- 1University of Gdansk, Intercollegiate Faculty of Biotechnology of University of Gdansk and Medical University of Gdansk, Gdansk, Poland
- 2Department of in vitro Studies, Institute of Biotechnology and Molecular Medicine, Gdansk, Poland
- 3Radcliffe Department of Medicine, University of Oxford, Oxford, United Kingdom
Extracellular vesicles (EVs), and especially exosomes, have been shown to mediate information exchange between distant cells; this process directly affects the biological characteristics and functionality of the recipient cell. As such, EVs significantly contribute to the shaping of immune responses in both physiology and disease states. While vesicles secreted by immune cells are often implicated in the allergic process, growing evidence indicates that EVs from non-immune cells, produced in the stroma or epithelia of the organs directly affected by inflammation may also play a significant role. In this review, we provide an overview of the mechanisms of allergy to which those EVs contribute, with a particular focus on small EVs (sEVs). Finally, we also give a clinical perspective regarding the utilization of the EV-mediated communication route for the benefit of allergic patients.
Introduction
During evolution multicellular organisms developed diverse methods of communication including a direct cell-to-cell contact, which allows for receptor-ligand interactions as well as the release of active mediators providing intercellular information transfer between donor to recipient cells. These include both soluble molecules and extracellular vesicles (EVs) capable of travelling long distances within the body. EVs which comprise apoptotic bodies (AP; 100-5000 nm), ectosomes or shedding microvesicles (MV; 100–1000 nm), secreted mid-body remnants (sMB-Rs; 200-600 nm) and exosomes (50–150 nm) are a group of heterogeneous structures (1, 2) surrounded by a lipid bilayer. EVs are released from practically all cell types including epithelial cells, fibroblasts, mesenchymal cells, dendritic cells (DCs), B cells, T cells, mast cells and tumor cells, among others. The presence of EVs has also been shown in multiple body fluids, including saliva (3), plasma (4, 5), breast milk (6), urine (7), bronchoalveolar lavage (8, 9) and malignant effusions (10–12). The complete biological effects of EVs are not yet well understood, but it is known that MVs and exosomes can bind to cells through several mechanisms, including receptor-mediated endocytosis, direct fusion, phagocytosis, and caveolae- or clathrin-mediated endocytosis and transfer their content to the recipient cell (1, 13). It has also been shown that alveolar epithelial cells internalize MVs via fluid-phase endocytosis but not via the well-known receptor-mediated EV endocytosis (14); MV uptake has endocytic basis which is energy-consuming and requires cytoskeletal rearrangement (15); receptor-mediated MV uptake has also been reported (16). Because of their morphological characteristics, exosomes are considered the EVs most pronouncely involved in the information exchange process. The uptake results in functional effects in recipients; hence EVs contribute to the complexity of communication stream between distant cells. Besides the size and density, EV heterogeneity also derives from their diverse cargo, making it arduous for researchers to determine their exact functions (17).
Given their ability to regulate physiological and pathological processes (18, 19) there is growing interest focused on the potential of EVs to serve as novel targets for the development of therapeutic and diagnostic strategies. The role of different EV subtypes largely depends on the type and activation state of a cell producing them (20). Exosomes have been found useful in diagnostics as possible biomarkers, e.g. in oncology and nephropathies (21, 22) and as novel therapeutic approach for treating various diseases, including those with a clear immunological pathomechanism, e.g. atopic dermatitis, asthma, arthritis (23–25). In those, EVs produced by the immune cells are the main focus of the EV field. However, multiple kinds of non-immune cells, often overlooked, have been shown as efficient EV sources; these are often significant contributors to the ongoing immune response. This review, therefore, discusses the role of non-immune cell-derived EVs in immune processes in allergy in contrast to the immune cell-derived EVs.
Extracellular Vesicles: Types and Biogenesis
EVs are most frequently categorized based on their biogenesis, and sub-grouped into three major types: exosomes, microvesicles and apoptotic bodies (Figure 1). Recently, a novel type of EVs, namely secreted midbody remnants (sMB-Rs) have also been described, along with yet another type of secreted nanoparticles, i.e. “exomeres”. While the former appear to be membranous structures and are likely true vesicles, a debate on the latter is ongoing (due to the lack of consensus we did not include exomers in Figure 1 and Table 1). The differences in the origin are directly reflected in the variations in the size, morphology, cargo and surface content of those EV populations (Table 1); however, they all likely play a role in cell-to-cell communication, transferring a variety of biological molecules, i.e. proteins, lipids, nucleic acids and small molecular mediators (18, 19, 75–80) to the recipient cell.
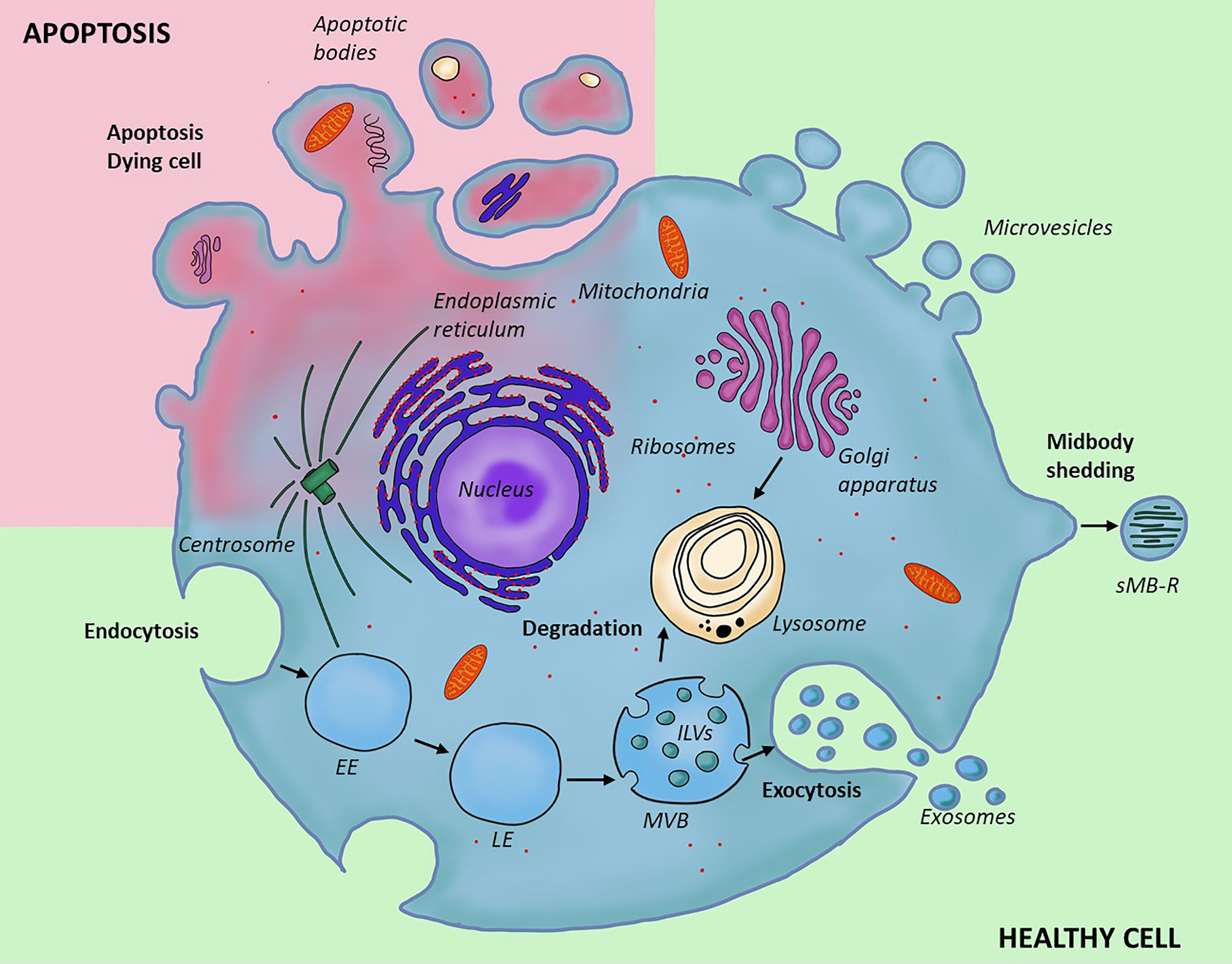
Figure 1 Different types and biogenesis of extracellular vesicles. Two types of EVs form through outward invagination of the plasma membrane; microvesicles and apoptotic bodies. The apoptotic bodies are larger and form in the context of programmed cell death; they enclose organelles removed from the cell during degradation, while microvesicles are produced by a healthy cell; their content is similar to that of the cytoplasm. Secreted midbody remnant is also secreted from the plasma membrane, but contain residual secreted midbody remnants are following cell division. In contrast to this, exosomes form through a distinct cellular pathway and within the endocytic system where inward budding of late endosome leads to the formation of a multivesicular body containing multiple intraluminal vesicles. The content of multivesicular bodies is either digested after fusion with lysosome (degradative pathway) or released into the extracellular space (secretory pathway). EE, early endosome; LE, late endosome; MVB, multivesicular body; ILVs, intraluminal vesicles; sMB-R, secreted midbody remnant.
Exosomes compose a population of small EVs (50-150 nm) (1). Due to their size and composition mainly consisting of lipids, these vesicles can squeeze between cells without damage and enter the circulation; this facilitates transfer of their cargo between cells at the longest distances (55, 81, 82). The exosomal wall composition reflects the biogenesis of those vesicles which have unique endocytic origin. Specifically, exosomes form at the level of late endosomes (LEs) which later progress into multivesicular bodies (MVBs) by accumulation of intraluminal vesicles (ILVs) generated through inward budding of the LE membrane (83). The formation of MVBs is mediated by two separate pathways; one involving a multimolecular machinery called endosomal sorting complex required for transport (ESCRT) and the other, dependent on a specific lipid composition of the endosomal membrane (84). ESCRT is a protein cascade consisting of approximately 30 proteins which are integrated into four subunits, namely ESCRT-0, ESCRT-I, ESCRT-II and ESCRT-III (83, 85, 86). The role of ESCRT-0 is to recognize and sequester ubiquitinated transmembrane proteins in the endosomal membrane which allows the ESCRT-I to bind to these ubiquitinated proteins and activate ESCRT-II to start oligomerization and generation of ESCRT-III. ESCRT-I and ESCRT-II complexes are implicated in the process of membrane deformation which leads to the membrane budding, and ESCRT-III components accomplish vesicle scission (1, 44, 87–89); the ESCRT pathway is ATP-dependent. To disassemble ESCRT subcomplexes from the endosomal membrane, the AAA (ATPases Associated with diverse cellular Activities); ATPase VPS4 (Vacuolar Protein Sorting 4), is required, which enzymatically accomplishes the membrane abscission (90–92). During MVB sorting an accessory factor, ALIX, is required for exosome secretion at the endosome to help sort membrane proteins into vesicles which later bud into MVBs (93, 94). Larios et al. have shown that ALIX- and ESCRT-III–dependent pathway promotes sorting and delivery of exosomal proteins (95). In contrast, the ESCRT-independent pathway relies on the process of converting membrane sphingolipids to ceramides by sphingomyelinase which is necessary for the inward budding and formation of ILVs (57, 96–98). Following the budding, MVBs which accumulate ILVs either fuse with the plasma membrane to release exosomes into the extracellular space via exocytosis (secretory pathway) or fuse with lysosomes and their content is digested by the lysosomal enzymes (degradative pathway) (99–101). The ESCRT-independent formation of ILVs in MVBs has been shown to be regulated by CD63 tetraspanin, which is particularly enriched intracellularly and is mostly localized in the endosomes and lysosomes, although in specialized cells it is also associated with lysosome-related organelles and their endosomal precursors (102, 103). Edgar et al. have shown that the formation of small ILVs requires CD63 (104).
Microvesicles (MVs) are vesicles generally larger than exosomes, with sizes in the 100-1000 nm range but some smaller MVs may be difficult to distinguish from exosomes purely based on the size. However, their biogenesis is completely unrelated; they originate through the processes of direct outward budding and fission of the plasma membrane into the extracellular space (105, 106); this explains why the MV surface markers largely depend on the composition of the plasma membrane (107). Based on the way of how the plasma membrane has emerged during the MV formation, MVs may contain various cell surface proteins, such as ARRDC1 (arrestin domain-containing protein 1) (108, 109), Bin-1 (ampiphysin) (110), EGFR (epidermal growth factor receptor), etc. (111). Released MVs may be taken up via receptor-mediated uptake (16, 112, 113) to transfer their cargo (surface receptors, lipids, proteins, mRNA, miRNA, infectious particles e.g. prions) to the target cells.
Apoptotic bodies (APs) are the largest subfraction of extracellular vesicles (100-5000 nm), formed and released when the cell undergoes programmed cell death, i.e. apoptosis (114, 115). Many changes occur to the cell during this process, including pronounced changes to the plasma membrane. Specifically, the blebbing generates various types of protrusions and APs form and may be released from those (116, 117). APs carry antigens and a variety of biomolecules, intracellular fragments, disrupted and degraded cellular organelles, membranes, released nucleic acids and cytosolic contents (75). APs have been shown to transfer their cargo and content between various cells (30, 118). Interestingly, the communication with the immune cells specifically is commonly mediated by vesicle-associated cytokines or damage-associated molecular patterns (DAMPs) (119). This includes mitochondria-derived N-formylated peptides (120), the nuclear protein High Mobility Group Box 1 (HMGB1) (121), histones (122), calcium-binding S100 proteins (123), heat shock proteins (HSPs) (124), ATP (125), uric acid (126), DNA/RNA and actin among many others (127). This richness of the cargo is perhaps not surprising, given the context of the cell death resulting in AP formation. Immune cells recognize these molecules via pathogen recognition receptors (PRRs) and drive inflammatory responses (117, 128, 129). APs act locally and are removed from the extracellular environment during phagocytosis by macrophages (117, 130, 131).
Very recent developments in the EV field brought identification of two new types of nanoparticles, i.e. exomeres and secreted midbody remnants. Given their novel nature, these are not yet well described, both in relation to their structure and function, however certain aspects are already known which positions these nanoparticles in the interest of the EV field.
Secreted midbody remnants (sMB-Rs), with sizes in the 200-600 nm range have been described as particles generated during the cell division. Specifically, these are generated at the time when daughter cells are still connected with intercellular cytoplasmic bridge; this bridge is cut during the cytokinesis by a transient organelle called midbody which anchors SNARE and exocyst complexes (50, 132). As a consequence, one of the nascent cells retains these midbody remnants and discards them either by autophagy (133) or releases them in the form of secreted vesicles; sMB-Rs. It has been documented that these nanoparticles are distinct from exosomes and shed microvesicles (51, 52). While generated as a byproduct during the cell division, sMB-Rs may also convey messages when internalized, as shown for fibroblasts, in which sMB-Rs promote cellular transformation into an invasive phenotype (2).
Exomeres, with their size at the ≤50 nm mark are the smallest secreted nanoparticles described so far. They also have very distinct characteristics; of all, the lack of a limiting membrane is the most evident differential feature. Exomeres seem to be involved in cargo transport and have been shown to contain proteins, lipids and nucleic acids, which provide functional outcome by receiving cells. Currently, however there is a debate whether exomers should be classified as “vesicles” and the EV field is awaiting specific recommendations in this regard (134–136).
It should be noted that while distinct types of EVs can be described by their origin pathway, as well as a set of specific characteristics including the size, marker profile and cargo content, the technical caveats and lack of very unique markers available to unambiguously define every EV population, it is virtually impossible to specify the origin of the EVs, unless these are imaged during secretion. Therefore, following the recommendation of the International Society for Extracellular Vesicles (ISEV) for the purpose of this review we have used the terms “small” and medium/large EVs” (sEVs and m/lEVs) throughout instead of the original description published in the referenced papers unless the populations are very well defined according to the ISEV guidelines (137).
Immune Cell-Derived EVs in Immunity
Recent progress in the EV field determined that thorough understanding of the EV biology and function is pivotal for our comprehension of immune-driven diseases, including the pathogenesis of allergy. Here, immune cell-derived EVs emerge as important contributors to immune responses, in both the innate and adaptive immunity arms and it may be useful to explore their potential as diagnostic and therapeutic tools. In the innate immunity pathways EVs provided by NK cells, macrophages and neutrophils mediate early host recognition and elimination of invading pathogens. In the adaptive arm, EVs are capable of activating B cells for antibody responses as well as providing both the direct and indirect antigen-specific stimulation to T cells. For the former, class I and class II MHC molecule-enriched EVs from antigen-pulsed DC are able to act as a display system for antigen presentation to cytotoxic and helper T cells (138). Moreover, it has been shown recently that the responses induced by exosomes (defined as tetraspanin and syntenin-positive sEVs) are by far superior in comparison to those obtained from MVs (distinguished as actinin-1-positive, syntenin-negative) (34), further highlighting the distinctive features resulting from the unique exosomal biogenesis pathway, encompassing the MHC-reach cellular compartments. As far as the indirect presentation is concerned, antigen or antigen/MHC complex transfer is also engaged, as well as cross-priming and cross-dressing presentation pathways. These topics have been extensively covered already in excellent publications (138–144). Hence, since the focus of this review is the EVs secreted by non-immune cells which are often overlooked but also extensively participate in immune responses, their contribution will be presented next.
Non-Immune Cell-Derived EVs in Immunity
While not as potent, in some respects, as the EVs secreted from the immune cells, the EVs that are produced by the non-immune cell types have also been shown to exert many distinct roles in the immune system. These non-immune EV-mediated pathways include contribution to both innate and adaptive immunity, ranging from the activating to inhibitory roles (Figure 2). As with any cells, the relative impact depends on the type and the activation state of the donor cell, in parallel to the functionality observed at the cellular level. Next section will discuss the ways in which those non-immune cell-derived EVs participate in the mechanisms of the innate and adaptive immunity.
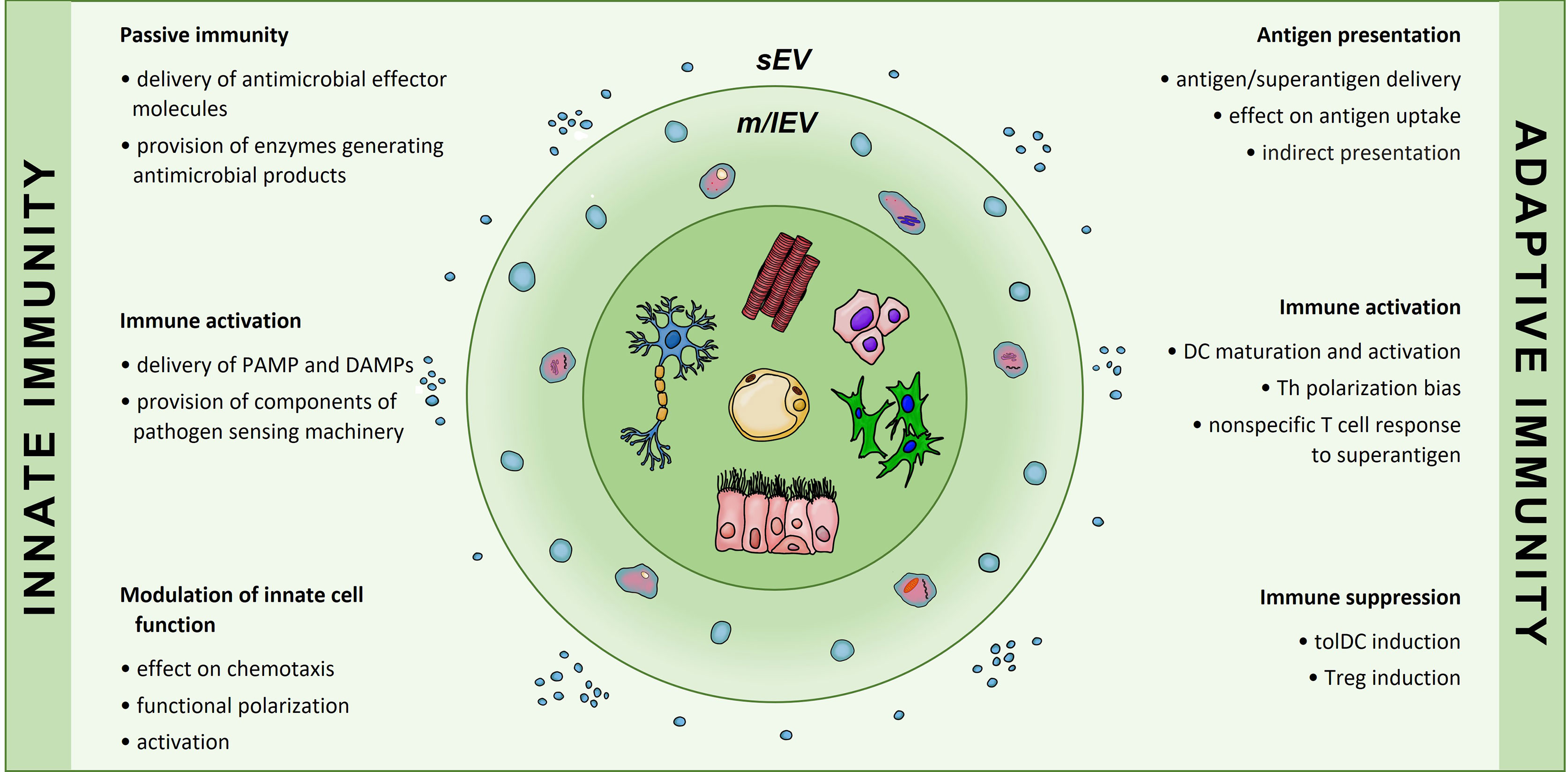
Figure 2 Involvement of non-immune cell-secreted extracellular vesicles in immunological processes of innate and adaptive immunity. Extracellular vesicles produced by cells of non-immune origin participate in exchange of information that contributes to immune responses. In the innate arm EVs enable passive immunity and may both induce activation and modulate innate cell function. In the adaptive arm EVs may influence antigen presentation, affect dendritic cell differentiation and phenotype; they have also been implicated in T cell polarization into Th or Treg subsets. sEVs, small EVs; m/lEVs, medium/large EVs.
Non-Immune Cell-Derived EVs in Innate Immunity
EVs secreted by non-immune cells provide mechanisms of innate control and consist a link between innate immunity and allergic diseases (145). For example, Hu et al. have shown that the activation of the TLR4 signaling results in enhanced luminal sEV production and shuttling of the epithelial antimicrobial peptides (cathelicidin-37 and β-defensin 2) from the gastrointestinal epithelium (146). Nasal mucosa-derived sEVs were also shown to carry proteins involved in the innate immune responses, including inducible nitric oxide synthase (NOS2) which exerts antimicrobial function (147). Similarly to this, Nocera et al. have shown that the secretion of basal nasal mucosa-derived sEVs and the expression of exosomal NO is increased after TLR4-stimulation by lipopolysaccharide. Interestingly, mucosa-derived sEVs had microbiocidal properties and were capable of transferring their immunoprotective cargo to naive epithelial cells to confer passive immunity to recipient cells in the setting of chronic rhinosinusitis (148).
Non-immune cell-derived sEVs can also interfere with the NOD-dependent signaling. Specifically, Vaccari et al. have shown that the expression of the components of the nucleotide-binding-and-oligomerization domain (NOD)-like receptor protein-1 (NLRP-1) inflammasome are increased in the spinal cord motor neurons and cortical neurons after trauma. Interestingly, NLPR-1 inflammasome proteins were found in cerebrospinal fluid-derived sEVs after spinal cord injury and traumatic brain-injured patients. The authors have shown that sEVs derived from neurons loaded with short-interfering RNA against caspase recruitment domain (CARD) can deliver their cargo and reduce inflammasome activation following spinal cord injury in rodents (149). Following this, Li et al. have demonstrated the ability of hepatocyte-derived sEVs (expressing exosome-associated tetraspanins) to induce acute liver injury in severe heat stress by activating the NOD-like receptor signaling pathway in hepatocytes (150). This pathway seems to provide a link between visceral organs and the central nervous system (CNS) as shown in a hepatic ischemia-reperfusion injury model. Liver transplantation may result in neuronal injury and cognitive dysfunction (151); Zhang et al. have demonstrated that circulating sEVs play critical role in hippocampal and cortical injury through regulating neuronal pyroptosis in rats. The authors have shown that neuronal pyroptotic cell death may be caused by sEVs through TLR4 activation of NLRP3 inflammasome (152).
Exosomal transfer of pathogen recognition pathway components may convey the message to the immune cells, such as monocytes and macrophages. Specifically, Mills et al. have shown that poly(I:C) stimulation induces the release of tenascin C-rich sEV from airway epithelial cells; these may potentiate airway inflammation by promoting cytokine production in macrophages (153). Furthermore, airway epithelial cell-derived sEVs have been shown to induce proliferation and infiltration of undifferentiated macrophages into the lungs under the influence of IL-13 in a murine model (20). In contrast, mesenchymal stem cells (MSC)-derived sEVs are capable of inhibiting macrophage chemotaxis (154), altering the M1/M2 balance (155), inhibiting M1 via miR-147 (156) and stimulating the M2 polarization in monocytes (157).
Non-Immune Cell Derived-EVs in Adaptive Immunity
Multiple studies indicate that non-immune cells, in the steady state, secrete EVs that execute immunoregulatory roles in adaptive immunity. For example, bone marrow MSC-derived EVs have been shown to suppress the Th2/Th17-mediated airway hyperresponsiveness and lung inflammation in a model of Aspergillus hyphal extract-induced allergic airway inflammation (158). Indeed, MSC-derived sEVs have shown immunosuppressive effects on several types of immune cells (159); including inhibition of B cell and DC proliferation (25), B cell maturation (160), and induction of T regulatory cells (Treg) (161–163). More specifically, Gomzikova et al. have demonstrated that MSC-derived EVs alter DC maturation and functional state (164); the antigen uptake by immature DCs was attenuated and the stimulation rendered DCs with a semi-mature phenotype after LPS exposure (165). These phenotypic changes were accompanied by a functional shift in the cytokine production profile from inflammatory to immunoregulatory (164), suggesting that those sEVs could promote tolerogenic DC (tolDC) induction. MSC-derived EVs have been also shown to reduce inflammatory cytokine (IL-23 and IL-22) production (166), enhancing the anti-inflammatory phenotype and regulatory lymphocyte proliferation, and the ability to produce IL-10 and TGF-β (167). Proliferation of T cells has also been shown to decrease after MSC-derived EV treatment in vitro, accompanied by a downregulation in IFN-γ and TNF-α (168). The study by Shigemoto-Kuroda et al. also confirmed that MSC-derived EVs have the ability to suppress Th1 and Th17 development, inhibit antigen presenting cell activation and increase expression of the immunosuppressive cytokine IL-10 (169). In a limited model, murine epidermal keratinocyte-derived sEVs (flotillin and Alix-positive) failed to induce T cell immune response despite some phenotypic effects on DC (170). However, when the donor cells are subjected to IFN-γ activation, keratinocyte-derived sEVs (of exosomal marker characteristics) may act as a transfer vehicle for T cell stimulation by Staphylococcal aureus enterotoxin B. Specifically, in this context HaCaT keratinocytes were shown to produce sEVs that contain MHC class I and class II and were able to drive nonspecific proliferation of CD4+ and CD8+ T cells in vitro (171). This suggests that the relative contribution of non-immune cell-derived sEVs (and potentially other EVs) to the adaptive immunity and T cell reactivity may change depending on the stimulation received by the donor cell; further evidence supports this (172).
Interesting are the results by Admyre et al. who have demonstrated that human breast milk contains sEVs which reveal immunomodulatory features inhibiting T cell cytokine production from PBMC and increasing the number of Foxp3+CD4+CD25+ Tregs in this semi-allogenic system (173, 174). Based on the content of surface molecules, in comparison to the DC-derived sEVs, these sEVs originate from either macrophages and lymphocytes in the breast milk or rather breast epithelial cells (173, 175). To support this, Herwijnen et al. have also shown that human milk-derived EVs contain novel EV-associated bioactive proteins that have distinct functions from other milk proteins; this suggests a novel mechanism of cellular communication between the mother and newborn (176).
The Role of Non-immune Cell-Derived EVs in Allergic Conditions
Many studies have been performed to investigate the involvement of non-immune cell-derived EVs content/cargo with different clinical manifestations of allergy; in this section current research regarding the role and function of non-immune cell-derived EVs in allergic conditions is reviewed. Certainly, for the outcome in allergic inflammation much depends on the source of EVs as summarized in Figure 3.
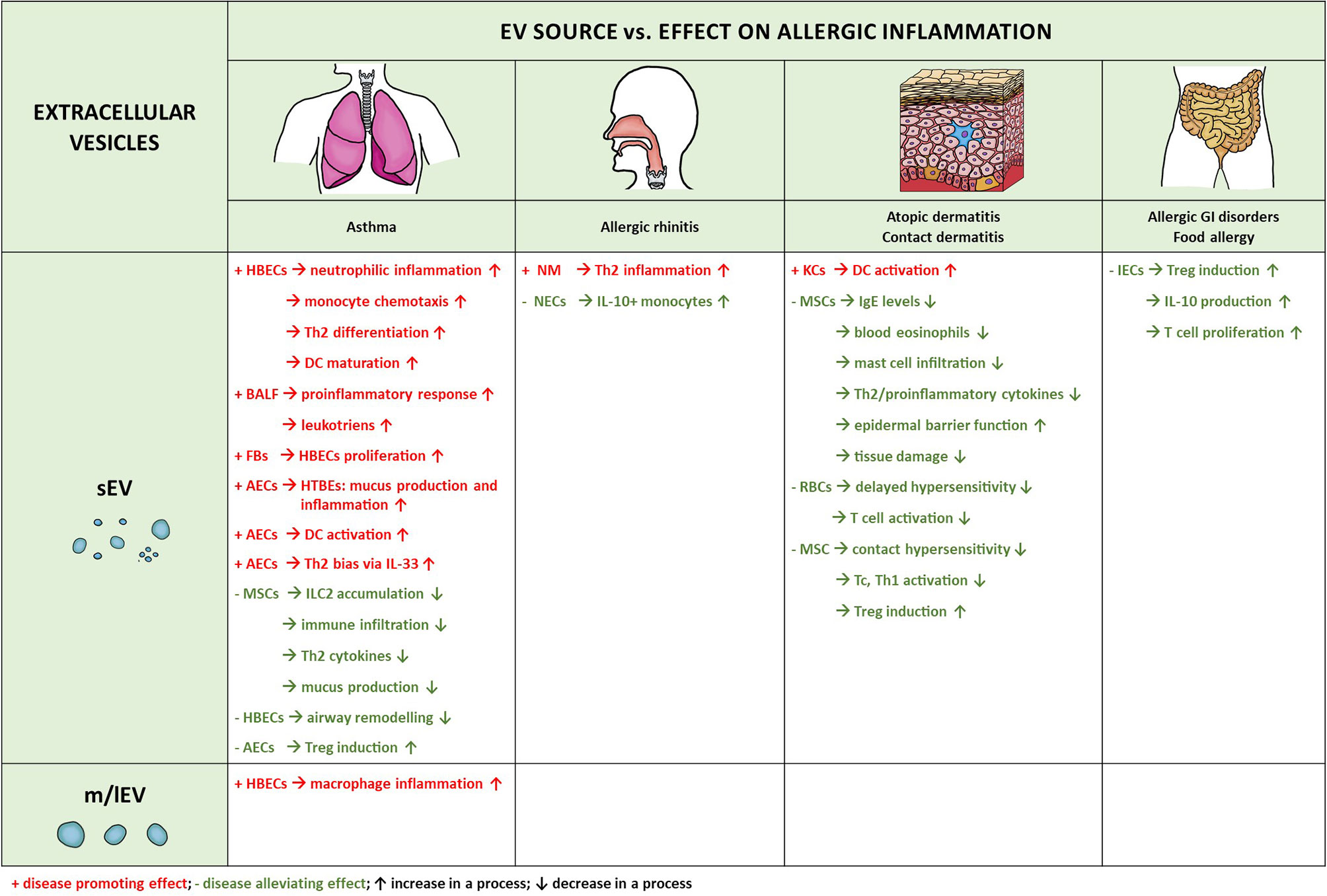
Figure 3 Extracellular vesicles produced by non-immune cells and their involvement in allergic diseases. Microvesicles and exosomes are the two types of extracellular vesicles which have been implicated in the pathogenesis of allergic inflammation. There is significant predominance of the exosomal involvement, likely due to the phenotypic characteristics and physical properties of these vesicles, enabling more without damage and entering the circulation for long-distance delivery. HBECs, human bronchial epithelial cells; BALF, bronchoalveolar lavage fluid; NM, nasal mucus; NECs, nasal epithelial cells; AECs, airway epithelial cells; HTBEs, human tracheobronchial cells; RBCs, red blood cells; IECs, intestinal epithelial cells; KCs, keratinocytes; FBs, fibroblasts; MSCs, mesenchymal stem cells. ↑ increase in a process; ↓ decrease in a process; + disease promoting effect; - disease alleviating effect.
Asthma
EVs contribute to the asthma pathogenesis via various mechanisms, related to both inflammation and pathological remodeling (177) and there are interesting interdependencies that can be observed. Specifically, it has been shown that fibroblasts-derived EVs secreted by cells obtained from severe asthmatics increase proliferation of bronchial epithelial cells (HBECs) in comparison to those in healthy individuals, due to a decrease in the TGF-β2 content (178). Vice versa, vesicular transfer between epithelial cells and fibroblasts which includes inositol polyphosphate 4-phosphatase type I A (INPP4A) cargo, may regulate inflammation and airway remodeling (179). Further to that, Gupta et al. have shown that sEV transfer between airway epithelial cells (AECs) and human tracheobronchial cells (HTBEs) promotes expression several proteins which may contribute to allergic inflammation and exacerbation of asthma symptoms, i.e. gel-forming mucins (180), complement component C3, SERPIN3. The addition of an allergen source (house dust mite; HDM) to the AEC culture resulted in DC activation by secreted sEVs in vitro and increased airway inflammation in a murine model (181); the role for contactin-1 has been demonstrated. Furthermore, it has been also demonstrated that sEVs may be a vehicle of secretion for an important Th2-promoting cytokine, interleukin 33 (IL-33); the cytokine seams to decorate the EV surface rather than be included within the intraluminal cargo (182). At the same time, however, it has been also shown that CD83/OVA-carrying sEVs derived from those cells may promote Treg differentiation (183). Ax et al. have documented that HBECs increase the number of EVs released upon treatment mimicking asthma milieu which may contribute to establishing of the neutrophilic airway inflammation associated with Th17-driven asthma (184). Kulshreshtha et al. have shown that IL-13-treated epithelial cells secrete sEVs which stimulate proliferation and chemotaxis of monocytes; suppressing secretion of those sEVs in the lungs alleviates asthmatic inflammation in a murine model of bronchial asthma (20). Similarly, Lee et al. have shown that HBEC-derived m/lEVs may promote macrophage-mediated inflammation upon hyperoxia-mediated lung injury via miR-221 and/or miR-320a (185). Moreover, three additional miRNAs, i.e. miR-92b, miR-34a and miR-210 found in the sEVs secreted by HBECs have been suggested to have possible roles in regulating Th2 differentiation and DCs maturation in asthma, indicating that airway epithelial miRNA secretion via sEVs might be even more implicated in the development of the disease (186). In agreement with this, bronchoalveolar lavage fluid (BALF)-derived EVs isolated from LPS-treated mice drive a mixed Th1/Th17 cell response and enhance production of the Th1/Th17-polarizing cytokines (IL-12p70 and IL-6) by lung DCs in an asthmatic mouse model but are more tolerogenic if the animals are devoid of the LPS stimulation (70). Specifically, Paredes et al. have shown that asthmatic BALF-derived sEVs which carry tetraspanins and MHC class II molecules might reflect increased levels of antigen-presenting capacity and suggest that these sEVs might contribute to the inflammation by increasing cytokine and leukotriene production in AECs (187). Asthmatic patients also have altered sEV proteomic characteristics and eicosanoid profile which is shown to exert pro-inflammatory functions in vitro. Specifically, Hough et al. have shown that BALF-derived EVs contain lipids, such as ceramides, sphingosines, prostaglandins and leukotrienes which have been previously identified to drive inflammation in asthma (188). In asthmatic conditions, BALF-derived EVs also exhibit particular miRNA profiles (189) and carry the biosynthetic machinery for the leukotriene biosynthesis pathway (187, 188). In agreement with this, in a human study, EVs isolated from the nasal secretions of children with asthma and chronic rhinitis promoted trafficking of primary monocytes, NK cells and neutrophils thanks to the changes in the exosomal proteome contributing to the alterations in the immune-related functions (147). These effects can be contrasted with a healthy lungs, as demonstrated in an animal model by Wan et al., who have shown that EVs isolated from the lungs of healthy mice contain immunosuppressive cytokines TGF-β1 and IL-10 which inhibit T helper cell proliferation and relieve asthmatic symptoms in mice (190). The immunomodulatory effect of EVs was also demonstrated by Prado et al. who have shown that intranasal administration of sEVs isolated from BALF of mice tolerized against major pollen allergen in the murine airway inflammation model (Ole e1) induces tolerance and protects naïve mice against allergic sensitization (191).
Finally, innate lymphoid cells type 2 (ILC2s) which are large contributors to the Th2-dominated allergic inflammation in the airways (192) can also be targeted by EV-mediated suppression. Specifically, systemic administration of MSC-derived sEVs resulted in the reduction in the ILC2 accumulation, inflammatory cell infiltration and mucus production in the lung, a reduction in the levels of Th2 cytokines, and alleviation of airway hyperresponsiveness in a mouse model of asthma. It seems that this sEV-mediated preventive effect was conveyed by the transfer of miR-146a-5p (193).
Allergic Rhinitis
Allergic rhinitis (AR) is a disease manifesting as type I allergic hypersensitivity within the nasal mucosa (194), and is characterized by chronic inflammation (195). The imbalance between the Th1 and Th2 differentiation is involved in the development of AR which is suggested to be partly regulated by sEVs. Zhu et al. have reported expression of a long-noncoding RNA (Lnc) GAS5 in the nasal mucus-derived sEVs in AR and in the ovalbumin-stimulated nasal epithelial cell (NEC)-derived sEVs. Here, this Lnc RNA promoted suppression of Th1 cell differentiation and induced Th2 differentiation upon treatment with nasal mucus (NM)-derived sEVs. A potential mechanism seems to involve the regulation of Enhancer of Zeste Homolog 2 (regulating proliferation and differentiation processes, including mediating proliferation and apoptosis of allogeneic T cells), and inhibition of T-bet expression by long-noncoding RNA GAS5 (196).
NEC-derived exosomal miR-146a induces the expression of IL-10 in monocytes in the murine model which seems to suppress allergic reactions downstream. Specifically, IL-10+ monocytes have an immune suppressor effect on the CD4+ effector T cells and the Th2 polarization in this model of AR (197). Interestingly, the alterations in the miRNA profile obtained from NM-derived EVs of AR patients showed intrinsic dysregulation of EV miRNA content in the disease. Wu et al. have demonstrated significant enrichment of certain biological and cellular processes within these differentially expressed miRNA signatures, namely B-cell receptor signaling pathway, natural killer cell-mediated cytotoxicity and T-cell receptor signaling, among others, implying that vesicular miRNAs exert regulatory function in AR. When investigated in more detail, B cell receptor signaling pathway-related miR-30-5p and miR-199b-3p were significantly increased, also miR-874 and miR-28-3p were significantly down-regulated in EVs from nasal mucus in AR (198).
Atopic Dermatitis and Contact Allergy
Atopic dermatitis (AD) is a chronic inflammatory skin disorder associated with the epidermal barrier disruption, eczematous cutaneous lesions and severe pruritus. AD pathogenesis is complex and characterized by cytokine production predominantly mediated by Th2 cells and ILC2 (199), but also involving innate and Th17 and Th22 components (200).
The importance of keratinocytes of the skin in the disease pathogenesis has been highlighted by the findings demonstrating that insufficiency in the epidermal barrier is key component (201). However, only one study so far has investigated the impact of EVs secreted by keratinocytes in the context of allergic inflammation (170). Here, using a murine allergy model, the authors noticed some signs of DC activation upon exposure to an antigen (OVA peptide) transferred by sEV from secreting keratinocytes. At the same time, however, they failed to detect any changes in the T cell reactivity to this peptide antigen.
Besides that, little is known about EV secretion from other cells in the skin with relation to AD, with more focus directed towards potential new therapies. In this regard, it has been reported that intravenous/subcutaneous administration of human adipose tissue-derived MSC-derived sEVs (showing exosomal characteristics) ameliorate AD symptoms in vivo (in a mouse model); the levels of serum IgE, the number of eosinophils in the blood, and the infiltration of mast cells were also shown to be reduced after the treatment. Such sEVs also reduced mRNA levels of IL-4, IL-31, IL-23, and TNF-α in the skin lesions demonstrating that their systemic administration may ameliorate AD-like symptoms through the regulation of inflammatory responses and expression of inflammatory cytokines in the tissue (25). Shin et al. have shown that exosomes-resembling sEVs derived from human adipose tissue-derived MSCs may significantly restore the epidermal barrier function in AD by inducing de novo synthesis of ceramides and modulating multiple gene expression programme, including the effects on differentiation of keratinocytes, lipid metabolism, cell cycle, and immune response (202). MSC-derived sEVs were shown to inhibit local inflammatory reaction and reduce tissue damage in atopic eczema (203). Hence, the evidence suggests that MSC-derived sEVs could potentially offer a promising cell-free therapeutic option for AD patients.
Contact allergy and contact sensitization is a common form of a delayed type hypersensitivity to small contact allergens. Contact allergy often develops after repeated or prolonged topical exposure to a particular sensitizing agent (204–206). Nazimek et al. have shown that intravenous administration of syngeneic mouse red blood cells leads to the EV generation that suppresses directed delayed type hypersensitivity in a miRNA-150-dependent manner; specifically, the syngeneic mouse red blood cell-derived EVs decreased T cell activation and enhanced their apoptosis (207). Similarly, human umbilical cord MSC-derived EVs were demonstrated to ameliorate and prevent the pathology of contact hypersensitivity in mice. Specifically, these EVs had a suppressive effect on both CD8+ cytotoxic cells and CD4+ Th1 cells, including the effect on TNF-α and IFN-γ production, induction of Tregs and the level of secreted IL-10 (208).
Food Allergy and Allergic Inflammation in the Gastrointestinal Tract
Food allergy is a manifestation of an abnormal immune response to food or food additives (209) which is a complex process involving multiple cellular and molecular mechanisms. It has been shown that early exposure to allergens via the gastrointestinal route promotes tolerance (210, 211). It is not clear how much EVs are involved in this process, however, animal models suggest that there could be some contribution. Specifically, intestinal epithelial cells (IECs) subjected to OVA release sEVs that carry IL-10 and OVA/MHC class II complexes recognized by OVA-specific TCR-bearing CD4+ T cells. Here, OVA-specific CD4+ T cells represent type 1 Tregs, produce IL-10 and show immune suppressive effects on effector T cell proliferation. The proposed mechanism involved the role of vasoactive intestinal peptides, which seemed to be required for this effect (212, 213). Furthermore, Treg bias has been also observed following a sEV-mediated transfer of food allergens into the mesenteric lymph nodes (MLNs) of mice, in contrast to a direct transfer of those allergens, which promoted Th2 responses (214); the results also highlighted the role of exosomal integrin αvβ6 as a protective molecule. Finally, given that the diverse composition of the gut microbiome has been shown to be critical in food allergy prevention (215), antigen and mediator transfer via EVs secreted by IECs may be also involved in the elimination of pathogenic bacteria to prevent intestinal dysbiosis (146).
Clinical Perspectives
Growing attention has been given to EVs as mediators in both physiological conditions and pathology, including the role in allergic diseases. Extensive research has been carried out showing the capacity of EVs to regulate homeostasis and immune functions in the allergic microenvironment. Alterations in exosomal content in allergic conditions have been shown to distinguish between physiological and diseased states suggesting the potential use of sEVs as biomarkers in the search of diagnostic tools for allergic diseases, for example in asthma phenotype subgrouping (216). Naturally-occurring sEVs can be also potentially used as drugs themselves, supporting healing process, e.g. MSC-derived sEVs participating in wound healing and regeneration of the lung tissue; this highlights the possible use of these sEVs in allergic airway remodeling (158, 202, 217). Several studies have proposed treatment strategies in animal models of allergic disease as summarized in Table 2. There are also examples of the use of sEVs as compound carriers are now being investigated as a naturally derived drug delivery systems (DDSs) with a favorable biocompatibility profile, but sEVs can be also potentially used to deliver non-drug anti-inflammatory agents including miRNAs (e.g. let-7-miRNAs). Indeed, there have been already several clinical trials in the past and more are now ongoing which investigate a potential of using EVs for the benefit of allergic patients (Table 3).
In summary, non-immune cell-derived EVs contribute to allergic inflammation in the tissue location and potentially systemically; they have a great potential to become a valuable diagnostic option as well as a novel target for allergy therapy. Such EVs are slowly introduced into the clinic within the setting of clinical trials which investigate the feasibility of such an approach.
Author Contributions
LH and DG-O wrote the paper. EC, DG-O, and LH prepared the figures. All authors contributed to the article and approved the submitted version.
Funding
This work was supported by POIR.04.04.00-00-21FA/16-00 project, carried out within the First TEAM programme of the Foundation for Polish Science co-financed by the European Union under the European Regional Development Fund and National Science Centre, Poland - UMO-2016/23/P/NZ6/04056, this project has received funding from the European Union’s Horizon 2020 research and innovation programme under the Marie Skłodowska-Curie grant agreement No. 665778, as a part of POLONEZ Fellowship.
Conflict of Interest
The authors declare that the research was conducted in the absence of any commercial or financial relationships that could be construed as a potential conflict of interest.
Publisher’s Note
All claims expressed in this article are solely those of the authors and do not necessarily represent those of their affiliated organizations, or those of the publisher, the editors and the reviewers. Any product that may be evaluated in this article, or claim that may be made by its manufacturer, is not guaranteed or endorsed by the publisher.
References
1. Mathieu M, Martin-Jaular L, Lavieu G, Théry C. Specificities of Secretion and Uptake of Exosomes and Other Extracellular Vesicles for Cell-to-Cell Communication. Nat Cell Biol (2019) 21:9–17. doi: 10.1038/s41556-018-0250-9
2. Rai A, Greening DW, Xu R, Chen M, Suwakulsiri W, Simpson RJ. Secreted Midbody Remnants Are a Class of Extracellular Vesicles Molecularly Distinct From Exosomes and Microparticles. Commun Biol (2021) 4:400. doi: 10.1038/s42003-021-01882-z
3. Iwai K, Minamisawa T, Suga K, Yajima Y, Shiba K. Isolation of Human Salivary Extracellular Vesicles by Iodixanol Density Gradient Ultracentrifugation and Their Characterizations. J Extracell Vesicles (2016) 5:1–17. doi: 10.3402/jev.v5.30829
4. Baranyai T, Herczeg K, Onódi Z, Voszka I, Módos K, Marton N, et al. Isolation of Exosomes From Blood Plasma: Qualitative and Quantitative Comparison of Ultracentrifugation and Size Exclusion Chromatography Methods. PLoS One (2015) 10:1–13. doi: 10.1371/journal.pone.0145686
5. Serrano-Pertierra E, Oliveira-Rodríguez M, Rivas M, Oliva P, Villafani J, Navarro A, et al. Characterization of Plasma-Derived Extracellular Vesicles Isolated by Different Methods: A Comparison Study. Bioengineering (2019) 6:1–13. doi: 10.3390/bioengineering6010008
6. Foster BP, Balassa T, Benen TD, Dominovic M, Elmadjian GK, Florova V, et al. Extracellular Vesicles in Blood, Milk and Body Fluids of the Female and Male Urogenital Tract and With Special Regard to Reproduction. Crit Rev Clin Lab Sci (2016) 53:379–95. doi: 10.1080/10408363.2016.1190682
7. Bryzgunova OE, Zaripov MM, Skvortsova TE, Lekchnov EA, Grigor’eva AE, Zaporozhchenko IA, et al. Comparative Study of Extracellular Vesicles From the Urine of Healthy Individuals and Prostate Cancer Patients. PLoS One (2016) 11:1–17. doi: 10.1371/journal.pone.0157566
8. Gregson AL, Hoji A, Injean P, Poynter ST, Briones C, Palchevskiy V, et al. Altered Exosomal RNA Profiles in Bronchoalveolar Lavage From Lung Transplants With Acute Rejection. Am J Respir Crit Care Med (2015) 192:1490–503. doi: 10.1164/rccm.201503-0558OC
9. Harms A, Fuehner T, Warnecke G, Haverich A, Gottlieb J, Trummer A. Epithelial and Erythrocyte Microvesicles From Bronchoalveolar Lavage Fluid Are Elevated and Associated With Outcome in Chronic Lung Allograft Dysfunction. Transplantation (2015) 99:2394–400. doi: 10.1097/TP.0000000000000881
10. Gamperl H, Plattfaut C, Freund A, Quecke T, Theophil F, Gieseler F. Extracellular Vesicles From Malignant Effusions Induce Tumor Cell Migration: Inhibitory Effect of LMWH Tinzaparin. Cell Biol Int (2016) 40:1050–61. doi: 10.1002/cbin.10645
11. Halin Bergström S, Hägglöf C, Thysell E, Bergh A, Wikström P, Lundholm M. Extracellular Vesicles From Metastatic Rat Prostate Tumors Prime the Normal Prostate Tissue to Facilitate Tumor Growth. Sci Rep (2016) 6:1–12. doi: 10.1038/srep31805
12. Aubertin K, Silva AKA, Luciani N, Espinosa A, Djemat A, Charue D, et al. Massive Release of Extracellular Vesicles From Cancer Cells After Photodynamic Treatment or Chemotherapy. Sci Rep (2016) 6:1–11. doi: 10.1038/srep35376
13. Huang-Doran I, Zhang CY, Vidal-Puig A. Extracellular Vesicles: Novel Mediators of Cell Communication In Metabolic Disease. Trends Endocrinol Metab (2017) 28:3–18. doi: 10.1016/j.tem.2016.10.003
14. Schneider DJ, Speth JM, Penke LR, Wettlaufer SH, Swanson JA, Peters-Golden M. Mechanisms and Modulation of Microvesicle Uptake in a Model of Alveolar Cell Communication. J Biol Chem (2017) 292:20897–910. doi: 10.1074/jbc.M117.792416
15. Ajikumar A, Long MB, Heath PR, Wharton SB, Ince PG, Ridger VC, et al. Neutrophil-Derived Microvesicle Induced Dysfunction of Brain Microvascular Endothelial Cells. vitro. Int J Mol Sci (2019) 20:1–17. doi: 10.3390/ijms20205227
16. Liu YP, Tong C, Dispenzieri A, Federspiel MJ, Russell SJ, Peng KW. Polyinosinic Acid Decreases Sequestration and Improves Systemic Therapy of Measles Virus. Cancer Gene Ther (2012) 19:202–11. doi: 10.1038/cgt.2011.82
17. van der Pol E, Böing AN, Harrison P, Sturk A, Nieuwland R. Classification, Functions, and Clinical Relevance of Extracellular Vesicles. Pharmacol Rev (2012) 64:676–705. doi: 10.1124/pr.112.005983
18. Rak J. Microparticles in Cancer. Semin Thromb Hemost (2010) 36:888–906. doi: 10.1055/s-0030-1267043
19. Kahlert C. Exosomes in Tumor Microenvironment Influence Cancer Progression and Metastasis. J Mol Med (2014) 91:431–7. doi: 10.1007/s00109-013-1020-6.Exosomes
20. Kulshreshtha A, Ahmad T, Agrawal A, Ghosh B. Proinflammatory Role of Epithelial Cell-Derived Exosomes in Allergic Airway Inflammation. J Allergy Clin Immunol (2013) 131:1194–203.e14. doi: 10.1016/j.jaci.2012.12.1565
21. Masaoutis C, Al Besher S, Koutroulis I, Theocharis S. Exosomes in Nephropathies: A Rich Source of Novel Biomarkers. Dis Markers (2020) 2020:8897833. doi: 10.1155/2020/8897833
22. Dear JW, Street JM, Bailey MA. Urinary Exosomes: A Reservoir for Biomarker Discovery and Potential Mediators of Intrarenal Signalling. Proteomics (2013) 13:1572–80. doi: 10.1002/pmic.201200285
23. Yang C, Robbins PD. Immunosuppressive Exosomes: A New Approach for Treating Arthritis. Int J Rheumatol (2012) 2012:573528. doi: 10.1155/2012/573528
24. Mortaz E, Alipoor SD, Varahram M, Jamaati H, Garssen J, Mumby SE, et al. Exosomes in Severe Asthma: Update in Their Roles and Potential in Therapy. BioMed Res Int (2018) 2018:2862187. doi: 10.1155/2018/2862187
25. Cho BS, Kim JO, Ha DH, Yi YW. Exosomes Derived From Human Adipose Tissue-Derived Mesenchymal Stem Cells Alleviate Atopic Dermatitis. Stem Cell Res Ther (2018) 9:1–5. doi: 10.1186/s13287-018-0939-5
26. King MA, Radicchi-Mastroianni MA. Effects of Caspase Inhibition on Camptothecin-Induced Apoptosis of HL-60 Cells. Cytometry (2002) 49(1):28–35. doi: 10.1002/cyto.10141
27. Mirochnik Y, Kwiatek A, Volpert O. Thrombospondin and Apoptosis: Molecular Mechanisms and Use for Design of Complementation Treatments. Curr Drug Targets (2008) 9:851–62. doi: 10.2174/138945008785909347
28. Baudino L, Sardini A, Ruseva MM, Fossati-Jimack L, Cook HT, Scott D, et al. C3 Opsonization Regulates Endocytic Handling of Apoptotic Cells Resulting in Enhanced T-Cell Responses to Cargo-Derived Antigens. Proc Natl Acad Sci U S A (2014) 111:1503–8. doi: 10.1073/pnas.1316877111
29. Shin S, Han D, Park MC, Mun JY, Choi J, Chun H, et al. Separation of Extracellular Nanovesicles and Apoptotic Bodies From Cancer Cell Culture Broth Using Tunable Microfluidic Systems. Sci Rep (2017) 7:1–8. doi: 10.1038/s41598-017-08826-w
30. Bergsmedh A, Szeles A, Henriksson M, Bratt A, Folkman MJ, Spetz AL, et al. Horizontal Transfer of Oncogenes by Uptake of Apoptotic Bodies. Proc Natl Acad Sci U S A (2001) 98:6407–11. doi: 10.1073/pnas.101129998
31. Hristov M, Erl W, Linder S, Weber PC. Apoptotic Bodies From Endothelial Cells Enhance the Number and Initiate the Differentiation of Human Endothelial Progenitor Cells In Vitro. Blood (2004) 104:2761–6. doi: 10.1182/blood-2003-10-3614
32. Crescitelli R, Lässer C, Szabó TG, Kittel A, Eldh M, Dianzani I, et al. Distinct RNA Profiles in Subpopulations of Extracellular Vesicles: Apoptotic Bodies, Microvesicles and Exosomes. J Extracell Vesicles (2013) 2. doi: 10.3402/jev.v2i0.20677
33. Battistelli M, Falcieri E. Apoptotic Bodies: Particular Extracellular Vesicles Involved in Intercellular Communication. Biol (Basel) (2020) 9(1):21. doi: 10.3390/biology9010021
34. Wahlund CJE, Güclüler G, Hiltbrunner S, Veerman RE, Näslund TI, Gabrielsson S. Exosomes From Antigen-Pulsed Dendritic Cells Induce Stronger Antigen-Specific Immune Responses Than Microvesicles In Vivo. Sci Rep (2017) 7:1–9. doi: 10.1038/s41598-017-16609-6
35. Dolo V, Adobati E, Canevari S, Picone MA, Vittorelli ML. Membrane Vesicles Shed Into the Extracellular Medium by Human Breast Carcinoma Cells Carry Tumor-Associated Surface Antigens. Clin Exp Metastasis (1995) 13:277–86. doi: 10.1007/BF00133483
36. Mobarrez F, Abraham-Nordling M, Aguilera-Gatica K, Friberg I, Antovic A, Pisetsky DS, et al. The Expression of Microvesicles in the Blood of Patients With Graves’ Disease and its Relationship to Treatment. Clin Endocrinol (Oxf) (2016) 84:729–35. doi: 10.1111/cen.12872
37. Del Conde I, Shrimpton CN, Thiagarajan P, López JA. Tissue-Factor-Bearing Microvesicles Arise From Lipid Rafts and Fuse With Activated Platelets to Initiate Coagulation. Blood (2005) 106:1604–11. doi: 10.1182/blood-2004-03-1095
38. Dolo V, D’Ascenzo S, Giusti I, Millimaggi D, Taraboletti G, Pavan A. Shedding of Membrane Vesicles by Tumor and Endothelial Cells, in: Italian Journal of Anatomy and Embryology (Ital J Anat Embryol) . (Accessed June 23, 2021).
39. Muralidharan-Chari V, Clancy J, Plou C, Romao M, Chavrier P, Raposo G, et al. ARF6-Regulated Shedding of Tumor Cell-Derived Plasma Membrane Microvesicles. Curr Biol (2009) 19:1875–85. doi: 10.1016/j.cub.2009.09.059
40. Zhang H, Yang F, Guo Y, Wang L, Fang F, Wu H, et al. The Contribution of Chronic Intermittent Hypoxia to OSAHS: From the Perspective of Serum Extracellular Microvesicle Proteins. Metabolism (2018) 85:97–108. doi: 10.1016/j.metabol.2018.02.012
41. Xu R, Greening DW, Rai A, Ji H, Simpson RJ. Highly-Purified Exosomes and Shed Microvesicles Isolated From the Human Colon Cancer Cell Line LIM1863 by Sequential Centrifugal Ultrafiltration Are Biochemically and Functionally Distinct. Methods (2015) 87:11–25. doi: 10.1016/j.ymeth.2015.04.008
42. Waldenström A, Gennebäck N, Hellman U, Ronquist G. Cardiomyocyte Microvesicles Contain DNA/RNA and Convey Biological Messages to Target Cells. PLoS One (2012) 7:e34653. doi: 10.1371/journal.pone.0034653
43. Hong BS, Cho JH, Kim H, Choi EJ, Rho S, Kim J, et al. Colorectal Cancer Cell-Derived Microvesicles Are Enriched in Cell Cycle-Related mRNAs That Promote Proliferation of Endothelial Cells. BMC Genomics (2009) 10:1–13. doi: 10.1186/1471-2164-10-556
44. Tricarico C, Clancy J, D’Souza-Schorey C. Biology and Biogenesis of Shed Microvesicles. Small GTPases (2017) 8:220–32. doi: 10.1080/21541248.2016.1215283
45. Al-Nedawi K, Meehan B, Micallef J, Lhotak V, May L, Guha A, et al. Intercellular Transfer of the Oncogenic Receptor EGFRvIII by Microvesicles Derived From Tumour Cells. Nat Cell Biol (2008) 10:619–24. doi: 10.1038/ncb1725
46. Hawari FI, Rouhani FN, Cui X, Yu ZX, Buckley C, Kaler M, et al. Release of Full-Length 55-kDa TNF Receptor 1 in Exosome-Like Vesicles: A Mechanism for Generation of Soluble Cytokine Receptors. Proc Natl Acad Sci U S A (2004) 101:1297–302. doi: 10.1073/pnas.0307981100
47. Baran J, Baj-Krzyworzeka M, Weglarczyk K, Szatanek R, Zembela M, Barbasz J, et al. Circulating Tumour-Derived Microvesicles in Plasma of Gastric Cancer Patients. Cancer Immunol Immunother (2010) 59:841–50. doi: 10.1007/s00262-009-0808-2
48. Mack M, Kleinschmidt A, Brühl H, Klier C, Nelson PJ, Cihak J, et al. Transfer of the Chemokine Receptor CCR5 Between Cells by Membrane- Derived Microparticles: A Mechanism for Cellular Human Immunodeficiency Virus 1 Infection. Nat Med (2000) 6:769–75. doi: 10.1038/77498
49. Singhto N, Vinaiphat A, Thongboonkerd V. Discrimination of Urinary Exosomes From Microvesicles by Lipidomics Using Thin Layer Liquid Chromatography (TLC) Coupled With MALDI-TOF Mass Spectrometry. Sci Rep (2019) 9:1–11. doi: 10.1038/s41598-019-50195-z
50. Gromley A, Yeaman C, Rosa J, Redick S, Chen C-T, Mirabelle S, et al. Centriolin Anchoring of Exocyst and SNARE Complexes at the Midbody Is Required for Secretory-Vesicle-Mediated Abscission. Cell (2005) 123:75–87. doi: 10.1016/j.cell.2005.07.027
51. Ettinger AW, Wilsch-Bräuninger M, Marzesco AM, Bickle M, Lohmann A, Maliga Z, et al. Proliferating Versus Differentiating Stem and Cancer Cells Exhibit Distinct Midbody-Release Behaviour. Nat Commun (2011) 2:503. doi: 10.1038/ncomms1511
52. Dubreuil V, Marzesco AM, Corbeil D, Huttner WB, Wilsch-Bräuninger M. Midbody and Primary Cilium of Neural Progenitors Release Extracellular Membrane Particles Enriched in the Stem Cell Marker Prominin-1. J Cell Biol (2007) 176(4):483–95. doi: 10.1083/jcb.200608137
53. Heijnen BHFG, Schiel AE, Fijnheer R, Geuze HJ, Sixma JJ. Activated Platelets Release Two Types of Membrane Vesicles Microvesicles by Surface Shedding and Exosomes Derived From Exocytosis of Multivesicular Bodies and Alpha-Granules. Blood (1999) 94(11):3791–9.
54. Guescini M, Genedani S, Stocchi V, Agnati LF. Astrocytes and Glioblastoma Cells Release Exosomes Carrying mtDNA. J Neural Transm (2010) 117:1–4. doi: 10.1007/s00702-009-0288-8
55. Valadi H, Ekström K, Bossios A, Sjöstrand M, Lee JJ, Lötvall JO. Exosome-Mediated Transfer of mRNAs and microRNAs Is a Novel Mechanism of Genetic Exchange Between Cells. Nat Cell Biol (2007) 9:654–9. doi: 10.1038/ncb1596
56. Al-Nedawi K, Meehan B, Kerbel RS, Allison AC, Rak A. Endothelial Expression of Autocrine VEGF Upon the Uptake of Tumor-Derived Microvesicles Containing Oncogenic EGFR. Proc Natl Acad Sci U S A (2009) 106:3794–9. doi: 10.1073/pnas.0804543106
57. Trajkovic K. Ceramide Triggers Budding of Exosome Vesicles Into Multivesicular Endosomes [Science (1244)]. Science (80-) (2008) 320:179. doi: 10.1126/science.320.5873.179
58. Lespagnol A, Duflaut D, Beekman C, Blanc L, Fiucci G, Marine J-C, et al. Exosome Secretion, Including the DNA Damage-Induced P53-Dependent Secretory Pathway, Is Severely Compromised in TSAP6/Steap3-Null Mice. Cell Death Differ (2008) 15:1723–33. doi: 10.1038/cdd.2008.104
59. Chairoungdua A, Smith DL, Pochard P, Hull M, Caplan MJ. Exosome Release of β-Catenin: A Novel Mechanism That Antagonizes Wnt Signaling. J Cell Biol (2010) 190:1079–91. doi: 10.1083/jcb.201002049
60. Gastpar R, Gehrmann M, Bausero MA, Asea A, Gross C, Schroeder JA, et al. Heat Shock Protein 70 Surface-Positive Tumor Exosomes Stimulate Migratory and Cytolytic Activity of Natural Killer Cells. Cancer Res (2005) 65:5238–47. doi: 10.1158/0008-5472.CAN-04-3804
61. Keller S, Rupp C, Stoeck A, Runz S, Fogel M, Lugert S, et al. CD24 Is a Marker of Exosomes Secreted Into Urine and Amniotic Fluid. Kidney Int (2007) 72:1095–102. doi: 10.1038/sj.ki.5002486
62. Mattera VS, Pereyra Gerber P, Glisoni R, Ostrowski M, Verstraeten SV, Pasquini JM, et al. Extracellular Vesicles Containing the Transferrin Receptor as Nanocarriers of Apotransferrin. J Neurochem (2020) 155:327–38. doi: 10.1111/jnc.15019
63. Zhang J, Hawari FI, Shamburek RD, Adamik B, Kaler M, Islam A, et al. Circulating TNFR1 Exosome-Like Vesicles Partition With the LDL Fraction of Human Plasma. Biochem Biophys Res Commun (2008) 366:579–84. doi: 10.1016/j.bbrc.2007.12.011
64. Shen B, Wu N, Yang M, Gould SJ. Protein Targeting to Exosomes/Microvesicles by Plasma Membrane Anchors. J Biol Chem (2011) 286:14383–95. doi: 10.1074/jbc.M110.208660
65. Jansen FH, Krijgsveld J, Van Rijswijk A, Van Den Bemd GJ, Van Den Berg MS, Van Weerden WM, et al. Exosomal Secretion of Cytoplasmic Prostate Cancer Xenograft-Derived Proteins. Mol Cell Proteomics (2009) 8:1192–205. doi: 10.1074/mcp.M800443-MCP200
66. Rana S, Yue S, Stadel D, Zöller M. Toward Tailored Exosomes: The Exosomal Tetraspanin Web Contributes to Target Cell Selection. Int J Biochem Cell Biol (2012) 44:1574–84. doi: 10.1016/j.biocel.2012.06.018
67. Kahlert C, Melo SA, Protopopov A, Tang J, Seth S, Koch O, et al. Identification of Doublestranded Genomic Dna Spanning All Chromosomes With Mutated KRAS and P53 DNA in the Serum Exosomes of Patients With Pancreatic Cancer. J Biol Chem (2014) 289:3869–75. doi: 10.1074/jbc.C113.532267
68. Cazzoli R, Buttitta F, Di Nicola M, Malatesta S, Marchetti A, Rom WN, et al. MicroRNAs Derived From Circulating Exosomes as Noninvasive Biomarkers for Screening and Diagnosing Lung Cancer. J Thorac Oncol (2013) 8:1156–62. doi: 10.1097/JTO.0b013e318299ac32
69. Zhou X, Zhu W, Li H, Wen W, Cheng W, Wang F, et al. Diagnostic Value of a Plasma microRNA Signature in Gastric Cancer: A microRNA Expression Analysis. Sci Rep (2015) 5:1–13. doi: 10.1038/srep11251
70. Haraszti RA, Didiot M-C, Sapp E, Leszyk J, Shaffer SA, Rockwell HE, et al. Journal of Extracellular Vesicles High-Resolution Proteomic and Lipidomic Analysis of Exosomes and Microvesicles From Different Cell Sources) High-Resolution Proteomic and Lipidomic Analysis of Exosomes and Microvesicles From Different Cell Sources. J Extracell Vesicles (2016) 5:32570. doi: 10.3402/jev.v5.32570
71. Mallegol J, Van Niel G, Lebreton C, Lepelletier Y, Candalh C, Dugave C, et al. T84-Intestinal Epithelial Exosomes Bear MHC Class II/Peptide Complexes Potentiating Antigen Presentation by Dendritic Cells. Gastroenterology (2007) 132:1866–76. doi: 10.1053/j.gastro.2007.02.043
72. Admyre C, Grunewald J, Thyberg J, Bripenäck S, Tornling G, Eklund A, et al. Exosomes With Major Histocompatibility Complex Class II and Co-Stimulatory Molecules Are Present in Human BAL Fluid. Eur Respir J (2003) 22:578–83. doi: 10.1183/09031936.03.00041703
73. Hoshino A, costa-Silva b, Shen T-L, rodrigues G, Hashimoto A, Tesic mm, et al. Tumour Exosome Integrins Determine Organotropic Metastasis. Nature (2015) 527(7578):329–35. doi: 10.1038/nature15756
74. Hosseini-Beheshti E, Pham S, Adomat H, Li N, Tomlinson Guns ES. Exosomes as Biomarker Enriched Microvesicles: Characterization of Exosomal Proteins Derived From a Panel of Prostate Cell Lines With Distinct AR Phenotypes. Mol Cell Proteomics (2012) 11:863–85. doi: 10.1074/mcp.M111.014845
75. Zhang Y, Liu Y, Liu H, Tang WH. Exosomes: Biogenesis, Biologic Function and Clinical Potential. Cell Biosci (2019) 9:1–18. doi: 10.1186/s13578-019-0282-2
76. Desrochers LM, Bordeleau F, Reinhart-King CA, Cerione RA, Antonyak MA. Microvesicles Provide a Mechanism for Intercellular Communication by Embryonic Stem Cells During Embryo Implantation. Nat Commun (2016) 7:11958. doi: 10.1038/ncomms11958
77. Kawamura Y, Yamamoto Y, Sato TA, Ochiya T. Extracellular Vesicles as Trans-Genomic Agents: Emerging Roles in Disease and Evolution. Cancer Sci (2017) 108:824–30. doi: 10.1111/cas.13222
78. Paolicelli RC, Bergamini G, Rajendran L. Cell-To-Cell Communication by Extracellular Vesicles: Focus on Microglia. Neuroscience (2019) 405:148–57. doi: 10.1016/j.neuroscience.2018.04.003
79. Maia J, Caja S, Strano Moraes MC, Couto N, Costa-Silva B. Exosome-Based Cell-Cell Communication in the Tumor Microenvironment. Front Cell Dev Biol (2018) 6:18. doi: 10.3389/fcell.2018.00018
80. Lässer C, Jang SC, Lötvall J. Subpopulations of Extracellular Vesicles and Their Therapeutic Potential. Mol Aspects Med (2018) 60:1–14. doi: 10.1016/j.mam.2018.02.002
81. Pegtel DM, Cosmopoulos K, Thorley-Lawson DA, Van Eijndhoven MAJ, Hopmans ES, Lindenberg JL, et al. Functional Delivery of Viral miRNAs via Exosomes. Proc Natl Acad Sci U.S.A. (2010) 107:6328–33. doi: 10.1073/pnas.0914843107
82. Mathivanan S, Ji H, Simpson RJ. Exosomes: Extracellular Organelles Important in Intercellular Communication. J Proteomics (2010) 73:1907–20. doi: 10.1016/j.jprot.2010.06.006
84. Raposo G, Stoorvogel W. Extracellular Vesicles: Exosomes, Microvesicles, and Friends. J Cell Biol (2013) 200:373–83. doi: 10.1083/jcb.201211138
85. Colombo M, Raposo G, Théry C. Biogenesis, Secretion, and Intercellular Interactions of Exosomes and Other Extracellular Vesicles. Annu Rev Cell Dev Biol (2014) 30:255–89. doi: 10.1146/annurev-cellbio-101512-122326
86. Raiborg C, Stenmark H. The ESCRT Machinery in Endosomal Sorting of Ubiquitylated Membrane Proteins. Nature (2009) 458:445–52. doi: 10.1038/nature07961
87. Stahl PD, Raposo G. Exosomes and Extracellular Vesicles: The Path Forward. Essays Biochem (2018) 62:119–24. doi: 10.1042/EBC20170088
88. Hurley JH, Hanson PI. Membrane Budding and Scission by ESCRT Machinery: It's all in the neck. Nat Rev Mol Cell Biol (2011) 11:556–66. doi: 10.1038/nrm2937
89. Souza-Schorey C, Schorey JS. Regulation and Mechanisms of Extracellular Vesicle Biogenesis and Secretion. Essays Biochem (2018) 62:125–33. doi: 10.1042/EBC20170078
90. Henne WM, Stenmark H, Emr SD. Molecular Mechanisms of the Membrane Sculpting ESCRT Pathway. Cold Spring Harb Perspect Biol (2013) 5:a016766. doi: 10.1101/cshperspect.a016766
91. Babst M, Wendland B, Estepa EJ, Emr SD. The Vps4p AAA ATPase Regulates Membrane Association of a Vps Protein Complex Required for Normal Endosome Function. EMBO J (1998) 17:2982–93. doi: 10.1093/emboj/17.11.2982
92. Hasegawa T, Konno M, Baba T, Sugeno N, Kikuchi A, Kobayashi M, et al. The AAA-ATPase VPS4 Regulates Extracellular Secretion and Lysosomal Targeting of α-Synuclein. PLoS One (2011) 6:29460. doi: 10.1371/journal.pone.0029460
93. Gill DJ, Teo H, Sun J, Perisic O, Veprintsev DB, Vallis Y, et al. Structural Studies of Phosphoinositide 3-Kinasedependent Traffic to Multivesicular Bodies. Biochem Soc Symp (2007) 74:47–57. doi: 10.1042/BSS0740047
94. Hurley JH, Emr SD. The ESCRT Complexes: Structure and Mechanism of a Membrane-Trafficking Network. Annu Rev Biophys Biomol Struct (2006) 35:277–98. doi: 10.1146/annurev.biophys.35.040405.102126
95. Larios J, Mercier V, Roux A, Gruenberg J. ALIX- And ESCRT-III-Dependent Sorting of Tetraspanins to Exosomes. J Cell Biol (2020) 219(3):e201904113. doi: 10.1083/jcb.201904113
96. McNally EK, Brett CL. The Intralumenal Fragment Pathway Mediates ESCRT-Independent Surface Transporter Down-Regulation. Nat Commun (2018) 9:5358. doi: 10.1038/s41467-018-07734-5
97. Kajimoto T, Okada T, Miya S, Zhang L, Nakamura SI. Ongoing Activation of Sphingosine 1-Phosphate Receptors Mediates Maturation of Exosomal Multivesicular Endosomes. Nat Commun (2013) 4:2712. doi: 10.1038/ncomms3712
98. Verderio C, Gabrielli M, Giussani P. Role of Sphingolipids in the Biogenesis and Biological Activity of Extracellular Vesicles. J Lipid Res (2018) 59:1325–40. doi: 10.1194/jlr.R083915
99. Raposo G, Nijman HW, Stoorvogel W, Leijendekker R, Harding CV, Melief CJM, et al. B Lymphocytes Secrete Antigen-Presenting Vesicles. J Exp Med (1996) 183:1161–72. doi: 10.1084/jem.183.3.1161
100. Davies BA, Lee JRE, Oestreich AJ, Katzmann DJ. Membrane Protein Targeting to the MVB/Lysosome. Chem Rev (2009) 109:1575–86. doi: 10.1021/cr800473s
101. Manfredi F, Bonito P, Arenaccio C, Anticoli S, Federico M. Lentiviral Vectors and Exosomes as Gene and Protein Delivery Tools. (2016) 1448:249–60. doi: 10.1007/978-1-4939-3753-0
102. van Niel G, Charrin S, Simoes S, Romao M, Rochin L, Saftig P, et al. The Tetraspanin CD63 Regulates ESCRT-Independent and -Dependent Endosomal Sorting During Melanogenesis. Dev Cell (2011) 21:708–21. doi: 10.1016/j.devcel.2011.08.019
103. Pols MS, Klumperman J. Trafficking and Function of the Tetraspanin CD63. Exp Cell Res (2009) 315:1584–92. doi: 10.1016/j.yexcr.2008.09.020
104. Edgar JR, Eden ER, Futter CE. Hrs- and CD63-Dependent Competing Mechanisms Make Different Sized Endosomal Intraluminal Vesicles. Traffic (2014) 15:197–211. doi: 10.1111/tra.12139
105. Cocucci E, Racchetti G, Meldolesi J. Shedding Microvesicles: Artefacts No More. Trends Cell Biol (2009) 19:43–51. doi: 10.1016/j.tcb.2008.11.003
106. Abels ER, Breakefield XO. Introduction to Extracellular Vesicles: Biogenesis, RNA Cargo Selection, Content, Release, and Uptake. Cell Mol Neurobiol (2016) 36:301–12. doi: 10.1007/s10571-016-0366-z
107. Zha QB, Yao YF, Ren ZJ, Li XJ, Tang JH. Extracellular Vesicles: An Overview of Biogenesis, Function, and Role in Breast Cancer. Tumor Biol (2017) 39(2):1010428317691182. doi: 10.1177/1010428317691182
108. Kuo L, Freed EO. ARRDC1 as a Mediator of Microvesicle Budding. Proc Natl Acad Sci U S A (2012) 109:4025–6. doi: 10.1073/pnas.1201441109
109. Nabhan JF, Hu R, Oh RS, Cohen SN, Lu Q. Formation and Release of Arrestin Domain-Containing Protein 1-Mediated Microvesicles (ARMMs) at Plasma Membrane by Recruitment of TSG101 Protein. Proc Natl Acad Sci U S A (2012) 109:4146–51. doi: 10.1073/pnas.1200448109
110. Stahl PD, Raposo G. Extracellular Vesicles: Exosomes and Microvesicles, Integrators of Homeostasis. Physiol (Bethesda) (2019) 34:169–77. doi: 10.1152/physiol.00045.2018
111. Al-Nedawi K, Meehan B, Rak J. Microvesicles: Messengers and Mediators of Tumor Progression. Cell Cycle (2009) 8:2014–8. doi: 10.4161/cc.8.13.8988
112. Lösche W, Scholz T, Temmler U, Oberle V, Claus RA. Platelet-Derived Microvesicles Transfer Tissue Factor to Monocytes But Not to Neutrophils. Platelets (2004) 15:109–15. doi: 10.1080/09537100310001649885
113. Turola E, Furlan R, Bianco F, Matteoli M, Verderio C. Microglial Microvesicle Secretion and Intercellular Signaling. Front Physiol (2012) 3:149. doi: 10.3389/fphys.2012.00149
114. Kerr JFR, Harmon B, Searle J. An Electron Microscope Study of Cell Deletion in the Anuran Tadpole Tail During Spontaneous Metamorphosis With Special Reference to Apoptosis of Striated Muscle Fibres. J Cell Sci (1974) 14:571–85.
115. Elmore S. Apoptosis: A Review of Programmed Cell Death. Toxicol Pathol (2007) 35:495–516. doi: 10.1080/01926230701320337
116. Moss DK, Betin VM, Malesinski SD, Lane JD. A Novel Role for Microtubules in Apoptotic Chromatin Dynamics and Cellular Fragmentation. J Cell Sci (2006) 119:2362–74. doi: 10.1242/jcs.02959
117. Caruso S, Poon IKH. Apoptotic Cell-Derived Extracellular Vesicles: More Than Just Debris. Front Immunol (2018) 28(9):1486. doi: 10.3389/fimmu.2018.01486
118. Samos J, García-Olmo DC, Picazo MG, Rubio-Vitaller A, García-Olmo D. Circulating Nucleic Acids in Plasma/Serum and Tumor Progression: Are Apoptotic Bodies Involved? An Experimental Study in a Rat Cancer Model. Ann N Y Acad Sci (2006) 1075:165–73. doi: 10.1196/annals.1368.022
119. Eppensteiner J, Davis RP, Barbas AS, Kwun J, Lee J. Immunothrombotic Activity of Damage-Associated Molecular Patterns and Extracellular Vesicles in Secondary Organ Failure Induced by Trauma and Sterile Insults. Front Immunol (2018) 9:190. doi: 10.3389/fimmu.2018.00190
120. Zhang Q, Raoof M, Chen Y, Sumi Y, Sursal T, Junger W, et al. Circulating Mitochondrial DAMPs Cause Inflammatory Responses to Injury. Nature (2010) 464:104–7. doi: 10.1038/nature08780
121. Scaffidi P, Misteli T, Bianchi ME. Release of Chromatin Protein HMGB1 by Necrotic Cells Triggers Inflammation. Nature (2002) 418:191–5. doi: 10.1038/nature00858
122. Westman J, Papareddy P, Dahlgren MW, Chakrakodi B, Norrby-Teglund A, Smeds E, et al. Extracellular Histones Induce Chemokine Production in Whole Blood Ex Vivo and Leukocyte Recruitment In Vivo. PLoS Pathog (2015) 11(12):e1005319. doi: 10.1371/journal.ppat.1005319
123. Hofmann MA, Drury S, Fu C, Qu W, Taguchi A, Lu Y, et al. RAGE Mediates a Novel Proinflammatory Axis: A Central Cell Surface Receptor for S100/calgranulin Polypeptides. Cell (1999) 97:889–901. doi: 10.1016/S0092-8674(00)80801-6
124. Quintana FJ, Cohen IR. Heat Shock Proteins as Endogenous Adjuvants in Sterile and Septic Inflammation. J Immunol (2005) 175:2777–82. doi: 10.4049/jimmunol.175.5.2777
125. Bours MJL, Swennen ELR, Di Virgilio F, Cronstein BN, Dagnelie PC. Adenosine 5′-Triphosphate and Adenosine as Endogenous Signaling Molecules in Immunity and Inflammation. Pharmacol Ther (2006) 112:358–404. doi: 10.1016/j.pharmthera.2005.04.013
126. Kono H, Chen CJ, Ontiveros F, Rock KL. Uric Acid Promotes an Acute Inflammatory Response to Sterile Cell Death in Mice. J Clin Invest (2010) 120:1939–49. doi: 10.1172/JCI40124
127. Sangiuliano B, Pérez NM, Moreira DF, Belizário JE. Cell Death-Associated Molecular-Pattern Molecules: Inflammatory Signaling and Control. Mediators Inflammation (2014) 2014:821043. doi: 10.1155/2014/821043
128. Krysko DV, Agostinis P, Krysko O, Garg AD, Bachert C, Lambrecht BN, et al. Emerging Role of Damage-Associated Molecular Patterns Derived From Mitochondria in Inflammation. Trends Immunol (2011) 32:157–64. doi: 10.1016/j.it.2011.01.005
129. Roh JS, Sohn DH. Origin and List of Damps. Immune Netw (2018) 18:1–14. doi: 10.4110/in.2018.18.e27
130. Westman J, Grinstein S, Marques PE. Phagocytosis of Necrotic Debris at Sites of Injury and Inflammation. Front Immunol (2020) 10:3030. doi: 10.3389/fimmu.2019.03030
131. Erwig LP, Henson PM. Clearance of Apoptotic Cells by Phagocytes. Cell Death Differ (2008) 15:243–50. doi: 10.1038/sj.cdd.4402184
132. Goss JW, Toomre DK. Both Daughter Cells Traffic and Exocytose Membrane at the Cleavage Furrow During Mammalian Cytokinesis. J Cell Biol (2008) 181:1047–54. doi: 10.1083/jcb.200712137
133. Pohl C, Jentsch S. Midbody Ring Disposal by Autophagy Is a Post-Abscission Event of Cytokinesis. Nat Cell Biol (2009) 11:65–70. doi: 10.1038/ncb1813
134. Zhang H, Freitas D, Kim HS, Fabijanic K, Li Z, Chen H, et al. Identification of Distinct Nanoparticles and Subsets of Extracellular Vesicles by Asymmetric Flow Field-Flow Fractionation. Nat Cell Biol (2018) 20:332–43. doi: 10.1038/s41556-018-0040-4
135. Zhang Q, Higginbotham JN, Jeppesen DK, Yang YP, Li W, McKinley ET, et al. Transfer of Functional Cargo in Exomeres. Cell Rep (2019) 27:940–54. doi: 10.1016/j.celrep.2019.01.009
136. Anand S, Samuel M, Mathivanan S. Exomeres: A New Member of Extracellular Vesicles Family. Subcellular Biochemistry (2021) 97:89–97. doi: 10.1007/978-3-030-67171-6_5
137. Théry C, Witwer KW, Aikawa E, Alcaraz MJ, Anderson JD, Andriantsitohaina R, et al. Minimal Information for Studies of Extracellular Vesicles 2018 (MISEV2018): A Position Statement of the International Society for Extracellular Vesicles and Update of the MISEV2014 Guidelines. J Extracell Vesicles (2018) 7(1):1535750. doi: 10.1080/20013078.2018.1535750
138. Théry C, Ostrowski M, Segura E. Membrane Vesicles as Conveyors of Immune Responses. Nat Rev Immunol (2009) 9:581–93. doi: 10.1038/nri2567
139. Huang F, Jia H, Zou Y, Yao Y, Deng Z. Exosomes: An Important Messenger in the Asthma Inflammatory Microenvironment. J Int Med Res (2020) 48:30006052090322. doi: 10.1177/0300060520903220
140. Demkow U, Stelmaszczyk-Emmel A. Extracellular Vesicles in Allergic Rhinitis and Asthma and Laboratory Possibilities for Their Assessment. Int J Mol Sci (2021) 22:1–13. doi: 10.3390/ijms22052273
141. Paul D. Robbins and Adrian E. Morelli. Regulation of Immune Responses by Extracellular Vesicles. Nat Rev Immunol (2014) 14:195–208. doi: 10.1038/nri3622
142. Kalluri R, LeBleu VS. The Biology, Function, and Biomedical Applications of Exosomes. Sci (80-) (2020) 367(6478):eaau6977. doi: 10.1126/science.aau6977
143. Zhou X, Xie F, Wang L, Zhang L, Zhang S, Fang M, et al. The Function and Clinical Application of Extracellular Vesicles in Innate Immune Regulation. Cell Mol Immunol (2020) 17(4):323–34. doi: 10.1038/s41423-020-0391-1
144. Hough KP, Deshane JS. Exosomes in Allergic Airway Diseases. Curr Allergy Asthma Rep (2019) 19:1–8. doi: 10.1007/s11882-019-0857-3
145. Prescott SL. Allergy Takes its Toll: The Role of Toll-Like Receptors in Allergy Pathogenesis. World Allergy Organ J (2008) 1:4–8. doi: 10.1097/wox.0b013e3181625d9f
146. Hu G, Gong AY, Roth AL, Huang BQ, Ward HD, Zhu G, et al. Release of Luminal Exosomes Contributes to TLR4-Mediated Epithelial Antimicrobial Defense. PloS Pathog (2013) 9(4):e1003261. doi: 10.1371/journal.ppat.1003261
147. Lässer C, O’Neil SE, Shelke GV, Sihlbom C, Hansson SF, Gho YS, et al. Exosomes in the Nose Induce Immune Cell Trafficking and Harbour an Altered Protein Cargo in Chronic Airway Inflammation. J Transl Med (2016) 14:1–14. doi: 10.1186/s12967-016-0927-4
148. Nocera AL, Mueller SK, Stephan JR, Hing L, Seifert P, Han X, et al. Exosome Swarms Eliminate Airway Pathogens and Provide Passive Epithelial Immunoprotection Through Nitric Oxide. J Allergy Clin Immunol (2019) 143:1525–35.e1. doi: 10.1016/j.jaci.2018.08.046
149. Pablo De Rivero Vaccari J, Iii FB, Adamczak S, Lee SW, Perez Barcena J, Wang MY, et al. Exosome-Mediated Inflammasome Signaling After Central Nervous System Injury Hhs Public Access. J Neurochem (2016) 136:39–48. doi: 10.1111/jnc.13036
150. Li Y, Zhu X, Zhang M, Tong H, Su L. Heatstroke-Induced Hepatocyte Exosomes Promote Liver Injury by Activating the NOD-Like Receptor Signaling Pathway in Mice. PeerJ (2019) 2019:e8216. doi: 10.7717/peerj.8216
151. Bronster DJ, Emre S, Boccagni P, Sheiner PA, Schwartz ME, Miller CM. Central Nervous System Complications in Liver Transplant Recipients - Incidence, Timing, and Long-Term Follow-Up. Clin Transplant (2000) 14:1–7. doi: 10.1034/j.1399-0012.2000.140101.x
152. Zhang L, Liu H, Jia L, Lyu J, Sun Y, Yu H, et al. Exosomes Mediate Hippocampal and Cortical Neuronal Injury Induced by Hepatic Ischemia-Reperfusion Injury Through Activating Pyroptosis in Rats. Oxid Med Cell Longev (2019) 2019:3753485. doi: 10.1155/2019/3753485
153. Mills JT, Schwenzer A, Marsh EK, Edwards MR, Sabroe I, Midwood KS, et al. Airway Epithelial Cells Generate Pro-Inflammatory Tenascin-C and Small Extracellular Vesicles in Response to TLR3 Stimuli and Rhinovirus Infection. Front Immunol (2019) 10:1987. doi: 10.3389/fimmu.2019.01987
154. Hu B, Chen S, Zou M, He Z, Shao S, Liu B. Effect of Extracellular Vesicles on Neural Functional Recovery and Immunologic Suppression After Rat Cerebral Apoplexy. Cell Physiol Biochem (2016) 40:155–62. doi: 10.1159/000452533
155. Drommelschmidt K, Serdar M, Bendix I, Herz J, Bertling F, Prager S, et al. Mesenchymal Stem Cell-Derived Extracellular Vesicles Ameliorate Inflammation-Induced Preterm Brain Injury. Brain Behav Immun (2017) 60:220–32. doi: 10.1016/j.bbi.2016.11.011
156. Ruppert KA, Nguyen TT, Prabhakara KS, Toledano Furman NE, Srivastava AK, Harting MT, et al. Human Mesenchymal Stromal Cell-Derived Extracellular Vesicles Modify Microglial Response and Improve Clinical Outcomes in Experimental Spinal Cord Injury. Sci Rep (2018) 8:1–12. doi: 10.1038/s41598-017-18867-w
157. Farinazzo A, Angiari S, Turano E, Bistaffa E, Dusi S, Ruggieri S, et al. Nanovesicles From Adipose-Derived Mesenchymal Stem Cells Inhibit T Lymphocyte Trafficking and Ameliorate Chronic Experimental Autoimmune Encephalomyelitis. Sci Rep (2018) 8:1–11. doi: 10.1038/s41598-018-25676-2
158. Cruz FF, Borg ZD, Goodwin M, Sokocevic D, Wagner DE, Coffey A, et al. Systemic Administration of Human Bone Marrow-Derived Mesenchymal Stromal Cell Extracellular Vesicles Ameliorates Aspergillus Hyphal Extract-Induced Allergic Airway Inflammation in Immunocompetent Mice. Stem Cells Transl Med (2015) 4:1302–16. doi: 10.5966/sctm.2014-0280
159. Burrello J, Monticone S, Gai C, Gomez Y, Kholia S, Camussi G. Stem Cell-Derived Extracellular Vesicles and Immune-Modulation. Front Cell Dev Biol (2016) 4:83. doi: 10.3389/fcell.2016.00083
160. Kordelas L, Rebmann V, Ludwig AK, Radtke S, Ruesing J, Doeppner TR, et al. MSC-Derived Exosomes: A Novel Tool to Treat Therapy-Refractory Graft-Versus-Host Disease. Leukemia (2014) 28:970–3. doi: 10.1038/leu.2014.41
161. Fujii S, Miura Y, Fujishiro A, Shindo T, Shimazu Y, Hirai H, et al. Graft-Versus-Host Disease Amelioration by Human Bone Marrow Mesenchymal Stromal/Stem Cell-Derived Extracellular Vesicles Is Associated With Peripheral Preservation of Naive T Cell Populations. Stem Cells (2018) 36:434–45. doi: 10.1002/stem.2759
162. Hosseini Shamili F, Alibolandi M, Rafatpanah H, Abnous K, Mahmoudi M, Kalantari M, et al. Immunomodulatory Properties of MSC-Derived Exosomes Armed With High Affinity Aptamer Toward Mylein as a Platform for Reducing Multiple Sclerosis Clinical Score. J Control Release (2019) 299:149–64. doi: 10.1016/j.jconrel.2019.02.032
163. Zhang Q, Fu L, Liang Y, Guo Z, Wang L, Ma C, et al. Exosomes Originating From MSCs Stimulated With TGF-β and IFN-γ Promote Treg Differentiation. J Cell Physiol (2018) 233:6832–40. doi: 10.1002/jcp.26436
164. Gomzikova MO, James V, Rizvanov AA. Therapeutic Application of Mesenchymal Stem Cells Derived Extracellular Vesicles for Immunomodulation. Front Immunol (2019) 10:2663. doi: 10.3389/fimmu.2019.02663
165. Reis M, Mavin E, Nicholson L, Green K, Dickinson AM, Wang X. Mesenchymal Stromal Cell-Derived Extracellular Vesicles Attenuate Dendritic Cell Maturation and Function. Front Immunol (2018) 9:2538. doi: 10.3389/fimmu.2018.02538
166. Hyvärinen K, Holopainen M, Skirdenko V, Ruhanen H, Lehenkari P, Korhonen M, et al. Mesenchymal Stromal Cells and Their Extracellular Vesicles Enhance the Anti-Inflammatory Phenotype of Regulatory Macrophages by Downregulating the Production of Interleukin (IL)-23 and IL-22. Front Immunol (2018) 9:771. doi: 10.3389/fimmu.2018.00771
167. Mokarizadeh A, Delirezh N, Morshedi A, Mosayebi G, Farshid AA, Mardani K. Microvesicles Derived From Mesenchymal Stem Cells: Potent Organelles for Induction of Tolerogenic Signaling. Immunol Lett (2012) 147:47–54. doi: 10.1016/j.imlet.2012.06.001
168. van den Akker F, Vrijsen KR, Deddens JC, Buikema JW, Mokry M, van Laake LW, et al. Suppression of T Cells by Mesenchymal and Cardiac Progenitor Cells Is Partly Mediated. via extracellular vesicles. Heliyon (2018) 4:e00642. doi: 10.1016/j.heliyon.2018.e00642
169. Shigemoto-Kuroda T, Oh JY, Kim D, Jeong HJ, Park SY, Lee HJ, et al. MSC-Derived Extracellular Vesicles Attenuate Immune Responses in Two Autoimmune Murine Models: Type 1 Diabetes and Uveoretinitis. Stem Cell Rep (2017) 8:1214–25. doi: 10.1016/j.stemcr.2017.04.008
170. Kotzerke K, Mempel M, Aung T, Wulf GG, Urlaub H, Wenzel D, et al. Immunostimulatory Activity of Murine Keratinocyte-Derived Exosomes. Exp Dermatol (2013) 22(10):650–5. doi: 10.1111/exd.12230
171. Cai XW, Zhu R, Ran L, Li YQ, Huang K, Peng J, et al. A Novel Non-Contact Communication Between Human Keratinocytes and T Cells: Exosomes Derived From Keratinocytes Support Superantigen-Induced Proliferation of Resting T Cells. Mol Med Rep (2017) 16:7032–8. doi: 10.3892/mmr.2017.7492
172. Shin TS, Kim JH, Kim YS, Jeon SG, Zhu Z, Gho YS, et al. Extracellular Vesicles Are Key Intercellular Mediators in the Development of Immune Dysfunction to Allergens in the Airways. Allergy Eur J Allergy Clin Immunol (2010) 65:1256–65. doi: 10.1111/j.1398-9995.2010.02359.x
173. Admyre C, Johansson SM, Qazi KR, Filén J-J, Lahesmaa R, Norman M, et al. Exosomes With Immune Modulatory Features Are Present in Human Breast Milk. J Immunol (2007) 179:1969–78. doi: 10.4049/jimmunol.179.3.1969
174. Lluis A, Depner M, Gaugler B, Saas P, Casaca VI, Raedler D, et al. Increased Regulatory T-Cell Numbers Are Associated With Farm Milk Exposure and Lower Atopic Sensitization and Asthma in Childhood. J Allergy Clin Immunol (2014) 133(2):551–9. doi: 10.1016/j.jaci.2013.06.034
175. Xanthou M. Immune Protection of Human Milk. Biol Neonate (1998) 74:121–33. doi: 10.1159/000014018
176. Van Herwijnen MJC, Zonneveld MI, Goerdayal S, Hoen ENMN, Garssen J, Stahl B, et al. Comprehensive Proteomic Analysis of Human Milk-Derived Extracellular Vesicles Unveils a Novel Functional Proteome Distinct From Other Milk Components. Mol Cell Proteomics (2016) 15:3412–23. doi: 10.1074/mcp.M116.060426
177. Willart M, Hammad H. Lung Dendritic Cell-Epithelial Cell Crosstalk in Th2 Responses to Allergens. Curr Opin Immunol (2011) 23:772–7. doi: 10.1016/j.coi.2011.09.008
178. Haj-Salem I, Plante S, Gounni AS, Rouabhia M, Chakir J. Fibroblast-Derived Exosomes Promote Epithelial Cell Proliferation Through TGF-β2 Signalling Pathway in Severe Asthma. Allergy Eur J Allergy Clin Immunol (2018) 73:178–86. doi: 10.1111/all.13234
179. Khanna K, Chaudhuri R, Aich J, Pattnaik B, Panda L, Prakash YS, et al. Secretory Inositol Polyphosphate 4-Phosphatase Protects Against Airway Inflammation and Remodeling. Am J Respir Cell Mol Biol (2019) 60:399–412. doi: 10.1165/rcmb.2017-0353OC
180. Gupta R, Radicioni G, Abdelwahab S, Dang H, Carpenter J, Chua M, et al. Intercellular Communication Between Airway Epithelial Cells Is Mediated by Exosome-Like Vesicles. Am J Respir Cell Mol Biol (2019) 60:209–20. doi: 10.1165/rcmb.2018-0156OC
181. Zhang M, Yu Q, Tang W, Wu Y, Lv J, Sun L, et al. Epithelial Exosomal Contactin-1 Promotes Monocyte-Derived Dendritic Cell–Dominant T-Cell Responses in Asthma. J Allergy Clin Immunol (2021) 4:S0091-6749(21)00720-X. doi: 10.1016/j.jaci.2021.04.025
182. Katz-Kiriakos E, Steinberg DF, Kluender CE, Osorio OA, Newsom-Stewart C, Baronia A, et al. Epithelial IL-33 Appropriates Exosome Trafficking for Secretion in Chronic Airway Disease. JCI Insight (2021) 6(4):e136166. doi: 10.1172/JCI.INSIGHT.136166
183. Mo LH, Luo XQ, Yang G, Liu JQ, Yang LT, Liu ZQ, et al. Epithelial Cell-Derived CD83 Restores Immune Tolerance in the Airway Mucosa by Inducing Regulatory T-Cell Differentiation. Immunology (2021) 163:310–22. doi: 10.1111/imm.13317
184. Ax E, Ax E, Jevnikar Z, Cvjetkovic A, Malmhäll C, Olsson H, et al. T2 and T17 Cytokines Alter the Cargo and Function of Airway Epithelium-Derived Extracellular Vesicles. Respir Res (2020) 21:1–14. doi: 10.1186/s12931-020-01402-3
185. Lee H, Zhang D, Zhu Z, Dela Cruz CS, Jin Y. Epithelial Cell-Derived Microvesicles Activate Macrophages and Promote Inflammation via Microvesicle-Containing microRNAs. Sci Rep (2016) 66:35250. doi: 10.1038/srep35250
186. Bartel S, La Grutta S, Cilluffo G, Perconti G, Bongiovanni A, Giallongo A, et al. Human Airway Epithelial Extracellular Vesicle miRNA Signature Is Altered Upon Asthma Development. Allergy Eur J Allergy Clin Immunol (2020) 75:346–56. doi: 10.1111/all.14008
187. Torregrosa Paredes P, Esser J, Admyre C, Nord M, Rahman QK, Lukic A, et al. Bronchoalveolar Lavage Fluid Exosomes Contribute to Cytokine and Leukotriene Production in Allergic Asthma. Allergy Eur J Allergy Clin Immunol (2012) 67:911–9. doi: 10.1111/j.1398-9995.2012.02835.x
188. Hough KP, Wilson LS, Trevor JL, Strenkowski JG, Maina N, Kim Y, et al. Unique Lipid Signatures of Extracellular Vesicles From the Airways of Asthmatics. Sci Rep (2018) 8:1–16. doi: 10.1038/s41598-018-28655-9
189. Levänen B, Bhakta NR, Torregrosa Paredes P, Barbeau R, Hiltbrunner S, Pollack JL, et al. Altered microRNA Profiles in Bronchoalveolar Lavage Fluid Exosomes in Asthmatic Patients. J Allergy Clin Immunol (2013) 131:894–903. doi: 10.1016/j.jaci.2012.11.039
190. Wan S, Wang S, Weng L, Zhang G, Lin Z, Fei X, et al. Cd8α + CD11c + Extracellular Vesicles in the Lungs Control Immune Homeostasis of the Respiratory Tract via TGF-β1 and IL-10. J Immunol (2018) 200(5):1651–60. doi: 10.4049/jimmunol.1701447
191. Prado N, Marazuela EG, Segura E, Fernández-García H, Villalba M, Théry C, et al. Exosomes From Bronchoalveolar Fluid of Tolerized Mice Prevent Allergic Reaction. J Immunol (2008) 181:1519–25. doi: 10.4049/jimmunol.181.2.1519
192. Helfrich S, Mindt BC, Fritz JH, Duerr CU. Group 2 Innate Lymphoid Cells in Respiratory Allergic Inflammation. Front Immunol (2019) 10:930. doi: 10.3389/fimmu.2019.00930
193. Fang SB, Zhang HY, Wang C, He BX, Liu XQ, Meng XC, et al. Small Extracellular Vesicles Derived From Human Mesenchymal Stromal Cells Prevent Group 2 Innate Lymphoid Cell-Dominant Allergic Airway Inflammation Through Delivery of miR-146a-5p. J Extracell Vesicles (2020) 9(1):1723260. doi: 10.1080/20013078.2020.1723260
194. Zou W, Liu G, Zhang J. Secretome From Bone Marrow Mesenchymal Stem Cells: A Promising, Cell-Free Therapy for Allergic Rhinitis. Med Hypotheses (2018) 121:124–6. doi: 10.1016/j.mehy.2018.09.016
195. Dhong HJ. Classification of Allergic Rhinitis: What is Most Suitable in Korea? Allergy Asthma Immunol Res (2013) 5:65–7. doi: 10.4168/aair.2013.5.2.65
196. Zhu X, Wang X, Wang Y, Zhao Y. Exosomal Long Non-Coding RNA GAS5 Suppresses Th1 Differentiation and Promotes Th2 Differentiation via Downregulating EZH2 and T-Bet in Allergic Rhinitis. Mol Immunol (2020) 118:30–9. doi: 10.1016/j.molimm.2019.11.009
197. Luo X, Han M, Liu J, Wang Y, Luo X, Zheng J, et al. Epithelial Cell-Derived Micro RNA-146a Generates Interleukin-10-Producing Monocytes to Inhibit Nasal Allergy. Sci Rep (2015) 5:1–10. doi: 10.1038/srep15937
198. Wu G, Yang G, Zhang R, Xu G, Zhang L, Wen W, et al. Altered microRNA Expression Profiles of Extracellular Vesicles in Nasal Mucus From Patients With Allergic Rhinitis. Allergy Asthma Immunol Res (2015) 7:449–57. doi: 10.4168/aair.2015.7.5.449
199. Salimi M, Barlow JL, Saunders SP, Xue L, Gutowska-Owsiak D, Wang X, et al. A Role for IL-25 and IL-33-Driven Type-2 Innate Lymphoid Cells in Atopic Dermatitis. J Exp Med (2013) 210:2939–50. doi: 10.1084/jem.20130351
200. Moy AP, Murali M, Kroshinsky D, Duncan LM, Nazarian RM. Immunologic Overlap of Helper T-Cell Subtypes 17 and 22 in Erythrodermic Psoriasis and Atopic Dermatitis. JAMA Dermatol (2015) 151:753–60. doi: 10.1001/jamadermatol.2015.2
201. Kim BE, Leung DYM. Significance of Skin Barrier Dysfunction in Atopic Dermatitis. Allergy Asthma Immunol Res (2018) 10:207–15. doi: 10.4168/aair.2018.10.3.207
202. Shin K-O, Ha DH, Kim JO, Crumrine DA, Meyer JM, Wakefield JS, et al. Exosomes From Human Adipose Tissue-Derived Mesenchymal Stem Cells Promote Epidermal Barrier Repair by Inducing De Novo Synthesis of Ceramides in Atopic Dermatitis. Cells (2020) 9:680. doi: 10.3390/cells9030680
203. Wang M, Zhao Y, Zhang Q. Human Mesenchymal Stem Cell-Derived Exosomes Accelerate Wound Healing of Mice Eczema. J Dermatolog Treat (2020) 0:000. doi: 10.1080/09546634.2020.1820935
204. Uter W, Werfel T, White IR, Johansen JD. Contact Allergy: A Review of Current Problems From a Clinical Perspective. Int J Environ Res Public Health (2018) 15(6):1108. doi: 10.3390/ijerph15061108
205. Ljubojevic Hadzavdic S, Pustišek N, Žužul K, Švigir A. Contact Allergy: An Update. G Ital di Dermatologia e Venereol (2018) 153:419–28. doi: 10.23736/S0392-0488.17.05844-8
206. Simonsen AB, Johansen JD, Deleuran M, Mortz CG, Sommerlund M. Contact Allergy in Children With Atopic Dermatitis: A Systematic Review. Br J Dermatol (2017) 177:395–405. doi: 10.1111/bjd.15628
207. Nazimek K, Bustos-Morán E, Blas-Rus N, Nowak B, Ptak W, Askenase PW, et al. Syngeneic Red Blood Cell–Induced Extracellular Vesicles Suppress Delayed-Type Hypersensitivity to Self-Antigens in Mice. Clin Exp Allergy (2019) 49:1487–99. doi: 10.1111/cea.13475
208. Guo L, Lai P, Wang Y, Huang T, Chen X, Luo C, et al. Extracellular Vesicles From Mesenchymal Stem Cells Prevent Contact Hypersensitivity Through the Suppression of Tc1 and Th1 Cells and Expansion of Regulatory T Cells. Int Immunopharmacol (2019) 74:105663. doi: 10.1016/j.intimp.2019.05.048
209. Kim JH, Jeun EJ, Hong CP, Kim SH, Jang MHMS, Lee EJ, et al. Extracellular Vesicle-Derived Protein From Bifidobacterium Longum Alleviates Food Allergy Through Mast Cell Suppression. J Allergy Clin Immunol (2016) 137:507–16.e8. doi: 10.1016/j.jaci.2015.08.016
210. Du Toit G, Tsakok T, Lack S, Lack G. Prevention of Food Allergy. J Allergy Clin Immunol (2016) 137:998–1010. doi: 10.1016/j.jaci.2016.02.005
211. Schmetterer KG, Pickl WF. The IL–10/STAT3 Axis: Contributions to Immune Tolerance by Thymus and Peripherally Derived Regulatory T-Cells. Eur J Immunol (2017) 47:1256–65. doi: 10.1002/eji.201646710
212. Zeng HT, Liu JQ, Zhao M, Yu D, Yang G, Mo LH, et al. Exosomes Carry IL-10 and Antigen/MHC II Complexes to Induce Antigen-Specific Oral Tolerance. Cytokine (2020) 133:155176. doi: 10.1016/j.cyto.2020.155176
213. Zeng H, Zhang R, Jin B, Chen L. Type 1 Regulatory T Cells: A New Mechanism of Peripheral Immune Tolerance. Cell Mol Immunol (2015) 12:566–71. doi: 10.1038/cmi.2015.44
214. Chen X, Song C-H, Feng B-S, Li T-L, Li P, Zheng P-Y, et al. Intestinal Epithelial Cell-Derived Integrin αβ6 Plays an Important Role in the Induction of Regulatory T Cells and Inhibits an Antigen-Specific Th2 Response. J Leukoc Biol (2011) 90:751–9. doi: 10.1189/jlb.1210696
215. Zhao W, Ho H, Bunyavanich S. The Gut Microbiome in Food Allergy. Ann Allergy Asthma Immunol (2019) 122:276–82. doi: 10.1016/j.anai.2018.12.012
216. Sinha A, Yadav AK, Chakraborty S, Kabra SK, Lodha R, Kumar M, et al. Exosome-Enclosed microRNAs in Exhaled Breath Hold Potential for Biomarker Discovery in Patients With Pulmonary Diseases. J Allergy Clin Immunol (2013) 132(1):219–22. doi: 10.1016/j.jaci.2013.03.035
217. De Castro LL, Xisto DG, Kitoko JZ, Cruz FF, Olsen PC, Redondo PAG, et al. Human Adipose Tissue Mesenchymal Stromal Cells and Their Extracellular Vesicles Act Differentially on Lung Mechanics and Inflammation in Experimental Allergic Asthma. Stem Cell Res Ther (2017) 8:1–12. doi: 10.1186/s13287-017-0600-8
218. Gon Y, Maruoka S, Inoue T, Kuroda K, Yamagishi K, Kozu Y, et al. Selective Release of miRNAs via Extracellular Vesicles Is Associated With House-Dust Mite Allergen-Induced Airway Inflammation. Clin Exp Allergy (2017) 47:1586–98. doi: 10.1111/CEA.13016
Keywords: extracellular vesicles, exosomes, cellular communication, immune responses, allergy, asthma, atopic dermatitis, allergic rhinitis
Citation: Hovhannisyan L, Czechowska E and Gutowska-Owsiak D (2021) The Role of Non-Immune Cell-Derived Extracellular Vesicles in Allergy. Front. Immunol. 12:702381. doi: 10.3389/fimmu.2021.702381
Received: 29 April 2021; Accepted: 31 July 2021;
Published: 19 August 2021.
Edited by:
Yann Lamarre, University of São Paulo, BrazilReviewed by:
Rong Xu, Monash University, AustraliaRobert Weil, U1135 Centre d’Immunologie et de Maladies Infectieuses (INSERM), France
Copyright © 2021 Hovhannisyan, Czechowska and Gutowska-Owsiak. This is an open-access article distributed under the terms of the Creative Commons Attribution License (CC BY). The use, distribution or reproduction in other forums is permitted, provided the original author(s) and the copyright owner(s) are credited and that the original publication in this journal is cited, in accordance with accepted academic practice. No use, distribution or reproduction is permitted which does not comply with these terms.
*Correspondence: Danuta Gutowska-Owsiak, ZGFudXRhLmd1dG93c2thLW93c2lha0B1Zy5lZHUucGw=