al. HIF-1α Is a Key Regulator in Potentiating Suppressor Activity and Limiting the Microbicidal Capacity of MDSC-Like Cells During Visceral Leishmaniasis. PloS Pathog (2017) 13:e1006616. doi: 10.1371/journal.ppat.1006616
81. Osorio EY, Medina-Colorado AA, Travi BL, Melby PC. In-Situ Proliferation Contributes to the Accumulation of Myeloid Cells in the Spleen During Progressive Experimental Visceral Leishmaniasis. PloS One (2020) 15:e0242337. doi: 10.1371/journal.pone.0242337
82. Carneiro MB, Lopes ME, Hohman LS, Romano A, David BA, Kratofil R, et al. Th1-Th2 Cross-Regulation Controls Early Leishmania Infection in the Skin by Modulating the Size of the Permissive Monocytic Host Cell Reservoir. Cell Host Microbe (2020) 27:752–68.e7. doi: 10.1016/j.chom.2020.03.011
83. Rosas LE, Snider HM, Barbi J, Satoskar AA, Lugo-Villarino G, Keiser T, et al. Cutting Edge: STAT1 and T-Bet Play Distinct Roles in Determining Outcome of Visceral Leishmaniasis Caused by Leishmania Donovani. J Immunol (2006) 177:22–5. doi: 10.4049/jimmunol.177.1.22
84. Varikuti S, Volpedo G, Saljoughian N, Hamza OM, Halsey G, Ryan NM, et al. The Potent ITK/BTK Inhibitor Ibrutinib Is Effective for the Treatment of Experimental Visceral Leishmaniasis Caused by Leishmania Donovani. J Infect Dis (2019) 219:599–608. doi: 10.1093/infdis/jiy552
85. Glennie ND, Volk SW, Scott P. Skin-Resident CD4+ T Cells Protect Against Leishmania Major by Recruiting and Activating Inflammatory Monocytes. PloS Pathog (2017) 13:e1006349. doi: 10.1371/journal.ppat.1006349
86. Romano A, Carneiro MBH, Doria NA, Roma EH, Ribeiro-Gomes FL, Inbar E, et al. Divergent Roles for Ly6C+CCR2+CX3CR1+ Inflammatory Monocytes During Primary or Secondary Infection of the Skin With the Intra-Phagosomal Pathogen Leishmania Major. PloS Pathog (2017) 13:e1006479. doi: 10.1371/journal.ppat.1006479
87. Soudja SM, Ruiz AL, Marie JC, Lauvau G. Inflammatory Monocytes Activate Memory CD8(+) T and Innate NK Lymphocytes Independent of Cognate Antigen During Microbial Pathogen Invasion. Immunity (2012) 37:549–62. doi: 10.1016/j.immuni.2012.05.029
88. De Koker S, Van Hoecke L, De Beuckelaer A, Roose K, Deswarte K, Willart MA, et al. Inflammatory Monocytes Regulate Th1 Oriented Immunity to CpG Adjuvanted Protein Vaccines Through Production of IL-12. Sci Rep (2017) 7:5986. doi: 10.1038/s41598-017-06236-6
89. Moreira ML, Costa-Pereira C, Alves ML, Marteleto BH, Ribeiro VM, Peruhype-Magalhães V, et al. Vaccination Against Canine Leishmaniosis Increases the Phagocytic Activity, Nitric Oxide Production and Expression of Cell Activation/Migration Molecules in Neutrophils and Monocytes. Vet Parasitol (2016) 220:33–45. doi: 10.1016/j.vetpar.2016.02.009
90. Araújo MS, de Andrade RA, Sathler-Avelar R, Teixeira-Carvalho A, Andrade MC, Vianna LR, et al. T-Cell-Derived Cytokines, Nitric Oxide Production by Peripheral Blood Monocytes and Seric Anti-Leishmania (Leishmania) Chagasi IgG Subclass Patterns Following Immunization Against Canine Visceral Leishmaniasis Using Leishvaccine and Leishmune. Vaccine (2009) 27:1008–17. doi: 10.1016/j.vaccine.2008.11.104
91. Quintin J, Saeed S, Martens JHA, Giamarellos-Bourboulis EJ, Ifrim DC, Logie C, et al. Candida Albicans Infection Affords Protection Against Reinfection via Functional Reprogramming of Monocytes. Cell Host Microbe (2012) 12:223–32. doi: 10.1016/j.chom.2012.06.006
92. Guerrini V, Bruiners N, Gennaro ML. Mtorc1 Links Cellular Metabolism and Immune Functions in Mycobacterium Tuberculosis Infection and BCG Vaccination. Value BCG TNF Autoimmunity Acad Press (2018) pp:155–70. doi: 10.1016/B978-0-12-814603-3.00010-0
93. Dos Santos JC, Barroso de Figueiredo AM, Teodoro Silva MV, Cirovic B, de Bree LCJ, Damen MSMA, et al. β-Glucan-Induced Trained Immunity Protects Against Leishmania Braziliensis Infection: A Crucial Role for IL-32. Cell Rep (2019) 28:2659–72.e6. doi: 10.1016/j.celrep.2019.08.004
94. Khan N, Downey J, Sanz J, Kaufmann E, Blankenhaus B, Pacis A, et al. M. Tuberculosis Reprograms Hematopoietic Stem Cells to Limit Myelopoiesis and Impair Trained Immunity. Cell (2020) 183:752–70.e22. doi: 10.1016/j.cell.2020.09.062
95. van Zandbergen G, Klinger M, Mueller A, Dannenberg S, Gebert A, Solbach W, et al. Cutting Edge: Neutrophil Granulocyte Serves as a Vector for Leishmania Entry Into Macrophages. J Immunol (2004) 173:6521–5. doi: 10.4049/jimmunol.173.11.6521
96. Gregory DJ, Godbout M, Contreras I, Forget G, Olivier M. A Novel Form of NF-kappaB Is Induced by Leishmania Infection: Involvement in Macrophage Gene Expression. Eur J Immunol (2008) 38:1071–81. doi: 10.1002/eji.200737586
97. Teixeira CR, Teixeira MJ, Gomes RB, Santos CS, Andrade BB, Raffaele-Netto I, et al. Saliva From Lutzomyia Longipalpis Induces CC Chemokine Ligand 2/Monocyte Chemoattractant Protein-1 Expression and Macrophage Recruitment. J Immunol (2005) 175:8346–53. doi: 10.4049/jimmunol.175.12.8346
98. Tuon FF, Amato VS, Bacha HA, Almusawi T, Duarte MI, Amato Neto V. Toll-Like Receptors and Leishmaniasis. Infect Immun (2008) 76:866–72. doi: 10.1128/IAI.01090-07
99. Rostami MN, Khamesipour A. Potential Biomarkers of Immune Protection in Human Leishmaniasis. Med Microbiol Immunol (2021) 210:81–100. doi: 10.1007/s00430-021-00703-8
100. Polando R, Dixit UG, Carter CR, Jones B, Whitcomb JP, Ballhorn W, et al. The Roles of Complement Receptor 3 and Fcγ Receptors During Leishmania Phagosome Maturation. J Leukoc Biol (2013) 93:921–32. doi: 10.1189/jlb.0212086
101. Kane MM, Mosser DM. The Role of IL-10 in Promoting Disease Progression in Leishmaniasis. J Immunol (2001) 166:1141–7. doi: 10.4049/jimmunol.166.2.1141
102. Sutterwala FS, Noel GJ, Salgame P, Mosser DM. Reversal of Proinflammatory Responses by Ligating the Macrophage Fcgamma Receptor Type I. J Exp Med (1998) 188:217–22. doi: 10.1084/jem.188.1.217
103. Hawn TR, Ozinsky A, Underhill DM, Buckner FS, Akira S, Aderem A. Leishmania Major Activates IL-1 Alpha Expression in Macrophages Through a MyD88-Dependent Pathway. Microbes Infect (2002) 4:763–71. doi: 10.1016/S1286-4579(02)01596-4
104. Isnard A, Shio MT, Olivier M. Impact of Leishmania Metalloprotease GP63 on Macrophage Signaling. Front Cell Infect Microbiol (2012) 2:72. doi: 10.3389/fcimb.2012.00072
105. Kumar A, Das S, Mandal A, Verma S, Abhishek K, Kumar V, et al. Leishmania Infection Activates Host mTOR for Its Survival by M2 Macrophage Polarization. Parasite Immunol (2018) 40:e12586. doi: 10.1111/pim.12586
106. Saha A, Basu M, Ukil A. Recent Advances in Understanding Leishmania Donovani Infection: The Importance of Diverse Host Regulatory Pathways. IUBMB Life (2018) 70:593–601. doi: 10.1002/iub.1759
107. Bogdan C. Macrophages as Host, Effector and Immunoregulatory Cells in Leishmaniasis: Impact of Tissue Micro-Environment and Metabolism. Cytokine X (2020) 2:100041. doi: 10.1016/j.cytox.2020.100041
108. Italiani P, Boraschi D. From Monocytes to M1/M2 Macrophages: Phenotypical vs. Functional Differentiation. Front Immunol (2014) 5:514. doi: 10.3389/fimmu.2014.00514
109. Loria-Cervera EN, Andrade-Narvaez F. The Role of Monocytes/Macrophages in Leishmania Infection: A Glance at the Human Response. Acta Trop (2020) 207:105456. doi: 10.1016/j.actatropica.2020.105456
111. Bronte V, Zanovello P. Regulation of Immune Responses by L-Arginine Metabolism. Nat Rev Immunol (2005) 5:641–54. doi: 10.1038/nri1668
112. Yang J, Zhang L, Yu C, Yang XF, Wang H. Monocyte and Macrophage Differentiation: Circulation Inflammatory Monocyte as Biomarker for Inflammatory Diseases. Biomark Res (2014) 2:1. doi: 10.1186/2050-7771-2-1
113. Paloque L, Perez-Berezo T, Abot A, Dalloux-Chioccioli J, Bourgeade-Delmas S, Le Faouder P, et al. Polyunsaturated Fatty Acid Metabolites: Biosynthesis in. J Lipid Res (2019) 60:636–47. doi: 10.1194/jlr.M091736
114. Subramanian A, Sarkar RR. Revealing the Mystery of Metabolic Adaptations Using a Genome Scale Model of Leishmania Infantum. Sci Rep (2017) 7:10262. doi: 10.1038/s41598-017-10743-x
115. Saunders EC, Ng WW, Kloehn J, Chambers JM, Ng M, McConville MJ. Induction of a Stringent Metabolic Response in Intracellular Stages of Leishmania Mexicana Leads to Increased Dependence on Mitochondrial Metabolism. PloS Pathog (2014) 10:e1003888. doi: 10.1371/journal.ppat.1003888
116. Saunders EC, Ng WW, Chambers JM, Ng M, Naderer T, Krömer JO, et al. Isotopomer Profiling of Leishmania Mexicana Promastigotes Reveals Important Roles for Succinate Fermentation and Aspartate Uptake in Tricarboxylic Acid Cycle (TCA) Anaplerosis, Glutamate Synthesis, and Growth. J Biol Chem (2011) 286:27706–17. doi: 10.1074/jbc.M110.213553
117. Titos E, Rius B, González-Périz A, López-Vicario C, Morán-Salvador E, Martínez-Clemente M, et al. Resolvin D1 and Its Precursor Docosahexaenoic Acid Promote Resolution of Adipose Tissue Inflammation by Eliciting Macrophage Polarization Toward an M2-Like Phenotype. J Immunol (2011) 187:5408–18. doi: 10.4049/jimmunol.1100225
118. Araújo-Santos T, Andrade BB, Gil-Santana L, Luz NF, Dos Santos PL, de Oliveira FA, et al. Anti-Parasite Therapy Drives Changes in Human Visceral Leishmaniasis-Associated Inflammatory Balance. Sci Rep (2017) 7:4334. doi: 10.1038/s41598-017-04595-8
119. Orecchioni M, Ghosheh Y, Pramod AB, Ley K. Macrophage Polarization: Different Gene Signatures in M1(LPS+) vs. Classically and M2(LPS-) vs. Alternatively Activated Macrophages. Front Immunol (2019) 10:1084. doi: 10.3389/fimmu.2019.01084
120. Van den Bossche J, O'Neill LA, Menon D. Macrophage Immunometabolism: Where Are We (Going)? Trends Immunol (2017) 38:395–406. doi: 10.1016/j.it.2017.03.001
121. Baardman J, Verberk SGS, Prange KHM, van Weeghel M, van der Velden S, Ryan DG, et al. A Defective Pentose Phosphate Pathway Reduces Inflammatory Macrophage Responses During Hypercholesterolemia. Cell Rep (2018) 25:2044–52.e5. doi: 10.1016/j.celrep.2018.10.092
122. Nagy C, Haschemi A. Time and Demand Are Two Critical Dimensions of Immunometabolism: The Process of Macrophage Activation and the Pentose Phosphate Pathway. Front Immunol (2015) 6:164. doi: 10.3389/fimmu.2015.00164
123. Moreira D, Rodrigues V, Abengozar M, Rivas L, Rial E, Laforge M, et al. Leishmania Infantum Modulates Host Macrophage Mitochondrial Metabolism by Hijacking the SIRT1-AMPK Axis. PloS Pathog (2015) 11:e1004684. doi: 10.1371/journal.ppat.1004684
124. Wanasen N, MacLeod CL, Ellies LG, Soong L. L-Arginine and Cationic Amino Acid Transporter 2B Regulate Growth and Survival of Leishmania Amazonensis Amastigotes in Macrophages. Infect Immun (2007) 75:2802–10. doi: 10.1128/IAI.00026-07
125. Carneiro PP, Conceição J, Macedo M, Magalhães V, Carvalho EM, Bacellar O. The Role of Nitric Oxide and Reactive Oxygen Species in the Killing of Leishmania Braziliensis by Monocytes From Patients With Cutaneous Leishmaniasis. PloS One (2016) 11:e0148084. doi: 10.1371/journal.pone.0148084
126. Wei XQ, Charles IG, Smith A, Ure J, Feng GJ, Huang FP, et al. Altered Immune Responses in Mice Lacking Inducible Nitric Oxide Synthase. Nature (1995) 375:408–11. doi: 10.1038/375408a0
127. Bhardwaj S, Srivastava N, Sudan R, Saha B. Leishmania Interferes With Host Cell Signaling to Devise a Survival Strategy. J BioMed Biotechnol (2010) 2010:109189. doi: 10.1155/2010/109189
128. Nandan D, Reiner NE. Attenuation of Gamma Interferon-Induced Tyrosine Phosphorylation in Mononuclear Phagocytes Infected With Leishmania Donovani: Selective Inhibition of Signaling Through Janus Kinases and Stat1. Infect Immun (1995) 63:4495–500. doi: 10.1128/iai.63.11.4495-4500.1995
129. Abu-Dayyeh I, Shio MT, Sato S, Akira S, Cousineau B, Olivier M. Leishmania-Induced IRAK-1 Inactivation Is Mediated by SHP-1 Interacting With an Evolutionarily Conserved KTIM Motif. PloS Negl Trop Dis (2008) 2:e305. doi: 10.1371/journal.pntd.0000305
130. Pathak MK, Yi T. Sodium Stibogluconate Is a Potent Inhibitor of Protein Tyrosine Phosphatases and Augments Cytokine Responses in Hemopoietic Cell Lines. J Immunol (2001) 167:3391–7. doi: 10.4049/jimmunol.167.6.3391
131. Turco SJ, Späth GF, Beverley SM. Is Lipophosphoglycan a Virulence Factor? A Surprising Diversity Between Leishmania Species. Trends Parasitol (2001) 17:223–6. doi: 10.1016/S1471-4922(01)01895-5
132. Ilg T. Lipophosphoglycan Is Not Required for Infection of Macrophages or Mice by Leishmania Mexicana. EMBO J (2000) 19:1953–62. doi: 10.1093/emboj/19.9.1953
133. Nylen S, Sacks D. Interleukin-10 and the Pathogenesis of Human Visceral Leishmaniasis. Trends Immunol (2007) 28:378–84. doi: 10.1016/j.it.2007.07.004
134. Hailu A, van Baarle D, Knol GJ, Berhe N, Miedema F, Kager PA. T Cell Subset and Cytokine Profiles in Human Visceral Leishmaniasis During Active and Asymptomatic or Sub-Clinical Infection With Leishmania Donovani. Clin Immunol (2005) 117:182–91. doi: 10.1016/j.clim.2005.06.015
135. Nylén S, Maurya R, Eidsmo L, Manandhar KD, Sundar S, Sacks D. Splenic Accumulation of IL-10 mRNA in T Cells Distinct From CD4+ CD25+ (Foxp3) Regulatory T Cells in Human Visceral Leishmaniasis. J Exp Med (2007) 204:805–17. doi: 10.1084/jem.20061141
136. Ansari NA, Saluja S, Salotra P. Elevated Levels of Interferon-γ, Interleukin-10, and Interleukin-6 During Active Disease in Indian Kala Azar. Clin Immunol (2006) 119:339–45. doi: 10.1016/j.clim.2006.01.017
137. Kurkjian KM, Mahmutovic AJ, Kellar KL, Haque R, Bern C, Secor WE. Multiplex Analysis of Circulating Cytokines in the Sera of Patients With Different Clinical Forms of Visceral Leishmaniasis. Cytometry Part A (2006) 69:353–8. doi: 10.1002/cyto.a.20256
138. Caldas A, Favali C, Aquino D, Vinhas V, Van Weyenbergh J, Brodskyn C, et al. Balance of IL-10 and Interferon-γ Plasma Levels in Human Visceral Leishmaniasis: Implications in the Pathogenesis. BMC Infect Dis (2005) 5:113. doi: 10.1186/1471-2334-5-113
139. Verma S, Kumar R, Katara GK, Singh LC, Negi NS, Ramesh V, et al. Quantification of Parasite Load in Clinical Samples of Leishmaniasis Patients: IL-10 Level Correlates With Parasite Load in Visceral Leishmaniasis. PloS One (2010) 5:e10107. doi: 10.1371/journal.pone.0010107
140. Sarkar A, Saha P, Mandal G, Mukhopadhyay D, Roy S, Singh SK, et al. Monitoring of Intracellular Nitric Oxide in Leishmaniasis: Its Applicability in Patients With Visceral Leishmaniasis. Cytometry A (2011) 79:35–45. doi: 10.1002/cyto.a.21001
141. Abebe T, Takele Y, Weldegebreal T, Cloke T, Closs E, Corset C, et al. Arginase Activity - a Marker of Disease Status in Patients With Visceral Leishmaniasis in Ethiopia. PloS Negl Trop Dis (2013) 7:e2134. doi: 10.1371/journal.pntd.0002134
142. Stäger S, Alexander J, Carter KC, Brombacher F, Kaye PM. Both Interleukin-4 (IL-4) and IL-4 Receptor Alpha Signaling Contribute to the Development of Hepatic Granulomas With Optimal Antileishmanial Activity. Infect Immun (2003) 71:4804–7. doi: 10.1128/IAI.71.8.4804-4807.2003
143. Lage DP, Ribeiro PAF, Dias DS, Mendonca DVC, Ramos FF, Carvalho LM, et al. A Candidate Vaccine for Human Visceral Leishmaniasis Based on a Specific T Cell Epitope-Containing Chimeric Protein Protects Mice Against Leishmania Infantum Infection. NPJ Vaccines (2020) 5:75. doi: 10.1038/s41541-020-00224-0
144. Tosyali OA, Allahverdiyev A, Bagirova M, Abamor ES, Aydogdu M, Dinparvar S, et al. Nano-Co-Delivery of Lipophosphoglycan With Soluble and Autoclaved Leishmania Antigens Into PLGA Nanoparticles: Evaluation of In Vitro and In Vivo Immunostimulatory Effects Against Visceral Leishmaniasis. Mater Sci Eng C Mater Biol Appl (2021) 120:111684. doi: 10.1016/j.msec.2020.111684
145. Kumar S, Zutshi S, Patidar A, Bodhale N, Roy S, Sarkar A, et al. LmjMAPK10 Offers Protection Against Leishmania Donovani Infection. Parasite Immunol (2020) 42:e12687. doi: 10.1111/pim.12687
146. Petitdidier E, Pagniez J, Pissarra J, Holzmuller P, Papierok G, Vincendeau P, et al. Peptide-Based Vaccine Successfully Induces Protective Immunity Against Canine Visceral Leishmaniasis. NPJ Vaccines (2019) 4:49. doi: 10.1038/s41541-019-0144-2
147. Walker PS, Scharton-Kersten T, Rowton ED, Hengge U, Bouloc A, Udey MC, et al. Genetic Immunization With Glycoprotein 63 cDNA Results in a Helper T Cell Type 1 Immune Response and Protection in a Murine Model of Leishmaniasis. Hum Gene Ther (1998) 9:1899–907. doi: 10.1089/hum.1998.9.13-1899
148. Iijima N, Iwasaki A. T Cell Memory. A Local Macrophage Chemokine Network Sustains Protective Tissue-Resident Memory CD4 T Cells. Science (2014) 346:93–8. doi: 10.1126/science.1257530
149. Kaye PM, Cruz I, Picado A, Van Bocxlaer K, Croft SL. Leishmaniasis Immunopathology-Impact on Design and Use of Vaccines, Diagnostics and Drugs. Semin Immunopathol (2020) 42:247–64. doi: 10.1007/s00281-020-00788-y
150. Birnbaum R, Craft N. Innate Immunity and Leishmania Vaccination Strategies. Dermatol Clin (2011) 29:89–102. doi: 10.1016/j.det.2010.08.014
151. Bhattacharya P, Dey R, Dagur PK, Kruhlak M, Ismail N, Debrabant A, et al. Genetically Modified Live Attenuated Leishmania Donovani Parasites Induce Innate Immunity Through Classical Activation of Macrophages That Direct the Th1 Response in Mice. Infect Immun (2015) 83:3800–15. doi: 10.1128/IAI.00184-15
152. Saiga H, Nieuwenhuizen N, Gengenbacher M, Koehler AB, Schuerer S, Moura-Alves P, et al. The Recombinant BCG Δurec::Hly Vaccine Targets the AIM2 Inflammasome to Induce Autophagy and Inflammation. J Infect Dis (2015) 211:1831–41. doi: 10.1093/infdis/jiu675
153. Rennick LJ, de Vries RD, Carsillo TJ, Lemon K, van Amerongen G, Ludlow M, et al. Live-Attenuated Measles Virus Vaccine Targets Dendritic Cells and Macrophages in Muscle of Nonhuman Primates. J Virol (2015) 89:2192–200. doi: 10.1128/JVI.02924-14
154. Sen S, Roy K, Mukherjee S, Mukhopadhyay R, Roy S. Restoration of Ifnγr Subunit Assembly, Ifnγ Signaling and Parasite Clearance in Leishmania Donovani Infected Macrophages: Role of Membrane Cholesterol. PloS Pathog (2011) 7:e1002229. doi: 10.1371/journal.ppat.1002229
155. Chakraborty D, Banerjee S, Sen A, Banerjee KK, Das P, Roy S. Leishmania Donovani Affects Antigen Presentation of Macrophage by Disrupting Lipid Rafts. J Immunol (2005) 175:3214–24. doi: 10.4049/jimmunol.175.5.3214
156. Ghosh J, Guha R, Das S, Roy S. Liposomal Cholesterol Delivery Activates the Macrophage Innate Immune Arm to Facilitate Intracellular Leishmania Donovani Killing. Infect Immun (2014) 82:607–17. doi: 10.1128/IAI.00583-13
157. Roy K, Mandloi S, Chakrabarti S, Roy S. Cholesterol Corrects Altered Conformation of MHC-II Protein in Leishmania Donovani Infected Macrophages: Implication in Therapy. PloS Negl Trop Dis (2016) 10:e0004710. doi: 10.1371/journal.pntd.0004710
158. Gannavaram S, Bhattacharya P, Siddiqui A, Ismail N, Madhavan S, Nakhasi HL. miR-21 Expression Determines the Early Vaccine Immunity Induced by. Front Immunol (2019) 10:2273. doi: 10.3389/fimmu.2019.02273
159. Feijó D, Tibúrcio R, Ampuero M, Brodskyn C, Tavares N. Dendritic Cells and Leishmania Infection: Adding Layers of Complexity to a Complex Disease. J Immunol Res (2016) 2016:3967436. doi: 10.1155/2016/3967436
160. Geijtenbeek TB, van Vliet SJ, Engering A, Hart BA't, van Kooyk Y. Self- and Nonself-Recognition by C-Type Lectins on Dendritic Cells. Annu Rev Immunol (2004) 22:33–54. doi: 10.1146/annurev.immunol.22.012703.104558
161. Tiburcio R, Nunes S, Nunes I, Rosa Ampuero M, Silva IB, Lima R, et al. Molecular Aspects of Dendritic Cell Activation in Leishmaniasis: An Immunobiological View. Front Immunol (2019) 10:227. doi: 10.3389/fimmu.2019.00227
162. Sacramento L, Trevelin SC, Nascimento MS, Lima-Junior DS, Costa DL, Almeida RP, et al. Toll-Like Receptor 9 Signaling in Dendritic Cells Regulates Neutrophil Recruitment to Inflammatory Foci Following Leishmania Infantum Infection. Infect Immun (2015) 83:4604–16. doi: 10.1128/IAI.00975-15
163. Gorak PM, Engwerda CR, Kaye PM. Dendritic Cells, But Not Macrophages, Produce IL-12 Immediately Following Leishmania Donovani Infection. Eur J Immunol (1998) 28:687–95. doi: 10.1002/(SICI)1521-4141(199802)28:02<687::AID-IMMU687>3.0.CO;2-N
164. Maroof A, Kaye PM. Temporal Regulation of Interleukin-12p70 (IL-12p70) and IL-12-Related Cytokines in Splenic Dendritic Cell Subsets During Leishmania Donovani Infection. Infect Immun (2008) 76:239–49. doi: 10.1128/IAI.00643-07
165. Basu A, Chakrabarti G, Saha A, Bandyopadhyay S. Modulation of CD11C+ Splenic Dendritic Cell Functions in Murine Visceral Leishmaniasis: Correlation With Parasite Replication in the Spleen. Immunology (2000) 99:305–13. doi: 10.1046/j.1365-2567.2000.00939.x
166. Ato M, Maroof A, Zubairi S, Nakano H, Kakiuchi T, Kaye PM. Loss of Dendritic Cell Migration and Impaired Resistance to Leishmania Donovani Infection in Mice Deficient in CCL19 and CCL21. J Immunol (2006) 176:5486–93. doi: 10.4049/jimmunol.176.9.5486
167. Hammami A, Abidin BM, Heinonen KM, Stäger S. HIF-1α Hampers Dendritic Cell Function and Th1 Generation During Chronic Visceral Leishmaniasis. Sci Rep (2018) 8:3500. doi: 10.1038/s41598-018-21891-z
168. Stanley AC, Dalton JE, Rossotti SH, MacDonald KP, Zhou Y, Rivera F, et al. VCAM-1 and VLA-4 Modulate Dendritic Cell IL-12p40 Production in Experimental Visceral Leishmaniasis. PloS Pathog (2008) 4:e1000158. doi: 10.1371/journal.ppat.1000158
169. Varikuti S, Verma C, Holcomb E, Jha BK, Viana A, Maryala R, et al. MicroRNA-21 Deficiency Promotes the Early Th1 Immune Response and Resistance Toward Visceral Leishmaniasis. J Immunol (2021) 207:1322–32. doi: 10.4049/jimmunol.2001099
170. Varikuti S, Verma C, Natarajan G, Oghumu S, Satoskar AR. MicroRNA155 Plays a Critical Role in the Pathogenesis of Cutaneous Leishmania Major Infection by Promoting a Th2 Response and Attenuating Dendritic Cell Activity. Am J Pathol (2021) 191:809–16. doi: 10.1016/j.ajpath.2021.01.012
171. Markikou-Ouni W, Drini S, Bahi-Jaber N, Chenik M, Meddeb-Garnaoui A. Immunomodulatory Effects of Four Leishmania Infantum Potentially Excreted/Secreted Proteins on Human Dendritic Cells Differentiation and Maturation. PloS One (2015) 10:e0143063. doi: 10.1371/journal.pone.0143063
172. Silverman JM, Clos J, Horakova E, Wang AY, Wiesgigl M, Kelly I, et al. Leishmania Exosomes Modulate Innate and Adaptive Immune Responses Through Effects on Monocytes and Dendritic Cells. J Immunol (2010) 185:5011–22. doi: 10.4049/jimmunol.1000541
173. Pearce EJ, Everts B. Dendritic Cell Metabolism. Nat Rev Immunol (2015) 15:18–29. doi: 10.1038/nri3771
174. Gaber T, Strehl C, Buttgereit F. Metabolic Regulation of Inflammation. Nat Rev Rheumatol (2017) 13:267–79. doi: 10.1038/nrrheum.2017.37
175. Krawczyk CM, Holowka T, Sun J, Blagih J, Amiel E, DeBerardinis RJ, et al. Toll-Like Receptor-Induced Changes in Glycolytic Metabolism Regulate Dendritic Cell Activation. Blood (2010) 115:4742–9. doi: 10.1182/blood-2009-10-249540
176. Everts B, Amiel E, Huang SC, Smith AM, Chang CH, Lam WY, et al. TLR-Driven Early Glycolytic Reprogramming via the Kinases TBK1-IKKε Supports the Anabolic Demands of Dendritic Cell Activation. Nat Immunol (2014) 15:323–32. doi: 10.1038/ni.2833
177. Jantsch J, Chakravortty D, Turza N, Prechtel AT, Buchholz B, Gerlach RG, et al. Hypoxia and Hypoxia-Inducible Factor-1 Alpha Modulate Lipopolysaccharide-Induced Dendritic Cell Activation and Function. J Immunol (2008) 180:4697–705. doi: 10.4049/jimmunol.180.7.4697
178. Hammami A, Charpentier T, Smans M, Stäger S. IRF-5-Mediated Inflammation Limits CD8+ T Cell Expansion by Inducing HIF-1α and Impairing Dendritic Cell Functions During Leishmania Infection. PloS Pathog (2015) 11:e1004938. doi: 10.1371/journal.ppat.1004938
179. Mesquita I, Ferreira C, Moreira D, Kluck GEG, Barbosa AM, Torrado E, et al. The Absence of HIF-1α Increases Susceptibility to Leishmania Donovani Infection via Activation of BNIP3/mTOR/SREBP-1c Axis. Cell Rep (2020) 30:4052–64.e7. doi: 10.1016/j.celrep.2020.02.098
180. Moll H. Antigen Delivery by Dendritic Cells. Int J Med Microbiol (2004) 294:337–44. doi: 10.1016/j.ijmm.2004.03.003
181. Fajardo-Moser M, Berzel S, Moll H. Mechanisms of Dendritic Cell-Based Vaccination Against Infection. Int J Med Microbiol (2008) 298:11–20. doi: 10.1016/j.ijmm.2007.07.003
182. Remer KA, Apetrei C, Schwarz T, Linden C, Moll H. Vaccination With Plasmacytoid Dendritic Cells Induces Protection Against Infection With Leishmania Major in Mice. Eur J Immunol (2007) 37:2463–73. doi: 10.1002/eji.200636780
183. Agallou M, Pantazi E, Tsiftsaki E, Toubanaki DK, Gaitanaki C, Smirlis D, et al. Induction of Protective Cellular Immune Responses Against Experimental Visceral Leishmaniasis Mediated by Dendritic Cells Pulsed With the N-Terminal Domain of Leishmania Infantum Elongation Factor-2 and CpG Oligodeoxynucleotides. Mol Immunol (2018) 103:7–20. doi: 10.1016/j.molimm.2018.08.004
184. Banerjee A, Bhattacharya P, Dagur PK, Karmakar S, Ismail N, Joshi AB, et al. Live Attenuated Leishmania Donovani Centrin Gene-Deleted Parasites Induce IL-23-Dependent IL-17-Protective Immune Response Against Visceral Leishmaniasis in a Murine Model. J Immunol (2018) 200:163–76. doi: 10.4049/jimmunol.1700674
185. Nico D, Martins Almeida F, Maria Motta J, Soares Dos Santos Cardoso F, Freire-de-Lima CG, Freire-de-Lima L, et al. NH36 and F3 Antigen-Primed Dendritic Cells Show Preserved Migrating Capabilities and CCR7 Expression and F3 Is Effective in Immunotherapy of Visceral Leishmaniasis. Front Immunol (2018) 9:967. doi: 10.3389/fimmu.2018.00967
186. Naqvi N, Ahuja K, Selvapandiyan A, Dey R, Nakhasi H, Puri N. Role of Mast Cells in Clearance of Leishmania Through Extracellular Trap Formation. Sci Rep (2017) 7:13240. doi: 10.1038/s41598-017-12753-1
187. Abraham SN, Malaviya R. Mast Cells in Infection and Immunity. Infect Immun (1997) 65:3501–8. doi: 10.1128/iai.65.9.3501-3508.1997
188. Galli SJ, Maurer M, Lantz CS. Mast Cells as Sentinels of Innate Immunity. Curr Opin Immunol (1999) 11:53–9. doi: 10.1016/S0952-7915(99)80010-7
189. Reiner SL, Locksley RM. The Regulation of Immunity to Leishmania Major. Annu Rev Immunol (1995) 13:151–77. doi: 10.1146/annurev.iy.13.040195.001055
190. Weber A, Knop J, Maurer M. Pattern Analysis of Human Cutaneous Mast Cell Populations by Total Body Surface Mapping. Br J Dermatol (2003) 148:224–8. doi: 10.1046/j.1365-2133.2003.05090.x
191. von Stebut E, Metz M, Milon G, Knop J, Maurer M. Early Macrophage Influx to Sites of Cutaneous Granuloma Formation Is Dependent on MIP-1alpha /Beta Released From Neutrophils Recruited by Mast Cell-Derived TNFalpha. Blood (2003) 101:210–5. doi: 10.1182/blood-2002-03-0921
192. Maurer M, Lopez Kostka S, Siebenhaar F, Moelle K, Metz M, Knop J, et al. Skin Mast Cells Control T Cell-Dependent Host Defense in Leishmania Major Infections. FASEB J (2006) 20:2460–7. doi: 10.1096/fj.06-5860com
193. Dey R, Natarajan G, Bhattacharya P, Cummings H, Dagur PK, Terrazas C, et al. Characterization of Cross-Protection by Genetically Modified Live-Attenuated Leishmania Donovani Parasites Against Leishmania Mexicana. J Immunol (2014) 193:3513–27. doi: 10.4049/jimmunol.1303145
194. Naqvi N, Srivastava R, Naskar P, Puri N. Mast Cells Modulate Early Responses to Mycobacterium Bovis Bacillus Calmette-Guerin by Phagocytosis and Formation of Extracellular Traps. Cell Immunol (2021) 365:104380. doi: 10.1016/j.cellimm.2021.104380
195. Fang Y, Xiang Z. Roles and Relevance of Mast Cells in Infection and Vaccination. J BioMed Res (2016) 30:253–63. doi: 10.7555/JBR.30.20150038
196. Willows S, Kulka M. Harnessing the Power of Mast Cells in Unconventional Immunotherapy Strategies and Vaccine Adjuvants. Cells (2020) 9:2713. doi: 10.3390/cells9122713
197. Huang B, Huang S, Chen Y, Zheng H, Shen J, Lun ZR, et al. Mast Cells Modulate Acute Toxoplasmosis in Murine Models. PloS One (2013) 8:e77327. doi: 10.1371/journal.pone.0077327
198. Meuser-Batista M, Corrêa JR, Carvalho VF, de Carvalho Britto CF, Moreira OC, Batista MM, et al. Mast Cell Function and Death in Trypanosoma Cruzi Infection. Am J Pathol (2011) 179:1894–904. doi: 10.1016/j.ajpath.2011.06.014
199. Fu Z, Akula S, Thorpe M, Hellman L. Highly Selective Cleavage of TH2-Promoting Cytokines by the Human and the Mouse Mast Cell Tryptases, Indicating a Potent Negative Feedback Loop on TH2 Immunity. Int J Mol Sci (2019) 20:5147. doi: 10.3390/ijms20205147
200. Bjorkstrom NK, Strunz B, Ljunggren HG. Natural Killer Cells in Antiviral Immunity. Nat Rev Immunol (2021) 1–12. doi: 10.1038/s41577-021-00558-3
201. Rai AK, Thakur CP, Kumar P, Saini S, Kureel AK, Kumari S, et al. Decrease in the Frequency of Circulating CD56(+)CD161(+) NK Cells in Human Visceral Leishmaniasis. Immunol Invest (2018) 47:125–34. doi: 10.1080/08820139.2017.1402925
202. Becker I, Salaiza N, Aguirre M, Delgado J, Carrillo-Carrasco N, Kobeh LG, et al. Leishmania Lipophosphoglycan (LPG) Activates NK Cells Through Toll-Like Receptor-2. Mol Biochem Parasitol (2003) 130:65–74. doi: 10.1016/S0166-6851(03)00160-9
203. Liese J, Schleicher U, Bogdan C. TLR9 Signaling Is Essential for the Innate NK Cell Response in Murine Cutaneous Leishmaniasis. Eur J Immunol (2007) 37:3424–34. doi: 10.1002/eji.200737182
204. Fernandez-Figueroa EA, Imaz-Rosshandler I, Castillo-Fernandez JE, Miranda-Ortiz H, Fernandez-Lopez JC, Becker I, et al. Down-Regulation of TLR and JAK/STAT Pathway Genes Is Associated With Diffuse Cutaneous Leishmaniasis: A Gene Expression Analysis in NK Cells From Patients Infected With Leishmania Mexicana. PloS Negl Trop Dis (2016) 10:e0004570. doi: 10.1371/journal.pntd.0004570
205. Pereira LI, Dorta ML, Pereira AJ, Bastos RP, Oliveira MA, Pinto SA, et al. Increase of NK Cells and Proinflammatory Monocytes Are Associated With the Clinical Improvement of Diffuse Cutaneous Leishmaniasis After Immunochemotherapy With BCG/Leishmania Antigens. Am J Trop Med Hyg (2009) 81:378–83. doi: 10.4269/ajtmh.2009.81.378
206. Satoskar AR, Stamm LM, Zhang X, Satoskar AA, Okano M, Terhorst C, et al. Mice Lacking NK Cells Develop an Efficient Th1 Response and Control Cutaneous Leishmania Major Infection. J Immunol (1999) 162:6747–54.
207. Kirkpatrick CE, Farrell JP. Leishmaniasis in Beige Mice. Infect Immun (1982) 38:1208–16. doi: 10.1128/iai.38.3.1208-1216.1982
208. Bogdan C. Natural Killer Cells in Experimental and Human Leishmaniasis. Front Cell Infect Microbiol (2012) 2:69. doi: 10.3389/fcimb.2012.00069
209. Laabs EM, Wu W, Mendez S. Vaccination With Live Leishmania Major and CpG DNA Promotes Interleukin-2 Production by Dermal Dendritic Cells and NK Cell Activation. Clin Vaccine Immunol (2009) 16:1601–6. doi: 10.1128/CVI.00249-09
210. Kleinnijenhuis J, Quintin J, Preijers F, Joosten LA, Jacobs C, Xavier RJ, et al. BCG-Induced Trained Immunity in NK Cells: Role for Non-Specific Protection to Infection. Clin Immunol (2014) 155:213–9. doi: 10.1016/j.clim.2014.10.005
211. Hammer Q, Romagnani C. About Training and Memory: NK-Cell Adaptation to Viral Infections. Adv Immunol (2017) 133:171–207. doi: 10.1016/bs.ai.2016.10.001
212. Krijgsman D, Hokland M, Kuppen PJK. The Role of Natural Killer T Cells in Cancer-A Phenotypical and Functional Approach. Front Immunol (2018) 9:367. doi: 10.3389/fimmu.2018.00367
213. Amprey JL, Im JS, Turco SJ, Murray HW, Illarionov PA, Besra GS, et al. A Subset of Liver NK T Cells Is Activated During Leishmania Donovani Infection by CD1d-Bound Lipophosphoglycan. J Exp Med (2004) 200:895–904. doi: 10.1084/jem.20040704
Keywords: innate immunity, vaccine, metabolic regulation, visceral leishmaniasis, live attenuated leishmania vaccines, trained immunity, metabolomics, immune-regulation
Citation: Volpedo G, Pacheco-Fernandez T, Bhattacharya P, Oljuskin T, Dey R, Gannavaram S, Satoskar AR and Nakhasi HL (2021) Determinants of Innate Immunity in Visceral Leishmaniasis and Their Implication in Vaccine Development. Front. Immunol. 12:748325. doi: 10.3389/fimmu.2021.748325
Received: 27 July 2021; Accepted: 24 September 2021;
Published: 12 October 2021.
Edited by:
Esther Von Stebut, University of Cologne, GermanyReviewed by:
Pedro Cecílio, National Institute of Allergy and Infectious Diseases (NIH), United StatesDavid M. Mosser, University of Maryland, College Park, United States
Copyright © 2021 Volpedo, Pacheco-Fernandez, Bhattacharya, Oljuskin, Dey, Gannavaram, Satoskar and Nakhasi. This is an open-access article distributed under the terms of the Creative Commons Attribution License (CC BY). The use, distribution or reproduction in other forums is permitted, provided the original author(s) and the copyright owner(s) are credited and that the original publication in this journal is cited, in accordance with accepted academic practice. No use, distribution or reproduction is permitted which does not comply with these terms.
*Correspondence: Hira L. Nakhasi, aGlyYS5uYWtoYXNpQGZkYS5oaHMuZ292; Sreenivas Gannavaram, U3JlZW5pdmFzLmdhbm5hdmFyYW1AZmRhLmhocy5nb3Y=; Abhay R. Satoskar, QWJoYXkuc2F0b3NrYXJAb3N1bWMuZWR1
†These authors have contributed equally to this work