- 1Department of Pediatric Respiratory Medicine, Immunology and Critical Care Medicine, Charité – Universitätsmedizin Berlin, Corporate Member of Freie Universität Berlin and Humboldt-Universität zu Berlin, Berlin, Germany
- 2Berlin Institute of Health (BIH) at Charité – Universitätsmedizin Berlin, BIH-Center for Regenerative Therapies (BCRT), Berlin, Germany
- 3Berlin Center for Advanced Therapies (BeCAT), Charité - Universitätsmedizin Berlin, Corporate Member of Freie Universität Berlin and Humboldt-Universität zu Berlin, Berlin, Germany
- 4Institute of Medical Immunology, Charité – Universitätsmedizin Berlin, Corporate Member of Freie Universität Berlin and Humboldt-Universität zu Berlin, Berlin, Germany
- 5Department of Veterinary Medicine, Institute of Veterinary Pathology, Freie Universität Berlin, Berlin, Germany
- 6Department of Nephrology and Internal Intensive Care Medicine, Charité – Universitätsmedizin Berlin, Corporate Member of Freie Universität Berlin and Humboldt-Universität zu Berlin, Berlin, Germany
Reshaping the immune balance by adoptive transfer of regulatory T-cells (Tregs) has emerged as a promising strategy to combat undesired immune reactions, including in Graft-versus-Host Disease (GvHD), which is the most lethal non-relapse complication of allogeneic hematopoietic stem cell transplantation. Currently however, little is known about the potentially inhibitory in vivo effects of conventional immunosuppressive drugs, which are routinely used to treat GvHD, on adoptively transferred Tregs. Here we demonstrate drug-specific effects of the conventional immunosuppressive drugs Cyclosporine A, Mycophenolate mofetil and methylprednisolone on adoptively transferred Tregs in a humanized NOD/SCID/IL2Rgamma-/- GvHD mouse model. The clinical course of GvHD and postmortem organ histology, including cellular organ infiltration, showed that co-administration of Cyclosporine A and Tregs is highly beneficial as it enhanced Treg accumulation at inflammatory sites like lung and liver. Similarly, co-administration of Mycophenolate mofetil and Tregs improved clinical signs of GvHD. In contrast, co-administration of methylprednisolone and Tregs resulted in reduced Treg recruitment to inflammatory sites and the fast deterioration of some animals. Consequently, when clinical trials investigating safety and efficacy of adjunctive Treg therapy in GvHD are designed, we suggest co-administering Cyclosporine A, whereas high doses of glucocorticosteroids should be avoided.
Introduction
Allogeneic hematopoietic stem cell transplantation (HSCT) is a curative treatment option for many patients with malignant and non-malignant diseases of the hematopoietic system. Graft-versus Host-Disease (GvHD) however, still constitutes one of the most lethal post-transplant complications of HSCT (1). While pharmacologic immunosuppression has dramatically improved short-term outcomes, long-term morbidity and mortality is still poor in patients suffering from GvHD. Despite the beneficial factor of graft-versus leukemia effects in patients with malignant underlying disease, transplantation related mortality rises from 5-13% in patients without GvHD to 51-75% in patients suffering from any form of GvHD (2). The current paradigm holds that GvHD is primarily a T-cell mediated disease caused by donor effector-T-lymphocytes recognizing allogenic structures as non-self in the host, and thereby eliciting their proinflammatory and cytolytic program. Our understanding of immunoregulatory mechanisms profoundly advanced with the identification of thymic derived regulatory T-cells (tTregs) as physiologic counterplayers of effector T-cells and critical mediators of immune homeostasis after T-cell activation by self- and non-self structures (3). tTregs are characterized by high expression of CD25 and the transcription factor FoxP3, and characteristically low expression of CD127. All three proteins are established as markers for Treg diagnostics. A numeric loss of tTregs is associated not only with a higher incidence and severity of GvHD (4–8) but also with autoimmune diseases (9–11). In contrast, therapeutic benefits can be achieved by enrichment of circulating tTregs after HSCT (12–19) and in the context of solid organ transplantation (20–22) and autoimmune diseases such as hepatitis C vasculitis (23), systemic lupus erythematosus or Type 1 diabetes (24–26).
Recent technological developments have enabled the purification and ex vivo expansion of Tregs on a large scale and under clinical grade conditions. This has allowed the translation of adoptive tTreg transfer from animal models to clinical protocols. In order to investigate safety and efficacy, Tregs will have to be administered as adjunctive therapy in addition to current state of the art protocols in early clinical trial phases. Therefore, in the context of HSCT, Treg transfer will have to be combined with the conventional immunosuppressive drugs (cISD) Cyclosporine A (CSA), mycophenolate mofetil (MMF) and prednisolone or methylprednisolone (MP) as components of prophylactic as well as first-, second- and third-line treatment regimens.
The question of “if” and “how” cISD influence the function and frequency of tTregs in vivo has previously been addressed, but some of the data are contradictory. Furthermore, there is little data available describing the effects of these drugs on the in vivo fate of ex vivo manipulated, adoptively transferred tTregs (27). Direct comparison of individual cISD in a clinical setting is difficult, since standard immunosuppressive regimens in HSCT but also for solid organ transplantation are comprised of a combination of at least two agents. Therefore, most data investigating individual drug-specific effects were generated by in vitro or animal experiments. The prevailing opinion assigns a beneficial effect of mTOR (Rapamycin, Everolimus) (28) and histone deacetylase inhibition (29, 30) but also low-dose Interleukin-2 (18, 23, 31–36) and anti-thymocyte globulin (37, 38) to tTreg maturation, function and survival. In contrast, the calcineurin inhibitors (CNI) CSA and Tacrolimus (20, 27, 28, 39–48), and blockage of CTLA-4 (49) and Interleukin-2 (50) seems to hamper both tTreg development and function. Even less consistent data exists on the effect of complement inhibitors, steroids (51–56) and MMF (43, 57). The effect of cISD on ex vivo expanded and adoptively transferred tTregs are just now starting to receive attention, however, study designs and results are diverse.
Therefore, the aim of this study was to determine the drug-specific influence of the standard prophylactic and therapeutic first-, second- and third-line drugs, CSA, MMF and MP used in GvHD therapy, on adoptively transferred Tregs. In order to investigate the pharmacological effects of cISD at the cellular level in vivo, as well as to obtain a deeper understanding of beneficial combinations of cISD with adjunctive Treg therapy in the clinical setting of GvHD, a humanized GvHD mouse model was employed. Insights obtained from this work will pave the way for rational design of prospective Phase I and II clinical trials investigating safety and efficacy of adjunctive Treg therapy in patients with acute and chronic GvHD.
Materials and Methods
Cell Isolation
Peripheral blood mononuclear cells (PBMC) were isolated from freshly collected blood of healthy volunteers or from buffy coats by Ficoll density-gradient centrifugation (PAA Laboratories, Pasching, Austria). Treg isolation was performed using the CD4+CD25+ Treg isolation kit (Miltenyi Biotech, Bergisch Gladbach, Germany) according to the manufacturer’s recommendations. This study was approved by the Charité-Universitätsmedizin Berlin ethics committee (Institutional Review Board) and all blood donors provided written informed consent.
Treg Expansion
Freshly isolated Tregs were polyclonally expanded as described previously (13). Briefly, Tregs were cultured in X-VIVO15 medium (Lonza, Basel, Switzerland) containing 10% serum, 500 U/mL human recombinant IL-2 (Chiron Behring, Marburg, Germany) and 100 nM rapamycin (Sigma–Aldrich, St. Louis, MO) and stimulated with CD3/CD28 Treg expansion beads (Miltenyi Biotech). Cells were restimulated every 3-4 days. After 3-weeks expansion time tTreg product purity was assessed by specific staining of CD3-APC750 (Clone UCHT1), CD4-APC (Clone 13B8.2), CD8-A700 (Clone B9.11), CD25-PeCy7 (Clone B1.49.9) from Beckman Coulter, Krefeld Germany) and FoxP3-A488 (Clone 259D/C7, Beckton Dickinson, Heidelberg, Germany). Mean Treg purity after isolation was 80% for CD3+CD4+ and 60% for CD3+CD4+CD25high cells. After isolation Tregs were expanded in cell culture medium supplemented with IL-2 and rapamycin in order to promote Treg expansion and suppress expansion of non-Treg cells. The final Treg products consisted of ~90% CD3+CD4+ T-cells with a frequency of 94% CD25highFoxP3+ Tregs.
Humanized Animal Experiments for Xenogenic GVHD
The adult offspring of NOD/SCID/IL-2Rgamma-/- mice, originally purchased from Charles River Laboratories (Research Models and Services, Sulzfeld, Germany), were used according to the approval by the Landesamt für Gesundheit und Soziales Berlin (G0483/09). Mice were maintained under specific pathogen-free conditions. Xenogenic GvHD was induced, after sublethal radiation (300 cGy), by single intravenous injection of 3x106 human PBMCs/mouse. Ex vivo expanded human tTregs, that originate from the same donor as the PBMCs used to induce GvHD, were administered i.v. at a single dose of 1.5x106, 3x106, or 6x106, equivalent to a PBMC:tTreg ratio of 2:1, 1:1 and 1:2. Additionally 1.5x106 tTregs were combined with each of the cISD. Conventional immunosuppressive therapy was administered daily from day+1 until mice were sacrificed on day+35 or sacrificed due to progressive disease: Cyclosporine A 4 mg/kg s.c. (Novartis Pharma GmbH, Nürnberg, Germany), Mycophenolate Mofetil 0.5 mg p.o. (MMF, CellCept® 1g/5ml Roche Pharma, Grenzach-Wyhlen Germany), Methylprednisolone 20 mg/kg/d i.p. daily (MP, Urbason ® solubile 32 mg Sanofi Aventis Deutschland GmbH, Frankfurt am Main, Germany). cISD dose finding was based on our own experience (20) and previously published data (58–60) in order to mimic the clinical situation with serum levels similar to target levels used in human patients. During the experimental setup we aimed for equal distribution of male and female animals in all groups (Supplementary Figure S1). The first group included mice that received no form of therapy after the PBMC administration. To further control for the effect of tTregs alone, tTregs were administered to a second control group without prior administration of PBMCs. Animals were monitored daily for body weight and clinical signs of GvHD (behavior, fur alterations, skin inflammation, hunched back, Supplementary Table ST1) and scored as either no GvHD (0); mild GvHD (1 – 1.5), moderate GvHD (2 – 2.5) or severe GvHD (3). Livers, lungs, spleens and intestines were collected on day+35 or when clinical signs required euthanasia. Euthanasia due to a poor clinical condition was performed when body weight decreased by >20% of initial body weight or at a clinical score of 3. Details of experimental setup are depicted in Figure 1 and days of analysis further listed in Table 1.
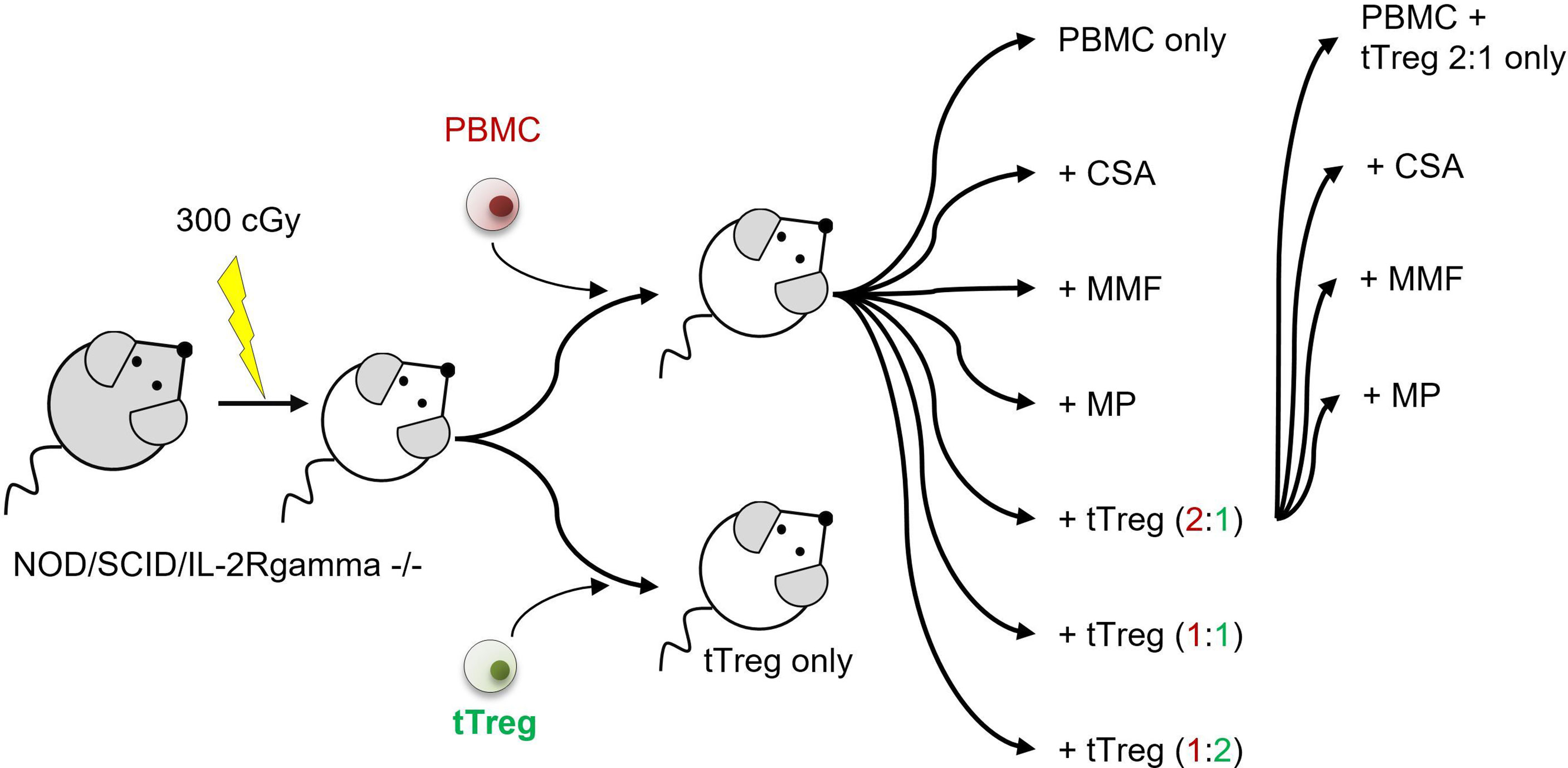
Figure 1 Experimental setup. NOD/SCID/IL-2Rgamma-/- mice were sub-lethally radiated with 300 cGy (day -1). Xenogenic GvHD was induced by intravenous application of 3x106 human PBMCs. GvHD control mice did not receive further therapy. tTreg control mice received 3x106 ex vivo expanded tTregs. If indicated tTregs were simultaneously administered with PBMCs at a dosage of 1.5x106 (PBMC:tTreg ration of 2:1), 3x106 (PBMC:tTreg ratio of 1:1) or 6x106 (PBMC:tTreg ratio of 1:2). Mice that received either PBMCs alone or in combination with tTregs were further treated daily with the cISD CSA (4 mg/kg/d s.c.), MMF (0.5 mg/d p.o) or MP (20 mg/kg/d i.p.) starting day +1 after cell administration. Animals were monitored daily for body weight and clinical signs of GvHD (behavior, fur alterations, skin inflammation, hunched back). Liver, spleen, and lungs were collected on day +35 or when clinical signs required euthanasia.
Organ Flow-Cytometry
After animals were sacrificed their organs were collected, divided and either fixed in 5% formalin for histologic analysis or resuspended in saline for direct organ flow cytometry. To isolate cells for FACS analysis, organs were mechanically disrupted and further digested by DNAse and collagenase (Sigma Aldrich, Germany). Subsequently, erythrocytes were lysed and remaining cells were washed in PBS and culture medium. Lung sample cells were flushed through 100 µm and 70 µm pore sized filters before staining. Hepatic samples were centrifuged at 50 xg for 3 minutes after cellular disruption and collagen digestion. Most hepatocytes sedimented whilst lymphocytes remained in the supernatant. The supernatant was collected and spun at 200xg for 2 minutes. The lymphocytes sedimented and were subsequently collected. Lymphocytes were additionally enriched by density centrifugation using Lymphocyte Separation Medium (LSM1077, PAA Laboratories, Pasching, Austria) before erythrocytes were lysed and cells subjected to the routine staining procedure. Antibodies were titrated to establish optimal staining conditions before use: CD3-APC-A750 (Clone UCHT1), CD4-APC (Clone 13B8.2), CD8-APC-A700 (Clone B9.11), CD19-ECD (Clone J3-119), CD25-PeCy7 (Clone B1.49.9), CD45-PacificBlue (Clone J33), CD56-PE (Clone N901), CD127-APC-A700 (Clone R34.34) from Beckman Coulter, Krefeld Germany; CD45 mouse-PerCP-Cy5.5 (Clone 30-F11, Biolegend, Germany); L/D PacificOrange (Life Technologies GmbH, Darmstadt, Germany); FoxP3-A488 (Clone 259D/c7), Ki67-PerCP-Cy5.5 (Clone B56) from Beckton Dickinson, Heidelberg, Germany. Flow cytometry was performed using Beckman Coulter NAVIOS cytometer. Data were analyzed using Kaluza® software (Beckman Coulter). For staining, up to 2x106 cells were suspended in 100 µl FACS buffer and unspecific Fc receptors were blocked using FcR blocking reagent (Miltenyi Biotech GmbH, Germany). Extracellular staining was performed as indicated. Cells were fixed and permeabilized using FoxP3 staining buffer set purchased from eBioscience, San Diego. Subsequently, cells were intracellularly stained, washed and analyzed by flow cytometry.
Histology
Tissue samples of liver, lung, spleen, and kidney were fixed in 5% formalin, embedded in paraffin, and cut into 5 µm-thick slices. Slices were dewaxed and stained with hematoxylin/eosin. For microscopy an Olympus BX41 (Olympus, Hamburg, Germany) microscope with a 20-fold magnification lens was used. The grade of infiltration of human cells was calculated based on a semi-quantitative scoring system. The scoring system ranged from 0 (no); 1 (mild); 2 (moderate) up to 3 (massive) infiltration by inflammatory cells.
Statistics
Unless stated otherwise, data are presented as scatter plots showing individual values and means ± SEM. Statistical significance was determined by Kaplan-Meier analysis and Log-rank test (Mantel-Cox-Test) or One-Way-ANOVA with Tukey correction. Significance levels are shown as indicated in the legends.
Data Sharing
Most of the data are presented in the manuscript text, figures, and legends. Any additional data will be made available upon request by the corresponding author (sybille.landwehr-kenzel(at)charite.de).
Results
Co-Administration of CSA or MMF With Adoptive Treg Transfer Improves the Clinical Course of GvHD
To investigate the clinical effect of conventional immunosuppressive drugs on adjunctive tTreg therapy, GvHD was induced in NOD/SCID/IL-2Rgamma-/- mice by infusion of 3x106 human peripheral blood mononuclear cells (PBMCs) (Figure 1, Table 1 and Figures 2A–M). Severe GvHD, substantial weight loss and a higher GvHD score were observed in all mice (Figures 2A, B, J). The timing of clinical GvHD manifestation resembled the clinical course known from human HSCT, with disease onset usually occurring during the first 15 days after transplantation. Mice showed signs of GvHD such as hunched posture, scurfy hair and overall reduced general condition. One control group received only tTregs in the absence of PBMCs (Figures 2A, B). These mice did not show clinical signs of GvHD (Figures 2A, B). Following PBMC-mediated GvHD induction mice were treated once with escalating doses of ex vivo expanded tTregs in the absence of immunosuppressive drugs. Remarkably, monotherapy with tTregs led to a statistically significant dose-dependent reduction in weight loss (Figure 2A) and reduced GvHD activity (Figure 2J). In line, overall survival was significantly improved by higher doses of tTregs (Figures 2B, I, J, K–M and Table 1). Alternatively, mice were treated daily with the cISD CSA (4 mg/kg s.c.), MMF (0.5 mg p.o.) or Methylprednisolone (MP, 20 mg/kg i.p.) (Figures 2C, D). cISD dosages were based on previously published data in order to reach target serum levels similar to target trough levels in the clinical setting (20, 58–60). To investigate the effects of co-administered cISD on adoptively transferred tTregs, the lowest tTreg dose (PBMC: tTreg = 2:1) was combined with CSA, MMF or MP (Figures 2A–J). Mice that received any of the cISD alone suffered from substantial weight loss and in case of MMF monotherapy, showed partially reduced survival (Figures 2C, D, I, J and Supplementary Figure S2). Despite their poor clinical condition, some mice receiving CSA or MMF alone did not fulfill the endpoint criteria for euthanasia (Figures 2D, I, J). Their prolonged survival was associated with a lower clinical GvHD score (Figures 2I, J). CSA or MMF administration in addition to tTreg therapy, substantially improved the clinical course of GvHD (Figures 2E, F, H–J and Table 1). In contrast, co-administration of tTreg and MP was associated with fast deterioration of all animals and a poor clinical outcome (Figures 2G, H-J). Most importantly, combined therapy with tTreg/CSA was clinically more effective than any other treatment approaches investigated (Figures 2A–J and Supplementary Figure S2), resulting in minimal GvHD activity (Figure 2H) and 100% survival (Figures 2H, I). In summary, tTreg monotherapy ameliorated the clinical signs of GvHD and improved overall survival, whilst co-administration of CSA or MMF in addition to tTregs significantly improved the clinical outcome, even when combined with the lowest investigated tTregs dose.
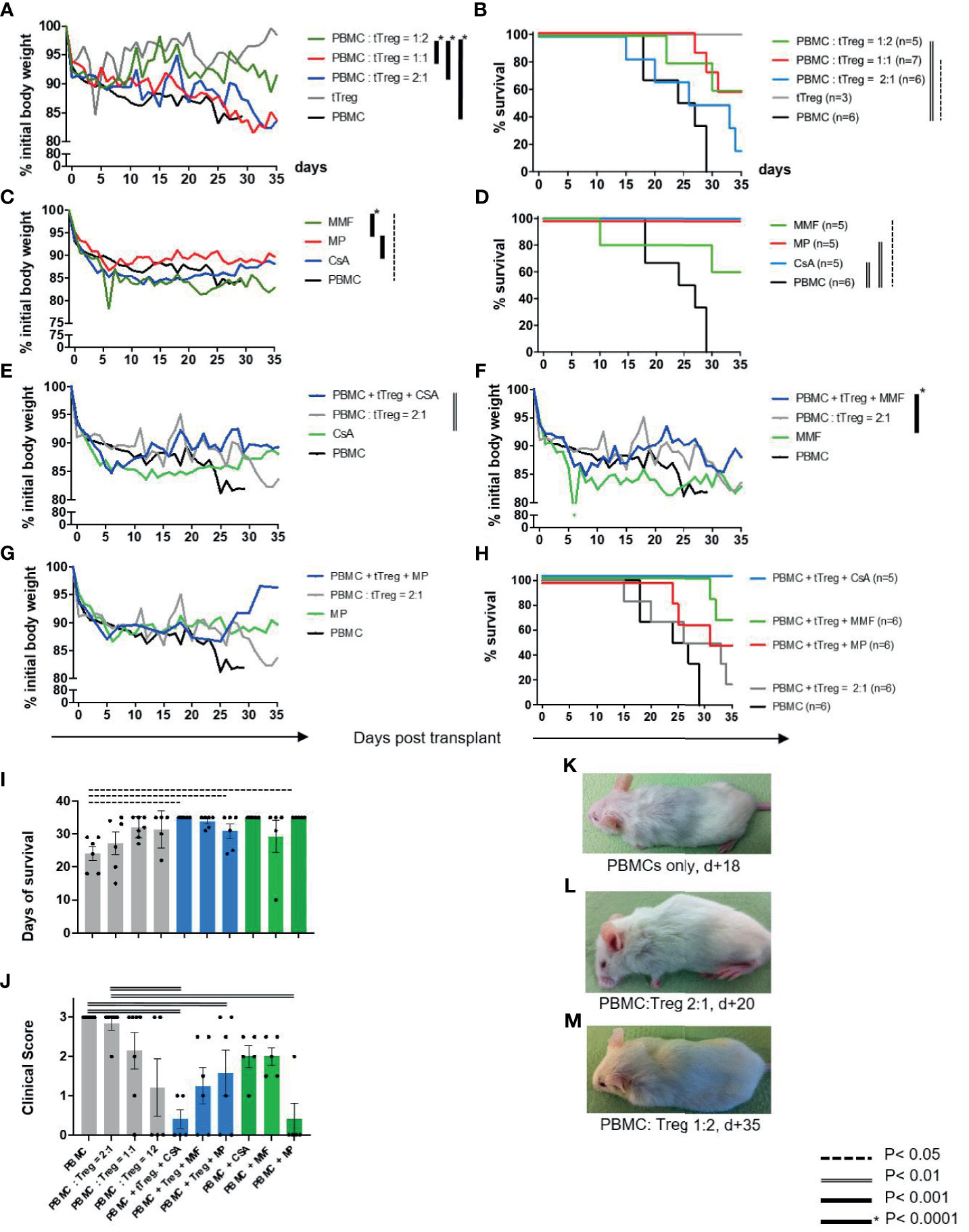
Figure 2 Clinical course and survival rates. As described in Figure 1 GvHD was induced in NOD/SCID/IL-2Rgamma -/- mice by infusion of 3x106 human PBMC [GvHD control, (A–M)]. tTreg control mice received ex vivo expanded human tTreg alone (3x106) but no PBMCs [tTreg, (A, B)]. (A, B) Mice were treated with escalating doses of ex vivo expanded tTregs at a ratio of 2:1 (n=6), 1:1 (n=7) or 1:2 (n=5). (C, D) Alternatively, mice were treated with the cISD CSA (4 mg/kg s.c.), MMF (0.5 mg p. o.) or MP (20 mg/kg i.p.) (n=5) alone. (E–H) After GvHD induction by PBMCs mice were treated with tTregs in combination with CSA (E, H), MMF (F, H) or MP (G, H). (J) Clinical assessment further included clinical signs of GvHD (behavior, fur alterations, skin inflammation, hunched back, Supplementary Table ST1) and was quantified as either no GvHD (0); mild GvHD (1 – 1.5), moderate GvHD (2 – 2.5) or severe GvHD (3). (K–M) Representative images demonstrating the clinical condition of mice treated with no [(K), d+18], low-dose [(L), d+20] or high dose [(M), d+35] tTregs are shown. Depicted are mean weight curves as percentage of initial body weight (A, C, E–G) and Kaplan-Meier-Survival curves (B, D, H). Individual body weight values are depicted in the Supplementary Figure S2. To support survival curves, days of survival are further listed in Table 1. Statistical analysis of weight curves was performed as One-Way-ANOVA with Tukey correction for multiple comparison. Survival data were statistically analyzed using Log-Rank (Mantel-Cox) test. CSA, Cyclosporine A; MMF, Mycophenolate Mofetil; MP, Methylprednisolone.
Combined Treatment of tTreg Therapy With CSA Reduces Lymphocytic Infiltration and Tissue Inflammation
Histopathologic organ morphology was assessed at the time of euthanasia in liver, lungs (Figure 3) and the intestine by microscopic analysis of tissue architecture, lymphocyte infiltration and signs of acute inflammation (Figures 3A–Q). To histologically semi-quantify these results, human CD3+ cells were stained and quantified in all samples of (P) livers and (Q) lungs. In line with previously published data (61–63) indicating that the lack of chemotherapeutic conditioning protects from intestinal GvHD, we did not find intestinal infiltrates or alternative signs of GvHD (Supplementary Figure S3). Accordingly, intestinal samples were not further analyzed here. In contrast, both the liver and lungs of animals that received PBMCs but not GvHD therapy showed moderate to high grade signs of GvHD (Figures 3A, B). In particular, we found hepatic bile duct damage with dense lymphocyte infiltration, inflammation and lymphocyte ballooning (Figure 3A). Lung pathology showed a significant loss of alveolar tissue architecture and was dominated by massive intra-alveolar and intraepithelial lymphocyte infiltration with inflammation of the pulmonary epithelium and apoptotic bodies (Figure 3B). Co-administration of CSA and tTregs (Figures 3D, F), and to a slightly lesser extent CSA monotherapy (Figures 3C, E), significantly reduced the histopathological grade of GvHD (Figures 3P, Q). This effect was characterized by preserved tissue architecture, mild lymphocyte infiltration and almost complete resolution of inflammation in both the liver and lung. Both MMF and MP administered as monotherapy or combined with tTregs only partially reduced lymphocyte infiltration but ameliorated signs of inflammation and largely preserved organ architecture (Figures 3G–K). In summary, therapy with both MMF and MP alone or in combination with tTregs was inferior to CSA and CSA/tTreg therapy. Accordingly, the beneficial effect of tTreg therapy on clinical parameters as described in Figure 2 correlated with reduced lymphocytic organ infiltration.
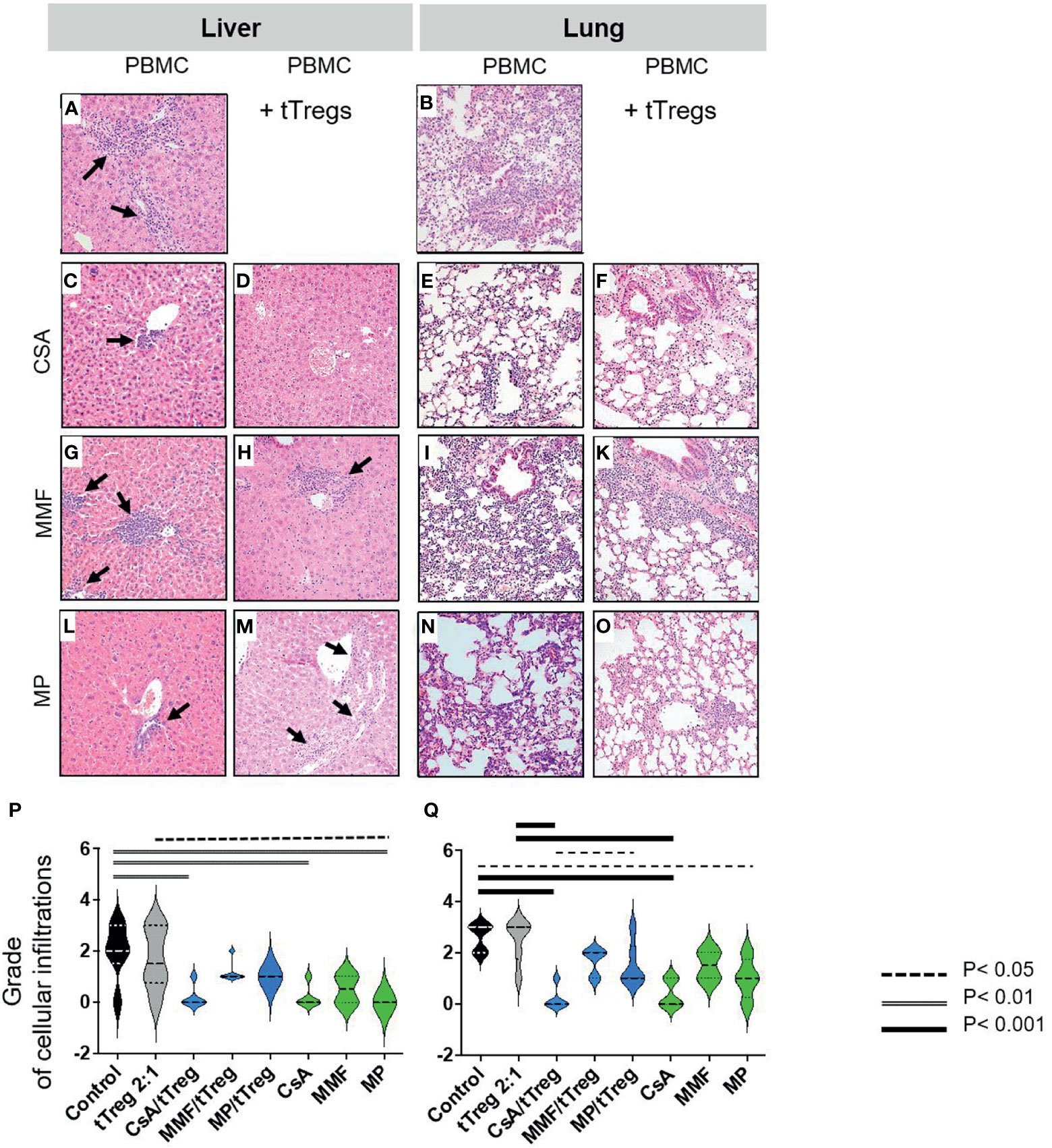
Figure 3 Histopathologic changes of liver and lung. Liver and lungs of mice described in Figure 1 were stained with Hematoxylin/Eosin (HE). Depicted are (A–O) representative images from liver and lung samples at 20-fold magnification and (A, B) moderate to high-grade cellular infiltration and inflammation in liver and lungs of animals that received PBMCs but no GvHD therapy. (A) Liver shows significant bile duct damage, grade 3 lobular inflammation with dense lymphocyte infiltration and lymphocyte ballooning (arrows). (B) Lung tissue shows massive intra-alveolar and intraepithelial lymphocyte infiltration with inflammation of the pulmonary epithelium and apoptotic bodies as well as loss of alveolar tissue architecture. (C–F) Only mild lymphocyte infiltration and almost complete resolution of inflammation with preserved tissue architecture in both liver (C, D) and lung (E, F) were observed in mice treated with CSA (C, E) or CSA + Tregs (D, F). (G, H) Significant reduction of hepatic lymphocyte infiltration and inflammation after MMF monotherapy; moderate infiltration of lymphocytes and signs of inflammation in mice which received combined MMF/tTreg therapy with preserved hepatic tissue architecture. (I–K) Only partial reduction of lymphocyte infiltration and inflammation in lung of mice which received MMF alone or in combination with tTregs. (I) Dense lymphocyte infiltration leading to diffuse alveolar damage and septal edema. (K) Alveolar architecture partially preserved after MMF/tTreg co-administration. (L–O) Significant reduction of cellular infiltration and preserved tissue architecture in both liver and lung upon treatment by MP alone or in combination with tTregs. (P, Q) shows quantification of lymphocyte infiltration of liver (P) and lung (Q). Statistical analysis was performed as One-Way-ANOVA with Tukey correction for multiple comparisons. Statistical significance levels are indicated as p < 0.05 (dotted line), p < 0.01 (dashed line), p < 0.001 (bold line), p < 0.0001 (solid line with asterix). CSA, Cyclosporine A; MMF, Mycophenolate Mofetil; MP, Methylprednisolone.
The superior efficacy of CSA and CSA/Treg co-administration as compared to the alternative treatment regimen tested became clearer when cellular infiltrates were analyzed in more detail (Figures 4, 5), yielding statistically significant differences.
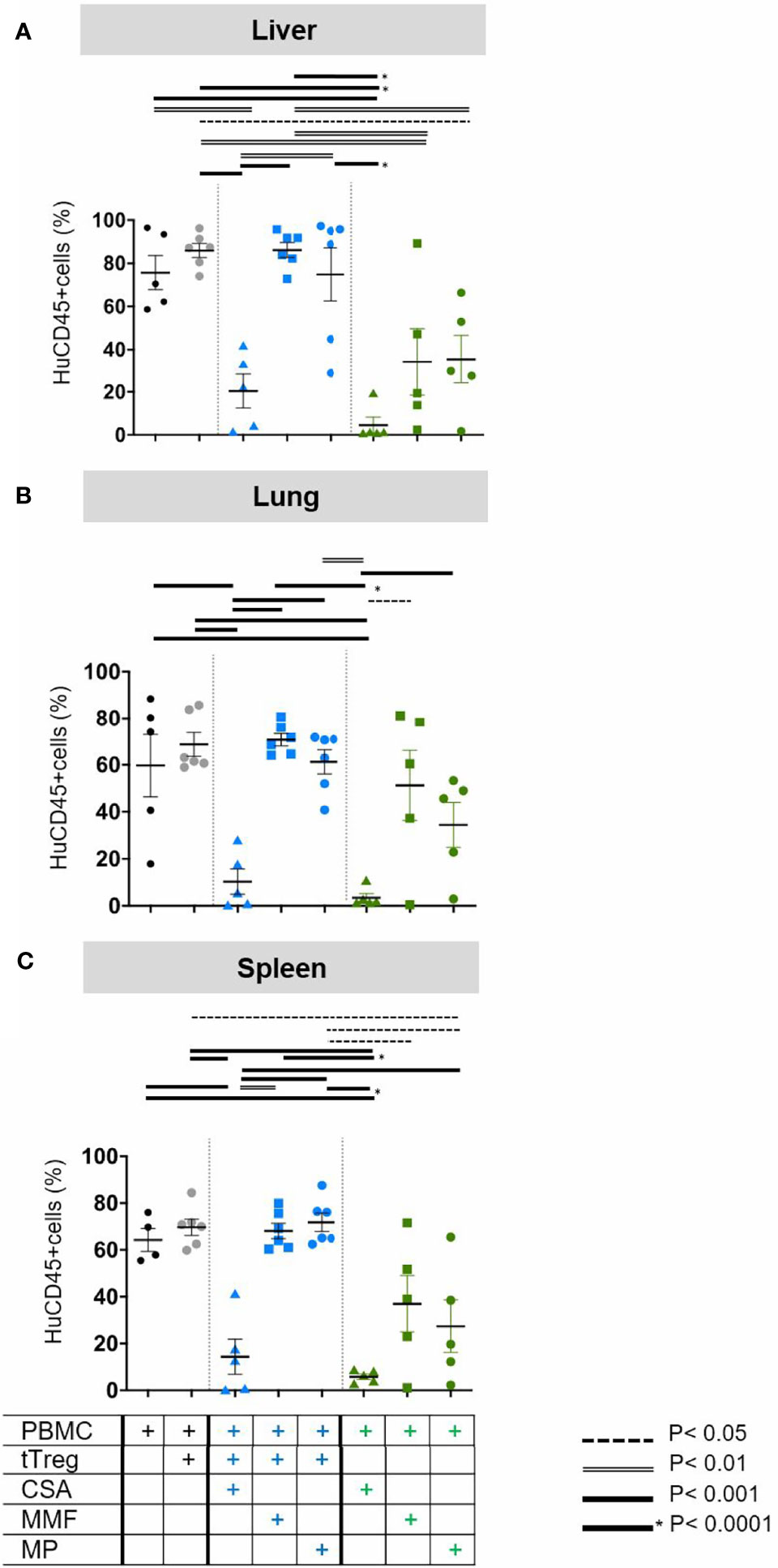
Figure 4 Quantification of human leukocyte infiltration. As described in Figure 1 GvHD was induced by 3x106 human PBMCs in NOD/SCID/IL2Rgamma -/- mice. Mice were subsequently treated with (i) the individual cISD (green symbols), CSA (4 mg/kg s.c., green triangles), MMF (0.5 mg p.o., green squares) or MP (20 mg/kg i.p., green dots) (n=5) alone (n=5), (ii) with ex vivo expanded tTregs at a PBMC:tTreg ratio of 2:1 (n=6, grey dots), or (iii) with a combination of cISD and low dose tTregs (PBMC:tTreg ratio 2:1; CSA/tTreg n=5, blue triangles; MMF/tTreg, blue squares; MP/tTreg n=6, blue dots). Postmortem liver (A), lung (B) and spleen (C) were analyzed by flow cytometry to quantify the amount of infiltrating CD45+ cells. Depicted are individual values and means ± SEM. Statistical analysis was performed as One-Way-ANOVA with Tukey correction for multiple comparison. Statistical significance levels are indicated as p < 0.05 (dotted line), p < 0.01 (dashed line), p < 0.001 (bold line), p < 0.0001 (solid line with asterix). CSA, Cyclosporine A; MMF, Mycophenolate Mofetil; MP, Methylprednisolone.
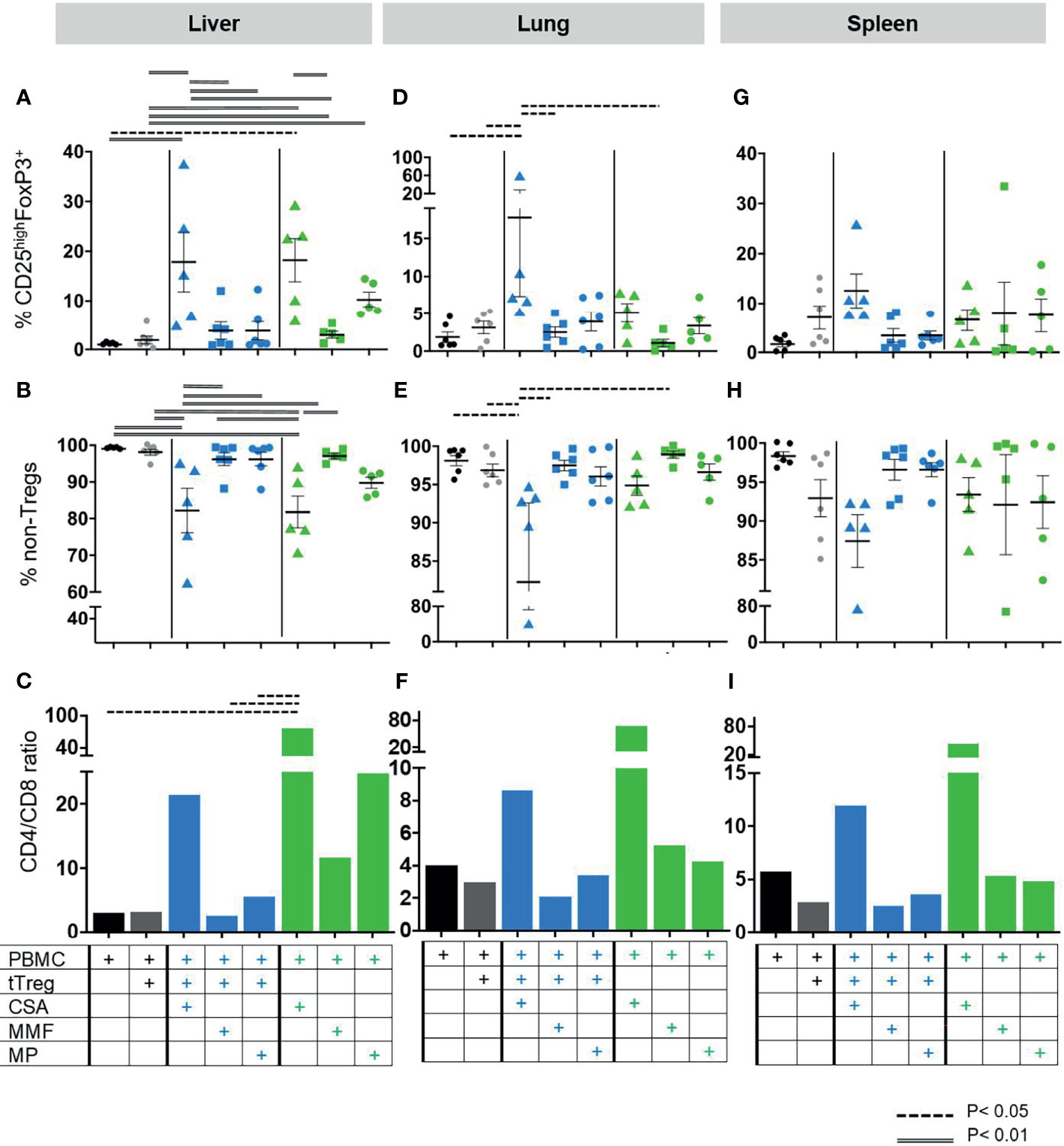
Figure 5 Composition of lymphocytic organ infiltrates. As described in Figure 1 GvHD was induced by 3x106 human PBMCs in NOD/SCID/IL2Rgamma-/- mice. Mice were subsequently treated with (i) the cISD (green symbols) CSA (4 mg/kg s.c., triangles), MMF (0.5 mg p.o, squares) or MP (20 mg/kg i.p., dots) (n=5) alone (n=5), (ii) with ex vivo expanded tTregs at a PBMC:tTreg ratio of 2:1 (n=6, grey dots), or (iii) with a combination of cISD and low dose tTregs (PBMC:tTreg ratio 2:1; CSA/tTreg n=5; MMF/tTreg, n=6; MP/tTreg n=6, blue symbols). Organ samples from liver, lung and spleen were further analyzed by flow cytometry to assess lymphocytic infiltration and proliferation. All samples were analyzed directly after harvesting as outlined in Table 1. To this end human live lymphocytes were identified as human CD45+ cells and subsequently gated as CD3+ cells. CD3+ T-lymphocytes were subdivided into CD4+ and CD4- cells. Treg cells were identified as CD25highFoxP3+ cells within the CD4+ compartment (A, D, G), while the remaining CD4+ cells were considered as non-Treg CD4+ T-cells (B, E, H), CD8+ T-cells were identified as CD3+CD4-. Subsequently CD4/CD8 ratios were analyzed for each group. (C, F, I). Depicted are individual values and mean values ± SEM. Statistical analysis was performed as One-Way-ANOVA with Tukey correction for multiple comparisons. Statistical significance levels are indicated as p < 0.05 (dashed line), p < 0.01 (doubled line), p < 0.001 (solid line). CSA, Cyclosporine A; MMF, Mycophenolate Mofetil; MP, Methylprednisolone.
To more accurately quantify cellular infiltration and further characterize the phenotype of lymphocytic infiltrates, liver (Figure 4A), lung (Figure 4B) and spleen (Figure 4C) tissues were analyzed by flow cytometry. In line with clinical experience, which classifies the liver as primary target organ of GvHD, we found massive infiltration by human CD45+ leukocytes in the liver (Figure 4A, black dots), but also in lungs (Figure 4B, black dots) and the spleen (Figure 4C, black dots). The mildly increased frequency of human leukocytes in case of low-dose Treg monotherapy, reflected the increased amount of circulating human cells but failed to reduce the frequency of infiltrating cells (Figures 4A–C, grey dots). Addition of CSA to Treg therapy significantly reduced organ infiltration in the liver, lung and spleen (Figures 4A–C, blue triangles). In contrast, neither tTreg/MMF (Figures 4A–C blue squares) nor tTreg/MP (Figures 4A–C, blue dots) co-administration was sufficient to reduce the hepatic, pulmonary or splenic infiltration of human leukocytes as compared to the untreated PBMC control (Figures 4A–C, black dots). Reassuringly, if only tTregs but no PBMCs were administered we did not observe infiltration of human cells into any organ (data not shown).
tTreg Recruitment to GvHD Target Tissues Is Promoted by CSA Co-Administration
As PBMC induced GvHD resulted in high frequencies of human leukocytes in the liver, lungs, and spleen, we further aimed to differentiate the composition of leukocytic organ infiltrates and assess the effect of cISD on tTregs in samples from livers (Figures 5A–C), lungs (Figures 5D–F) and spleens (Figures 5G–I). To this end these organ samples were analyzed for the specific frequency of CD4+CD25highFoxP3+ tTreg (Figures 5A, D, G) and CD4+ non-Tregs (Figures 5B, E, H). Additionally, the CD4/CD8 ratio was assessed in all samples (Figures 5C, F, I). Cellular infiltrates in all organs consisted of mainly CD3+CD4+ non-Treg lymphocytes (Figures 5B, E, H). In line with our clinical observations, co-administration of CSA and Tregs led to strong recruitment of circulating CD4+CD25highFoxP3+ Tregs to liver, lungs and spleens (Figures 5A, D, G) and substantially reduced the frequency of CD3+CD4+ non-Treg lymphocytes in these organs (Figures 5B, E, H). This effect was superior to any other therapeutic approach in lungs and spleens Figures 5D, E, G, H) and, in hepatic tissues, co-administration of CSA and Tregs was comparable to CSA monotherapy (Figures 5A, B). In line with the survival data presented in Figure 2, co-administration of tTregs and MP (Figures 5A, G) substantially reduced the hepatic and splenic proportion of tTregs as compared to MP monotherapy (Figures 5A, G). Similarly, combined administration of Treg and CSA reduced the frequency of infiltrating CD8 T-cells as expressed by increased CD4/CD8 ratios in these organs and was with this respect superior to both Treg/MMF and Treg/MP therapy (Figures 5C, F, I).
Discussion
The main focus of this study was to assess if any of the cISD used in first and second line GvHD prophylaxis or therapy either hamper or support the immunomodulatory functions of adoptively transferred tTregs in vivo. To this end we employed a humanized GvHD mouse model and found that in vivo survival and function of adoptively transferred tTregs are supported by combining them with CSA therapy, leading to significant reduction of harmful lymphocytic organ infiltration and limited clinical progression of GvHD. Similarly, the clinical course of mice treated with MMF and low-dose tTregs significantly improved. Conversely, we found that co-administration of steroids and tTregs negatively impacts the histological state in the studied tissues and the clinical outcome of GvHD.
During acute GvHD, glucocorticoids are usually administered at high doses to control allo-specific immune responses and tapered thereafter to the minimal dose required to prevent GvHD recurrence. The mechanism of action is mediated by DNA-binding steroid-receptor complexes that form in the cytosol and translocate to the nucleus where they directly interfere with the transcription factors AP-1 and NFkB (64, 65). In-depth data on the direct effects of corticosteroids on tTregs are lacking. Previous studies have shown that corticosteroids increase the frequency of circulating Tregs (51–53), and may amplify expansion of Tregs in an IL-2 (54, 55) or dendritic cell (66–70) dependent manner. These data however were not consistently confirmed (56) and we can only speculate on whether the ex vivo manipulation and expansion protocol of tTregs or the setting of GvHD might be responsible for the observed differences. Another reason for the aforementioned discrepancies might be the imprecise definition of Tregs, with some groups including induced Tregs in their analysis, while others used additional markers such as CD127, CD45RA and HLA-DR. However, steroid-induced FoxP3 expression (71) seems to not correlate with suppressive activity of these cells (72), and after recruitment to an inflamed tissue environment, these cells might exert effector T-cell function and promote a corticosteroid-dependent condition in patients suffering from GvHD. Last but not least, glucocorticoid-induced T-cell apoptosis is well described on a functional level, but is not well understood on a molecular level (73, 74). In light of the detrimental effect of MP on the clinical outcome we would strongly suggest minimizing the use of glucocorticoids in the case of tTreg therapy.
MMF is typically used in combination with prednisone and CSA. MMF is converted to its pharmacologically active form, Mycophenolic acid (MPA), by enteric and hepatic bioactivation. MPA inhibits proliferation of B- and T-lymphocytes primarily via the enzyme inosine monophosphate dehydrogenase, which is crucial for de novo purine synthesis. Substantial data on the specific effects of MPA on tTreg function and survival are insufficient as, in most studies, MMF was combined with CSA or steroids, but according to prevailing opinion MMF is compatible with adoptive tTreg transfer (43, 57, 75). A beneficial effect of MMF was postulated by the observation that the amount of circulating tTregs is significantly higher in kidney transplant patients treated with a combined therapy consisting of CSA, steroids and MMF as compared to patients who received CSA, steroids and Everolimus; however, it should be to taken into account that in this study CSA trough levels were significantly higher in the MMF treated group (46). In conclusion, we present, for the first time, data that indicates MMF combined with adoptively transferred tTregs may work synergistically to ameliorate the clinical signs of GvHD, which was reflected in histologically reduced cellular infiltration in the lung and liver. We were, however, unable to quantitatively confirm the additive effect of MMF and tTregs on a cellular level by organ flow cytometry, most likely due to the technical limitations of small organ anatomy and the overall limited cell number yield during organ preparation.
Calcineurin inhibitors, in particular CSA, are one of the most commonly used immunosuppressive compounds for maintenance immunosuppression in transplantation medicine, irrespective of the transplanted organ. By inhibition of the intracellular phosphatase calcineurin, cytosolic activation and nuclear translocation of the nuclear factor of activated T-cells is reduced, thereby hampering de novo synthesis of proinflammatory cytokines such as IL-2. Accordingly, the current paradigm holds that under most circumstances CSA leads to reduced de novo conversion of allospecific Tregs (45), reduced frequency of circulating tTregs (46), and reduced suppressive capacities (47). However, these results were generated in the clinical setting of solid organ or hematopoietic transplantation where cISD are administered at high doses. Therefore, conceptually different approaches were necessary to recognize that low-dose CSA can increase the frequency of circulating tTregs and promote tTreg function and survival (47, 76–78). In line with these findings, we previously reported that tTreg therapy can replace permanent pharmacologic immunosuppression only if tTreg transfer was combined with early-phase, short-term and low-dose CNI treatment (20). Within a Phase I/IIa clinical trial, we were able to further demonstrate that autologous Treg therapy allows tapering of standard triple immunosuppression in kidney transplanted patients to CNI (Tacrolimus) monotherapy if Tregs are administered early after kidney transplantation (79). The mechanism behind this was previously elucidated by Ruppert and colleagues, who showed that Tregs resist CSA-induced cell death via CD44-mediated signaling pathways in a dose-dependent fashion (39). In contrast to our data presented here, Zeiser et al., suggested that coadministration of CSA and Tregs hampers the suppressive capacity of tTregs (57). The authors further describe that the combination of CSA and tTregs strongly reduced survival in a GvHD mouse model, whereas this was not the case when tTregs were combined with MMF. However, the model employed by Zeiser et al., does not recapitulate the clinical state where we face either ongoing GvHD or the post-transplant phase with a particularly high risk of clinically relevant allo-responses (57). Additionally, Zeiser et al., administered 10 mg/kg CSA while in the model presented here, we use only 4 mg/kg body weight, which more closely corresponds to the clinical dosage in human patients (20, 58, 59). Therefore, it is perhaps unsurprising that in our present study we were able to confirm the beneficial effect of CSA and tTreg co-administration.
In summary, we have demonstrated that the efficacy of ex vivo pre-activated tTregs to control GvHD reactions by alloreactive T-cells is increased when combined with the calcineurin inhibitor CSA and, to a lesser extent, when combined with MMF. In contrast, the combination of tTregs with steroids in this context reduced tTreg treatment efficacy. In our mouse model, GvHD is due to clonal expansion of naïve xenoreactive T-cells and their differentiation to pathogenic effector T-cells. The data support synergistic inhibitory effects of CSA and tTreg on this process, whilst the functionality of pre-activated tTregs used for adoptive transfer appears to be resistant to CSA. The model presented here has one limitation: in vivo expansion of human tTreg in the humanized mouse graft is inherently poor, therefore, a relatively high tTreg/Teff ratio (e.g. >1:2) is necessary to see beneficial effects, confirming findings from numerous other groups (80–83). Thus, the use of CSA supports tTreg activity, but in a xenogenic mouse model high cell doses are necessary to compensate for poor tTreg clonal expansion. To date, the numbers of adoptively transferred tTregs required to obtain beneficial effects in a human context remains unclear. However, we conclude that CSA should be the agent of choice when adoptive transfer of a sufficient number of tTregs for GvHD is scheduled. MMF may be a further beneficial combination partner if dual or alternative therapy is required. Steroids should be avoided or, if necessary, be restricted to minimal doses.
Data Availability Statement
The raw data supporting the conclusions of this article will be made available by the authors, without undue reservation.
Ethics Statement
The studies involving human participants were reviewed and approved by Charité-Universitätsmedizin Berlin, Ethics Committee (Institutional Review Board), Charitéplatz 1, 10117 Berlin. The patients/participants provided their written informed consent to participate in this study. The animal study was reviewed and approved by Landesamt für Gesundheit und Soziales Berlin (G0483/09), Berlin, Germany.
Author Contributions
SL-K supervised the project, designed the experiments, performed some experiments, analyzed the data, and wrote the manuscript. AZ, AF, HH, and IS-K performed and analyzed experiments. MS-H contributed to the data analysis and assisted in writing the manuscript. RK performed the histological analysis of solid organs. H-DV and PR led the project, designed the research, assisted in data interpretation, and contributed the writing of the manuscript. All authors contributed to the article and approved the submitted version.
Funding
This project has received funding from the European Union’s Horizon 2020 Research and Innovation Programme under grant agreement No 825392 (RESHAPE) and from the European Union, Seventh Framework Programme [FP7/2007-2013], under grant agreement n° HEALTH-F5-2010-260687 The ONE Study. The project was further funded by the German Federal Ministry of Education and Research (BMBF) under grant agreement with the Berlin Institute of Health (BIH) and BIH Center for Regenerative Therapies (BCRT). SL-K was supported by a personal “Clinical Scientist” grant (BSRT/BCRT) and the Rahel-Hirsch-Habilitation Grant (Charité). The funders had no role in considering the study design or in the collection, analysis, interpretation of data, writing of the report, or decision to submit the article for publication.
Conflict of Interest
The authors declare that the research was conducted in the absence of any commercial or financial relationships that could be construed as a potential conflict of interest.
Publisher’s Note
All claims expressed in this article are solely those of the authors and do not necessarily represent those of their affiliated organizations, or those of the publisher, the editors and the reviewers. Any product that may be evaluated in this article, or claim that may be made by its manufacturer, is not guaranteed or endorsed by the publisher.
Acknowledgments
We are grateful to M. Streitz (Institute of Medical Immunology, Charité University Medicine Berlin) for technical flow cytometry assistance. We thank Nicola Brindle, Horst von Bernuth, and Mirjam Völler for careful proof-reading.
Supplementary Material
The Supplementary Material for this article can be found online at: https://www.frontiersin.org/articles/10.3389/fimmu.2021.716629/full#supplementary-material
References
1. Ghimire S, Weber D, Mavin E, Wang XN, Dickinson AM, Holler E. Pathophysiology of GvHD and Other HSCT-Related Major Complications. Front Immunol (2017) 8:79. doi: 10.3389/fimmu.2017.00079
2. Weisdorf D, Zhang M-J, Arora M, Horowitz MM, Rizzo JD, Eapen M. Graft-Versus-Host Disease Induced Graft-Versus-Leukemia Effect: Greater Impact on Relapse and Disease-Free Survival After Reduced Intensity Conditioning. Biol Blood Marrow Transplant (2012) 18:1727–33. doi: 10.1016/j.bbmt.2012.06.014
3. Sakaguchi S, Sakaguchi N, Asano M, Itoh M, Toda M. Immunologic Self-Tolerance Maintained by Activated T Cells Expressing IL-2 Receptor Alpha-Chains (CD25). Breakdown of a Single Mechanism of Self-Tolerance Causes Various Autoimmune Diseases. J Immunol (1995) 186:3808–21.
4. Li Q, Zhai Z, Xu X, Shen Y, Zhang A, Sun Z, et al. Decrease of CD4+CD25+ Regulatory T Cells and TGF-? At Early Immune Reconstitution Is Associated to the Onset and Severity of Graft-Versus-Host Disease Following Allogeneic Haematogenesis Stem Cell Transplantation. Leuk Res (2010) 34:1158–68. doi: 10.1016/j.leukres.2010.03.017
5. Bremm M, Huenecke S, Lehrnbecher T, Ponstingl E, Mueller R, Heinze A, et al. Advanced Flowcytometric Analysis of Regulatory T Cells: CD127 Downregulation Early Post Stem Cell Transplantation and Altered Treg/CD3+CD4+-Ratio in Severe GvHD or Relapse. J Immunol Methods (2011) 373:36–44. doi: 10.1016/j.jim.2011.07.018
6. Zorn E, Kim HT, Lee SJ, Floyd BH, Litsa D, Arumugarajah S, et al. Reduced Frequency of FOXP3+ CD4+CD25+ Regulatory T Cells in Patients With Chronic Graft-Versus-Host Disease. Blood (2005) 106:2903–11. doi: 10.1182/blood-2005-03-1257
7. Beres AJ, Drobyski WR. The Role of Regulatory T Cells in the Biology of Graft Versus Host Disease. Front Immunol (2013) 4:163. doi: 10.3389/fimmu.2013.00163
8. McIver Z, Melenhorst JJ, Wu C, Grim A, Ito S, Cho I, et al. Donor Lymphocyte Count and Thymic Activity Predict Lymphocyte Recovery and Outcomes After Matched-Sibling Hematopoietic Stem Cell Transplant. Haematologica (2013) 98:346–52. doi: 10.3324/haematol.2012.072991
9. Dejaco C, Duftner C, Grubeck-Loebenstein B, Schirmer M. Imbalance of Regulatory T Cells in Human Autoimmune Diseases. Immunology (2006) 117:289–300. doi: 10.1111/j.1365-2567.2005.02317.x
10. Akdis M, Verhagen J, Taylor A, Karamloo F, Karagiannidis C, Crameri R, et al. Immune Responses in Healthy and Allergic Individuals Are Characterized by a Fine Balance Between Allergen-Specific T Regulatory 1 and T Helper 2 Cells. J Exp Med (2004) 199:1567–75. doi: 10.1084/jem.20032058
11. Sakaguchi S, Ono M, Setoguchi R, Yagi H, Hori S, Fehervari Z, et al. Foxp3+ CD25+ CD4+ Natural Regulatory T Cells in Dominant Self-Tolerance and Autoimmune Disease. Immunol Rev (2006) 212:8–27. doi: 10.1111/j.0105-2896.2006.00427.x
12. Trzonkowski P, Dukat-Mazurek A, Bieniaszewska M, Marek-Trzonkowska N, Dobyszuk A, Juścińska J, et al. Treatment of Graft-Versus-Host Disease With Naturally Occurring T Regulatory Cells. BioDrugs (2013) 27:605–14. doi: 10.1007/s40259-013-0050-5
13. Landwehr-Kenzel S, Issa F, Luu S-H, Schmück M, Lei H, Zobel A, et al. Novel GMP-Compatible Protocol Employing an Allogeneic B Cell Bank for Clonal Expansion of Allospecific Natural Regulatory T Cells. Am J Transplant (2014) 14:594–606. doi: 10.1111/ajt.12629
14. Edinger M. Regulatory T Cells for the Prevention of Graft-Versus-Host Disease: Professionals Defeat Amateurs. Eur J Immunol (2009) 39:2966–8. doi: 10.1002/eji.200940030
15. Trzonkowski P, Bieniaszewska M, Juścińska J, Dobyszuk A, Krzystyniak A, Marek N, et al. First-in-Man Clinical Results of the Treatment of Patients With Graft Versus Host Disease With Human Ex Vivo Expanded CD4+CD25+CD127- T Regulatory Cells. Clin Immunol (2009) 133:22–6. doi: 10.1016/j.clim.2009.06.001
16. Di Ianni M, Falzetti F, Carotti A, Terenzi A, Castellino F, Del Papa B, et al. Tregs Prevent GVHD and Promote Immune Reconstitution in HLA-Haploidentical Transplantation. Blood (2011) 117:3921–8. doi: 10.1182/blood-2010-10-311894
17. Brunstein CG, Miller JS, Cao Q, McKenna DH, Hippen KL, Curtsinger J, et al. Infusion of Ex Vivo Expanded T Regulatory Cells in Adults Transplanted With Umbilical Cord Blood: Safety Profile and Detection Kinetics. Blood (2011) 117:1061–70. doi: 10.1182/blood-2010-07-293795
18. Koreth J, Kim HT, Jones KT, Lange PB, Reynolds CG, Chammas MJ, et al. Efficacy, Durability, and Response Predictors of Low-Dose Interleukin-2 Therapy for Chronic Graft-Versus-Host Disease. Blood (2017) 128:130–8. doi: 10.1182/blood-2016-02-702852.The
19. Koreth J, Matsuoka K, Kim H, McDonough S, Bindra B, Alyea EP, et al. Interleukin-2 and Regulatory T Cells in Graft-Versus-Host Disease. N Engl J Med (2011) 365:2055–66. doi: 10.1056/NEJMoa1108188
20. Siepert A, Ahrlich S, Vogt K, Appelt C, Stanko K, Kühl A, et al. Permanent CNI Treatment for Prevention of Renal Allograft Rejection in Sensitized Hosts Can Be Replaced by Regulatory T Cells. Am J Transplant (2012) 12:2384–94. doi: 10.1111/j.1600-6143.2012.04143.x
21. Vaikunthanathan T, Safinia N, Boardman D, Lechler RI, Lombardi G. Regulatory T Cells: Tolerance Induction in Solid Organ Transplantation. Clin Exp Immunol (2017) 189(2):197–210. doi: 10.1111/cei.12978
22. Schließer U, Streitz M, Sawitzki B. Tregs : Application for Solid-Organ Transplantation. Curr Opin Organ Transplant (2012) 17:34–41. doi: 10.1097/MOT.0b013e32834ee69f
23. Saadoun D, Rosenzwajg M, Joly F, Six A, Carrat F, Thibault V, et al. Regulatory T-Cell Responses to Low-Dose Interleukin-2 in HCV-Induced Vasculitis. N Engl J Med (2011) 365:2067–77. doi: 10.1056/NEJMoa1105143
24. Humrich JY, von Spee-Mayer C, Siegert E, Alexander T, Hiepe F, Radbruch A, et al. Rapid Induction of Clinical Remission by Low- Dose Interleukin-2 in a Patient With Refractory SLE. BMJ (2015) 74:791–2. doi: 10.1136/annrheumdis-2014-206506
25. Hartemann A, Bensimon G, Payan CA, Jacqueminet S, Bourron O, Nicolas N, et al. Low-Dose Interleukin 2 in Patients With Type 1 Diabetes : A Phase 1 / 2 Randomised , Double-Blind , Placebo-Controlled Trial. Lancet (2013) 1:295–305. doi: 10.1016/S2213-8587(13)70113-X
26. Bluestone JA, Buckner JH, Fitch M, Gitelman SE, Gupta S, Hellerstein MK, et al. Type 1 Diabetes Immunotherapy Using Polyclonal Regulatory T Cells. Sci Transl Med (2015) 7:315ra189. doi: 10.1126/scitranslmed.aad4134
27. Scottà C, Fanelli G, Hoong SJ, Romano M, Lamperti EN, Sukthankar M, et al. Impact of Immunosuppressive Drugs on the Therapeutic Efficacy of Ex Vivo Expanded Human Regulatory T Cells. Haematologica (2016) 101:91–100. doi: 10.3324/haematol.2015.128934
28. San Segundo D, Ruiz JC, Fernández-Fresnedo G, Izquierdo M, Gómez-Alamillo C, Cacho E, et al. Calcineurin Inhibitors Affect Circulating Regulatory T Cells in Stable Renal Transplant Recipients. Transplant Proc (2006) 38:2391–3. doi: 10.1016/j.transproceed.2006.08.081
29. Choi SW, Gatza E, Hou G, Sun Y, Whitfield J, Song Y, et al. Histone Deacetylase Inhibition Regulates Inflammation and Enhances Tregs After Allogeneic Hematopoietic Cell Transplantation in Humans. Blood (2015) 125:815–9. doi: 10.1182/blood
30. Tao R, de Zoeten EF, Ozkaynak E, Chen C, Wang L, Porrett PM, et al. Deacetylase Inhibition Promotes the Generation and Function of Regulatory T Cells. Nat Med (2007) 13:1299–307. doi: 10.1038/nm1652
31. Pham MN, von Herrath MG, Vela JL. Antigen-Specific Regulatory T Cells and Low Dose of IL-2 in Treatment of Type 1 Diabetes. Front Immunol (2015) 6:651. doi: 10.3389/fimmu.2015.00651
32. Matsuoka K, Koreth J, Kim HT, Bascug G, McDonough S, Kawano Y, et al. Low-Dose Interleukin-2 Therapy Restores Regulatory T Cell Homeostasis in Patients With Chronic Graft-Versus-Host Disease. Sci Transl Med (2013) 5:179ra43. doi: 10.1126/scitranslmed.3005265
33. Castela E, Le Duff F, Butori C, Ticchioni M, Hofman P, Bahadoran P, et al. Effects of Low-Dose Recombinant Interleukin 2 to Promote T-Regulatory Cells in Alopecia Areata. JAMA Dermatol (2014) 150:748–51. doi: 10.1001/jamadermatol.2014.504
34. Rosenzwajg M, Churlaud G, Mallone R, Six A, Dérian N, Chaara W, et al. Low-Dose Interleukin-2 Fosters a Dose-Dependent Regulatory T Cell Tuned Milieu in T1D Patients. J Autoimmun (2015) 58:48–58. doi: 10.1016/j.jaut.2015.01.001
35. Kennedy-Nasser A a, Ku S, Castillo-Caro P, Hazrat Y, Wu M-F, Liu H, et al. Ultra Low-Dose IL-2 for GVHD Prophylaxis After Allogeneic Hematopoietic Stem Cell Transplantation Mediates Expansion of Regulatory T Cells Without Diminishing Antiviral and Antileukemic Activity. Clin Cancer Res (2014) 20:2215–25. doi: 10.1158/1078-0432.CCR-13-3205
36. Ito S, Bollard CM, Carlsten M, Melenhorst JJ, Biancotto A, Wang E, et al. Ultra-Low Dose Interleukin-2 Promotes Immune- Modulating Function of Regulatory T Cells and Natural Killer Cells in Healthy Volunteers. Mol Ther (2014) 22:1388–95. doi: 10.1038/mt.2014.50
37. Gurkan S, Luan Y, Dhillon N, Allam SR, Montague T, Bromberg JS, et al. Immune Reconstitution Following Rabbit Antithymocyte Globulin. Am J Transplant (2010) 10:2132–41. doi: 10.1111/j.1600-6143.2010.03210.x
38. Grafals M, Smith B, Murakami N, Trabucco A, Hamill K, Marangos E, et al. Immunophenotyping and Efficacy of Low Dose ATG in non-Sensitized Kidney Recipients Undergoing Early Steroid Withdrawal: A Randomized Pilot Study. PloS One (2014) 9:e104408. doi: 10.1371/journal.pone.0104408
39. Ruppert SM, Falk BA, Long SA, Bollyky PL. Regulatory T Cells Resist Cyclosporine-Induced Cell Death via CD44-Mediated Signaling Pathways. Int J Cell Biol (2015) 2015:1–10. doi: 10.1155/2015/614297
40. Presser D, Sester U, Mohrbach J, Janssen M, Köhler H, Sester M. Differential Kinetics of Effector and Regulatory T Cells in Patients on Calcineurin Inhibitor–Based Drug Regimens. Kidney Int (2009) 76:557–66. doi: 10.1038/ki.2009.198
41. Segundo D, Ruiz JC, Cacho E, Rodrigo E, Palomar R, Lo M. Calcineurin Inhibitors, But Not Rapamycin, Reduce Percentages of CD4+CD25+FoxP3+ Regulatory T Cells in Renal Transplant Recipients. Transplantation (2006) 82:550–7. doi: 10.1097/01.tp.0000229473.95202.50
42. Gallon L, Traitanon O, Sustento-Reodica N, Leventhal J, Ansari MJ, Gehrau RC, et al. Cellular and Molecular Immune Profiles in Renal Transplant Recipients After Conversion From Tacrolimus to Sirolimus. Kidney Int (2015) 87:828–38. doi: 10.1038/ki.2014.350
43. Demirkiran A, Sewgobind VD, van der Weijde J, Kok A, Baan CC, Kwekkeboom J, et al. Conversion From Calcineurin Inhibitor to Mycophenolate Mofetil-Based Immunosuppression Changes the Frequency and Phenotype of CD4+FOXP3+ Regulatory T Cells. Transplantation (2009) 87:1062–8. doi: 10.1097/TP.0b013e31819d2032
44. Pascual J, Bloom D, Torrealba J, Brahmbhatt R, Chang Z, Sollinger HW, et al. Calcineurin Inhibitor Withdrawal After Renal Transplantation With Alemtuzumab: Clinical Outcomes and Effect on T-Regulatory Cells. Am J Transplant (2008) 8:1529–36. doi: 10.1111/j.1600-6143.2008.02260.x
45. Gao W, Lu Y, El Essawy B, Oukka M, Kuchroo VK, Strom TB. Contrasting Effects of Cyclosporine and Rapamycin in De Novo Generation of Alloantigen-Specific Regulatory T Cells. Am J Transpl (2007) 7:1722–32. doi: 10.1111/j.1600-6143.2007.01842.x
46. Fourtounas C, Dousdampanis P, Sakellaraki P, Rodi M, Georgakopoulos T, Vlachojannis JG, et al. Different Immunosuppressive Combinations on T-Cell Regulation in Renal Transplant Recipients. Am J Nephrol (2010) 32:1–9. doi: 10.1159/000313940
47. Miroux C, Morales O, Ghazal K, Ben Othman S, de Launoit Y, Pancré V, et al. In Vitro Effects of Cyclosporine A and Tacrolimus on Regulatory T-Cell Proliferation and Function. Transplantation (2012) 94:123–31. doi: 10.1097/TP.0b013e3182590d8f
48. Kawai M, Kitade H, Mathieu C, Waer M, Pirenne J. Inhibitory and Stimulatory Effects of Cyclosporine A on the Development of Regulatory T Cells In Vivo. Transplantation (2005) 79:1073–7. doi: 10.1097/01.TP.0000153505.73700.32
49. Bluestone J, Liu W, Yabu JM, Laszik ZG, Putnam A, Belingheri M, et al. The Effect of Costimulatory and Interleukin 2 Receptor Blockade on Regulatory T Cells in Renal Transplantation. Am J Transplant (2008) 8:2086–96. doi: 10.1111/j.1600-6143.2008.02377.x
50. Zhao T, Yang C, Xue Y, Qiu YY, Hu L, Qiu Y, et al. Impact of Basiliximab on the Proportion of Regulatory T Cells and Their Subsets Early After Renal Transplantation: A Preliminary Report. Transplantation (2012) 178:175–8. doi: 10.1016/j.transproceed.2011.11.026
51. Seissler N, Schmitt E, Hug F, Sommerer C, Zeier M, Schaier M, et al. Methylprednisolone Treatment Increases the Proportion of the Highly Suppressive HLA-DR + -Treg-Cells in Transplanted Patients. Transpl Immunol (2012) 27(4):157–61. doi: 10.1016/j.trim.2012.09.003
52. Braitch M, Harikrishnan S, Robins RA, Nichols C, Fahey AJ, Showe L, et al. Glucocorticoids Increase CD4CD25 Cell Percentage and Foxp3 Expression in Patients With Multiple Sclerosis. Acta Neurol Scand (2009) 119:239–45. doi: 10.1111/j.1600-0404.2008.01090.x
53. Karagiannidis C, Akdis M, Holopainen P, Woolley NJ, Hense G, Rückert B, et al. Glucocorticoids Upregulate FOXP3 Expression and Regulatory T Cells in Asthma. J Allergy Clin Immunol (2004) 114:1425–33. doi: 10.1016/j.jaci.2004.07.014
54. Chen X, Oppenheim J, Winkler-Pickett R, Ortaldo J, Howard O M Zac. Glucocorticoid Amplifies IL-2-Dependent Expansion of Functional FoxP3+CD4+CD25+ T Regulatory CellsIn Vivo and Enhances Their Capacity to Suppress EAE. Eur J Immunol (2006) 36:2139–49. doi: 10.1002/eji.200635873
55. Xu L, Xu Z, Xu M. Glucocorticoid Treatment Restores the Impaired Suppressive Function of Regulatory T Cells in Patients With Relapsing-Remitting Multiple Sclerosis. Clin Exp Immunol (2009) 158:26–30. doi: 10.1111/j.1365-2249.2009.03987.x
56. Sbiera S, Dexneit T, Reichardt SD, Michel KD, van den Brandt J, Schmull S, et al. Influence of Short-Term Glucocorticoid Therapy on Regulatory T Cells In Vivo. PloS One (2011) 6:e24345. doi: 10.1371/journal.pone.0024345
57. Zeiser R, Nguyen VH, Beilhack A, Buess M, Schulz S, Baker J, et al. Inhibition of CD4+CD25+ Regulatory T-Cell Function by Calcineurin-Dependent Interleukin-2 Production. Blood (2006) 108:390–9. doi: 10.1182/blood-2006-01-0329
58. Noll BD, Coller JK, Somogyi AA, Morris RG, Russ GR, Hesselink DA, et al. Measurement of Cyclosporine A in Rat Tissues and Human Kidney Transplant Biopsies - A Method Suitable for Small (<1 Mg) Samples. Ther Drug Monit (2011) 33:688–93. doi: 10.1097/FTD.0b013e318236315d
59. Haug M, Fritz M, Dan O, Lorenz RR, Wimberley S, Strome M. Cyclosporine Dose, Serum Trough Levels, and Allograft Preservation in a Rat Model of Laryngeal Transplantation. Ann Otol Rhinol Laryngol (2003) 112:506–10. doi: 10.1177/000348940311200604
60. Brandhorst G, Brehmer F, Petrova DT, Gross O, Miosge N, Armstrong VW, et al. Mycophenolic Acid Predose Concentrations and Renal Function in a Mouse Model for Progressive Renal Fibrosis. Ther Drug Monit (2010) 32:73–8. doi: 10.1097/FTD.0b013e3181c91fc4
61. Nalle SC, Zuo L, Ong MLDM, Singh G, Worthylake AM, Choi W, et al. Graft-Versus-Host Disease Propagation Depends on Increased Intestinal Epithelial Tight Junction Permeability. J Clin Invest (2019) 129:902–14. doi: 10.1172/JCI98554
62. Heimesaat MM, Nogai A, Bereswill S, Plickert R, Fischer A, Loddenkemper C, et al. MyD88/TLR9 Mediated Immunopathology and Gut Microbiota Dynamics in a Novel Murine Model of Intestinal Graft-Versus-Host Disease. Gut (2010) 59:1079–87. doi: 10.1136/gut.2009.197434
63. Hill GR, Crawford JM, Cooke KR, Brinson YS, Pan L, Ferrara JLM. Total Body Irradiation and Acute Graft-Versus-Host Disease: The Role of Gastrointestinal Damage and Inflammatory Cytokines. Blood (1997) 90:3204–13. doi: 10.1182/blood.v90.8.3204
64. McKay LI, Cidlowski JA. Molecular Control of Immune/Inflammatory Responses: Interactions Between Nuclear Factor-?B and Steroid Receptor-Signaling Pathways. Endocr Rev (1999) 20:435–59. doi: 10.1210/edrv.20.4.0375
65. Coutinho AE, Chapman KE. The Anti-Inflammatory and Immunosuppressive Effects of Glucocorticoids, Recent Developments and Mechanistic Insights. Mol Cell Endocrinol (2011) 335:2–13. doi: 10.1016/j.mce.2010.04.005
66. Calmette J, Ellouze M, Tran T, Karaki S, Ronin E, Capel F, et al. Glucocorticoid-Induced Leucine Zipper Enhanced Expression in Dendritic Cells Is Sufficient To Drive Regulatory T Cells Expansion In Vivo. J Immunol (2014) 193(12):5863–72. doi: 10.4049/jimmunol.1400758
67. Mahnke K, Johnson TS, Ring S, Enk AH. Tolerogenic Dendritic Cells and Regulatory T Cells: A Two-Way Relationship. J Dermatol Sci (2007) 46:159–67. doi: 10.1016/j.jdermsci.2007.03.002
68. Rutella S, Lemoli RM. Regulatory T Cells and Tolerogenic Dendritic Cells: From Basic Biology to Clinical Applications. Immunol Lett (2004) 94:11–26. doi: 10.1016/j.imlet.2004.04.015
69. Stoltze ET, Lauer S, Poeschel S, Melms A, Brucklacher-Waldert V, Rosenkranz D, et al. Myasthenia Gravis Patients Dendritic Cells and a Regulatory Milieu in Prednisolone Treatment Induces Tolerogenic Prednisolone Treatment Induces Tolerogenic Dendritic Cells and a Regulatory Milieu in Myasthenia Gravis Patients 1. June J Immunol Mater Suppl J Immunol (2017) 183:841–8. doi: 10.4049/jimmunol.0802046
70. Luther C, Adamopoulou E, Stoeckle C, Brucklacher-Waldert V, Rosenkranz D, Stoltze L, et al. Prednisolone Treatment Induces Tolerogenic Dendritic Cells and a Regulatory Milieu in Myasthenia Gravis Patients. J Immunol (2009) 183(2):841–8. doi: 10.4049/jimmunol.0802046
71. Rudra D, deRoos P, Chaudhry A, Niec RE, Arvey A, Samstein RM, et al. Transcription Factor Foxp3 and its Protein Partners Form a Complex Regulatory Network. Nat Immunol (2012) 13:1010–9. doi: 10.1038/ni.2402
72. Prado C, Gómez J, López P, de Paz B, Gutiérrez C, Suárez A. Dexamethasone Upregulates FOXP3 Expression Without Increasing Regulatory Activity. Immunobiology (2011) 216:386–92. doi: 10.1016/j.imbio.2010.06.013
73. Ashwell JD, Lu FWM, Vacchio MS. Glucocorticoids in T Cell Development and Function. Annu Rev Immunol (2000) 18:309–45. doi: 10.1146/annurev.immunol.18.1.309
74. Herold MJ, McPherson KG, Reichardt HM. Glucocorticoids in T Cell Apoptosis and Function. Cell Mol Life Sci (2006) 63:60–72. doi: 10.1007/s00018-005-5390-y
75. Abadja F, Videcoq C, Alamartine E, Berthoux F, Mariat C. Differential Effect of Cyclosporine and Mycophenolic Acid on the Human Regulatory T Cells and TH-17 Cells Balance. Transplant Proc (2009) 41:3367–70. doi: 10.1016/j.transproceed.2009.08.031
76. Brandt C, Pavlovic V, Radbruch A, Worm M, Baumgrass R. Low-Dose Cyclosporine A Therapy Increases the Regulatory T Cell Population in Patients With Atopic Dermatitis. Allergy (2009) 64:1588–96. doi: 10.1111/j.1398-9995.2009.02054.x
77. Hijnen D, Haeck I, van Kraats AA, Nijhuis E, de Bruin-Weller MS, Bruijnzeel-Koomen CAFM, et al. Cyclosporin A Reduces CD4+CD25+ Regulatory T-Cell Numbers in Patients With Atopic Dermatitis. J Allergy Clin Immunol (2009) 124:856–8. doi: 10.1016/j.jaci.2009.07.056
78. Baumgrass R, Brandt C, Wegner F, Abdollahnia M, Worm M. Low-Dose, But Not High-Dose, Cyclosporin A Promotes Regulatory T-Cell Induction, Expansion, or Both. J Allergy Clin Immunol (2010) 126:183–4. doi: 10.1016/j.jaci.2010.04.032
79. Roemhild A, Otto NM, Moll G, Abou-El-Enein M, Kaiser D, Bold G, et al. Regulatory T Cells for Minimising Immune Suppression in Kidney Transplantation: Phase I/IIa Clinical Trial. BMJ (2020) 371:m3734. doi: 10.1136/bmj.m3734
80. McDonald-Hyman C, Flynn R, Panoskaltsis-Mortari A, Peterson N, MacDonald KPA, Hill GR, et al. Therapeutic Regulatory T-Cell Adoptive Transfer Ameliorates Established Murine Chronic GVHD in a CXCR5-Dependent Manner. Blood (2016) 128:1013–7. doi: 10.1182/blood-2016-05-715896
81. Leveque-El Mouttie L, Koyama M, Le Texier L, Markey KA, Cheong M, Kuns RD, et al. Corruption of Dendritic Cell Antigen Presentation During Acute GVHD Leads to Regulatory T-Cell Failure and Chronic GVHD. Blood (2016) 128:794–804. doi: 10.1182/blood-2015-11-680876
82. Riegel C, Boeld TJ, Doser K, Huber E, Hoffmann P, Edinger M. Efficient Treatment of Murine Acute GvHD by In Vitro Expanded Donor Regulatory T Cells. Leukemia (2020) 34:895–908. doi: 10.1038/s41375-019-0625-3
Keywords: transplantation, regulatory T-cells, adoptive cell therapy, tolerance, drug interaction, Graft-versus-Host Disease, immunosuppressive drugs
Citation: Landwehr-Kenzel S, Zobel A, Schmitt-Knosalla I, Forke A, Hoffmann H, Schmueck-Henneresse M, Klopfleisch R, Volk H-D and Reinke P (2021) Cyclosporine A but Not Corticosteroids Support Efficacy of Ex Vivo Expanded, Adoptively Transferred Human Tregs in GvHD. Front. Immunol. 12:716629. doi: 10.3389/fimmu.2021.716629
Received: 28 May 2021; Accepted: 02 September 2021;
Published: 11 October 2021.
Edited by:
Benny J. Chen, Duke University, United StatesReviewed by:
Robert B. Levy, University of Miami, United StatesBryon Johnson, Medical College of Wisconsin, United States
Copyright © 2021 Landwehr-Kenzel, Zobel, Schmitt-Knosalla, Forke, Hoffmann, Schmueck-Henneresse, Klopfleisch, Volk and Reinke. This is an open-access article distributed under the terms of the Creative Commons Attribution License (CC BY). The use, distribution or reproduction in other forums is permitted, provided the original author(s) and the copyright owner(s) are credited and that the original publication in this journal is cited, in accordance with accepted academic practice. No use, distribution or reproduction is permitted which does not comply with these terms.
*Correspondence: Sybille Landwehr-Kenzel, c3liaWxsZS5sYW5kd2Voci1rZW56ZWxAY2hhcml0ZS5kZQ==; orcid.org/0000-0002-4836-7659