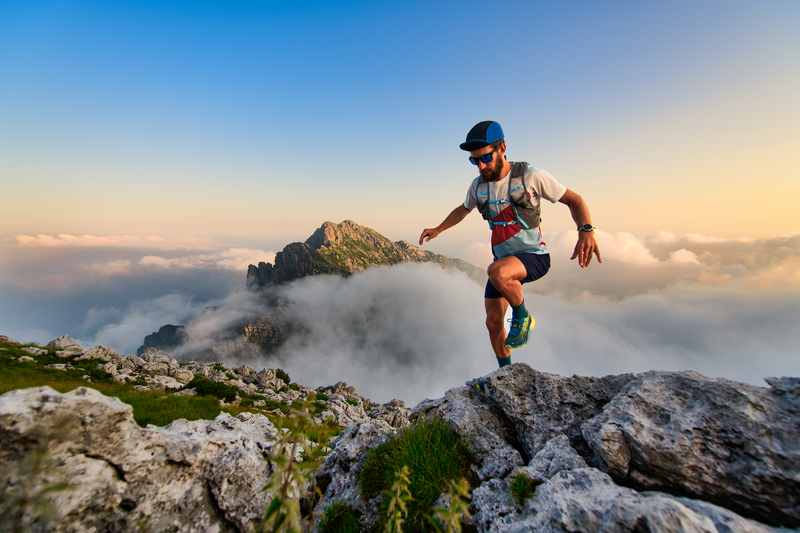
95% of researchers rate our articles as excellent or good
Learn more about the work of our research integrity team to safeguard the quality of each article we publish.
Find out more
REVIEW article
Front. Immunol. , 05 August 2021
Sec. Multiple Sclerosis and Neuroimmunology
Volume 12 - 2021 | https://doi.org/10.3389/fimmu.2021.714179
This article is part of the Research Topic A Microbial View of Central Nervous System Disorders: Interplay Between Microorganisms, Neuroinflammation and Behavior View all 9 articles
This article prosecutes a case against the zoonotic pathogen Mycobacterium avium ss. paratuberculosis (MAP) as a precipitant of Alzheimer’s disease (AD). Like the other major neurodegenerative diseases AD is, at its core, a proteinopathy. Aggregated extracellular amyloid protein plaques and intracellular tau protein tangles are the recognized protein pathologies of AD. Autophagy is the cellular housekeeping process that manages protein quality control and recycling, cellular metabolism, and pathogen elimination. Impaired autophagy and cerebral insulin resistance are invariant features of AD. With a backdrop of age-related low-grade inflammation (inflammaging) and heightened immune risk (immunosenescence), infection with MAP subverts glucose metabolism and further exhausts an already exhausted autophagic capacity. Increasingly, a variety of agents have been found to favorably impact AD; they are agents that promote autophagy and reduce insulin resistance. The potpourri of these therapeutic agents: mTOR inhibitors, SIRT1 activators and vaccines are seemingly random until one recognizes that all these agents also suppress intracellular mycobacterial infection. The zoonotic mycobacterial MAP causes a common fatal enteritis in ruminant animals. Humans are exposed to MAP from contaminated food products and from the environment. The enteritis in animals is called paratuberculosis or Johne’s disease; in humans, it is the putative cause of Crohn’s disease. Beyond Crohn’s, MAP is associated with an increasing number of inflammatory and autoimmune diseases: sarcoidosis, Blau syndrome, autoimmune diabetes, autoimmune thyroiditis, multiple sclerosis, and rheumatoid arthritis. Moreover, MAP has been associated with Parkinson’s disease. India is one county that has extensively studied the human bio-load of MAP; 30% of more than 28,000 tested individuals were found to harbor, or to have harbored, MAP. This article asserts an unfolding realization that MAP infection of humans 1) is widespread in its presence, 2) is wide-ranging in its zoonosis and 3) provides a plausible link connecting MAP to AD.
Common age-related neurodegenerative diseases are Alzheimer’s disease (AD), Parkinson’s disease (PD), Huntington’s disease (HD) and amyotrophic lateral sclerosis (ALS). While these diseases are distinct clinical entities, at their core, they are all proteinopathies and share a common feature: misfolded and aggregated proteins. For AD, the proteins are amyloid and tau; for PD, synuclein; for HD, Htt; and for ALS, TDP-43 (1). In disease, these proteins lose their physiological functions, aggregate and acquire neurotoxic potential (2). In AD, impairment of protein elimination is central to amyloid accumulation; there is stable production, but inadequate amyloid clearance (3).
Autophagy is the conserved phylogenetic mechanism critical for intracellular clearance and recycling of aged and/or damaged protein elements occurring in all cell types, including neurons. In the brain, astrocytes and subtypes of microglia play important “janitorial” roles in the phagocytosis and subsequent autophagic elimination of neurotoxic proteins (4).
Autophagy is also critical for the regulation of a wide range of immune responses including innate immunity, inflammation, and antibacterial defense (5, 6). When pathogenic microbes are the target of autophagy the process is called “xenophagy,” a form of selective autophagy (7).
Consideration of an infectious contribution to AD is not new. The “usual suspects” are herpes simplex virus 1 (HSV-1), Cytomegalovirus CMV), Borrelia burgdorferi, Chlamydia pneumoniae, Helicobacter pylori and Porphyromonas gingivalis (8, 9). Giving credence to a microbial cause of AD is the recognition that amyloid is an antimicrobial peptide (AMP), and the accumulation of this AMP may be reflective of an increasing infectious burden (10, 11). Indeed, the risk of AD appears to increase as the number of concurrent infections increases (12).
This article presents an additional potential AD precipitant, the zoonotic pathogen Mycobacterium avium ss. paratuberculosis (MAP). MAP has been killing livestock, contaminating food products and has been associated with human inflammatory and autoimmune diseases at a steadily increasing global rate for 100 years (13–15). Moreover, MAP can contribute to cellular and metabolic invariant features of AD; namely dysfunctional autophagy and insulin resistance (Figure 1).
Figure 1 MAP can impair autophagy and promote insulin resistance; these respectively, are the cellular and metabolic changes seen in Alzheimer’s disease. Mycobacterium avium subspecies paratuberculosis – MAP is a notable bacterium responsible for disease of animals, contamination of food and water and associated with an increasingly long list of human diseases.
Before an in-depth discussion of MAP and AD, it is relevant to review systemic states that are at the intersection of age and AD: inflammaging and immunosenescence.
Inflammaging, coined by Franceschi in 2001 (16) refers to a low-grade inflammatory state manifest by 1) expression of genes linked to inflammation 2) higher levels of cytokines in serum and 3) activation of nuclear factor (NF) signaling, the master regulator of inflammatory responses (17–19). Inflammaging is associated with a decline in autophagic capacity impairing cellular housekeeping which leads to protein aggregation and accumulation of dysfunctional mitochondria (20). This age-related phenotype is a risk factor for morbidity and mortality of the elderly and is implicated in the pathogenesis of a number of human maladies including type-2 diabetes, coronary artery disease and Alzheimer’s (21, 22).
Paralleling inflammaging is an age-related reduction in immune competency termed immunosenescence (23). Immunosenescence not only lessens the capacity to respond to infections but also contributes to a number of age-related non-infectious diseases (24, 25). While aging of the immune system has been often described as a shift from naive lymphocytes to memory lymphocytes, that shift is relative; aging itself is not the sole determinant of an accumulation of memory T cells and B cells. For instance, cytomegalovirus infection is associated with an acceleration of immunosenescence (26) as well as increased risk of mycobacterial infection (27, 28).
There is a bi-directional communication, a crosstalk, between the gut microbiota and CNS; the gut-brain-microbiome axis is increasingly understood to play a major role in the pathogenesis of many neurodegenerative disorders including Alzheimer’s disease (AD) (29).
There is increasing evidence suggesting that the development of the hallmark pathologic features of AD, amyloid plaque, and tau tangles, can be linked to microbes (30). This is tied to the aforementioned function of amyloid as an antimicrobial peptide (AMP). Studies found that amyloid exerts antimicrobial activity against eight common, clinically relevant microorganisms. Previously unrecognized as an AMP, this contrasting model of amyloid-mediated pathology has important implications for ongoing and future AD treatment strategies (31). Though none of the tested microbes were mycobacteria, it is hoped that this manuscript would prompt future testing of mycobacteria, specifically MAP.
While there are known factors associated with AD: cerebral ischemia, hypertension, type 2 diabetes metabolic syndrome, high and low body weight, tobacco use and traumatic brain injury (32), change to the microbiome is considered a newly added factor (33–35).
Microbial residents of the intestine are able to modulate the activities of distant sites including the brain via bidirectional communication of the GI tract through interactions between the enteric nervous system (ENS) and central nervous system (CNS) (36, 37). Metabolic products of a healthy microbiome are required for the optimal function of the CNS (38).
The largest reservoir of tissue macrophages in the body is contained within the gastrointestinal system. Macrophage response to bacterial presence results in phagocytosis. The response to MAP, however, is blunted due to the fact that MAP prevents phago-lysomal maturation allowing MAP to persist within the macrophage (39).
There is broad acceptance of mycobacterial presence in pulmonary disease, certainly for tuberculosis, and increasingly so for non-tuberculous mycobacteria (40). Conversely, it is unlikely that MAP would be reported in an enteric microbiome report: molecular targets fail to report or under report MAP as it is in low abundance and very difficult to extract from the cell (41, 42); the target genetic sequences are not MAP specific; assays are performed on the gut contents, not the submucosa where MAP thrives (43, 44); MAP overcomes its host, not by sheer numbers but by MAP’s own virulence factors (45).
The proposed mechanism by which MAP promotes AD is the following: MAP persists by inhibition of phagosome maturation, inhibition of toll-like receptor signaling and inhibition of interferon-gamma (IFN-γ) signaling by MAP (13), altered composition of the enteric microbial community by MAP “bio-load” causes and increased mucosal inflammation which loosens epithelial tight junctions, “leaky gut” (46, 47). This permits MAP and/or its byproducts to enter the circulation that contribute to a permeable blood-brain barrier (BBB) allowing their introduction into the brain wherein they produce inflammation and amyloid accumulation/aggregation promoting AD (30).
Although nascent, the study of gut bacteria metabolites upon the brain is an area of active investigation (48). In addition to regulation of both the intestinal barrier and the BBB, several gut microbiota metabolites are able to cross both the intestinal barrier and the BBB presenting a means of communication between the microbiota and the brain. An animal study looking at dietary polyphenols showed minimum absorption with the remainder extensively metabolized by the gut microbiota. Twenty-three polyphenol microbial metabolites were isolated then given intravenously; the brain was found to be a targeted organ for ten of the metabolites (49). Short-chain fatty acids (SCFAs), such as butyrate, are speculated to have a key role in microbiome brain communication; the SCFAs are produced in the gut by bacterial fermentation of dietary fiber (50). These key products of microbiome fermentation may directly or indirectly mediate these interactions via signaling routes including immune, endocrine neural and humoral pathways (50).
Humans lack the pathway to produce essential aromatic amino acids; for instance, humans rely on gut bacteria for biosynthesis of phenylalanine, tyrosine, and tryptophan. Multiple studies demonstrate reduced plasma tryptophan levels in probable AD and in clinical AD (51). MAP infection results in upregulation of the enzyme that controls tryptophan metabolism; indoleamine 2,3 –dioxygenase (IDO) levels are increased in MAP infected monocytes, infected ileum and in peripheral blood of infected ruminants. IDO breaks down tryptophan reflected by decreased plasma tryptophan levels and correlated with onset of clinical paratuberculosis (52). This offers a conceptual link between MAP infection and AD.
A recent longitudinal study measuring nine cytokines of 298 cognitively normal elderly found that a higher IFN-γ level was associated with slower cognitive decline independent of amyloid deposition (53). IFNγ plays a central role in immune defense against a variety of intracellular pathogens, including mycobacteria (54). MAP, specifically, inhibits IFN-γ signaling (13), presenting an additional plausible mechanism for the association or MAP and AD.
Only one country, India, has assessed the “bio-load” of MAP in a human population study: over 30% of nearly 30,000 tested positive for MAP: this represents a composite result: 1/3 of serum ELISA tests were positive (past or present exposure), 8.8% of blood samples tested positive by PCR and 22.4 of stool samples tested positive by PCR (55).
There are greater than 140 known Mycobacterium species; most of which are considered non-pathogenic or “environmental” (56). M. tuberculosis maintains such a prominent place within the species that all others are referred to as non-tuberculous mycobacteria (NTM), many of which have clinical significance particularly in immunocompromised individuals (57).
Based upon genetic sequencing, the mycobacteria responsible for tuberculosis, leprosy and paratuberculosis, are proposed to have gone through an “evolutionary bottleneck” about 10,000 years ago. It is speculated that this was due, in part, to the domestication of and living closely with animals (58). Two of these mycobacteria are well known and studied: tuberculosis has claimed more lives than any other bacterium and a third of the world population is latently infection with M. tuberculosis (59). M. leprae, responsible for leprosy, is literally biblical in presence and continues today. Official World Health Organization figures report there were more than 202,000 new cases in 2019 (60).
The third mycobacterial agent emerging through this evolutionary bottleneck, MAP, is the long-recognized cause of Johne’s disease. MAP infection known also as paratuberculosis, and recognized worldwide, is an enteric inflammatory infectious disease, mostly studied in ruminant animals: cattle, sheep, and goats.
It is very difficult to diagnose the MAP infection in the early, subclinical stage of the disease.
MAP will colonize the intestines of infected animals for years while the animal exhibits no symptoms. However, sub-clinically infected animals continue to shed MAP bacilli in their milk (61) and feces contaminating pastures, the environment, and the food chain (62).
A majority of the dairy herds in the United States and Europe have infected animals within the herd (63). Indeed, according to the USDA, the herd-level prevalence of MAP infection in US dairy herds has markedly increased from 21.6% in 1996 to 91.1% in 2007 (64).
As noted, paratuberculosis is a global disease. Extensive testing in India describes an increasing MAP “bio-load” in cattle (43%), buffalo (36%), goats (23%) and sheep (41%). Moreover, in this same geographic area, 30.8% of 28,291 humans (via serum ELISA, blood PCR and stool PCR) tested positive for MAP (48). Similarly, testing of ruminants in Saudi Arabia found MAP: 26% of sheep, 27% of goats, 30% of cattle and 15% of camels (65).
Milk and related dairy products are considered to be the primary source of MAP infection in humans (66); products from pasteurized milk constitute a risk as pasteurization only reduces the MAP load originally present in milk (66, 67). MAP is present in yogurt (68), cheese (69), muscle meat (70) and hamburger (71).
Though the link of MAP zoonosis to Crohn’s disease has been controversial for over one hundred years (13), validation of this association has come from studies showing Crohn’s disease resolution with anti-mycobacterial therapy targeted against MAP (72–75). Moreover, MAP is now linked to an increasing list of inflammatory and autoimmune diseases (13, 76). To date, MAP has been causally associated with granulomatous diseases: Crohn’s (77), sarcoidosis (78, 79) and Blau syndrome (80). Through molecular mimicry from mycobacterial heat shock protein (hsp65) (81), MAP induces autoantibodies in autoimmune diabetes (T1D) (82), multiple sclerosis (83, 84), autoimmune thyroiditis (85), lupus (86), rheumatoid arthritis (87, 88) and possibly, Sjogren’s syndrome (89).
Neuronal homeostasis is dependent upon autophagy; the soma of a neuron is the primary site for the degradative pathways while the axon, which extends to synaptic sites as far as a meter away, traffics the lysosome cargo to the soma to complete the degradative process (90). Axonal lysosomes are abundant but are separated from the soma by a selectivity filter that regulates trafficking of the lysosomes to the soma (91). Evidence suggests that amyloid deposits cause a local impairment of retrograde axonal transport of lysosomes leading the further amyloid accumulation.
Although an enormous effort has been given to develop AD therapies, there has been little success in finding effective treatments. Currently FDA-approved cholinesterase inhibitors and memantine, while addressing some AD symptoms, lack the ability to slow or stop disease progression (92). Considering the impact of dysfunctional autophagy on both pathogenic mycobacterial infection and AD, perhaps the most compelling argument for mycobacterial involvement in AD can be found in reviewing therapeutic agents that have been found to favorably impact AD and mycobacterial infection. A complex interaction between macrophages and pathogenic mycobacterial agents determines the outcome of an infection with these organisms (93). For example, MAP has the ability to retard lysosomal maturation by limiting acidification which improves its virulence and facilitates its survival (45). The remainder of this section identifies therapeutic agents that boost autophagy and by doing so, have potential benefit in both pathogenic mycobacterial infection and AD.
The mammalian target of rapamycin (mTOR) signaling pathway is a well described controller of autophagy. Rapamycin and related “rapalogs” are protein kinases that inhibit mTOR (94). As neurons are small, polarized and are post-mitotic they are sensitive to the accumulation of aggregated and damaged cellular proteins and as such are dependent upon efficient autophagy for survival (95). Mounting evidence suggests that AD may be related to mTOR protein synthesis and impaired autophagy (96, 97). Rapamycin is also an effective inhibitor of MAP; its benefit may have unknowingly been an anti-MAP antibiotic (98).
Everolimus, as a rapalog, inhibits mTOR and boots autophagy. It has been studied and showed benefit in animal models of AD (99, 100) as well as in mycobacterial infection (101, 102). This includes MAP infection wherein, like with rapamycin, it may act as an anti-MAP antibiotic (98). Both rapamycin and everolimus are from the macrolide antibiotic family of medications, amongst the most potent anti M. avium antibiotic families (103).
The silent information regulator 1 (SIRT1) function is linked to cellular metabolism and is activated by L-serine (104). SIRT1 induces autophagy (105) and plays a critical role in controlling mycobacterial disease (106). Activation of SIRT1 reduces the intracellular growth of both drug-susceptible and drug-resistant strains of M. tuberculosis and induces phagosome maturation fusion and autophagy in a SIRT1-dependent manner (107). Serine, a dietary amino acid, is currently being studied for early stage AD: ClinicalTrials.gov Identifier: NCT03062449, (108).
Vitamin D has had an increasingly recognized roll in an expanding variety of diseases including mycobacterial disease and AD; calcitriol causes a dose-dependent inhibition of MAP (109). Calcitriol induces the synthesis of the archetypical antimicrobial peptide LL-37, (cathelicidin), which enhances autophagy (110–113). Specific binding interactions between LL-37 and amyloid complexes may inhibit amyloid aggregation (114).
A 1992 epidemiological study revealed that Japanese patients treated with anti-mycobacterial drugs for leprosy had a significantly lower incidence of AD dementia compared with an untreated group (115). Moreover, subsequent histological analyses indicated that non-demented, treated Japanese leprosy patients aged over 70 years showed significantly lower levels of senile plaques in the brain than age-matched non-demented non-leprosy subjects (116, 117). These studies have brought attention to the anti-mycobacterial drugs rifampin and dapsone. Dapsone, an anti-leprosy drug, has neuroprotective effects (118); there is a single reported case of an individual who recovered from AD to mild cognitive impairment, MCI, while on dapsone (119).
Rifampin may have the greatest potential as a repurposed drug for AD (120). Rifampicin is a well-known antibiotic used in the treatment of mycobacterial infections including tuberculosis and leprosy. Rifampin is available for oral and intravenous use; rifampin induces autophagy (121). Clinical trials of therapies that target amyloid-β in patients with AD have revealed that initiating therapy after the onset of clinical symptoms has little effect on cognitive function (122–124) suggesting that preventive therapy should start prior to clinical symptoms. Treatment efforts with rifampin failed in cohorts of mild and moderate AD individuals (125). However, when rifampin was used in preclinical and prodromal AD, it showed preventative effects (126). This amplifies the need for novel plasma biomarkers that identify AD risk which then can be used in clinical trials of individuals with prodromal AD (127). As attractive as repurposed rifampin may be for AD clinical trials, it is notably hepatotoxic (128) and has multiple adverse drug-drug interactions (129).
The nasal route of drug administration has several advantages over oral or intravenous administration, which include non-invasiveness, self-administration, shorter time to onset of effect and higher bioavailability due to avoidance of hepatic first-pass metabolism (130).
Intranasal rifampin delivery has direct access to the brain due to the olfactory and trigeminal neural pathways that connect the nasal mucosa with the brain (131). Moreover, rifampin has advantages for the treatment of AD as it can cross the BBB preventing production of amyloid aggregates as well as amyloid-associated cellular toxicity (132, 133). The permeability-glycoprotein (P-gp) is considered the most important transporter modulating the entry of drugs into the central nervous system (CNS) (134); rifampin, as a potent P-gp inducer, facilitates reduced accumulation of amyloid (135). Thus, intranasal rifampin can access the brain directly via ante grade olfactory nerve access, retrograde trigeminal nerve access and via a favored permeability status through the BBB.
A further benefit of the anti-mycobacterial agent rifampin, as suggested from animal studies, is that it works favorably in carriers of the ApoE4 allele (136). Also, as with AD, rifampin has a neuroprotective role in Parkinson’s disease (137, 138). MAP has also been associated with Parkinson’s disease (139, 140).
Accumulating evidence indicates that AD is an age-related, metabolic disease. Impaired cerebral glucose metabolism is an invariant pathophysiological feature in AD and its occurrence precedes cognitive dysfunction and pathological alterations even for decades (141). Compared with age-matched controls, AD individuals show regional glucose metabolism impairment in parieto-temporal lobe, posterior cingulate cortex, and the frontal areas during disease progression (142). Some investigators refer to AD as “Type 3 diabetes mellitus” (143). Insulin has been implicated in clearance of amyloid across the BBB, in tau phosphorylation, and in memory via its effects on synaptic function and long-term potentiation (144).
There is a proposed bi-directional relationship between insulin resistance and mycobacterial infection (145, 146); this occurs to the degree that insulin resistance could be considered both a biomarker and risk factor for active mycobacterial infection (147). Insulin resistance is associated with Alzheimer’s disease reflected in a two to five fold increased probability of a type 2 diabetic developing AD (148).
As with rifampin, enhancing brain insulin function with intranasal delivery may be a viable approach to ameliorating AD symptoms and attenuating AD-related pathophysiologic processes (149); this is accomplished without perturbation of the peripheral glucose level as little of the intranasal peptide reaches the peripheral circulation (150). Unlike rifampin, cerebral insulin treatment appears to have less benefit for those carrying the ApoE4 allele(s) (151).
The primary use of BCG is for the prevention of tuberculosis (152). There is increasing evidence that BCG provides protection against NTM infections (153–155). This extends to leprosy (156). This is unsurprising as BCG, a live attenuated vaccine, shares epitopes with mycobacteria other than tuberculosis (157).
A recent population study found an inverse relationship between the incidence of Alzheimer’s disease and BCG vaccination. The populations studied showed a lower prevalence of AD in countries with high BCG coverage. The authors hypothesized that exposure to BCG decreases the prevalence of AD due to a modulation of the immune system. They proposed testing their hypothesis by evaluating bladder cancer patients who received BCG comparing them to bladder cancer patients for whom BCG was not part of their recommended treatment (158). They found that bladder cancer patients treated with BCG were significantly less likely to develop AD compared to those not similarly treated. The mean age at diagnosis of bladder cancer was 68 years. AD was diagnosed at a mean age of 84 years. BCG dramatically reduced the risk of developing AD. Those treated with BCG had four-fold less risk for developing AD compared to patients not treated with BCG. The authors state that confirmation of their retrospective study would support prospective studies of BCG in AD (159). A follow up multi-cohort study again showed protective benefit of intravesicular BCG and risk of AD; it also showed protection against Parkinson’s disease (160). Increasingly appreciated is the protective benefit of not only BCG, but also other live-attenuated vaccines against all-cause infection (161–163).
BCG vaccination for autoimmune diseases type 1 diabetes (T1D) and multiple sclerosis (MS) have shown benefit in these disparate diseases; both diseases associated with MAP: T1D (164, 165) and MS (166, 167) The positive response to BCG in T1D (168) and MS (169), may be due to a mitigation by BCG of the consequences of MAP infection.
The role of microbial agents in AD is gaining recognition. The current and projected demographics of AD is dire and mandates broad approaches to mitigate the impact AD, not only for individuals and families, but also for global social and health systems. MAP is closely related to the greatest pathogen in human history, M. tuberculosis, a microbe that continues to latently infect one third of the world population. MAP may have a role in AD. This article suggests steps to further investigate this potentially fertile line of inquiry: 1) determine population-based MAP “bio-load”, 2) use optimized blood-based biomarkers to determine AD risk, 3) test for MAP in those with elevated AD risk vs. healthy controls. Concurrently, interventions could be initiated to 1) eliminate MAP from animals, the environment, and the food chain, 2) initiate clinical trials to test iterations of anti-mycobacterial agents shown to have benefit for AD. Parsimoniously, when searching for new directions in the efforts against AD, look at the MAP.
The author confirms being the sole contributor of this work and has approved it for publication.
The author declares that the research was conducted in the absence of any commercial or financial relationships that could be construed as a potential conflict of interest.
All claims expressed in this article are solely those of the authors and do not necessarily represent those of their affiliated organizations, or those of the publisher, the editors and the reviewers. Any product that may be evaluated in this article, or claim that may be made by its manufacturer, is not guaranteed or endorsed by the publisher.
1. Boland B, Yu WH, Corti O, Mollereau B, Henriques A, Bezard E, et al. Promoting the Clearance of Neurotoxic Proteins in Neurodegenerative Disorders of Ageing. Nat Rev Drug Discov (2018) 17(9):660–88. doi: 10.1038/nrd.2018.109
2. Menzies FM, Fleming A, Caricasole A, Bento CF, Andrews SP, Ashkenazi A, et al. Autophagy and Neurodegeneration: Pathogenic Mechanisms and Therapeutic Opportunities. Neuron (2017) 93(5):1015–34. doi: 10.1016/j.neuron.2017.01.022
3. Mawuenyega KG, Sigurdson W, Ovod V, Munsell L, Kasten T, Morris JC, et al. Decreased Clearance of CNS Beta-Amyloid in Alzheimer’s Disease. Science (2010) 330(6012):1774. doi: 10.1126/science.1197623
4. Ferrer I. Diversity of Astroglial Responses Across Human Neurodegenerative Disorders and Brain Aging. Brain Pathol (2017) 27(5):645–74. doi: 10.1111/bpa.12538
5. Pareja ME, Colombo MI. Autophagic Clearance of Bacterial Pathogens: Molecular Recognition of Intracellular Microorganisms. Front Cell Infect Microbiol (2013) 3:54. doi: 10.3389/fcimb.2013.00054
6. Bah A, Vergne I. Macrophage Autophagy and Bacterial Infections. Front Immunol (2017) 8:1483. doi: 10.3389/fimmu.2017.01483
7. Ma Y, Galluzzi L, Zitvogel L, Kroemer G. Autophagy and Cellular Immune Responses. Immunity (2013) 39(2):211–27. doi: 10.1016/j.immuni.2013.07.017
8. Panza F, Lozupone M, Solfrizzi V, Watling M, Imbimbo BP. Time to Test Antibacterial Therapy in Alzheimer’s Disease. Brain (2019) 142(10):2905–29. doi: 10.1093/brain/awz244
9. Shoemark DK, Allen SJ. The Microbiome and Disease: Reviewing the Links Between the Oral Microbiome, Aging, and Alzheimer’s Disease. J Alzheimers Dis (2015) 43(3):725–38. doi: 10.3233/JAD-141170
10. Alonso R, Pisa D, Fernández-Fernández AM, Carrasco L. Infection of Fungi and Bacteria in Brain Tissue From Elderly Persons and Patients With Alzheimer’s Disease. Front Aging Neurosci (2018) 10:159. doi: 10.3389/fnagi.2018.00159
11. Fülöp T, Itzhaki RF, Balin BJ, Miklossy J, Barron AE. Role of Microbes in the Development of Alzheimer’s Disease: State of the Art - An International Symposium Presented at the 2017 IAGG Congress in San Francisco. Front Genet (2018) 9:362. doi: 10.3389/fgene.2018.00362
12. Bu XL, Yao XQ, Jiao SS, Zeng F, Liu YH, Xiang Y, et al. A Study on the Association Between Infectious Burden and Alzheimer’s Disease. Eur J Neurol (2015) 22(12):1519–25. doi: 10.1111/ene.12477
13. Ekundayo TC, Okoh AI. Systematic Assessment of Mycobacterium Avium Subspecies Paratuberculosis Infections From 1911-2019: A Growth Analysis of Association With Human Autoimmune Diseases. Microorganisms (2020) 8(8):1212. doi: 10.3390/microorganisms8081212
14. Sechi LA, Dow CT. Mycobacterium Avium Ss. Paratuberculosis Zoonosis - The Hundred Year War - Beyond Crohn’s Disease. Front Immunol (2015) 6:96. doi: 10.3389/fimmu.2015.00096
15. Agrawal G, Borody TJ, Chamberlin W. ‘Global Warming’ to Mycobacterium Avium Subspecies Paratuberculosis. Future Microbiol (2014) 9(7):829–32. doi: 10.2217/fmb.14.52
16. Franceschi C, Bonafè M, Valensin S, Olivieri F, De Luca M, Ottaviani E, et al. Inflamm-Aging. An Evolutionary Perspective on Immunosenescence. Ann NY Acad Sci (2000) 908:244–54. doi: 10.1111/j.1749-6632.2000.tb06651.x
17. de Magalhães JP, Curado J, Church GM. Meta-Analysis of Age-Related Gene Expression Profiles Identifies Common Signatures of Aging. Bioinformatics (2009) 25(7):875–81. doi: 10.1093/bioinformatics/btp073
18. Singh T, Newman AB. Inflammatory Markers in Population Studies of Aging. Ageing Res Rev (2011) 10(3):319–29. doi: 10.1016/j.arr.2010.11.002
19. Salminen A, Huuskonen J, Ojala J, Kauppinen A, Kaarniranta K, Suuronen T. Activation of Innate Immunity System During Aging: NF-kB Signaling Is the Molecular Culprit of Inflamm-Aging. Ageing Res Rev (2008) 7(2):83–105. doi: 10.1016/j.arr.2007.09.002
20. Salminen A, Kaarniranta K, Kauppinen A. Inflammaging: Disturbed Interplay Between Autophagy and Inflammasomes. Aging (Albany NY) (2012) 4(3):166–75. doi: 10.18632/aging.100444
21. Lindholm E, Bakhtadze E, Cilio C, Agardh E, Groop L, Agardh CD, et al. TNF and AGER Polymorphisms and Late Diabetic Complications. PloS One (2008) 3(6):e2546. doi: 10.1371/journal.pone.0002546
22. Holmes C, Cunningham C, Zotova E, Woolford J, Dean C, Kerr S, et al. Systemic Inflammation and Disease Progression in Alzheimer Disease. Neurology (2009) 73(10):768–74. doi: 10.1212/WNL.0b013e3181b6bb95
23. Martorana A, Bulati M, Buffa S, Pellicanò M, Caruso C, Candore G, et al. Immunosenescence, Inflammation and Alzheimer’s Disease. Longev Healthspan (2012) 1:8. doi: 10.1186/2046-2395-1-8
24. Fulop T, Witkowski JM, Pawelec G, Alan C, Larbi A. On the Immunological Theory of Aging. Interdiscip Top Gerontol (2014) 39:163–76. doi: 10.1159/000358904
25. Fülöp T, Dupuis G, Witkowski JM, Larbi A. The Role of Immunosenescence in the Development of Age-Related Diseases. Rev Invest Clin (2016) 68(2):84–91.
26. Wertheimer AM, Bennett MS, Park B, Uhrlaub JL, Martinez C, Pulko V, et al. Aging and Cytomegalovirus Infection Differentially and Jointly Affect Distinct Circulating T Cell Subsets in Humans. J Immunol (2014) 192(5):2143–55. doi: 10.4049/jimmunol.1301721
27. Terrazzini N, Bajwa M, Vita S, Thomas D, Smith H, Vescovini R, et al. Cytomegalovirus Infection Modulates the Phenotype and Functional Profile of the T-Cell Immune Response to Mycobacterial Antigens in Older Life. Exp Gerontol (2014) 54(100):94–100. doi: 10.1016/j.exger.2013.12.007
28. Müller J, Tanner R, Matsumiya M, Snowden MA, Landry B, Satti I, et al. Cytomegalovirus Infection Is a Risk Factor for Tuberculosis Disease in Infants. JCI Insight (2019) 4(23):e130090. doi: 10.1172/jci.insight.130090
29. Chandra S, Alam MT, Dey J, Sasidharan BCP, Ray U, Srivastava AK, et al. Healthy Gut, Healthy Brain: The Gut Microbiome in Neurodegenerative Disorders. Curr Top Med Chem (2020) 20(13):1142–53. doi: 10.2174/1568026620666200413091101
30. Bulgart HR, Neczypor EW, Wold LE, Mackos AR. Microbial Involvement in Alzheimer Disease Development and Progression. Mol Neurodegener (2020) 15(1):42. doi: 10.1186/s13024-020-00378-4
31. Soscia SJ, Kirby JE, Washicosky KJ, Tucker SM, Ingelsson M, Hyman B, et al. The Alzheimer’s Disease-Associated Amyloid Beta-Protein Is an Antimicrobial Peptide. PloS One (2010) 5(3):e9505. doi: 10.1371/journal.pone.0009505
32. Reitz C, Mayeux R. Alzheimer Disease: Epidemiology, Diagnostic Criteria, Risk Factors and Biomarkers. Biochem Pharmacol (2014) 88(4):640–51. doi: 10.1016/j.bcp.2013.12.024
33. Köhler CA, Maes M, Slyepchenko A, Berk M, Solmi M, Lanctôt KL, et al. The Gut-Brain Axis, Including the Microbiome, Leaky Gut and Bacterial Translocation: Mechanisms and Pathophysiological Role in Alzheimer’s Disease. Curr Pharm Des (2016) 22(40):6152–66. doi: 10.2174/1381612822666160907093807
34. Golde TE. Alzheimer Disease: Host Immune Defense, Amyloid-β Peptide and Alzheimer Disease. Nat Rev Neurol (2016) 12(8):433–4. doi: 10.1038/nrneurol.2016.105
35. Pistollato F, Sumalla Cano S, Elio I, Masias Vergara M, Giampieri F, Battino M. Role of Gut Microbiota and Nutrients in Amyloid Formation and Pathogenesis of Alzheimer Disease. Nutr Rev (2016) 74(10):624–34. doi: 10.1093/nutrit/nuw023
36. Carabotti M, Scirocco A, Maselli MA, Severi C. The Gut-Brain Axis: Interactions Between Enteric Microbiota, Central and Enteric Nervous Systems. Ann Gastroenterol (2015) 28(2):203–9.
37. Giau VV, Wu SY, Jamerlan A, An SSA, Kim SY, Hulme J. Gut Microbiota and Their Neuroinflammatory Implications in Alzheimer’s Disease. Nutrients (2018) 10(11):1765. doi: 10.3390/nu10111765
38. Erny D, Hrabě de Angelis AL, Jaitin D, Wieghofer P, Staszewski O, David E, et al. Host Microbiota Constantly Control Maturation and Function of Microglia in the CNS. Nat Neurosci (2015) 18(7):965–77. doi: 10.1038/nn.4030
39. Basler T, Brumshagen C, Beineke A, Goethe R, Bäumer W. Mycobacterium Avium Subspecies Impair Dendritic Cell Maturation. Innate Immun (2013) 19(5):451–61. doi: 10.1177/1753425912470291
40. Cowman SA, James P, Wilson R, Cookson WOC, Moffatt MF, Loebinger MR. Profiling Mycobacterial Communities in Pulmonary Nontuberculous Mycobacterial Disease. PloS One (2018) 13(12):e0208018. doi: 10.1371/journal.pone.0208018
41. Beinhauerova M, Beinhauerova M, McCallum S, Sellal E, Ricchi M, O’Brien R, et al. Development of a Reference Standard for the Detection and Quantification of Mycobacterium Avium Subsp. Paratuberculosis by Quantitative PCR. Sci Rep (2021) 11(1):11622. doi: 10.1038/s41598-021-90789-0
42. Epperson LE, Strong M. A Scalable, Efficient, and Safe Method to Prepare High Quality DNA From Mycobacteria and Other Challenging Cells. J Clin Tuberc Other Mycobact Dis (2020) 19:100150. doi: 10.1016/j.jctube.2020.100150
43. Chiodini RJ, Dowd SE, Davis B, Galandiuk S, Chamberlin WM, Kuenstner JT, et al. Crohn’s Disease may be Differentiated Into 2 Distinct Biotypes Based on the Detection of Bacterial Genomic Sequences and Virulence Genes Within Submucosal Tissues. J Clin Gastroenterol (2013) 47(7):612–20. doi: 10.1097/MCG.0b013e31827b4f94
44. Suárez Fernández G. Enteritis Paratuberculosa Y Enfermedad De Crohn [Paratuberculose Enteritis and Crohn’s Disease]. An R Acad Nac Med (Madr) (2007) 124(2):221–34; discussion 234-40. Spanish.
45. Souza C, Davis WC, Eckstein TM, Sreevatsan S, Weiss DJ. Mannosylated Lipoarabinomannans From Mycobacterium Avium Subsp. Paratuberculosis Alters the Inflammatory Response by Bovine Macrophages and Suppresses Killing of Mycobacterium Avium Subsp. Avium Organisms. PloS One (2013) 8(9):e75924. doi: 10.1371/journal.pone.0075924
46. Leblhuber F, Geisler S, Steiner K, Fuchs D, Schütz B. Elevated Fecal Calprotectin in Patients With Alzheimer’s Dementia Indicates Leaky Gut. J Neural Transm (Vienna) (2015) 122(9):1319–22. doi: 10.1007/s00702-015-1381-9
47. Thevaranjan N, Puchta A, Schulz C, Naidoo A, Szamosi JC, Verschoor CP, et al. Age-Associated Microbial Dysbiosis Promotes Intestinal Permeability, Systemic Inflammation, and Macrophage Dysfunction. Cell Host Microbe (2017) 21(4):455–66.e4. doi: 10.1016/j.chom.2017.03.002
48. Tran SM, Mohajeri MH. The Role of Gut Bacterial Metabolites in Brain Development, Aging and Disease. Nutrients (2021) 13(3):732. doi: 10.3390/nu13030732
49. Gasperotti M, Passamonti S, Tramer F, Masuero D, Guella G, Mattivi F, et al. Fate of Microbial Metabolites of Dietary Polyphenols in Rats: Is the Brain Their Target Destination? ACS Chem Neurosci (2015) 6(8):1341–52. doi: 10.1021/acschemneuro.5b00051
50. Dalile B, Van Oudenhove L, Vervliet B, Verbeke K. The Role of Short-Chain Fatty Acids in Microbiota-Gut-Brain Communication. Nat Rev Gastroenterol Hepatol (2019) 16(8):461–78. doi: 10.1038/s41575-019-0157-3
51. Paley EL, Merkulova-Rainon T, Faynboym A, Shestopalov VI, Aksenoff I. Geographical Distribution and Diversity of Gut Microbial NADH:Ubiquinone Oxidoreductase Sequence Associated With Alzheimer’s Disease. J Alzheimers Dis (2018) 61(4):1531–40. doi: 10.3233/JAD-170764
52. Plain KM, de Silva K, Earl J, Begg DJ, Purdie AC, Whittington RJ. Indoleamine 2,3-Dioxygenase, Tryptophan Catabolism, and Mycobacterium Avium Subsp. Paratuberculosis: A Model for Chronic Mycobacterial Infections. Infect Immun (2011) 79(9):3821–32. doi: 10.1128/IAI.05204-11
53. Yang HS, Zhang C, Carlyle BC, Zhen SY, Trombetta BA, Schultz AP, et al. Plasma IL-12/IFN-γ Axis Predicts Cognitive Trajectories in Cognitively Unimpaired Older Adults. Alzheimers Dement (2021). doi: 10.1002/alz.12399
54. Dorman SE, Holland SM. Mutation in the Signal-Transducing Chain of the Interferon-Gamma Receptor and Susceptibility to Mycobacterial Infection. J Clin Invest (1998) 101(11):2364–9. doi: 10.1172/JCI2901
55. Chaubey KK, Singh SV, Gupta S, Singh M, Sohal JS, Kumar N, et al. Mycobacterium Avium Subspecies Paratuberculosis - an Important Food Borne Pathogen of High Public Health Significance With Special Reference to India: An Update. Vet Q (2017) 37(1):282–99. doi: 10.1080/01652176.2017.1397301
56. Porvaznik I, Solovič I, Mokrý J. Non-Tuberculous Mycobacteria: Classification, Diagnostics, and Therapy. Adv Exp Med Biol (2017) 944:19–25. doi: 10.1007/5584_2016_45
57. Gopalaswamy R, Shanmugam S, Mondal R, Subbian S. Of Tuberculosis and non-Tuberculous Mycobacterial Infections - A Comparative Analysis of Epidemiology, Diagnosis and Treatment. J BioMed Sci (2020) 27(1):74. doi: 10.1186/s12929-020-00667-6
58. Frothingham R. Evolutionary Bottlenecks in the Agents of Tuberculosis, Leprosy, and Paratuberculosis. Med Hypotheses (1999) 52(2):95–9. doi: 10.1054/mehy.1997.0622
59. Available at: https://www.who.int/en/news-room/fact-sheets/detail/tuberculosis (Accessed 7.1.2021).
60. Available at: https://www.who.int/en/news-room/fact-sheets/detail/leprosy (Accessed 7.1.2021).
61. Shankar H, Singh SV, Singh PK, Singh AV, Sohal JS, Greenstein RJ. Presence, Characterization, and Genotype Profiles of Mycobacterium Avium Subspecies Paratuberculosis From Unpasteurized Individual and Pooled Milk, Commercial Pasteurized Milk, and Milk Products in India by Culture, PCR, and PCR-REA Methods. Int J Infect Dis (2010) 14(2):e121–6. doi: 10.1016/j.ijid.2009.03.031
62. Waddell L, Rajić A, Stärk K, McEwen SA. Mycobacterium Avium Ssp. Paratuberculosis Detection in Animals, Food, Water and Other Sources or Vehicles of Human Exposure: A Scoping Review of the Existing Evidence. Prev Vet Med (2016) 132:32–48. doi: 10.1016/j.prevetmed.2016.08.003
63. Stabel JR. Johne’s Disease: A Hidden Threat. J Dairy Sci (1998) 81(1):283–8. doi: 10.3168/jds.S0022-0302(98)75577-8
64. Lombard JE, Gardner IA, Jafarzadeh SR, Fossler CP, Harris B, Capsel RT, et al. Herd-Level Prevalence of Mycobacterium Avium Subsp. Paratuberculosis Infection in United States Dairy Herds in 2007. Prev Vet Med (2013) 108(2-3):234–8. doi: 10.1016/j.prevetmed.2012.08.006
65. Elsohaby I, Fayez M, Alkafafy M, Refaat M, Al Marri T, Alaql FA, et al. Serological and Molecular Characterization of Mycobacterium Avium Subsp. Paratuberculosis (MAP) From Sheep, Goats, Cattle and Camels in the Eastern Province, Saudi Arabia. Animals (Basel) (2021) 11(2):323. doi: 10.3390/ani11020323
66. Gill CO, Saucier L, Meadus WJ. Mycobacterium Avium Subsp. Paratuberculosis in Dairy Products, Meat, and Drinking Water. J Food Prot (2011) 74(3):480–99. doi: 10.4315/0362-028X.JFP-10-301
67. Eltholth MM, Marsh VR, Van Winden S, Guitian FJ. Contamination of Food Products With Mycobacterium Avium Paratuberculosis: A Systematic Review. J Appl Microbiol (2009) 107(4):1061–71. doi: 10.1111/j.1365-2672.2009.04286.x
68. Van Brandt L, Coudijzer K, Herman L, Michiels C, Hendrickx M, Vlaemynck G. Survival of Mycobacterium Avium Ssp. Paratuberculosis in Yoghurt and in Commercial Fermented Milk Products Containing Probiotic Cultures. J Appl Microbiol (2011) 110(5):1252–61. doi: 10.1111/j.1365-2672.2011.04979.x
69. Galiero A, Fratini F, Mataragka A, Turchi B, Nuvoloni R, Ikonomopoulos J, et al. Detection of Mycobacterium Avium Subsp. Paratuberculosis in Cheeses From Small Ruminants in Tuscany. Int J Food Microbiol (2016) 217:195–9. doi: 10.1016/j.ijfoodmicro.2015.10.029
70. Alonso-Hearn M, Molina E, Geijo M, Vazquez P, Sevilla I, Garrido JM, et al. Isolation of Mycobacterium Avium Subsp. Paratuberculosis From Muscle Tissue of Naturally Infected Cattle. Foodborne Pathog Dis (2009) 6(4):513–8. doi: 10.1089/fpd.2008.0226
71. Hammer P, Walte HG, Matzen S, Hensel J, Kiesner C. Inactivation of Mycobacterium Avium Subsp. Paratuberculosis During Cooking of Hamburger Patties. J Food Prot (2013) 76(7):1194–201. doi: 10.4315/0362-028X.JFP-12-474
72. Qasem A, Elkamel E, Naser SA. Anti-MAP Triple Therapy Supports Immunomodulatory Therapeutic Response in Crohn’s Disease Through Downregulation of NF-κB Activation in the Absence of MAP Detection. Biomedicines (2020) 8(11):513. doi: 10.3390/biomedicines8110513
73. Agrawal G, Clancy A, Huynh R, Borody T. Profound Remission in Crohn’s Disease Requiring No Further Treatment for 3-23 Years: A Case Series. Gut Pathog (2020) 12:16. doi: 10.1186/s13099-020-00355-8
74. Savarino E, Bertani L, Ceccarelli L, Bodini G, Zingone F, Buda A, et al. Antimicrobial Treatment With the Fixed-Dose Antibiotic Combination RHB-104 for Mycobacterium Avium Subspecies Paratuberculosis in Crohn’s Disease: Pharmacological and Clinical Implications. Expert Opin Biol Ther (2019) 19(2):79–88. doi: 10.1080/14712598.2019.1561852
75. Borody TJ, Bilkey S, Wettstein AR, Leis S, Pang G, Tye S. Anti-Mycobacterial Therapy in Crohn’s Disease Heals Mucosa With Longitudinal Scars. Dig Liver Dis (2007) 39(5):438–44. doi: 10.1016/j.dld.2007.01.008
76. Dow CT, Sechi LA. Cows Get Crohn’s Disease and They’re Giving Us Diabetes. Microorganisms (2019) 7(10):466. doi: 10.3390/microorganisms7100466
77. Kuenstner JT, Naser S, Chamberlin W, Borody T, Graham DY, McNees A, et al. The Consensus From the Mycobacterium Avium Ssp. Paratuberculosis (MAP) Conference 2017. Front Public Health (2017) 5:208. doi: 10.3389/fpubh.2017.00208
78. Celler BG. Case Study: Cardiac Sarcoidosis Resolved With Mycobacterium Avium Paratuberculosis Antibiotics (MAP). Sarcoidosis Vasc Diffuse Lung Dis (2018) 35(2):171–7. doi: 10.36141/svdld.v35i2.6769
79. Reid JD, Chiodini RJ. Serologic Reactivity Against Mycobacterium Paratuberculosis Antigens in Patients With Sarcoidosis. Sarcoidosis (1993) 10(1):32–5.
80. Dow CT, Ellingson JL. Detection of Mycobacterium Avium Ss. Paratuberculosis in Blau Syndrome Tissues. Autoimmune Dis (2010) 2011:127692. doi: 10.4061/2010/127692
81. Dow CT. M. Paratuberculosis Heat Shock Protein 65 and Human Diseases: Bridging Infection and Autoimmunity. Autoimmune Dis (2012) 2012:150824. doi: 10.1155/2012/150824
82. Naser SA, Thanigachalam S, Dow CT, Collins MT. Exploring the Role of Mycobacterium Avium Subspecies Paratuberculosis in the Pathogenesis of Type 1 Diabetes Mellitus: A Pilot Study. Gut Pathog (2013) 5:14. doi: 10.1186/1757-4749-5-14
83. Cossu D, Masala S, Sechi LA. A Sardinian Map for Multiple Sclerosis. Future Microbiol (2013) 8(2):223–32. doi: 10.2217/fmb.12.135
84. Bo M, Niegowska M, Arru G, Sechi E, Mariotto S, Mancinelli C, et al. Mycobacterium Avium Subspecies Paratuberculosis and Myelin Basic Protein Specific Epitopes Are Highly Recognized by Sera From Patients With Neuromyelitis Optica Spectrum Disorder. J Neuroimmunol (2018) 318:97–102. doi: 10.1016/j.jneuroim.2018.02.013
85. Sisto M, Cucci L, D’Amore M, Dow TC, Mitolo V, Lisi S. Proposing a Relationship Between Mycobacterium Avium Subspecies Paratuberculosis Infection and Hashimoto’s Thyroiditis. Scand J Infect Dis (2010) 42(10):787–90. doi: 10.3109/00365541003762306
86. Dow CT. Detection of M. Paratuberculosis Bacteremia in a Child With Lupus Erythematosus and Sjogren’s Syndrome. Autoimmun Infect Dis (2016) 2(1). doi: 10.16966/2470-1025.111
87. Bo M, Erre GL, Bach H, Slavin YN, Manchia PA, Passiu G, et al. PtpA and PknG Proteins Secreted by Mycobacterium Avium Subsp. Paratuberculosis Are Recognized by Sera From Patients With Rheumatoid Arthritis: A Case-Control Study. J Inflamm Res (2019) 12:301–8. doi: 10.2147/JIR.S220960
88. Bo M, Erre GL, Niegowska M, Piras M, Taras L, Longu MG, et al. Interferon Regulatory Factor 5 Is a Potential Target of Autoimmune Response Triggered by Epstein-barr Virus and Mycobacterium Avium Subsp. Paratuberculosis in Rheumatoid Arthritis: Investigating a Mechanism of Molecular Mimicry. Clin Exp Rheumatol (2018) 36(3):376–81.
89. Dow CT, Chan ED. What Is the Evidence That Mycobacteria Are Associated With the Pathogenesis of Sjogren’s Syndrome? J Transl Autoimmun (2021) 4:100085. doi: 10.1016/j.jtauto.2021.100085
90. Stavoe AKH, Holzbaur ELF. Axonal Autophagy: Mini-Review for Autophagy in the CNS. Neurosci Lett (2019) 697:17–23. doi: 10.1016/j.neulet.2018.03.025
91. Gowrishankar S, Yuan P, Wu Y, Schrag M, Paradise S, Grutzendler J, et al. Massive Accumulation of Luminal Protease-Deficient Axonal Lysosomes at Alzheimer’s Disease Amyloid Plaques. Proc Natl Acad Sci USA (2015) 112(28):E3699–708. doi: 10.1073/pnas.1510329112
92. Iqbal UH, Zeng E, Pasinetti GM. The Use of Antimicrobial and Antiviral Drugs in Alzheimer’s Disease. Int J Mol Sci (2020) 21(14):4920. doi: 10.3390/ijms21144920
93. Weiss DJ, Evanson OA, Deng M, Abrahamsen MS. Sequential Patterns of Gene Expression by Bovine Monocyte-Derived Macrophages Associated With Ingestion of Mycobacterial Organisms. Microb Pathog (2004) 37(4):215–24. doi: 10.1016/j.micpath.2004.07.001
94. Ballou LM, Lin RZ. Rapamycin and mTOR Kinase Inhibitors. J Chem Biol (2008) 1(1-4):27–36. doi: 10.1007/s12154-008-0003-5
95. Tooze SA, Schiavo G. Liaisons Dangereuses: Autophagy, Neuronal Survival and Neurodegeneration. Curr Opin Neurobiol (2008) 18(5):504–15. doi: 10.1016/j.conb.2008.09.015
96. Bové J, Martínez-Vicente M, Vila M. Fighting Neurodegeneration With Rapamycin: Mechanistic Insights. Nat Rev Neurosci (2011) 12(8):437–52. doi: 10.1038/nrn3068
97. Cai Z, Zhao B, Li K, Zhang L, Li C, Quazi SH, et al. Mammalian Target of Rapamycin: A Valid Therapeutic Target Through the Autophagy Pathway for Alzheimer’s Disease? J Neurosci Res (2012) 90(6):1105–18. doi: 10.1002/jnr.23011
98. Greenstein RJ, Su L, Juste RA, Brown ST. On the Action of Cyclosporine A, Rapamycin and Tacrolimus on M. Avium Including Subspecies Paratuberculosis. PloS One (2008) 3(6):e2496. doi: 10.1371/journal.pone.0002496
99. Cassano T, Magini A, Giovagnoli S, Polchi A, Calcagnini S, Pace L, et al. Early Intrathecal Infusion of Everolimus Restores Cognitive Function and Mood in a Murine Model of Alzheimer’s Disease. Exp Neurol (2019) 311:88–105. doi: 10.1016/j.expneurol.2018.09.011
100. Fanoudi S, Hosseini M, Alavi MS, Boroushaki MT, Hosseini A, Sadeghnia HR. Everolimus, a Mammalian Target of Rapamycin Inhibitor, Ameliorated Streptozotocin-Induced Learning and Memory Deficits Via Neurochemical Alterations in Male Rats. EXCLI J (2018) 17:999–1017. doi: 10.17179/excli2018-1626
101. Cerni S, Shafer D, To K, Venketaraman V. Investigating the Role of Everolimus in Mtor Inhibition and Autophagy Promotion as a Potential Host-Directed Therapeutic Target in Mycobacterium Tuberculosis Infection. J Clin Med (2019) 8(2):232. doi: 10.3390/jcm8020232
102. Ashley D, Hernandez J, Cao R, To K, Yegiazaryan A, Abrahem R, et al. Antimycobacterial Effects of Everolimus in a Human Granuloma Model. J Clin Med (2020) 9(7):2043. doi: 10.3390/jcm9072043
103. Barrow WW, Wright EL, Goh KS, Rastogi N. Activities of Fluoroquinolone, Macrolide, and Aminoglycoside Drugs Combined With Inhibitors of Glycosylation and Fatty Acid and Peptide Biosynthesis Against Mycobacterium Avium. Antimicrob Agents Chemother (1993) 37(4):652–61. doi: 10.1128/AAC.37.4.652
104. Sim WC, Kim DG, Lee W, Sim H, Choi YJ, Lee BH. Activation of SIRT1 by L-serine Increases Fatty Acid Oxidation and Reverses Insulin Resistance in C2C12 Myotubes. Cell Biol Toxicol (2019) 35(5):457–70. doi: 10.1007/s10565-019-09463-x
105. Ren Q, Hu Z, Jiang Y, Tan X, Botchway BOA, Amin N, et al. Sirt1 Protects Against Apoptosis by Promoting Autophagy in the Oxygen Glucose Deprivation/Reperfusion-Induced Injury. Front Neurol (2019) 10:1289. doi: 10.3389/fneur.2019.01289
106. Yang H, Hu J, Chen YJ, Ge B. Role of Sirt1 in Innate Immune Mechanisms Against Mycobacterium Tuberculosis Via the Inhibition of TAK1 Activation. Arch Biochem Biophys (2019) 667:49–58. doi: 10.1016/j.abb.2019.04.006
107. Cheng CY, Gutierrez NM, Marzuki MB, Lu X, Foreman TW, Paleja B, et al. Host Sirtuin 1 Regulates Mycobacterial Immunopathogenesis and Represents a Therapeutic Target Against Tuberculosis. Sci Immunol (2017) 2(9):eaaj1789. doi: 10.1126/sciimmunol.aaj1789
108. Metcalf JS, Dunlop RA, Powell JT, Banack SA, Cox PA. L-Serine: A Naturally-Occurring Amino Acid With Therapeutic Potential. Neurotox Res (2018) 33(1):213–21. doi: 10.1007/s12640-017-9814-x
109. Greenstein RJ, Su L, Brown ST. Vitamins A & D Inhibit the Growth of Mycobacteria in Radiometric Culture. PloS One (2012) 7(1):e29631. doi: 10.1371/journal.pone.0029631
110. Shapira Y, Agmon-Levin N, Shoenfeld Y. Mycobacterium Tuberculosis, Autoimmunity, and Vitamin D. Clin Rev Allergy Immunol (2010) 38(2-3):169–77. doi: 10.1007/s12016-009-8150-1
111. Jayedi A, Rashidy-Pour A, Shab-Bidar S. Vitamin D Status and Risk of Dementia and Alzheimer’s Disease: A Meta-Analysis of Dose-Response †. Nutr Neurosci (2019) 22(11):750–9. doi: 10.1080/1028415X.2018.1436639
112. Morello M, Pieri M, Zenobi R, Talamo A, Stephan D, Landel V, et al. The Influence of Vitamin D on Neurodegeneration and Neurological Disorders: A Rationale for its Physio-pathological Actions. Curr Pharm Des (2020) 26(21):2475–91. doi: 10.2174/1381612826666200316145725
113. Periyasamy KM, Ranganathan UD, Tripathy SP, Bethunaickan R. Vitamin D - A Host Directed Autophagy Mediated Therapy for Tuberculosis. Mol Immunol (2020) 127:238–44. doi: 10.1016/j.molimm.2020.08.007
114. De Lorenzi E, Chiari M, Colombo R, Cretich M, Sola L, Vanna R, et al. Evidence That the Human Innate Immune Peptide LL-37 may be a Binding Partner of Amyloid-β and Inhibitor of Fibril Assembly. J Alzheimers Dis (2017) 59(4):1213–26. doi: 10.3233/JAD-170223
115. McGeer PL, Harada N, Kimura H, McGeer EG, Schulzer M. Prevalence of Dementia Amongst Elderly Japanese With Leprosy: Apparent Effect of Chronic Drug Therapy. Dementia (1992) 3:146–9. doi: 10.1159/000107010
116. Namba Y, Kawatsu K, Izumi S, Ueki A, Ikeda K. Neurofibrillary Tangles and Senile Plaques in Brain of Elderly Leprosy Patients. Lancet (1992) 340:978. doi: 10.1016/0140-6736(92)92870-L
117. Chui DH, Tabira T, Izumi S, Koya G, Ogata J. Decreased B-Amyloid and Increased Abnormal Tau Deposition in the Brain of Aged Patients With Leprosy. Am J Pathol (1994) 145:771–5.
118. Yang N, Li L, Li Z, Ni C, Cao Y, Liu T, et al. Protective Effect of Dapsone on Cognitive Impairment Induced by Propofol Involves Hippocampal Autophagy. Neurosci Lett (2017) 649:85–92. doi: 10.1016/j.neulet.2017.04.019
119. Liang Y, Zheng D, Peng S, Lin D, Jing X, Zeng Z, et al. Rifampicin Attenuates Rotenone-Treated Microglia Inflammation Via Improving Lysosomal Function. Toxicol In Vitro (2020) 63:104690. doi: 10.1016/j.tiv.2019.104690
120. Umeda T, Ono K, Sakai A, Yamashita M, Mizuguchi M, Klein WL, et al. Rifampicin Is a Candidate Preventive Medicine Against Amyloid-β and Tau Oligomers. Brain (2016) 139(Pt 5):1568–86. doi: 10.1093/brain/aww042
121. Lee JH, Choi SH, Lee CJ, Oh SS. Recovery of Dementia Syndrome Following Treatment of Brain Inflammation. Dement Geriatr Cogn Dis Extra (2020) 10(1):1–12. doi: 10.1159/000504880
122. Imbimbo BP, Giardina GA. γ-Secretase Inhibitors and Modulators for the Treatment of Alzheimer’s Disease: Disappointments and Hopes. Curr Top Med Chem (2011) 11(12):1555–70. doi: 10.2174/156802611795860942
123. Sperling RA, Jack CR Jr, Aisen PS. Testing the Right Target and Right Drug at the Right Stage. Sci Transl Med (2011) 3(111):111cm33. doi: 10.1126/scitranslmed.3002609
124. Panza F, Logroscino G, Imbimbo BP, Solfrizzi V. Is There Still Any Hope for Amyloid-Based Immunotherapy for Alzheimer’s Disease? Curr Opin Psychiatry (2014) 27(2):128–37. doi: 10.1097/YCO.0000000000000041
125. Loeb MB, Molloy DW, Smieja M, Standish T, Goldsmith CH, Mahony J, et al. A Randomized, Controlled Trial of Doxycycline and Rifampin for Patients With Alzheimer’s Disease. J Am Geriatr Soc (2004) 52(3):381–7. doi: 10.1111/j.1532-5415.2004.52109.x
126. Iizuka T, Morimoto K, Sasaki Y, Kameyama M, Kurashima A, Hayasaka K, et al. Preventive Effect of Rifampicin on Alzheimer Disease Needs at Least 450 Mg Daily for 1 Year: An FDG-PET Follow-Up Study. Dement Geriatr Cogn Dis Extra (2017) 7(2):204–14. doi: 10.1159/000477343
127. West T, Kirmess KM, Meyer MR, Holubasch MS, Knapik SS, Hu Y, et al. A Blood-Based Diagnostic Test Incorporating Plasma Aβ42/40 Ratio, ApoE Proteotype, and Age Accurately Identifies Brain Amyloid Status: Findings From a Multi Cohort Validity Analysis. Mol Neurodegener (2021) 16(1):30. doi: 10.1186/s13024-021-00451-6
128. Available at: https://livertox.nih.gov/Rifampin.htm (Accessed 7.1.21).
129. Available at: https://www.drugs.com/drug-interactions/rifampin.html (Accessed 5.1.21).
130. Erdő F, Bors LA, Farkas D, Bajza Á, Gizurarson S. Evaluation of Intranasal Delivery Route of Drug Administration for Brain Targeting. Brain Res Bull (2018) 143:155–70. doi: 10.1016/j.brainresbull.2018.10.009
131. Jiang Y, Li Y, Liu X. Intranasal Delivery: Circumventing the Iron Curtain to Treat Neurological Disorders. Expert Opin Drug Deliv (2015) 12(11):1717–25. doi: 10.1517/17425247.2015.1065812
132. Tomiyama T, Asano S, Suwa Y, Morita T, Kataoka K, Mori H, et al. Rifampicin Prevents the Aggregation and Neurotoxicity of Amyloid Beta Protein In Vitro. Biochem Biophys Res Commun (1994) 204(1):76–83. doi: 10.1006/bbrc.1994.2428
133. Tomiyama T, Shoji A, Kataoka K, Suwa Y, Asano S, Kaneko H, et al. Inhibition of Amyloid Beta Protein Aggregation and Neurotoxicity by Rifampicin. Its Possible Function as a Hydroxyl Radical Scavenger. J Biol Chem (1996) 271(12):6839–44. doi: 10.1074/jbc.271.12.6839
134. Sun H, Dai H, Shaik N, Elmquist WF. Drug Efflux Transporters in the CNS. Adv Drug Deliv Rev (2003) 55(1):83–105. doi: 10.1016/s0169-409x(02)00172-2
135. Abuznait AH, Cain C, Ingram D, Burk D, Kaddoumi A. Up-Regulation of P-glycoprotein Reduces Intracellular Accumulation of Beta Amyloid: Investigation of P-glycoprotein as a Novel Therapeutic Target for Alzheimer’s Disease. J Pharm Pharmacol (2011) 63(8):1111–8. doi: 10.1111/j.2042-7158.2011.01309.x
136. Lin AL, Parikh I, Yanckello LM, White RS, Hartz AMS, Taylor CE, et al. APOE Genotype-Dependent Pharmacogenetic Responses to Rapamycin for Preventing Alzheimer’s Disease. Neurobiol Dis (2020) 139:104834. doi: 10.1016/j.nbd.2020.104834
137. Bi W, Zhu L, Jing X, Liang Y, Tao E. Rifampicin and Parkinson’s Disease. Neurol Sci (2013) 34(2):137–41. doi: 10.1007/s10072-012-1156-0
138. Li J, Zhu M, Rajamani S, Uversky VN, Fink AL. Rifampicin Inhibits Alpha-Synuclein Fibrillation and Disaggregates Fibrils. Chem Biol (2004) 11(11):1513–21. doi: 10.1016/j.chembiol.2004.08.025
139. Dow CT. M. Paratuberculosis and Parkinson’s Disease–Is This a Trigger. Med Hypotheses (2014) 83(6):709–12. doi: 10.1016/j.mehy.2014.09.025
140. Arru G, Caggiu E, Paulus K, Sechi GP, Mameli G, Sechi LA. Is There a Role for Mycobacterium Avium Subspecies Paratuberculosis in Parkinson’s Disease? J Neuroimmunol (2016) 293:86–90. doi: 10.1016/j.jneuroim.2016.02.016
141. Chen Z, Zhong C. Decoding Alzheimer’s Disease From Perturbed Cerebral Glucose Metabolism: Implications for Diagnostic and Therapeutic Strategies. Prog Neurobiol (2013) 108:21–43. doi: 10.1016/j.pneurobio.2013.06.004
142. Minoshima S, Giordani B, Berent S, Frey KA, Foster NL, Kuhl DE. Metabolic Reduction in the Posterior Cingulate Cortex in Very Early Alzheimer’s Disease. Ann Neurol (1997) 42(1):85–94. doi: 10.1002/ana.410420114
143. de la Monte SM, Wands JR. Alzheimer’s Disease Is Type 3 Diabetes-Evidence Reviewed. J Diabetes Sci Technol (2008) 2(6):1101–13. doi: 10.1177/193229680800200619
144. Ribe EM, Lovestone S. Insulin Signaling in Alzheimer’s Disease and Diabetes: From Epidemiology to Molecular Links. J Intern Med (2016) 280(5):430–42. doi: 10.1111/joim.12534
145. Jeon CY, Harries AD, Baker MA, Hart JE, Kapur A, Lönnroth K, et al. Bi-Directional Screening for Tuberculosis and Diabetes: A Systematic Review. Trop Med Int Health (2010) 15(11):1300–14. doi: 10.1111/j.1365-3156.2010.02632.x
146. Philips L, Visser J, Nel D, Blaauw R. The Association Between Tuberculosis and the Development of Insulin Resistance in Adults With Pulmonary Tuberculosis in the Western Sub-District of the Cape Metropole Region, South Africa: A Combined Cross-Sectional, Cohort Study. BMC Infect Dis (2017) 17(1):570. doi: 10.1186/s12879-017-2657-5
147. Mao F, Chen T, Zhao Y, Zhang C, Bai B, Zhao S, et al. Insulin Resistance: A Potential Marker and Risk Factor for Active Tuberculosis? Med Hypotheses (2011) 77(1):66–8. doi: 10.1016/j.mehy.2011.03.025
148. Biessels GJ, Despa F. Cognitive Decline and Dementia in Diabetes Mellitus: Mechanisms and Clinical Implications. Nat Rev Endocrinol (2018) 14(10):591–604. doi: 10.1038/s41574-018-0048-7
149. Craft S, Claxton A, Baker LD, Hanson AJ, Cholerton B, Trittschuh EH, et al. Effects of Regular and Long-Acting Insulin on Cognition and Alzheimer’s Disease Biomarkers: A Pilot Clinical Trial. J Alzheimers Dis (2017) 57(4):1325–34. doi: 10.3233/JAD-161256
150. Born J, Lange T, Kern W, McGregor GP, Bickel U, Fehm HL. Sniffing Neuropeptides: A Transnasal Approach to the Human Brain. Nat Neurosci (2002) 5(6):514–6. doi: 10.1038/nn849
151. Reger MA, Watson GS, Frey WH 2nd, Baker LD, Cholerton B, Keeling ML, et al. Effects of Intranasal Insulin on Cognition in Memory-Impaired Older Adults: Modulation by APOE Genotype. Neurobiol Aging (2006) 27(3):451–8. doi: 10.1016/j.neurobiolaging.2005.03.016
152. Griffin JF, Chinn DN, Rodgers CR, Mackintosh CG. Optimal Models to Evaluate the Protective Efficacy of Tuberculosis Vaccines. Tuberculosis (2001) 81:133–9. doi: 10.1054/tube.2000.0271
153. Abate G, Hamzabegovic F, Eickhoff CS, Hoft DF. BCG Vaccination Induces M. Avium and M. Abscessus Cross-Protective Immunity. Front Immunol (2019) 10:234. doi: 10.3389/fimmu.2019.00234
154. Kontturi A, Soini H, Ollgren J, Salo E. Increase in Childhood Nontuberculous Mycobacterial Infections After Bacille Calmette-Guérin Coverage Drop: A Nationwide, Population-Based Retrospective Study, Finland, 1995-2016. Clin Infect Dis (2018) 67:1256–61. doi: 10.1093/cid/ciy241
155. Trnka L, Danková D, Svandová E. Six Years’ Experience With the Discontinuation of BCG Vaccination. 4. Protective Effect of BCG Vaccination Against the Mycobacterium Avium Intracellulare Complex. Tuber Lung Dis (1994) 75:348–52. doi: 10.1016/0962-8479(94)90080-9
156. Setia MS, Steinmaus C, Ho CS, Rutherford GW. The Role of BCG in Prevention of Leprosy: A Meta-Analysis. Lancet Infect Dis (2006) 6:162–70. doi: 10.1016/S1473-3099(06)70412-1
157. Zimmermann P, Finn A, Curtis N. Does BCG Vaccination Protect Against Nontuberculous Mycobacterial Infection? A Systematic Review and Meta-Analysis. J Infect Dis (2018) 218:679–87. doi: 10.1093/infdis/jiy207
158. Gofrit ON, Bercovier H, Klein BY, Cohen IR, Ben-Hur T, Greenblatt CL. Can Immunization With Bacillus Calmette-Guérin (BCG) Protect Against Alzheimer’s Disease? Med Hypotheses (2019) 123:95–7. doi: 10.1016/j.mehy.2019.01.007
159. Gofrit ON, Klein BY, Cohen IR, Ben-Hur T, Greenblatt CL, Bercovier H. Bacillus Calmette-Guérin (BCG) Therapy Lowers the Incidence of Alzheimer’s Disease in Bladder Cancer Patients. PloS One (2019) 14(11):e0224433. doi: 10.1371/journal.pone.0224433
160. Klinger D, Hill BL, Barda N, Halperin E, Gofrit ON, Greenblatt CL, et al. Bladder Cancer Immunotherapy by BCG Is Associated With a Significantly Reduced Risk of Alzheimer’s Disease and Parkinson’s Disease. Vaccines (2021) 9(5):491. doi: 10.3390/vaccines9050491
161. de Bree LCJ, Koeken VACM, Joosten LAB, Aaby P, Benn CS, van Crevel R, et al. Non-Specific Effects of Vaccines: Current Evidence and Potential Implications. Semin Immunol (2018) 39:35–43. doi: 10.1016/j.smim.2018.06.002
162. Palgen JL, Feraoun Y, Dzangué-Tchoupou G, Joly C, Martinon F, Le Grand R, et al. Optimize Prime/Boost Vaccine Strategies: Trained Immunity as a New Player in the Game. Front Immunol (2021) 12:612747. doi: 10.3389/fimmu.2021.612747
163. Moulson AJ, Av-Gay Y. BCG Immunomodulation: From the ‘Hygiene Hypothesis’ to COVID-19. Immunobiology (2021) 226(1):152052. doi: 10.1016/j.imbio.2020.152052
164. Sechi LA, Rosu V, Pacifico A, Fadda G, Ahmed N, Zanetti S. Humoral Immune Responses of Type 1 Diabetes Patients to Mycobacterium Avium Subsp. Paratuberculosis Lend Support to the Infectious Trigger Hypothesis. Clin Vaccine Immunol (2008) 15(2):320–6. doi: 10.1128/CVI.00381-07
165. Rosu V, Ahmed N, Paccagnini D, Gerlach G, Fadda G, Hasnain SE, et al. Specific Immunoassays Confirm Association of Mycobacterium Avium Subsp. Paratuberculosis With Type-1 But Not Type-2 Diabetes Mellitus. PloS One (2009) 4(2):e4386. doi: 10.1371/journal.pone.0004386
166. Cossu D, Cocco E, Paccagnini D, Masala S, Ahmed N, Frau J, et al. Association of Mycobacterium Avium Subsp. Paratuberculosis With Multiple Sclerosis in Sardinian Patients. PloS One (2011) 6(4):e18482. doi: 10.1371/journal.pone.0018482
167. Otsubo S, Cossu D, Eda S, Otsubo Y, Sechi LA, Suzuki T, et al. Seroprevalence of IgG1 and IgG4 Class Antibodies Against Mycobacterium Avium Subsp. Paratuberculosis in Japanese Population. Foodborne Pathog Dis (2015) 12(10):851–6. doi: 10.1089/fpd.2015.1956
168. Kühtreiber WM, Faustman DL. BCG Therapy for Type 1 Diabetes: Restoration of Balanced Immunity and Metabolism. Trends Endocrinol Metab (2019) 30(2):80–92. doi: 10.1016/j.tem.2018.11.006
Keywords: Alzheimer’s disease, autophagy, insulin resistance, Mycobacterium avium ss. paratuberculosis, MAP, rifampin, Johne’s disease, Crohn’s disease
Citation: Dow CT (2021) Warm, Sweetened Milk at the Twilight of Immunity - Alzheimer’s Disease - Inflammaging, Insulin Resistance, M. paratuberculosis and Immunosenescence. Front. Immunol. 12:714179. doi: 10.3389/fimmu.2021.714179
Received: 24 May 2021; Accepted: 09 July 2021;
Published: 05 August 2021.
Edited by:
Davide Cossu, Juntendo University, JapanReviewed by:
Michael Thomas Collins, University of Wisconsin-Madison, United StatesCopyright © 2021 Dow. This is an open-access article distributed under the terms of the Creative Commons Attribution License (CC BY). The use, distribution or reproduction in other forums is permitted, provided the original author(s) and the copyright owner(s) are credited and that the original publication in this journal is cited, in accordance with accepted academic practice. No use, distribution or reproduction is permitted which does not comply with these terms.
*Correspondence: Coad Thomas Dow, Q3RvbWRvd0BnbWFpbC5jb20=
Disclaimer: All claims expressed in this article are solely those of the authors and do not necessarily represent those of their affiliated organizations, or those of the publisher, the editors and the reviewers. Any product that may be evaluated in this article or claim that may be made by its manufacturer is not guaranteed or endorsed by the publisher.
Research integrity at Frontiers
Learn more about the work of our research integrity team to safeguard the quality of each article we publish.