- 1Faculty of Medicine and Health Sciences, Collaborative Antwerp Psychiatric Research Institute (CAPRI), University of Antwerp, Antwerp, Belgium
- 2Scientific Initiative of Neuropsychiatric and Psychopharmacological Studies (SINAPS), University Psychiatric Centre Duffel, Duffel, Belgium
- 3Laboratory of Medical Biochemistry, University of Antwerp, Antwerp, Belgium
- 4INSERM U955, Equipe Psychiatrie Translationnelle, Créteil, France
- 5Fondation FondaMental - Hôpital Albert Chenevier - Pôle Psychiatrie, Créteil, France
- 6AP-HP, Hôpitaux Universitaires Henri Mondor, DHU Pepsy, Pôle de Psychiatrie et d’Addictologie, Créteil, France
- 7Université Paris Est Créteil, Faculté de Médecine, Creteil, France
- 8Department of Psychiatry and Psychotherapy, Ludwig-Maximilians-University, München, Germany
Objective: Disturbances in the kynurenine pathway have been implicated in the pathophysiology of psychotic and mood disorders, as well as several other psychiatric illnesses. It remains uncertain however to what extent metabolite levels detectable in plasma or serum reflect brain kynurenine metabolism and other disease-specific pathophysiological changes. The primary objective of this systematic review was to investigate the concordance between peripheral and central (CSF or brain tissue) kynurenine metabolites. As secondary aims we describe their correlation with illness course, treatment response, and neuroanatomical abnormalities in psychiatric diseases.
Methods: We performed a systematic literature search until February 2021 in PubMed. We included 27 original research articles describing a correlation between peripheral and central kynurenine metabolite measures in preclinical studies and human samples from patients suffering from neuropsychiatric disorders and other conditions. We also included 32 articles reporting associations between peripheral KP markers and symptom severity, CNS pathology or treatment response in schizophrenia, bipolar disorder or major depressive disorder.
Results: For kynurenine and 3-hydroxykynurenine, moderate to strong concordance was found between peripheral and central concentrations not only in psychiatric disorders, but also in other (patho)physiological conditions. Despite discordant findings for other metabolites (mainly tryptophan and kynurenic acid), blood metabolite levels were associated with clinical symptoms and treatment response in psychiatric patients, as well as with observed neuroanatomical abnormalities and glial activity.
Conclusion: Only kynurenine and 3-hydroxykynurenine demonstrated a consistent and reliable concordance between peripheral and central measures. Evidence from psychiatric studies on kynurenine pathway concordance is scarce, and more research is needed to determine the validity of peripheral kynurenine metabolite assessment as proxy markers for CNS processes. Peripheral kynurenine and 3-hydroxykynurenine may nonetheless represent valuable predictive and prognostic biomarker candidates for psychiatric disorders.
1 Introduction
Immune dysregulation plays an important role in the pathophysiology of several psychiatric disorders. Mood and psychotic disorders exhibit peripheral and central immune abnormalities, such as increased peripheral pro-inflammatory cytokine levels (1–3) and up- or downregulated central nervous system (CNS) glial responses (4–7). Immune mechanisms are further known to modulate psychiatric symptom development and illness course. Specifically, inflammation-induced depressive symptomatology has been observed in healthy volunteers and patients recently remitted from major depression (8, 9), while add-on anti-inflammatory drugs improve residual symptoms in patients with major depressive disorder (MDD) and psychotic disorders. This effect is particularly observed if patients present with a basally increased peripheral pro-inflammatory cytokine profile (10, 11).
For over half a century, disruption of the kynurenine pathway (KP) has been proposed as a mechanistic link between immune disturbances and psychiatric pathology and symptomatology (12, 13). Since the early 1990s, increased efforts and better analytical methods have further disclosed the role of tryptophan (TRP), kynurenine (KYN) and their downstream metabolites in psychotic and mood disorders. Two meta-analyses (14–16) have demonstrated that a.o. peripheral tryptophan, kynurenine and kynurenic acid levels are at least partially downregulated in mood and psychotic disorders, whereas the limited number of studies focusing on cerebrospinal fluid (CSF) and brain tissue demonstrate unaltered or even increased KP metabolite concentrations (especially kynurenic acid) in these disorders (17–20). Most clinical studies to date quantified kynurenine metabolite concentrations in peripheral blood. Nonetheless, fundamental knowledge about the interrelation between kynurenine metabolites in CNS and peripheral blood and, importantly, their bidirectional transport across the blood-brain barrier remains incomplete. Peripheral KP metabolite quantifications may not represent concentrations in CNS tissue, as evidenced by divergent research results. Consequently, the validity of peripheral kynurenine metabolite assessment as biomarkers for human neuropsychiatric illnesses has been questioned (14, 21, 22).
1.1 Overview of the Kynurenine Pathway
Tryptophan (TRP) is an essential amino acid mainly known as the precursor of serotonin (5-HT) and melatonin. The first, rate-limiting, step of the pathway is the conversion of TRP to KYN by the enzymes indoleamine 2,3-dioxygenase (IDO) and tryptophan 2,3-dioxygenase (TDO) (Figure 1). TDO, which is mainly found in the liver and also in the brain (23–26), metabolizes 95% of whole-body TRP into KYN, of which the liver contributes 90%. Under normal physiological conditions, TDO in liver tissue will consume most of diet-derived TRP, and as such is the main source of KYN throughout the body (27). TDO is considered a housekeeping enzyme: excess TRP is diverted to the Krebs cycle to generate energy (26). The enzyme is induced by glucocorticoids to fulfill energy needs under stressful conditions and is thus activated by psychophysiological stress by cortisol release (28). Moreover, TDO is inhibited by a reduction in nicotinamide, activated by heme and stabilized by TRP (26).
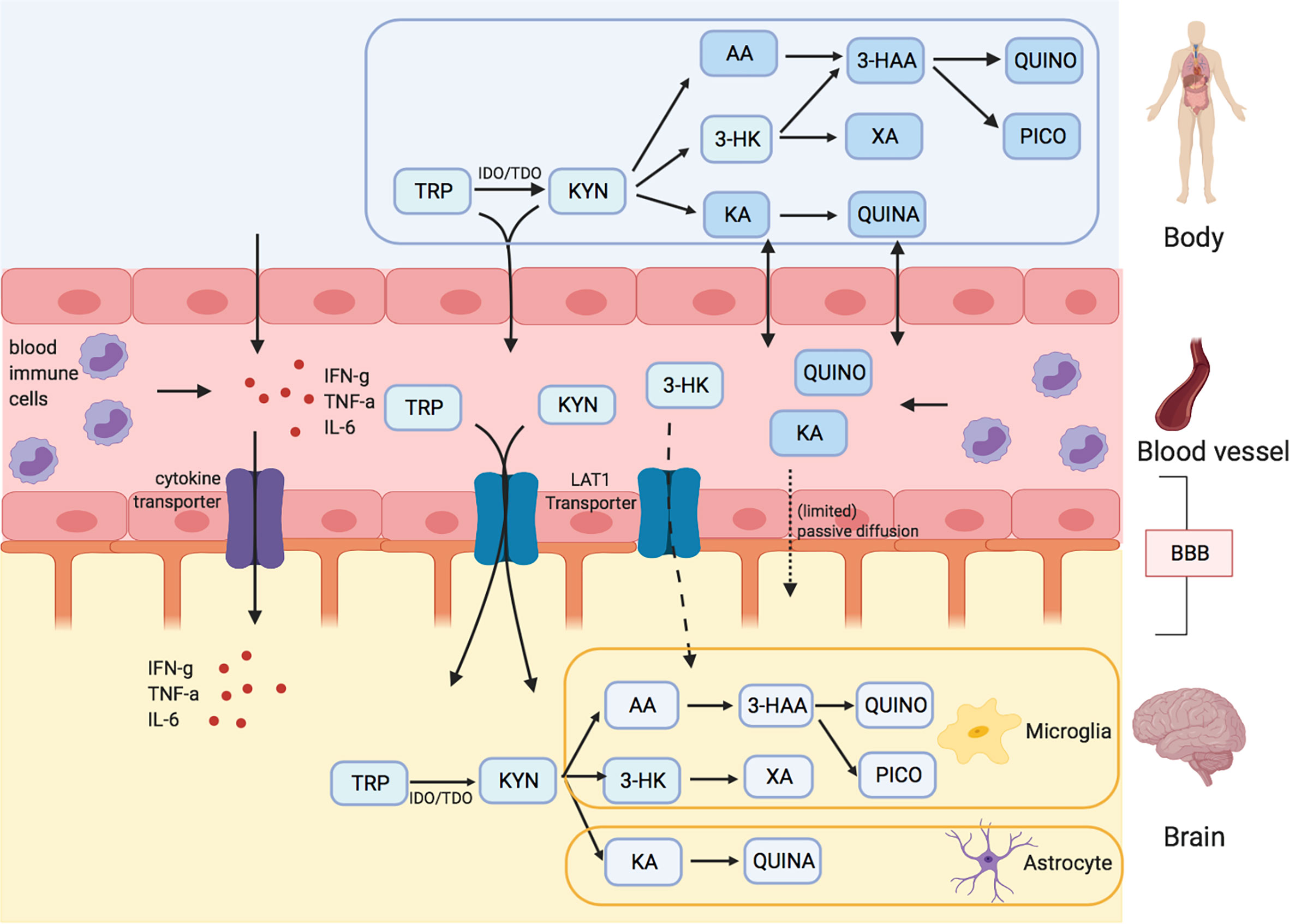
Figure 1 Kynurenine metabolites and the blood brain barrier (BBB). Tryptophan (TRP) and kynurenine (KYN), and to a lesser degree 3-hydrox kynurenine (3-HK) are actively transported into the brain over LAT1 transporters. Downstream metabolites of the kynurenine pathway (KP), like quinolinic acid (QUINO) and kynurenic acid (KA), cannot make use of these transporters, but (probably limited) passive diffusion of these metabolites over the BBB is possible. Anthranilic acid and 3-hydroxy anthranilic acid (not shown in figure) may equally pass the blood brain barrier through passive diffusion, much like QUINO. In the brain, microglia are responsible for the production of metabolites 3-HK and QUINO, whereas astrocytes produce KA. Peripheral production of these KP metabolites is done by blood immune cells, such as blood monocytes (PBMC) and other organs, including liver and kidney. The gut microbiome, which plays a role in psychiatric illness through the gut-brain axis, also affects KP metabolization.
Activity of IDO is low in non-pathological conditions but, unlike TDO, can be downregulated by anti-inflammatory cytokines (29) and upregulated by pro-inflammatory cytokines [mainly interferon gamma (IFN-g), but also tumor necrosis factor alpha (TNF-a)] (30) and psychological stress (31).
In the brain, downstream metabolization of KYN occurs through divergent routes in microglia and astrocytes. Kynurenine 3-monooxygenase (KMO), only active in cerebral microglia, metabolizes KYN into 3-hydroxykynurenine (3-HK), an N-methyl D-aspartate (NDMA) receptor agonist (32). Metabolites further downstream from 3-HK include quinolinic acid (QUINO), another NMDA-receptor agonist, and picolinic acid (PICO), which in contrast antagonizes the NMDA-receptor. It is assumed that additional microglial QUINO can be produced in parallel via catabolization of KYN to anthranilic acid (AA), although this was not supported by Giorgini et al. as QUINO levels were almost non-existent in KMO deficient mice (AA) (33). Quinolinic acid phosphoribosyltransferase (QPRT) further degrades QUINO to niacin, a form of vitamin B3.
In astrocytes, KYN is metabolized by kynurenine aminotransferases (KAT) to kynurenic acid (KA), another NMDA-receptor antagonist. However, a portion of this astrocyte produced KYN will fuel macrophages and microglia to produce QUINO (34). KA is considered a neuroprotective metabolite due to its antagonism effect on the excitatory NMDA receptor. However, abnormally elevated KA has been put forward as the mechanism causing glutamate hypofunction by sustained NMDA receptor antagonism, which can lead to psychotic symptoms and cognitive and social impairments in MDD and SCZ (35–37).
In the periphery, catabolization through KMO and KAT both occurs albeit at different rates depending on multiple factors such as the relative abundance of the enzymes in specific tissues, substrate concentration and affinity, pH, bioavailability of cofactors, cosubstrates and competing substrates (26). As the majority of studies investigating these enzymes are performed in vivo, these factors are often not taken into account resulting in a simplification of the actual enzyme physiology (38). KMO is mostly present in liver, kidney, macrophages and monocytes (39), while KAT is active in liver, kidney, placenta, heart and macrophages (40). Pro-inflammatory cytokines like IFN-g also have a strong stimulating effect on KMO, in the brain as well as in the periphery.
1.2 Study Objectives
As the primary objective of this systematic review, we will investigate the correlation coefficients between peripheral and central kynurenine metabolite concentrations in preclinical research and in human samples of varying origins. As secondary objectives, associations between peripheral KP measures and (endo)phenotypic measures (symptom severity, treatment response and CNS abnormalities) in psychiatric illness will be described to appraise the value of KP metabolites as prognostic and predictive biomarkers. In order to provide a better understanding of the factors influencing central and peripheral KP metabolites, we will discuss the (patho)physiological impact on KP bioavailability and blood-brain-barrier (BBB) transport in healthy and immune-activated physiological states.
2 Methodology
We performed a pubmed-based literature systematic search (January 1968 - February 2021) using the following search string: ((tryptophan OR kynuren* OR “quinolinic” OR “xanthurenic acid” OR “anthranilic acid”) AND (“serum” OR “plasma” OR “blood”) AND (“brain tissue” OR “BBB” OR “blood brain barrier” OR “blood-brain-barrier” OR “CSF” OR “cerebrospinal fluid” OR “postmortem” OR “post-mortem” OR “MRI” OR “fMRI” OR “PET” OR “DTI”) NOT (review [Publication Type])). Eligible papers were extracted from the PubMed database using the following inclusion criteria: 1) English language articles published in peer-reviewed journals, 2) Human studies including patients with a major psychiatric disorder reporting correlation coefficients between peripheral and central KP metabolites or association measures between at least one peripheral KP metabolite and symptom severity or brain imaging disturbances or treatment outcome, or 3) Human studies including healthy controls or non-psychiatric patients reporting correlation coefficients between peripheral and central KP metabolites, or 4) In-vivo or postmortem assessment of at least one KP metabolite peripherally and centrally.
Two authors independently performed the literature search (M.M., K.S.). This search strategy yielded 1078 records that, with 19 additional records found through cross-referencing resulted in a total of 1097 records that were screened based on title and abstract. After exclusion of 868 irrelevant records, full articles were evaluated of 229 papers, ultimately leading to 59 papers that were included in the systematic review.
We included 27 original research articles describing a correlation between peripheral and central kynurenine metabolite measures in preclinical studies and human samples from healthy controls and patients suffering from neuropsychiatric disorders and other conditions. Correlation coefficients of peripheral-central KP metabolites along with the p-values, sample type, sample size and pathology type were extracted from the articles. The strength of concordance between peripheral and central KP measures was evaluated as a function of correlation measures, i.e. discordance = r <.20; weak concordance = .20 ≤ r ≤ -.39; moderate concordance = .40 ≤ r ≤ -.59 and strong concordance = r ≥.60 (41, 42).
We also included 32 articles reporting associations between peripheral KP markers and symptom severity, CNS pathology or treatment response in schizophrenia (SCZ), bipolar disorder (BD) or major depressive disorder (MDD).
See Figure 2 for PRISMA Flow Diagram [based on (43)].
3 Concordance of Peripheral and Central KP Metabolite Assessments
(Table 1) provides an overview of the included studies investigating correlations between peripheral and central kynurenine metabolite concentrations in both human and preclinical research. Since only a handful of studies (n=4) reported on these correlations in psychiatric populations, we additionally listed findings in healthy subjects and non-psychiatric diseases (n=14). It should be noted that the available psychiatric studies only concern depressed and bipolar patients, as until date no study has scrutinized this association in schizophrenic patients.
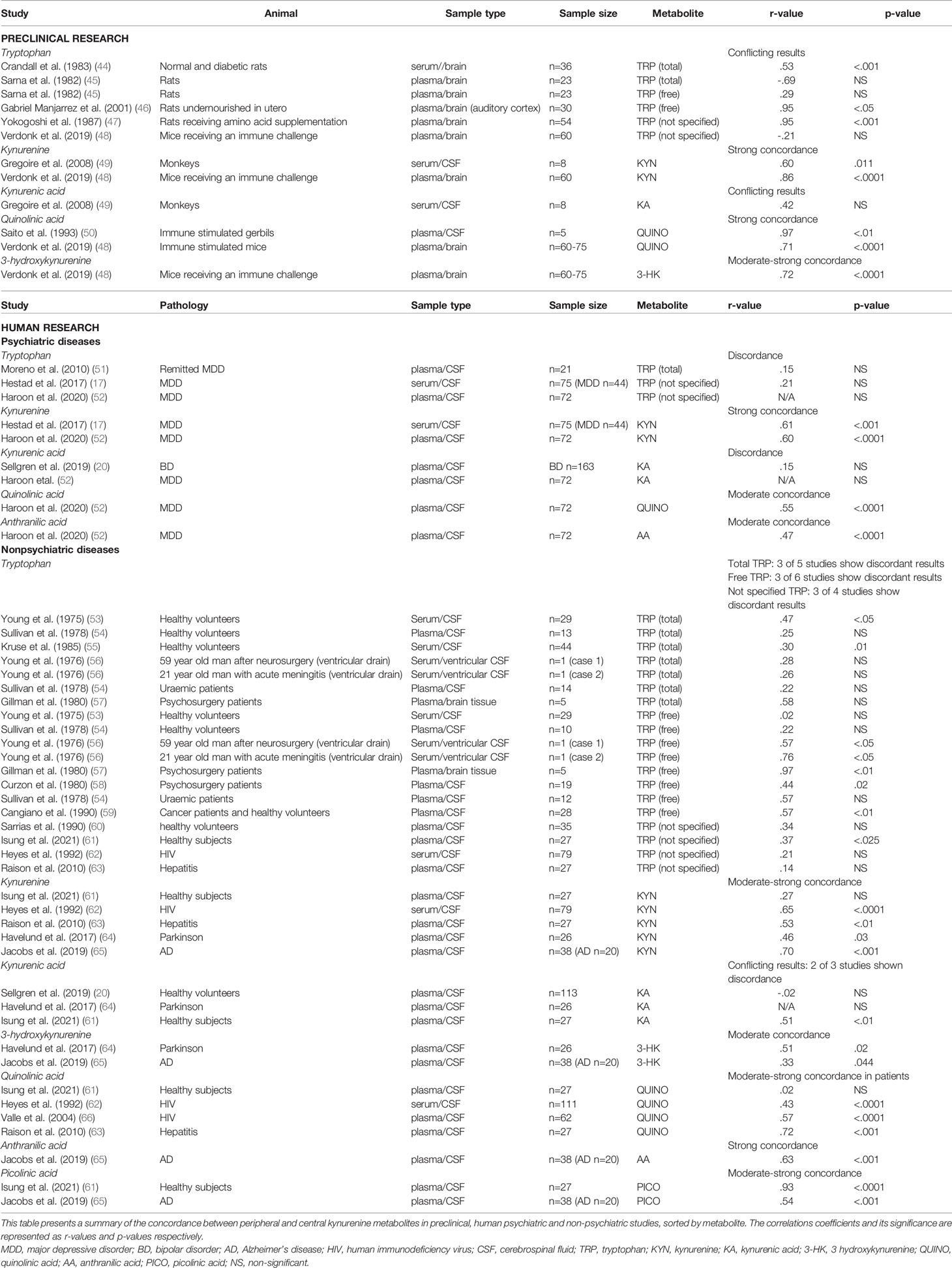
Table 1 Overview of studies investigating correlations between blood-based and CSF kynurenine pathway metabolites.
3.1 Preclinical Findings
Overall, animal studies showed divergent results on peripheral-central TRP correlations (see Table 1) (44–48). In immune challenged mice (i.e. lipopolysaccharide) (48) plasma and brain parenchyma KYN levels strongly correlated (r=.86; p<.001), in contrast to TRP levels (r=-.21). Similarly, 3-HK displayed very good inter-tissue correlations (r=.72; p>.001). Animal studies (rats, mice, rabbits) using (supraphysiological) stimulation of the immune system showed very high between-tissue correlations for QUINO and KA (r>.70) in plasma, CSF and brain tissue (48, 50). When directly comparing CSF and serum of monkeys receiving a kynurenine 3-hydroxylase inhibitor, both kynurenine and KA correlated between CSF and serum (r=0.60 and 0.43, respectively) (49).
In amino acid supplemented rats, a significant increase in brain TRP concentrations was observed, accompanied by strong plasma/brain correlations (47). Goeden (67) demonstrated that administration of kynurenine to pregnant female rats leads to very comparable increases of KYN (9-10 fold), KA (3-6 fold) and 3-HK (15-17 fold) in the maternal placenta, fetal plasma and brain. In contrast, administration of KA to the pregnant dams increased KA levels in placenta and plasma, but not the fetal plasma or brain. Interestingly, whereas a 7-day treatment with systemic KA in rats led to increased KA concentrations in both plasma and CSF but not in the other metabolites, acute KA administration did alter both TRP and several KP metabolite serum levels (68, 69).
In rats subjected to chronically unpredicted mild stress during 5 weeks, significant increases in KYN were observed in the colon, as well as in the cortex and hippocampus. Additionally, KA levels were increased in colon, whereas a decrease was seen in the cortex and hippocampus. Colonic KYN was significantly correlated with hippocampal KYN (r=.6154; p=.0066), KA (r=−.5787; p=.0119) and 3-HK (r=.5050; p=.0325) and negatively correlated to cortical KA (r=-.6717; p=.0023) (70).
Of note, kynurenine metabolite production seems to vary between species, e.g. between rats and gerbils (71), between mouse and human brain (29), so findings from animal models cannot be generalized to the human brain.
3.2 Psychiatric Research
Strong correlations were found between CSF and peripheral measures of KYN in MDD (n=75; r=.61; p<.001 (17) (n=72; r=.60; p=<.0001) (52). Peripheral TRP concentrations, however, did not correlate with central assessments (17, 51, 52), although it should be noted that these studies did not measure free TRP. Notably, the serotonin branch utilizes 10% of the blood-based TRP but on average half of the cerebral TRP, which may partially explain its low correlations with central concentrations. In contrast, peripheral assessments of KA (KAT-branch) did not correlate with CSF measures of the same metabolite in a large sample of bipolar patients (n=163) (20). Plasma concentrations of downstream metabolites of the KMO-branch QUINO and AA also showed a moderate concordance with CSF values in MDD (n=72; r=.55, resp. r=.47; all p-values <.0001) (52).
Although peripheral and central kynurenines have not been compared directly yet in schizophrenic patients, a recent meta-analysis showed that schizophrenia is associated with lower plasma KYN levels but higher CSF KYN and KA (72), which may suggest that peripheral and central KYN are not necessarily correlated in psychotic illness.
However, with only 4 psychiatric studies available, the currently available data do not allow calculation of quantitative meta-analytic summary statistics.
3.3 Non-Psychiatric Research
Similar to animal studies and in MDD, both total and free TRP correlation mostly show discordance in studies with healthy participants. Peripheral and central levels of kynurenine metabolites were compared in healthy participants (n=27) either after an intense physical activity or after a 4-week training program. At baseline, PICO levels were strongly correlated between plasma and CSF (r=.93; n=27; p<.0001), whereas a weak correlation was seen for the other kynurenine metabolites. After exercise, however, discordance ensued for KYN (r=-.22; NS), QUINO (r=-.09; NS) and KYNA (r=-.05; NS) (61).
In line with the results in MDD, peripheral KYN levels showed a moderate-to-strong concordances with CSF values in HIV patients (n=79; r=.65; p<.0001) (62), Alzheimer patients (n=20; r=.70; p<.001) (65), hepatitis C (n=27; r=.46; p<.01) (63) and Parkinson patients (n=26; r=.46; p=.03) (64), whereas both free and total peripheral TRP measures again showed conflicting results (free TRP: 3 of 6 studies show discordance; total TRP: 3 of 5 studies show discordance; not specified TRP: 3 of 4 studies show discordance) (53–60, 62, 63).
Peripheral 3-HK assessments also tend to reflect central concentrations of the same metabolite, albeit more modestly, as plasma and CSF 3-HK concentrations correlated weakly in Alzheimer’s (r=.33; p=.044) (65) and moderately in Parkinson’s disease (r=.51; p=.02) (64).
Further downstream the KMO-branch, QUINO and KA were most frequently studied. Blood and CSF assessments of microglia-based metabolites (AA, 3-HK, PICO, QUINO) equally tended to intercorrelate irrespective of the underlying illness (see Table 1), with moderate-to-strong concordances for QUINO (r-values ranging from.43 to.57; all p-values below.001) (62, 63, 66) and weak-to-moderate for 3-HK (r-values ranging between.33 and.51; all p-values <.05) (64, 65). Although based on a singly study, plasma AA and PICO showed a high (n=20; r=.63; p<.001) and moderate (n=20; r=.54; p<.001) concordance with CSF levels in Alzheimer patients (65). However, it should be noted that all investigated patient groups had hepatitis or neurological or HIV-related pathology, which may not be reflective of the concentrations and correlations found in psychiatric patients. Serum QUINO in HIV patients for example (73) ranged between 200-8000 nM/L, whereas that in schizophrenia and MDD more modestly ranged from 200 to 600 nM/L (74–76).
In line with findings in BD, KA measures in blood did not mirror central values in patients with Parkinson’s disease and healthy volunteers, although this discordance could be explained by the use of a peripheral aromatic amino acid decarboxylase inhibitor along with L-dopa which inhibit both KAT and KYNY enzymes in the periphery (20, 64).
In conclusion, human studies support several findings from preclinical samples, namely positive correlation coefficients between peripheral and central KYN and 3-HK levels, in contrast to TRP and KA. Free TRP has been preferred by several researchers over total TRP to correlate with CSF values (54, 56, 59, 77), although this could not be confirmed with the current results. Furthermore, hepatitis and HIV patients and immune stimulated rodents showed high inter-tissue correlation values of QUINO, possibly caused by inflammation in both compartments. However, further studies are necessary to confirm these interpretations.
4 Link Between Peripheral KP Markers and Endo-phenotypical Markers of Psychiatric Illness
As a secondary objective of this review, we aimed to develop a better understanding of the relationships between peripheral KP markers and several relevant clinical features such as symptom severity and treatment response.
4.1 Correlations Between Peripheral KP Markers and Clinical Symptomatology in Psychiatric Illness
An increased KYN/TRP ratio has been associated with higher depression severity scores in MDD patients (78, 79), with the presence of suicidality (80) and with manic symptomatology (81), irrespective of pharmacological treatments. Increased KYN/TRP has equally been linked to reduced cognitive performance in schizophrenia, MDD, panic disorder and aging (17, 76) (Table 2). In contrast, symptom severity in depression did not correlate with free or total plasma TRP levels (82).
Increased peripheral QUINO concentrations have been associated with depressive symptom severity in major depression and postpartum depression and in healthy volunteers receiving an inflammatory challenge (10, 83–85), as have increased AA and decreased KA. Decreased KA has also been associated with negative symptomatology in schizophrenia patients (86), although it is important to note that these low KA concentrations could be attributable to extremely low KYN levels (<200ng/ml instead of normal values around 2µg/ml) possibly caused by food intake which is known to lower KYN values (87). It should be noted that these associations were not consistently present (63, 88).
A shift towards the neurotoxic branch has equally been associated to affect cognition, as increased 3-HK activity was associated with poor memory performance in unipolar depressive and bipolar disorder (74, 89). This is in contrast with the negative correlation between KA and social cognition demonstrated by Huang (90). Although serum 3-HK and XA values were lowered in SCZ and BD compared to healthy controls (HCs), these metabolites did not correlate with symptom severity (91).
4.2 Correlations Between Peripheral KP Markers and CNS Physiology in Psychiatric Illness
A higher KYN/TRP ratio has been associated with loss of grey and white matter integrity in bipolar disorder (92) and schizophrenia (93) as well as with lower dorsolateral prefrontal cortex (DLPFC) volumes in schizophrenia (76), lower frontal white matter glutamate levels (93) and reduced striatal volumes in MDD (94).
Low plasma KA concentrations and KA/QUINO ratios have been associated with reduced connectivity, white matter integrity and cortical thickness and hippocampal volume in MDD (95–97) and bipolar disorder (75, 98) as well as increased glial cell activation [as assessed by Positron Emission Tomography using radioligand (18F)-PBR111] in schizophrenia (99). Surprisingly, a positive correlation between neurotoxic QUINO and increased connectivity in MDD was equally shown (95).
A small sample of melancholic depressed adolescents (n=7) showed strong correlations (r>.90) between plasma KYN and 3-HAA with brain choline, a cell membrane turnover biomarker, in the striatum with magnetic resonance spectroscopy (MRS) (100). Please find the summary of this paragraph in Table 2.
4.3 Link Between Peripheral KP Markers and Treatment Response in Psychiatric Illness
In mood disorders, antidepressant treatment with an selective serotonin reuptake inhibitor (SSRI) (48), ketamine (48, 101), electroconvulsive therapy (ECT) (79, 102) or real time functional magnetic resonance imaging (RT fMRI) neurofeedback training (103) typically have similar effects on the KP pathway as evidenced by overall lowering of QUINO and 3-HK levels (typically thought to be more neurotoxic in nature), as well as increases in KA, which is seen as neuroprotective. These KP changes, as well as baseline high QUINO and low KA levels were predictive for treatment response or remission (48, 101, 104). Whereas plasma TRP levels did not predict treatment response on lithium nor amitriptyline, a subnormal ratio of TRP to other amino acids competing with the LAT1 transporter predicted better outcome in depressed individuals (105). Moreover, antidepressant treatment has been shown to decrease IFN-g expression as well as IDO activity in the brain and in peripheral blood mononuclear cells (PBMCs) (106), leading to reduced overall activation of the kynurenine pathway, mirrored by reduced KYN levels after antidepressant treatment (48). It remains to be investigated whether the antidepressant effect of these treatments is mediated by their impact on kynurenine pathway dynamics. Nonetheless, these results suggest that KP abnormalities may be useful as predictive biomarkers for treatment response.
Antipsychotics have equally shown KP modulating effects in schizophrenia patients. Lowered TRP and KA (107, 108) and increased 3-HK levels (108) normalized after antipsychotic treatment. Cao et al. reported lower KYN levels in unmedicated schizophrenic patients in their meta-analysis, whereas higher KYN levels existed during and after treatment with antipsychotics (72). This also accords with earlier findings by our group, which showed that decreased levels of QUINO and 3-HK in unmedicated psychotic patients tend to normalize after antipsychotic treatment (86).
5 Peripheral and Central Factors Influencing Kynurenine Metabolite Concentrations
The following sections describe the bioavailability and blood brain barrier transport of KP metabolites in normal and immune-activated conditions, which is often the case in psychiatric illness.
5.1 Mechanisms of Entrance of Tryptophan and Kynurenines Into the Brain
The blood-brain barrier (BBB) separates the central nervous system from peripheral circulation and regulates the exchange between these two compartments, protecting the brain from harmful or toxic compounds circulating in the blood, while supplying the brain with nutrients (27).
Tryptophan and kynurenine easily pass the BBB, actively transported by the large neutral amino acid transporter (LAT1) in competition with other essential amino acids such as valine, isoleucine, leucine, tyrosine and phenylalanine (109, 110). LAT1 is ubiquitously expressed on both apical and basolateral sides of the endothelial membranes, as well as on neurons, microglia and astrocytes (111, 112). Although relative concentration differences may be present depending on the compound (113, 114), the transporters provide bidirectional transport to maintain equilibrium of amino acid distribution across both sides of the BBB (115). Preclinical research suggests that 60-78% of the cerebral pool of KYN is imported from the periphery (109, 116, 117) and that TRP transport over the BBB declines with older age (45, 118).
In addition to TRP and KYN, 3-HK is also actively transported over the BBB by LAT1, albeit to a much lesser degree (109, 117). Still, animal research shows that the uptake of systemically administered 3-HK was seven- to eight-fold higher in the brain than in other tissues (119), arguing for efficient metabolite transport over the BBB. AA, another precursor for QUINO (Figure 1), can also pass the BBB easily, presumably by passive diffusion (117, 120).
By contrast, QUINO, 3-HAA and KA are not actively transported over the BBB, restricting brain uptake to passive diffusion (120), which supposedly is very limited due to these compounds’ polar nature (117). This is confirmed by preclinical studies where systemically administered neurotoxic doses of KA and QUINO had no effect on rats (121), while equal doses of a synthetic KA variant that easily passes the BBB instantly killed all animals (122). As they cross the BBB very poorly, cerebral concentrations of KA and QUINO are therefore considered mainly to derive from local production (117). In contrast, several gerbil studies show subcutaneously infused radiolabeled QUINO made up 50-70% of the QUINO brain pool (116, 123), challenging the notion that passive QUINO diffusion over the BBB is limited. However, it is unclear to what extent these findings are extrapolatable to humans.
Cholesterol and fatty acid composition of cell membranes determines membrane fluidity and as a consequence the efficiency of the transport function (124). Data from several studies suggest that cholesterol disturbances are associated with psychiatric disease, including MDD and schizophrenia (125–128). Also, chronic hypertension and insulin seem to facilitate TRP brain uptake in rats (129, 130). However, the role of these factors in altered BBB permeability in psychiatric patients needs further clarification.
5.2 Bioavailability and Transport of Kynurenine Metabolites
As TRP, KYN and KA are known to be loosely bound to human serum albumin (HSA), these compounds first need to be stripped off of HSA in order to be transported to the central nervous system (131–134). In fact, 80-95% of plasma TRP is bound to human serum albumin (HSA), leaving only a small percentage as free TRP (114, 135). Therefore, factors influencing the albumin concentration or interacting with the binding sites have a major impact on the bound/unbound ratio and, consequently, on transportation over the BBB (136, 137). For example, low HSA may occur during liver and kidney disease, prolonged inadequate food intake and in a pro-inflammatory state. Lower TRP in turn affects albumin synthesis (135, 138). Moreover, fatty acids and several drugs, such as salicylates, are able to displace TRP from its binding site, although these mechanisms are difficult to evaluate in vivo (135, 139, 140).
Additionally, it is important to bear in mind that KP enzyme expression and/or activity is changed in various diseases [for review, see (26)].
5.3 Peripheral-Central Neuroimmune Crosstalk and the Effect on the Kynurenine Pathway
Although the brain is considered as an immune-privileged organ since tissue grafts survive when implanted into the CNS parenchyma, the BBB has shown to be permeable to inflammatory proteins and cells under inflammatory conditions, which are able to activate immune responses in the CNS. Several mechanisms may result in crosstalk between the peripheral and central immune system, influencing the functional link between the peripheral and central KP metabolism.
Pro-inflammatory cytokines, which activate the kynurenine pathway both peripherally and centrally, pass the BBB easily through cytokine-specific transporters and circumventricular organs (CVOs) (141), the latter being highly permeable and isolated brain areas characterized by efficient neurohumoral exchange (142). However, the complex interactions between the peripheral and central immune system need further clarification. Cytokine-stimulated activation of the kynurenine pathway in the brain may be mirrored in peripheral tissue, and theoretically peripheral assessments of metabolites such as QUINO or KA could indirectly reflect the situation in brain tissue. During immune activation in the CNS, over 98% of brain-located KYN and QUINO could derive from local production (116). However, Guillemin and colleagues (143) demonstrated that human monocytes and monocyte-derived macrophages can produce up to 19 times more QUINO than activated microglia (143). This is also in line with findings of Espey (32), who showed that synthesis of QUINO by microglia in epilepsy patients was approximately 15% of that produced by monocyte-derived macrophages retrieved from brain tissue. In acute liver failure, an 11-fold increase in QUINO plasma concentrations was mirrored by 1-4-fold elevations in postmortem cerebral tissue, again arguing for more potent QUINO production capacities in peripheral tissue (144). In this line, QUINO concentrations over a range of pathological conditions are systematically higher in blood compared to the CNS with blood/CSF ratios of 14:1 in humans, 19:1 in rodents and up to 52:1 in nonhuman primates, [for review, see (145)]. This can also have relevance to the CNS, as infiltrating activated macrophages could be the most potent QUINO source during brain inflammation (143).
Chronic inflammation leads to an enhanced release of pro-inflammatory cytokines and other components that may alter the microvascular permeability, resulting in a so-called ‘leaky’ blood-brain barrier, which is associated with increased permeability for activated monocytes that may migrate to brain tissue, thus exacerbating neuroinflammation (27).
BBB integrity was shown to be affected in 14-29% of treatment resistant patients with mood and psychotic spectrum disorders (146) and was suggested to be associated with negative symptoms in schizophrenia (147). However, very few psychiatric studies focused on differentiating resident microglia from blood-derived macrophages that migrated to the brain, so little is known about a potentially changed macrophage presence in psychiatric brain tissue and their impact on neuroinflammatory abnormalities. Nonetheless, an invasion of macrophages in brain tissue in 40% of schizophrenic patients in a high inflammatory state as found by Cai and colleagues (148), could alter local QUINO concentrations drastically, given the previously mentioned superior ability of macrophages to produce QUINO compared to microglia. Importantly, QUINO increases result in astrocytic apoptosis, which may further impact BBB integrity (149).
As for KA, early results in an animal model may suggest that (supraphysiological) increased levels of peripheral KA could alter BBB permeability in itself, and in this way penetrate the BBB to reach the brain (150, 151). However, the relevance of these data to humans in general and to KP metabolite concentrations reached in psychiatric illnesses specifically is not clear.
The CNS in turn keeps the peripheral immune activity in check by a number of neuronal control mechanisms. First, although both physiological and psychological stress activates the paraventricular nucleus in order to adapt rapidly to threats of homeostasis, the hypothalamo-pituitary-adrenocortical (HPA) stress response has self-regulating abilities through glucocorticoid negative feedback loops (152, 153). Second, stress-induced cortisol activates multiple physiological reactions, including the induction of TDO. On the other hand, acute cortisol release has anti-inflammatory effects, as it leads to the production of anti-inflammatory cytokines (154). However, persistent elevations of cortisol downregulate the expression of the glucocorticoid receptor which results in glucocorticoid resistance, leading to a pro-inflammatory state as evidenced by elevated IL-6 and TNF-a levels (155).
Interestingly, increased cortisol or treatment with dexamethasone has been associated with low TRP plasma levels in treatment resistant schizophrenics (156) and MDD (157–159).
6 Discussion
Disturbances in the KP are thought to be involved in the pathophysiology of several psychiatric illnesses, such as psychotic and mood disorders. Whereas these abnormalities are easily measured in plasma/serum, empirical evidence of BBB transportation dynamics of the different metabolites under physiological and pathological conditions is limited. The general consensus has been that TRP, KYN and maybe 3-HK easily cross the BBB whereas other downstream metabolites (QUINO, 3-HAA, AA, KA) do not. Nonetheless, this theory has been based on a single study (117) that investigated BBB KP metabolite transport in rats. Evidently, findings in rodents are not always extrapolatable to humans. Kynurenine pathway enzymes might be more active in the brain of higher species (160) and interspecies differences in the KP have been demonstrated (71, 117). The present review was designed to summarize the available correlation coefficients between peripheral and central kynurenine metabolite concentrations.
In clinical studies, KYN and, to a lesser degree, 3-HK, correlate well between blood and CSF samples, irrespective of underlying diagnosis. This is unsurprising, as both metabolites are actively transported over the BBB. However, although TRP equally passes the BBB easily, CSF and peripheral samples taken from the same individual do not always correlate well in human studies for both free and total TRP, especially with regard to more recent studies. High TRP-binding to blood albumin may explain low correlations to central TRP levels. More than 90% of the peripheral TRP is metabolized into KYN, while brain TRP is equally divided over the serotonergic and the kynurenine pathway (69) Additionally, IDO is more represented in the brain, whereas TDO is mainly responsible for metabolization in the periphery; this may contribute to differential levels between CNS and blood. As TRP is an essential amino acid, active transport into the brain may alter the ratio of these molecules between CSF/brain and blood. Downstream metabolites in both branches of the pathway cannot easily pass the BBB due to a lack of active transportation. Yet despite relying solely on passive diffusion, peripheral and central concentrations of QUINO and the precursor AA have shown correlations of moderate strength in neurological (Parkinson’s disease, Alzheimer’s disease) and infectious disorders (HIV, hepatitis C). In psychiatric disorders however, there generally is a lack of available evidence on CSF concentrations of kynurenine metabolites. Interestingly, out of all KP compounds, peripheral KA levels seem to be the least predictive and even diametrically opposed to those in the CSF of depressed and bipolar patients.
Overall, recent meta-analyses and individual psychiatric studies investigating KP metabolites in either peripheral or central samples have shown divergent results across both sides of the BBB (Figure 3). Several reasons can be proposed for these discrepancies. It is to be considered that central and peripheral aberrations may reflect different processes in the human body. Under physiological circumstances for example, intense physical exercise causes transient changes in the KP both in the periphery and centrally (61, 161, 162). KP metabolization in tissue macrophages, PBMCs and other immune cells contribute substantially to peripheral concentrations, whereas central levels of downstream metabolites are mostly determined by the lower enzymatic activity in astrocytes and microglia. Moreover, somatic comorbidities such as autoimmune illnesses, metabolic syndrome, an altered microbiome and hepatic or renal dysfunction (163, 164) may impact peripheral and central KP metabolite concentrations. Even though stress and pro-inflammatory cytokines (IFN-g, TNF-a) equally activate the pathway in peripheral and central tissue (123), it is possible that the correlation between peripheral and CNS KP findings depends on the type and level of inflammation. While high-level inflammation and/or BBB disintegrity may lead to parallel changes in CNS and blood KP (Table 1), this is not necessarily the case in the chronic mild inflammatory conditions found in psychiatric illness (Figure 3). The observed positive correlations in blood-CSF QUINO concentrations in MDD, hepatitis and HIV, but not in healthy volunteers, could be attributable to the inflammatory conditions in these illnesses damaging the BBB. On the other hand, peripheral findings in psychiatric illness do represent valuable biomarkers, associated with symptom severity and treatment response, as well as other core biological features of the disorder as discussed in section 6. This corroborates the notion of psychiatric illnesses as ‘whole-body disorders’ rather than brain disorders (165).
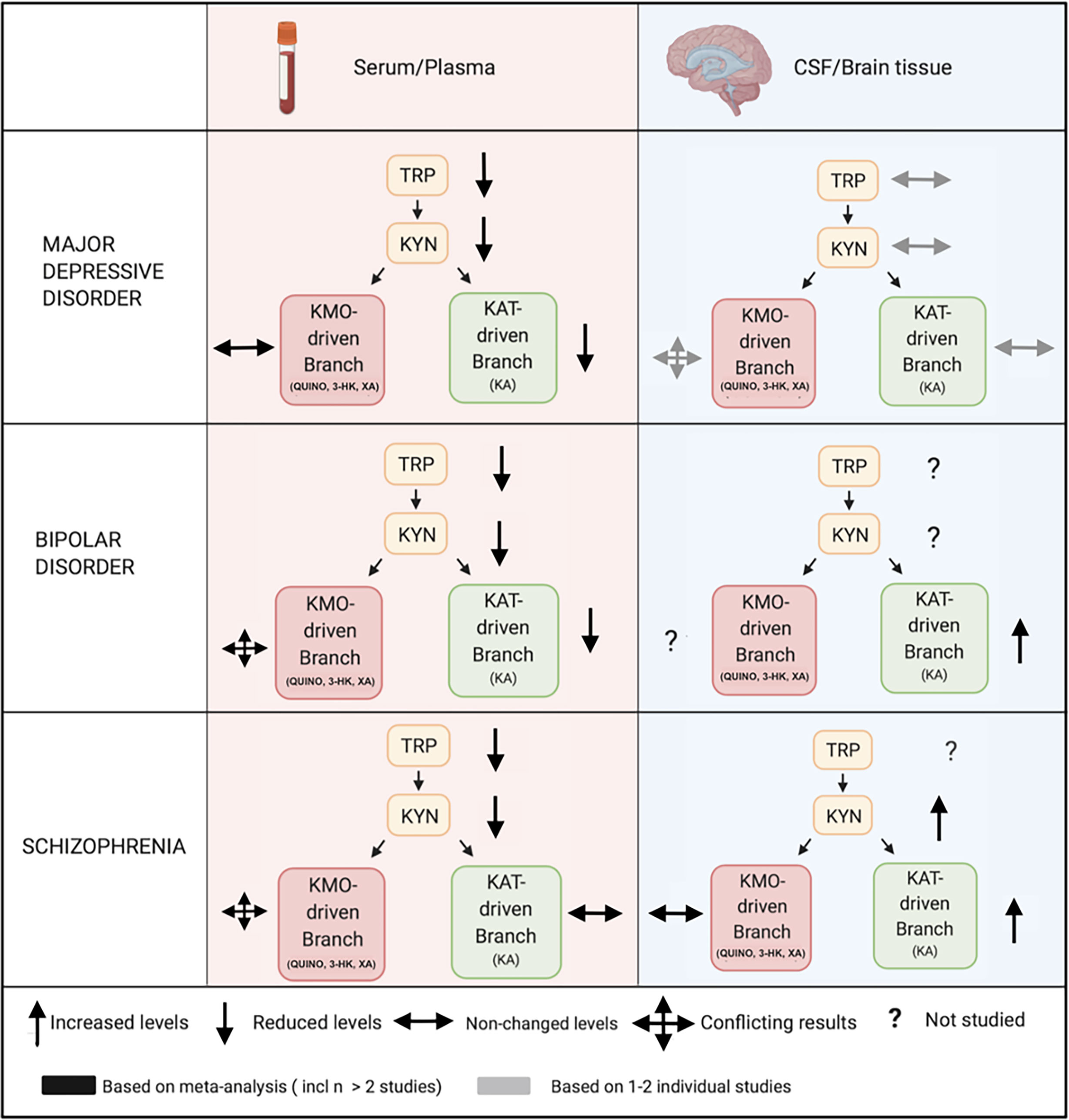
Figure 3 Comparing peripheral (serum/plasma) and central (CSF/brain tissue) kynurenine pathway findings in major psychiatric disorders. Legend: In mood disorders, peripheral studies demonstrate decreased measures of blood TRP, KYN and a downregulation of the KAT-driven branch in the periphery, which is possibly explained by a decreased availability of TRP to the KP. Central studies investigating cerebrospinal fluid (CSF) or brain tissue are less conclusive, but are very limited. In schizophrenia, peripheral findings are much less clear downstream with very conflicting results. Central studies in schizophrenia, also limited and mainly based on CSF research on KA, equally suggest an activation of the pathway, reflected by KYN increases, accompanied by a shift towards the astrocyte-derived branch. KP, kynurenine pathway; 3-HK, 3-hydroxy-kynurenine; KA, kynurenic acid; KYN, kynurenine; QUINO, quinolinic acid; TRP, tryptophan; XA, xanthurenic acid; KMO, kynurenine 3-monooxygenase; KAT, kynurenine aminotransferase.
Psychiatric diagnoses are typically based on clinical phenotypes which are now widely accepted to represent a heterogeneous group of biotypes. Several biological dysregulations (immune, neuroendocrine, metabolic,…) are therefore nonspecific, overlapping between diagnostic categories. Kynurenine abnormalities may thus vary over studies depending on the representation of the different biotypes in these studies. Symptomatic state (14), medication status and use of illicit substances - in particular THC - (86, 108, 166) may also impact KP alterations, further adding to the variability. The complex interaction between KP and the dimensional aspects of different psychiatric syndromes needs further scrutiny. An increased KYN/TRP ratio as well as low KA and/or high QUINO emerge as true transdiagnostic blood-based trait markers across the three major psychiatric disorders. A similarly strong overlap has recently been demonstrated in the polygenic risk factors related to these disorders (167) (see Table 2).
Methodological issues should also be considered. Study sample sizes have systematically been small, especially in those investigating central KP metabolites. A recent meta-analysis (19) demonstrated that schizophrenia-related KYN findings in CSF were based on a total of 60 patients over 3 studies, and KA on a total of 148 patients over 4 studies. Although the role of QUINO in the pathophysiology of schizophrenia has been a favored and frequently repeated hypothesis in many opinion papers and reviews, no study has actually investigated QUINO in the CSF of schizophrenia patients to date. Similarly, the KP was investigated in the CSF of below 100 MDD patients (17–19). Postmortem studies equally tend to include smaller sample sizes (168–170). The fact that data on microglia-driven metabolites appear more mixed than KA levels, may simply result from the fact that these metabolites have hardly been investigated. Another methodological issue is that some metabolites (e.g. QUINO) are present in very low ranges (200-600 nM/L) and QUINO findings over studies differ with factors up to one million, which may reflect bioanalytical inaccuracies related to the assays’ lower detection limits that are nonetheless rarely acknowledged in these papers (171). In this line, the smaller ranges of KP metabolite alterations found in psychiatric illnesses (75, 76) may need higher sample sizes in order to achieve sufficient statistical power compared to studies investigating neurological and infectious illnesses, which are accompanied by high-grade systemic responses and more profound blood-brain barrier disintegrety (63, 65, 73). Another issue is that CSF findings may not reflect brain KP metabolism in situ, especially in the context of a ‘leaky’ brain, as CSF may be more representative of KP enzyme activity in periventricular macrophages rather than parenchymal glial cells. Moreover, the total volume of the obtained CSF and the exact intervertebral height have a significant impact on protein concentration as the concentration decreases when descending along the vertebral column (172).
Finally, KP enzyme activity is typically estimated using metabolite ratios. For example, TDO/IDO activity is typically calculated from KYN/TRP ratios. Actual assessment of enzyme activity (e.g. in isolated cell types such as PBMCs) or genetic expression of these enzymes may be more relevant and representative.
In conclusion, KYN and 3-HK measured in plasma or serum seem to reflect their concentrations in brain tissue, but this relationship is less clear in TRP and more downstream metabolites of the KP. Even if peripheral concentrations do not correlate with central measures in psychiatric illness, they are not necessarily without merit as relevant biomarkers of phenotypical features and treatment response. Nonetheless, many potential confounders may contribute to diverging central and peripheral assessments, and more fundamental research is needed to clarify these issues. Future studies should investigate 1) to what extent KP metabolites pass BBB in humans (e.g. by use of radioactive labelling), 2) whether CSF concentrations reflect in situ brain abnormalities and to what extent these KP abnormalities are systemic or region-dependent in the brain, 3) how well CSF and blood concentrations of microglial branch metabolites intercorrelate in mood and psychotic disorders and 4) what the impact is of medication, symptom status and illness phase on KP abnormalities. Finally, we strongly recommend future studies investigating the KP in psychiatric illness to assess (at least) the following metabolites: TRP, KYN, QUINO, 3-HK and KA.
Data Availability Statement
The original contributions presented in the study are included in the article. Further inquiries can be directed to the corresponding author.
Author Contributions
KS, LP, VC, and MM equally contributed to the study design of this review. KS and MM performed the literature search, interpreted the data and wrote the manuscript. LP, VC, and RV profoundly ameliorated the manuscript by adding important intellectual content. All authors contributed to the article and approved the submitted version.
Conflict of Interest
The authors declare that the research was conducted in the absence of any commercial or financial relationships that could be construed as a potential conflict of interest.
Publisher’s Note
All claims expressed in this article are solely those of the authors and do not necessarily represent those of their affiliated organizations, or those of the publisher, the editors and the reviewers. Any product that may be evaluated in this article, or claim that may be made by its manufacturer, is not guaranteed or endorsed by the publisher.
References
1. Miller AH, Maletic V, Raison CL. Inflammation and its Discontents: The Role of Cytokines in the Pathophysiology of Major Depression. Biol Psychiatry (2009) 65(9):732–41. doi: 10.1016/j.biopsych.2008.11.029
2. Hudson ZD, Miller BJ. Meta-Analysis of Cytokine and Chemokine Genes in Schizophrenia. Clin Schizophr Relat Psychoses (2018) 12(3):121–9B. doi: 10.3371/CSRP.HUMI.070516
3. van den Ameele S, van Diermen L, Staels W, Coppens V, Dumont G, Sabbe B, et al. The Effect of Mood-Stabilizing Drugs on Cytokine Levels in Bipolar Disorder: A Systematic Review. J Affect Disord (2016) 203:364–73. doi: 10.1016/j.jad.2016.06.016
4. Ottoy J, De Picker L, Verhaeghe J, Deleye S, Wyffels L, Kosten L, et al. F-PBR111 PET Imaging in Healthy Controls and Schizophrenia: Test-Retest Reproducibility and Quantification of Neuroinflammation. J Nucl Med (2018) 59(8):1267–74. doi: 10.2967/jnumed.117.203315
5. De Picker LJ, Morrens M, Chance SA, Boche D. Microglia and Brain Plasticity in Acute Psychosis and Schizophrenia Illness Course: A Meta-Review. Front Psychiatry (2017) 8:238. doi: 10.3389/fpsyt.2017.00238
6. Haarman BCMB, Riemersma-Van der Lek RF, de Groot JC, Ruhé HGE, Klein HC, Zandstra TE, et al. Neuroinflammation in Bipolar Disorder - A [(11)C]-(R)-PK11195 Positron Emission Tomography Study. Brain Behav Immun (2014) 40:219–25. doi: 10.1016/j.bbi.2014.03.016
7. De Picker LJ, Haarman BCM. Applicability, Potential and Limitations of TSPO PET Imaging as a Clinical Immunopsychiatry Biomarker. Eur J Nucl Med Mol Imaging [Internet] (2021) 48:1–10. doi: 10.1007/s00259-021-05308-0
8. Cassiers LLM, Niemegeers P, Fransen E, Morrens M, De Boer P, Van Nueten L, et al. Neuroendocrine and Inflammatory Effects of Childhood Trauma Following Psychosocial and Inflammatory Stress in Women With Remitted Major Depressive Disorder. Brain Sci [Internet] (2019) 9(12):1–13. doi: 10.3390/brainsci9120375
9. Niemegeers P, De Boer P, Dumont GJH, Van Den Eede F, Fransen E, Claes SJ, et al. Differential Effects of Inflammatory and Psychosocial Stress on Mood, Hypothalamic-Pituitary-Adrenal Axis, and Inflammation in Remitted Depression. Neuropsychobiology (2016) 74(3):150–8. doi: 10.1159/000466698
10. Kruse JL, Cho JH-J, Olmstead R, Hwang L, Faull K, Eisenberger NI, et al. Kynurenine Metabolism and Inflammation-Induced Depressed Mood: A Human Experimental Study. Psychoneuroendocrinology (2019) 109:104371. doi: 10.1016/j.psyneuen.2019.104371
11. Nettis MA, Lombardo G, Hastings C, Zajkowska Z, Mariani N, Nikkheslat N, et al. Augmentation Therapy With Minocycline in Treatment-Resistant Depression Patients With Low-Grade Peripheral Inflammation: Results From a Double-Blind Randomised Clinical Trial. Neuropsychopharmacology (2021) 46(5):939–48. doi: 10.1038/s41386-020-00948-6
12. Faurbye A. The Role of Amines in the Etiology of Schizophrenia. Compr Psychiatry (1968) 9(2):155–77. doi: 10.1016/S0010-440X(68)80051-3
13. Lapin IP, Oxenkrug GF. Intensification of the Central Serotoninergic Processes as a Possible Determinant of the Thymoleptic Effect. Lancet (1969) 1(7586):132–6. doi: 10.1016/S0140-6736(69)91140-4
14. Morrens M, De Picker L, Kampen JK, Coppens V. Blood-Based Kynurenine Pathway Alterations in Schizophrenia Spectrum Disorders: A Meta-Analysis. Schizophr Res (2020) 223:43–52. doi: 10.1016/j.schres.2020.09.007
15. Marx W, McGuinness AJ, Rocks T, Ruusunen A, Cleminson J, Walker AJ, et al. The Kynurenine Pathway in Major Depressive Disorder, Bipolar Disorder, and Schizophrenia: A Meta-Analysis of 101 Studies. Mol Psychiatry [Internet] (2020) 25:1–21. doi: 10.1038/s41380-020-00951-9
16. Hebbrecht K, Skorobogatov K, Giltay EJ, Coppens V, De Picker L, Morrens M. Tryptophan Catabolites in Bipolar Disorder: A Meta-Analysis. Front Immunol (2021) 12:667179. doi: 10.3389/fimmu.2021.667179
17. Hestad KA, Engedal K, Whist JE, Farup PG. The Relationships Among Tryptophan, Kynurenine, Indoleamine 2,3-Dioxygenase, Depression, and Neuropsychological Performance. Front Psychol (2017) 8:1561. doi: 10.3389/fpsyg.2017.01561
18. Erhardt S, Lim CK, Linderholm KR, Janelidze S, Lindqvist D, Samuelsson M, et al. Connecting Inflammation With Glutamate Agonism in Suicidality [Internet]. Neuropsychopharmacology (2013) 38:743–52. doi: 10.1038/npp.2012.248
19. Wang AK, Miller BJ. Meta-Analysis of Cerebrospinal Fluid Cytokine and Tryptophan Catabolite Alterations in Psychiatric Patients: Comparisons Between Schizophrenia, Bipolar Disorder, and Depression. Schizophr Bull (2018) 44(1):75–83. doi: 10.1093/schbul/sbx035
20. Sellgren CM, Gracias J, Jungholm O, Perlis RH, Engberg G, Schwieler L, et al. Peripheral and Central Levels of Kynurenic Acid in Bipolar Disorder Subjects and Healthy Controls. Transl Psychiatry (2019) 9(1):37. doi: 10.1038/s41398-019-0378-9
21. Arnone D, Saraykar S, Salem H, Teixeira AL, Dantzer R, Selvaraj S. Role of Kynurenine Pathway and its Metabolites in Mood Disorders: A Systematic Review and Meta-Analysis of Clinical Studies. Neurosci Biobehav Rev (2018) 92:477–85. doi: 10.1016/j.neubiorev.2018.05.031
22. Ogyu K, Kubo K, Noda Y, Iwata Y, Tsugawa S, Omura Y, et al. Kynurenine Pathway in Depression: A Systematic Review and Meta-Analysis. Neurosci Biobehav Rev (2018) 90:16–25. doi: 10.1016/j.neubiorev.2018.03.023
23. Dang Y, Dale WE, Brown OR. Comparative Effects of Oxygen on Indoleamine 2,3-Dioxygenase and Tryptophan 2,3-Dioxygenase of the Kynurenine Pathway [Internet]. Free Radical Biol Med (2000) 28:615–24. doi: 10.1016/s0891-5849(99)00272-5
24. Miller CL, Llenos IC, Dulay JR, Barillo MM, Yolken RH, Weis S. Expression of the Kynurenine Pathway Enzyme Tryptophan 2,3-Dioxygenase is Increased in the Frontal Cortex of Individuals With Schizophrenia. Neurobiol Dis (2004) 15(3):618–29. doi: 10.1016/j.nbd.2003.12.015
25. Ohira K, Hagihara H, Toyama K, Takao K, Kanai M, Funakoshi H, et al. Expression of Tryptophan 2,3-Dioxygenase in Mature Granule Cells of the Adult Mouse Dentate Gyrus. Mol Brain (2010) 3(1):26. doi: 10.1186/1756-6606-3-26
26. Badawy AA-B. Kynurenine Pathway of Tryptophan Metabolism: Regulatory and Functional Aspects. Int J Tryptophan Res (2017) 10:1178646917691938. doi: 10.1177/1178646917691938
27. Ascoli BM, Géa LP, Colombo R, Barbé-Tuana FM, Kapczinski F, Rosa AR. The Role of Macrophage Polarization on Bipolar Disorder: Identifying New Therapeutic Targets. Aust N Z J Psychiatry (2016) 50(7):618–30. doi: 10.1177/0004867416642846
28. Fuertig R, Azzinnari D, Bergamini G, Cathomas F, Sigrist H, Seifritz E, et al. Mouse Chronic Social Stress Increases Blood and Brain Kynurenine Pathway Activity and Fear Behaviour: Both Effects are Reversed by Inhibition of Indoleamine 2,3-Dioxygenase. Brain Behav Immun (2016) 54:59–72. doi: 10.1016/j.bbi.2015.12.020
29. Guillemin GJ. Quinolinic Acid, the Inescapable Neurotoxin. FEBS J (2012) 279(8):1356–65. doi: 10.1111/j.1742-4658.2012.08485.x
30. Dang Y, Dale WE, Brown OR. Effects of Oxygen on Kynurenine-3-Monooxygenase Activity [Internet]. Redox Rep (2000) 5:81–4. doi: 10.1179/135100000101535564
31. Dostal CR, Sulzer MC, Kelley KW, Freund GG, McCusker RH. Glial and Tissue-Specific Regulation of Kynurenine Pathway Dioxygenases by Acute Stress of Mice [Internet]. Neurobiol Stress (2017) 7:1–15. doi: 10.1016/j.ynstr.2017.02.002
32. Espey MG, Chernyshev ON, Reinhard JF Jr, Namboodiri MA, Colton CA. Activated Human Microglia Produce the Excitotoxin Quinolinic Acid. Neuroreport (1997) 8(2):431–4. doi: 10.1097/00001756-199701200-00011
33. Giorgini F, Huang S-Y, Sathyasaikumar KV, Notarangelo FM, Thomas MAR, Tararina M, et al. Targeted Deletion of Kynurenine 3-Monooxygenase in Mice: A New Tool for Studying Kynurenine Pathway Metabolism in Periphery and Brain. J Biol Chem (2013) 288(51):36554–66. doi: 10.1074/jbc.M113.503813
34. Guillemin GJ, Kerr SJ, Smythe GA, Smith DG, Kapoor V, Armati PJ, et al. Kynurenine Pathway Metabolism in Human Astrocytes: A Paradox for Neuronal Protection. J Neurochem (2001) 78(4):842–53. doi: 10.1046/j.1471-4159.2001.00498.x
35. Brown SJ, Huang X-F, Newell KA. The Kynurenine Pathway in Major Depression: What We Know and Where to Next. Neurosci Biobehav Rev (2021) 127:917–27. doi: 10.1016/j.neubiorev.2021.05.018
36. Javitt DC, Zukin SR. Recent Advances in the Phencyclidine Model of Schizophrenia. Am J Psychiatry (1991) 148(10):1301–8. doi: 10.1176/ajp.148.10.1301
37. Moghaddam B, Krystal JH. Capturing the Angel in “Angel Dust”: Twenty Years of Translational Neuroscience Studies of NMDA Receptor Antagonists in Animals and Humans. Schizophr Bull (2012) 38(5):942–9. doi: 10.1093/schbul/sbs075
38. Schwarcz R, Stone TW. The Kynurenine Pathway and the Brain: Challenges, Controversies and Promises. Neuropharmacology (2017) 112(Pt B):237–47. doi: 10.1016/j.neuropharm.2016.08.003
39. Smith JR, Jamie JF, Guillemin GJ. Kynurenine-3-Monooxygenase: A Review of Structure, Mechanism, and Inhibitors. Drug Discov Today (2016) 21(2):315–24. doi: 10.1016/j.drudis.2015.11.001
40. Han Q, Cai T, Tagle DA, Li J. Structure, Expression, and Function of Kynurenine Aminotransferases in Human and Rodent Brains. Cell Mol Life Sci (2010) 67(3):353–68. doi: 10.1007/s00018-009-0166-4
41. Bora E. Peripheral Inflammatory and Neurotrophic Biomarkers of Cognitive Impairment in Schizophrenia: A Meta-Analysis - CORRIGENDUM. Psychol Med (2020) 1:1971–9. doi: 10.1017/S0033291720001701
42. Peterson RA, Brown SP. On the Use of Beta Coefficients in Meta-Analysis. J Appl Psychol (2005) 90(1):175–81. doi: 10.1037/0021-9010.90.1.175
43. Moher D, Liberati A, Tetzlaff J, Altman DG, PRISMA Group. Preferred Reporting Items for Systematic Reviews and Meta-Analyses: The PRISMA Statement. Int J Surg (2010) 8(5):336–41. doi: 10.1016/j.ijsu.2010.02.007
44. Crandall EA, Fernstrom JD. Effect of Experimental Diabetes on the Levels of Aromatic and Branched-Chain Amino Acids in Rat Blood and Brain. Diabetes (1983) 32(3):222–30. doi: 10.2337/diab.32.3.222
45. Sarna GS, Tricklebank MD, Kantamaneni BD, Hunt A, Patel AJ, Curzon G. Effect of Age on Variables Influencing the Supply of Tryptophan to the Brain. J Neurochem (1982) 39(5):1283–90. doi: 10.1111/j.1471-4159.1982.tb12567.x
46. Gabriel Manjarrez G, Hernández ZE, Robles OA, González RM, Hernández RJ. Developmental Impairment of Auditory Evoked N1/P2 Component in Rats Undernourished In Utero: Its Relation to Brain Serotonin Activity. Brain Res Dev Brain Res (2001) 127(2):149–55. doi: 10.1016/S0165-3806(01)00129-8
47. Yokogoshi H, Iwata T, Ishida K, Yoshida A. Effect of Amino Acid Supplementation to Low Protein Diet on Brain and Plasma Levels of Tryptophan and Brain 5-Hydroxyindoles in Rats. J Nutr (1987) 117(1):42–7. doi: 10.1093/jn/117.1.42
48. Verdonk F, Petit A-C, Abdel-Ahad P, Vinckier F, Jouvion G, de Maricourt P, et al. Microglial Production of Quinolinic Acid as a Target and a Biomarker of the Antidepressant Effect of Ketamine. Brain Behav Immun (2019) 81:361–73. doi: 10.1016/j.bbi.2019.06.033
49. Gregoire L, Rassoulpour A, Guidetti P, Samadi P, Bedard P, Izzo E, et al. Prolonged Kynurenine 3-Hydroxylase Inhibition Reduces Development of Levodopa-Induced Dyskinesias in Parkinsonian Monkeys [Internet]. Behav Brain Res (2008) 186:161–7. doi: 10.1016/j.bbr.2007.08.007
50. Saito K, Crowley JS, Markey SP, Heyes MP. A Mechanism for Increased Quinolinic Acid Formation Following Acute Systemic Immune Stimulation. J Biol Chem (1993) 268(21):15496–503. doi: 10.1016/S0021-9258(18)82284-0
51. Moreno FA, Parkinson D, Palmer C, Castro WL, Misiaszek J, El Khoury A, et al. CSF Neurochemicals During Tryptophan Depletion in Individuals With Remitted Depression and Healthy Controls. Eur Neuropsychopharmacol (2010) 20(1):18–24. doi: 10.1016/j.euroneuro.2009.10.003
52. Haroon E, Welle JR, Woolwine BJ, Goldsmith DR, Baer W, Patel T, et al. Associations Among Peripheral and Central Kynurenine Pathway Metabolites and Inflammation in Depression. Neuropsychopharmacology (2020) 45(6):998–1007. doi: 10.1038/s41386-020-0607-1
53. Young SN, Lal S, Sourkes TL, Feldmuller F, Aronoff A, Martin JB. Relationships Between Tryptophan in Serum and CSF, and 5-Hydroxyindoleacetic Acid in CSF of Man: Effect of Cirrhosis of Liver and Probenecid Administration. J Neurol Neurosurg Psychiatry (1975) 38(4):322–30. doi: 10.1136/jnnp.38.4.322
54. Sullivan PA, Murnaghan D, Callaghan N, Kantamaneni BD, Curzon G. Cerebral Transmitter Precursors and Metabolites in Advanced Renal Disease. J Neurol Neurosurg Psychiatry (1978) 41(7):581–8. doi: 10.1136/jnnp.41.7.581
55. Kruse T, Reiber H, Neuhoff V. Amino Acid Transport Across the Human Blood-CSF Barrier. J Neurol Sci (1985) 70(2):129–38. doi: 10.1016/0022-510X(85)90082-6
56. Young SN, Lal S, Feldmuller F, Sourkes TL, Ford RM, Kiely M, et al. Parallel Variation of Ventricular CSF Tryptophan and Free Serum Tryptophan in Man. J Neurol Neurosurg Psychiatry (1976) 39(1):61–5. doi: 10.1136/jnnp.39.1.61
57. Gillman PK, Bartlett JR, Bridges PK, Kantamaneni BD, Curzon G. Relationships Between Tryptophan Concentrations in Human Plasma, Cerebrospinal Fluid and Cerebral Cortex Following Tryptophan Infusion. Neuropharmacology (1980) 19(12):1241–2. doi: 10.1016/0028-3908(80)90217-8
58. Curzon G, Kantamaneni BD, Van Boxel P, Gillman PK, Bartlett JR, Bridges PK. Substances Related to 5-Hydroxytryptamine in Plasma and in Lumbar and Ventricular Fluids of Psychiatric Patients. Acta Psychiatr Scand Suppl (1980) 280:3–20. doi: 10.1111/acps.1980.61.s280.3
59. Cangiano C, Cascino A, Ceci F, Laviano A, Mulieri M, Muscaritoli M, et al. Plasma and CSF Tryptophan in Cancer Anorexia. J Neural Transm Gen Sect (1990) 81(3):225–33. doi: 10.1007/BF01245044
60. Sarrias MJ, Cabré P, Martínez E, Artigas F. Relationship Between Serotoninergic Measures in Blood and Cerebrospinal Fluid Simultaneously Obtained in Humans. J Neurochem (1990) 54(3):783–6. doi: 10.1111/j.1471-4159.1990.tb02319.x
61. Isung J, Granqvist M, Trepci A, Huang J, Schwieler L, Kierkegaard M, et al. Differential Effects on Blood and Cerebrospinal Fluid Immune Protein Markers and Kynurenine Pathway Metabolites From Aerobic Physical Exercise in Healthy Subjects. Sci Rep (2021) 11(1):1669. doi: 10.1038/s41598-021-81306-4
62. Heyes MP, Brew BJ, Saito K, Quearry BJ, Price RW, Lee K, et al. Inter-Relationships Between Quinolinic Acid, Neuroactive Kynurenines, Neopterin and Beta 2-Microglobulin in Cerebrospinal Fluid and Serum of HIV-1-Infected Patients. J Neuroimmunol (1992) 40(1):71–80. doi: 10.1016/0165-5728(92)90214-6
63. Raison CL, Dantzer R, Kelley KW, Lawson MA, Woolwine BJ, Vogt G, et al. CSF Concentrations of Brain Tryptophan and Kynurenines During Immune Stimulation With IFN-Alpha: Relationship to CNS Immune Responses and Depression. Mol Psychiatry (2010) 15(4):393–403. doi: 10.1038/mp.2009.116
64. Havelund JF, Andersen AD, Binzer M, Blaabjerg M, Heegaard NHH, Stenager E, et al. Changes in Kynurenine Pathway Metabolism in Parkinson Patients With L-DOPA-Induced Dyskinesia. J Neurochem (2017) 142(5):756–66. doi: 10.1111/jnc.14104
65. Jacobs KR, Lim CK, Blennow K, Zetterberg H, Chatterjee P, Martins RN, et al. Correlation Between Plasma and CSF Concentrations of Kynurenine Pathway Metabolites in Alzheimer’s Disease and Relationship to Amyloid-β and Tau. Neurobiol Aging (2019) 80:11–20. doi: 10.1016/j.neurobiolaging.2019.03.015
66. Valle M, Price RW, Nilsson A, Heyes M, Verotta D. CSF Quinolinic Acid Levels are Determined by Local HIV Infection: Cross-Sectional Analysis and Modelling of Dynamics Following Antiretroviral Therapy. Brain (2004) 127(Pt 5):1047–60. doi: 10.1093/brain/awh130
67. Goeden N, Notarangelo FM, Pocivavsek A, Beggiato S, Bonnin A, Schwarcz R. Prenatal Dynamics of Kynurenine Pathway Metabolism in Mice: Focus on Kynurenic Acid. Dev Neurosci (2017) 39(6):519–28. doi: 10.1159/000481168
68. Zádor F, Nagy-Grócz G, Dvorácskó S, Bohár Z, Cseh EK, Zádori D, et al. Long-Term Systemic Administration of Kynurenic Acid Brain Region Specifically Elevates the Abundance of Functional CB1 Receptors in Rats [Internet]. Neurochem Int (2020) 138:104752. doi: 10.1016/j.neuint.2020.104752
69. Badawy AA-B, Bano S. Tryptophan Metabolism in Rat Liver After Administration of Tryptophan, Kynurenine Metabolites, and Kynureninase Inhibitors. Int J Tryptophan Res (2016) 9:51–65. doi: 10.4137/IJTR.S38190
70. Li C-C, Jiang N, Gan L, Zhao M-J, Chang Q, Liu X-M, et al. Peripheral and Cerebral Abnormalities of the Tryptophan Metabolism in the Depression-Like Rats Induced by Chronic Unpredicted Mild Stress. Neurochem Int (2020) :138:104771. doi: 10.1016/j.neuint.2020.104771
71. Heyes MP, Saito K, Chen CY, Proescholdt MG, Nowak TS Jr, Li J, et al. Species Heterogeneity Between Gerbils and Rats: Quinolinate Production by Microglia and Astrocytes and Accumulations in Response to Ischemic Brain Injury and Systemic Immune Activation. J Neurochem (1997) 69(4):1519–29. doi: 10.1046/j.1471-4159.1997.69041519.x
72. Cao B, Chen Y, Ren Z, Pan Z, McIntyre RS, Wang D. Dysregulation of Kynurenine Pathway and Potential Dynamic Changes of Kynurenine in Schizophrenia: A Systematic Review and Meta-Analysis. Neurosci Biobehav Rev (2021) 123:203–14. doi: 10.1016/j.neubiorev.2021.01.018
73. Heyes MP, Brew BJ, Martin A, Price RW, Salazar AM, Sidtis JJ, et al. Quinolinic Acid in Cerebrospinal Fluid and Serum in HIV-1 Infection: Relationship to Clinical and Neurological Status. Ann Neurol (1991) 29(2):202–9. doi: 10.1002/ana.410290215
74. Young KD, Drevets WC, Dantzer R, Teague TK, Bodurka J, Savitz J. Kynurenine Pathway Metabolites are Associated With Hippocampal Activity During Autobiographical Memory Recall in Patients With Depression. Brain Behav Immun (2016) 56:335–42. doi: 10.1016/j.bbi.2016.04.007
75. Meier TB, Drevets WC, Wurfel BE, Ford BN, Morris HM, Victor TA, et al. Relationship Between Neurotoxic Kynurenine Metabolites and Reductions in Right Medial Prefrontal Cortical Thickness in Major Depressive Disorder. Brain Behav Immun (2016) 53:39–48. doi: 10.1016/j.bbi.2015.11.003
76. Kindler J, Lim CK, Weickert CS, Boerrigter D, Galletly C, Liu D, et al. Dysregulation of Kynurenine Metabolism is Related to Proinflammatory Cytokines, Attention, and Prefrontal Cortex Volume in Schizophrenia. Mol Psychiatry [Internet] (2019) 25:2860–72. doi: 10.1038/s41380-019-0401-9
77. Badawy AA-B A-B, Badawy A. Mechanisms of Elevation of Rat Brain Tryptophan Concentration by Various Doses of Salicylate [Internet]. Br J Pharmacol (1982) 76:211–3. doi: 10.1111/j.1476-5381.1982.tb09208.x
78. Gabbay V, Ely BA, Babb J, Liebes L. The Possible Role of the Kynurenine Pathway in Anhedonia in Adolescents. J Neural Transm (2012) 119(2):253–60. doi: 10.1007/s00702-011-0685-7
79. Ryan KM, Allers KA, McLoughlin DM, Harkin A. Tryptophan Metabolite Concentrations in Depressed Patients Before and After Electroconvulsive Therapy. Brain Behav Immun (2020) 83:153–62. doi: 10.1016/j.bbi.2019.10.005
80. Bradley KAL, Case JAC, Khan O, Ricart T, Hanna A, Alonso CM, et al. The Role of the Kynurenine Pathway in Suicidality in Adolescent Major Depressive Disorder. Psychiatry Res (2015) 227(2-3):206–12. doi: 10.1016/j.psychres.2015.03.031
81. Myint AM, Kim Y-K, Verkerk R, Park SH, Scharpé S, Steinbusch HWM, et al. Tryptophan Breakdown Pathway in Bipolar Mania. J Affect Disord (2007) 102(1-3):65–72. doi: 10.1016/j.jad.2006.12.008
82. Russ MJ, Ackerman SH, Banay-Schwartz M, Shindledecker RD, Gerard PS. Plasma Tryptophan to Large Neutral Amino Acid Ratios in Depressed and Normal Subjects. J Affect Disord (1990) 19(1):9–14. doi: 10.1016/0165-0327(90)90003-Q
83. Liu H, Ding L, Zhang H, Mellor D, Wu H, Zhao D, et al. The Metabolic Factor Kynurenic Acid of Kynurenine Pathway Predicts Major Depressive Disorder [Internet]. Front Psychiatry (2018) 9:1–9. doi: 10.3389/fpsyt.2018.00552
84. Achtyes E, Keaton SA, Smart L, Burmeister AR, Heilman PL, Krzyzanowski S, et al. Inflammation and Kynurenine Pathway Dysregulation in Post-Partum Women With Severe and Suicidal Depression. Brain Behav Immun (2020) 83:239–47. doi: 10.1016/j.bbi.2019.10.017
85. Öztürk M, Yalın Sapmaz Ş, Kandemir H, Taneli F, Aydemir Ö. The Role of the Kynurenine Pathway and Quinolinic Acid in Adolescent Major Depressive Disorder. Int J Clin Pract (2020) e13739. doi: 10.1111/ijcp.13739
86. De Picker L, Fransen E, Coppens V, Timmers M, de Boer P, Oberacher H, et al. Immune and Neuroendocrine Trait and State Markers in Psychotic Illness: Decreased Kynurenines Marking Psychotic Exacerbations. Front Immunol [Internet] (2020) 10:1–12. doi: 10.3389/fimmu.2019.02971
87. Badawy AA-B, A-B Badawy A, Doughrty DM, Marsh-Richard DM, Steptoe A. Activation of Liver Tryptophan Pyrrolase Mediates the Decrease in Tryptophan Availability to the Brain After Acute Alcohol Consumption by Normal Subjects [Internet]. Alcohol Alcoholism (2009) 44:267–71. doi: 10.1093/alcalc/agp005
88. Brundin L, Sellgren CM, Lim CK, Grit J, Pålsson E, Landén M, et al. An Enzyme in the Kynurenine Pathway That Governs Vulnerability to Suicidal Behavior by Regulating Excitotoxicity and Neuroinflammation. Transl Psychiatry (2016) 6(8):e865. doi: 10.1038/tp.2016.133
89. Platzer M, Dalkner N, Fellendorf FT, Birner A, Bengesser SA, Queissner R, et al. Tryptophan Breakdown and Cognition in Bipolar Disorder. Psychoneuroendocrinology (2017) 81:144–50. doi: 10.1016/j.psyneuen.2017.04.015
90. Huang X, Ding W, Wu F, Zhou S, Deng S, Ning Y. Increased Plasma Kynurenic Acid Levels are Associated With Impaired Attention/Vigilance and Social Cognition in Patients With Schizophrenia. Neuropsychiatr Dis Treat (2020) 16:263–71. doi: 10.2147/NDT.S239763
91. Steen NE, Dieset I, Hope S, Vedal TSJ, Smeland OB, Matson W, et al. Metabolic Dysfunctions in the Kynurenine Pathway, Noradrenergic and Purine Metabolism in Schizophrenia and Bipolar Disorders. Psychol Med (2020) 50(4):595–606. doi: 10.1017/S0033291719000400
92. Poletti S, Melloni E, Aggio V, Colombo C, Valtorta F, Benedetti F, et al. Grey and White Matter Structure Associates With the Activation of the Tryptophan to Kynurenine Pathway in Bipolar Disorder. J Affect Disord (2019) 259:404–12. doi: 10.1016/j.jad.2019.08.034
93. Chiappelli J, Postolache TT, Kochunov P, Rowland LM, Wijtenburg SA, Shukla DK, et al. Tryptophan Metabolism and White Matter Integrity in Schizophrenia. Neuropsychopharmacology (2016) 41(10):2587–95. doi: 10.1038/npp.2016.66
94. Savitz J, Dantzer R, Meier TB, Wurfel BE, Victor TA, McIntosh SA, et al. Activation of the Kynurenine Pathway is Associated With Striatal Volume in Major Depressive Disorder. Psychoneuroendocrinology (2015) 62:54–8. doi: 10.1016/j.psyneuen.2015.07.609
95. DeWitt SJ, Bradley KA, Lin N, Yu C, Gabbay V. A Pilot Resting-State Functional Connectivity Study of the Kynurenine Pathway in Adolescents With Depression and Healthy Controls [Internet]. J Affect Disord (2018) 227:752–8. doi: 10.1016/j.jad.2017.11.040
96. Savitz J, Drevets WC, Smith CM, Victor TA, Wurfel BE, Bellgowan PSF, et al. Putative Neuroprotective and Neurotoxic Kynurenine Pathway Metabolites are Associated With Hippocampal and Amygdalar Volumes in Subjects With Major Depressive Disorder. Neuropsychopharmacology (2015) 40(2):463–71. doi: 10.1038/npp.2014.194
97. Doolin K, Allers KA, Pleiner S, Liesener A, Farrell C, Tozzi L, et al. Altered Tryptophan Catabolite Concentrations in Major Depressive Disorder and Associated Changes in Hippocampal Subfield Volumes. Psychoneuroendocrinology (2018) 95:8–17. doi: 10.1016/j.psyneuen.2018.05.019
98. Poletti S, Myint AM, Schüetze G, Bollettini I, Mazza E, Grillitsch D, et al. Kynurenine Pathway and White Matter Microstructure in Bipolar Disorder. Eur Arch Psychiatry Clin Neurosci (2018) 268(2):157–68. doi: 10.1007/s00406-016-0731-4
99. De Picker L, Ottoy J, Verhaeghe J, Deleye S, Wyffels L, Fransen E, et al. State-Associated Changes in Longitudinal [F]-PBR111 TSPO PET Imaging of Psychosis Patients: Evidence for the Accelerated Ageing Hypothesis? Brain Behav Immun (2019) 77:46–54. doi: 10.1016/j.bbi.2018.11.318
100. Gabbay V, Liebes L, Katz Y, Liu S, Mendoza S, Babb JS, et al. The Kynurenine Pathway in Adolescent Depression: Preliminary Findings From a Proton MR Spectroscopy Study. Prog Neuropsychopharmacol Biol Psychiatry (2010) 34(1):37–44. doi: 10.1016/j.pnpbp.2009.09.015
101. Zhou Y, Zheng W, Liu W, Wang C, Zhan Y, Li H, et al. Antidepressant Effect of Repeated Ketamine Administration on Kynurenine Pathway Metabolites in Patients With Unipolar and Bipolar Depression [Internet]. Brain Behavior Immun (2018) 74:205–12. doi: 10.1016/j.bbi.2018.09.007
102. Guloksuz S, Arts B, Walter S, Drukker M, Rodriguez L, Myint A-M, et al. The Impact of Electroconvulsive Therapy on the Tryptophan-Kynurenine Metabolic Pathway. Brain Behav Immun (2015) 48:48–52. doi: 10.1016/j.bbi.2015.02.029
103. Tsuchiyagaito A, Smith JL, El-Sabbagh N, Zotev V, Misaki M, Al Zoubi O, et al. Real-Time fMRI Neurofeedback Amygdala Training may Influence Kynurenine Pathway Metabolism in Major Depressive Disorder. NeuroImage Clin (2021) 29:102559. doi: 10.1016/j.nicl.2021.102559
104. Sun Y, Drevets W, Turecki G, Li QS. The Relationship Between Plasma Serotonin and Kynurenine Pathway Metabolite Levels and the Treatment Response to Escitalopram and Desvenlafaxine. Brain Behav Immun (2020) 87:404–12. doi: 10.1016/j.bbi.2020.01.011
105. Møller SE, Honoré P, Larsen OB. Tryptophan and Tyrosine Ratios to Neutral Amino Acids in Endogenous Depression. Relation to Antidepressant Response to Amitriptyline and Lithium + L-Tryptophan. J Affect Disord (1983) 5(1):67–79. doi: 10.1016/0165-0327(83)90038-1
106. Brooks AK, Janda TM, Lawson MA, Rytych JL, Smith RA, Ocampo-Solis C, et al. Desipramine Decreases Expression of Human and Murine Indoleamine-2,3-Dioxygenases. Brain Behav Immun (2017) 62:219–29. doi: 10.1016/j.bbi.2017.02.010
107. Kim Y-K, Myint A-M, Verkerk R, Scharpe S, Steinbusch H, Leonard B. Cytokine Changes and Tryptophan Metabolites in Medication-Naïve and Medication-Free Schizophrenic Patients. Neuropsychobiology (2009) 59(2):123–9. doi: 10.1159/000213565
108. Myint AM, Schwarz MJ, Verkerk R, Mueller HH, Zach J, Scharpé S, et al. Reversal of Imbalance Between Kynurenic Acid and 3-Hydroxykynurenine by Antipsychotics in Medication-Naïve and Medication-Free Schizophrenic Patients. Brain Behav Immun (2011) 25(8):1576–81. doi: 10.1016/j.bbi.2011.05.005
109. Gál EM, Sherman AD. Synthesis and Metabolism of L-Kynurenine in Rat Brain. J Neurochem (1978) 30(3):607–13. doi: 10.1111/j.1471-4159.1978.tb07815.x
110. Pardridge WM. Kinetics of Competitive Inhibition of Neutral Amino Acid Transport Across the Blood-Brain Barrier. J Neurochem (1977) 28(1):103–8. doi: 10.1111/j.1471-4159.1977.tb07714.x
111. Boado RJ, Li JY, Nagaya M, Zhang C, Pardridge WM. Selective Expression of the Large Neutral Amino Acid Transporter at the Blood-Brain Barrier. Proc Natl Acad Sci U S A (1999) 96(21):12079–84. doi: 10.1073/pnas.96.21.12079
112. Sánchez del Pino MM, Peterson DR, Hawkins RA. Neutral Amino Acid Transport Characterization of Isolated Luminal and Abluminal Membranes of the Blood-Brain Barrier. J Biol Chem (1995) 270(25):14913–8. doi: 10.1074/jbc.270.25.14913
113. Albrecht J, Zielińska M. Exchange-Mode Glutamine Transport Across CNS Cell Membranes. Neuropharmacology (2019) 161:107560. doi: 10.1016/j.neuropharm.2019.03.003
114. Knudsen GM, Pettigrew KD, Patlak CS, Hertz MM, Paulson OB. Asymmetrical Transport of Amino Acids Across the Blood-Brain Barrier in Humans. J Cereb Blood Flow Metab (1990) 10(5):698–706. doi: 10.1038/jcbfm.1990.123
115. Nakatani Y, Sato-Suzuki I, Tsujino N, Nakasato A, Seki Y, Fumoto M, et al. Augmented Brain 5-HT Crosses the Blood-Brain Barrier Through the 5-HT Transporter in Rat. Eur J Neurosci (2008) 27(9):2466–72. doi: 10.1111/j.1460-9568.2008.06201.x
116. Kita T, Morrison PF, Heyes MP, Markey SP. Effects of Systemic and Central Nervous System Localized Inflammation on the Contributions of Metabolic Precursors to the L-Kynurenine and Quinolinic Acid Pools in Brain. J Neurochem (2002) 82(2):258–68. doi: 10.1046/j.1471-4159.2002.00955.x
117. Fukui S, Schwarcz R, Rapoport SI, Takada Y, Smith QR. Blood-Brain Barrier Transport of Kynurenines: Implications for Brain Synthesis and Metabolism. J Neurochem (1991) 56(6):2007–17. doi: 10.1111/j.1471-4159.1991.tb03460.x
118. Tang JP, Melethil S. Effect of Aging on the Kinetics of Blood-Brain Barrier Uptake of Tryptophan in Rats. Pharm Res (1995) 12(7):1085–91. doi: 10.1023/A:1016283003747
119. Speciale C, Schwarcz R. Uptake of Kynurenine Into Rat Brain Slices. J Neurochem (1990) 54(1):156–63. doi: 10.1111/j.1471-4159.1990.tb13296.x
120. Kitt TM, Spector R. Transport of Quinolinic Acid Into Rabbit and Rat Brain. Neurochem Res (1987) 12(7):625–8. doi: 10.1007/BF00971011
121. Foster AC, Miller LP, Oldendorf WH, Schwarcz R. Studies on the Disposition of Quinolinic Acid After Intracerebral or Systemic Administration in the Rat. Exp Neurol (1984) 84(2):428–40. doi: 10.1016/0014-4886(84)90239-5
122. Robotka H, Németh H, Somlai C, Vécsei L, Toldi J. Systemically Administered Glucosamine-Kynurenic Acid, But Not Pure Kynurenic Acid, is Effective in Decreasing the Evoked Activity in Area CA1 of the Rat Hippocampus. Eur J Pharmacol (2005) 513(1-2):75–80. doi: 10.1016/j.ejphar.2005.02.043
123. Savitz J. The Kynurenine Pathway: A Finger in Every Pie. Mol Psychiatry (2020) 25(1):131–47. doi: 10.1038/s41380-019-0414-4
124. Dickens D, Chiduza GN, Wright GSA, Pirmohamed M, Antonyuk SV, Hasnain SS. Modulation of LAT1 (SLC7A5) Transporter Activity and Stability by Membrane Cholesterol. Sci Rep (2017) 7:43580. doi: 10.1038/srep43580
125. Yao JK, van Kammen DP. Red Blood Cell Membrane Dynamics in Schizophrenia. I. Membrane Fluidity. Schizophr Res (1994) 11(3):209–16. doi: 10.1016/0920-9964(94)90014-0
126. Olusi SO, Fido AA. Serum Lipid Concentrations in Patients With Major Depressive Disorder. Biol Psychiatry (1996) 40(11):1128–31. doi: 10.1016/S0006-3223(95)00599-4
127. Parekh A, Smeeth D, Milner Y, Thuret S. The Role of Lipid Biomarkers in Major Depression [Internet]. Healthcare (2017) 5:5. doi: 10.3390/healthcare5010005
128. Sobczak S, Honig A, Christophe A, Maes M, Helsdingen RWC, De Vriese SA, et al. Lower High-Density Lipoprotein Cholesterol and Increased Omega-6 Polyunsaturated Fatty Acids in First-Degree Relatives of Bipolar Patients. Psychol Med (2004) 34(1):103–12. doi: 10.1017/S0033291703001090
129. Tang J-P, Xu Z-Q, Douglas FL, Rakhit A, Melethil S. Increased Blood-Brain Barrier Permeability of Amino Acids in Chronic Hypertension. Life Sci (1993) 53(25):PL417–20. doi: 10.1016/0024-3205(93)90033-Y
130. Tagliamonte A, DeMontis MG, Olianas M, Onali PL, Gessa GL. Possible Role of Insulin in the Transport of Tyrosine and Tryptophan From Blood to Brain. Adv Exp Med Biol (1976) 69:89–94. doi: 10.1007/978-1-4684-3264-0_7
131. Cunningham VJ, Hay L, Stoner HB. The Binding of L-Tryptophan to Serum Albumins in the Presence of Non-Esterified Fatty Acids. Biochem J (1975) 146(3):653–8. doi: 10.1042/bj1460653
132. Etinger A, Kumar SR, Ackley W, Soiefer L, Chun J, Singh P, et al. Correction: The Effect of Isohydric Hemodialysis on the Binding and Removal of Uremic Retention Solutes. PloS One (2018) 13(7):e0200980. doi: 10.1371/journal.pone.0200980
133. Cangiano C, Cardelli P, Peverini P, Giglio RM, Laviano A, Fava A, et al. Effect of Kynurenine on Tryptophan-Albumin Binding in Human Plasma. Adv Exp Med Biol (1999) 467:279–82. doi: 10.1007/978-1-4615-4709-9_35
134. Yuwiler A, Oldendorf WH, Geller E, Braun L. Effect of Albumin Binding and Amino Acid Competition on Tryptophan Uptake Into Brain. J Neurochem (1977) 28(5):1015–23. doi: 10.1111/j.1471-4159.1977.tb10664.x
135. Badawy AA-B, Guillemin G. The Plasma [Kynurenine]/[Tryptophan] Ratio and Indoleamine 2,3-Dioxygenase: Time for Appraisal. Int J Tryptophan Res (2019) 12:1178646919868978. doi: 10.1177/1178646919868978
136. Bloxam DL, Tricklebank MD, Patel AJ, Curzon G. Effects of Albumin, Amino Acids, and Clofibrate on the Uptake of Tryptophan by the Rat Brain. J Neurochem (1980) 34(1):43–9. doi: 10.1111/j.1471-4159.1980.tb04619.x
137. Pardridge WM. Tryptophan Transport Through the Blood-Brain Barrier: In Vivo Measurement of Free and Albumin-Bound Amino Acid. Life Sci (1979) 25(17):1519–28. doi: 10.1016/0024-3205(79)90378-3
138. Straus DS, Marten NW, Hayden JM, Burke EJ. Protein Restriction Specifically Decreases the Abundance of Serum Albumin and Transthyretin Nuclear Transcripts in Rat Liver. J Nutr (1994) 124(7):1041–51. doi: 10.1093/jn/124.7.1041
139. Yang F, Zhang Y, Liang H. Interactive Association of Drugs Binding to Human Serum Albumin. Int J Mol Sci (2014) 15(3):3580–95. doi: 10.3390/ijms15033580
140. Blomstrand E, Celsing F, Newsholme EA. Changes in Plasma Concentrations of Aromatic and Branched-Chain Amino Acids During Sustained Exercise in Man and Their Possible Role in Fatigue. Acta Physiol Scand (1988) 133(1):115–21. doi: 10.1111/j.1748-1716.1988.tb08388.x
141. Małkiewicz MA, Szarmach A, Sabisz A, Cubała WJ, Szurowska E, Winklewski PJ. Blood-Brain Barrier Permeability and Physical Exercise. J Neuroinflamm (2019) 16(1):15. doi: 10.1186/s12974-019-1403-x
142. Gross PM, Weindl A. Peering Through the Windows of the Brain. J Cereb Blood Flow Metab (1987) 7(6):663–72. doi: 10.1038/jcbfm.1987.120
143. Guillemin GJ, Smith DG, Smythe GA, Armati PJ, Brew BJ. Expression of the Kynurenine Pathway Enzymes in Human Microglia and Macrophages. Adv Exp Med Biol (2003) 527:105–12. doi: 10.1007/978-1-4615-0135-0_12
144. Basile AS, Saito K, al-Mardini H, Record CO, Hughes RD, Harrison P, et al. The Relationship Between Plasma and Brain Quinolinic Acid Levels and the Severity of Hepatic Encephalopathy. Gastroenterology (1995) 108(3):818–23. doi: 10.1016/0016-5085(95)90456-5
145. Morrison PF, Morishige GM, Beagles KE, Heyes MP. Quinolinic Acid is Extruded From the Brain by a Probenecid-Sensitive Carrier System: A Quantitative Analysis. J Neurochem (1999) 72(5):2135–44. doi: 10.1046/j.1471-4159.1999.0722135.x
146. Bechter K, Reiber H, Herzog S, Fuchs D, Tumani H, Maxeiner HG. Cerebrospinal Fluid Analysis in Affective and Schizophrenic Spectrum Disorders: Identification of Subgroups With Immune Responses and Blood-CSF Barrier Dysfunction. J Psychiatr Res (2010) 44(5):321–30. doi: 10.1016/j.jpsychires.2009.08.008
147. Müller N, Ackenheil M. Immunoglobulin and Albumin Content of Cerebrospinal Fluid in Schizophrenic Patients: Relationship to Negative Symptomatology. Schizophr Res (1995) 14(3):223–8. doi: 10.1016/0920-9964(94)00045-A
148. Cai HQ, Catts VS, Webster MJ, Galletly C, Liu D, O’Donnell M, et al. Increased Macrophages and Changed Brain Endothelial Cell Gene Expression in the Frontal Cortex of People With Schizophrenia Displaying Inflammation. Mol Psychiatry [Internet] (2018) 25:761–75. doi: 10.1038/s41380-018-0235-x
149. Braidy N, Grant R, Adams S, Brew BJ, Guillemin GJ. Mechanism for Quinolinic Acid Cytotoxicity in Human Astrocytes and Neurons [Internet]. Neurotoxicity Res (2009) 16:77–86. doi: 10.1007/s12640-009-9051-z
150. Reynolds DS, Morton AJ. Changes in Blood-Brain Barrier Permeability Following Neurotoxic Lesions of Rat Brain can be Visualised With Trypan Blue. J Neurosci Methods (1998) 79(1):115–21. doi: 10.1016/S0165-0270(97)00168-4
151. Oláh G, Herédi J, Menyhárt A, Czinege Z, Nagy D, Fuzik J, et al. Unexpected Effects of Peripherally Administered Kynurenic Acid on Cortical Spreading Depression and Related Blood-Brain Barrier Permeability. Drug Des Devel Ther (2013) :7:981–7. doi: 10.2147/DDDT.S44496
152. Sawchenko PE, Brown ER, Chan RK, Ericsson A, Li HY, Roland BL, et al. The Paraventricular Nucleus of the Hypothalamus and the Functional Neuroanatomy of Visceromotor Responses to Stress. Prog Brain Res (1996) 107:201–22. doi: 10.1016/S0079-6123(08)61866-X
153. Herman JP, Cullinan WE. Neurocircuitry of Stress: Central Control of the Hypothalamo-Pituitary-Adrenocortical Axis. Trends Neurosci (1997) 20(2):78–84. doi: 10.1016/S0166-2236(96)10069-2
154. Agarwal SK, Marshall GD Jr. Dexamethasone Promotes Type 2 Cytokine Production Primarily Through Inhibition of Type 1 Cytokines. J Interferon Cytokine Res (2001) 21(3):147–55. doi: 10.1089/107999001750133159
155. Perrin AJ, Horowitz MA, Roelofs J, Zunszain PA, Pariante CM. Glucocorticoid Resistance: Is It a Requisite for Increased Cytokine Production in Depression? A Systematic Review and Meta-Analysis. Front Psychiatry (2019) 10:423. doi: 10.3389/fpsyt.2019.00423
156. Lee M, Jayathilake K, Dai J, Meltzer HY. Decreased Plasma Tryptophan and Tryptophan/Large Neutral Amino Acid Ratio in Patients With Neuroleptic-Resistant Schizophrenia: Relationship to Plasma Cortisol Concentration. Psychiatry Res (2011) 185(3):328–33. doi: 10.1016/j.psychres.2010.07.013
157. Joseph MS, Brewerton TD, Reus VI, Stebbins GT. Plasma L-Tryptophan/Neutral Amino Acid Ratio and Dexamethasone Suppression in Depression. Psychiatry Res (1984) 11(3):185–92. doi: 10.1016/0165-1781(84)90067-2
158. Maes M, Vandewoude M, Schotte C, Maes L, Martin M, Scharpe S, et al. The Relationships Between the Cortisol Responses to Dexamethasone and to L-5-HTP, and the Availability of L-Tryptophan in Depressed Females. Biol Psychiatry (1990) 27(6):601–8. doi: 10.1016/0006-3223(90)90527-9
159. Maes M, Jacobs MP, Suy E, Minner B, Leclercq C, Christiaens F, et al. Suppressant Effects of Dexamethasone on the Availability of Plasma L-Tryptophan and Tyrosine in Healthy Controls and in Depressed Patients. Acta Psychiatr Scand (1990) 81(1):19–23. doi: 10.1111/j.1600-0447.1990.tb06443.x
160. Vezzani A, Gramsbergen JB, Speciale C, Schwarcz R. Production of Quinolinic Acid and Kynurenic Acid by Human Glioma. Adv Exp Med Biol (1991) 294:691–5. doi: 10.1007/978-1-4684-5952-4_95
161. Struder HK, Hollmann W, Platen P, Duperly J, Fischer HG, Weber K. Alterations in Plasma Free Tryptophan and Large Neutral Amino Acids do Not Affect Perceived Exertion and Prolactin During 90 Min of Treadmill Exercise. Int J Sports Med (1996) 17(2):73–9. doi: 10.1055/s-2007-972811
162. Agudelo LZ, Femenía T, Orhan F, Porsmyr-Palmertz M, Goiny M, Martinez-Redondo V, et al. Skeletal Muscle PGC-1α1 Modulates Kynurenine Metabolism and Mediates Resilience to Stress-Induced Depression. Cell (2014) 159(1):33–45. doi: 10.1016/j.cell.2014.07.051
163. Gao J, Xu K, Liu H, Liu G, Bai M, Peng C, et al. Impact of the Gut Microbiota on Intestinal Immunity Mediated by Tryptophan Metabolism [Internet]. Front Cell Infection Microbiol (2018) 8:1–22. doi: 10.3389/fcimb.2018.00013
164. Morrens M, Coppens V, Walther S. Do Immune Dysregulations and Oxidative Damage Drive Mood and Psychotic Disorders? Neuropsychobiology (2019) 79:1–4. doi: 10.1159/000496622
165. Shivakumar V, Kalmady SV, Venkatasubramanian G, Ravi V, Gangadhar BN. Do Schizophrenia Patients Age Early? [Internet]. Asian J Psychiatry (2014) 10:3–9. doi: 10.1016/j.ajp.2014.02.007
166. Secci ME, Mascia P, Sagheddu C, Beggiato S, Melis M, Borelli AC, et al. Astrocytic Mechanisms Involving Kynurenic Acid Control Δ-Tetrahydrocannabinol-Induced Increases in Glutamate Release in Brain Reward-Processing Areas. Mol Neurobiol (2019) 56(5):3563–75. doi: 10.1007/s12035-018-1319-y
167. Brainstorm Consortium, Anttila V, Bulik-Sullivan B, Finucane HK, Walters RK, Bras J, et al. Analysis of Shared Heritability in Common Disorders of the Brain. Sci [Internet] (2018) 360(6395):209–23. doi: 10.1126/science.aap8757
168. Clark SM, Pocivavsek A, Nicholson JD, Notarangelo FM, Langenberg P, McMahon RP, et al. Reduced Kynurenine Pathway Metabolism and Cytokine Expression in the Prefrontal Cortex of Depressed Individuals. J Psychiatry Neurosci (2016) 41(6):386–94. doi: 10.1503/jpn.150226
169. Wonodi I, Stine OC, Sathyasaikumar KV, Roberts RC, Mitchell BD, Hong LE, et al. Downregulated Kynurenine 3-Monooxygenase Gene Expression and Enzyme Activity in Schizophrenia and Genetic Association With Schizophrenia Endophenotypes. Arch Gen Psychiatry (2011) 68(7):665–74. doi: 10.1001/archgenpsychiatry.2011.71
170. Sathyasaikumar KV, Stachowski EK, Wonodi I, Roberts RC, Rassoulpour A, McMahon RP, et al. Impaired Kynurenine Pathway Metabolism in the Prefrontal Cortex of Individuals With Schizophrenia. Schizophr Bull (2011) 37(6):1147–56. doi: 10.1093/schbul/sbq112
171. Young S. Bioanalytical Inaccuracy: A Threat to the Integrity and Efficiency of Research [Internet]. J Psychiatry Neurosci (2010) 35:3–6. doi: 10.1503/jpn.090171
Keywords: kynurenine, blood-brain barrier, immune, tryptophan, psychiatry, inflammation, CSF
Citation: Skorobogatov K, De Picker L, Verkerk R, Coppens V, Leboyer M, Müller N and Morrens M (2021) Brain Versus Blood: A Systematic Review on the Concordance Between Peripheral and Central Kynurenine Pathway Measures in Psychiatric Disorders. Front. Immunol. 12:716980. doi: 10.3389/fimmu.2021.716980
Received: 29 May 2021; Accepted: 08 September 2021;
Published: 23 September 2021.
Edited by:
Yvette Mándi, University of Szeged, HungaryReviewed by:
Imola Wilhelm, Biological Research Centre, HungaryJanos Tajti, University of Szeged, Hungary
Copyright © 2021 Skorobogatov, De Picker, Verkerk, Coppens, Leboyer, Müller and Morrens. This is an open-access article distributed under the terms of the Creative Commons Attribution License (CC BY). The use, distribution or reproduction in other forums is permitted, provided the original author(s) and the copyright owner(s) are credited and that the original publication in this journal is cited, in accordance with accepted academic practice. No use, distribution or reproduction is permitted which does not comply with these terms.
*Correspondence: Katrien Skorobogatov, a2F0cmllbi5za29yb2JvZ2F0b3ZAZW1tYXVzLmJl