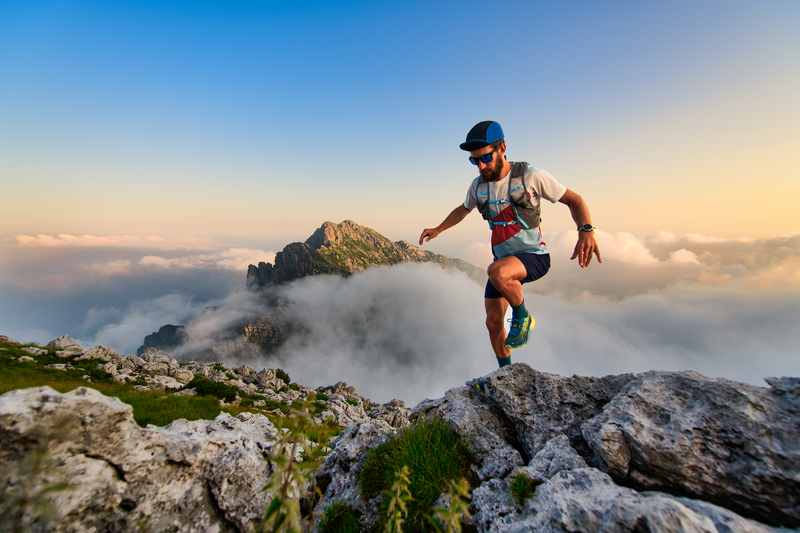
95% of researchers rate our articles as excellent or good
Learn more about the work of our research integrity team to safeguard the quality of each article we publish.
Find out more
REVIEW article
Front. Immunol. , 01 October 2021
Sec. Cytokines and Soluble Mediators in Immunity
Volume 12 - 2021 | https://doi.org/10.3389/fimmu.2021.738697
The severe respiratory consequences of the coronavirus disease 2019 (COVID-19) pandemic have prompted the urgent need for novel therapies. Cell-based therapies, primarily using mesenchymal stromal cells (MSCs), have demonstrated safety and potential efficacy in the treatment of critical illness, particularly sepsis and acute respiratory distress syndrome (ARDS). However, there are limited preclinical data for MSCs in COVID-19. Recent studies have shown that MSCs could decrease inflammation, improve lung permeability, enhance microbe and alveolar fluid clearance, and promote lung epithelial and endothelial repair. In addition, MSC-based therapy has shown promising effects in preclinical studies and phase 1 clinical trials in sepsis and ARDS. Here, we review recent advances related to MSC-based therapy in the context of sepsis and ARDS and evaluate the potential value of MSCs as a therapeutic strategy for COVID-19.
The coronavirus disease 2019 (COVID-19) pandemic, caused by severe acute respiratory syndrome coronavirus 2 (SARS-CoV-2), is rapidly and continuously spreading globally and can result in serious significant respiratory morbidity and mortality (1, 2). Although most patients with COVID-19 present with mild respiratory tract infection, severe pneumonia and acute respiratory distress syndrome (ARDS) have been described in 19% of reported cases, and the overall mortality is approximately 49% (3). The most common symptoms of SARS-CoV-2 infection are cough, fever, fatigue, headache, myalgia, and diarrhea. Approximately 1 week after the onset of symptoms, patients become severely ill when dyspnea and hypoxemia appear, and progressive respiratory failure subsequently develops (4). The main reason for severe COVID-19 disease is aberrant immune host response accompanied by a “cytokine storm.” More precisely, it involves the excessive activation and dysregulation of CD8+ T cells and immoderate pulmonary recruitment of immune cells, as well as the overproduction of pro-inflammatory cytokines such as interleukin 2 (IL-2), IL-6, IL-7, and tumor necrosis factor (TNF) (5). Additionally, endothelial dysfunction and immunothrombosis are also regarded as critical pathogenic mechanisms leading to severe COVID-19 (6) (Figure 1).
Figure 1 Mechanisms of lung injury in COVID-19 and potential therapeutic effects of MSCs in COVID-19-related respiratory lung injury. The mechanisms of lung injury in COVID-19 include, but are not limited to, aberrant immune host responses accompanied by a “cytokine storm,” excessive neutrophil activation, dysregulation of immune cells, endothelial dysfunction, and immunothrombosis formation. The potential therapeutic effects of MSCs in COVID-19 involve multiple mechanisms via their secretion of soluble paracrine factors and possible mitochondrial transfer. MSCs can enhance microbe clearance, resolve inflammation, modulate immunity, improve lung permeability, increase alveolar fluid clearance, and promote lung epithelial and endothelial repair. SARS-CoV-2, severe acute respiratory syndrome coronavirus 2; MSCs, mesenchymal stromal cells; ATI, alveolar type 1 cells; ATII, alveolar type 2 cells; TNF, tumor necrosis factor; IL, interleukin; ACE2, angiotensin-converting enzyme 2; PGE2, prostaglandin E2; KGF, keratinocyte growth factor; TSG-6, TNF-stimulated gene 6; HGF, hepatocyte growth factor; EGF, epidermal growth factor; EVs, extracellular vesicles; NETs, neutrophil extracellular traps; RBCs, red blood cells.
Without effective antiviral medications, current therapeutic approaches are limited to aggressive standard supportive care and treatment of any other co-infections. More recently, a growing number of clinical investigations of cell-based therapies, primarily involving mesenchymal stromal cells (MSCs), have demonstrated safety and possible efficacy in the treatment of critical illness, particularly in sepsis and ARDS (7–15). Recent preclinical data in models of sepsis/ARDS and relevant related clinical studies of MSC administration in patients with ARDS may contribute to a better understanding of the potential MSC-based cell therapy approaches for COVID-19. Therefore, our review focused on MSCs as a potential therapeutic agent for COVID-19 on the basis of the current evidence from sepsis and ARDS.
MSCs were originally characterized in bone marrow stromal cells and are now the best-described and most widely used cells for cell-based therapies. According to the International Society of Cellular Therapy, MSCs are defined by the following minimal criteria: 1) must be adherent to plastic; 2) must positively present CD105, CD90, and CD73 and must be lacking CD45, CD34, CD14, CD11b, CD79 alpha, CD19, and HLA-DR surface makers; and 3) must have a differentiation potential in in vitro conditions (16).
MSCs were originally identified in the bone marrow, and the most recent studies have focused on the effects of bone marrow-derived MSCs. However, MSCs can also be isolated from several tissues such as umbilical cord/fetal blood, placenta, amniotic fluid, and adipose tissue (17–21). Recent studies on rodent models have shown that adipose tissue-derived MSCs minimized ischemia–reperfusion lung injury by suppressing oxidative stress and inflammatory reaction (22); improved phagocytosis and the bactericidal functions of macrophages in a model of Pseudomonas aeruginosa pneumonia (23); attenuated radiation-induced lung injury through anti-inflammation, anti-fibrosis, and anti-apoptosis mechanisms (24); and abrogated bleomycin-induced lung fibrosis by significantly reducing collagen deposition at an early stage (25). Moreover, it was also reported that umbilical cord-derived MSCs can ameliorate hyperoxia-induced lung injury in newborn rats with bronchopulmonary dysplasia and improve the bacterial clearance and survival in neonatal septic rats (26, 27).
There are many advantages of MSCs as an attractive therapeutic candidate. Firstly, they express low levels of human leukocyte antigen (HLA)-associated proteins, which lead to the absence of immunosuppression by allogeneic MSCs as stimulators (28). Secondly, MSCs can be easily isolated from host tissue and expanded stably and rapidly in vitro, which enables sufficient quantities for clinical use (29). Thirdly, the homing capacity of MSCs to the injury sites contributes to their persistence in the target tissues (30). Moreover, MSCs can be trapped in the lungs by systemic administration, thus prolonging their persistence in the lungs, which may be of most benefit in lung disease treatment (30). Fourthly, the safety profile of MSCs was demonstrated by phase 1 clinical trials in patients with ARDS and sepsis (7–15). Finally, growing evidence shows that MSCs exert anti-inflammatory and anti-apoptotic effects in response to injury (31).
Paracrine secretion is a well-known mechanism for MSCs to exhibit their potential benefits (32). A paracrine mechanism of the small mediators secreted by MSCs was suggested to exert the effects of immune modulation, inflammation resolution, and injury restoration. Subsequent studies revealed that several paracrine factors are involved in MSC secretomes, including LL-37 (antimicrobial peptides) (33), keratinocyte growth factor (KGF, enhanced alveolar fluid clearance) (34, 35), angiopoietin-1 (Ang-1, restored epithelial cell permeability) (36), hepatocyte growth factor (HGF, restored endothelial cell permeability) (37–39), and lipoxin A4 (LXA4, alleviated inflammation) (36). For example, in an in vitro experiment, conditioned medium (CM) from Escherichia coli-stimulated MSCs, compared with unstimulated MSCs, showed remarkable antimicrobial activity against E. coli and P. aeruginosa, followed by a significantly higher level of LL-37 being observed in MSCs after bacterial challenge. However, E. coli-stimulated MSCs pre-incubated with the anti-LL-37 antibody resulted in a decrease in bacterial clearance. A subsequent in vivo experiment also displayed a consistent result, suggesting that the antimicrobial activity of MSCs is mainly attributed to the secretion of the antimicrobial peptide LL-37 (33). In addition, a study by Wang et al. (38) reported that the ability of bone marrow-derived MSCs to stabilize the endothelial barrier was partly affected by HGF. Lipopolysaccharide (LPS)-induced endothelial paracellular and transcellular permeabilities were improved after treatment with MSC macrovesicles (MSC-MVs), accompanied by more expressions of the endothelial intercellular junction proteins VE-cadherin and occludin and less endothelial apoptosis. However, these effects were removed after HGF gene knockdown in MSC-MVs. Carrying plenty of cytokines with specific function, MSCs were shown to be of great benefit to the resolution of inflammation and the repair of acute lung injury (ALI).
Mitochondrial transfer is also considered as one of the important mechanisms underlying the protective effects of MSCs (40). MSCs can aid the repair of lung injury through the transfer of mitochondria, which results in increased alveolar ATP concentration (41). Moreover, mitochondrial transfer from MSCs to alveolar macrophages leads to the enhancement of phagocytic activity, thus exerting antimicrobial effects in ARDS (42). Additionally, MSCs can promote an anti-inflammatory and phagocytic macrophage phenotype via extracellular vesicle-mediated mitochondrial transfer (43). Finally, MSCs can donate cytoplasmic content and mitochondria to repair damaged bronchial epithelial cells (44). However, the microenvironment is crucial for effective MSC therapy. It was reported that hypercapnic acidosis induces mitochondrial dysfunction and impairs the ability of MSCs to promote distal lung epithelial repair (45, 46), which may lead to the invalidation of MSCs in patients who develop hypercapnia. Another study further illustrated that the administration of MSC extracellular vesicles with dysfunctional mitochondria had no effect in attenuating LPS-induced ALI, whereas normal MSC extracellular vesicles were able to restore mitochondrial respiration and improve barrier integrity (47).
In COVID-19 patients who develop acute respiratory failure requiring intubation and mechanical ventilation, sepsis and sepsis shock can also develop. Sepsis is a systemic inflammatory response to infection that is characterized by the imbalance between pro-inflammation and anti-inflammation (48, 49). Recently, MSCs have been shown to be effective in modulating such processes, which may be applicable to COVID-19.
Sepsis is a multifaceted host response to an infecting pathogen. The clearance of bacteria with antibiotics is one of the most important treatments for sepsis (50, 51). Beyond antibiotics, MSCs were recently reported to play a crucial role in clearing bacteria in sepsis (52, 53). In in vivo experimental models of sepsis, MSC delivery was shown to be significantly greater in the clearance of bacteria via enhancing the phagocytotic activity of neutrophils, monocytes, and macrophages (54–56). In addition, MSCs could secrete antimicrobial factors such as LL-37 (33, 57) and lipocalin 2 (53) to reduce bacterial growth (in colony forming units). This bactericidal effect may be the result of the enhancement of NOX2-dependent reactive oxygen species (ROS) production by MSCs (55). Furthermore, MSC extracellular vesicles suppressed the activity of multidrug resistance-associated protein 1 (MRP1) through the transfer of miR-145, thereby resulting in antimicrobial activity in E. coli-induced pneumonia in C57BL/6 mice through LTB4/BLT1 signaling (58).
Aside from bacterial infection in sepsis, viral infection is an independent risk factor for sepsis and septic shock (59, 60). However, the antiviral effect of MSCs remains controversial in preclinical studies. MSCs are susceptible to H1N1, H5N1, and H9N5 avian influenza virus, which may lead to cell death and induce the immune dysregulation of MSCs by releasing high levels of TNF-α, IL-6, MCP-1, and MIP-1β (61–63). Moreover, MSC therapy was shown to prolong the disruption of the alveolar–capillary barrier and failed to improve outcomes in experimental severe influenza in mice (64, 65). In contrast, more recent studies of MSCs have reported their protective effects in influenza virus-induced ALI in mice (66–69). The inconsistent results on the antiviral effects of MSCs may have been dependent on the different types of influenza virus and MSCs, animal models, ages, and the methods of administration. A study by Michael et al. showed that MSC treatment was beneficial in aged mice (8–12 months of age), but not in young mice (6–8 weeks of age) with H5N1 influenza virus infection (66). To avoid infection of MSCs by the influenza virus, Mahesh et al. applied MSCs derived from extracellular vesicles for the treatment of influenza-infected pigs, which showed great benefits in attenuating ALI and may provide a cell-free therapeutic strategy for influenza in humans (68). Loy et al. reported that umbilical cord MSCs (UC-MSCs) with greater growth factor secretion of Ang-1 and HGF outperformed bone marrow-derived MSCs in restoring the impaired alveolar fluid clearance and protein permeability of H5N1-infected alveolar epithelial cells (69). Although SARS-CoV-2, a coronavirus, may differ from influenza virus, the use of MSCs in COVID-19 should be fully considered according to the double-edged sword of MSC application in influenza.
Fungal causes of sepsis have increased rapidly worldwide (59, 70). However, there remains a lack of information regarding MSC treatment in fungal infection. It was reported that a subset of single colony-derived MSCs producing IL-17 was capable of inhibiting the growth of Candida albicans both in vitro and in vivo (71). Thus, the potential antifungal effect of MSCs for sepsis and ALI warrants rapid investigation.
The reduction and resolution of inflammation is of vital importance in sepsis. MSCs seem to be promising in this respect. It was reported that MSC administration contributed to a reduction in the pro-inflammatory responses (TNF-α, MIP-2, and IL-6) to endotoxins while upregulating the anti-inflammatory cytokine IL-10 (72–78). A network analysis of transcriptional responses showed that more than 4,000 genes were significantly altered in MSC-treated mice, which indicated that the protective effect of MSCs in sepsis was not limited to a single mediator (79). Of these, TNF-α stimulated gene 6 (TSG-6) may serve as a potential biomarker to predict the efficacy of MSCs in modulating inflammation because MSCs with high levels of TSG-6 exert an enhanced anti-inflammatory efficacy (80–82). However, MSCs are highly sensitive to their microenvironment (46). Under excessive inflammatory circumstances, they may undergo apoptosis through inflammation-induced autophagy (83). Conversely, MSC-conditioned media with an anti-IL6 antibody was more effective in augmenting the promotion of an anti-inflammatory monocyte phenotype (84). In addition, MSCs could restrict NLRP3 inflammasome activation, which suppressed the generation of mitochondrial ROS (85). Moreover, MSC-conditioned media contributed to a reduction in the activity of NF-kB and MMP-9 in neutrophils from patients with sepsis-related ARDS, thus exhibiting great potential in the treatment of inflammatory disorders (86).
Immunological dysfunction is also an important clinical manifestation of sepsis and septic shock (87, 88). MSCs were shown to possess immunomodulatory functions in sepsis. MSCs expressing toll-like receptor 4 (TLR4) and myeloid differentiation primary response gene-88 (MyD88) are essential for releasing prostaglandin E2 (PGE2), which can reprogram macrophages to increase the production of IL-10 (89, 90). Along with the prostaglandin E2 receptor, PGE2 is indispensable to optimal CD4+ T-cell activation and T-cell-mediated inflammatory responses (91). When PGE2 synthesis of human MSCs was inhibited, the MSC-mediated immunosuppressive effects on T cells were also negated (92). In this respect, MSCs may be useful in COVID-19 management where aberrant immune activation plays an important role in the disease progression (5). Moreover, circulating CD3+CD4+CD25+ regulatory T cells (Tregs) were significantly increased when MSCs were administered in a cecal ligation and puncture (CLP)-induced sepsis rat model (93). Furthermore, the immunosuppressive capacity of Tregs can inhibit the production of pro-inflammatory cytokines such as IL-6 and TNF-α (93, 94). In addition, MSCs exhibited immunosuppressive effects by reducing the level of immunoglobulin production and chemokines that are related to recruiting neutrophils in lung B cells (95). When IL-1β was pretreated, the immunomodulatory properties of MSCs could be effectively enhanced (96). MSC-mediated immunomodulation during sepsis was likely to be associated with the MyD88–NFκB signaling pathway (74).
Characterized by the acute onset of non-cardiogenic pulmonary edema, hypoxemia, and diffuse alveolar–capillary membrane damage, ARDS is a common cause of respiratory failure in critically ill patients (97). Recently, a growing number of studies have suggested that MSCs may be a potential therapy for ARDS by improving lung permeability, increasing alveolar fluid clearance, and promoting lung epithelial and endothelial repair.
Epithelial injury is critical in the development of ALI. Alveolar epithelial cells may be the first to be affected by pulmonary ARDS (caused by pneumonia, acid aspiration, and toxic gas inhalation), which may contribute to the loss of the alveolar epithelial barrier integrity and thus increase lung permeability (98). MSCs were reported to show benefits in improving lung epithelial permeability, such as restoring the permeability of alveolar type II epithelial cells through the secretion of Ang-1 (36). Additionally, sodium transport and epithelial permeability were recovered by conditioned media from MSCs via the secretion of KGF in an in vitro model of acute alveolar injury (99), while the intrapulmonary delivery of MSCs contributed to significantly decreasing the levels of bronchoalveolar lavage protein and improving the alveolar epithelial permeability in endotoxin-induced ALI in mice (72). The improvement in alveolar epithelial permeability by MSCs may be a result of the activation of Wnt3a-induced Wnt/β-catenin signaling (100).
Similarly, pulmonary vascular endothelial cells may be the first to be injured by extrapulmonary ARDS (resulting from sepsis, trauma, or hemorrhagic shock), which may lead to dysfunction of the pulmonary vascular endothelial barrier and, thus, increase lung permeability (101). It was reported that MSCs inhibit pulmonary vascular endothelial permeability by preserving critical vascular endothelial barrier proteins (VE-cadherin, occludin-1, and claudin-1) in the lungs after hemorrhagic shock (102, 103). Adipose-derived stem cells also attenuated pulmonary microvascular hyperpermeability in a sheep model of smoke inhalation-induced ALI (104). MSC treatment for endotoxin-induced lung injury improves the lung endothelial barrier permeability partly by secreting KGF and HGF (37, 38, 105). The capacity of MSCs to inhibit vascular permeability is likely through the modulation of VE-cadherin/β-catenin signaling (106).
Alveolar fluid clearance is usually impaired in patients with ARDS (107) and contributes to lung edema and hypoxemia. MSC treatments were reported to play a significant role in clearing alveolar fluid. In an in vivo mouse model of E. coli endotoxin-induced ALI, bone marrow-derived MSCs were shown to reduce extravascular lung water by 60% and total protein levels by 66% (108). In a sheep model of ALI, MSCs also attenuated the lung water content and edema (104, 109). In an ex vivo perfused human lung endotoxin-induced ALI model, MSCs increased the alveolar fluid clearance partly by restoring sodium transport (105). The above benefits of MSC treatments may have originated from the paracrine signaling of KGF, which contributed to restoring the effects of the α-epithelial sodium channel (α-ENaC) (99, 110, 111).
The promotion of lung epithelial and endothelial cell repair is of crucial importance in ARDS treatment. MSC-based therapy may be the breaking point in lung repair and regeneration. MSC therapy enhances pulmonary epithelial wound healing and restores lung structure by secreting KGF in ventilator-induced lung injury (34). MSCs can also improve the viability of BEAS-2B cells and inhibit LPS-induced apoptosis, which may be due to the increased expression of proliferating cell nuclear antigen (PCNA) and KGF and the reduced expression of caspase-3 (112). In addition, receptor tyrosine kinase-like orphan receptor 2 (ROR2)- (113), β-catenin- (114), and KGF-overexpressing (115) MSCs significantly promoted their proliferation and differentiation into type II alveolar epithelial cells, which demonstrated much better therapeutic effects than did native MSCs alone. Furthermore, MSCs can mitigate LPS-induced injury in murine lung epithelial cells and reverse the epithelial–mesenchymal transition by blocking the activation of the NF-κB and Hedgehog pathways (116). Finally, the repair and regeneration potential of MSCs may be induced by the stimulation of pro-inflammatory cytokines (i.e., TNF-α and IL-1β) and the activation of Jun N-terminal kinase (JNK) and p38 mitogen-activated protein kinase (MAPK) (117, 118).
According to statistical data generated early in the COVID-19 pandemic, 14% of cases were classified as severe COVID-19 and 5% were determined as critical with organ failure (3). Severe pneumonia and ARDS have been described in the reported COVID-19 cases. In parallel with proper ventilation management, dexamethasone, antiviral agents, convalescent plasma, and IL-1/6 inhibitors, MSCs are also considered a promising candidate therapy for COVID-19-related ARDS (4, 119). It was reported that MSCs from the bone marrow, amniotic fluid, and adipose tissue were all resistant to SARS-CoV-2 infection due to the low expressions of angiotensin-converting enzyme 2 (ACE-2) and transmembrane protease serine subtype 2 (TMPRSS2) on the cell surface under both steady-state and inflammatory conditions (120, 121), which supported the potential applicability of MSCs for COVID-19 treatment (Figure 1).
It was reported that MSCs were well tolerated in ARDS patients without severe adverse events in phase 1 and phase 2a clinical trials (13, 15). Even though adverse effects such as shivering, muscle contraction, and increased lactic acid dehydrogenase arose after the infusion of MSCs in a small minority of patients (122–124), multiple clinical studies have identified the tolerability and efficacy of MSCs in the treatment of COVID-19-related ARDS. Current clinical trials advise that it is safe to administer MSCs extracted from the umbilical cord, placenta, or menstrual blood to severely and critically ill COVID-19 patients (122–132), always intravenously, with one to three infusions and 106–108 cells per dose.
Leng et al. (128) reported the earliest clinical trial (ChiCTR2000029990) of MSC therapy against COVID-19. In one dose of 1 × 106 cells/kg, clinical grade MSCs were intravenously transplanted to seven patients with COVID-19 pneumonia (one of the critical type, four of the severe type, and two of the common type), and three patients in the control group received placebo treatment. Without any observed adverse effects, the symptoms (high fever, weakness, and shortness of breath) and oxygen saturations significantly improved within 2–4 days after MSC transplantation in all patients. More importantly, it was suggested that MSCs were valuable in suppressing virus-induced cytokine storms. Compared with the placebo control group, the overactivated cytokine-secreting immune cells disappeared in 3–6 days and regulatory dendritic cells dramatically increased in the group that received MSC treatment. Meanwhile, the serum pro-inflammatory cytokine TNF-α was significantly decreased and the anti-inflammatory cytokine IL-10 was remarkably increased after MSC transplantation. Of note is that all seven patients were put forward for this pilot study after no improvements were observed under standard treatment, and thus, it is not surprising that patients in the placebo group showed negative results. Therefore, the functions of MSCs should be identified in a larger population.
In a multicenter, open-label, non-randomized, parallel controlled phase 1 exploratory trial in China (ChiCTR2000029606) (125), 44 severe or critical COVID-19 patients were enrolled. Among them, 26 patients received allogeneic, menstrual blood-derived MSC therapy (three infusions totaling 9 × 107 MSCs, one infusion every other day), while 18 patients received only concomitant medication. After 1 month of follow-up, it was observed that the mortality between the two groups was significantly different (7.69% in the MSC group vs. 33.33% in the control group, p = 0.048). Compared with the control group, the symptoms of patients in the experimental group were quickly alleviated, with expiratory dyspnea significantly improving during MSC infusion on day 1 (p = 0.016), day 3 (p = 0.040), and day 5 (p = 0.031). Chest imaging results also showed improvements in the experimental group in the first month after MSC infusion. In addition, Lanzoni et al. (132) reported a double-blind, phase 1/2a, randomized controlled trial of UC-MSC therapy against COVID-19. A total of 24 patients who were diagnosed with COVID-19-related ARDS within 24 h were randomized 1:1 to either a UC-MSC treatment group (two intravenous infusions of 100 ± 20 × 106 UC-MSCs, on days 0 and 3) or a control group (two infusions of vehicle solution). The results reflected that UC-MSC treatment resulted in significant reductions of inflammatory cytokines on day 6 and improved survival (91% vs. 42%, p = 0.015) during a 31-day follow-up. In another double-blind, multicenter, phase 1 randomized controlled trial (NCT04457609) (130) in 40 critically ill COVID-19 patients, 20 patients received a single intravenous infusion of 1 × 106/kg UC-MSCs in 100 ml saline (0.9%) solution (SS) and 20 patients received 100 ml 0.9% SS as the control group. The median time from intensive care unit (ICU) enrollment to MSC treatment was 8 days (range = 2–30 days). During 30 days of observation after MSC administration, the results revealed that UC-MSC treatment was significantly associated with a higher survival rate, with particular benefit in patients with comorbidities. In conclusion, MSC therapy is a safe approach against COVID-19 disease and shows promising benefits for severely ill COVID-19 patients at a progression stage. However, the observation periods of these studies were short and the sample size was small; thus, whether MSC therapy has long-term benefits warrants further research.
In a study (NCT04288102) by Shi et al. (131), a randomized, double-blind, placebo-controlled phase 2 trial was performed in 100 severe COVID-19 patients who were randomly assigned to receive either UC-MSCs (4 × 107 cells per infusion) or placebo on days 0, 3, and 6. According to the analyses of both radiologists and lung imaging artificial intelligence software, the results of high-resolution chest CT imaging revealed that the proportions of solid component lesion volumes were significantly reduced in the MSC treatment group, but not in the placebo group, after 28 days of follow-up. The results of a 6-min walk test also indicated better restoration in patients treated with UC-MSCs. However, with a median time from symptom onset to study baseline of approximately 45 days, most of these patients were at the convalescent stage when joining this trial, which might account for the lack of significant reduction in the duration of oxygen therapy, dyspnea scores, cytokine levels, or chemokine levels. Nonetheless, COVID-19 patients might benefit from MSC therapy, even in the convalescent stage.
Several clinical studies were performed to assess the safety and effectiveness of MSCs in COVID-19 disease (Table 1). However, these preclinical trials seemed to be less convincing due to the small sample sizes ranging from 5 to 100. Because of the emergency nature of the COVID-19 outbreak and the ethical limitations, some of these studies failed to strictly follow the principles of standard clinical trials, such as randomization, blindness, and comparisons. In addition, the patients enrolled in these clinical studies were at different stages of severe COVID-19 disease, which resulted in greater heterogeneity and different results. In particular, given that intravascular coagulation and thromboembolism are considered as leading causes of fatality in COVID-19, the application of MSCs remains controversial because variable levels of highly procoagulant tissue factor (TF/CD142) are expressed by MSCs (133). Furthermore, the time to starting MSC treatment, the cell dosage, and the interval duration have not been fully determined. Hence, further high-quality randomized clinical trials are needed to provide evidence regarding the application of MSCs in COVID-19-related ARDS, and hundreds of clinical trials registered on (https://clinicaltrials.gov/) may offer assistance with this.
Table 1 Clinical trials of mesenchymal stromal cells (MSCs) in acute respiratory distress syndrome (ARDS) and sepsis.
The homing capacity and the systemic distribution of MSCs contribute to the persistence of MSCs in the lungs (26). Moreover, interesting work has been undertaken on therapeutic factors, such as Ang-1, KGF, and HGF, in ARDS and sepsis. However, MSCs alone or “protected mediators” alone may not be sufficient to rectify the disorder of the microenvironment, particularly in severe ARDS and septic shock. Therefore, there is a strong rationale for gene-modified MSCs with critical molecules to “rescue” lung injury and sepsis. It was reported that Ang-1-transfected MSCs could further improve both alveolar inflammation and permeability in septic mice (134, 135). In addition, ROR2-overexpressing MSCs led to more significant effects than did the native MSC treatment in ARDS mice, including the retention of MSCs in the lung, differentiation into alveolar type 2 (ATII) cells, and promotion of lung repair (113). Moreover, gene-modified MSCs also showed further benefits in LPS-induced sepsis and ALI treatment than did native MSCs alone, including overexpressing β-catenin, KGF, HGF, IL-10, the E-prostanoid 2 receptor, CXCR4 receptor, soluble IL-1 receptor-like-1 (sST2), ACE2, TGFβ1, CXCR7, LL-37, heme oxygenase-1, angiotensin II type 2 receptor (AT2R), Miro1, and 7ND (dominant-negative inhibitor of CCL2) (114, 115, 136–148) (Table 2). Aside from the above-mentioned coding genes, the modification of non-coding genes, microRNAs, is an alternative approach. For instance, Wei et al. (150) reported that miR-377-3p released by UC-MSCs suppressed the expression of the target gene RPTOR, and RPTOR silencing played a pivotal role in enhancing autophagy-related proteins. Administration of UC-MSCs with overexpression of miR-377-3p activated the process of autophagy in alveolar epithelial cells under LPS treatment and finally dramatically ameliorated inflammation both in vitro and in vivo. Besides, miR-124-3p transferred by MSC-derived exosomes was observed to improve oxidative stress injury and suppress inflammatory response, thus was beneficial to attenuate traumatic ALI in rats (151). It was also reported that the transduction of miRNA let-7d (with anti-fibrotic effects) promoted recovery from bleomycin-associated lung injury (149). However, there are no reports on which genes should be overexpressed or knocked out in different models of sepsis and ARDS, and it is not clear whether double or triple knockouts of specific genes overexpressed in MSCs are better than a single gene. There is also no report of whether genetically modified MSCs are safe or effective for use in the human body. Therefore, further preclinical studies are required to elucidate the exact role of gene-modified MSCs in different microenvironments.
Despite the promising effects of MSCs in both preclinical and phase 1 clinical trials, the adverse effects of MSC treatment should not be ignored. MSCs are highly adherent to membrane oxygenators, which led to a rapid decline in oxygenator performance during extracorporeal membrane oxygenation (ECMO) in a sheep model of ALI (152, 153). In addition, human MSC administration showed a lack of efficacy in influenza-mediated lung injury in mice (64) and in improving the survival of mice with staphylococcal toxic shock syndrome (154). Senescent MSCs were also unable to show protection in a murine model of LPS-induced lethal endotoxemia (155). In some reports, MSCs were indicated to enhance neutrophil recruitment and its bactericidal functions through TNF-α and IL-1β signaling (156, 157). However, these findings highlight MSCs as a potential risk factor because aberrant neutrophil activation contributes to disease severity and local tissue damage in COVID-19 through the amplification of hypercytokinemia and the formation of neutrophil extracellular traps (NETs) (158). Furthermore, the encouraging findings from animal experiments do not guarantee their efficacy in clinical trials. It was reported that biomarkers of inflammation (IL-6 and IL-8) at 5 days and the length of hospital stay, ventilator-free days, and ICU-free days at 28 days were similar between the MSC treatment and saline placebo groups in a randomized controlled trial of MSCs for ARDS (14).
Clinical trials of MSCs for ARDS and COVID-19 treatment demonstrated safety and tolerance (see Table 1). However, both published trials contained very few patients, and much work remains to be undertaken before MSCs can be used in the clinic and even for COVID-19. Firstly, there are various sources of MSCs (including tissues and donors), and the best source needs to be identified. Secondly, the preparation of MSCs (including the culture conditions, passage, and storage) should be standardized and consistent. Thirdly, the best MSC dose, timing, and delivery remain to be further clarified. Finally, the etiologies of sepsis and ARDS vary; thus, optimal treatment with MSCs may depend on different microenvironments. Future studies should take the above suggestions into full consideration and carefully investigate them.
A growing number of preclinical studies have shown encouraging findings for MSC-based therapy in sepsis and ARDS treatment. Phase 1 clinical trials have also reported that MSCs are safe and well tolerated in ARDS patients. Nevertheless, experimental studies and clinical trials are still needed to fully understand the optimal MSC application in sepsis/ARDS and COVID-19.
ZX, YH, and JZ prepared the original draft and reviewed and edited the manuscript. WH, XL, YL, NZ, and LS reviewed and edited the manuscript. XD prepared the tables and figures. All authors contributed to the article and approved the submitted version.
The study was funded by the National Natural Science Foundation of China (81870069, 81970071, and 82070084), the Natural Science Foundation of Guangdong Province (2020A1515011459 and 2021A1515012565), the Science and Technology Program of Guangzhou (202102010157), the State Key Laboratory of Respiratory Disease Independent Program (SKLRD-Z-202108), Emergency Key Program of Guangzhou Laboratory (EKPG21-17) and Natural Science Foundation of Guangdong Province (2020A1515011459).
YL is an editor of Frontiers in Immunology.
The remaining authors declare that the research was conducted in the absence of any commercial or financial relationships that could be construed as a potential conflict of interest.
All claims expressed in this article are solely those of the authors and do not necessarily represent those of their affiliated organizations, or those of the publisher, the editors and the reviewers. Any product that may be evaluated in this article, or claim that may be made by its manufacturer, is not guaranteed or endorsed by the publisher.
1. Guan WJ, Ni ZY, Hu Y, Liang WH, Ou CQ, He JX, et al. Clinical Characteristics of Coronavirus Disease 2019 in China. N Engl J Med (2020) 382(18):1708–20. doi: 10.1056/NEJMoa2002032
2. Phelan AL, Katz R, Gostin LO. The Novel Coronavirus Originating in Wuhan, China: Challenges for Global Health Governance. JAMA (2020) 323(8):709–10. doi: 10.1001/jama.2020.1097
3. Wu Z, McGoogan JM. Characteristics of and Important Lessons From the Coronavirus Disease 2019 (COVID-19) Outbreak in China: Summary of a Report of 72 314 Cases From the Chinese Center for Disease Control and Prevention. JAMA (2020) 323(13):1239–42. doi: 10.1001/jama.2020.2648
4. Berlin DA, Gulick RM, Martinez FJ. Severe Covid-19. N Engl J Med (2020) 383(25):2451–60. doi: 10.1056/NEJMcp2009575
5. Tay MZ, Poh CM, Rénia L, MacAry PA, Ng LFP. The Trinity of COVID-19: Immunity, Inflammation and Intervention. Nat Rev Immunol (2020) 20(6):363–74. doi: 10.1038/s41577-020-0311-8
6. Bonaventura A, Vecchié A, Dagna L, Martinod K, Dixon DL, Van Tassell BW, et al. Endothelial Dysfunction and Immunothrombosis as Key Pathogenic Mechanisms in COVID-19. Nat Rev Immunol (2021) 21(5):319–29. doi: 10.1038/s41577-021-00536-9
7. Yip HK, Fang WF, Li YC, Lee FY, Lee CH, Pei SN, et al. Human Umbilical Cord-Derived Mesenchymal Stem Cells for Acute Respiratory Distress Syndrome. Crit Care Med (2020) 48(5):e391–e9. doi: 10.1097/ccm.0000000000004285
8. Chen J, Hu C, Chen L, Tang L, Zhu Y, Xu X, et al. Clinical Study of Mesenchymal Stem Cell Treatment for Acute Respiratory Distress Syndrome Induced by Epidemic Influenza A (H7N9) Infection: A Hint for COVID-19 Treatment. Engineering (Beijing) (2020) 6(10):1153–61. doi: 10.1016/j.eng.2020.02.006
9. Schlosser K, Wang JP, Dos Santos C, Walley KR, Marshall J, Fergusson DA, et al. Effects of Mesenchymal Stem Cell Treatment on Systemic Cytokine Levels in a Phase 1 Dose Escalation Safety Trial of Septic Shock Patients. Crit Care Med (2019) 47(7):918–25. doi: 10.1097/CCM.0000000000003657
10. Perlee D, van Vught LA, Scicluna BP, Maag A, Lutter R, Kemper EM, et al. Intravenous Infusion of Human Adipose Mesenchymal Stem Cells Modifies the Host Response to Lipopolysaccharide in Humans: A Randomized, Single-Blind, Parallel Group, Placebo Controlled Trial. Stem Cells (2018) 36(11):1778–88. doi: 10.1002/stem.2891
11. McIntyre LA, Stewart DJ, Mei SHJ, Courtman D, Watpool I, Granton J, et al. Cellular Immunotherapy for Septic Shock. A Phase I Clinical Trial. Am J Respir Crit Care Med (2018) 197(3):337–47. doi: 10.1164/rccm.201705-1006OC
12. He X, Ai S, Guo W, Yang Y, Wang Z, Jiang D, et al. Umbilical Cord-Derived Mesenchymal Stem (Stromal) Cells for Treatment of Severe Sepsis: Aphase 1 Clinical Trial. Transl Res (2018) 199:52–61. doi: 10.1016/j.trsl.2018.04.006
13. Wilson JG, Liu KD, Zhuo H, Caballero L, McMillan M, Fang X, et al. Mesenchymal Stem (Stromal) Cells for Treatment of ARDS: A Phase 1 Clinical Trial. Lancet Respir Med (2015) 3(1):24–32. doi: 10.1016/s2213-2600(14)70291-7
14. Zheng G, Huang L, Tong H, Shu Q, Hu Y, Ge M, et al. Treatment of Acute Respiratory Distress Syndrome With Allogeneic Adipose-Derived Mesenchymal Stem Cells: A Randomized, Placebo-Controlled Pilot Study. Respir Res (2014) 15(1):39. doi: 10.1186/1465-9921-15-39
15. Matthay MA, Calfee CS, Zhuo H, Thompson BT, Wilson JG, Levitt JE, et al. Treatment With Allogeneic Mesenchymal Stromal Cells for Moderate to Severe Acute Respiratory Distress Syndrome (START Study): A Randomised Phase 2a Safety Trial. Lancet Respir Med (2019) 7(2):154–62. doi: 10.1016/s2213-2600(18)30418-1
16. Dominici M, Le Blanc K, Mueller I, Slaper-Cortenbach I, Marini F, Krause D, et al. Minimal Criteria for Defining Multipotent Mesenchymal Stromal Cells. The International Society for Cellular Therapy Position Statement. Cytotherapy (2006) 8(4):315–7. doi: 10.1080/14653240600855905
17. Zuk PA, Zhu M, Ashjian P, De Ugarte DA, Huang JI, Mizuno H, et al. Human Adipose Tissue Is a Source of Multipotent Stem Cells. Mol Biol Cell (2002) 13(12):4279–95. doi: 10.1091/mbc.E02-02-0105
18. Campagnoli C, Roberts IA, Kumar S, Bennett PR, Bellantuono I, Fisk NM. Identification of Mesenchymal Stem/Progenitor Cells in Human First-Trimester Fetal Blood, Liver, and Bone Marrow. Blood (2001) 98(8):2396–402. doi: 10.1182/blood.V98.8.2396
19. In 't Anker PS, Scherjon SA, Kleijburg-van der Keur C, Noort WA, Claas FH, Willemze R, et al. Amniotic Fluid as a Novel Source of Mesenchymal Stem Cells for Therapeutic Transplantation. Blood (2003) 102(4):1548–9. doi: 10.1182/blood-2003-04-1291
20. Chamberlain G, Fox J, Ashton B, Middleton J. Concise Review: Mesenchymal Stem Cells: Their Phenotype, Differentiation Capacity, Immunological Features, and Potential for Homing. Stem Cells (2007) 25(11):2739–49. doi: 10.1634/stemcells.2007-0197
21. Nazarov I, Lee JW, Soupene E, Etemad S, Knapik D, Green W, et al. Multipotent Stromal Stem Cells From Human Placenta Demonstrate High Therapeutic Potential. Stem Cells Trans Med (2012) 1(5):359–72. doi: 10.5966/sctm.2011-0021
22. Sun CK, Leu S, Hsu SY, Zhen YY, Chang LT, Tsai CY, et al. Mixed Serum-Deprived and Normal Adipose-Derived Mesenchymal Stem Cells Against Acute Lung Ischemia-Reperfusion Injury in Rats. Am J Trans Res (2015) 7(2):209–31.
23. Mao YX, Xu JF, Seeley EJ, Tang XD, Xu LL, Zhu YG, et al. Adipose Tissue-Derived Mesenchymal Stem Cells Attenuate Pulmonary Infection Caused by Pseudomonas Aeruginosa via Inhibiting Overproduction of Prostaglandin E2. Stem Cells (2015) 33(7):2331–42. doi: 10.1002/stem.1996
24. Jiang X, Jiang X, Qu C, Chang P, Zhang C, Qu Y, et al. Intravenous Delivery of Adipose-Derived Mesenchymal Stromal Cells Attenuates Acute Radiation-Induced Lung Injury in Rats. Cytotherapy (2015) 17(5):560–70. doi: 10.1016/j.jcyt.2015.02.011
25. Reddy M, Fonseca L, Gowda S, Chougule B, Hari A, Totey S. Human Adipose-Derived Mesenchymal Stem Cells Attenuate Early Stage of Bleomycin Induced Pulmonary Fibrosis: Comparison With Pirfenidone. Int J Stem Cells (2016) 9(2):192–206. doi: 10.15283/ijsc16041
26. Hou C, Peng D, Gao L, Tian D, Dai J, Luo Z, et al. Human Umbilical Cord-Derived Mesenchymal Stem Cells Protect From Hyperoxic Lung Injury by Ameliorating Aberrant Elastin Remodeling in the Lung of O2-Exposed Newborn Rat. Biochem Biophys Res Commun (2018) 495(2):1972–9. doi: 10.1016/j.bbrc.2017.12.055
27. Zhu Y, Xu L, Collins JJP, Vadivel A, Cyr-Depauw C, Zhong S, et al. Human Umbilical Cord Mesenchymal Stromal Cells Improve Survival and Bacterial Clearance in Neonatal Sepsis in Rats. Stem Cells Dev (2017) 26(14):1054–64. doi: 10.1089/scd.2016.0329
28. Le Blanc K, Tammik C, Rosendahl K, Zetterberg E, Ringden O. HLA Expression and Immunologic Properties of Differentiated and Undifferentiated Mesenchymal Stem Cells. Exp Hematol (2003) 31(10):890–6. doi: 10.1016/S0301-472X(03)00110-3
29. Laffey JG, Matthay MA. Fifty Years of Research in ARDS. Cell-Based Therapy for Acute Respiratory Distress Syndrome. Biology and Potential Therapeutic Value. Am J Respir Crit Care Med (2017) 196(3):266–73. doi: 10.1164/rccm.201701-0107CP
30. De Becker A, Riet IV. Homing and Migration of Mesenchymal Stromal Cells: How to Improve the Efficacy of Cell Therapy? World J Stem Cells (2016) 8(3):73–87. doi: 10.4252/wjsc.v8.i3.73
31. Walter J, Ware LB, Matthay MA. Mesenchymal Stem Cells: Mechanisms of Potential Therapeutic Benefit in ARDS and Sepsis. Lancet Respir Med (2014) 2(12):1016–26. doi: 10.1016/s2213-2600(14)70217-6
32. Qin H, Zhao A. Mesenchymal Stem Cell Therapy for Acute Respiratory Distress Syndrome: From Basic to Clinics. Protein Cell (2020) 11(10):707–22. doi: 10.1007/s13238-020-00738-2
33. Krasnodembskaya A, Song Y, Fang X, Gupta N, Serikov V, Lee JW, et al. Antibacterial Effect of Human Mesenchymal Stem Cells Is Mediated in Part From Secretion of the Antimicrobial Peptide LL-37. Stem Cells (2010) 28(12):2229–38. doi: 10.1002/stem.544
34. Curley GF, Hayes M, Ansari B, Shaw G, Ryan A, Barry F, et al. Mesenchymal Stem Cells Enhance Recovery and Repair Following Ventilator-Induced Lung Injury in the Rat. Thorax (2012) 67(6):496–501. doi: 10.1136/thoraxjnl-2011-201059
35. Lee JW, Krasnodembskaya A, McKenna DH, Song Y, Abbott J, Matthay MA. Therapeutic Effects of Human Mesenchymal Stem Cells in Ex Vivo Human Lungs Injured With Live Bacteria. Am J Respir Crit Care Med (2013) 187(7):751–60. doi: 10.1164/rccm.201206-0990OC
36. Fang X, Neyrinck AP, Matthay MA, Lee JW. Allogeneic Human Mesenchymal Stem Cells Restore Epithelial Protein Permeability in Cultured Human Alveolar Type II Cells by Secretion of Angiopoietin-1. J Biol Chem (2010) 285(34):26211–22. doi: 10.1074/jbc.M110.119917
37. Hu S, Li J, Xu X, Liu A, He H, Xu J, et al. The Hepatocyte Growth Factor-Expressing Character Is Required for Mesenchymal Stem Cells to Protect the Lung Injured by Lipopolysaccharide In Vivo. Stem Cell Res Ther (2016) 7(1):66. doi: 10.1186/s13287-016-0320-5
38. Wang H, Zheng R, Chen Q, Shao J, Yu J, Hu S. Mesenchymal Stem Cells Microvesicles Stabilize Endothelial Barrier Function Partly Mediated by Hepatocyte Growth Factor (HGF). Stem Cell Res Ther (2017) 8(1):211. doi: 10.1186/s13287-017-0662-7
39. Lu Z, Chang W, Meng S, Xu X, Xie J, Guo F, et al. Mesenchymal Stem Cells Induce Dendritic Cell Immune Tolerance via Paracrine Hepatocyte Growth Factor to Alleviate Acute Lung Injury. Stem Cell Res Ther (2019) 10(1):372. doi: 10.1186/s13287-019-1488-2
40. Fan XL, Zhang Y, Li X, Fu QL. Mechanisms Underlying the Protective Effects of Mesenchymal Stem Cell-Based Therapy. Cell Mol Life Sci CMLS (2020) 77(14):2771–94. doi: 10.1007/s00018-020-03454-6
41. Islam MN, Das SR, Emin MT, Wei M, Sun L, Westphalen K, et al. Mitochondrial Transfer From Bone-Marrow-Derived Stromal Cells to Pulmonary Alveoli Protects Against Acute Lung Injury. Nat Med (2012) 18(5):759–65. doi: 10.1038/nm.2736
42. Jackson MV, Morrison TJ, Doherty DF, McAuley DF, Matthay MA, Kissenpfennig A, et al. Mitochondrial Transfer via Tunneling Nanotubes Is an Important Mechanism by Which Mesenchymal Stem Cells Enhance Macrophage Phagocytosis in the In Vitro and In Vivo Models of ARDS. Stem Cells (2016) 34(8):2210–23. doi: 10.1002/stem.2372
43. Morrison TJ, Jackson MV, Cunningham EK, Kissenpfennig A, McAuley DF, O'Kane CM, et al. Mesenchymal Stromal Cells Modulate Macrophages in Clinically Relevant Lung Injury Models by Extracellular Vesicle Mitochondrial Transfer. Am J Respir Crit Care Med (2017) 196(10):1275–86. doi: 10.1164/rccm.201701-0170OC
44. Sinclair KA, Yerkovich ST, Hopkins PM, Chambers DC. Characterization of Intercellular Communication and Mitochondrial Donation by Mesenchymal Stromal Cells Derived From the Human Lung. Stem Cell Res Ther (2016) 7(1):91. doi: 10.1186/s13287-016-0354-8
45. Fergie N, Todd N, McClements L, McAuley D, O'Kane C, Krasnodembskaya A. Hypercapnic Acidosis Induces Mitochondrial Dysfunction and Impairs the Ability of Mesenchymal Stem Cells to Promote Distal Lung Epithelial Repair. FASEB J (2019) 33(4):5585–98. doi: 10.1096/fj.201802056R
46. Islam D, Huang Y, Fanelli V, Delsedime L, Wu S, Khang J, et al. Identification and Modulation of Microenvironment Is Crucial for Effective Mesenchymal Stromal Cell Therapy in Acute Lung Injury. Am J Respir Crit Care Med (2019) 199(10):1214–24. doi: 10.1164/rccm.201802-0356OC
47. Silva JD, Su Y, Calfee CS, Delucchi KL, Weiss D, McAuley DF, et al. MSC Extracellular Vesicles Rescue Mitochondrial Dysfunction and Improve Barrier Integrity in Clinically Relevant Models of ARDS. Eur Respir J (2020) 58(1):2002978. doi: 10.1183/13993003.02978-2020
48. Singer M, Deutschman CS, Seymour CW, Shankar-Hari M, Annane D, Bauer M, et al. The Third International Consensus Definitions for Sepsis and Septic Shock (Sepsis-3). Jama (2016) 315(8):801–10. doi: 10.1001/jama.2016.0287
49. American College of Chest Physicians/Society of Critical Care Medicine Consensus Conference: Definitions for Sepsis and Organ Failure and Guidelines for the Use of Innovative Therapies in Sepsis. Crit Care Med (1992) 20(6):864–74.
50. Kumar A, Zarychanski R, Light B, Parrillo J, Maki D, Simon D, et al. Early Combination Antibiotic Therapy Yields Improved Survival Compared With Monotherapy in Septic Shock: A Propensity-Matched Analysis. Crit Care Med (2010) 38(9):1773–85. doi: 10.1097/CCM.0b013e3181eb3ccd
51. Howell MD, Davis AM. Management of Sepsis and Septic Shock. JAMA (2017) 317(8):847–8. doi: 10.1001/jama.2017.0131
52. Mei SH, Haitsma JJ, Dos Santos CC, Deng Y, Lai PF, Slutsky AS, et al. Mesenchymal Stem Cells Reduce Inflammation While Enhancing Bacterial Clearance and Improving Survival in Sepsis. Am J Respir Crit Care Med (2010) 182(8):1047–57. doi: 10.1164/rccm.201001-0010OC
53. Gupta N, Krasnodembskaya A, Kapetanaki M, Mouded M, Tan X, Serikov V, et al. Mesenchymal Stem Cells Enhance Survival and Bacterial Clearance in Murine Escherichia Coli Pneumonia. Thorax (2012) 67(6):533–9. doi: 10.1136/thoraxjnl-2011-201176
54. Krasnodembskaya A, Samarani G, Song Y, Zhuo H, Su X, Lee JW, et al. Human Mesenchymal Stem Cells Reduce Mortality and Bacteremia in Gram-Negative Sepsis in Mice in Part by Enhancing the Phagocytic Activity of Blood Monocytes. Am J Physiol Lung Cell Mol Physiol (2012) 302(10):L1003–13. doi: 10.1152/ajplung.00180.2011
55. Rabani R, Volchuk A, Jerkic M, Ormesher L, Garces-Ramirez L, Canton J, et al. Mesenchymal Stem Cells Enhance NOX2 Dependent ROS Production and Bacterial Killing in Macrophages During Sepsis. Eur Respir J (2018) 51(4):1702021. doi: 10.1183/13993003.02021-2017
56. Hall SR, Tsoyi K, Ith B, Padera RF Jr., Lederer JA, Wang Z, et al. Mesenchymal Stromal Cells Improve Survival During Sepsis in the Absence of Heme Oxygenase-1: The Importance of Neutrophils. Stem Cells (2013) 31(2):397–407. doi: 10.1002/stem.1270
57. Devaney J, Horie S, Masterson C, Elliman S, Barry F, O'Brien T, et al. Human Mesenchymal Stromal Cells Decrease the Severity of Acute Lung Injury Induced by E. Coli in the Rat. Thorax (2015) 70(7):625–35. doi: 10.1136/thoraxjnl-2015-206813
58. Hao Q, Gudapati V, Monsel A, Park JH, Hu S, Kato H, et al. Mesenchymal Stem Cell-Derived Extracellular Vesicles Decrease Lung Injury in Mice. J Immunol (2019) 203(7):1961–72. doi: 10.4049/jimmunol.1801534
59. Martin GS. Sepsis, Severe Sepsis and Septic Shock: Changes in Incidence, Pathogens and Outcomes. Expert Rev Anti-Infective Ther (2012) 10(6):701–6. doi: 10.1586/eri.12.50
60. Miggins M, Hasan A, Hohmann S, Southwick F, Casella G, Schain D, et al. The Potential Influence of Common Viral Infections Diagnosed During Hospitalization Among Critically Ill Patients in the United States. PLoS One (2011) 6(4):e18890. doi: 10.1371/journal.pone.0018890
61. Khatri M, O'Brien TD, Goyal SM, Sharma JM. Isolation and Characterization of Chicken Lung Mesenchymal Stromal Cells and Their Susceptibility to Avian Influenza Virus. Dev Comp Immunol (2010) 34(4):474–9. doi: 10.1016/j.dci.2009.12.008
62. Thanunchai M, Kanrai P, Wiboon-Ut S, Puthavathana P, Hongeng S, Thitithanyanont A. Tropism of Avian Influenza A (H5N1) Virus to Mesenchymal Stem Cells and CD34+ Hematopoietic Stem Cells. PLoS One (2013) 8(12):e81805. doi: 10.1371/journal.pone.0081805
63. Khatri M, Saif YM. Influenza Virus Infects Bone Marrow Mesenchymal Stromal Cells In Vitro: Implications for Bone Marrow Transplantation. Cell Transplant (2013) 22(3):461–8. doi: 10.3727/096368912x656063
64. Gotts JE, Abbott J, Matthay MA. Influenza Causes Prolonged Disruption of the Alveolar-Capillary Barrier in Mice Unresponsive to Mesenchymal Stem Cell Therapy. Am J Physiol Lung Cell Mol Physiol (2014) 307(5):L395–406. doi: 10.1152/ajplung.00110.2014
65. Darwish I, Banner D, Mubareka S, Kim H, Besla R, Kelvin DJ, et al. Mesenchymal Stromal (Stem) Cell Therapy Fails to Improve Outcomes in Experimental Severe Influenza. PLoS One (2013) 8(8):e71761. doi: 10.1371/journal.pone.0071761
66. Chan MC, Kuok DI, Leung CY, Hui KP, Valkenburg SA, Lau EH, et al. Human Mesenchymal Stromal Cells Reduce Influenza A H5N1-Associated Acute Lung Injury In Vitro and In Vivo. Proc Natl Acad Sci U S A (2016) 113(13):3621–6. doi: 10.1073/pnas.1601911113
67. Li Y, Xu J, Shi W, Chen C, Shao Y, Zhu L, et al. Mesenchymal Stromal Cell Treatment Prevents H9N2 Avian Influenza Virus-Induced Acute Lung Injury in Mice. Stem Cell Res Ther (2016) 7(1):159. doi: 10.1186/s13287-016-0395-z
68. Khatri M, Richardson LA, Meulia T. Mesenchymal Stem Cell-Derived Extracellular Vesicles Attenuate Influenza Virus-Induced Acute Lung Injury in a Pig Model. Stem Cell Res Ther (2018) 9(1):17. doi: 10.1186/s13287-018-0774-8
69. Loy H, Kuok DIT, Hui KPY, Choi MHL, Yuen W, Nicholls JM, et al. Therapeutic Implications of Human Umbilical Cord Mesenchymal Stromal Cells in Attenuating Influenza A(H5N1) Virus-Associated Acute Lung Injury. J Infect Dis (2019) 219(2):186–96. doi: 10.1093/infdis/jiy478
70. Martin GS, Mannino DM, Eaton S, Moss M. The Epidemiology of Sepsis in the United States From 1979 Through 2000. N Engl J Med (2003) 348(16):1546–54. doi: 10.1056/NEJMoa022139
71. Yang R, Liu Y, Kelk P, Qu C, Akiyama K, Chen C, et al. A Subset of IL-17(+) Mesenchymal Stem Cells Possesses Anti-Candida Albicans Effect. Cell Res (2013) 23(1):107–21. doi: 10.1038/cr.2012.179
72. Gupta N, Su X, Popov B, Lee JW, Serikov V, Matthay MA. Intrapulmonary Delivery of Bone Marrow-Derived Mesenchymal Stem Cells Improves Survival and Attenuates Endotoxin-Induced Acute Lung Injury in Mice. J Immunol (2007) 179(3):1855–63. doi: 10.4049/jimmunol.179.3.1855
73. Luo CJ, Zhang FJ, Zhang L, Geng YQ, Li QG, Hong Q, et al. Mesenchymal Stem Cells Ameliorate Sepsis-Associated Acute Kidney Injury in Mice. Shock (2014) 41(2):123–9. doi: 10.1097/shk.0000000000000080
74. Wu KH, Wu HP, Chao WR, Lo WY, Tseng PC, Lee CJ, et al. Time-Series Expression of Toll-Like Receptor 4 Signaling in Septic Mice Treated With Mesenchymal Stem Cells. Shock (2016) 45(6):634–40. doi: 10.1097/shk.0000000000000546
75. Asami T, Ishii M, Namkoong H, Yagi K, Tasaka S, Asakura T, et al. Anti-Inflammatory Roles of Mesenchymal Stromal Cells During Acute Streptococcus Pneumoniae Pulmonary Infection in Mice. Cytotherapy (2018) 20(3):302–13. doi: 10.1016/j.jcyt.2018.01.003
76. Weil BR, Manukyan MC, Herrmann JL, Wang Y, Abarbanell AM, Poynter JA, et al. Mesenchymal Stem Cells Attenuate Myocardial Functional Depression and Reduce Systemic and Myocardial Inflammation During Endotoxemia. Surgery (2010) 148(2):444–52. doi: 10.1016/j.surg.2010.03.010
77. Pedrazza L, Cubillos-Rojas M, de Mesquita FC, Luft C, Cunha AA, Rosa JL, et al. Mesenchymal Stem Cells Decrease Lung Inflammation During Sepsis, Acting Through Inhibition of the MAPK Pathway. Stem Cell Res Ther (2017) 8(1):289. doi: 10.1186/s13287-017-0734-8
78. Gonzalez-Rey E, Anderson P, Gonzalez MA, Rico L, Buscher D, Delgado M. Human Adult Stem Cells Derived From Adipose Tissue Protect Against Experimental Colitis and Sepsis. Gut (2009) 58(7):929–39. doi: 10.1136/gut.2008.168534
79. dos Santos CC, Murthy S, Hu P, Shan Y, Haitsma JJ, Mei SH, et al. Network Analysis of Transcriptional Responses Induced by Mesenchymal Stem Cell Treatment of Experimental Sepsis. Am J Pathol (2012) 181(5):1681–92. doi: 10.1016/j.ajpath.2012.08.009
80. Lee RH, Yu JM, Foskett AM, Peltier G, Reneau JC, Bazhanov N, et al. TSG-6 as a Biomarker to Predict Efficacy of Human Mesenchymal Stem/Progenitor Cells (hMSCs) in Modulating Sterile Inflammation In Vivo. Proc Natl Acad Sci U S A (2014) 111(47):16766–71. doi: 10.1073/pnas.1416121111
81. Lee RH, Pulin AA, Seo MJ, Kota DJ, Ylostalo J, Larson BL, et al. Intravenous hMSCs Improve Myocardial Infarction in Mice Because Cells Embolized in Lung Are Activated to Secrete the Anti-Inflammatory Protein TSG-6. Cell Stem Cell (2009) 5(1):54–63. doi: 10.1016/j.stem.2009.05.003
82. Wang G, Cao K, Liu K, Xue Y, Roberts AI, Li F, et al. Kynurenic Acid, an IDO Metabolite, Controls TSG-6-Mediated Immunosuppression of Human Mesenchymal Stem Cells. Cell Death Differ (2018) 25(7):1209–23. doi: 10.1038/s41418-017-0006-2
83. Dang S, Yu ZM, Zhang CY, Zheng J, Li KL, Wu Y, et al. Autophagy Promotes Apoptosis of Mesenchymal Stem Cells Under Inflammatory Microenvironment. Stem Cell Res Ther (2015) 6:247. doi: 10.1186/s13287-015-0245-4
84. Abreu SC, Rolandsson Enes S, Dearborn J, Goodwin M, Coffey A, Borg ZD, et al. Lung Inflammatory Environments Differentially Alter Mesenchymal Stromal Cell Behavior. Am J Physiol Lung Cell Mol Physiol (2019) 317(6):L823–l31. doi: 10.1152/ajplung.00263.2019
85. Li S, Wu H, Han D, Ma S, Fan W, Wang Y, et al. A Novel Mechanism of Mesenchymal Stromal Cell-Mediated Protection Against Sepsis: Restricting Inflammasome Activation in Macrophages by Increasing Mitophagy and Decreasing Mitochondrial ROS. Oxid Med Cell Longevity (2018) 2018:15. doi: 10.1155/2018/3537609
86. Su VY, Lin CS, Hung SC, Yang KY. Mesenchymal Stem Cell-Conditioned Medium Induces Neutrophil Apoptosis Associated With Inhibition of the NF-kappaB Pathway in Endotoxin-Induced Acute Lung Injury. Int J Mol Sci (2019) 20(9):2208. doi: 10.3390/ijms20092208
87. Deutschman CS, Tracey KJ. Sepsis: Current Dogma and New Perspectives. Immunity (2014) 40(4):463–75. doi: 10.1016/j.immuni.2014.04.001
88. Hotchkiss RS, Monneret G, Payen D. Sepsis-Induced Immunosuppression: From Cellular Dysfunctions to Immunotherapy. Nat Rev Immunol (2013) 13(12):862–74. doi: 10.1038/nri3552
89. Nemeth K, Leelahavanichkul A, Yuen PS, Mayer B, Parmelee A, Doi K, et al. Bone Marrow Stromal Cells Attenuate Sepsis via Prostaglandin E(2)-Dependent Reprogramming of Host Macrophages to Increase Their Interleukin-10 Production. Nat Med (2009) 15(1):42–9. doi: 10.1038/nm.1905
90. Zhu H, Xiong Y, Xia Y, Zhang R, Tian D, Wang T, et al. Therapeutic Effects of Human Umbilical Cord-Derived Mesenchymal Stem Cells in Acute Lung Injury Mice. Sci Rep (2017) 7:39889. doi: 10.1038/srep39889
91. Sreeramkumar V, Hons M, Punzón C, Stein JV, Sancho D, Fresno M, et al. Efficient T-Cell Priming and Activation Requires Signaling Through Prostaglandin E2 (EP) Receptors. Immunol Cell Biol (2016) 94(1):39–51. doi: 10.1038/icb.2015.62
92. Aggarwal S, Pittenger MF. Human Mesenchymal Stem Cells Modulate Allogeneic Immune Cell Responses. Blood (2005) 105(4):1815–22. doi: 10.1182/blood-2004-04-1559
93. Chao YH, Wu HP, Wu KH, Tsai YG, Peng CT, Lin KC, et al. An Increase in CD3+CD4+CD25+ Regulatory T Cells After Administration of Umbilical Cord-Derived Mesenchymal Stem Cells During Sepsis. PLoS One (2014) 9(10):e110338. doi: 10.1371/journal.pone.0110338
94. Zhu J, Feng B, Xu Y, Chen W, Sheng X, Feng X, et al. Mesenchymal Stem Cells Alleviate LPS-Induced Acute Lung Injury by Inhibiting the Proinflammatory Function of Ly6C(+) CD8(+) T Cells. Cell Death Dis (2020) 11(10):829. doi: 10.1038/s41419-020-03036-1
95. Feng B, Zhu J, Xu Y, Chen W, Sheng X, Feng X, et al. Immunosuppressive Effects of Mesenchymal Stem Cells on Lung B Cell Gene Expression in LPS-Induced Acute Lung Injury. Stem Cell Res Ther (2020) 11(1):418. doi: 10.1186/s13287-020-01934-x
96. Song Y, Dou H, Li X, Zhao X, Li Y, Liu D, et al. Exosomal miR-146a Contributes to the Enhanced Therapeutic Efficacy of Interleukin-1beta-Primed Mesenchymal Stem Cells Against Sepsis. Stem Cells (2017) 35(5):1208–21. doi: 10.1002/stem.2564
97. Matthay MA, Zemans RL, Zimmerman GA, Arabi YM, Beitler JR, Mercat A, et al. Acute Respiratory Distress Syndrome. Nat Rev Dis Primers (2019) 5(1):1–18. doi: 10.1038/s41572-019-0069-0
98. Bhattacharya J, Matthay MA. Regulation and Repair of the Alveolar-Capillary Barrier in Acute Lung Injury. Annu Rev Physiol (2013) 75:593–615. doi: 10.1146/annurev-physiol-030212-183756
99. Goolaerts A, Pellan-Randrianarison N, Larghero J, Vanneaux V, Uzunhan Y, Gille T, et al. Conditioned Media From Mesenchymal Stromal Cells Restore Sodium Transport and Preserve Epithelial Permeability in an In Vitro Model of Acute Alveolar Injury. Am J Physiol Lung Cell Mol Physiol (2014) 306(11):L975–85. doi: 10.1152/ajplung.00242.2013
100. Zhang J, Shao Y, He D, Zhang L, Xu G, Shen J. Evidence That Bone Marrow-Derived Mesenchymal Stem Cells Reduce Epithelial Permeability Following Phosgene-Induced Acute Lung Injury via Activation of Wnt3a Protein-Induced Canonical Wnt/Beta-Catenin Signaling. Inhal Toxicol (2016) 28(12):572–9. doi: 10.1080/08958378.2016.1228720
101. Pelosi P, D'Onofrio D, Chiumello D, Paolo S, Chiara G, Capelozzi VL, et al. Pulmonary and Extrapulmonary Acute Respiratory Distress Syndrome Are Different. Eur Respir J Suppl (2003) 42:48s–56s. doi: 10.1183/09031936.03.00420803
102. Pati S, Gerber MH, Menge TD, Wataha KA, Zhao Y, Baumgartner JA, et al. Bone Marrow Derived Mesenchymal Stem Cells Inhibit Inflammation and Preserve Vascular Endothelial Integrity in the Lungs After Hemorrhagic Shock. PLoS One (2011) 6(9):e25171. doi: 10.1371/journal.pone.0025171
103. Potter DR, Miyazawa BY, Gibb SL, Deng X, Togaratti PP, Croze RH, et al. Mesenchymal Stem Cell-Derived Extracellular Vesicles Attenuate Pulmonary Vascular Permeability and Lung Injury Induced by Hemorrhagic Shock and Trauma. J Trauma Acute Care Surg (2018) 84(2):245–56. doi: 10.1097/TA.0000000000001744
104. Ihara K, Fukuda S, Enkhtaivan B, Trujillo R, Perez-Bello D, Nelson C, et al. Adipose-Derived Stem Cells Attenuate Pulmonary Microvascular Hyperpermeability After Smoke Inhalation. PLoS One (2017) 12(10):e0185937. doi: 10.1371/journal.pone.0185937
105. Lee JW, Fang X, Gupta N, Serikov V, Matthay MA. Allogeneic Human Mesenchymal Stem Cells for Treatment of E. Coli Endotoxin-Induced Acute Lung Injury in the Ex Vivo Perfused Human Lung. Proc Natl Acad Sci U S A (2009) 106(38):16357–62. doi: 10.1073/pnas.0907996106
106. Pati S, Khakoo AY, Zhao J, Jimenez F, Gerber MH, Harting M, et al. Human Mesenchymal Stem Cells Inhibit Vascular Permeability by Modulating Vascular Endothelial Cadherin/Beta-Catenin Signaling. Stem Cells Dev (2011) 20(1):89–101. doi: 10.1089/scd.2010.0013
107. Ware LB, Matthay MA. Alveolar Fluid Clearance Is Impaired in the Majority of Patients With Acute Lung Injury and the Acute Respiratory Distress Syndrome. Am J Respir Crit Care Med (2001) 163(6):1376–83. doi: 10.1164/ajrccm.163.6.2004035
108. Hao Q, Zhu YG, Monsel A, Gennai S, Lee T, Xu F, et al. Study of Bone Marrow and Embryonic Stem Cell-Derived Human Mesenchymal Stem Cells for Treatment of Escherichia Coli Endotoxin-Induced Acute Lung Injury in Mice. Stem Cells Trans Med (2015) 4(7):832–40. doi: 10.5966/sctm.2015-0006
109. Asmussen S, Ito H, Traber DL, Lee JW, Cox RA, Hawkins HK, et al. Human Mesenchymal Stem Cells Reduce the Severity of Acute Lung Injury in a Sheep Model of Bacterial Pneumonia. Thorax (2014) 69(9):819–25. doi: 10.1136/thoraxjnl-2013-204980
110. Li JW, Wu X. Mesenchymal Stem Cells Ameliorate LPS-Induced Acute Lung Injury Through KGF Promoting Alveolar Fluid Clearance of Alveolar Type II Cells. Eur Rev Med Pharmacol Sci (2015) 19(13):2368–78.
111. McAuley DF, Curley GF, Hamid UI, Laffey JG, Abbott J, McKenna DH, et al. Clinical Grade Allogeneic Human Mesenchymal Stem Cells Restore Alveolar Fluid Clearance in Human Lungs Rejected for Transplantation. Am J Physiol Lung Cell Mol Physiol (2014) 306(9):L809–15. doi: 10.1152/ajplung.00358.2013
112. Xiang B, Chen L, Wang X, Zhao Y, Wang Y, Xiang C. Transplantation of Menstrual Blood-Derived Mesenchymal Stem Cells Promotes the Repair of LPS-Induced Acute Lung Injury. Int J Mol Sci (2017) 18(4):689. doi: 10.3390/ijms18040689
113. Cai SX, Liu AR, Chen S, He HL, Chen QH, Xu JY, et al. The Orphan Receptor Tyrosine Kinase ROR2 Facilitates MSCs to Repair Lung Injury in ARDS Animal Model. Cell Transplant (2016) 25(8):1561–74. doi: 10.3727/096368915x689776
114. Cai SX, Liu AR, Chen S, He HL, Chen QH, Xu JY, et al. Activation of Wnt/beta-Catenin Signalling Promotes Mesenchymal Stem Cells to Repair Injured Alveolar Epithelium Induced by Lipopolysaccharide in Mice. Stem Cell Res Ther (2015) 6:65. doi: 10.1186/s13287-015-0060-y
115. Chen J, Li C, Gao X, Li C, Liang Z, Yu L, et al. Keratinocyte Growth Factor Gene Delivery via Mesenchymal Stem Cells Protects Against Lipopolysaccharide-Induced Acute Lung Injury in Mice. PLoS One (2013) 8(12):e83303. doi: 10.1371/journal.pone.0083303
116. Xiao K, He W, Guan W, Hou F, Yan P, Xu J, et al. Mesenchymal Stem Cells Reverse EMT Process Through Blocking the Activation of NF-kappaB and Hedgehog Pathways in LPS-Induced Acute Lung Injury. Cell Death Dis (2020) 11(10):863. doi: 10.1038/s41419-020-03034-3
117. Broekman W, Amatngalim GD, de Mooij-Eijk Y, Oostendorp J, Roelofs H, Taube C, et al. TNF-Alpha and IL-1beta-Activated Human Mesenchymal Stromal Cells Increase Airway Epithelial Wound Healing In Vitro via Activation of the Epidermal Growth Factor Receptor. Respir Res (2016) 17:3. doi: 10.1186/s12931-015-0316-1
118. Chen J, Li Y, Hao H, Li C, Du Y, Hu Y, et al. Mesenchymal Stem Cell Conditioned Medium Promotes Proliferation and Migration of Alveolar Epithelial Cells Under Septic Conditions In Vitro via the JNK-P38 Signaling Pathway. Cell Physiol Biochem Int J Exp Cell Physiol Biochem Pharmacol (2015) 37(5):1830–46. doi: 10.1159/000438545
119. Khoury M, Cuenca J, Cruz FF, Figueroa FE, Rocco PRM, Weiss DJ. Current Status of Cell-Based Therapies for Respiratory Virus Infections: Applicability to COVID-19. Eur Respir J (2020) 55(6):2000858. doi: 10.1183/13993003.00858-2020
120. Schafer R, Spohn G, Bechtel M, Bojkova D, Baer PC, Kuci S, et al. Human Mesenchymal Stromal Cells Are Resistant to SARS-CoV-2 Infection Under Steady-State, Inflammatory Conditions and in the Presence of SARS-CoV-2-Infected Cells. Stem Cell Rep (2021) 16(3):419–27. doi: 10.1016/j.stemcr.2020.09.003
121. Avanzini MA, Mura M, Percivalle E, Bastaroli F, Croce S, Valsecchi C, et al. Human Mesenchymal Stromal Cells do Not Express ACE2 and TMPRSS2 and Are Not Permissive to SARS-CoV-2 Infection. Stem Cells Transl Med (2021) 10(4):636–42. doi: 10.1002/sctm.20-0385
122. Iglesias M, Butrón P, Torre-Villalvazo I, Torre-Anaya EA, Sierra-Madero J, Rodriguez-Andoney JJ, et al. Mesenchymal Stem Cells for the Compassionate Treatment of Severe Acute Respiratory Distress Syndrome Due to COVID 19. Aging Dis (2021) 12(2):360–70. doi: 10.14336/ad.2020.1218
123. Hashemian SR, Aliannejad R, Zarrabi M, Soleimani M, Vosough M, Hosseini SE, et al. Mesenchymal Stem Cells Derived From Perinatal Tissues for Treatment of Critically Ill COVID-19-Induced ARDS Patients: A Case Series. Stem Cell Res Ther (2021) 12(1):91. doi: 10.1186/s13287-021-02165-4
124. Shu L, Niu C, Li R, Huang T, Wang Y, Huang M, et al. Treatment of Severe COVID-19 With Human Umbilical Cord Mesenchymal Stem Cells. Stem Cell Res Ther (2020) 11(1):361. doi: 10.1186/s13287-020-01875-5
125. Xu X, Jiang W, Chen L, Xu Z, Zhang Q, Zhu M, et al. Evaluation of the Safety and Efficacy of Using Human Menstrual Blood-Derived Mesenchymal Stromal Cells in Treating Severe and Critically Ill COVID-19 Patients: An Exploratory Clinical Trial. Clin Trans Med (2021) 11(2):e297. doi: 10.1002/ctm2.297
126. Meng F, Xu R, Wang S, Xu Z, Zhang C, Li Y, et al. Human Umbilical Cord-Derived Mesenchymal Stem Cell Therapy in Patients With COVID-19: A Phase 1 Clinical Trial. Signal Transduct Target Ther (2020) 5(1):172. doi: 10.1038/s41392-020-00286-5
127. Feng Y, Huang J, Wu J, Xu Y, Chen B, Jiang L, et al. Safety and Feasibility of Umbilical Cord Mesenchymal Stem Cells in Patients With COVID-19 Pneumonia: A Pilot Study. Cell Prolif (2020) 53(12):e12947. doi: 10.1111/cpr.12947
128. Leng Z, Zhu R, Hou W, Feng Y, Yang Y, Han Q, et al. Transplantation of ACE2(-) Mesenchymal Stem Cells Improves the Outcome of Patients With COVID-19 Pneumonia. Aging Dis (2020) 11(2):216–28. doi: 10.14336/ad.2020.0228
129. Sengupta V, Sengupta S, Lazo A, Woods P, Nolan A, Bremer N. Exosomes Derived From Bone Marrow Mesenchymal Stem Cells as Treatment for Severe COVID-19. Stem Cells Dev (2020) 29(12):747–54. doi: 10.1089/scd.2020.0080
130. Dilogo IH, Aditianingsih D, Sugiarto A, Burhan E, Damayanti T, Sitompul PA, et al. Umbilical Cord Mesenchymal Stromal Cells as Critical COVID-19 Adjuvant Therapy: A Randomized Controlled Trial. Stem Cells Transl Med (2021) 10(9):1279–87. doi: 10.1002/sctm.21-0046
131. Shi L, Huang H, Lu X, Yan X, Jiang X, Xu R, et al. Effect of Human Umbilical Cord-Derived Mesenchymal Stem Cells on Lung Damage in Severe COVID-19 Patients: A Randomized, Double-Blind, Placebo-Controlled Phase 2 Trial. Signal Transduct Target Ther (2021) 6(1):58. doi: 10.1038/s41392-021-00488-5
132. Lanzoni G, Linetsky E, Correa D, Messinger Cayetano S, Alvarez RA, Kouroupis D, et al. Umbilical Cord Mesenchymal Stem Cells for COVID-19 Acute Respiratory Distress Syndrome: A Double-Blind, Phase 1/2a, Randomized Controlled Trial. Stem Cells Transl Med (2021) 10(5):660–73. doi: 10.1002/sctm.20-0472
133. George MJ, Prabhakara K, Toledano-Furman NE, Gill BS, Wade CE, Cotton BA, et al. Procoagulant In Vitro Effects of Clinical Cellular Therapeutics in a Severely Injured Trauma Population. Stem Cells Transl Med (2020) 9(4):491–8. doi: 10.1002/sctm.19-0206
134. Mei SH, McCarter SD, Deng Y, Parker CH, Liles WC, Stewart DJ. Prevention of LPS-Induced Acute Lung Injury in Mice by Mesenchymal Stem Cells Overexpressing Angiopoietin 1. PLoS Med (2007) 4(9):e269. doi: 10.1371/journal.pmed.0040269
135. Florian M, Wang JP, Deng Y, Souza-Moreira L, Stewart DJ, Mei SHJ. Gene Engineered Mesenchymal Stem Cells: Greater Transgene Expression and Efficacy With Minicircle vs. Plasmid DNA Vectors in a Mouse Model of Acute Lung Injury. Stem Cell Res Ther (2021) 12(1):184. doi: 10.1186/s13287-021-02245-5
136. Wang C, Lv D, Zhang X, Ni ZA, Sun X, Zhu C. Interleukin-10-Overexpressing Mesenchymal Stromal Cells Induce a Series of Regulatory Effects in the Inflammatory System and Promote the Survival of Endotoxin-Induced Acute Lung Injury in Mice Model. DNA Cell Biol (2018) 37(1):53–61. doi: 10.1089/dna.2017.3735
137. Han J, Lu X, Zou L, Xu X, Qiu H. E-Prostanoid 2 Receptor Overexpression Promotes Mesenchymal Stem Cell Attenuated Lung Injury. Hum Gene Ther (2016) 27(8):621–30. doi: 10.1089/hum.2016.003
138. Chen J, Zhang X, Xie J, Xue M, Liu L, Yang Y, et al. Overexpression of TGFbeta1 in Murine Mesenchymal Stem Cells Improves Lung Inflammation by Impacting the Th17/Treg Balance in LPS-Induced ARDS Mice. Stem Cell Res Ther (2020) 11(1):311. doi: 10.1186/s13287-020-01826-0
139. Shao Y, Zhou F, He D, Zhang L, Shen J. Overexpression of CXCR7 Promotes Mesenchymal Stem Cells to Repair Phosgene-Induced Acute Lung Injury in Rats. BioMed Pharmacother (2019) 109:1233–9. doi: 10.1016/j.biopha.2018.10.108
140. Li Z, Song Y, Yuan P, Guo W, Hu X, Xing W, et al. Antibacterial Fusion Protein BPI21/LL-37 Modification Enhances the Therapeutic Efficacy of hUC-MSCs in Sepsis. Mol Ther (2020) 28(8):1806–17. doi: 10.1016/j.ymthe.2020.05.014
141. Chen X, Wu S, Tang L, Ma L, Wang F, Feng H, et al. Mesenchymal Stem Cells Overexpressing Heme Oxygenase-1 Ameliorate Lipopolysaccharide-Induced Acute Lung Injury in Rats. J Cell Physiol (2019) 234(5):7301–19. doi: 10.1002/jcp.27488
142. He HL, Liu L, Chen QH, Cai SX, Han JB, Hu SL, et al. MSCs Modified With ACE2 Restore Endothelial Function Following LPS Challenge by Inhibiting the Activation of RAS. J Cell Physiol (2015) 230(3):691–701. doi: 10.1002/jcp.24794
143. Yang JX, Zhang N, Wang HW, Gao P, Yang QP, Wen QP. CXCR4 Receptor Overexpression in Mesenchymal Stem Cells Facilitates Treatment of Acute Lung Injury in Rats. J Biol Chem (2015) 290(4):1994–2006. doi: 10.1074/jbc.M114.605063
144. Zhang X, Chen J, Xue M, Tang Y, Xu J, Liu L, et al. Overexpressing P130/E2F4 in Mesenchymal Stem Cells Facilitates the Repair of Injured Alveolar Epithelial Cells in LPS-Induced ARDS Mice. Stem Cell Res Ther (2019) 10(1):74. doi: 10.1186/s13287-019-1169-1
145. Xu XP, Huang LL, Hu SL, Han JB, He HL, Xu JY, et al. Genetic Modification of Mesenchymal Stem Cells Overexpressing Angiotensin II Type 2 Receptor Increases Cell Migration to Injured Lung in LPS-Induced Acute Lung Injury Mice. Stem Cells Transl Med (2018) 7(10):721–30. doi: 10.1002/sctm.17-0279
146. Ahmad T, Mukherjee S, Pattnaik B, Kumar M, Singh S, Kumar M, et al. Miro1 Regulates Intercellular Mitochondrial Transport & Enhances Mesenchymal Stem Cell Rescue Efficacy. EMBO J (2014) 33(9):994–1010. doi: 10.1002/embj.201386030
147. Wang H, Yang YF, Zhao L, Xiao FJ, Zhang QW, Wen ML, et al. Hepatocyte Growth Factor Gene-Modified Mesenchymal Stem Cells Reduce Radiation-Induced Lung Injury. Hum Gene Ther (2013) 24(3):343–53. doi: 10.1089/hum.2012.177
148. Saito S, Nakayama T, Hashimoto N, Miyata Y, Egashira K, Nakao N, et al. Mesenchymal Stem Cells Stably Transduced With a Dominant-Negative Inhibitor of CCL2 Greatly Attenuate Bleomycin-Induced Lung Damage. Am J Pathol (2011) 179(3):1088–94. doi: 10.1016/j.ajpath.2011.05.027
149. Huleihel L, Sellares J, Cardenes N, Álvarez D, Faner R, Sakamoto K, et al. Modified Mesenchymal Stem Cells Using miRNA Transduction Alter Lung Injury in a Bleomycin Model. Am J Physiol Lung Cell Mol Physiol (2017) 313(1):L92–l103. doi: 10.1152/ajplung.00323.2016
150. Wei X, Yi X, Lv H, Sui X, Lu P, Li L, et al. MicroRNA-377-3p Released by Mesenchymal Stem Cell Exosomes Ameliorates Lipopolysaccharide-Induced Acute Lung Injury by Targeting RPTOR to Induce Autophagy. Cell Death Dis (2020) 11(8):657. doi: 10.1038/s41419-020-02857-4
151. Li QC, Liang Y, Su ZB. Prophylactic Treatment With MSC-Derived Exosomes Attenuates Traumatic Acute Lung Injury in Rats. Am J Physiol Lung Cell Mol Physiol (2019) 316(6):L1107–L17. doi: 10.1152/ajplung.00391.2018
152. Millar JE, von Bahr V, Malfertheiner MV, Ki KK, Redd MA, Bartnikowski N, et al. Administration of Mesenchymal Stem Cells During ECMO Results in a Rapid Decline in Oxygenator Performance. Thorax (2018) 74(2):194–6. doi: 10.1136/thoraxjnl-2017-211439
153. Millar JE, Bartnikowski N, Passmore MR, Obonyo NG, Malfertheiner MV, von Bahr V, et al. Combined Mesenchymal Stromal Cell Therapy and Extracorporeal Membrane Oxygenation in Acute Respiratory Distress Syndrome. A Randomized Controlled Trial in Sheep. Am J Respir Crit Care Med (2020) 202(3):383–92. doi: 10.1164/rccm.201911-2143OC
154. Kim H, Darwish I, Monroy MF, Prockop DJ, Liles WC, Kain KC. Mesenchymal Stromal (Stem) Cells Suppress Pro-Inflammatory Cytokine Production But Fail to Improve Survival in Experimental Staphylococcal Toxic Shock Syndrome. BMC Immunol (2014) 15:1. doi: 10.1186/1471-2172-15-1
155. Sepulveda JC, Tome M, Fernandez ME, Delgado M, Campisi J, Bernad A, et al. Cell Senescence Abrogates the Therapeutic Potential of Human Mesenchymal Stem Cells in the Lethal Endotoxemia Model. Stem Cells (2014) 32(7):1865–77. doi: 10.1002/stem.1654
156. Hackel A, Aksamit A, Bruderek K, Lang S, Brandau S. TNF-α and IL-1β Sensitize Human MSC for IFN-γ Signaling and Enhance Neutrophil Recruitment. Eur J Immunol (2021) 51(2):319–30. doi: 10.1002/eji.201948336
157. Wang LT, Wang HH, Chiang HC, Huang LY, Chiu SK, Siu LK, et al. Human Placental MSC-Secreted IL-1β Enhances Neutrophil Bactericidal Functions During Hypervirulent Klebsiella Infection. Cell Rep (2020) 32(13):108188. doi: 10.1016/j.celrep.2020.108188
Keywords: COVID-19, MSCs therapies, ARDS, sepsis, SARS-CoV-2
Citation: Xu Z, Huang Y, Zhou J, Deng X, He W, Liu X, Li Y, Zhong N and Sang L (2021) Current Status of Cell-Based Therapies for COVID-19: Evidence From Mesenchymal Stromal Cells in Sepsis and ARDS. Front. Immunol. 12:738697. doi: 10.3389/fimmu.2021.738697
Received: 09 July 2021; Accepted: 13 September 2021;
Published: 01 October 2021.
Edited by:
Aldo Tagliabue, Italian National Research Council, ItalyReviewed by:
Saikat Majumder, University of Pittsburgh, United StatesCopyright © 2021 Xu, Huang, Zhou, Deng, He, Liu, Li, Zhong and Sang. This is an open-access article distributed under the terms of the Creative Commons Attribution License (CC BY). The use, distribution or reproduction in other forums is permitted, provided the original author(s) and the copyright owner(s) are credited and that the original publication in this journal is cited, in accordance with accepted academic practice. No use, distribution or reproduction is permitted which does not comply with these terms.
*Correspondence: Ling Sang, c29ueXNhbmc5OTlAYWxpeXVuLmNvbQ==; Nanshan Zhong, bmFuc2hhbkB2aXAuMTYzLmNvbQ==
†These authors have contributed equally to this work
Disclaimer: All claims expressed in this article are solely those of the authors and do not necessarily represent those of their affiliated organizations, or those of the publisher, the editors and the reviewers. Any product that may be evaluated in this article or claim that may be made by its manufacturer is not guaranteed or endorsed by the publisher.
Research integrity at Frontiers
Learn more about the work of our research integrity team to safeguard the quality of each article we publish.