- 1Department of Medicine, Duke University Medical Center, Durham, NC, United States
- 2Department of Pharmacology and Cancer Biology, Duke University Medical Center, Durham, NC, United States
- 3Division of Hematology, Department of Internal Medicine, The Ohio State University Comprehensive Cancer Center, Columbus, OH, United States
Vaccinia virus (VV) is the most studied member of the poxvirus family, is responsible for the successful elimination of smallpox worldwide, and has been developed as a vaccine vehicle for infectious diseases and cancer immunotherapy. We have previously shown that the unique potency of VV in the activation of CD8+ T cell response is dependent on efficient activation of the innate immune system through Toll-like receptor (TLR)-dependent and -independent pathways. However, it remains incompletely defined what regulate CD8+ T cell response to VV infection. In this study, we showed that γδT cells play an important role in promoting CD8+ T cell response to VV infection. We found that γδT cells can directly present viral antigens in the context of MHC-I for CD8+ T cell activation to VV in vivo, and we further demonstrated that cell-intrinsic MyD88 signaling in γδT cells is required for activation of γδT cells and CD8+ T cells. These results illustrate a critical role for γδT cells in the regulation of adaptive T cell response to viral infection and may shed light on the design of more effective vaccine strategies based on manipulation of γδT cells.
Highlights
Targeting the immune systems has powerful potentials to treat many disorders, such as cancers and viral infections. By understanding how the immune system responds to model infections, we can better determine strategies to manipulate our immune systems. Vaccinia virus is responsible for the worldwide elimination of smallpox and produces one of the longest immune responses known in humans. We know from previous findings that NK cells are required for initial immune response and CD8+ T cells are required for the elimination of the virus. How CD8+ T cells are activated in response to vaccinia virus is not fully understood. This manuscript found that γδT cells activate CD8+ T cells in response to vaccinia virus infection through MyD88 pathway.
Introduction
Vaccinia virus (VV), an enveloped double-stranded DNA virus, is a member of the Orthopoxvirus genus of the Poxviridae family. It has approximately a 200kb genome that encodes all the proteins required for cytoplasmic viral replication in host cells (1). It is responsible for the worldwide elimination of smallpox, and as a result has been developed as recombinant vaccine vehicle for infectious diseases and cancer immunotherapy (2). It is unique among viral agents to be able to elicit both potent and long-lasting immunity (3). Though its natural route of infection is via the skin, many studies have noted that intraperitoneal, intravenous, and intramuscular modes of VV inoculation provides similar clinical efficacy in both mice and humans (4–6).
We have previously shown that the unique potency of intraperitoneal VV inoculation in the activation of CD8+ T cell responses is dependent on efficient activation of the innate immune system through Toll-like receptor (TLR)-dependent and -independent pathways (7, 8). Specifically, we have demonstrated that intrinsic TLR2-MyD88 (myeloid differentiation factor 88) signaling in CD8+ T cells is critical for clonal expansion and long-lived memory formation (9). In addition, TLR-independent production of type I interferons (IFNs) is also important for efficient CD8+ T cell responses (7, 10). However, despite these advances, the mechanisms by conventional antigen-presenting cells are unable to fully explain the unique potency of VV in the activation of CD8+ T cell responses.
γδT cells are a unique population of lymphocytes that exert a strong influence on the immune system (11). Previous studies have also shown that there are several subpopulations of γδT cells with distinct functions. However, a definitive system to categorize the different subpopulations has remained elusive. Initial proposals to define γδT cells based on their different TCR expression in both mice and humans have had to be revisited (12–17). As a result, we assessed the effects of γδT cells as a population in this study.
γδT cells act as a bridge between the innate and adaptive immune responses, with characteristics of both. They can exert direct cytotoxicity and enhance the adaptive immune responses (18–21). Studies have found that γδT cells are important in the immune response against many mycobacterial, parasitic, and viral infections (22–35). Similarly, previous studies have demonstrated that γδT cells express CD80 and CD86 at similar levels to professional antigen presenting cells and is able to promote CD8+ T cells activation (19, 20). However, it remains largely unknown exactly how γδT cells promote adaptive immune responses.
In this study, we found that γδT cells play a critical role in promoting CD8+ T cell response to VV infection in vivo. We showed that activation of γδT cells by VV presented viral antigens in the context of MHC class I for CD8+ T cell activation. We further demonstrated that cell-intrinsic MyD88 signaling in γδT cells is required for γδT cell activation and CD8+ T cell responses. These results demonstrated a critical role for γδT cells in the regulation of CD8+ T cell response to viral infection and may shed light on the design of more effective vaccine strategies based on manipulation of γδT cells.
Materials and Methods
Animals
Eight- to ten-week-old C57BL/6, δTCR-/-, OT-1, and β2m-/- mice were purchased from The Jackson Laboratory. MyD88-/- on C57BL/6 background were kindly provided by Shizuo Akira (Osaka University, Osaka, Japan). All experiments involving the use of mice were done in accordance with protocols approved by the Animal Care and Use Committee at Duke University and the Ohio State University.
Vaccinia Virus
Western Reserve (WR) strain of VV was purchased from American Type Culture Collection (Manassas, VA). Recombinant VV-OVA was provided by Jonathan Yewdell at NIH. The viruses were grown in TK-143B cells and purified by centrifugation through a 35% sucrose cushion as previously described (36). The titer was determined by plaque assay on TK-143B cells and subsequently stored at -80˚C until use. For in vivo studies, 5x106 pfu of live VV in 0.1mL Tris-Cl was injected into mice intraperitoneally, unless otherwise specified.
DC Culture
Femurs and tibiae of mice were harvested and bone marrow cells were flushed with DC medium (RPMI-1640 with 5% fetal bovine serum [FBS], 2mM L-glutamine, 10mM HEPES, 50µM β-mercaptoethanol, 100 IU/mL penicillin, and 100 IU/mL streptomycin), as previously described (36). After lysis of red blood cells with ACK lysis buffer (Gibco Life Technologies, Waltham, MA), the bone marrow cells were cultured in 6-well plates at density of 3x106 cells/mL in 3mL DC medium in the presence of mouse granulocyte macrophage-colony stimulating factor (GM-CSF; 1000 U/mL; R&D Systems, Minneapolis, MN) and interleukin 4 (IL-4; 500 U/mL; R&D Systems). GM-CSF and IL-4 were replenished on day 2 and 4. On day 5, DCs were harvested, and CD11c+ DCs were transferred onto a new 24-well plate at a density of 0.85 x 106 cells/mL in 2mL DC media.
Isolation of γδT Cells
Splenocytes were harvested from C57BL/6 mice 2 days after peritoneal inoculation with VV. γδT cells were isolated from harvested splenocytes with pan-T cell microbeads, followed by anti-γδTCR microbeads (Miltenyi Biotec, Auburn, CA). The isolated γδT cells were assessed via flow cytometry for confirmation.
Isolation of Immune Cells From Peritoneal Cavity
On the day of harvest, mice were euthanized in accordance with protocols approved by the Animal Care and Use Committee at Duke University and the Ohio State University. A midline incision was made along the Linea alba, without disrupting the peritoneal membrane. After exposing the outer peritoneum, a 18G needle and syringe with 4mL of 1x phosphate buffered saline (PBS) was injected into the abdomen, followed by gentle agitation. The intraperitoneal fluid was then isolated for further analysis.
CD8+ T Cell Proliferation Assay
CD8+ T cells were isolated from splenocytes of OT-I mice on C57BL/6 background using anti-CD8a microbeads (Miltenyi Biotec), and then fluorescently labeled with carboxyfluorescein succinimidyl ester (CFSE). Labeled CD8+ T cells and OVA-I peptide were then cocultured with matured DCs or VV-activated γδT cells at 1:1 ratio in 96 well plates. The cells were incubated at 37˚C for 72 hours, and then assessed via flow cytometry.
Adoptive Transfer of γδT Cells
Naïve γδT cells were isolated from pooled spleens and lymph nodes of wild-type or MyD88-/- mice on C57BL/6 background, with pan-T cell microbeads, followed by anti-γδTCR microbeads (Miltenyi Biotec). The isolated cells were confirmed via flow cytometry and suspended in 1xPBS. The cells were then injected intravenously via the tail vein into δTCR-/- or MyD88-/- mice on C57BL/6 background at 1x106 cells/mouse, unless otherwise specified.
Antibodies and Flow Cytometry Analysis
The list of used antibodies is provided in Table 1. Cells were suspended in 1xPBS buffer with 2% heat-inactivated FBS and 0.1% sodium azide. After staining, cells were washed twice, and analyzed with FACSCanto flow cytometer (BD Biosciences) using FlowJo software (BD Biosciences).
Intracellular Cytokine Staining
Splenocytes were re-stimulated specifically for CD8+ T cells with 2 µg/mL B8R peptide (TSYKFESV, MBL International) with 5 µg/mL Brefeldin A (Invitrogen) for 5 hours at 2 µg/mL at 37°C. Splenocytes or mesenteric lymph node cells were stimulated specifically for γδT cells with 50 ng/mL Ionomycin, 100 ng/mL PMA, and 5 µg/mL Brefeldin A for 3 hours at 37°C. After staining with cell surface markers, the cells were fixed and permeabilized with Cytoperm/Cytofix solution (BD Biosciences) for 20 minutes and incubated with anti-IFN-γ antibodies for 30 minutes. The cells were washed twice with Permeabilization buffer (BD Biosciences) and analyzed with a FACSCanto flow cytometer using FlowJo software (BD Biosciences).
MHC/Peptide Tetramer
The VV-specific epitope B8R20–27, TSYKFESV, is a synthetic peptide based on modified vaccinia virus Ankara (MVA) sequence (37). Peptide MHC I tetramers consisting of B8R20–27/Kb conjugated to phycoerythrin (PE) Mallophycocyanin were obtained from the NIH Tetramer Core Facility (Emory University, Atlanta, GA, USA). Cells were stained with the tetramer for 30 minutes at room temperature in the dark together with surface staining and subsequently analyzed by flow cytometry.
Plaque Assay
Viral load in the peritoneum is measured by plaque-forming assay as described (38). Mice were euthanized 3 days after infection, and the peritoneum is washed with PBS, and stored at -80˚C. Peritoneum washings were homogenized with bead homogenizer (MP Biomedical, Irvine, CA), and serial dilutions were performed to determine virus titers by plaque assay on confluent TK-143B cells.
VV Quantitative Real-Time PCR
3 days post-VV inoculation, total DNA was isolated from peritoneal fluid as previously described (39). Real-time quantitative PCR was used to analyzed VV E3L gene in duplicates using SYBR Green Real-Time PCR Master Mix (Bio-Rad). PCR conditions were 50˚C for 2 minutes, 95˚C for 10 minutes, 40 cycles of 95˚C for 15 seconds, and 60˚C for 50 seconds, followed by a melt curve capture on CFX96 Touch Real-Time PCR Detection System (Bio-Rad, Hercules, CA). Primer sequences are provided in Table 2. Relative gene expression levels for each respective gene were calculated using threshold cycle (2-ΔΔCT) and normalized to GAPDH.
Statistical Analysis
Results are expressed as mean ± SEM. Differences between groups were examined for statistical significance using ANOVA with post-hoc t-test, Mann-Whitney test, or unpaired student t-test. P-values less than 0.05 are significant.
Results
γδT cells Are Required for CD8+ T Cell Response to VV
To address whether γδT cells play a role in regulating CD8+ T cell responses, we first examined the activation status of γδT cells in response to VV infection in vivo. C57BL/6 mice were injected with VV intraperitoneally, and at different time points after infection, γδT cells were examined for IFN-γ production.
We found that in both spleen (Figure 1A) and peritoneal cavity (Figure 1B), IFN-γ+ γδT cell count reached its peak around day 4 following VV infection, with subsequent decline in the days following. This contrasts with VV-specific CD8+ T cell response in that B8R+ IFN-γ+ CD8+ T cell count reached its peak around day 7 (Figure 1). These results indicated that the activation of γδT cells peaked prior to that of CD8+ T cells. We found that naïve γδT cells secreted IFN-γ after stimulation, therefore we subsequently determined IFN-γ gating within each experiment against fluorescence minus one (FMO) controls (Figures 1C, D), and between inoculated versus naïve mice (data not shown).
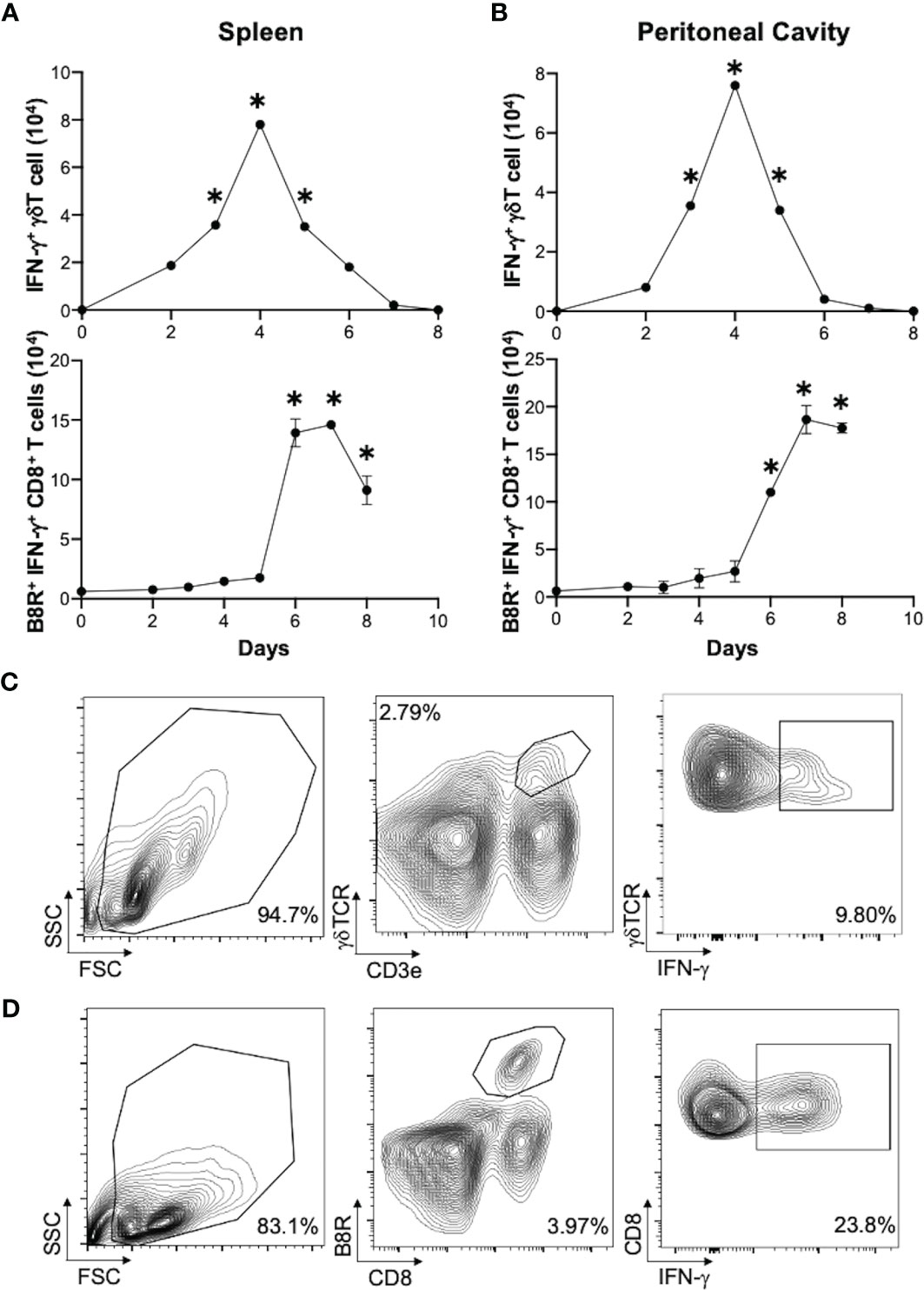
Figure 1 Activation of γδT cells in response to VV infection. 5 x 106 pfu of VV were injected into wild-type C57BL/6 mice intraperitoneally. 2, 3, 4, 5, 6, 7 and 8 days after infection, CD8+ T cells from spleen and peritoneal cavity were assayed for IFN-γ production by intracellular staining by FACS, and IFN-γ+ γδT cells and CD8+ T cells from the spleen (A) spleen and peritoneal cavity (B) are shown. IFN-γ+ γδT cells are first gated on CD3e+ and γδTCR+, then assessed on IFN-γ expression. VV-specific B8R+ IFN-γ+ CD8+ T cells were first gated on B8R+ CD8+ T cells, and then assessed for IFN-γ expression. The mean of each time point is plotted. Representative of 2 independent studies, each with 3 biological replicates. Gating strategy used to generate the data are represented by (C, D). The gating for IFN-γ+ γδT cells is determined against IFN-γ+ γδT cells from control naïve mice for each experiment. Mann-Whitney test, *P < 0.05.
We next determined if γδT cells play a role in CD8+ T cell response to VV. We inoculated wild-type (WT) and δTCR-/- C57BL/6 mice intraperitoneally with VV and assessed for VV-specific B8R+ CD8+ T cell activation 7 days post-inoculation. B8R is a VV epitope that is recognized by VV-specific CD8+ T cells; B8R+ CD8+ T cells are specifically activated by VV. At 3 days post-transfer, we could only minimally detect the adoptively transferred γδT cells on flow cytometry, compared to δTCR-/- mice without adoptive transfer (Figures 2A, B; Supplementary Figure 1). However, we found that there was a significant decrease in peritoneal VV titer in δTCR-/- mice adoptively transferred with WT γδT cells, compared to VV-inoculated δTCR-/- mice alone (Supplementary Figure 2; P < 0.01). Similarly, we found that there is a significant decrease in VV-specific B8R+ (Figures 2C, D) and functional IFN-γ+ (Figures 2E, F) CD8+ T cells in δTCR-/- mice that lack γδT cells at 7 days post-transfer, compared to that of WT mice (P < 0.005). We subsequently found that this defect can be rescued with adoptively transferred WT γδT cells. However, VV inoculation of δTCR-/- mice with adoptive transfer of WT γδT cells had significantly greater VV-specific B8R+ and IFN-γ+ CD8+ T cells, compared to that of δTCR-/- mice with VV inoculation alone (P < 0.005). VV-specific B8R+ and IFN-γ+ CD8+ T cell response in δTCR-/- with adoptive transfer of WT γδT cells following VV inoculation also approximated the same response as WT mice with VV inoculation alone (P not significant). This suggested that γδT cells play a critical role in CD8+ T cell activation following VV infection.
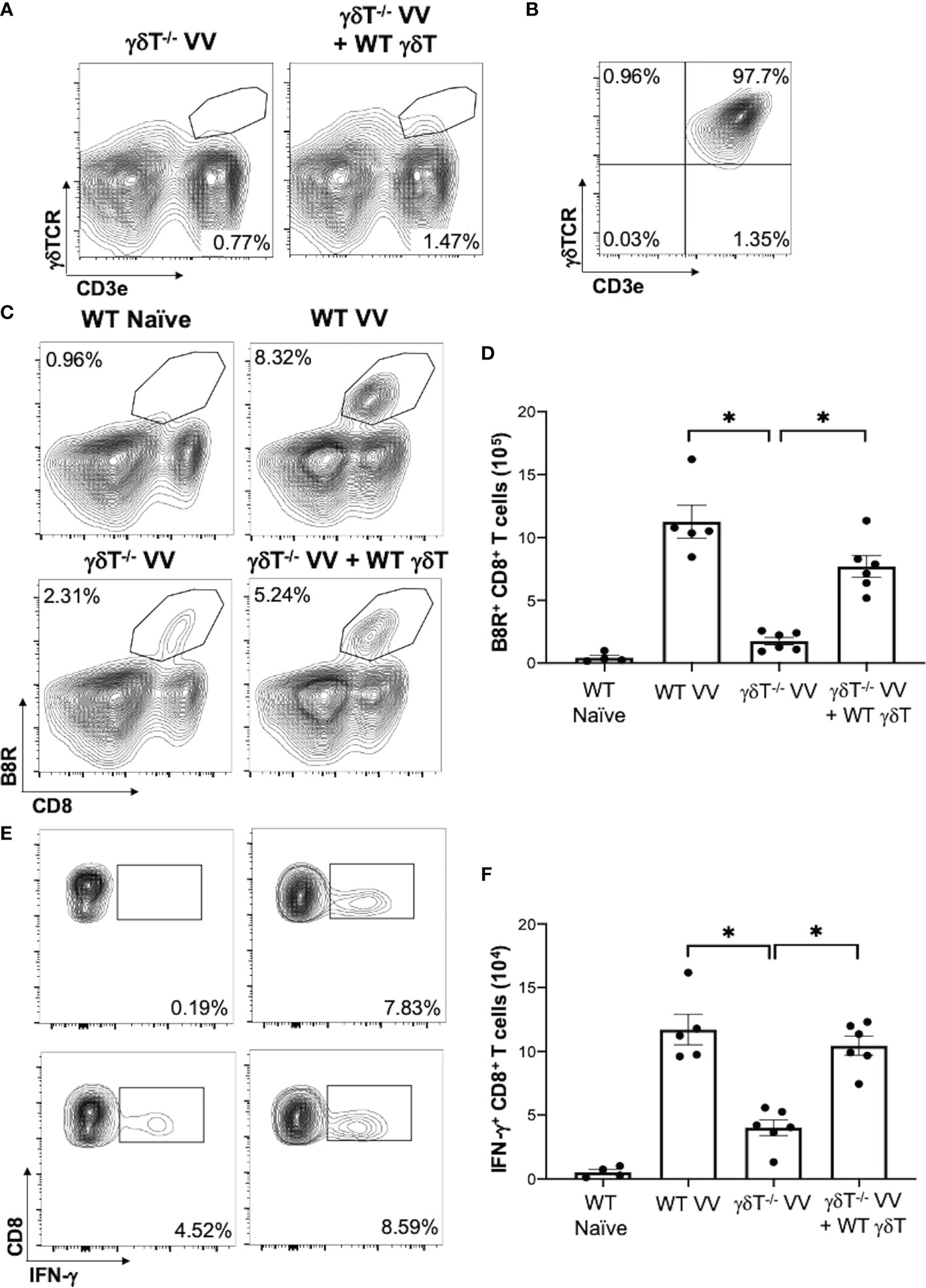
Figure 2 γδT cells is required for CD8+ T cell response to VV. 5 x 106 pfu of VV were injected intraperitoneally into wild-type C57BL/6 (WT) or δTCR-/- mice (γδT-/-). Concurrently, a different population of δTCR-/- mice were also adoptively transferred with 1 x 106 WT γδT cells (γδT-/- VV + WT γδT), followed by VV inoculation. (A) 3 days post-adoptive transfer and VV inoculation, there is a detectable, but nonsignificant, population of γδT cells in the mesenteric lymph nodes. (B) Representative plot of purified γδT cells used for adoptive transfer. 7 days post-inoculation, the spleens were harvested and stained for B8R+ CD8+ T cells by tetramer and IFN-γ+ CD8+ T cells by intracellular staining. (C) Representative FACS plots first gated on CD8+ CD4- T cells and then plotted against B8R+ and CD8+ T cells for B8R+ CD8+ T cells. (E) Representative FACS plots first gated on CD8+ CD4- T cells and then assessed for IFN-γ expression. (D, F) Quantification of FACS plots. Values are mean ± SEM, representative of 3 independent studies, each with at least 3 biological replicates. ANOVA with post-hoc t-test, *P < 0.005.
VV Activates γδT Cells to Present MHC-I Peptide and Upregulate CD80 and CD86
To determine how γδT cells promote the activation CD8+ T cells to VV infection, we explored whether γδT cells contributed to signals that are required to activate CD8+ T cells: 1) direct presentation of VV-specific peptide on MHC-I, 2) co-stimulation with CD80 and CD86 ligands, and 3) cytokines release (40–42). To assess peptide presentation on MHC-I, we inoculated WT mice with VV or VV encoded with OVA (VV-OVA). We then assessed γδT cells for expression of H2Kb specific for SIINFEKL peptide on MHC-I. We found that there is an increase in H2Kb SIINFEKL+ γδT cells in mice inoculated with VV-OVA, compared to that of naïve or mice inoculated with VV (Figure 3A; P < 0.05).
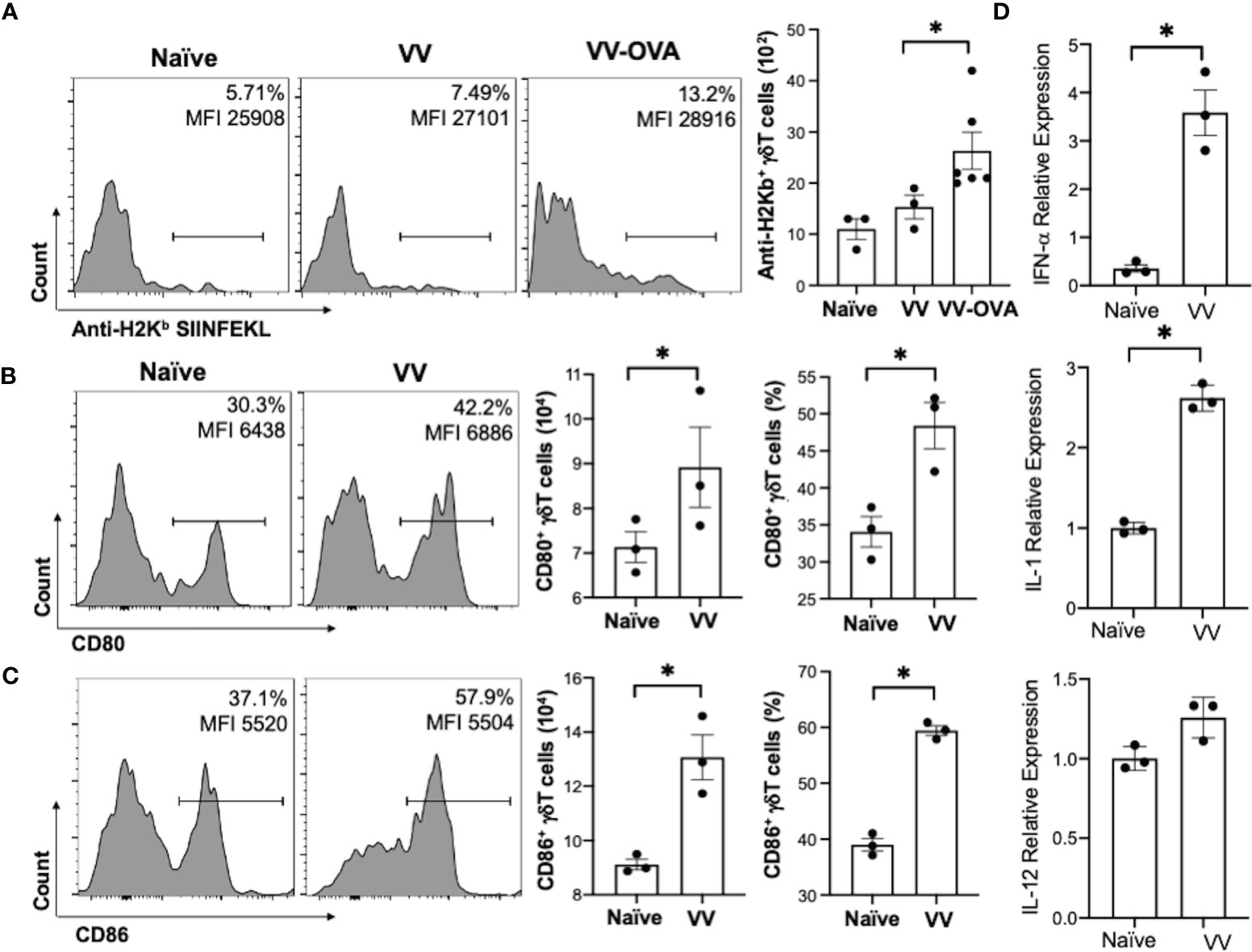
Figure 3 VV activates γδT cells to present peptide on MHC-I and upregulate CD80/CD86 expression. C57BL/6 mice were inoculated intraperitoneally with 5 x 106 pfu of VV or VV encoding OVA (VV-OVA). (A) Representative FACS plots first gated on CD3e+ and γδTCR+ T cells, and then assessed for SIINFEKL peptide expression on mouse MHC-I H2Kb via anti-H2Kb SIINFEKL in spleens harvested 2 days post-infection. (B, C) 4 days post-inoculation, splenocytes were extracted and stained for CD3e+ and γδTCR+ T cells. The gated cells were then assessed for (B) CD80 and (C) CD86 expression. (D) 4 days following VV inoculation, γδT cells were isolated from splenocytes from naïve and VV-inoculated WT mice with MACS. Total RNA was extracted from the isolated cells and assessed by qRT-PCR for IFN-a, IL-1, and IL-12 expression. Values are mean ± SEM, representative of 3 independent studies, each with at least 3 biological replicates. ANOVA with post-hoc t-test, *P < 0.05.
We also found that following VV infection, there is an increase in CD80 and CD86 expression on the surface of γδT cells by flow cytometry. CD86 is expressed first as the initial co-stimulatory ligand, and CD80 is expressed after antigen-presenting-cell activation (43). We found that 4 days post-inoculation, there is a corresponding increase in CD80 (Figure 3B; P < 0.05), and a significant increase in CD86 (Figure 3C; P < 0.01). These results suggests that γδT cells could provide the necessary signals for CD8+ T cell activation after VV infection.
Signal 3 of effector CD8+ T cell activation is mainly associated with type I interferon, IL-1, and IL-12 (40, 44, 45). 4 days post-VV inoculation, we found that there is a significant increase in expression of IL-1 and IFN-α in γδT cells compared to that of naïve γδT cells (Figure 3D; P < 0.001). γδT cells also secrete a basal level of IL-12 that does not change following VV inoculation but is significantly decreased following depletion of MyD88 (Supplementary Figure 3; P < 0.001). This suggests that γδT cells can provide the necessary signals for CD8+ T cell activation after VV infection.
γδT Cells Also Directly Activate CD8+ T Cells via MHC-I
We next explored whether γδT cells directly activates CD8+ T cell following VV infection in vivo. To determine if VV can activate γδT cells to act as antigen presenting cells, we isolated γδT cells from WT C57BL/6 mice that had been inoculated intraperitoneally with VV 48 hours prior. CD8+ T cells were obtained from OT-I mice on C57BL/6 background and pulsed with CFSE. The CFSE-labeled CD8+ T cells were then co-cultured with the VV-activated γδT cells and OVA-I peptide. 72 hours after co-incubation, CD8+ T cell proliferation was assayed by CFSE dilution. As a control, the CD8+ T cells were also incubated with matured DCs and OVA-I peptide. We found that CD8+ T cells proliferated at a similar magnitude when cocultured with VV-activated γδT cells as with matured DCs (Figure 4A). The same proliferation is not seen if CD8+ T cells are incubated with γδT cells or OT-I peptide alone. This suggests that VV can activate γδT cells to become antigen presenting cells.
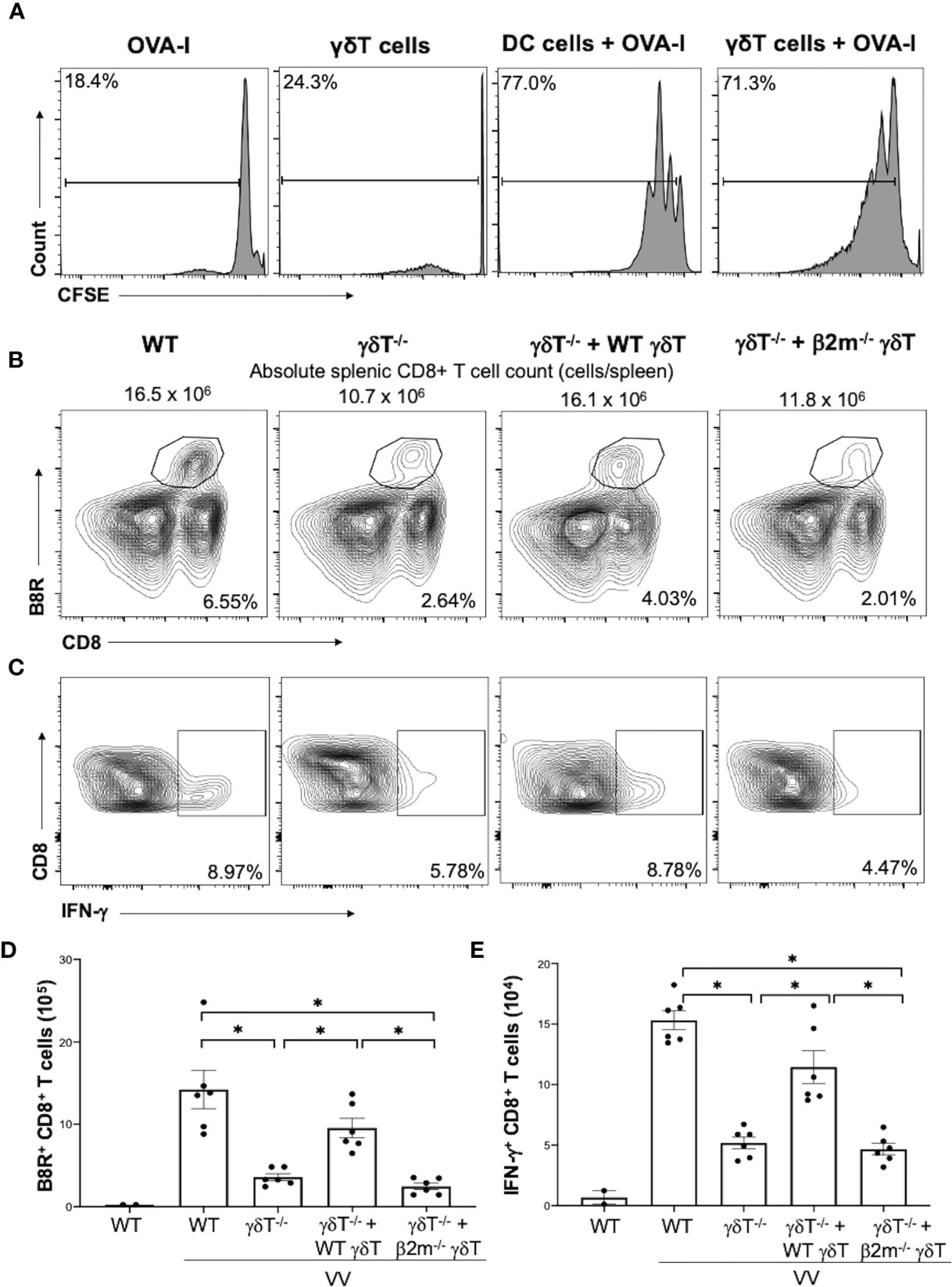
Figure 4 γδT cells directly activate CD8+ T cells via MHC-I. CD8+ T cells were obtained from splenocytes of OT-I+ mice via magnetic-activated cell sorting. (A) The CD8+ T cells were labeled with Carboxyfluorescein diacetate succinimidyl ester (CSFE) and measured for proliferation. The labeled cells were co-cultured in vitro with OVA-I peptide plus LPS-matured dendritic cells or VV-activated γδT cells for 3 days, at a ratio of 1:1. Cells were harvested and stained for CD8 and CD3e. Control CD8+ T cells were incubated with OT-I peptide or γδT cells alone. They were subsequently assessed via flow cytometry for CSFE. (B, C) 5 x 106 pfu of VV was inoculated intraperitoneally into WT or δTCR-/- mice, with and without adoptive transfer of 1 x106 cells of WT or β2m-/- γδT cells. 7 days post-inoculation, splenocytes were stained for CD8+ CD4- lymphocytes, and assessed for B8R+ and IFN-γ+ CD8+ T cells. (B) Representative FACS plots first gated on gated on CD8+ CD4- T cells and then plotted against B8R+ and CD8+ T cells for B8R+ CD8+ T cells. (C) Representative FACS plots first gated on CD8+ CD4- T cells and then assessed for IFN-γ expression. (D, E) Quantification of FACS plots. Values are mean ± SEM, representative of 3 independent studies. ANOVA with post-hoc t-test, *P < 0.005.
We then determined if γδT cells functionally acts as professional APCs via MHC-I by assessing if CD8+ T cell activation could be rescued via adoptive transfer of β2m-/- γδT cells. β2m is a necessary component of MHC-I. We found that adoptive transfer of WT γδT cells into δTCR-/- followed by VV infection significantly increased percentages and cell count of B8R+ and IFN-γ+ CD8+ T cells compared to δTCR-/- with VV infection alone (Figures 4B–E; P < 0.001). However, β2m-/- γδT cells adoptive transfer into δTCR-/- followed by VV infection resulted in similar percentages and cell count of B8R+ and IFN-γ+ CD8+ T cells as δTCR-/- with VV infection alone (Figures 4B–E; P < 0.001). We found that adoptive transfer of β2m-/- γδT cells was also unable to rescue the change in absolute CD8+ T cell count, compared to adoptive transfer of WT γδT into δTCR-/- mice (Supplementary Figure 4; P < 0.05). This suggests that γδT cells also activate CD8+ T cells via presentation of epitope on MHC-I for CD8+ T cell recognition.
MyD88 Signaling in γδT Cells Promotes CD8+ T Cell Response to VV
To determine how VV activates γδT cells for antigen presentation, we screened mice defective for innate signaling pathways and assessed for γδT cell frequency and IFN-γ+ γδT cell functional activation. VV was inoculated in WT, IFN-αβR-/-, IFN-γR-/-, TNF-αR-/-, and MyD88-/- mice and splenic cells were assessed via flow cytometry 4 days afterwards. We found that there was a significant deficit in γδT cell expansion and IFN-γ secretion in MyD88-/- mice (Figure 5), but not in mice with other defective signaling pathways. This suggests that MyD88 signaling is required for VV activation of γδT cells.
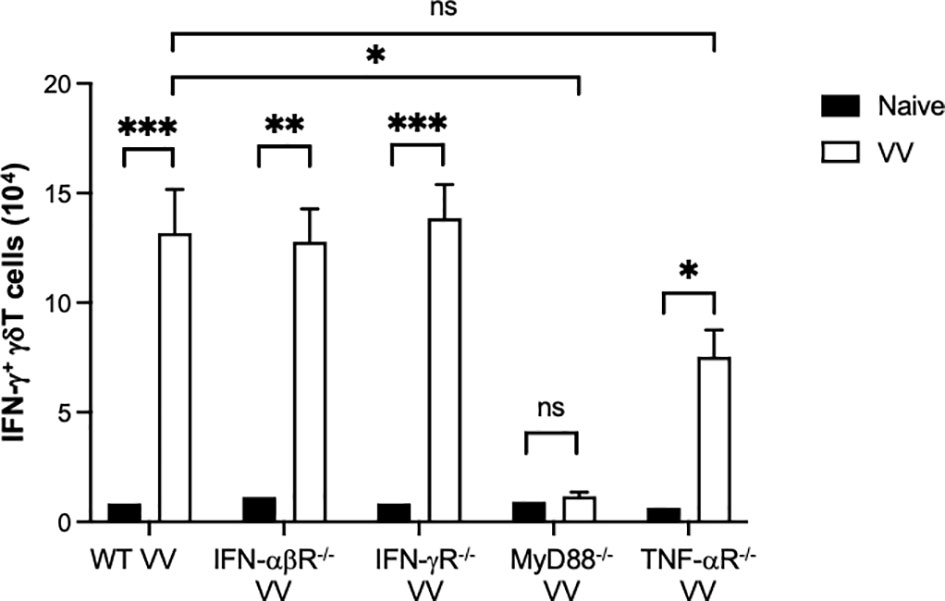
Figure 5 In vivo screen for signaling pathways required for γδT cell activation following VV infection. Wild-type (WT), IFN-αβ receptor deficient (IFN-αβR-/-), IFN-γ receptor deficient (IFN-γR-/-), MyD88 deficient (MyD88-/-), and TNF- α receptor deficient (TNF- αR-/-) mice were inoculated with VV intraperitoneally and splenocytes were harvested 4 days after inoculation. Harvested cells were gated for CD3e+ γδTCR+ T cells and subsequently assessed IFN-γ positivity. Unpaired student t-test, ***P < 0.005, **P < 0.01, *P < 0.05. NS, non significant.
We next addressed if intrinsic MyD88 activation in γδT cells is sufficient for VV activation, opposed to signaling from other cells, we adoptively transferred WT γδT cells into MyD88-/- mice and assessed for γδT cell activation. We found that adoptive transfer of WT γδT cells into MyD88-/- mice is sufficient to rescue γδT cell expansion and IFN-γ+ secretion (Figure 6; P < 0.001), suggesting intrinsic MyD88 signaling is required for activation of γδT cells by VV.
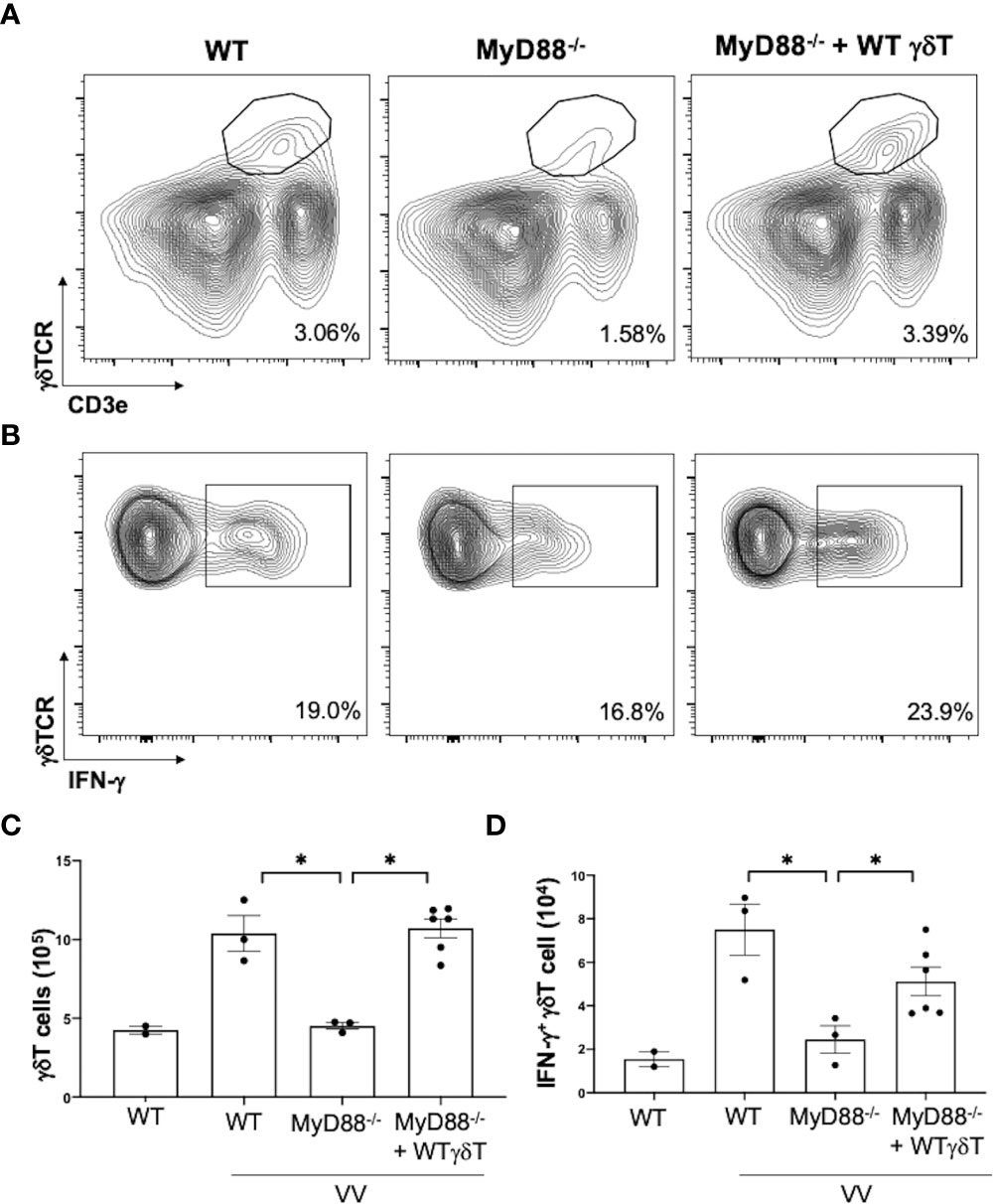
Figure 6 Intrinsic MyD88 signaling is required for activation of γδT cells by VV. 5 x 106 pfu of VV was inoculated into WT and MyD88-/- mice with and without adoptive transfer of 1 x 106 cells of WT γδT cells. Mice splenocytes were harvested 4 days after inoculation and assessed via flow cytometry. (A) Representative FACS plots of live cells plotted for CD3e+ γδTCR+ splenocytes, 4 days after VV inoculation. (B) Representative FACS plots first gated on CD3e+ γδTCR+ splenocytes, and then assessed for IFN-γ positivity. The gating for IFN-γ is determined against γδT cells from control naïve mice for each experiment. (C) Summary graph of a representative experiment for positive CD3e+ γδTCR+ T cells in mice, 4 days post-VV inoculation. (D) Summary graph of a representative experiment for IFN-γ+ CD3e+ γδTCR+ T cells in mice, 4 days post-VV inoculation. Representative of 3 independent experiments. ANOVA with post-hoc t-test, *P < 0.001.
To investigated if MyD88 signaling in γδT cells is needed for subsequent CD8+ T cell activation, we adoptively transferred WT or MyD88-/- γδT cells into δTCR-/- mice and found that there was similarly γδT cell reconstitution (Figure 7A; nonsignificant), but there was a significant decrease in VV-specific B8R+ and IFN-γ+ CD8+ T cell expansion in δTCR-/- adoptively transferred with MyD88-/- γδT cells when compared to that of WT γδT cells (Figures 7B–E; P < 0.005).
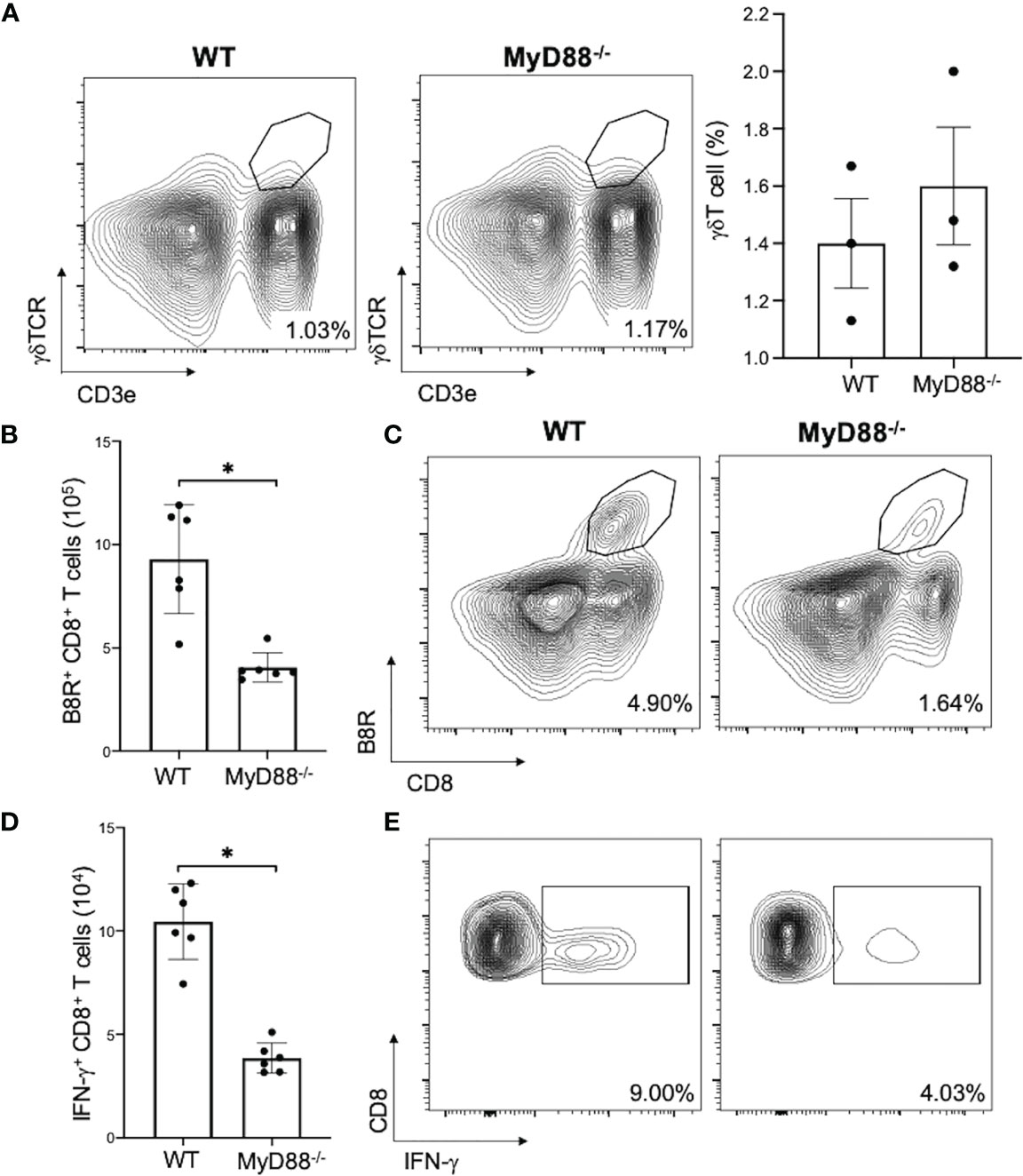
Figure 7 MyD88 is required for CD8+ T cell activation by γδT cells. δTCR-/- mice were adoptively transferred with WT or MyD88-/- γδT cells and inoculated with VV. (A) 3 days after inoculation, splenocytes were analyzed for gdT cells by FACS plots and percentages of gdT cells are shown. 7 days after inoculation, splenocytes were obtained and stained for CD8+ CD4- lymphocytes and plotted via flow cytometry for B8R+ CD8+ T cells (B, C) or IFN-γ+ CD8+ T cells. (D, E) Unpaired student T-test, *P < 0.005. Each panel is representative of 3 independent studies.
Discussion
Here we showed that VV can activate γδT cells via the MyD88 signaling pathway. We further showed that VV-activated γδT cells can present antigens to activate and induce VV-specific CD8+ T cell response. Our results further demonstrate MyD88 has a critical role in VV activation of γδT cells to promote specific CD8+ T cell response.
γδT cells represents approximately 0.7% of the peripheral blood T cells and play an important role in the integration of the innate and adaptive immune system (18). Previous studies have shown that although activation of NK cells is critical for the initial control of VV infection (7, 8), efficient activation of CD8+ T cell response is required for the eradication of VV infection (9). What promotes the activation of CD8+ T cell response to VV infection remains incompletely defined. Understanding how γδT cells activate CD8+ T cells will better elucidate the mechanisms that govern CD8+ T cell activation and how to better employ them for future strategies in vaccination or immunotherapy.
γδT cells play an active role in the control of parasitic, bacterial, and viral infections, such as malaria, Listeria monocytogenes, Salmonella, EBV, and HSV (22, 24–27, 46–48). Unlike other innate immune cells, γδT cells require activation by various antigens prior to exhibiting cytotoxic characteristics (49). Currently, most strategies that target γδT cells employ phosphoantigens to activate γδT cells. However, recent evidence suggests that phosphoantigen activation is nonspecific, and induce both inflammatory and anti-inflammatory functions in the targeted cells (50–53). The goal is therefore to investigate a method that would preferentially activate one subpopulation of γδT cells. Previous studies have found that different infections result in 2 main differential responses in IFN-γ and IL-17 expression (54, 55). However, categorization of γδT cell subpopulations remains controversial (13, 16). Given the long-lasting immunity that VV produce in clinical evaluations, understanding how VV activates γδT cells to produce IFN-γ could provide insights into strategies to shifting γδT cells towards cytotoxic immunity overall.
In this study, we demonstrate that γδT cells is required for the full activation of CD8+ T cells after VV infection. δTCR-/- mice have deficient VV-specific CD8+ T cell proliferation and functional response. We find that deficiency of γδT cells results in over 3-fold decrease in CD8+ T cell response. Given previous studies that demonstrate γδT cells’ influence on the immune system, it is possible that γδT cells may be directly responsible for CD8+ T cell activation following VV infection (19, 20, 56). To investigate this possibility, we examined whether VV upregulates the 3 conventional signals of CD8+ T cell activation in γδT cells. We found that following infection with VV-OVA, SIINFEKL peptide is present on MHC-I on the surface of γδT cells. We also found that there is a significant decrease in CD8+ T cell activation in δTCR-/- mice adoptively transferred with deficient MHC-I γδT cells, compared to that transferred with wild-type γδT cells. Similarly, there is a significant increase in expression of CD86, IFN-α, and IL-1 in γδT cells following VV infection for signals 2 and 3 that are required for CD8+ T cell activation. This suggests that γδT cells can provide all 3 signals necessary for CD8+ T cell activation, and that γδT cells directly influence CD8+ T cell activation following VV infection.
To determine how VV activates γδT cells for antigen presentation, we assessed γδT cell activity in mice with deficiencies in IFN-αβ, IFN-γ, TNF-α, and MyD88 signaling. We found that only mice with global deficient MyD88 signaling presented with impaired γδT cell response to VV infection. To determine if MyD88 expression in γδT cells (cell-intrinsic) or in other cells (cell-extrinsic) is responsible for γδT cell activation, we investigated whether the γδT cell deficiency in MyD88-/- mice are due to signaling from other cells that require MyD88 signaling or MyD88 signaling in γδT cells alone. We found that MyD88-/- mice that were adoptively transferred with WT γδT cells exhibited normalized γδT cells response to VV. This indicates that VV could activate γδT cells via MyD88-associated PRRs. Additionally, when MyD88-/- γδT cells are adoptively transferred to δTCR-/- mice, there is a deficient CD8+ T cell response. This means that MyD88 activation on γδT cells is required for CD8+ T cell response.
In conclusion, our study reveals that VV activates γδT cells via MyD88 signaling pathway, which leads to direct antigen presentation to CD8+ T cells. In vivo, this bridge between the innate and adaptive immune pathways plays a critical role in the activation of CD8+ T cell response to VV. Furthermore, we demonstrate that cell-intrinsic MyD88 signaling in γδT cells is required for activation of CD8+ T cells. These results demonstrate a critical role for γδT cells in the regulation of adaptive T cell response to viral infection and may shed light on the design of more effective vaccine strategies based on manipulation of γδT cells.
Data Availability Statement
The raw data supporting the conclusions of this article will be made available by the authors, without undue reservation.
Ethics Statement
The animal study was reviewed and approved by The Animal Care and Use Committees at Duke University and The Ohio State University.
Author Contributions
YY conceived the study and supervised the work. RD performed the experiments. YY, RD, and XH designed experiments, analyzed and interpreted data. RD and YY wrote the manuscript, and all authors reviewed and revised the manuscript, and approved the submitted version.
Funding
This work was supported by National Institutes of Health grants CA136934, CA186973 and CA193167 (to YY).
Conflict of Interest
The authors declare that the research was conducted in the absence of any commercial or financial relationships that could be construed as a potential conflict of interest.
Publisher’s Note
All claims expressed in this article are solely those of the authors and do not necessarily represent those of their affiliated organizations, or those of the publisher, the editors and the reviewers. Any product that may be evaluated in this article, or claim that may be made by its manufacturer, is not guaranteed or endorsed by the publisher.
Supplementary Material
The Supplementary Material for this article can be found online at: https://www.frontiersin.org/articles/10.3389/fimmu.2021.727046/full#supplementary-material
References
1. Fenner F, Henderson DA, Arita I, Jezek Z, Ladnyi ID. Small-Pox and Its Eradication. Geneva, Switzerland: World Health Organization (1988) p. 1371–409.
2. Falkner FG, Holzer GW. Vaccinia Viral/Retroviral Chimeric Vectors. Curr Gene Ther (2004) 4(4):417–26. doi: 10.2174/1566523043346101
3. Hammarlund E, Lewis MW, Hansen SG, Strelow LI, Nelson JA, Sexton GJ, et al. Duration of Antiviral Immunity After Smallpox Vaccination. Nat Med (2003) 9(9):1131–7. doi: 10.1038/nm917
4. McClain DJ, Harrison S, Yeager CL, Cruz J, Ennis FA, Gibbs P, et al. Immunologic Responses to Vaccinia Vaccines Administered by Different Parenteral Routes. J Infect Dis (1997) 175):756–63. doi: 10.1086/513968
5. Hanke T, Blanchard TJ, Schneider J, Ogg GS, Tan R, Becker M, et al. Immunogenicities of Intravenous and Intramuscular Administrations of Modified Vaccinia Virus Ankara-Based Multi-CTL Epitope Vaccine for Human Immunodeficiency Virus Type 1 in Mice. J Gen Virol (1998) 79(Pt 1):83–90. doi: 10.1099/0022-1317-79-1-83
6. Gnant MF, Puhlmann M, Bartlett DL, Alexander HR Jr. Regional Versus Systemic Delivery of Recombinant Vaccinia Virus as Suicide Gene Therapy for Murine Liver Metastases. Ann Surg (1999) 230(3):352–360. doi: 10.1097/00000658-199909000-00008
7. Zhu J, Martinez J, Huang X, Yang Y. Innate Immunity Against Vaccinia Virus is Mediated by TLR2 and Requires TLR-Independent Production of IFN-Beta. Blood (2007) 109(2):619–25. doi: 10.1182/blood-2006-06-027136
8. Martinez J, Huang X, Yang Y. Direct Action of Type I IFN on NK Cells is Required for Their Activation in Response to Vaccinia Viral Infection In Vivo. J Immunol (2008) 180(3):1592–7. doi: 10.4049/jimmunol.180.3.1592
9. Quigley M, Martinez J, Huang X, Yang Y. A Critical Role for Direct TLR2-MyD88 Signaling in CD8 T-Cell Clonal Expansion and Memory Formation Following Vaccinia Viral Infection. Blood (2009) 113(10):2256–64. doi: 10.1182/blood-2008-03-148809
10. Quigley M, Huang X, Yang Y. STAT1 Signaling in CD8 T Cells Is Required for Their Clonal Expansion and Memory Formation Following Viral Infection In Vivo. J Immunol (2008) 180(4):2158–64. doi: 10.4049/jimmunol.180.4.2158
11. Hayday AC. Gammadelta T Cells and the Lymphoid Stress-Surveillance Response. Immunity (2009) 31(2):184–96. doi: 10.1016/j.immuni.2009.08.006
12. Dodd J, Riffault S, Kodituwakku JS, Hayday AC, Openshaw PJ. Pulmonary V Gamma 4+ Gamma Delta T Cells Have Proinflammatory and Antiviral Effects in Viral Lung Disease. J Immunol (2009) 182(2):1174–81. doi: 10.4049/jimmunol.182.2.1174
13. Munoz-Ruiz M, Ribot JC, Grosso AR, Goncalves-Sousa N, Pamplona A, Pennington DJ, et al. TCR Signal Strength Controls Thymic Differentiation of Discrete Proinflammatory Gammadelta T Cell Subsets. Nat Immunol (2016) 17(6):721–7. doi: 10.1038/ni.3424
14. Lahn M, Kanehiro A, Takeda K, Terry J, Hahn YS, Aydintug MK, et al. MHC Class I-Dependent V Gamma 4(+) Pulmonary T Cells Regulate Alpha Beta T Cell-Independent Airway Responsiveness. Proc Natl Acad Sci USA (2002) 99(13):8850–5. doi: 10.1073/pnas.132519299
15. Casetti R, De Simone G, Sacchi A, Rinaldi A, Viola D, Agrati C, et al. Vgamma9Vdelta2 T-Cell Polyfunctionality Is Differently Modulated in HAART-Treated HIV Patients According to CD4 T-Cell Count. PloS One (2015) 10(7):e0132291. doi: 10.1371/journal.pone.0132291
16. O’Brien RL, Lahn M, Born WK, Huber SA. T Cell Receptor and Function Cosegregate in Gamma-Delta T Cell Subsets. Chem Immunol (2001) 79:1–28. doi: 10.1159/000058829
17. O’Brien RL, Roark CL, Jin N, Aydintug MK, French JD, Chain JL, et al. Gammadelta T-Cell Receptors: Functional Correlations. Immunol Rev (2007) 215:77–88. doi: 10.1111/j.1600-065X.2006.00477.x
18. Vantourout P, Hayday A. Six-Of-the-Best: Unique Contributions of Gammadelta T Cells to Immunology. Nat Rev Immunol (2013) 13(2):88–100. doi: 10.1038/nri3384
19. Brandes M, Willimann K, Moser B. Professional Antigen-Presentation Function by Human Gammadelta T Cells. Science (2005) 309(5732):264–8. doi: 10.1126/science.1110267
20. Brandes M, Willimann K, Bioley G, Levy N, Eberl M, Luo M, et al. Cross-Presenting Human Gammadelta T Cells Induce Robust CD8+ Alphabeta T Cell Responses. Proc Natl Acad Sci USA (2009) 106(7):2307–12. doi: 10.1073/pnas.0810059106
21. Cheng L, Cui Y, Shao H, Han G, Zhu L, Huang Y, et al. Mouse Gammadelta T Cells are Capable of Expressing MHC Class II Molecules, and of Functioning as Antigen-Presenting Cells. J Neuroimmunol (2008) 203(1):3–11. doi: 10.1016/j.jneuroim.2008.06.007
22. Webster DP, Dunachie S, Vuola JM, Berthoud T, Keating S, Laidlaw SM, et al. Enhanced T Cell-Mediated Protection Against Malaria in Human Challenges by Using the Recombinant Poxviruses FP9 and Modified Vaccinia Virus Ankara. Proc Natl Acad Sci USA (2005) 102(13):4836–41. doi: 10.1073/pnas.0406381102
23. Liu C, Emami SN, Pettersson J, Ranford-Cartwright L, Faye I, Parmryd I. Vgamma9Vdelta2 T Cells Proliferate in Response to Phosphoantigens Released From Erythrocytes Infected With Asexual and Gametocyte Stage Plasmodium Falciparum. Cell Immunol (2018) 334:11–9. doi: 10.1016/j.cellimm.2018.08.012
24. Sciammas R, Bluestone JA. HSV-1 Glycoprotein I-Reactive TCR Gamma Delta Cells Directly Recognize the Peptide Backbone in a Conformationally Dependent Manner. J Immunol (1998) 161(10):5187–92.
25. Puttur FK, Fernandez MA, White R, Roediger B, Cunningham AL, Weninger W, et al. Herpes Simplex Virus Infects Skin Gamma Delta T Cells Before Langerhans Cells and Impedes Migration of Infected Langerhans Cells by Inducing Apoptosis and Blocking E-Cadherin Downregulation. J Immunol (2010) 185(1):477–87. doi: 10.4049/jimmunol.0904106
26. Hiromatsu K, Yoshikai Y, Matsuzaki G, Ohga S, Muramori K, Matsumoto K, et al. A Protective Role of Gamma/Delta T Cells in Primary Infection With Listeria Monocytogenes in Mice. J Exp Med (1992) 175(1):49–56. doi: 10.1084/jem.175.1.49
27. Zhu Y, Wang H, Xu Y, Hu Y, Chen H, Cui L, et al. Human Gammadelta T Cells Augment Antigen Presentation in Listeria Monocytogenes Infection. Mol Med (2016) 22:737–46. doi: 10.2119/molmed.2015.00214
28. Kubota K, Kadoya Y. Innate IFN-Gamma-Producing Cells in the Spleen of Mice Early After Listeria Monocytogenes Infection: Importance of Microenvironment of the Cells Involved in the Production of Innate IFN-Gamma. Front Immunol (2011) 2:26. doi: 10.3389/fimmu.2011.00026
29. Chen ZW. Multifunctional Immune Responses of HMBPP-Specific Vgamma2Vdelta2 T Cells in M. Tuberculosis and Other Infections. Cell Mol Immunol (2013) 10(1):58–64. doi: 10.1038/cmi.2012.46
30. Qaqish A, Huang D, Chen CY, Zhang Z, Wang R, Li S, et al. Adoptive Transfer of Phosphoantigen-Specific Gammadelta T Cell Subset Attenuates Mycobacterium Tuberculosis Infection in Nonhuman Primates. J Immunol (2017) 198(12):4753–63. doi: 10.4049/jimmunol.1602019
31. Amezcua Vesely MC, Pallis P, Bielecki P, Low JS, Zhao J, Harman CCD, et al. Effector TH17 Cells Give Rise to Long-Lived TRM Cells That Are Essential for an Immediate Response Against Bacterial Infection. Cell (2019) 178(5):1176–1188.e1115. doi: 10.1016/j.cell.2019.07.032
32. Hermann E, Sucke B, Droste U, Meyer zum Buschenfelde KH. Klebsiella Pneumoniae-Reactive T Cells in Blood and Synovial Fluid of Patients With Ankylosing Spondylitis. Comparison With HLA-B27+ Healthy Control Subjects in a Limiting Dilution Study and Determination of the Specificity of Synovial Fluid T Cell Clones. Arthritis Rheum (1995) 38(9):1277–82. doi: 10.1002/art.1780380916
33. Wang T, Gao Y, Scully E, Davis CT, Anderson JF, Welte T, et al. Gamma Delta T Cells Facilitate Adaptive Immunity Against West Nile Virus Infection in Mice. J Immunol (2006) 177(3):1825–32. doi: 10.4049/jimmunol.177.3.1825
34. Moretto M, Durell B, Schwartzman JD, Khan IA. Gamma Delta T Cell-Deficient Mice Have a Down-Regulated CD8+ T Cell Immune Response Against Encephalitozoon Cuniculi Infection. J Immunol (2001) 166(12):7389–97. doi: 10.4049/jimmunol.166.12.7389
35. Nomura A, Matsuzaki G, Takada H, Hiromatsu K, Nabeshima S, Nakamura T, et al. The Role of Gammadelta T Cells in Induction of Bacterial Antigen-Specific Protective CD8+ Cytotoxic T Cells in Immune Response Against the Intracellular Bacteria Listeria Monocytogenes. Immunology (1998) 95(2):226–33. doi: 10.1046/j.1365-2567.1998.00593.x
36. Yang Y, Huang CT, Huang X, Pardoll DM. Persistent Toll-Like Receptor Signals are Required for Reversal of Regulatory T Cell-Mediated CD8 Tolerance. Nat Immunol (2004) 5(5):508–15. doi: 10.1038/ni1059
37. Tscharke DC, Karupiah G, Zhou J, Palmore T, Irvine KR, Haeryfar SM, et al. Identification of Poxvirus CD8+ T Cell Determinants to Enable Rational Design and Characterization of Smallpox Vaccines. J Exp Med (2005) 201(1):95–104. doi: 10.1084/jem.20041912
38. Xu R, Johnson AJ, Liggitt D, Bevan MJ. Cellular and Humoral Immunity Against Vaccinia Virus Infection of Mice. J Immunol (2004) 172(10):6265–71. doi: 10.4049/jimmunol.172.10.6265
39. Yang Y, Nunes FA, Berencsi K, Furth EE, Gonczol E, Wilson JM. Cellular Immunity to Viral Antigens Limits E1-Deleted Adenoviruses for Gene Therapy. Proc Natl Acad Sci USA (1994) 91(10):4407–11. doi: 10.1073/pnas.91.10.4407
40. Curtsinger JM, Johnson CM, Mescher MF. CD8 T Cell Clonal Expansion and Development of Effector Function Require Prolonged Exposure to Antigen, Costimulation, and Signal 3 Cytokine. J Immunol (2003) 171(10):5165–71. doi: 10.4049/jimmunol.171.10.5165
41. Curtsinger JM, Lins DC, Mescher MF. Signal 3 Determines Tolerance Versus Full Activation of Naive CD8 T Cells: Dissociating Proliferation and Development of Effector Function. J Exp Med (2003) 197(9):1141–51. doi: 10.1084/jem.20021910
42. Van den Hove LE, Van Gool SW, Vandenberghe P, Bakkus M, Thielemans K, Boogaerts MA, et al. CD40 Triggering of Chronic Lymphocytic Leukemia B Cells Results in Efficient Alloantigen Presentation and Cytotoxic T Lymphocyte Induction by Up-Regulation of CD80 and CD86 Costimulatory Molecules. Leukemia (1997) 11(4):572–80. doi: 10.1038/sj.leu.2400598
43. Sansom DM, Manzotti CN, Zheng Y. What’s the Difference Between CD80 and CD86? Trends Immunol (2003) 24(6):314–9. doi: 10.1016/S1471-4906(03)00111-X
44. Curtsinger JM, Valenzuela JO, Agarwal P, Lins D, Mescher MF. Type I IFNs Provide a Third Signal to CD8 T Cells to Stimulate Clonal Expansion and Differentiation. J Immunol (2005) 174(8):4465–9. doi: 10.4049/jimmunol.174.8.4465
45. Ben-Sasson SZ, Hogg A, Hu-Li J, Wingfield P, Chen X, Crank M, et al. Et Al: IL-1 Enhances Expansion, Effector Function, Tissue Localization, and Memory Response of Antigen-Specific CD8 T Cells. J Exp Med (2013) 210(3):491–502. doi: 10.1084/jem.20122006
46. Sciammas R, Bluestone JA. TCRgammadelta Cells and Viruses. Microbes Infect (1999) 1(3):203–12. doi: 10.1016/S1286-4579(99)80035-5
47. Hviid L, Smith-Togobo C, Willcox BE. Human Vdelta1(+) T Cells in the Immune Response to Plasmodium Falciparum Infection. Front Immunol (2019) 10:259. doi: 10.3389/fimmu.2019.00259
48. Howard J, Loizon S, Tyler CJ, Duluc D, Moser B, Mechain M, et al. The Antigen-Presenting Potential of Vgamma9Vdelta2 T Cells During Plasmodium Falciparum Blood-Stage Infection. J Infect Dis (2017) 215(10):1569–79. doi: 10.1093/infdis/jix149
49. Salerno A, Dieli F. Role of Gamma Delta T Lymphocytes in Immune Response in Humans and Mice. Crit Rev Immunol (1998) 18(4):327–57. doi: 10.1615/CritRevImmunol.v18.i4.30
50. Todaro M, D’Asaro M, Caccamo N, Iovino F, Francipane MG, Meraviglia S, et al. Efficient Killing of Human Colon Cancer Stem Cells by Gammadelta T Lymphocytes. J Immunol (2009) 182(11):7287–96. doi: 10.4049/jimmunol.0804288
51. Fichtner AS, Bubke A, Rampoldi F, Wilharm A, Tan L, Steinbruck L, et al. TCR Repertoire Analysis Reveals Phosphoantigen-Induced Polyclonal Proliferation of Vgamma9Vdelta2 T Cells in Neonates and Adults. J Leukoc Biol (2020) 107(6):1023–32. doi: 10.1002/JLB.1MA0120-427RR
52. Rei M, Goncalves-Sousa N, Lanca T, Thompson RG, Mensurado S, Balkwill FR, et al. Murine CD27(-) Vgamma6(+) Gammadelta T Cells Producing IL-17A Promote Ovarian Cancer Growth via Mobilization of Protumor Small Peritoneal Macrophages. Proc Natl Acad Sci USA (2014) 111(34):E3562–3570. doi: 10.1073/pnas.1403424111
53. Chen X, Shang W, Xu R, Wu M, Zhang X, Huang P, et al. Distribution and Functions of Gammadelta T Cells Infiltrated in the Ovarian Cancer Microenvironment. J Transl Med (2019) 17(1):144. doi: 10.1186/s12967-019-1897-0
54. Ribot JC, Chaves-Ferreira M, d’Orey F, Wencker M, Goncalves-Sousa N, Decalf J, et al. Cutting Edge: Adaptive Versus Innate Receptor Signals Selectively Control the Pool Sizes of Murine IFN-Gamma- or IL-17-Producing Gammadelta T Cells Upon Infection. J Immunol (2010) 185(11):6421–5. doi: 10.4049/jimmunol.1002283
55. Xu S, Han Y, Xu X, Bao Y, Zhang M, Cao X. IL-17A-Producing gammadeltaT Cells Promote CTL Responses Against Listeria Monocytogenes Infection by Enhancing Dendritic Cell Cross-Presentation. J Immunol (2010) 185(10):5879–87. doi: 10.4049/jimmunol.1001763
Keywords: γδT cells, vaccinia virus, CD8 T cells, innate immunity, adaptive immunity, MyD88 pathway
Citation: Dai R, Huang X and Yang Y (2021) γδT Cells Are Required for CD8+ T Cell Response to Vaccinia Viral Infection. Front. Immunol. 12:727046. doi: 10.3389/fimmu.2021.727046
Received: 18 June 2021; Accepted: 22 September 2021;
Published: 08 October 2021.
Edited by:
Kenth Gustafsson, University College London, United KingdomReviewed by:
Brian S Sheridan, Stony Brook University, United StatesRoberto Tinoco, University of California, Irvine, United States
Copyright © 2021 Dai, Huang and Yang. This is an open-access article distributed under the terms of the Creative Commons Attribution License (CC BY). The use, distribution or reproduction in other forums is permitted, provided the original author(s) and the copyright owner(s) are credited and that the original publication in this journal is cited, in accordance with accepted academic practice. No use, distribution or reproduction is permitted which does not comply with these terms.
*Correspondence: Yiping Yang, eWlwaW5nLnlhbmcyQG9zdW1jLmVkdQ==