- Department of Hematology and Oncology, Okayama University Hospital, Okayama, Japan
Allogeneic hematopoietic stem cell transplantation (allo-HSCT) is an evidence based- cellular immunotherapy for hematological malignancies. Immune reactions not only promote graft-versus-tumor effects that kill hematological malignant cells but also graft-versus-host disease (GVHD) that is the primary complication characterized by systemic organ damages consisting of T-cells and antigen presenting cells (APCs) activation. GVHD has long been recognized as an immunological reaction that requires an immunosuppressive treatment targeting immune cells. However immune suppression cannot always prevent GVHD or effectively treat it once it has developed. Recent studies using high-throughput sequencing technology investigated the impact of microbial flora on GVHD and provided profound insights of the mechanism of GVHD other than immune cells. Allo-HSCT affects the intestinal microbiota and microbiome-metabolome axis that can alter intestinal homeostasis and the severity of experimental GVHD. This axis can potentially be manipulated via dietary intervention or metabolites produced by intestinal bacteria affected post-allo-HSCT. In this review, we discuss the mechanism of experimental GVHD regulation by the complex microbial community-metabolites-host tissue axis. Furthermore, we summarize the major findings of microbiome-based immunotherapeutic approaches that protect tissues from experimental GVHD. Understanding the complex relationships between gut microbiota-metabolites-host tissues axis provides crucial insight into the pathogenesis of GVHD and advances the development of new therapeutic approaches.
Introduction
Hematological malignancies, such as leukemia, lead to high mortality, especially in the elderly. Allogeneic hematopoietic stem cell transplantation (allo-HSCT) is an immune therapy that has become widely used for the treatment of life-threatening hematological malignancies and congenic immune deficiencies (1). However, many complications following allo-HSCT occur due to immune-related reactions, the most challenging and serious of which is graft-versus-host disease (GVHD) (2). GVHD is composed of complexes of immune cells and tissues (3). After T-cells activation and migration to GVHD target organs, such as the lung, gut, liver and skin, cytotoxic T-cells and helper T-cells attack target cells in these target organs. Once GVHD develops, recipients require additional immunosuppressive therapies using steroids in addition to GVHD preventive immunosuppressants consisting of calcineurin inhibitors. However, GVHD is frequently refractory to standard steroid therapy and has a dismal prognosis, with only 5–30% overall survival (4). In steroid-refractory GVHD, novel treatment approaches include the use of anti-thymocyte globulin, extracorporeal photopheresis, mesenchymal stromal cells, mycophenolate mofetil, everolimus, sirolimus, etanercept, infliximab, and ruxolitinib (5). Intensified immunosuppressive therapies for aggravated GVHD suppress immune reactions and permit bacterial translocation from injured intestinal epithelium cells; this leads to subsequent systemic infections that increase the release of pathogen-associated molecular patterns (PAMPs) and damage associated molecular patterns (DAMPs). This negative feedback loop can result in fatal GVHD. In addition, excessive immunosuppression increases malignancy relapse after HSCT. Thus, immunosuppressive therapies are limited.
Current focus has been shifted to the target tissues, the third player in GVHD, in addition to APCs and T-cells (3). The role of tissue-intrinsic factors that might contribute to the regulation of GVHD severity has been largely overlooked. Tissue-specific programs contribute to target tissue resilience, repair, and regeneration and mitigate the severity of GVHD without altering the load or function of alloreactive immune cells (6). In this context, the gastro-intestinal (GI) tract has close interactions with the microbiota, and recent genomic microbial analyses have revealed intricate connections between the microbiota and various diseases. Particularly, in GVHD, disturbance of microbial composition, that is, dysbiosis, is strongly associated with poor outcomes after allo-HSCT (7). However, whether a causal relationship exists remains unclear. In this review, we focus on the connection among gut microbiota, microbial metabolites and intestinal environment that affect GVHD. We also discuss the recent findings on microbiome-based immunotherapeutics that affect or mitigate GVHD and enumerate the emerging strategies for the regulation of dysbiosis and microbial metabolites in the regulation of GVHD.
Gut Microbiota and Intestinal Epithelial Cells
A close relationship between microbiota and human health has been investigated. Microbiota refers to the community comprising trillions of microorganisms, including bacteria, fungi, and viruses, that symbiotically colonize the human body, most members of which reside in the gut and are largely nonpathogenic anaerobic commensal bacteria (8). This balance is finely tuned in the gastrointestinal (GI) tract and influenced by the environment, diet, and host factors, including host physiology (9). The mammalian GI tract is a relatively hypoxic tissue and has a steep oxygen (O2) gradient between the O2-rich lamina propria and the gut lumen, which is dominated by anaerobic organisms (10). Aerobic and facultative anaerobic bacteria have been suggested to consume oxygen in the distal intestine and maintain hypoxia in the lumen, leading to the colonization by strict anaerobes and the production of short-chain fatty acids (SCFAs) (11). Complex dietary carbohydrates (fiber) are broken down by these bacteria into digested fermentation products, that are absorbed by the host and utilized for host nutrition, immune development, and niche protection against enteric pathogens (12–19). Members of Clostridia and Bacteroidia are the obligate anaerobic bacteria dominating in the colon and capably digest any carbohydrate complex into fermentation products (20, 21). Facultative anaerobic bacteria such as Proteobacteria are not specialized in consuming fiber, and rather interfere with host nutrition by changing metabolites to carbon dioxide under oxygen (22, 23). Fermented metabolite effects have been observed in a neonatal mouse model with Clostridia species colonization that protected neonatal mice form virulent pathogens (24). Intestinal epithelial cells (IECs) are continuously renewed from the crypts where intestinal stem cells (ISCs) are located. ISCs divide and differentiate into transit-amplifying (TA) cells, IECs, enteroendocrine cells and goblet cells (25). In contrast to TA and ISCs that utilize glucose to obtain energy through glycolysis, IECs produce energy via mitochondrial β-oxidation of fatty acids and oxidative phosphorylation, both of which require oxygen (26, 27). Butyrate is a favored metabolic substrate that maintains epithelial energy homeostasis (26). Despite low O2 conditions, high butyrate levels result in the downregulation of the glycolytic pathway and the upregulation of mitochondrial respiration (28). This drastic change of energy production was triggered with the expression of peroxisome proliferator-activated receptor-γ (PPAR-γ), which is a nuclear receptor synthesized in differentiated IECs of rodents and humans (29). The energy metabolism in IECs consumes a high amount of oxygen, leading to low oxygen pressure in the lumen (30). This oxygen consumption in IECs permits epithelial hypoxia and helps to maintain anaerobic bacterial flora in the intestinal lumen (31). The hypoxic environment enables obligate anaerobic bacteria that produce carbohydrate metabolites to colonize and provide benefits to the host. Epithelial metabolism of SCFA is a primary determinant of ‘‘physiologic hypoxia’’ in the mucosa with O2 consumption and hypoxia-inducible factor (HIF) stabilization that promotes barrier function (32). Therefore, IECs are uniquely adapted to this hypoxic environment, and cells programmed by “physiological hypoxia” have been shown to tonally regulate barrier function (33). These mechanisms indicated that IECs influence the shaping of beneficial microbiota, and those microbiotas bring metabolic profits to the host. In this process IECs play a central role in maintaining gut homeostasis (Figure 1).
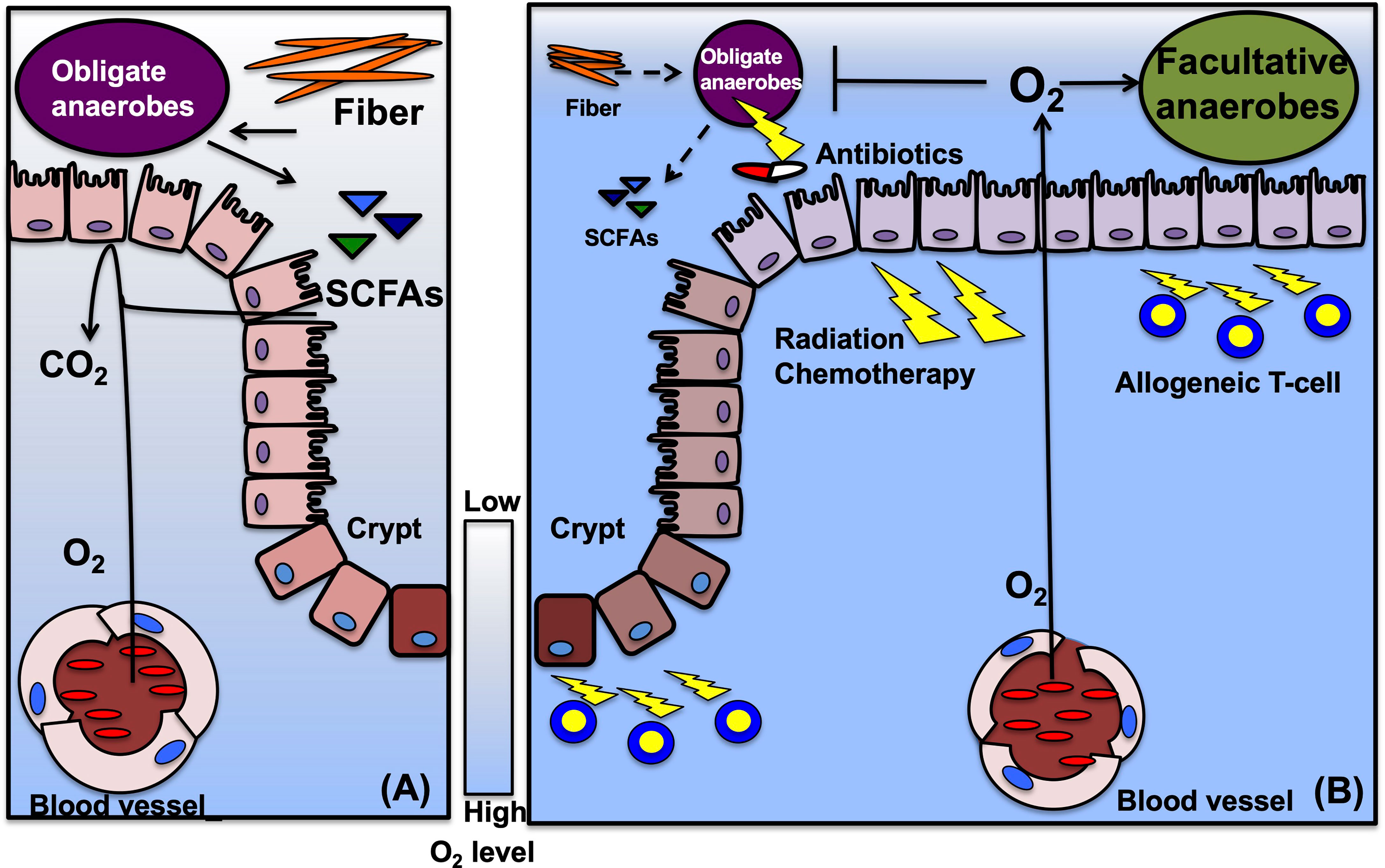
Figure 1 Suggestive mechanisms of epithelial metabolism and gut microbiota in HSCT. (A) Before HSCT, gut microbiota, especially obligate anaerobic bacteria, ferment fiber from diet into metabolites, such as SCFAs. Intestinal epithelial cells (IECs) metabolize short chain fatty acids (SCFAs) and consume oxygen to produce energy. High oxygen consumption limits the oxygen diffusion into the lumen and maintains hypoxia in the lumen. Hypoxic condition enables obligate anaerobes to keep growth. The scale bar indicates oxygen (O2) levels, usually between 3% to 10% in normal intestines. (B) During HSCT, conditioning treatments including irradiation and high-dose chemotherapy damages IECs, and injured IEC decreases oxygen consumption. Decreased dietary fiber and antibiotics treatments during HSCT disrupt microbiota composition, especially reduce obligate anaerobes, and deplete the fermentative products. Reduced SCFAs limit oxygen consumption to produce energy in IECs and permit oxygen diffusion into the gut lumen. Elevated oxygen concentration drives an expansion of facultative anaerobic bacteria and might lead to a contraction of obligate anaerobes. In GVHD, allogeneic T-cells promote IEC injuries and require antibiotics treatments, that could enhance and maintain dysbiosis in the gut.
The IECs and gut microbiota function in two distinct manners: protection against ingested pathogens and induction of tolerance to beneficial commensals. The intestinal immune system matured by microbiota stimulates the mucosa-producing cells (i.e., Paneth cells and goblet cells) to secrete antimicrobial peptides, antibodies, and mucin to form intestinal physical barriers, such as the mucus layer and tight junctions that regulates the relationship between the microbiota and the host (34). Germ-free mice have distinct gut features, such as altered intestinal morphology and slower turnover of IECs than conventional mice (35). After exposure to exogenous commensal bacteria, germ-free mice responded to intestinal bacteria. Four days post-exposure, innate immune responses and structural changes in the intestinal epithelial crypts were observed, i.e., increased TNF-α and interferon-γ (IFN-γ) expression and upregulation of MHC class I molecules (35). IECs in SPF mice also expressed MHC class II molecules that were deficient in germ free mice (36). Under the inflammatory status such as GVHD, gut microbiome stimulated IL-12 production from myeloid cells, resulting in increased expression of MHC class II on IECs through IFN-γ produced from lamina propria lymphocytes. In summary, the intestinal environment shapes and maintains the microbiota. Also, microbiota and those metabolites play important roles on both the function and metabolism in IECs.
Mechanism of Dysbiosis in Hematopoietic Cell Transplantation
Associations between the microbiota and HSCT outcomes have been extensively studied in the 1970s (37). According to these early studies, specific pathogen-free mice treated with antibiotics or germ-free mice that received HSCT developed less severe GVHD than their respective controls. These attempts were validated in HSCT patients with gut decontamination by antibiotics (38). Recently, broad-spectrum antibiotics that target anaerobic pathobionts have been revealed to increase GVHD-related mortality in mouse and human (39). Therefore, some antibiotics that perturb microbial composition were unsuitable for patients in HSCT settings. The microbial compositions of various anaerobic commensal intestinal bacterial species were robustly disturbed and reduced due to chemo/radiotherapy, antibiotics, or HSCT itself in patients (40). Decreased diversity and expansion of specific bacteria, including Enterococcus spp., were reported as risk factors that resulted in poor outcomes after HSCT (41). The mono-domination of Enterococcus was significantly associated with severe GVHD and treatment related mortality (TRM) in mice and human studies (42). These studies indicate the causative role of Enterococcus in the pathogenesis of GVHD. However, the mechanism of dysbiosis post-HSCT remains unclear (7, 43). Before and after HSCT, patients receive various interventions that are likely to contribute to the microbial dysbiosis. Importantly, in a mouse model of acute GVHD exposure to a GVHD-associated gut microbiome pre- and/or post- HSCT accelerated GVHD development and severity demonstrating the complex relationship between GVHD and microbiota (44). These factors could affect the intestinal microbial composition (Figure 1).
Radiotherapy
As a part of allo-HSCT, systemic irradiation is frequently used to kill fast-dividing leukemia/lymphoma cells and host myeloid cells/lymphocytes. However, radiation causes intestinal damage, and the potential correlation between dysbiosis and radiation-induced damage has been revealed (45, 46). Radiation induces oxidative stress via the generation of reactive oxygen species (ROS), including hydroxyl radical (OH), superoxide anion (O2−), and hydrogen peroxide (H2O2), resulting in the activation of cyclooxygenases (COX), nitric oxide synthases, lipoxygenases, and nicotinamide adenine dinucleotide phosphate oxidases. These products of oxidative stress cause DNA damage, inflammation, and apoptosis in IECs and affect the gut microbiota composition through homeostatic disturbances (45). The relationship between radiation enteritis and dysbiosis was observed in patients receiving radiotherapy. Relatively high abundances of Proteobacteria and Gammaproteobacteria, characterized by increased oxidative stress resistance in Enterobacteriaceae, Phyllobacteriaceae, and Beijerinckiaceae, and low abundance of Bacteroides with decreased Bacteroidaceae and Ruminococcaceae that were sensitive to oxidative stress, were detected (46). Recently, a multi-omics study reported Lachnospiraceae and Enterococcaceae as radioprotective microbes and elevated SCFA levels due to irradiation damage in mice receiving irradiation (47). Bifidobacterium can utilize indigestive fibers, such as fructose oligosaccharides (FOS), inulin-type fructans (ITF), and xylo-oligosaccharides (XOS), and cross-feed with lactate-converting bacteria and butyrate-producing bacteria, i.e., Eubacterium hallii and Anaerostipes caccae (48). Although Bifidobacterium cannot produce butyrate, it is associated with increased abundance of butyrogenic bacteria in a cross-feeding manner (49). Moreover, Eubacterium hallii L2-7 and Anaerostipes caccae L1-92 could not grow in starch but grew upon co-culture with Bifidobacterium adolescentis L2-32 to produce butyrate (50). Collectively, the abundance of specific bacterial species is reduced and eventually lost post irradiation before allo-HSCT.
Chemotherapy
Elaborating the purpose of repeated intensive chemotherapy before allo-HSCT reduces microbiota diversity in both human and mice and leads to the expansion of Escherichia Coli and Enterococcus spp (51). During allo-HSCT, disrupted gut microbial composition and diversity, including decreased abundances in Bifidobacterium, butyrate-producing Faecalibacterium and Lachnospiraceae, were highly associated with transplant-related mortality (7). Methotrexate treatments caused a significant reduction in the number of Bifidobacterium and Lactobacillus species and Escherichia coli in children with leukemia (52). Etoposide exhibits broad bacterial inhibitory activity that changes microbial composition in vitro (53). In leukemia patient data, it is suggested that chemotherapy induces dysbiosis (51). Healthy volunteers in the Human Microbiome Project (HMP) and patients with acute myeloid leukemia in the pre-induction phase showed no significant differences in terms of microbial diversity. After neutrophil recovery, microbial diversity in the leukemia group was significantly decreased and this dysbiosis was associated with an increased risk of infections and the use of broad-spectrum antibiotics. Within 3 months of anti-anaerobic antibiotic administrations including carbapenem and piperacillin-tazobactam, a significant reduction in microbial diversity was also observed before allo-HSCT in the recipients (54). Multiple intensive chemotherapies for acute leukemia patients also disrupt the microbial composition and promote the outgrowth of pathogenic bacteria such as Enterococcus (55). Another study demonstrated that pre-transplant microbial composition differed from the healthy controls due to decreased abundances of beneficial bacteria, Bifidobacterium, Faecalibacterium, and Lachnospiraceae (56). Overall, these findings suggest that pre-transplant microbe disruption increased HSCT comorbidity. However, the mechanisms of dysbiosis onset and the disruption of the microbiota by chemotherapy are still unknown. Furthermore, these studies could not separate the effects of HSCT from those of antibiotics (39).
Antibiotics
Chemotherapies for hematological malignancies cause high-grade neutropenia and require systemic antibiotic administration to prevent or treat life-threatening bacterial infections. The influence of antibiotics on the microbial composition should be accounted for. Recent microbiome studies have observed undesirable consequences in dysbiosis, including antibiotic resistance, pathogenic bacteria dominance, transient or profound loss of microbial diversity, increased susceptibility to infection, and the risk of recurrent infections, after antibiotic usage in cancer patients (57). However, immunocompromised individuals are at high risk of intestinal infections, which are difficult to treat without using broad-spectrum antibiotics (58). As reported by various studies, oxygen-tolerant species, Enterococcus, Enterobacteriaceae, Klebsiella spp., and Viridans streptococci, are most commonly translocated from damaged intestinal mucosal barrier due to chemotherapy, irradiation, and antibiotics to the bloodstream (59). Prophylactic antibiotics, such as quinolones, are usually utilized to prevent infections in patients receiving HSCT. Once they develop fever or infections, broad-spectrum antibiotics, such as piperacillin-tazobactam or meropenem, are prescribed (60). Compared to pre-transplant conditions, decreased Firmicutes and Actinobacteria and increased Bacteroides and Proteobacteria have been detected at 1week post-conditioning (61). Notably, the use of rifaximin could preserve gut beneficial commensals and improve patient outcomes (62). HSCT patients receiving rifaximin showed significantly lower TRM, prolonged OS, and a tendency for low acute GI-GVHD rates than historical controls. These observations remained unknown even in patients who developed neutropenic fever and received systemic antibiotics without an increase in sepsis rates or pathogenic bacterial colonization. Efforts to investigate and identify the antibiotics that could spare microbial compositions to reduce acute GI-GVHD and adverse outcomes have been attempted (63). A study in patients from Canada also assessed the effects of prophylactic and therapeutic antibiotic administration before day 0 of HSCT (64). The antibiotic-receiving group had a significantly higher incidence of acute GVHD (aGVHD) and shorter survival. The early administration of systemic antibiotics before engraftment was also associated with low 3-IS levels and reduced Clostridia abundance in the intestines, leading to higher TRM rates than those without systemic antibiotics before engraftment (65). These studies suggest the association of antibiotic usage affecting microbial diversity and GVHD-related outcomes. Piperacillin-tazobactam and meropenem, known as broad-spectrum antibiotics that are also effective against anaerobic bacteria, caused decreased microbiome diversity during transplantation in mice and humans (39, 66). In mouse models, these antibiotics lead to microbial injury with loss of colonic mucosa and intestinal barrier function caused by mucin-degrading bacteria, Akkermansia muciniphila (39). The load of anti-anaerobic antibiotic usage was correlated with a significant decrease in anti-inflammatory Clostridia (AIC) abundance and aGVHD in pediatric HSCT patients (67). This decrease was recovered by the administration of AIC after clindamycin treatment and improved survival in a mouse GVHD model (67, 68). Among the broad-spectrum antibiotics administered during HSCT, meropenem showed significantly decreased microbial diversity and a higher rate of GI-HVHD onset compared to no antibiotics, but cefepime did not change the diversity with a trend of increased GI-GVHD onset rates (69). In a pediatric study, anti-anaerobic antibiotics resulted in a significant decrease of SCFA-producing bacteria, especially butyrate-producing commensals and butyrate levels in the luminal content. These patients developed aGVHD with low butyrate levels in stools compared to patients without GVHD (70). Single bacterial taxa dominated by Enterococcus and Streptococcus was observed in two-thirds of patients with bloodstream infections around the time of engraftment (71). The significant elevation of enterococcus expansion was increased by metronidazole administration, and VRE bacteremia was significantly increased (3-fold and 9-fold, respectively). Integrating these studies, although antibiotic prophylaxis and systemic administration clearly improved TRM, especially infection-related mortality, we need comprehensive choices of antibiotic usage around HSCT periods comparing weights of disease status, donor sources, history of antibiotic usage, status of microbial injuries, and other risk factors.
Metabolic Changes in Colonocytes
IECs might control the homeostasis that shapes the microbiota to be beneficial (72). Dysbiosis that caused increased abundance of facultative anaerobic bacteria, is observed in the population consuming a high-fat Western-style diet, patients with inflammatory bowel disease, colorectal cancer, irritable bowel syndrome or necrotizing enterocolitis (73–80). It has been proposed that shift of microbial community from obligate to facultative anaerobic bacteria that can utilize oxygen could be associated with a disruption in aerobiosis, a concept “oxygen hypothesis” in mouse inflammatory bowel disease models (81, 82). This microbial shift might be associated with the colonocyte metabolic dysfunction (31). Disruption of gut homeostasis was observed in antibiotic treatment that altered IECs metabolism by depleting microbes that produce SCFAs in a mouse colitis model (19, 32). Decreased SCFAs increases the inflammatory tone of the colonic mucosa in mice (83). In the animal model, elevation of inflammatory signals shifts the metabolism in IECs toward an aerobic glycolysis, reduced oxygen consumption and high glucose consumption and high lactate release (84). These metabolic changes result in a loss of epithelial hypoxia (32). Increased oxygen concentration elevates the amount of oxygen in mucosal surface, therefore drives an expansion of facultative anaerobic bacteria (85). These associations between metabolic condition in IECs and dysbiosis are not validated in GVHD settings and the future investigations are required.
Butyrate and Lactase Pathway
Recent evidence has suggested that dysbiosis post allo-HSCT are related to the butyrate and lactase pathways (86). Butyrate is produced from non-digestible fiber by anaerobic bacterial species, including those belonging to Firmicutes, Lachnospiraceae, Ruminococcaceae, Lactobacillus spp., and Bifidobacterium adolescentis. Other bacterial phyla including Bacteroides, Actinobacteria, Fusobacteria, and Proteobacteria are also potent butyrate producers (87). These bacteria are sensitive to oxidative stress caused by chemotherapy and irradiation administered before HSCT, leading to their reduced abundances and the subsequent decrease in butyrate volume in the lumen. At the intestinal level, butyrate is a protective molecule against inflammation, a histone deacetylase inhibitor, and an energy source for epithelial cells (68, 88). Therefore, decreased butyrate concentration led to GVHD augmentation due to the disturbance of intestinal homeostasis and the activation of inflammatory environment in the intestine. Another mechanism that causes dysbiosis post-HSCT in GVHD patients involves the domination of the lactic acid bacteria (LAB) Enterococcus faecium in the gut (42). In GVHD patients, metabolism of lactose and galactose is impaired by the overexpression of enzymes and lactases (89). In adults, lactase is mainly produced by the microbiota, including species belonging to Actinobacteria, Proteobacteria, and Firmicutes (90). Each LAB has distinct enzymatic characteristics and behaves differently depending on each feature. LABs are mainly gram-positive, acid-tolerant bacteria from the genera Lactobacillus, Enterococcus, Streptococcus, Pediococcus, Lactococcus, and Oenococcus (91). They produce lactase that catalyzes the conversion of carbohydrates (such as lactose, glucose, sucrose, and galactose) into lactic acid and facilitates lactose absorption (92, 93). The reduction in the number of commensal LAB mediated by conditioning chemotherapy, radiotherapy, and antibiotics post-HSCT leads to high lactose concentrations. For example, Enterococci are a part of the normal intestinal microbiota and can cause clinical problems. Particularly, Enterococcus faecium and some Enterococcus faecalis encode genes related to lactose and galactose metabolism (42). Enterococcus faecium metabolizes citrate and lactose to produce lactate, whereas E. faecalis can produce superoxide and H2O2 that damage colonic epithelial cells (94, 95). These enterococci require lactose for growth in vitro (lactose auxotroph) and are associated with intestinal inflammation and damages (96). These pathways were observed following the expansion of Enterococci early after HSCT in patients with the increased risk of HSCT-related mortality (43). Butyrate and lactase pathway could be a target for treating dysbiosis in HSCT. The analysis of these pathways for TRM post HSCT has been reviewed in detail previously (89).
Microbial Metabolites in GVHD
The intestinal microbiota produces various microbial metabolites that maintain intestinal homeostasis (97, 98). These metabolites affect both IECs and immune cells in the intestine to maintain intestinal barrier function and host immune responses (97). Changes in microbial composition led to poor HSCT outcomes; however, to date, there is no conclusive evidence that changes in gut microbial metabolites affect GVHD severity and TRM.
Short-Chain Fatty Acids
SCFAs, including acetate, butyrate, and propionate, are products of fermented carbohydrates by anaerobic commensal bacteria Clostridia spp (99). SCFAs have multipotent effects on both IECs and intestinal immune cells (Figure 2). Particularly, butyrate serves as an energy source for IECs as demonstrated by reduced autophagy in SPF mice compared to germ-free mice (26). In addition, SCFAs preserve the intestinal mucosal barrier by supporting goblet cells via upregulating mucin-related genes. The supplementation of SCFA-producing bacteria in germ-free rats showed goblet cell maturation (100–102). SCFAs also maintain the IEC barrier integrity that prevents pathogenic bacterial translocation (103). Furthermore, they play an important role in innate immunity, neutrophils, mononuclear cells, macrophages, and dendritic cells and act as histone deacetylase (HDAC) inhibitors with anti-inflammatory effects (99, 101, 102). SCFAs also affect Tregs to promote differentiation and anti-inflammatory responses in a mouse colitis model (17). Moreover, anti-inflammatory IL-10 production from Foxp3-positive Tregs via HDAC inhibition was observed in germ-free mice with SCFA supplementation (18, 104). A high-fiber diet and acetate activated the inflammasome and promoted IL-18 production, which modulated epithelial repair in murine colitis model (105). Butyrate also increased IL-18 expression in IECs and IL-10 expression in DCs and macrophages, thereby inducing Treg differentiation in mice (106). Recently, a metabolomic analysis of murine intestine and contents revealed a significant reduction in butyrate in the IECs from GVHD mice and that the supplementation of butyrate or butyrate-producing bacteria ameliorated acute GVHD via HDAC inhibition and gut integrity maintenance (68, 107). Butyrate and propionate directly protected IECs via inflammasome activation through SCFA-sensing receptors in murine GVHD model (108). In humans, small clinical studies have found that the high fecal butyrate and propionate concentrations are decreased in acute GVHD patients and that high circulating butyrate and propionate concentrations in the blood are associated with protection from chronic GVHD (70, 107). Other studies examined fecal SCFA concentrations after allo-HSCT and reported that the concentrations of butyrate and other SCFAs correlate with the abundance of butyrate-producing bacteria in the intestinal microbiota and are higher in patients with resistance to lower tract respiratory infections (109). However, the effect of SCFAs on GVHD needs to be examined in patients after allogeneic HSCT.
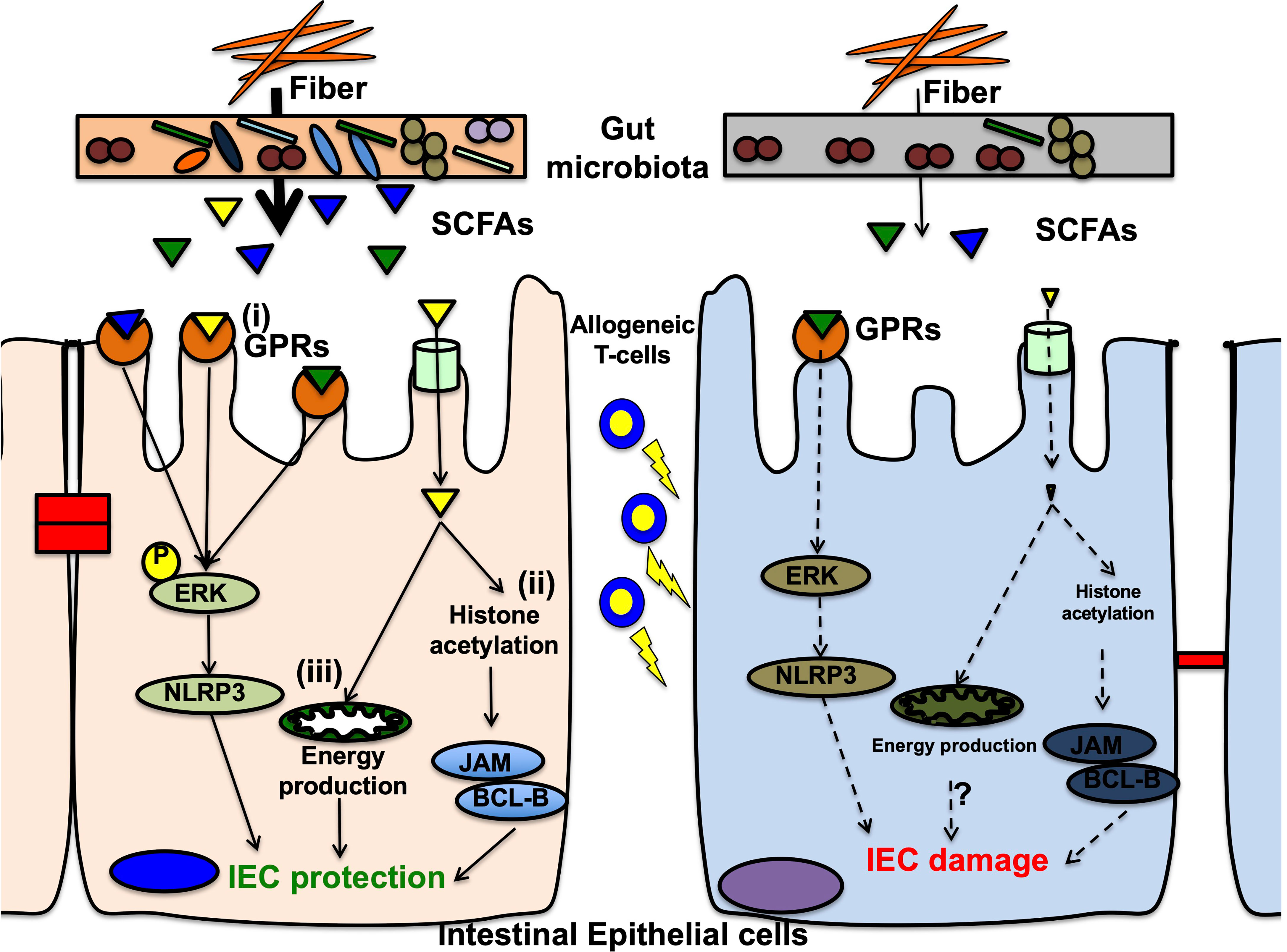
Figure 2 Mechanisms of short chain fatty acids on intestinal epithelial cells in the pathophysiology of gastrointestinal GVHD. In the normal condition, short chain fatty acids (SCFAs) that are metabolites from microbial fermentation of dietary fibers, protect intestinal epithelial cells (IECs) in at least 3ways. (i) G-protein-coupled receptors (GPRs) on IECs, especially GPR43, sensor SCFAs and signal ERK phosphorylation leading to subsequent NLRP3 inflammasome activation, that promotes IEC integrity. In graft-versus-host disease (GVHD), decreases of GPRs on IECs and reduced SCFAs from dysbiosis diminish intracellular signaling via ERK-NLRP3 pathway, resulting in IEC damages. (ii) One of SCFAs, butyrate acts as a histone deacetylase inhibitor, and increases various target gene expressions, including anti-apoptotic BCL-B and the junctional protein JAM (junctional adhesion molecule). These gene expressions result in decreased IEC apoptosis and enhanced junctional integrity, leading to IEC protection. In GVHD, reduced butyrate fails to protect histones from deacetylation and decreased target gene expression, resulting in low resistance against allogeneic T-cell injuries. (iii) Butyrate activates peroxisome proliferator-activated receptor-γ (PPAR-γ) signaling in IECs and promotes mitochondrial ß-oxidation that produce enough amount of energy to protect and maintain IECs from cellular damages. In GVHD, reduced butyrate might not activate PPAR-γ, resulting in mitochondrial dysfunction for energy production that are inappropriate for IEC protection against allogeneic T-cell injuries. This mechanism is studied in inflammatory bowel disease but not validated in GVHD. Further investigations are required.
During HSCT, recipients have problems that limit oral intake due to mucositis and receive total parenteral nutrition (TPN). In both mice and humans, TPN skewed immune responses to pro-inflammatory conditions (110, 111). Positive nutritional support using sucrose improved hematopoietic recovery compared to antibiotic-treated recipients in mice (112). These studies overall suggest the potential of specific diet elements (prebiotics) during HSCT. Resistant carbohydrate supplementation can stimulate SCFA production in the intestine as the microbiota can metabolize resistant starch (RS) (113). Among various formulations of RS, that derived from potatoes was identified as a potent candidate for increasing fecal butyrate levels in healthy adults (114). Remarkably, RS administration to allo-recipients around HSCT periods was demonstrated as feasible and safe and lead to increased fecal butyrate levels concomitant with high RS degrading and butyrate-producing bacteria in a human pilot study (115). However, the clinical data on the effects of prebiotics in acute GVHD are limited. Inulin and fructo-oligosaccharides have potential as prebiotics in inflammatory bowel disease patients as they led to the expansion of intestinal microbial diversity (116, 117). To determine the exact role of nutritional modulation using RS in the microbiome and metabolites affecting GVHD severity, clinical trials are currently ongoing (www.clinicaltrials.gov: NCT02763033). A clinical trial that evaluating the safety and tolerability of fructo-oligosaccharides in HSCT patients (NCT02805075) is also underway.
Bile Acids
Bile acid is a microbial metabolite affected by intestinal microbial composition (118). Primary bile acids are generated in the liver via cholesterol catabolism. These primary bile acids are conjugated to glycine and taurine in the final step of synthesis and are secreted into the intestine. Most bile acids are taken up by the IECs and transported to the liver (119). Secondary bile acids are converted by microbial enzymes from primary bile acids that were not absorbed. Two main receptors, the nuclear farnesoid X receptor (FXR) and G protein-coupled bile acid receptor 1 (GPBAR1; TGR5), are known. FXR-deficient mice showed abnormal IEC functions, thus causing bacterial translocation from the intestine (120). TGR5 signaling from bile acids activates macrophages and inhibits the production of pro-inflammatory cytokines TNF-α and IL-1 by inhibiting the nuclear factor-κB (121). Dietary fat-induced taurocholic acid in IL-10-deficient mice enhanced colitis in mice by altering the intestinal microbiota and pro-inflammatory Th1 responses (75). While bile acids are not well absorbed in patients with intestinal GVHD and serum sample analysis of allo-HSCT patients revealed altered bile acid concentrations, ursodeoxycholic acid, a secondary bile acid, is clinically used to reduce hepatic complications and GVHD (122–124). In a recent study, tauroursodeoxycholic acid, another bile acid, reduced the experimental murine GVHD severity (124). Tauroursodeoxycholic acid decreased antigen presentation on IECs, and the apoptosis of IECs without affecting the microbial composition and graft-versus-leukemia/lymphoma (GVL) effects. Taurine is a metabolite related to bile acids that stimulates the Nod-like receptor family protein domain containing 6 (NLRP6). NLRP6 stimulation leads to innate immune cell activation, increased apoptosis/necroptosis, inflammasome-related cytokine production, and promotion of intestinal epithelial integrity (125). Taurine has been reported to stimulate NLRP6 in IECs and augment GVHD severity in mice (126). These data suggest that bile acids are promising microbial metabolites that can be used to reduce GVHD severity.
Aryl Hydrocarbon Receptor Ligands
Aryl hydrocarbon receptor (AhR) modulates mucosal immune responses via the ligand-activated transcription factor. AhR ligands are produced from the endogenous and exogenous pathway in the body. In an endogenous pathway, they are produced through intestinal microbial metabolism. Lactobacilli, Fusobacterium, Bacteroides and Enterococcus faecalis convert the amino acid, tryptophan, into AhR ligands, such as indole-3-aldehyde and its derivatives (127, 128). In a human GVHD cohort, preserved microbial diversity and Clostridia spp were correlated with 3-IS concentrations in the urine (129). In serum metabolic comparisons of HLA identical donors and recipients, indolepropionate that was derived from microbiota, was a smaller amount in recipients at the time of GVHD onset (123). 3-IS is also known as a uremic toxin associated with adverse outcomes in renal disease patients. In this setting, monocytes respond to 3-IS through AhR pathway and release TNF-α, resulting in the pro-inflammatory condition with endothelial damage leading to cardiovascular disease (130). AhR ligands maintain IEC barrier functions. AhR-deficient mice have decreased levels of anti-microbial peptides and intestinal epithelial lymphocytes and reduced IEC turnover with altered microbial composition in mice (131). AhR also regulates innate immunity in the intestine by expanding the IL-22-producing retinoic acid receptor-related orphan receptor γt (RORγt)+ group 3 innate lymphoid cells (ILC3s) (132), thereby affecting adaptive immunity (133). In murine GVHD models, a few investigations were reported. Recipient mice that exposed to lethal irradiation or chemotherapeutic conditioning regimens had lower urinary concentrations of 3-IS (134). The supplementation of indole-3 carboxaldehyde, an indole derivative, ameliorated gut epithelial damages with reduced inflammatory cytokines, leading to improved survival. In murine GVHD models, AhR-deficient T-cells ameliorated GVHD through the expansion of peripherally induced Tregs (135). AhR ligand levels were decreased in patients at the onset of GVHD (123). However, the mechanism by which the AhR pathway affects GVHD in mice and humans remains unknown.
Tyrosine Derived Metabolites
Tyrosine derived metabolites are produced from tyrosine fermentation by microbiota in the large intestine (136). Tyrosine is one of the non-essential amino acids that is involved in catecholamine synthesis (137). The function of tyrosine has been well studied in brain physiological and pathological conditions (138). In a mouse GVHD model, the metabolic profiles in recipient mice have been determined (139). The low concentration of tyrosine in the gut was observed in GVHD mice, and the tyrosine derived metabolites were decreased in the mice that has less Blautia and Enterococcus. Dietary supplementation of tyrosine ameliorated GVHD and restored the microbial diversity. In GVHD patients, the metabolite markers in the serum have been investigated (140). Alteration of tyrosine derived metabolites, such as p-cresol sulfate and 3-phenylpropionate, were identified. Also, lysine and phenylalanine were suggested as the altered metabolites. To elucidate the importance of these metabolites, further research is necessary.
Choline Derived Metabolites
Choline derived trimethylamine N-oxide (TMAO) results from oxidation by hepatic flavin monooxygenases of trimethylamine (TMA) that is a microbial metabolite of choline and other choline containing compounds in the diet (141). TMAO is well known to play a role in the initiation of atherosclerosis and thrombosis in vascular inflammation and endothelial function (142). TMAO and choline induced the GVHD progression in a murine GVHD model (143). TMAO and choline induces M1 macrophages and M1-like cytokines in tissues and bone marrow via NLRP3. TMAO are produced by the wide range of bacteria, Anaerococcus hydrogenalis, Clostridium asparagiforme, Clostridium hathewayi, Clostridium sporogenes (144).
Riboflavin (Vitamin B2) Derived Metabolites
Riboflavin derived metabolites are also known to have an important role in the GVHD pathogenesis. Various bacteria including E. coli, Staphylococcus aureus and Pseudomonas aeruginosa, produce these metabolites (145). These metabolites expand the numbers of mucosal associated invariant T-cells (MAIT) (146). Vitamin B2/B9 derived metabolites are presented by MR1, the MHC class I-like molecule, to MAIT cells producing IFN-γ, IL-17 and antibacterial products. In mouse and human GVHD, studies involving riboflavin derived metabolites and GVHD have been accumulating, but there is still no direct evidence that the riboflavin concentration in feces has a direct impact on HSCT complications and outcomes (147–150). In cord blood transplantation, microbial expression of enzymes in the riboflavin synthesis pathway was associated with greater MAIT reconstitution after HSCT (151).
Polyamines
Polyamines are polycationic molecules found in the gastrointestinal tract and are produced by microbial metabolism. They have various biological functions, including IEC barrier function, innate immunity, pro-inflammatory cytokines, and adaptive immunity (152). Spermine, a polyamine, preserves TGF-β and IL-10 production by inhibiting pro-inflammatory cytokine production in activated macrophages (153). Moreover, the amino acid arginine is metabolized to produce polyamines and decreases the production of pro-inflammatory cytokines when administered with bifidobacterial LKM512 (154). The oral supplementation of spermine or spermidine promoted intraepithelial CD8+ T-cell maturation and CD4+ T-cell increase in the lamina propria and B cell increase in the rat spleen (155). However, the effects of polyamines on GVHD have not yet been well studied. The oral microbiome is suggested to play a role in pathological conditions and mucositis in allo-HSCT settings (156, 157). During allo-HSCT, oral microbiota derived metabolites are altered in patients with severe oral mucositis, and reduction of N-acetylputrescine and agmatine, metabolites involved in the polyamine pathway, were reported from salivary metabolic analysis in patients (158).
Fecal Microbiota Transplant
Alterations in intestinal microbiota composition and its contribution to allo-HSCT outcomes have been studied to determine the role of the intestinal microbiota in acute GVHD severity. The strategy of manipulating or improving dysbiosis post allo-HSCT via fecal microbiota transplant (FMT) were introduced in the HSCT field because FMT treatment for Clostridium difficile infection in non-HSCT settings has been successfully reported (159). Recently, the utility of FMT in patients with refractory GVHD after allo-HSCT has also been reported (160). After FMT treatment, dysbiosis improvement was observed as evidenced by increased beneficial bacteria and resolution of clinical symptoms (161). The effects of FMT from third parties were also assessed in a pilot study, and the feasibility and effects on microbiome diversity in recipients have been reported (162). An increase of Clostridiales abundance was correlated with a significant increase of 3-IS concentration in the urine in this study. In a randomized trial involving autologous FMT early after allo-HSCT without broad-spectrum antibiotics, the expansion and reestablishment of gut microbiota diversity in autologous FMT recipients was reported (163). Although only a limited number of studies regarding FMT and GVHD treatment have been conducted, they have reported similar results (164). FMT products were freshly processed or frozen until subsequent use. The routes of administration mainly include oral in packed capsules, nasogastric/nasoduodenal tubes, or enema. Most FMT recipients were treated using third-line or more therapies, whereas some received second-line therapy after steroid failure. Complete response rate was high upon treatment with second-line therapy. Responses to treatment were observed within an average 14 days, with a median of two FMT administrations. Changes in the stool microbiome were analyzed using bacterial sequences. FMT recipients had increased diversity and enrichment of Bacteroides, Lactobacillus, Bidifobacterium, and Faecalibacterium compared with pre-FMTs (160). These changes were observed only when anti-anaerobic systemic antibiotics were discontinued; however, the fourth generation cephem did not affect the efficacy of FMT.
The gut microbiome comprises communities of bacteria, fungi, and viruses that affect each other. Similar to bacterial dysbiosis, alterations in the gut viral community are also associated with gut GVHD (165). For instance, human herpes virus 6 was detected in the serum, and picornavirus in the stool of acute GVHD patients (166). The intestinal fungal composition (mycobiome) after HSCT remains not well investigated. The expansion of pathogenic Candida species was associated with a substantial loss of bacterial diversity, especially that of anaerobes, and increased the risk of fungal bloodstream infections (167). There is a paucity of data regarding the changes in the mycobiome and virome in allo-HSCT post-FMT (168). In a GVHD patient with repeated FMT treatments, longitudinal studies observed dynamic changes in the microbiome, mycobiome, and virome in the stool. In contrast to the expansion of intestinal microbial composition post-FMT, the gut mycobiome was first expanded and decreased after multiple FMTs. The gut virome community varied substantially over time with a stable increase in diversity. Furthermore, increased microbial diversity post-FMTs was consistently reported; however, the suggested mechanisms need to be elucidated, including the changes in the microbial metabolites and the key bacterial strains.
Overall, most studies have concluded that FMTs are generally safe and effective for steroid-refractory GVHD patients. However, infectious complications and deaths have been reported, including death due to transmitted drug-resistant bacteria from the FMT donor, bacteremia not from FMT products, diarrhea due to norovirus in the FMTs, and other infections, and were attributed to the immunocompromised states of the patients. Importantly, critical complications related to the recipient death have been reported, such as the transmission of extended spectrum beta-lactamase producing Escherichia coli that was proven using genomic sequencing from FMT products (169). Before the clinical application of FMTs, the preparation of FMT products should be standardized, and universal stool banks are warranted (170). The precise practical aspects of FMT treatments have been reviewed in detail elsewhere (171).
Future Perspectives
The effects of dysbiosis post-HSCT on GVHD have been investigated. Currently new studies on mycobiome and virome in the intestine post-HSCT are focused because intestinal environments were composed of various microorganisms other than microbiota. As viruses and fungi are also part of the gut microbiome, they are expected to provide a deeper understanding of the connection between the microbiome and GVHD. Microbial-derived metabolites, such as SCFAs and indoles, play critical roles in promoting intestinal homeostasis to overcome unwanted antibiotic influences and unfavorable outcomes after HSCT. There are various targets that can affect the results of HSCT, including microbial metabolites and specific microbial strains that potentiate as prebiotics, probiotics, or FMT in the clinical settings. All potential interventions are still under investigation or not yet determined. However, the mechanisms that cause dysbiosis post-HSCT, excluding broad-spectrum antibiotics, remain elusive. Because IECs utilize oxygen for energy, the intestinal environment has low oxygen levels (26, 89), resulting in the domination of anaerobic bacteria in the gut microbiota. As shown in an inflammatory bowel disease model, the metabolic change through PPAR-γ signaling in IECs might lead to dysbiosis in GVHD, which still require further investigation (19). The role of SCFAs in cell metabolism suggests that tissue damage can be reduced by directly intervening target cells without substantially affecting the immune system (68, 172). Tolerance mediated by tissue homeostasis reduces immunological damage from T-cells and other cells. In addition, GVHD and GVL can also be separated. Furthermore, the connection between GVL effects and dysbiosis should be explored to identify potential strategies that boost immune reactions.
Understanding the precise mechanisms and conditions in the intestinal environment can promote the development of prophylactic/therapeutic strategies targeting single or combined modalities (such as FMT or FMT + butyrate, combinations of metabolites) to reduce immunological reactions originating from the gut (Figure 3). Furthermore, investigating the crosstalk between local microbiota and injuries in target organs, such as the lungs, could unveil other strategies to prevent HSCT-related complications (173).
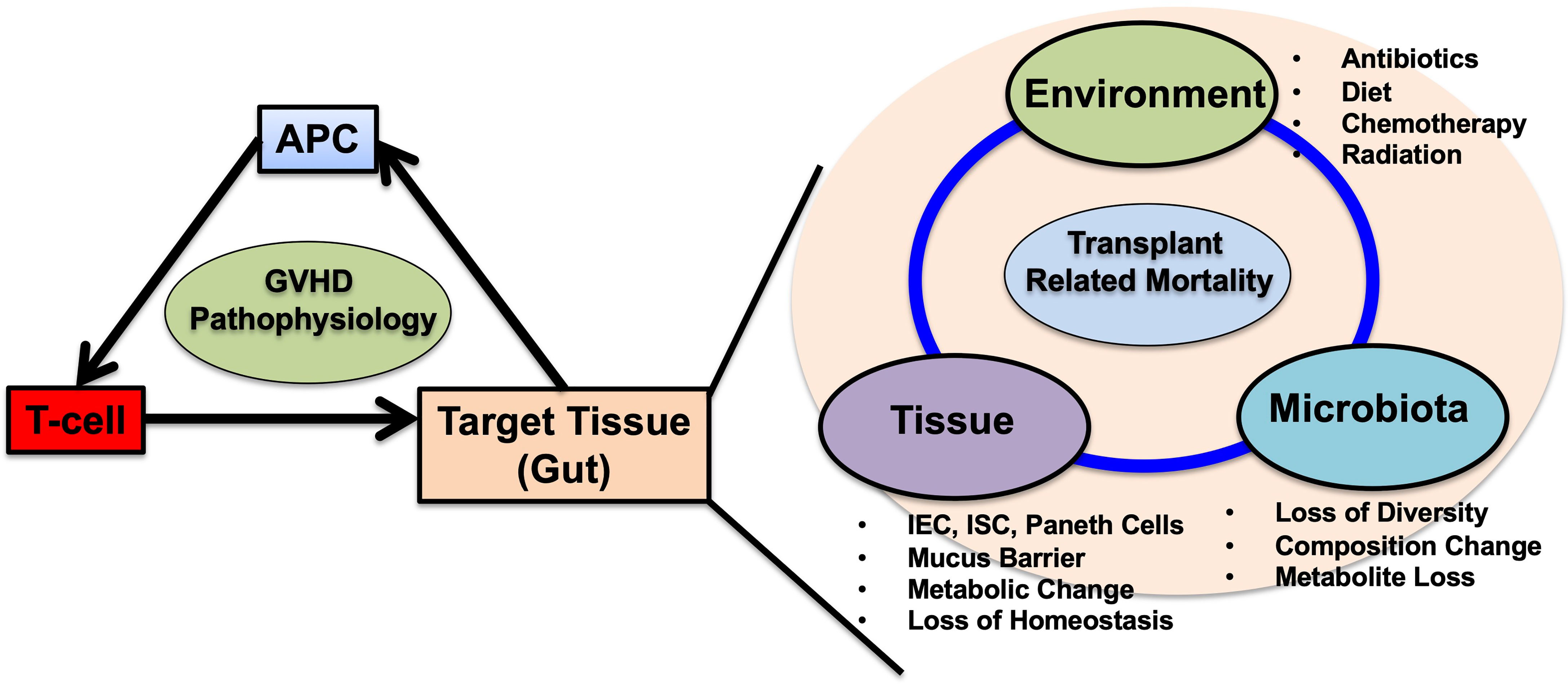
Figure 3 Schematic representation of acute GVHD pathophysiology and the multifactorial interactions in the gut target tissue. Historically, antigen presenting cells (APCs), donor-T cells and target tissues are the main factors in acute GVHD pathophysiology. Among these cells, APCs and T-cells are well studied and targeted for GVHD prevention and treatment. In gut GVHD, microbial dysbiosis are highly associated with transplant related mortality. Microbial dysbiosis is one aspect of multifactorial interactions in the gut during GVHD. In HSCT, conditioning regimens including chemotherapy and irradiation cause damages to intestinal epithelial cells (IECs), intestinal stem cells (ISCs), paneth cells mucus producing goblet cells. Disruption of gut microbiota already exists before HSCT, and prophylactic and systemic antibiotic treatments worsens the microbial dysbiosis with loss of diversity, composition changes along with increase of Enterococcus. Dysbiosis depletes microbial derived metabolites changes the IEC metabolism skewing to less tolerant against donor T-cell damages, postpones IEC repairs and mucus barrier restorations. Expansion of Pathogenic bacteria permits blood stream infection thorough injured IECs that necessitate systemic antibiotic administration, leading to further microbial disruptions. These factors including environment, tissue and microbiota falls into the vicious cycle leading to transplant related mortality. The questions remain whether which factor could be prioritized and be targeted for treatments including fecal microbiota transplantation (FMT), prebiotics, probiotics, the attentions of the usages of anti-anaerobic antibiotics and other interventions targeting tissues. Single or combined strategies are warranted to break the vicious cycle and improve HSCT outcomes.
Author Contributions
The author confirms being the sole contributor of this work and has approved it for publication.
Funding
This work was supported by JSPS KAKENHI Grant number JP20K22901, JP21H02904, The Kawasaki Foundation of Medical Science and Medical Welfare, The Ryobiteien Memorial Foundation, The MSD Life Science Foundation Public Interest Incorporated Foundation, The Okayama Medical Foundation, The SENSHIN Medical Research Foundation, The Kato Memorial Bioscience Foundation and the NOVARTIS Foundation (Japan) for the Promotion of Science.
Conflict of Interest
The author declares that the research was conducted in the absence of any commercial or financial relationships that could be construed as a potential conflict of interest.
Publisher’s Note
All claims expressed in this article are solely those of the authors and do not necessarily represent those of their affiliated organizations, or those of the publisher, the editors and the reviewers. Any product that may be evaluated in this article, or claim that may be made by its manufacturer, is not guaranteed or endorsed by the publisher.
References
1. Jenq RR, van den Brink MR. Allogeneic Haematopoietic Stem Cell Transplantation: Individualized Stem Cell and Immune Therapy of Cancer. Nat Rev Cancer (2010) 10:213–21. doi: 10.1038/nrc2804
2. Choi SW, Reddy P. Current and Emerging Strategies for the Prevention of Graft-Versus-Host Disease. Nat Rev Clin Oncol (2014) 11:536–47. doi: 10.1038/nrclinonc.2014.102
3. Ferrara JL, Levine JE, Reddy P, Holler E. Graft-Versus-Host Disease. Lancet (2009) 373:1550–61. doi: 10.1016/S0140-6736(09)60237-3
4. Zeiser R, Socié G, Blazar BR. Pathogenesis of Acute Graft-Versus-Host Disease: From Intestinal Microbiota Alterations to Donor T Cell Activation. Br J Haematol (2016) 175:191–207. doi: 10.1111/bjh.14295
5. Toubai T, Magenau J. Immunopathology and Biology-Based Treatment of Steroid-Refractory Graft-Versus-Host Disease. Blood (2020) 136:429–40. doi: 10.1182/blood.2019000953
6. Wu SR, Reddy P. Tissue Tolerance: A Distinct Concept to Control Acute GVHD Severity. Blood (2017) 129:1747–52. doi: 10.1182/blood-2016-09-740431
7. Peled JU, Gomes ALC, Devlin SM, Littmann ER, Taur Y, Sung AD, et al. Microbiota as Predictor of Mortality in Allogeneic Hematopoietic-Cell Transplantation. N Engl J Med (2020) 382:822–34. doi: 10.1056/NEJMoa1900623
8. Turnbaugh PJ, Ley RE, Hamady M, Fraser-Liggett CM, Knight R, Gordon JI. The Human Microbiome Project. Nature (2007) 449:804–10. doi: 10.1038/nature06244
9. David LA, Maurice CF, Carmody RN, Gootenberg DB, Button JE, Wolfe BE, et al. Diet Rapidly and Reproducibly Alters the Human Gut Microbiome. Nature (2014) 505:559–63. doi: 10.1038/nature12820
10. He G, Shankar RA, Chzhan M, Samouilov A, Kuppusamy P, Zweier JL. Noninvasive Measurement of Anatomic Structure and Intraluminal Oxygenation in the Gastrointestinal Tract of Living Mice With Spatial and Spectral EPR Imaging. Proc Natl Acad Sci USA (1999) 96:4586–91. doi: 10.1073/pnas.96.8.4586
11. Espey MG. Role of Oxygen Gradients in Shaping Redox Relationships Between the Human Intestine and Its Microbiota. Free Radic Biol Med (2013) 55:130–40. doi: 10.1016/j.freeradbiomed.2012.10.554
12. den Besten G, van Eunen K, Groen AK, Venema K, Reijngoud DJ, Bakker BM. The Role of Short-Chain Fatty Acids in the Interplay Between Diet, Gut Microbiota, and Host Energy Metabolism. J Lipid Res (2013) 54:2325–40. doi: 10.1194/jlr.R036012
13. Velázquez OC, Lederer HM, Rombeau JL. Butyrate and the Colonocyte. Production, Absorption, Metabolism, and Therapeutic Implications. Adv Exp Med Biol (1997) 427:123–34.
14. Atarashi K, Tanoue T, Shima T, Imaoka A, Kuwahara T, Momose Y, et al. Induction of Colonic Regulatory T Cells by Indigenous Clostridium Species. Science (2011) 331:337–41. doi: 10.1126/science.1198469
15. Arpaia N, Campbell C, Fan X, Dikiy S, van der Veeken J, deRoos P, et al. Metabolites Produced by Commensal Bacteria Promote Peripheral Regulatory T-Cell Generation. Nature (2013) 504:451–5. doi: 10.1038/nature12726
16. Campbell C, McKenney PT, Konstantinovsky D, Isaeva OI, Schizas M, Verter J, et al. Bacterial Metabolism of Bile Acids Promotes Generation of Peripheral Regulatory T Cells. Nature (2020) 581:475–9. doi: 10.1038/s41586-020-2193-0
17. Furusawa Y, Obata Y, Fukuda S, Endo TA, Nakato G, Takahashi D, et al. Commensal Microbe-Derived Butyrate Induces the Differentiation of Colonic Regulatory T Cells. Nature (2013) 504:446–50. doi: 10.1038/nature12721
18. Smith PM, Howitt MR, Panikov N, Michaud M, Gallini CA, Bohlooly YM, et al. The Microbial Metabolites, Short-Chain Fatty Acids, Regulate Colonic Treg Cell Homeostasis. Science (2013) 341:569–73. doi: 10.1126/science.1241165
19. Byndloss MX, Olsan EE, Rivera-Chávez F, Tiffany CR, Cevallos SA, Lokken KL, et al. Microbiota-Activated PPAR-γ Signaling Inhibits Dysbiotic Enterobacteriaceae Expansion. Science (2017) 357:570–5. doi: 10.1126/science.aam9949
20. Porter NT, Martens EC. The Critical Roles of Polysaccharides in Gut Microbial Ecology and Physiology. Annu Rev Microbiol (2017) 71:349–69. doi: 10.1146/annurev-micro-102215-095316
21. El Kaoutari A, Armougom F, Gordon JI, Raoult D, Henrissat B. The Abundance and Variety of Carbohydrate-Active Enzymes in the Human Gut Microbiota. Nat Rev Microbiol (2013) 11:497–504. doi: 10.1038/nrmicro3050
22. Bronner DN, Faber F, Olsan EE, Byndloss MX, Sayed NA, Xu G, et al. Genetic Ablation of Butyrate Utilization Attenuates Gastrointestinal Salmonella Disease. Cell Host Microbe (2018) 23:266–73.e4. doi: 10.1016/j.chom.2018.01.004
23. Spiga L, Winter MG, Furtado de Carvalho T, Zhu W, Hughes ER, Gillis CC, et al. An Oxidative Central Metabolism Enables Salmonella to Utilize Microbiota-Derived Succinate. Cell Host Microbe (2017) 22:291–301.e6. doi: 10.1016/j.chom.2017.07.018
24. Kim YG, Sakamoto K, Seo SU, Pickard JM, Gillilland MG 3rd, Pudlo NA, et al. Neonatal Acquisition of Clostridia Species Protects Against Colonization by Bacterial Pathogens. Science (2017) 356:315–9. doi: 10.1126/science.aag2029
25. Barker N, van de Wetering M, Clevers H. The Intestinal Stem Cell. Genes Dev (2008) 22:1856–64. doi: 10.1101/gad.1674008
26. Donohoe DR, Garge N, Zhang X, Sun W, O’Connell TM, Bunger MK, et al. The Microbiome and Butyrate Regulate Energy Metabolism and Autophagy in the Mammalian Colon. Cell Metab (2011) 13:517–26. doi: 10.1016/j.cmet.2011.02.018
27. Roediger WE. Role of Anaerobic Bacteria in the Metabolic Welfare of the Colonic Mucosa in Man. Gut (1980) 21:793–8. doi: 10.1136/gut.21.9.793
28. Blouin JM, Penot G, Collinet M, Nacfer M, Forest C, Laurent-Puig P, et al. Butyrate Elicits a Metabolic Switch in Human Colon Cancer Cells by Targeting the Pyruvate Dehydrogenase Complex. Int J Cancer (2011) 128:2591–601. doi: 10.1002/ijc.25599
29. Lefebvre M, Paulweber B, Fajas L, Woods J, McCrary C, Colombel JF, et al. Peroxisome Proliferator-Activated Receptor Gamma Is Induced During Differentiation of Colon Epithelium Cells. J Endocrinol (1999) 162:331–40. doi: 10.1677/joe.0.1620331
30. Furuta GT, Turner JR, Taylor CT, Hershberg RM, Comerford K, Narravula S, et al. Hypoxia-Inducible Factor 1-Dependent Induction of Intestinal Trefoil Factor Protects Barrier Function During Hypoxia. J Exp Med (2001) 193:1027–34. doi: 10.1084/jem.193.9.1027
31. Litvak Y, Byndloss MX, Tsolis RM, Bäumler AJ. Dysbiotic Proteobacteria Expansion: A Microbial Signature of Epithelial Dysfunction. Curr Opin Microbiol (2017) 39:1–6. doi: 10.1016/j.mib.2017.07.003
32. Kelly CJ, Zheng L, Campbell EL, Saeedi B, Scholz CC, Bayless AJ, et al. Crosstalk Between Microbiota-Derived Short-Chain Fatty Acids and Intestinal Epithelial HIF Augments Tissue Barrier Function. Cell Host Microbe (2015) 17:662–71. doi: 10.1016/j.chom.2015.03.005
33. Colgan SP, Taylor CT. Hypoxia: An Alarm Signal During Intestinal Inflammation. Nat Rev Gastroenterol Hepatol (2010) 7:281–7. doi: 10.1038/nrgastro.2010.39
34. Belkaid Y, Hand TW. Role of the Microbiota in Immunity and Inflammation. Cell (2014) 157:121–41. doi: 10.1016/j.cell.2014.03.011
35. Wu C, Sartor RB, Huang K, Tonkonogy SL. Transient Activation of Mucosal Effector Immune Responses by Resident Intestinal Bacteria in Normal Hosts is Regulated by Interleukin-10 Signalling. Immunology (2016) 148:304–14. doi: 10.1111/imm.12612
36. Koyama M, Mukhopadhyay P, Schuster IS, Henden AS, Hülsdünker J, Varelias A, et al. MHC Class II Antigen Presentation by the Intestinal Epithelium Initiates Graft-Versus-Host Disease and Is Influenced by the Microbiota. Immunity (2019) 51:885–98.e7. doi: 10.1016/j.immuni.2019.08.011
37. Jones JM, Wilson R, Bealmear PM. Mortality and Gross Pathology of Secondary Disease in Germfree Mouse Radiation Chimeras. Radiat Res (1971) 45:577–88. doi: 10.2307/3573066
38. Buckner CD, Clift RA, Sanders JE, Meyers JD, Counts GW, Farewell VT, et al. Protective Environment for Marrow Transplant Recipients: A Prospective Study. Ann Intern Med (1978) 89:893–901. doi: 10.7326/0003-4819-89-6-893
39. Shono Y, Docampo MD, Peled JU, Perobelli SM, Velardi E, Tsai JJ, et al. Increased GVHD-Related Mortality With Broad-Spectrum Antibiotic Use After Allogeneic Hematopoietic Stem Cell Transplantation in Human Patients and Mice. Sci Transl Med (2016) 8:339ra71. doi: 10.1126/scitranslmed.aaf2311
40. Eckburg PB, Bik EM, Bernstein CN, Purdom E, Dethlefsen L, Sargent M, et al. Diversity of the Human Intestinal Microbial Flora. Science (2005) 308:1635–8. doi: 10.1126/science.1110591
41. Köhler N, Zeiser R. Intestinal Microbiota Influence Immune Tolerance Post Allogeneic Hematopoietic Cell Transplantation and Intestinal GVHD. Front Immunol (2018) 9:3179. doi: 10.3389/fimmu.2018.03179
42. Stein-Thoeringer CK, Nichols KB, Lazrak A, Docampo MD, Slingerland AE, Slingerland JB, et al. Lactose Drives Enterococcus Expansion to Promote Graft-Versus-Host Disease. Science (2019) 366:1143–9. doi: 10.1126/science.aax3760
43. Peled JU, Devlin SM, Staffas A, Lumish M, Khanin R, Littmann ER, et al. Intestinal Microbiota and Relapse After Hematopoietic-Cell Transplantation. J Clin Oncol (2017) 35:1650–9. doi: 10.1200/JCO.2016.70.3348
44. Bowerman KL, Varelias A, Lachner N, Kuns RD, Hill GR, Hugenholtz P. Continuous Pre- and Post-Transplant Exposure to a Disease-Associated Gut Microbiome Promotes Hyper-Acute Graft-Versus-Host Disease in Wild-Type Mice. Gut Microbes (2020) 11:754–70. doi: 10.1080/19490976.2019.1705729
45. Saha SK, Lee SB, Won J, Choi HY, Kim K, Yang GM, et al. Correlation Between Oxidative Stress, Nutrition, and Cancer Initiation. Int J Mol Sci (2017) 18:1544. doi: 10.3390/ijms18071544
46. Wang Z, Wang Q, Wang X, Zhu L, Chen J, Zhang B, et al. Gut Microbial Dysbiosis Is Associated With Development and Progression of Radiation Enteritis During Pelvic Radiotherapy. J Cell Mol Med (2019) 23:3747–56. doi: 10.1111/jcmm.14289
47. Guo H, Chou WC, Lai Y, Liang K, Tam JW, Brickey WJ, et al. Multi-Omics Analyses of Radiation Survivors Identify Radioprotective Microbes and Metabolites. Science (2020) 370:eaay9097. doi: 10.1126/science.aay9097
48. Tuohy KM, Kolida S, Lustenberger AM, Gibson GR. The Prebiotic Effects of Biscuits Containing Partially Hydrolysed Guar Gum and Fructo-Oligosaccharides–A Human Volunteer Study. Br J Nutr (2001) 86:341–8. doi: 10.1079/BJN2001394
49. Falony G, Calmeyn T, Leroy F, De Vuyst L. Coculture Fermentations of Bifidobacterium Species and Bacteroides Thetaiotaomicron Reveal a Mechanistic Insight Into the Prebiotic Effect of Inulin-Type Fructans. Appl Environ Microbiol (2009) 75:2312–9. doi: 10.1128/AEM.02649-08
50. Belenguer A, Duncan SH, Calder AG, Holtrop G, Louis P, Lobley GE, et al. Two Routes of Metabolic Cross-Feeding Between Bifidobacterium Adolescentis and Butyrate-Producing Anaerobes From the Human Gut. Appl Environ Microbiol (2006) 72:3593–9. doi: 10.1128/AEM.72.5.3593-3599.2006
51. Galloway-Peña JR, Smith DP, Sahasrabhojane P, Ajami NJ, Wadsworth WD, Daver NG, et al. The Role of the Gastrointestinal Microbiome in Infectious Complications During Induction Chemotherapy for Acute Myeloid Leukemia. Cancer (2016) 122:2186–96. doi: 10.1002/cncr.30039
52. Huang Y, Yang W, Liu H, Duan J, Zhang Y, Liu M, et al. Effect of High-Dose Methotrexate Chemotherapy on Intestinal Bifidobacteria, Lactobacillus and Escherichia Coli in Children With Acute Lymphoblastic Leukemia. Exp Biol Med (Maywood) (2012) 237:305–11. doi: 10.1258/ebm.2011.011297
53. Bodet CA 3rd, Jorgensen JH, Drutz DJ. Antibacterial Activities of Antineoplastic Agents. Antimicrob Agents Chemother (1985) 28:437–9. doi: 10.1128/AAC.28.3.437
54. Mancini N, Greco R, Pasciuta R, Barbanti MC, Pini G, Morrow OB, et al. Enteric Microbiome Markers as Early Predictors of Clinical Outcome in Allogeneic Hematopoietic Stem Cell Transplant: Results of a Prospective Study in Adult Patients. Open Forum Infect Dis (2017) 4:ofx215. doi: 10.1093/ofid/ofx215
55. Rashidi A, Kaiser T, Shields-Cutler R, Graiziger C, Holtan SG, Rehman TU, et al. Dysbiosis Patterns During Re-Induction/Salvage Versus Induction Chemotherapy for Acute Leukemia. Sci Rep (2019) 9:6083. doi: 10.1038/s41598-019-42652-6
56. Kusakabe S, Fukushima K, Maeda T, Motooka D, Nakamura S, Fujita J, et al. Pre- and Post-Serial Metagenomic Analysis of Gut Microbiota as a Prognostic Factor in Patients Undergoing Haematopoietic Stem Cell Transplantation. Br J Haematol (2020) 188:438–49. doi: 10.1111/bjh.16205
57. Freifeld AG, Bow EJ, Sepkowitz KA, Boeckh MJ, Ito JI, Mullen CA, et al. Clinical Practice Guideline for the Use of Antimicrobial Agents in Neutropenic Patients With Cancer: 2010 Update by the Infectious Diseases Society of America. Clin Infect Dis (2011) 52:427–31. doi: 10.1093/cid/ciq147
58. Velasco E, Byington R, Martins CA, Schirmer M, Dias LM, Gonçalves VM. Comparative Study of Clinical Characteristics of Neutropenic and Non-Neutropenic Adult Cancer Patients With Bloodstream Infections. Eur J Clin Microbiol Infect Dis (2006) 25:1–7. doi: 10.1007/s10096-005-0077-8
59. Taur Y, Pamer EG. Microbiome Mediation of Infections in the Cancer Setting. Genome Med (2016) 8:40. doi: 10.1186/s13073-016-0306-z
60. Gafter-Gvili A, Fraser A, Paul M, van de Wetering M, Kremer L, Leibovici L. Antibiotic Prophylaxis for Bacterial Infections in Afebrile Neutropenic Patients Following Chemotherapy. Cochrane Database Syst Rev (2005) Cd004386. doi: 10.1002/14651858.CD004386.pub2
61. Montassier E, Batard E, Massart S, Gastinne T, Carton T, Caillon J, et al. 16s rRNA Gene Pyrosequencing Reveals Shift in Patient Faecal Microbiota During High-Dose Chemotherapy as Conditioning Regimen for Bone Marrow Transplantation. Microb Ecol (2014) 67:690–9. doi: 10.1007/s00248-013-0355-4
62. Weber D, Oefner PJ, Dettmer K, Hiergeist A, Koestler J, Gessner A, et al. Rifaximin Preserves Intestinal Microbiota Balance in Patients Undergoing Allogeneic Stem Cell Transplantation. Bone Marrow Transplant (2016) 51:1087–92. doi: 10.1038/bmt.2016.66
63. Weber D, Hiergeist A, Weber M, Dettmer K, Wolff D, Hahn J, et al. Detrimental Effect of Broad-Spectrum Antibiotics on Intestinal Microbiome Diversity in Patients After Allogeneic Stem Cell Transplantation: Lack of Commensal Sparing Antibiotics. Clin Infect Dis (2019) 68:1303–10. doi: 10.1093/cid/ciy711
64. Routy B, Letendre C, Enot D, Chénard-Poirier M, Mehraj V, Séguin NC, et al. The Influence of Gut-Decontamination Prophylactic Antibiotics on Acute Graft-Versus-Host Disease and Survival Following Allogeneic Hematopoietic Stem Cell Transplantation. Oncoimmunology (2017) 6:e1258506. doi: 10.1080/2162402X.2016.1258506
65. Weber D, Jenq RR, Peled JU, Taur Y, Hiergeist A, Koestler J, et al. Microbiota Disruption Induced by Early Use of Broad-Spectrum Antibiotics Is an Independent Risk Factor of Outcome After Allogeneic Stem Cell Transplantation. Biol Blood Marrow Transplant (2017) 23:845–52. doi: 10.1016/j.bbmt.2017.02.006
66. Morjaria S, Schluter J, Taylor BP, Littmann ER, Carter RA, Fontana E, et al. Antibiotic-Induced Shifts in Fecal Microbiota Density and Composition During Hematopoietic Stem Cell Transplantation. Infect Immun (2019) 87. doi: 10.1128/IAI.00206-19
67. Simms-Waldrip TR, Sunkersett G, Coughlin LA, Savani MR, Arana C, Kim J, et al. Antibiotic-Induced Depletion of Anti-Inflammatory Clostridia Is Associated With the Development of Graft-Versus-Host Disease in Pediatric Stem Cell Transplantation Patients. Biol Blood Marrow Transplant (2017) 23:820–9. doi: 10.1016/j.bbmt.2017.02.004
68. Mathewson ND, Jenq R, Mathew AV, Koenigsknecht M, Hanash A, Toubai T, et al. Gut Microbiome-Derived Metabolites Modulate Intestinal Epithelial Cell Damage and Mitigate Graft-Versus-Host Disease. Nat Immunol (2016) 17:505–13. doi: 10.1038/ni.3400
69. Lee SE, Lim JY, Ryu DB, Kim TW, Park SS, Jeon YW, et al. Alteration of the Intestinal Microbiota by Broad-Spectrum Antibiotic Use Correlates With the Occurrence of Intestinal Graft-Versus-Host Disease. Biol Blood Marrow Transplant (2019) 25:1933–43. doi: 10.1016/j.bbmt.2019.06.001
70. Romick-Rosendale LE, Haslam DB, Lane A, Denson L, Lake K, Wilkey A, et al. Antibiotic Exposure and Reduced Short Chain Fatty Acid Production After Hematopoietic Stem Cell Transplant. Biol Blood Marrow Transplant (2018) 24:2418–24. doi: 10.1016/j.bbmt.2018.07.030
71. Taur Y, Xavier JB, Lipuma L, Ubeda C, Goldberg J, Gobourne A, et al. Intestinal Domination and the Risk of Bacteremia in Patients Undergoing Allogeneic Hematopoietic Stem Cell Transplantation. Clin Infect Dis (2012) 55:905–14. doi: 10.1093/cid/cis580
72. Foster KR, Schluter J, Coyte KZ, Rakoff-Nahoum S. The Evolution of the Host Microbiome as an Ecosystem on a Leash. Nature (2017) 548:43–51. doi: 10.1038/nature23292
73. Shin NR, Whon TW, Bae JW. Proteobacteria: Microbial Signature of Dysbiosis in Gut Microbiota. Trends Biotechnol (2015) 33:496–503. doi: 10.1016/j.tibtech.2015.06.011
74. Rizzatti G, Lopetuso LR, Gibiino G, Binda C, Gasbarrini A. Proteobacteria: A Common Factor in Human Diseases. BioMed Res Int (2017) 2017:9351507. doi: 10.1155/2017/9351507
75. Devkota S, Wang Y, Musch MW, Leone V, Fehlner-Peach H, Nadimpalli A, et al. Dietary-Fat-Induced Taurocholic Acid Promotes Pathobiont Expansion and Colitis in Il10-/- Mice. Nature (2012) 487:104–8. doi: 10.1038/nature11225
76. Martinez-Medina M, Denizot J, Dreux N, Robin F, Billard E, Bonnet R, et al. Western Diet Induces Dysbiosis With Increased E Coli in CEABAC10 Mice, Alters Host Barrier Function Favouring AIEC Colonisation. Gut (2014) 63:116–24. doi: 10.1136/gutjnl-2012-304119
77. Morgan XC, Tickle TL, Sokol H, Gevers D, Devaney KL, Ward DV, et al. Dysfunction of the Intestinal Microbiome in Inflammatory Bowel Disease and Treatment. Genome Biol (2012) 13:R79. doi: 10.1186/gb-2012-13-9-r79
78. Arthur JC, Perez-Chanona E, Mühlbauer M, Tomkovich S, Uronis JM, Fan TJ, et al. Intestinal Inflammation Targets Cancer-Inducing Activity of the Microbiota. Science (2012) 338:120–3. doi: 10.1126/science.1224820
79. Krogius-Kurikka L, Lyra A, Malinen E, Aarnikunnas J, Tuimala J, Paulin L, et al. Microbial Community Analysis Reveals High Level Phylogenetic Alterations in the Overall Gastrointestinal Microbiota of Diarrhoea-Predominant Irritable Bowel Syndrome Sufferers. BMC Gastroenterol (2009) 9:95. doi: 10.1186/1471-230X-9-95
80. Normann E, Fahlén A, Engstrand L, Lilja HE. Intestinal Microbial Profiles in Extremely Preterm Infants With and Without Necrotizing Enterocolitis. Acta Paediatr (2013) 102:129–36. doi: 10.1111/apa.12059
81. Rigottier-Gois L. Dysbiosis in Inflammatory Bowel Diseases: The Oxygen Hypothesis. Isme J (2013) 7:1256–61. doi: 10.1038/ismej.2013.80
82. Rivera-Chávez F, Lopez CA, Bäumler AJ. Oxygen as a Driver of Gut Dysbiosis. Free Radic Biol Med (2017) 105:93–101. doi: 10.1016/j.freeradbiomed.2016.09.022
83. Spees AM, Wangdi T, Lopez CA, Kingsbury DD, Xavier MN, Winter SE, et al. Streptomycin-Induced Inflammation Enhances Escherichia Coli Gut Colonization Through Nitrate Respiration. mBio (2013) 4:e00430-13. doi: 10.1128/mBio.00430-13
84. Gillis CC, Hughes ER, Spiga L, Winter MG, Zhu W, Furtado de Carvalho T, et al. Dysbiosis-Associated Change in Host Metabolism Generates Lactate to Support Salmonella Growth. Cell Host Microbe (2018) 23:54–64.e6. doi: 10.1016/j.chom.2017.11.006
85. Reese AT, Cho EH, Klitzman B, Nichols SP, Wisniewski NA, Villa MM, et al. Antibiotic-Induced Changes in the Microbiota Disrupt Redox Dynamics in the Gut. Elife (2018) 7:e35987. doi: 10.7554/eLife.35987
86. Golob JL, DeMeules MM, Loeffelholz T, Quinn ZZ, Dame MK, Silvestri SS, et al. Butyrogenic Bacteria After Acute Graft-Versus-Host Disease (GVHD) are Associated With the Development of Steroid-Refractory GVHD. Blood Adv (2019) 3:2866–9. doi: 10.1182/bloodadvances.2019000362
87. Vital M, Howe AC, Tiedje JM. Revealing the Bacterial Butyrate Synthesis Pathways by Analyzing (Meta)Genomic Data. mBio (2014) 5:e00889. doi: 10.1128/mBio.00889-14
88. Clausen MR, Mortensen PB. Kinetic Studies on the Metabolism of Short-Chain Fatty Acids and Glucose by Isolated Rat Colonocytes. Gastroenterology (1994) 106:423–32. doi: 10.1016/0016-5085(94)90601-7
89. Litvak Y, Byndloss MX, Bäumler AJ. Colonocyte Metabolism Shapes the Gut Microbiota. Science (2018) 362:eaat9076. doi: 10.1126/science.aat9076
90. Long C, Liu Y, He L, Tan Q, Yu Z, Xiao N, et al. Bacterial Lactase Genes Diversity in Intestinal Mucosa of Mice With Dysbacterial Diarrhea Induced by Antibiotics. 3 Biotech (2018) 8:176. doi: 10.1007/s13205-018-1191-5
91. Makarova K, Slesarev A, Wolf Y, Sorokin A, Mirkin B, Koonin E, et al. Comparative Genomics of the Lactic Acid Bacteria. Proc Natl Acad Sci USA (2006) 103:15611–6. doi: 10.1073/pnas.0607117103
92. Rhimi M, Aghajari N, Jaouadi B, Juy M, Boudebbouze S, Maguin E, et al. Exploring the Acidotolerance of Beta-Galactosidase From Lactobacillus Delbrueckii Subsp. Bulgaricus: An Attractive Enzyme for Lactose Bioconversion. Res Microbiol (2009) 160:775–84. doi: 10.1016/j.resmic.2009.09.004
93. Russo P, Arena MP, Fiocco D, Capozzi V, Drider D, Spano G. Lactobacillus Plantarum With Broad Antifungal Activity: A Promising Approach to Increase Safety and Shelf-Life of Cereal-Based Products. Int J Food Microbiol (2017) 247:48–54. doi: 10.1016/j.ijfoodmicro.2016.04.027
94. Cabral ME, Abeijón Mukdsi MC, Medina de Figueroa RB, González SN. Citrate Metabolism by Enterococcus Faecium and Enterococcus Durans Isolated From Goat’s and Ewe’s Milk: Influence of Glucose and Lactose. Can J Microbiol (2007) 53:607–15. doi: 10.1139/W07-011
95. Huycke MM, Abrams V, Moore DR. Enterococcus Faecalis Produces Extracellular Superoxide and Hydrogen Peroxide That Damages Colonic Epithelial Cell DNA. Carcinogenesis (2002) 23:529–36. doi: 10.1093/carcin/23.3.529
96. Zitvogel L, Kroemer G. Immunostimulatory Gut Bacteria. Science (2019) 366:1077–8. doi: 10.1126/science.aaz7595
97. Bäckhed F, Ley RE, Sonnenburg JL, Peterson DA, Gordon JI. Host-Bacterial Mutualism in the Human Intestine. Science (2005) 307:1915–20. doi: 10.1126/science.1104816
98. Postler TS, Ghosh S. Understanding the Holobiont: How Microbial Metabolites Affect Human Health and Shape the Immune System. Cell Metab (2017) 26:110–30. doi: 10.1016/j.cmet.2017.05.008
99. Koh A, De Vadder F, Kovatcheva-Datchary P, Bäckhed F. From Dietary Fiber to Host Physiology: Short-Chain Fatty Acids as Key Bacterial Metabolites. Cell (2016) 165:1332–45. doi: 10.1016/j.cell.2016.05.041
100. Willemsen LE, Koetsier MA, van Deventer SJ, van Tol EA. Short Chain Fatty Acids Stimulate Epithelial Mucin 2 Expression Through Differential Effects on Prostaglandin E(1) and E(2) Production by Intestinal Myofibroblasts. Gut (2003) 52:1442–7. doi: 10.1136/gut.52.10.1442
101. Gaudier E, Jarry A, Blottière HM, de Coppet P, Buisine MP, Aubert JP, et al. Butyrate Specifically Modulates MUC Gene Expression in Intestinal Epithelial Goblet Cells Deprived of Glucose. Am J Physiol Gastrointest Liver Physiol (2004) 287:G1168–74. doi: 10.1152/ajpgi.00219.2004
102. Wrzosek L, Miquel S, Noordine ML, Bouet S, Joncquel Chevalier-Curt M, Robert V, et al. Bacteroides Thetaiotaomicron and Faecalibacterium Prausnitzii Influence the Production of Mucus Glycans and the Development of Goblet Cells in the Colonic Epithelium of a Gnotobiotic Model Rodent. BMC Biol (2013) 11:61. doi: 10.1186/1741-7007-11-61
103. Fukuda S, Toh H, Hase K, Oshima K, Nakanishi Y, Yoshimura K, et al. Bifidobacteria can Protect From Enteropathogenic Infection Through Production of Acetate. Nature (2011) 469:543–7. doi: 10.1038/nature09646
104. Atarashi K, Tanoue T, Oshima K, Suda W, Nagano Y, Nishikawa H, et al. Treg Induction by a Rationally Selected Mixture of Clostridia Strains From the Human Microbiota. Nature (2013) 500:232–6. doi: 10.1038/nature12331
105. Macia L, Tan J, Vieira AT, Leach K, Stanley D, Luong S, et al. Metabolite-Sensing Receptors GPR43 and GPR109A Facilitate Dietary Fibre-Induced Gut Homeostasis Through Regulation of the Inflammasome. Nat Commun (2015) 6:6734. doi: 10.1038/ncomms7734
106. Singh N, Gurav A, Sivaprakasam S, Brady E, Padia R, Shi H, et al. Activation of Gpr109a, Receptor for Niacin and the Commensal Metabolite Butyrate, Suppresses Colonic Inflammation and Carcinogenesis. Immunity (2014) 40:128–39. doi: 10.1016/j.immuni.2013.12.007
107. Markey KA, Schluter J, Gomes ALC, Littmann ER, Pickard AJ, Taylor BP, et al. The Microbe-Derived Short-Chain Fatty Acids Butyrate and Propionate are Associated With Protection From Chronic GVHD. Blood (2020) 136:130–6. doi: 10.1182/blood.2019003369
108. Fujiwara H, Docampo MD, Riwes M, Peltier D, Toubai T, Henig I, et al. Microbial Metabolite Sensor GPR43 Controls Severity of Experimental GVHD. Nat Commun (2018) 9:3674. doi: 10.1038/s41467-018-06048-w
109. Haak BW, Littmann ER, Chaubard JL, Pickard AJ, Fontana E, Adhi F, et al. Impact of Gut Colonization With Butyrate-Producing Microbiota on Respiratory Viral Infection Following Allo-HCT. Blood (2018) 131:2978–86. doi: 10.1182/blood-2018-01-828996
110. Wu Y, Kudsk KA, DeWitt RC, Tolley EA, Li J. Route and Type of Nutrition Influence IgA-Mediating Intestinal Cytokines. Ann Surg (1999) 229:662–7. doi: 10.1097/00000658-199905000-00008
111. Sigalet DL, Mackenzie SL, Hameed SM. Enteral Nutrition and Mucosal Immunity: Implications for Feeding Strategies in Surgery and Trauma. Can J Surg (2004) 47:109–16.
112. Staffas A, Burgos da Silva M, Slingerland AE, Lazrak A, Bare CJ, Holman CD, et al. Nutritional Support From the Intestinal Microbiota Improves Hematopoietic Reconstitution After Bone Marrow Transplantation in Mice. Cell Host Microbe (2018) 23:447–57.e4. doi: 10.1016/j.chom.2018.03.002
113. Venkataraman A, Sieber JR, Schmidt AW, Waldron C, Theis KR, Schmidt TM. Variable Responses of Human Microbiomes to Dietary Supplementation With Resistant Starch. Microbiome (2016) 4:33. doi: 10.1186/s40168-016-0178-x
114. Baxter NT, Schmidt AW, Venkataraman A, Kim KS, Waldron C, Schmidt TM. Dynamics of Human Gut Microbiota and Short-Chain Fatty Acids in Response to Dietary Interventions With Three Fermentable Fibers. mBio (2019) 10:e02566-18. doi: 10.1128/mBio.02566-18
115. Riwes M, Reddy P. Short Chain Fatty Acids: Postbiotics/metabolites and Graft Versus Host Disease Colitis. Semin Hematol (2020) 57:1–6. doi: 10.1053/j.seminhematol.2020.06.001
116. Lindsay JO, Whelan K, Stagg AJ, Gobin P, Al-Hassi HO, Rayment N, et al. Clinical, Microbiological, and Immunological Effects of Fructo-Oligosaccharide in Patients With Crohn’s Disease. Gut (2006) 55:348–55. doi: 10.1136/gut.2005.074971
117. Han K, Nam J, Xu J, Sun X, Huang X, Animasahun O, et al. Generation of Systemic Antitumour Immunity via the in Situ Modulation of the Gut Microbiome by an Orally Administered Inulin Gel. Nat BioMed Eng (2021). doi: 10.1038/s41551-021-00749-2
118. Russell DW. The Enzymes, Regulation, and Genetics of Bile Acid Synthesis. Annu Rev Biochem (2003) 72:137–74. doi: 10.1146/annurev.biochem.72.121801.161712
119. Monte MJ, Marin JJ, Antelo A, Vazquez-Tato J. Bile Acids: Chemistry, Physiology, and Pathophysiology. World J Gastroenterol (2009) 15:804–16. doi: 10.3748/wjg.15.804
120. Inagaki T, Moschetta A, Lee YK, Peng L, Zhao G, Downes M, et al. Regulation of Antibacterial Defense in the Small Intestine by the Nuclear Bile Acid Receptor. Proc Natl Acad Sci USA (2006) 103:3920–5. doi: 10.1073/pnas.0509592103
121. Pols TW, Nomura M, Harach T, Lo Sasso G, Oosterveer MH, Thomas C, et al. TGR5 Activation Inhibits Atherosclerosis by Reducing Macrophage Inflammation and Lipid Loading. Cell Metab (2011) 14:747–57. doi: 10.1016/j.cmet.2011.11.006
122. Joshi NM, Hassan S, Jasani P, Dixon S, Cavenagh JD, Oakervee HE, et al. Bile Acid Malabsorption in Patients With Graft-Versus-Host Disease of the Gastrointestinal Tract. Br J Haematol (2012) 157:403–7. doi: 10.1111/j.1365-2141.2011.09014.x
123. Michonneau D, Latis E, Curis E, Dubouchet L, Ramamoorthy S, Ingram B, et al. Metabolomics Analysis of Human Acute Graft-Versus-Host Disease Reveals Changes in Host and Microbiota-Derived Metabolites. Nat Commun (2019) 10:5695. doi: 10.1038/s41467-019-13498-3
124. Ruutu T, Eriksson B, Remes K, Juvonen E, Volin L, Remberger M, et al. Ursodeoxycholic Acid for the Prevention of Hepatic Complications in Allogeneic Stem Cell Transplantation. Blood (2002) 100:1977–83. doi: 10.1182/blood-2001-12-0159
125. Ghimire L, Paudel S, Jin L, Jeyaseelan S. The NLRP6 Inflammasome in Health and Disease. Mucosal Immunol (2020) 13:388–98. doi: 10.1038/s41385-020-0256-z
126. Toubai T, Fujiwara H, Rossi C, Riwes M, Tamaki H, Zajac C, et al. Host NLRP6 Exacerbates Graft-Versus-Host Disease Independent of Gut Microbial Composition. Nat Microbiol (2019) 4:800–12. doi: 10.1038/s41564-019-0373-1
127. Zelante T, Iannitti RG, Cunha C, De Luca A, Giovannini G, Pieraccini G, et al. Tryptophan Catabolites From Microbiota Engage Aryl Hydrocarbon Receptor and Balance Mucosal Reactivity via Interleukin-22. Immunity (2013) 39:372–85. doi: 10.1016/j.immuni.2013.08.003
128. Roager HM, Licht TR. Microbial Tryptophan Catabolites in Health and Disease. Nat Commun (2018) 9:3294. doi: 10.1038/s41467-018-05470-4
129. Weber D, Oefner PJ, Hiergeist A, Koestler J, Gessner A, Weber M, et al. Low Urinary Indoxyl Sulfate Levels Early After Transplantation Reflect a Disrupted Microbiome and Are Associated With Poor Outcome. Blood (2015) 126:1723–8. doi: 10.1182/blood-2015-04-638858
130. Kim HY, Yoo TH, Hwang Y, Lee GH, Kim B, Jang J, et al. Indoxyl Sulfate (IS)-Mediated Immune Dysfunction Provokes Endothelial Damage in Patients With End-Stage Renal Disease (ESRD). Sci Rep (2017) 7:3057. doi: 10.1038/s41598-017-03130-z
131. Li Y, Innocentin S, Withers DR, Roberts NA, Gallagher AR, Grigorieva EF, et al. Exogenous Stimuli Maintain Intraepithelial Lymphocytes via Aryl Hydrocarbon Receptor Activation. Cell (2011) 147:629–40. doi: 10.1016/j.cell.2011.09.025
132. Kiss EA, Vonarbourg C, Kopfmann S, Hobeika E, Finke D, Esser C, et al. Natural Aryl Hydrocarbon Receptor Ligands Control Organogenesis of Intestinal Lymphoid Follicles. Science (2011) 334:1561–5. doi: 10.1126/science.1214914
133. Schiering C, Wincent E, Metidji A, Iseppon A, Li Y, Potocnik AJ, et al. Feedback Control of AHR Signalling Regulates Intestinal Immunity. Nature (2017) 542:242–5. doi: 10.1038/nature21080
134. Swimm A, Giver CR, DeFilipp Z, Rangaraju S, Sharma A, Ulezko Antonova A, et al. Indoles Derived From Intestinal Microbiota Act via Type I Interferon Signaling to Limit Graft-Versus-Host Disease. Blood (2018) 132:2506–19. doi: 10.1182/blood-2018-03-838193
135. Dant TA, Lin KL, Bruce DW, Montgomery SA, Kolupaev OV, Bommiasamy H, et al. T-Cell Expression of AhR Inhibits the Maintenance of Pt(Reg) Cells in the Gastrointestinal Tract in Acute GVHD. Blood (2017) 130:348–59. doi: 10.1182/blood-2016-08-734244
136. de Loor H, Bammens B, Evenepoel P, De Preter V, Verbeke K. Gas Chromatographic-Mass Spectrometric Analysis for Measurement of P-Cresol and Its Conjugated Metabolites in Uremic and Normal Serum. Clin Chem (2005) 51:1535–8. doi: 10.1373/clinchem.2005.050781
137. Fernstrom JD, Fernstrom MH. Tyrosine, Phenylalanine, and Catecholamine Synthesis and Function in the Brain. J Nutr (2007) 137:1539S–47S. discussion 1548S. doi: 10.1093/jn/137.6.1539S
138. Kühn S, Düzel S, Colzato L, Norman K, Gallinat J, Brandmaier AM, et al. Food for Thought: Association Between Dietary Tyrosine and Cognitive Performance in Younger and Older Adults. Psychol Res (2019) 83:1097–106. doi: 10.1007/s00426-017-0957-4
139. Li X, Lin Y, Li X, Xu X, Zhao Y, Xu L, et al. Tyrosine Supplement Ameliorates Murine aGVHD by Modulation of Gut Microbiome and Metabolome. EBioMedicine (2020) 61:103048. doi: 10.1016/j.ebiom.2020.103048
140. Reikvam H, Hatfield K, Bruserud Ø. The Pretransplant Systemic Metabolic Profile Reflects a Risk of Acute Graft Versus Host Disease After Allogeneic Stem Cell Transplantation. Metabolomics (2016) 12:12. doi: 10.1007/s11306-015-0880-x
141. Janeiro MH, Ramírez MJ, Milagro FI, Martínez JA, Solas M. Implication of Trimethylamine N-Oxide (TMAO) in Disease: Potential Biomarker or New Therapeutic Target. Nutrients (2018) 10:1398. doi: 10.3390/nu10101398
142. Wang Z, Klipfell E, Bennett BJ, Koeth R, Levison BS, Dugar B, et al. Gut Flora Metabolism of Phosphatidylcholine Promotes Cardiovascular Disease. Nature (2011) 472:57–63. doi: 10.1038/nature09922
143. Wu K, Yuan Y, Yu H, Dai X, Wang S, Sun Z, et al. The Gut Microbial Metabolite Trimethylamine N-Oxide Aggravates GVHD by Inducing M1 Macrophage Polarization in Mice. Blood (2020) 136:501–15. doi: 10.1182/blood.2019003990
144. Romano KA, Vivas EI, Amador-Noguez D, Rey FE. Intestinal Microbiota Composition Modulates Choline Bioavailability From Diet and Accumulation of the Proatherogenic Metabolite Trimethylamine-N-Oxide. mBio (2015) 6:e02481. doi: 10.1128/mBio.02481-14
145. Peterson CT, Rodionov DA, Osterman AL, Peterson SN. B Vitamins and Their Role in Immune Regulation and Cancer. Nutrients (2020) 12:3380. doi: 10.3390/nu12113380
146. Gold MC, Lewinsohn DM. Co-Dependents: MR1-Restricted MAIT Cells and Their Antimicrobial Function. Nat Rev Microbiol (2013) 11:14–9. doi: 10.1038/nrmicro2918
147. Varelias A, Bunting MD, Ormerod KL, Koyama M, Olver SD, Straube J, et al. Recipient Mucosal-Associated Invariant T Cells Control GVHD Within the Colon. J Clin Invest (2018) 128:1919–36. doi: 10.1172/JCI91646
148. Bhattacharyya A, Hanafi LA, Sheih A, Golob JL, Srinivasan S, Boeckh MJ, et al. Graft-Derived Reconstitution of Mucosal-Associated Invariant T Cells After Allogeneic Hematopoietic Cell Transplantation. Biol Blood Marrow Transplant (2018) 24:242–51. doi: 10.1016/j.bbmt.2017.10.003
149. Kawaguchi K, Umeda K, Hiejima E, Iwai A, Mikami M, Nodomi S, et al. Influence of Post-Transplant Mucosal-Associated Invariant T Cell Recovery on the Development of Acute Graft-Versus-Host Disease in Allogeneic Bone Marrow Transplantation. Int J Hematol (2018) 108:66–75. doi: 10.1007/s12185-018-2442-2
150. Solders M, Erkers T, Gorchs L, Poiret T, Remberger M, Magalhaes I, et al. Mucosal-Associated Invariant T Cells Display a Poor Reconstitution and Altered Phenotype After Allogeneic Hematopoietic Stem Cell Transplantation. Front Immunol (2017) 8:1861. doi: 10.3389/fimmu.2017.01861
151. Konuma T, Kohara C, Watanabe E, Takahashi S, Ozawa G, Suzuki K, et al. Reconstitution of Circulating Mucosal-Associated Invariant T Cells After Allogeneic Hematopoietic Cell Transplantation: Its Association With the Riboflavin Synthetic Pathway of Gut Microbiota in Cord Blood Transplant Recipients. J Immunol (2020) 204:1462–73. doi: 10.4049/jimmunol.1900681
152. Di Martino ML, Campilongo R, Casalino M, Micheli G, Colonna B, Prosseda G. Polyamines: Emerging Players in Bacteria-Host Interactions. Int J Med Microbiol (2013) 303:484–91. doi: 10.1016/j.ijmm.2013.06.008
153. Zhang M, Wang H, Tracey KJ. Regulation of Macrophage Activation and Inflammation by Spermine: A New Chapter in an Old Story. Crit Care Med (2000) 28:N60–6. doi: 10.1097/00003246-200004001-00007
154. Kibe R, Kurihara S, Sakai Y, Suzuki H, Ooga T, Sawaki E, et al. Upregulation of Colonic Luminal Polyamines Produced by Intestinal Microbiota Delays Senescence in Mice. Sci Rep (2014) 4:4548. doi: 10.1038/srep04548
155. Pérez-Cano FJ, González-Castro A, Castellote C, Franch A, Castell M. Influence of Breast Milk Polyamines on Suckling Rat Immune System Maturation. Dev Comp Immunol (2010) 34:210–8. doi: 10.1016/j.dci.2009.10.001
156. Oku S, Takeshita T, Futatsuki T, Kageyama S, Asakawa M, Mori Y, et al. Disrupted Tongue Microbiota and Detection of Nonindigenous Bacteria on the Day of Allogeneic Hematopoietic Stem Cell Transplantation. PloS Pathog (2020) 16:e1008348. doi: 10.1371/journal.ppat.1008348
157. Lamont RJ, Koo H, Hajishengallis G. The Oral Microbiota: Dynamic Communities and Host Interactions. Nat Rev Microbiol (2018) 16:745–59. doi: 10.1038/s41579-018-0089-x
158. Shouval R, Eshel A, Dubovski B, Kuperman AA, Danylesko I, Fein JA, et al. Patterns of Salivary Microbiota Injury and Oral Mucositis in Recipients of Allogeneic Hematopoietic Stem Cell Transplantation. Blood Adv (2020) 4:2912–7. doi: 10.1182/bloodadvances.2020001827
159. Gupta S, Allen-Vercoe E, Petrof EO. Fecal Microbiota Transplantation: In Perspective. Therap Adv Gastroenterol (2016) 9:229–39. doi: 10.1177/1756283X15607414
160. Kakihana K, Fujioka Y, Suda W, Najima Y, Kuwata G, Sasajima S, et al. Fecal Microbiota Transplantation for Patients With Steroid-Resistant Acute Graft-Versus-Host Disease of the Gut. Blood (2016) 128:2083–8. doi: 10.1182/blood-2016-05-717652
161. Qi X, Li X, Zhao Y, Wu X, Chen F, Ma X, et al. Treating Steroid Refractory Intestinal Acute Graft-vs.-Host Disease With Fecal Microbiota Transplantation: A Pilot Study. Front Immunol (2018) 9:2195. doi: 10.3389/fimmu.2018.02195
162. DeFilipp Z, Peled JU, Li S, Mahabamunuge J, Dagher Z, Slingerland AE, et al. Third-Party Fecal Microbiota Transplantation Following Allo-HCT Reconstitutes Microbiome Diversity. Blood Adv (2018) 2:745–53. doi: 10.1182/bloodadvances.2018017731
163. Taur Y, Coyte K, Schluter J, Robilotti E, Figueroa C, Gjonbalaj M, et al. Reconstitution of the Gut Microbiota of Antibiotic-Treated Patients by Autologous Fecal Microbiota Transplant. Sci Transl Med (2018) 10:eaap9489. doi: 10.1126/scitranslmed.aap9489
164. Spindelboeck W, Schulz E, Uhl B, Kashofer K, Aigelsreiter A, Zinke-Cerwenka W, et al. Repeated Fecal Microbiota Transplantations Attenuate Diarrhea and Lead to Sustained Changes in the Fecal Microbiota in Acute, Refractory Gastrointestinal Graft-Versus-Host-Disease. Haematologica (2017) 102:e210–3. doi: 10.3324/haematol.2016.154351
165. Pichereau C, Desseaux K, Janin A, Scieux C, Peffault de Latour R, Xhaard A, et al. The Complex Relationship Between Human Herpesvirus 6 and Acute Graft-Versus-Host Disease. Biol Blood Marrow Transplant (2012) 18:141–4. doi: 10.1016/j.bbmt.2011.07.018
166. Legoff J, Resche-Rigon M, Bouquet J, Robin M, Naccache SN, Mercier-Delarue S, et al. The Eukaryotic Gut Virome in Hematopoietic Stem Cell Transplantation: New Clues in Enteric Graft-Versus-Host Disease. Nat Med (2017) 23:1080–5. doi: 10.1038/nm.4380
167. Zhai B, Ola M, Rolling T, Tosini NL, Joshowitz S, Littmann ER, et al. High-Resolution Mycobiota Analysis Reveals Dynamic Intestinal Translocation Preceding Invasive Candidiasis. Nat Med (2020) 26:59–64. doi: 10.1038/s41591-019-0709-7
168. Zhang F, Zuo T, Yeoh YK, Cheng FWT, Liu Q, Tang W, et al. Longitudinal Dynamics of Gut Bacteriome, Mycobiome and Virome After Fecal Microbiota Transplantation in Graft-Versus-Host Disease. Nat Commun (2021) 12:65. doi: 10.1038/s41467-020-20240-x
169. DeFilipp Z, Bloom PP, Torres Soto M, Mansour MK, Sater MRA, Huntley MH, et al. Coli Bacteremia Transmitted by Fecal Microbiota Transplant. N Engl J Med (2019) 381:2043–50. doi: 10.1056/NEJMoa1910437
170. Cammarota G, Ianiro G, Kelly CR, Mullish BH, Allegretti JR, Kassam Z, et al. International Consensus Conference on Stool Banking for Faecal Microbiota Transplantation in Clinical Practice. Gut (2019) 68:2111–21. doi: 10.1136/gutjnl-2019-319548
171. Henig I, Yehudai-Ofir D, Zuckerman T. The Clinical Role of the Gut Microbiome and Fecal Microbiota Transplantation in Allogeneic Stem Cell Transplantation. Haematologica (2020) 106:933–46. doi: 10.3324/haematol.2020.247395
172. Haring E, Uhl FM, Andrieux G, Proietti M, Bulashevska A, Sauer B, et al. Bile Acids Regulate Intestinal Antigen Presentation and Reduce Graft-Versus-Host Disease Without Impairing the Graft-Versus-Leukemia Effect. Haematologica (2020) 106:2132–46. doi: 10.3324/haematol.2019.242990
Keywords: graft-versus-host disease, microbial metabolite, dysbiosis, microbiota, allogeneic stem cell transplantation
Citation: Fujiwara H (2021) Crosstalk Between Intestinal Microbiota Derived Metabolites and Tissues in Allogeneic Hematopoietic Cell Transplantation. Front. Immunol. 12:703298. doi: 10.3389/fimmu.2021.703298
Received: 30 April 2021; Accepted: 09 August 2021;
Published: 27 August 2021.
Edited by:
Antiopi Varelias, The University of Queensland, AustraliaReviewed by:
Luisa Giaccone, University of Turin, ItalyQing Ge, Peking University, China
Jonathan Peled, Memorial Sloan Kettering Cancer Center, United States
Andrea Henden, The University of Queensland, Australia
Copyright © 2021 Fujiwara. This is an open-access article distributed under the terms of the Creative Commons Attribution License (CC BY). The use, distribution or reproduction in other forums is permitted, provided the original author(s) and the copyright owner(s) are credited and that the original publication in this journal is cited, in accordance with accepted academic practice. No use, distribution or reproduction is permitted which does not comply with these terms.
*Correspondence: Hideaki Fujiwara, cG1leDl2M3FAb2theWFtYS11LmFjLmpw