- 1Moores Cancer Center, University of California San Diego, La Jolla, CA, United States
- 2Department of Rheumatology, Graduate School of Medical and Dental Sciences, Tokyo Medical and Dental University (TMDU), Tokyo, Japan
- 3The Herbert Wertheim School of Public Health and Longevity, University of California San Diego, La Jolla, CA, United States
- 4Department of Medicine, University of California San Diego, La Jolla, CA, United States
As viruses continue to mutate the need for rapid high titer neutralizing antibody responses has been highlighted. To meet these emerging threats, agents that enhance vaccine adjuvant activity are needed that are safe with minimal local or systemic side effects. To respond to this demand, we sought small molecules that would sustain and improve the protective effect of a currently approved adjuvant, monophosphoryl lipid A (MPLA), a Toll-like receptor 4 (TLR4) agonist. A lead molecule from a high-throughput screen, (N-(4-(2,5-dimethylphenyl)thiazol-2-yl)-4-(piperidin-1-ylsulfonyl)benzamide, was identified as a hit compound that sustained NF-κB activation by a TLR4 ligand, lipopolysaccharide (LPS), after an extended incubation (16 h). In vitro, the resynthesized compound (2D216) enhanced TLR4 ligand-induced innate immune activation and antigen presenting function in primary murine bone marrow-derived dendritic cells without direct activation of T cells. In vivo murine vaccination studies demonstrated that compound 2D216 acted as a potent co-adjuvant when used in combination with MPLA that enhanced antigen-specific IgG equivalent to that of AS01B. The combination adjuvant MPLA/2D216 produced Th1 dominant immune responses and importantly protected mice from lethal influenza virus challenge. 2D216 alone or 2D216/MPLA demonstrated minimal local reactogenicity and no systemic inflammatory response. In summary, 2D216 augmented the beneficial protective immune responses of MPLA as a co-adjuvant and showed an excellent safety profile.
Introduction
Adjuvants are used to enhance the potencies of protein and peptide-based vaccines (1, 2). Common strategies in designing such adjuvants incorporate natural or semisynthetic components that stimulate the innate immune system. Ligands for pattern recognition receptors (PRRs) are critical activators of innate immunity. They include Toll-like receptors (TLRs) and C-type lectin receptors (CLRs), as well as the nucleotide-binding oligomerization domain (NOD)-like receptors (NLRs) and retinoic acid-inducible gene I (RIG-I) like receptors, which are being actively investigated for activity and safety as adjuvants (1). The FDA has approved a TLR4 ligand, monophosphoryl lipid A (MPLA), and a TLR9 ligand, CpG 1018, for clinical use as vaccine adjuvants (3–5). Despite these advances, single-agent adjuvants are not always sufficient to generate high titer protective antibody responses in vulnerable populations, including the elderly and immunocompromised hosts (3, 6, 7).
To address this medical need, we first examined published data on combinations of available adjuvants to augment protective immune responses. Preclinical data suggest that this is a promising avenue for the use of PRR ligands as adjuvants. The TLR4 adjuvant MPLA has been approved for use in co-adjuvant systems with AS04 (oil-in-water emulsion) and AS01B (saponin) (2, 8–10). Other co-adjuvant systems, including CAF01 with a CLR Mincle ligand and IC31 with a TLR9 ligand, ODN1, are currently being tested in clinical trials (11, 12).
The stimulation of PRRs commonly activates the NF-κB pathway, which plays a critical role in initiating signaling pathways that lead to the differentiation, maturation, and recruitment of antigen-presenting cells (APCs) (13). Thus, we postulated that small synthetic molecules that enhance NF-κB activation initiated by a TLR4 ligand could improve adjuvant efficacy. To obtain such molecules, we previously performed a cell-based high-throughput screening (HTS) campaign using the human monocytic cell line THP-1 equipped with an NF-κB fluorescence resonance energy transfer (FRET) reporter construct (14). Cells were simultaneously stimulated with lipopolysaccharide (LPS) as a TLR4 ligand and individual compounds from a small molecule compound library (~168K compounds) (14). A hit compound N-(4-(2,5-dimethylphenyl)thiazol-2-yl)-4-(piperidin-1-ylsulfonyl)benzamide (hereafter designated as compound 2D216) was identified that prevented decay of NF-κB activation by LPS at 16 h incubation, without increasing the peak NF-κB activation at 5 h incubation (14). This characteristic attribute of sustaining LPS-induced NF-κB activation without further increasing early peak NF-κB activity suggested that such compound may be a safe and an effective co-adjuvant for MPLA, an approved TLR4 adjuvant.
In this report, we examined the adjuvant activity of 2D216 alone and in combination with MPLA in vitro and in vivo. As a single agent, the compound showed minimal activity on APCs including dendritic cells and B cells and no direct activity on T cells. However, when combined with a TLR4 ligand, 2D216 enhanced the transcription of chemokines, cytokines, and surface molecules associated with antigen presenting function. In the preclinical immunization models, the combination adjuvant (MPLA/2D216) augmented antigen-specific IgG levels equivalently to that of AS01B and induced a minimal local intramuscular infiltration of inflammatory cells and systemic inflammatory response.
Materials and Methods
Cells and Reagents
Mouse bone marrow-derived dendritic cells (mBMDCs) were generated from the femurs and tibias of mice as previously described (15). Mouse CD4+ T cells and CD19+ B cells were isolated from splenocytes using EasySep Mouse CD4 T Cell Isolation Kit (ST-19852, STEMCELL Technologies, Vancouver, Canada) and mouse CD19 MicroBeads (130-121-301, Miltenyi Biotec, Bergisch Gladbach, Germany) according to the manufacturer’s protocol. Cells were cultured in RPMI supplemented with 10% FBS (Omega, Tarzana, CA, USA) and penicillin/streptomycin (100 unit/mL/100 μg/mL, Thermo Fisher Scientific, Waltham, MA). Compound 2D216 was synthesized in our laboratory, and purity was confirmed by LC/MS. Ovalbumin (OVA) was purchased from Worthington Biochemical (Lakewood, NJ, USA). LPS (LPS-EB Ultrapure tlrl-eblps) and MPLA (vac-mpla) were purchased from InvivoGen (San Diego, CA, United States). AS01B was purchased from GlaxoSmithKline (GSK, Middlesex, United Kingdom, Zoster Vaccine Recombinant, Adjuvanted SHINGRIX).
Animals
Six to eight-week-old BALB/c, C57BL/6, and DO11.10 mice were purchased from The Jackson Laboratory (Bar Harbor, ME, United States). Myd88-/- mice were a gift from Dr. Shizuo Akira (Osaka University, Osaka, Japan). Ticam1Lps2 mice were kindly provided by Dr. Bruce Beutler (University of Texas Southwestern Medical Center, Dallas, TX, USA). All animal experiments received prior approval by the UC San Diego Institutional Animal Care and Use Committee (IACUC). The influenza challenge study was performed by the Institute for Antiviral Research of Utah State University. The study was approved by IACUC of Utah State University.
nCounter Gene Expression Assay and Data Analysis
nCounter Mouse Immunology Panels (XT-CSO-MIM1-12, NanoString, Seattle, WA, United States), including a total of 561 genes, were used according to the manufacturer’s instruction. Briefly, mBMDCs (0.5×106 cells/mL) were treated with 0.5% dimethylsulfoxide (DMSO) as vehicle, 2D216 (5 μM), LPS (1 ng/mL), or LPS/2D216 for 5 h and total RNA was isolated. Data were acquired in triplicates, and the expression values were normalized using positive controls to eliminate platform related variation, negative controls to eliminate background effect, and housekeeping genes to remove variation due to sample input. Genes with zero counts were removed from group comparisons, linear models for microarray with the trend method were used to compare log2 expression values among groups. The Benjamini-Hochberg procedure was applied to control the false discovery rate (FDR). A gene was considered significantly changed if FDR < 0.05. Principal component analysis (PCA) was performed and plotted using Prism 9 software (GraphPad Software, San Diego, CA, United States) to assess similarity or dissimilarity between the datasets. Data are deposited and available through GEO with accession number GSE181134 (https://www.ncbi.nlm.nih.gov/geo/query/acc.cgi?acc=GSE181134:;!!LLK065n_VXAQ!yy4cAlugEz2j7waYDkyjNnKVpmEpSpvNg6DspkiEjVpYOpT_OMuq6sh4RWboK6I2wg$).
Cytokine ELISA
Wild type, Myd88-/- and TicamLps2 mBMDCs (0.5×106 cells/mL), mouse CD19+ B cells (1×106 cells/mL), and mouse CD4+ T cells (1×106 cells/mL) were treated as detailed in each figure legend, and the culture supernatants were tested for IL-12 p40/p70, TNF-α, IL-6, and IL-2 by ELISA. Antibodies and reagents are listed in Supplementary Table 1.
Antigen-Specific T Cell Proliferation Assay
mBMDCs (5×105 cells/mL) from BALB/c mice were treated with 2D216 (5 μM) with or without MPLA (100 ng/mL) overnight and OVA protein (10 μg/mL) were loaded for 4 h. CD4+ T cells were isolated from spleens of DO11.10 T cell receptor (TCR) transgenic mice using EasySep Mouse CD4 T Cell Isolation Kit (ST-19852, STEMCELL Technologies) and labeled with 5-(and -6)-carboxyfluorescein diacetate succinimidyl ester (CFSE, 4 μM, C34554, Molecular Probe, Eugene, OR, United States). mBMDCs were washed twice and co-cultured with equal numbers of CFSE-labelled CD4+ T cells (5×105 cells/mL) for 3 days. Supernatants were removed and stored at -20°C and later tested for IL-2 by ELISA. The T cells were stained with AF647-conjugated anti-DO11.10 TCR antibodies (BD Biosciences, United States) and analyzed with a MACSQuant Analyzer 10 (Miltenyi Biotec) for CSFE intensity in the TCR+ gated population. The % divided, the percent of the live CFSE-labelled gated T cells that entered division was calculated using FlowJo (version 10.6.1, Becton Dickinson, Ashland, OR, United States). Antibodies and reagents are listed in Supplementary Table 1. For direct activation of mouse primary CD4+ T cells, CD4+ T cells were treated with plate-bound anti-mouse CD3 (0.5 μg/mL, 16-0032-82 Thermo Fischer Scientific, Waltham, MA, United States) and soluble anti-mouse CD28 (1 μg/mL, Thermo Fischer Scientific, #16-0281-82) antibodies for 3 days.
Analysis of CD40 and CD86 Expression
For co-stimulatory molecules and cell surface activation makers, mBMDCs and CD19+ B cells were treated for 24 h as described in each figure legend. Antibodies and reagents are listed in Supplementary Table 1.
Immunoblot
mBMDC cells (5×105 cells/mL) cells were rested overnight and then treated with 5 µM 2D216 in the presence or absence of 1 ng/mL LPS for indicated time periods. Treated cells were lysed with PhosphoSafe Extraction Reagent (71296, EMD Millipore, Billerica, MA, United States) mixed with protease inhibitor cocktail (11697498001, Roche, Manheim, Germany) and 0.1% SDS. Protein concentrations were determined using Pierce BCA protein assay kit (23225, Thermo Fisher Scientific). The reduced and denatured cell lysates (10 µg protein) were separated by gel electrophoresis and transferred onto PVDF membranes. The membranes were blocked with 5% bovine serum albumin (BSA) in 0.1% Tween-20 Tris-buffered saline (TBST). The membranes were incubated in primary antibody and secondary antibody diluted in 5% - BSA- TBST and signals were visualized with SuperSignal™ West Dura Extended Duration Substrate (34075, Thermo Fisher Scientific). Anti-phospho NF-κB p65, and anti-phospho IRF3 (Ser396) and anti-actin were purchased from Cell Signaling Technology (Danvers, MA).
Formulation of Antigen and Adjuvant for In Vivo Studies
The adjuvant and antigen solutions for immunization were separately prepared and then mixed together. For the combined adjuvant 50µl 2D216 (40 mM stock solution in DMSO) was added to 5µL MPLA (20 µg/mL stock solution). Separately, antigen OVA was prepared by adding 4 µL of OVA (50 mg/mL stock solution in saline) to a final volume of 445 µL saline. OVA in saline was then added to the adjuvant in DMSO solution prepared above and the mixture was vortexed to obtain 0.5 mL of vaccine formulation for injection (10 doses). To verify the homogeneity and stability of the vaccine formulation, we analyzed the sample by optical imaging. A freshly prepared formulation (0.5 mL) was added to a 1mL quartz cuvette and filmed for 30 minutes using a high-resolution smartphone camera (Samsung Galaxy S10, Korea) mounted at a fixed distance. Frames captured at 0, 5, 10, 15, 20, 25, and 30 minutes were extracted using Adobe Premier Pro CS5 software and three regions of interest (ROIs) were demarcated using ImageJ (Ver 1.53e) and the mean pixel density was calculated for each ROI (Supplementary Figures 1A–D). The formulation of OVA with MPLA and 2D216 retained its mean density and homogeneity for 30 minutes unlike the positive control compound which demonstrated aggregation and increased density in the bottom ROI with gravity over time (Supplementary Figures 1B–D).
In Vivo Adjuvant Activity Study
BALB/c male and female mice (n = 5-10/group) were immunized in the right gastrocnemius muscle with model antigen ovalbumin (20 μg/mouse, Worthington Biochemicals, Lakewood, NJ, United States) mixed with 2D216 (200 nmol/mouse) and/or MPLA (10 ng/mouse) in a total volume of 50 μL on day 0 and day 21. 10% DMSO and AS01B (MPLA 1 μg and saponin QS-21 1 μg/mouse) were used as a vehicle and positive controls, respectively. On days 28 and 42, mice were bled, and OVA-specific IgG2a and IgG1 titers were measured by ELISA as previously described (16). For splenocyte re-stimulation analysis, spleens were harvested on day 28, and single-cell suspensions were prepared using MACS (Miltenyi Biotec). After red cell lysis using ammonium-chloride-potassium (ACK) lysing buffer (A1049201, Gibco, Waltham, MA, United States), splenocytes (5×106 cells/mL) were re-stimulated with 100 μg/mL OVA protein for 3 days. IFN-γ in supernatants were measured using mouse IFN-γ ELISA kit (R&D Systems, Minneapolis, MN, United States). For germinal center reaction analysis, draining lymph nodes, including popliteal and inguinal lymph nodes, were harvested on day 28, and single-cell suspensions were stained with fluorescent antibodies (Supplementary Table 1) subjected to flow cytometry analysis.
Maximal Feasible Dose Study
BALB/c female mice (n = 5/group) were intraperitoneally injected with 50 μ;g (110 nmol)/g mouse or 100 μg (220 nmol)/g mouse and body weights and behaviors were monitored for 7 days. Compound 2D216 was incorporated into a liposome formulation containing 2D216, 1,2-dioleoyl-3-phosphatidylcholine (DOPC, Avanti polar Lipids, St. Louis, MO, United States) and cholesterol (C8667, Sigma) with a mol % ratio of 10:80:10, respectively, using the thin-film rehydration method in our laboratory to achieve high concentrations (17).
Systemic and Local Reactogenicity Studies
BALB/c female mice (n = 3-5/group) were injected in the right gastrocnemius muscle with 2D216 (200 nmol/mouse) with or without MPLA (10 ng/mouse). AS01B was used as a control. After 2 and 24 h, mice were bled and sera were assessed for cytokine (TNF-α and IL-6) and C-reactive protein (CRP) levels by Luminex multiplex cytokine assay (Thermo Fischer Scientific) and ELISA. After 24 h, the right gastrocnemius muscles were harvested and histological sections with 5 μm thickness were stained with hematoxylin and eosin (H&E) and analyzed by BZ-X810 microscopy (KEYENCE, Osaka, Japan).
Influenza Challenge Test
The influenza challenge test was performed at the Institute for Antiviral Research of Utah State University as previously described (17). Briefly, BALB/c mice (n = 10/group) were intramuscularly immunized with 2D216 (100 nmol/mouse) with or without MPLA (1 μg/mouse) as an adjuvant and inactivated influenza A virus [IIAV, A/California/07/2009, (H1N1)pdm09] (0.3 μg/mouse) as an antigen in a total volume of 50 µL on day 0 and intranasally challenged with approximately 1×105 (3×LD50) cell culture infectious doses (CCID50) of homologous influenza A virus, (H1N1)pdm09 in 50 µL on day 21. Body weights and survival of mice were monitored. Virus neutralization and hemagglutination-inhibition (HAI) titers were determined by Antiviral Research of Utah State University as previously described (17).
Statistical Analysis
Data obtained in vitro studies are shown as means with standard deviation (SD), and in vivo data are presented as means with standard error of the mean (SEM). For in vitro data, Mann-Whitney U test to compare two groups was used. For multiple comparisons of in vivo data, one-way ANOVA with Dunnett’s post hoc test or Kruskal-Wallis tests with Dunn’s post hoc test was applied. For body weights, a last-value-carried-forward approach was used to impute missing values after a mouse was sacrificed, the average weight over time was used as an outcome for comparison, and Kruskal-Wallis tests with Dunn’s post hoc test was applied. A log-rank test was used to test for a significant difference between Kaplan-Meier survival curves. Prism 9 software (GraphPad Software) was used. A p-value less than 0.05 was considered statistically significant.
Results
2D216 Augments TLR4 Stimulation of Murine Bone Marrow-Derived Dendritic Cells
In the HTS campaign, we identified a hit compound N-(4-(2,5-dimethylphenyl)thiazol-2-yl)-4-(piperidin-1-ylsulfonyl)benzamide (2D216, Figure 1A), which prolonged NF-κB reporter activation induced by LPS, a TLR4 ligand, as a primary stimulus. Thus, we hypothesized that this compound might enhance the activation of APCs in combination with a TLR4 ligand (e.g., the FDA-approved adjuvant MPLA). To test this hypothesis, we resynthesized the compound and confirmed its purity (> 99%). To assess the innate immune activation profile of 2D216 in the presence and absence of TLR4 ligand, we examined mRNA transcript levels after stimulating primary mBMDCs by nCounter® gene expression assay (NanoString). Principle component analysis (PCA) indicated that 2D216 has a limited intrinsic activity that was distinct from vehicle (Veh) and LPS, and the combination of LPS/2D216 was clustered separately from LPS alone (Figure 1B). 2D216 alone upregulated expression (2 fold) of only six transcripts compared to Veh stimulated cells (Figure 1C). In contrast, the skewing volcano plots between LPS and LPS/2D216 demonstrated that expression levels of 22 genes were enhanced upon LPS/2D216 treatment (Figure 1D). These molecules included cell surface activation markers as well as chemokines and cytokines. We selected two analytes and confirmed that 2D216 significantly enhanced secretion of IL-12 and TNF-α by LPS co-stimulated mBMDCs, while 2D216 alone was insufficient for detectable cytokine release (Figure 1E and Supplementary Figures 2A–C).
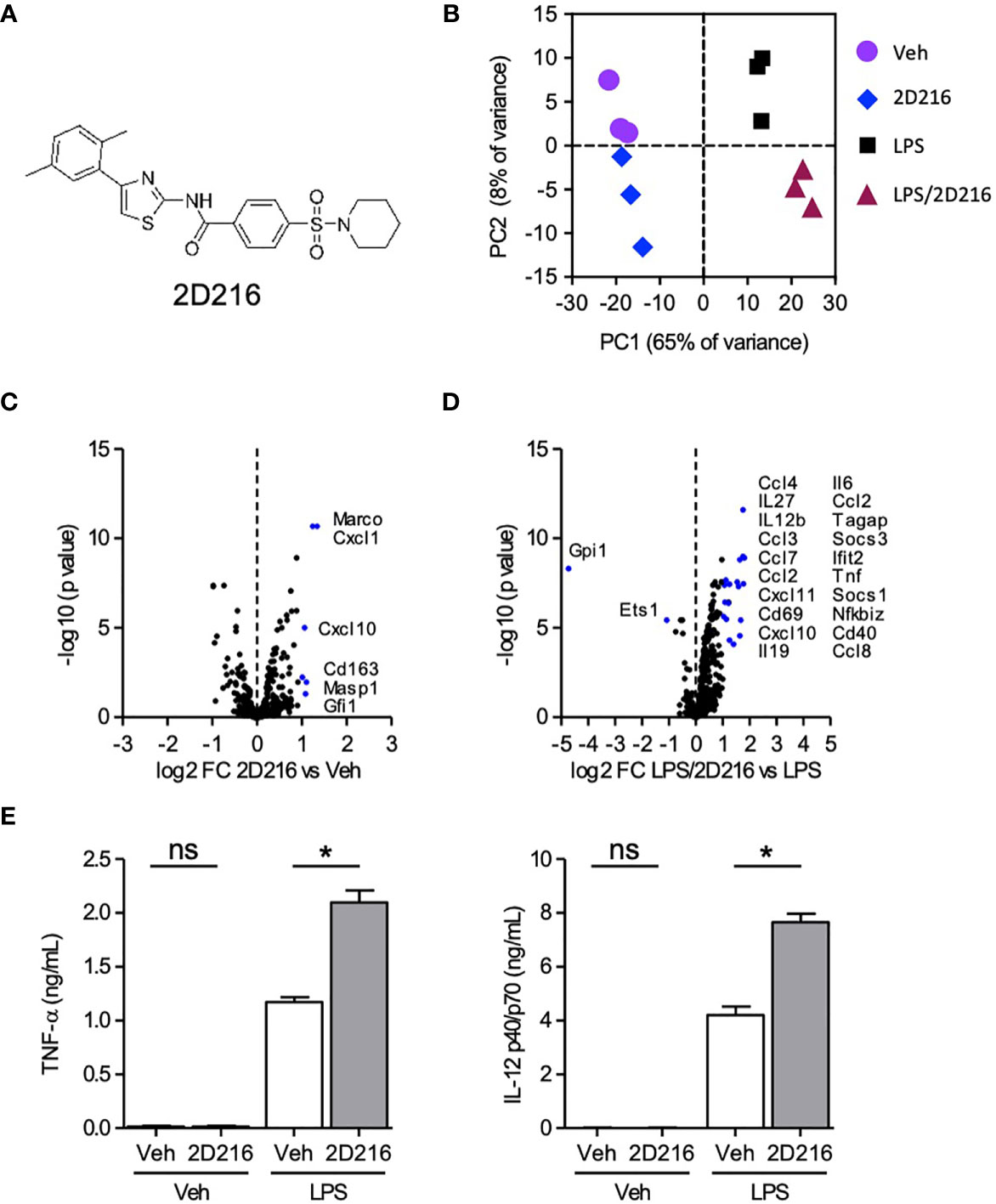
Figure 1 Augmentation of TLR4 ligand-mediated activation of mBMDCs by 2D216. (A) Chemical structure of compound 2D216. (B–D) nCounter gene expression analysis of genes upregulated by LPS/2D216 and LPS. mBMDCs (1×106 cells/mL) were incubated with vehicle (Veh), 2D216 (5 μM), LPS (1 ng/mL), or 2D216 (5 μM) plus LPS (1 ng/mL) for 5 h, and total RNAs were subjected to nanoString nCounter® Mouse Immunology Panel analysis containing 561 mouse immune-related genes. (B) PCA demonstrates the clustering of the treatments. (C) Genes significantly up-regulated by 2D216 compared to Veh and (D) LPS/2D216 compared with LPS alone are shown as blue dots (log2 FC > 1 and FDR < 0.05). (E) Cytokine secretion induced by 2D216. mBMDCs (0.5×106 cells/mL) were incubated with Veh, 2D216 (5 μM), LPS (1 ng/mL), or LPS (1 ng/mL)/2D216 (5 μM) plus LPS (1 ng/mL) for 20 h, and the levels of IL-12 p40/p70 and TNF-α in the LPS/2D216 culture supernatants were significantly greater than LPS alone. Data represent mean ± SD of triplicates of two independent experiments showing similar results. *p < 0.05 by Mann-Whitney U test compared to Veh/Veh or MPLA/Veh. ns, not significant.
TLRs signal through two major intermediaries: Myeloid differentiation primary response 88 (MyD88) and the TIR domain-containing adapter-inducing interferon-β (TRIF) protein. TLR4 is the only TLR that utilizes both pathways and TLR7 only utilizes MyD88 (13). We tested the ability of 2D216 to augment the cytokine production induced by LPS and a TLR7 ligand, 1V270 (16), in cells deficient in MyD88 and TRIF (Supplementary Figures 2A–C). 2D216 treatment enhanced the cytokine production in wild type cells for both ligands. There was no TNF or IL-12 production induced in the MyD88 cells, however the level of RANTES induced by LPS (through the TRIF pathway) was greater in the 2D216 treated cells. In the TicamLps2 (TRIF mutant) cells the 2D216 and 1V270 cotreated cells produced significantly higher TNF, and IL-12 than cells treated with 1V270 alone. These results indicate that 2D216 can enhance cytokine production for another TLR ligand and is not exclusive to TLR4. 2D216 does not stimulate phosphorylation of NF-κB p65 or interferon regulatory factor (IRF)3 (Supplementary Figure 2D and Supplementary Figures 3A–C) which further demonstrated that 2D216 is not active directly targeting MyD88, TRIF or their major downstream signaling pathways.
2D216 Enhances Activation of Antigen Presenting Cells and Does Not Directly Augment T Cell Activation In Vitro
2D216 had minimal intrinsic effects on mBMDC gene expression and cytokine production without a primary stimulus (Figure 1). 2D216, when used in conjunction with a TLR4 ligand, significantly upregulated surface markers for APC activation, CD40 and CD86, compared to either 2D216 or TLR4 ligand alone (Figures 2A, B). 2D216 also enhanced LPS-induced expression of activation markers (CD86 and CD69) and secretion of IL-6 in primary mouse splenic CD19+ B cells in vitro (Supplementary Figures 4A–C). To assess if 2D216 would be effective in enhancing antigen-presenting function and cognate T cell priming, mBMDCs stimulated by 2D216/MPLA were co-cultured in the presence of antigen with CFSE-labeled CD4+ T cells expressing OVA-specific T cell receptors (from DO11.10 mice) (Figures 2C, D). mBMDCs treated with OVA and MPLA/2D216 induced significantly higher T cell proliferation and IL-2 release than those by treatment of MPLA alone (Figures 2C, D). To examine the possibility that 2D216 directly activates T cells to enhance their proliferation, anti-CD3/anti-CD28 antibody-activated primary splenic CD4+ T cells were incubated with 2D216 (Figure 2E). 2D216 had no effect on proliferation and IL-2 release by T cells in the presence and absence of TCR engagements (Figure 2E). These results suggested that 2D216 likely augmented the APC activation by MPLA in the co-culture system with minimal effects on T cells.
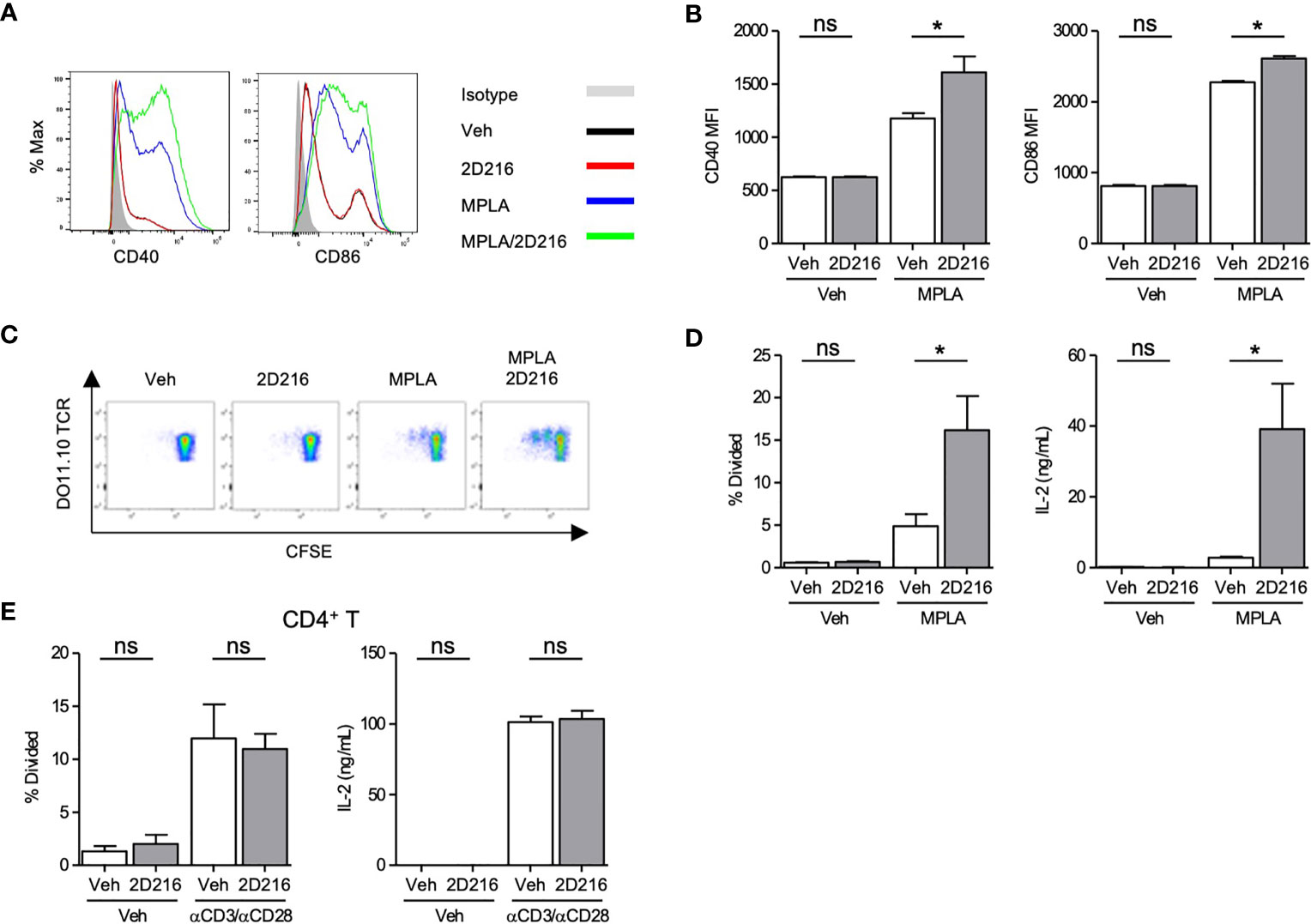
Figure 2 2D216 enhances MPLA-induced antigen presenting function in mBMDCs. (A, B) Co-stimulatory molecule expression induced by 2D216. mBMDCs (1×106 cells/mL) were incubated with vehicle (Veh), 2D216 (5 μM), MPLA (100 ng/mL), or 2D216 (5 μM) plus MPLA (100 ng/mL) for 24 h and expression of CD40 and CD86 were compared by mean fluorescent intensity (MFI) analyzed using flow cytometry, *p < 0.05 by Mann-Whitney U test compared to Veh/Veh or MPLA/Veh. (C) Representative plots of T cell proliferation with MPLA/2D216 treated mBMDCs in co-culture. mBMDCs (0.5×106 cells/mL) were treated with Veh, 2D216 (5 μM), MPLA (100 ng/mL), or MPLA (100 ng/mL)/2D216 (5 μM) overnight, and pulsed with OVA (10 μg/mL) for 4 h. Antigen-pulsed mBMDCs were then co-cultured with the same number of CFSE-labelled CD4+ DO11.10 T cells for 3 days. (D) T cell proliferation was determined by CFSE dilution. Percent T cells that divided relative to the original population is shown. IL-2 released in the culture supernatant was determined by ELISA. (E) Primary mouse splenic CD4+ T cells were isolated, CFSE-labeled and stimulated with plate-bound anti-CD3 antibodies (0.5 μg/mL) and soluble anti-CD28 antibodies (1 μg/mL) with or without 2D216 (5 μM) for 3 days. T cell proliferation was determined by CFSE dilution. IL-2 levels in the culture supernatant were measured by ELISA. Data represent mean ± SD of triplicates of two independent experiments showing similar results. *p < 0.05 by Mann-Whitney U test compared to Veh/Veh or MPLA/Veh. ns, not significant.
2D216 Enhances Th1-Biased Adjuvant Activity In Vivo
The above results demonstrated that 2D216 acted as a co-adjuvant with TLR4 ligands in mBMDCs and B cells in vitro. In vivo co-adjuvant activity of 2D216 was examined in male and female mice separately. Female BALB/c mice were intramuscularly (i.m.) immunized on days 0 and 21 with the model antigen OVA adjuvanted with 2D216 alone or in combination with MPLA (MPLA/2D216) (Figure 3A). Inoculation with 2D216 as a single adjuvant significantly enhanced the levels of anti-OVA IgG2a and IgG1 produced; however, a greater increase was seen in mice immunized with MPLA/2D216-adjuvanted OVA at both 28 and 42 days (Figures 3B, C). The levels of anti-OVA IgG2a demonstrated a decline at 42 days when 2D216 was used as a single adjuvant; however, anti-OVA IgG2a levels were sustained when used in combination with MPLA (Figure 3C).
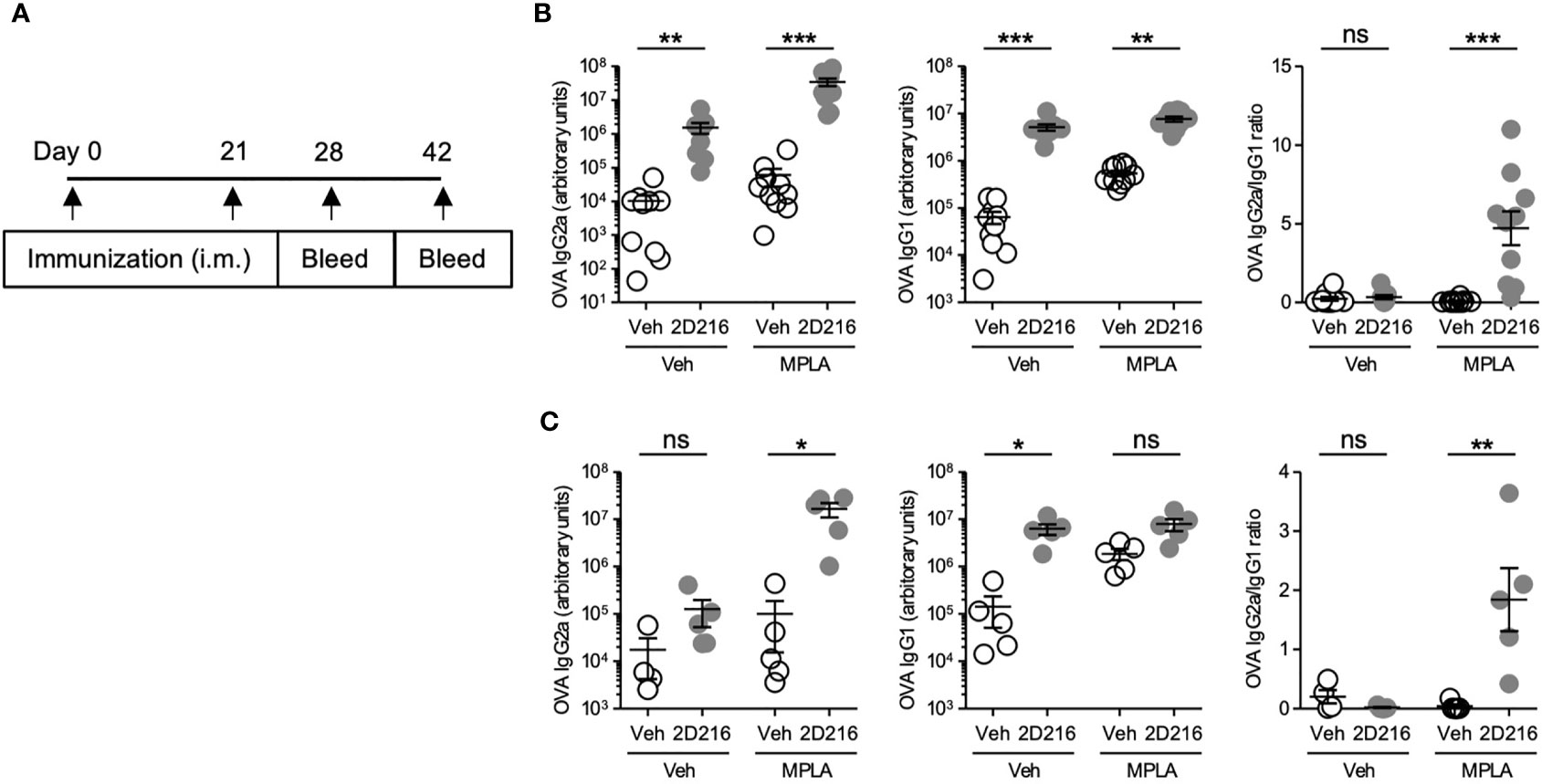
Figure 3 2D216 enhances MPLA-induced Th1-dominant adaptive immune responses in female mice. (A) Female BALB/c mice (n = 5-10/group) were i.m. immunized with OVA (20 μg/mouse) adjuvanted with vehicle (Veh; 10%DMSO in saline), 2D216 (200 nmol/mouse), MPLA (10 ng/mouse), or a combination (MPLA/2D216) and bled on day 28 and day 42. OVA-specific IgG2a and IgG1 levels in sera from day 28 (B) and day 42 (C) were assayed by ELISA. IgG2a/IgG1 ratio was calculated. Data shown are means ± SEM of two independent experiments showing similar results. *p < 0.05, **p < 0.01, ***p < 0.001 by Kruskal-Wallis tests with Dunn’s post hoc test. ns, not significant.
In a separate cohort, male BALB/c mice were immunized, i.m. on days 0 and 21, with OVA adjuvanted with 2D216 alone or in combination with MPLA (Figure 4A). An additional group with FDA-approved combination adjuvant AS01B (MPLA/saponin) was added as a comparator. On day 28, 2D216 enhanced MPLA-induced OVA-specific IgG2a by over 100 fold, while antigen-specific IgG1 production showed lesser increase in mice vaccinated with 2D216 alone compared to vehicle adjuvanted OVA (Figure 4B). The levels of anti-OVA antibodies were equivalent between mice immunized with the two combination adjuvants (AS01B as MPLA/saponin or MPLA/2D216) (Figure 4B). When IgG2a: IgG1 ratios were plotted to evaluate Th1/Th2 balance, 2D216 significantly enhanced Th1-biased immunoglobulin responses when combined with MPLA (Figure 4B). On day 28, the draining lymph nodes were removed and analyzed for follicular helper T cells (Tfh) and germinal center (GC) B cells, both of which are important for sustained humoral responses in draining lymph nodes (18) (Figures 4C, D). Immunization with 2D216 in combination with MPLA increased the proportions of Tfh cells compared to MPLA/Veh (Figure 4D, left panel), while 2D216 showed minimal effects on the GC B cell population (Figure 4D, right panel). When splenocytes harvested on day 28 were restimulated ex-vivo with OVA for 3 days, cells from mice immunized with the combination adjuvant, MPLA/2D216 and AS01B, secreted significantly higher levels of IFN-γ into the culture supernatants compared to the vehicle control (Figure 4E). These data further support that 2D216 augments Th1 responses induced by MPLA.
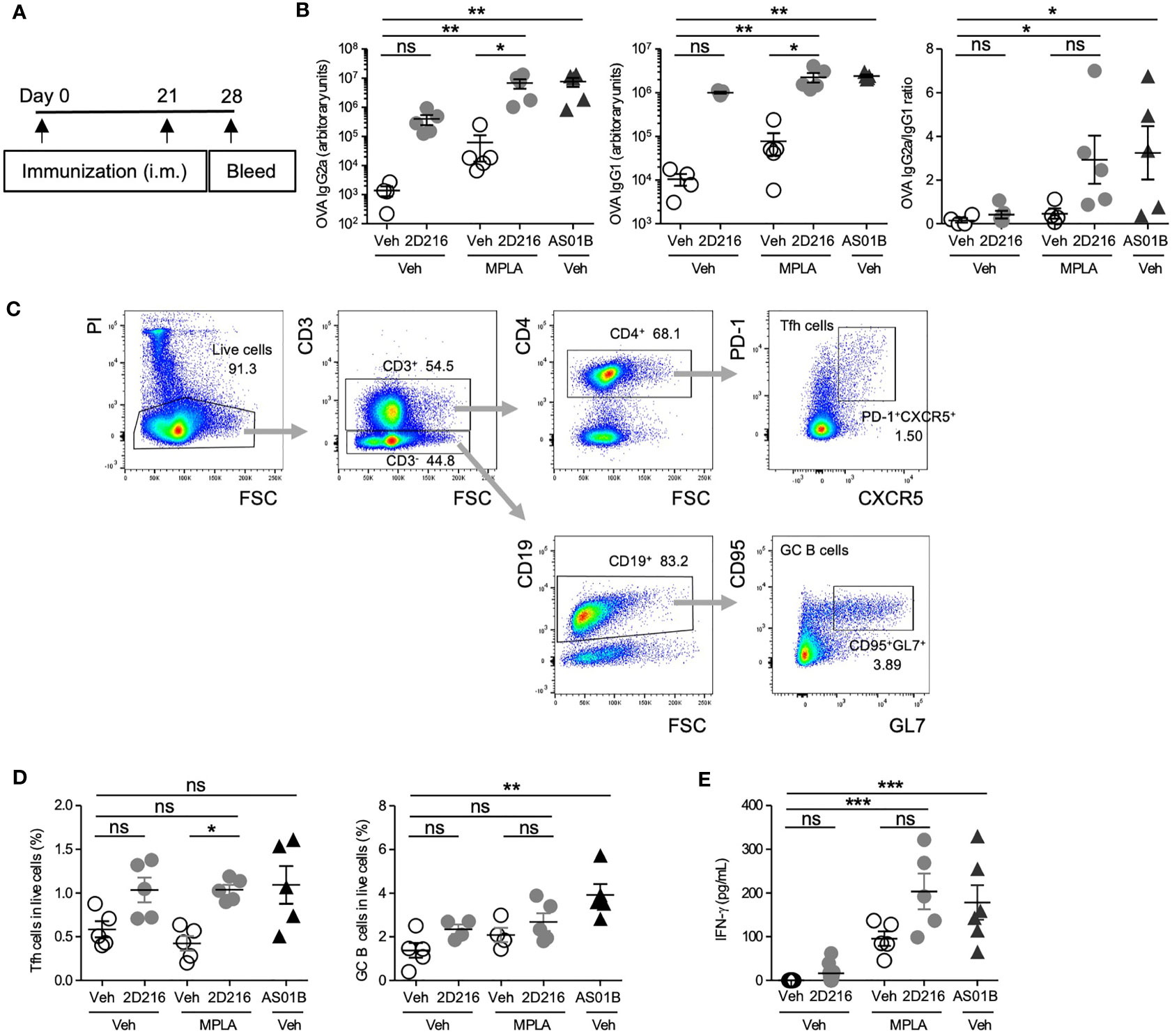
Figure 4 MPLA/2D216 induced Th1-dominant cellular immune responses in male mice. (A) Male BALB/c mice (n = 5/group) were i.m. immunized with OVA protein (20 μg/mouse) adjuvanted with vehicle (Veh;10%DMSO in saline), 2D216 (200 nmol/mouse), MPLA (10 ng/mouse), or MPLA/2D216, and bled on day 28. (B) OVA-specific IgG2a and IgG1 levels in sera on day 24 were assayed by ELISA. IgG2a/IgG1 ratios were calculated. (C, D) On day 28, draining lymph nodes were harvested, and Tfh and GC B cells were examined by flow cytometry analysis. The gating strategy is shown (C). Percent Tfh cells (CD3+CD4+PD-1+CXCR5+) and GC B cells (CD3-CD19+CD95+GL7+) in the gated live cells are shown (D). (E) On day 28, splenocytes (5×106 cells/mL) were harvested and re-stimulated with OVA (100 μg/mL) for 3 days. The levels of IFN-γ in the culture supernatants were measured by ELISA. Data shown are means ± SEM of two independent experiments showing similar results. *p < 0.05, **p < 0.01, ***p < 0.001 by Kruskal-Wallis tests with Dunn’s post hoc test. ns, not significant.
2D216 Shows Minimal Local and Systemic Reactogenicity
To address compound safety, a maximal feasible dose of 2D216 (100 μg/g mouse body weight) was intraperitoneally administered to the mice. The injection of highest dose of 2D216 had no effects on body weight and behavioral changes for 7 days (Figure 5A). To examine systemic and local reactogenicity of the combination adjuvant of MPLA/2D216, we i.m. immunized BALB/c mice with 2D216 alone or in combination with MPLA, and compared the effects to AS01B. Sera were collected at 2 and 24 h post-injection, and inflammatory markers, CRP, TNF-α and IL-6, were measured. 2D216 alone or a combination with MPLA did not result in an elevation of these inflammatory markers, while the use of AS01B significantly increased the levels of these inflammatory proteins in the sera of mice 24 h post-injection (Figures 5B, C). Muscle tissues at injection sites were harvested 24 h post-injection. The histologic examination showed that neither 2D216 alone nor in combination with MPLA resulted in excess inflammatory cell infiltration or muscle necrosis (Figure 5D). In contrast, inflammatory cell infiltration areas in the muscle tissues injected with AS01B were observed (Figure 5D). Collectively, these data indicate that the combination adjuvant of 2D216 and MPLA has an acceptable safety profile.
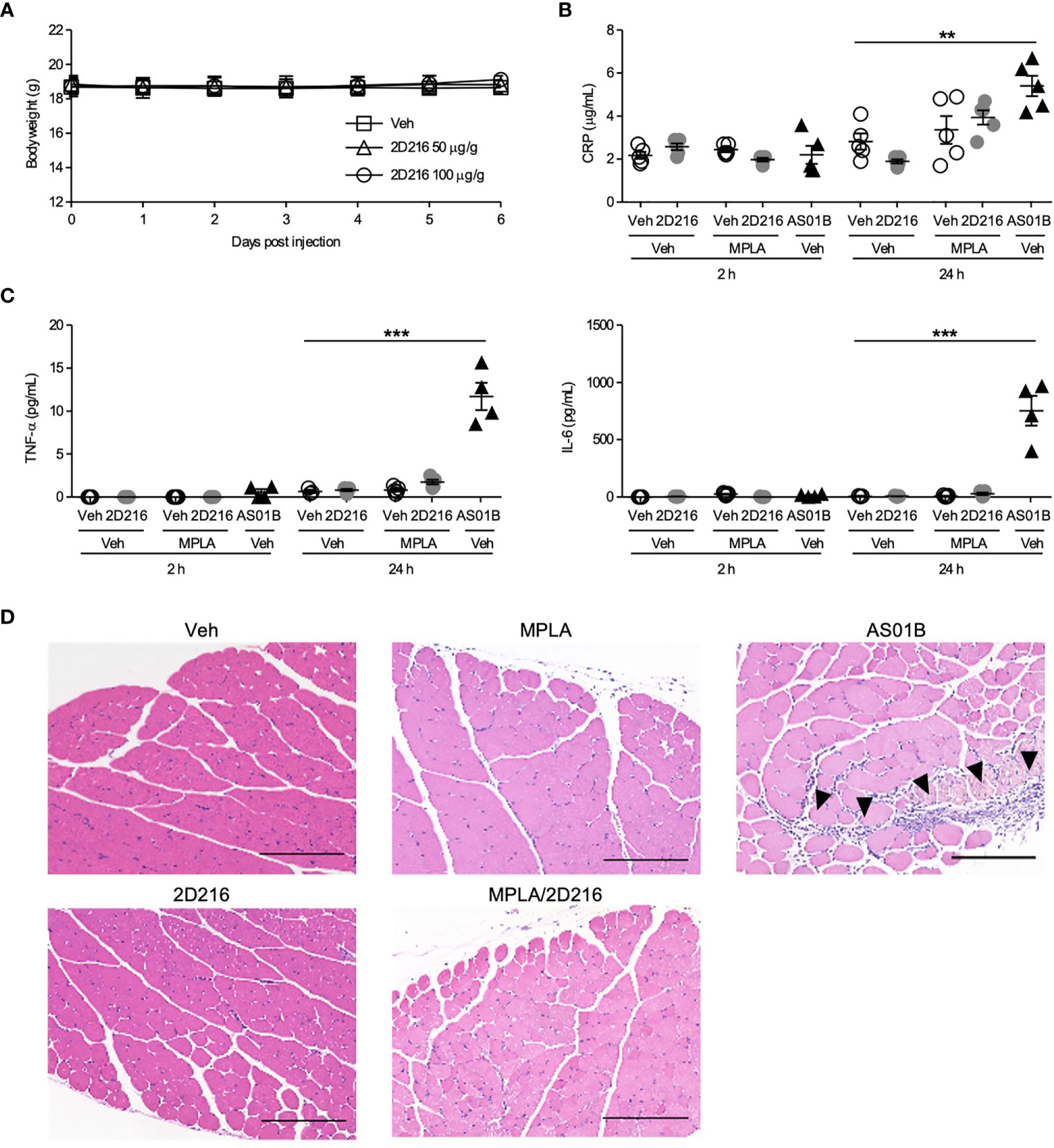
Figure 5 MPLA/2D216 shows a minimal local and systemic reactogenicity. (A) Maximal feasible dose test. BALB/c mice (n = 5/group) were intraperitoneally injected with vehicle (Veh; 10%DMSO in saline), or 2D216 (50 and 100 μg/g mouse) on day 0 and body weights were monitored for 7 days. (B–D) BALB/c mice (n = 3-5/group) were i.m. injected with Veh, 2D216 (200 nmol/mouse), MPLA (10 ng/mouse), MPLA/2D216 or AS01B (10 μL/mouse). Two and 24 h after the injection, sera were collected, and CRP (B), TNF-α (C), and IL-6 (C) levels were determined by ELISA. Data shown are means ± SEM of two independent experiments showing similar results. **p < 0.01, ***p < 0.001 by one-way ANOVA with Dunnett’s post hoc test compared to Veh/Veh. (D) Local reactogenicity. After 24 h, the injected muscles were harvested. Examples of H&E stained histological sections are shown (scale bar 200 μm). Arrowheads indicate the areas with inflammatory cell infiltration. ns, not significant.
Combination Adjuvant With 2D216 Protects Mice From Lethal Influenza Challenge
The adjuvant effects of MPLA/2D216 were further examined in a lethal influenza virus challenge model in mice. BALB/c mice were i.m. immunized once with inactivated influenza A virus (IIAV) [A/California/07/2009, (H1N1)pdmCal9] adjuvanted with Veh, MPLA, 2D216, and MPLA/2D216 on day 0 and were intranasally challenged with homologous influenza virus (H1N1)pdm09 on day 21 (Figure 6A). The combination of MPLA/2D216 significantly suppressed body weight loss after the virus challenge (Figure 6B). While MPLA improved the survival of mice from the viral challenge compared to the mice immunized with vehicle adjuvanted IIAV, 2D216 in combination with MPLA had the greatest potency in protecting the mice from the lethal virus challenge (Figure 6C). The viral neutralization titers from sera in the mice immunized with MPLA/2D216 adjuvanted IIAV were greater than those of the MPLA/Veh group, while 2D216 showed minimal effects on hemagglutinin inhibition titers (HAI) (Figure 6D). These results support that the combination of MPLA and 2D216 is a promising candidate as an effective co-adjuvant system.
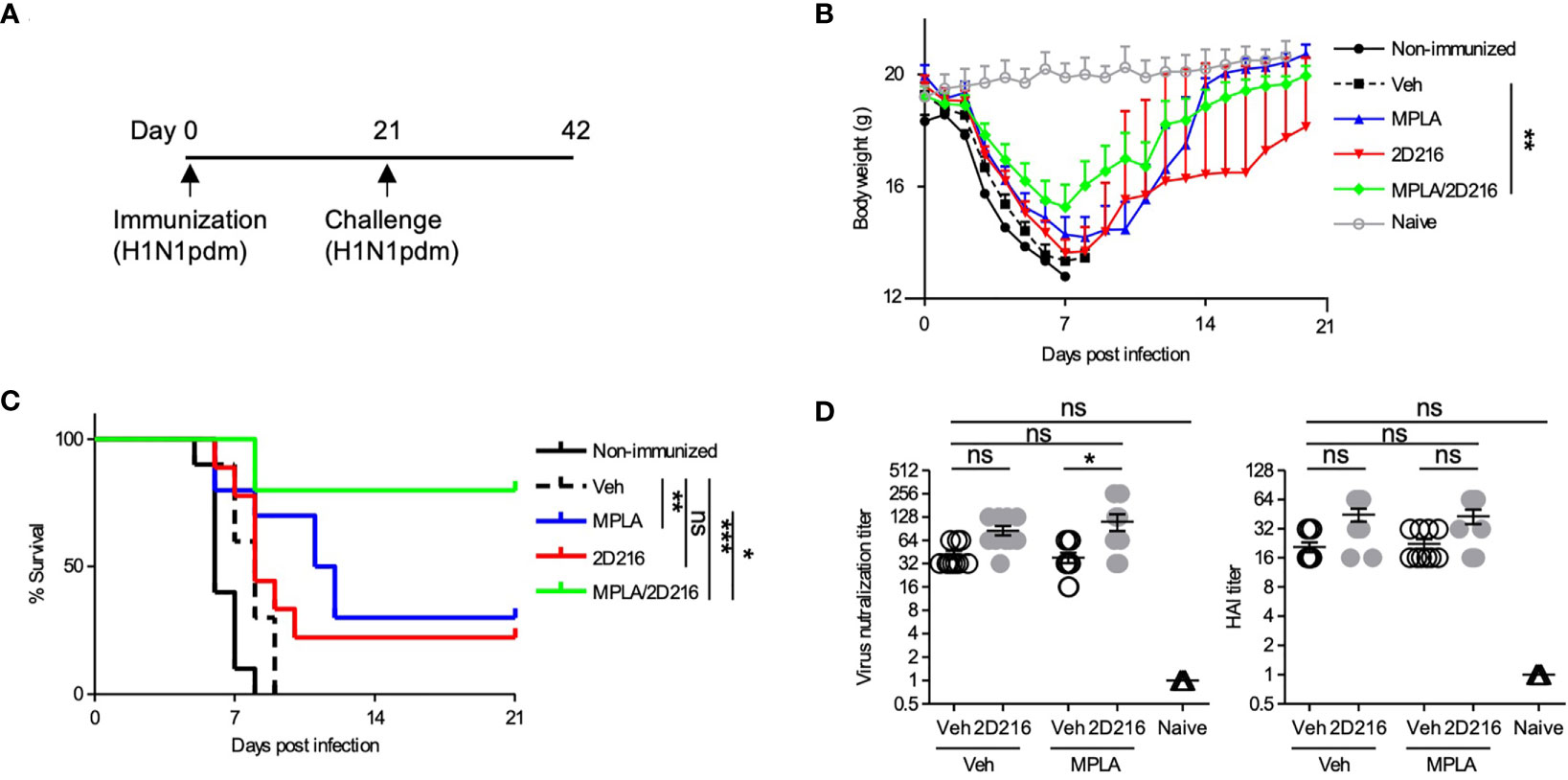
Figure 6 MPLA/2D216 adjuvanted IIAV vaccination protects mice from lethal influenza virus challenge. (A) BALB/c mice (n = 10/group) were immunized with IIAV adjuvanted with vehicle (Veh; 10%DMSO in saline), MPLA (1 μg/mouse), 2D216 (100 nmol/mouse), or MPLA/2D216 on day 0 and were infected with homologous influenza virus (H1N1)pdm09 on day 21. Body weights and survival were monitored for 21 days. (B) Body weights are indicated as mean ± SEM. For statistical analysis, last-value-carried-forward approach was used to impute missing values after a mouse died. The average weight over time was used as an outcome for comparison. *p < 0.05, **p < 0.01, ***p < 0.001 by Kruskal-Wallis tests with Dunn’s post hoc test. (C) Survival rates of mice post-challenge with homologous influenza virus. Kaplan-Meier curves with the log-rank test are shown. *p < 0.1, **p < 0.01, ***p < 0.0001, and ns, not significant. (D) The sera were collected from the mice prior to lethal challenge and tested for titer against the HA protein and viral neutralization. Data shown are means ± SEM. *p < 0.05, **p < 0.01, ***p < 0.001 by Kruskal-Wallis tests with Dunn’s post hoc test.
Discussion
Combination vaccines such as AS01B, including a TLR4 ligand, MPLA, have shown improved humoral and cellular responses and overcome reduced immune responses in the elderly for shingles and influenza (4, 7). However, undesired local and systemic reactogenicity can reduce compliance, especially if a second vaccination is required (4, 19, 20). To overcome this problem, we conducted HTS to identify non-toxic synthetic adjuvants that enhanced TLR4 ligand-mediated immune responses (14). Here we have described one of the compounds, 2D216, that enhanced immune responses triggered by TLR4 ligand MPLA in a cell type-specific manner. The combination of MPLA and 2D216 demonstrated Th1-biased humoral and cellular immune responses with less local and systemic reactogenicity and efficient protection of mice from lethal influenza challenge, suggesting that the combination adjuvant MPLA/2D216 will be a promising potent vaccine adjuvant.
Although this study demonstrated that 2D216 could augment cellular activation by TLR ligands we did not observe a dependence of the compound on the MyD88 or TRIF signaling pathways. The direct target is likely to be upstream directly proximal to or on the cell membrane. However, our studies revealed the interesting cell-type-specific action of 2D216. Concordantly, 2D216 enhanced LPS-induced activation and cytokine secretion in primary mouse CD19+ B cells, but not anti-CD3/anti-CD28 antibody-induced proliferation and cytokine secretion in primary mouse CD4+ T cells. Direct activation of B cells by vaccine adjuvants provides beneficial effects on host defense. For example, engagement of TLR7, C3d, and BAFF receptors on B cells by their ligands enhances antibody titer against influenza virus (21, 22). On the contrary, direct activation of T cells might lead to severe adverse effects such as cytokine storm (23–25). Hence, the activation of myeloid and B cells, but not T cells by 2D216, is desirable for safe vaccine adjuvants.
The OVA immunization experiment demonstrated that a combination vaccine of MPLA and 2D216 induced Th1-biased humoral and cellular immune responses. This finding was consistent with the in vitro observation that 2D216 enhanced MPLA-induced mBMDC activation such as IL-12 secretion. Inducing Th1-biased T cell responses, especially in the immune senescent elderly, is important for protection against influenza infection (26, 27). This can explain the superior protection by MPLA/2D216 combination compared to single treatment of each compound in mice against influenza challenge study. Although 2D216 alone had a minor effect on mBMDCs in vitro, it induced robust IgG1 titer in vivo. As reported for Alum and MF95 in vivo, some adjuvants induce primarily Th2 responses via multiple pathways, including depot effects, inflammasome activation, and effects on non-immune cells such as muscle cells (28–31). These pathways may contribute to Th2 responses elicited by 2D216 alone. Although these early studies are promising the addition of compound directly to MPLA in saline may have led to some inhomogeneity and microaggregation and future studies to improve the formulation may further enhance protective antibody responses.
In conclusion, we have identified and characterized the mode of action and in vivo adjuvanticity and safety of the new synthetic adjuvant 2D216. 2D216 enhanced innate immune responses induced by TLR4 ligand, MPLA. This co-adjuvant effect of 2D216 is cell type-specific, observed in myeloid and B cells but not T cells. In OVA immunization and influenza challenge studies, combination adjuvant, MPLA/2D216, induced Th1-biased humoral and cellular responses and protected mice from lethal viral challenge. MPLA/2D216 caused no local and systemic reactogenicity as compared to AS01B. Thus, 2D216 may become a promising candidate for future development as a vaccine co-adjuvant.
Data Availability Statement
The raw data supporting the conclusions of this article will be made available by the authors, without undue reservation.
Ethics Statement
Animal studies were reviewed and approved by UCSD IACUC and Utah State University IACUC.
Author Contributions
TS, DC, MCo, and TH designed the study. TS, YS, FS-K, SY, FL, THo and JS performed experiments. NS, MCh, PC, and HC synthesized the compound. TS, KM and MP performed statistical analyses. TS, DC, MC, and TH interpreted data and wrote the manuscript. All authors contributed to the article and approved the submitted version.
Funding
This study was supported by the National Institute of Health/National Institute of Allergy and Infectious Diseases under contracts HHSN272201400051C and 75N93019C00042 (Principal Investigator-DC).
Conflict of Interest
The authors declare that the research was conducted in the absence of any commercial or financial relationships that could be construed as a potential conflict of interest.
Publisher’s Note
All claims expressed in this article are solely those of the authors and do not necessarily represent those of their affiliated organizations, or those of the publisher, the editors and the reviewers. Any product that may be evaluated in this article, or claim that may be made by its manufacturer, is not guaranteed or endorsed by the publisher.
Supplementary Material
The Supplementary Material for this article can be found online at: https://www.frontiersin.org/articles/10.3389/fimmu.2021.701445/full#supplementary-material
References
1. Coffman RL, Sher A, Seder RA. Vaccine Adjuvants: Putting Innate Immunity to Work. Immunity (2010) 33:492–503. doi: 10.1016/j.immuni.2010.10.002
2. Tong NK, Beran J, Kee SA, Miguel JL, Sanchez C, Bayas JM, et al. Immunogenicity and Safety of an Adjuvanted Hepatitis B Vaccine in Pre-Hemodialysis and Hemodialysis Patients. Kidney Int (2005) 68:2298–303. doi: 10.1111/j.1523-1755.2005.00689.x
3. Del Giudice G, Rappuoli R, Didierlaurent AM. Correlates of Adjuvanticity: A Review on Adjuvants in Licensed Vaccines. Semin Immunol (2018) 39:14–21. doi: 10.1016/j.smim.2018.05.001
4. Laupeze B, Herve C, Di Pasquale A, Tavares Da Silva F. Adjuvant Systems for Vaccines: 13years of Post-Licensure Experience in Diverse Populations Have Progressed the Way Adjuvanted Vaccine Safety Is Investigated and Understood. Vaccine (2019) 37:5670–80. doi: 10.1016/j.vaccine.2019.07.098
5. Shi S, Zhu H, Xia X, Liang Z, Ma X, Sun B. Vaccine Adjuvants: Understanding the Structure and Mechanism of Adjuvanticity. Vaccine (2019) 37:3167–78. doi: 10.1016/j.vaccine.2019.04.055
6. Allen JC, Toapanta FR, Chen W, Tennant SM. Understanding Immunosenescence and Its Impact on Vaccination of Older Adults. Vaccine (2020) 38:8264–72. doi: 10.1016/j.vaccine.2020.11.002
7. Ciabattini A, Nardini C, Santoro F, Garagnani P, Franceschi C, Medaglini D. Vaccination in the Elderly: The Challenge of Immune Changes With Aging. Semin Immunol (2018) 40:83–94. doi: 10.1016/j.smim.2018.10.010
8. Cunningham AL, Lal H, Kovac M, Chlibek R, Hwang SJ, Diez-Domingo J, et al. Efficacy of the Herpes Zoster Subunit Vaccine in Adults 70 Years of Age or Older. N Engl J Med (2016) 375:1019–32. doi: 10.1056/NEJMoa1603800
9. Lal H, Poder A, Campora L, Geeraerts B, Oostvogels L, Vanden Abeele C, et al. Immunogenicity, Reactogenicity and Safety of 2 Doses of an Adjuvanted Herpes Zoster Subunit Vaccine Administered 2, 6 or 12 Months Apart in Older Adults: Results of a Phase III, Randomized, Open-Label, Multicenter Study. Vaccine (2018) 36:148–54. doi: 10.1016/j.vaccine.2017.11.019
10. Paavonen J, Naud P, Salmeron J, Wheeler CM, Chow SN, Apter D, et al. Efficacy of Human Papillomavirus (HPV)-16/18 AS04-Adjuvanted Vaccine Against Cervical Infection and Precancer Caused by Oncogenic HPV Types (PATRICIA): Final Analysis of a Double-Blind, Randomised Study in Young Women. Lancet (2009) 374:301–14. doi: 10.1016/S0140-6736(09)61248-4
11. Knudsen NP, Olsen A, Buonsanti C, Follmann F, Zhang Y, Coler RN, et al. Different Human Vaccine Adjuvants Promote Distinct Antigen-Independent Immunological Signatures Tailored to Different Pathogens. Sci Rep (2016) 6:19570. doi: 10.1038/srep19570
12. Pedersen GK, Andersen P, Christensen D. Immunocorrelates of CAF Family Adjuvants. Semin Immunol (2018) 39:4–13. doi: 10.1016/j.smim.2018.10.003
13. Kawai T, Akira S. Signaling to NF-kappaB by Toll-Like Receptors. Trends Mol Med (2007) 13:460–9. doi: 10.1016/j.molmed.2007.09.002
14. Chan M, Ahmadi A, Yao S, Sato-Kaneko F, Messer K, Pu M, et al. Identification of Biologically Active Pyrimido[5,4-B]Indoles That Prolong NF-kappaB Activation Without Intrinsic Activity. ACS Comb Sci (2017) 19:533–43. doi: 10.1021/acscombsci.7b00080
15. Datta SK, Redecke V, Prilliman KR, Takabayashi K, Corr M, Tallant T, et al. A Subset of Toll-Like Receptor Ligands Induces Cross-Presentation by Bone Marrow-Derived Dendritic Cells. J Immunol (2003) 170:4102–10. doi: 10.4049/jimmunol.170.8.4102
16. Chan M, Hayashi T, Kuy CS, Gray CS, Wu CC, Corr M, et al. Synthesis and Immunological Characterization of Toll-Like Receptor 7 Agonistic Conjugates. Bioconjug Chem (2009) 20:1194–200. doi: 10.1021/bc900054q
17. Sato-Kaneko F, Yao S, Lao FS, Shpigelman J, Messer K, Pu M, et al. A Novel Synthetic Dual Agonistic Liposomal TLR4/7 Adjuvant Promotes Broad Immune Responses in an Influenza Vaccine With Minimal Reactogenicity. Front Immunol (2020) 11:1207. doi: 10.3389/fimmu.2020.01207
18. Linterman MA, Hill DL. Can Follicular Helper T Cells be Targeted to Improve Vaccine Efficacy? F1000Res (2016) 5. doi: 10.12688/f1000research.7388.1
19. Herve C, Laupeze B, Del Giudice G, Didierlaurent AM, Tavares Da Silva F. The How’s and What’s of Vaccine Reactogenicity. NPJ Vaccines (2019) 4:39. doi: 10.1038/s41541-019-0132-6
20. Lecrenier N, Beukelaers P, Colindres R, Curran D, De Kesel C, De Saegher JP, et al. Development of Adjuvanted Recombinant Zoster Vaccine and Its Implications for Shingles Prevention. Expert Rev Vaccines (2018) 17:619–34. doi: 10.1080/14760584.2018.1495565
21. Sicard T, Kassardjian A, Julien JP. B Cell Targeting by Molecular Adjuvants for Enhanced Immunogenicity. Expert Rev Vaccines (2020) 19:1023–39. doi: 10.1080/14760584.2020.1857736
22. Vo HTM, Baudner BC, Sammicheli S, Iannacone M, D’Oro U, Piccioli D. Alum/Toll-Like Receptor 7 Adjuvant Enhances the Expansion of Memory B Cell Compartment Within the Draining Lymph Node. Front Immunol (2018) 9:641. doi: 10.3389/fimmu.2018.00641
23. Fajgenbaum DC, June CH. Cytokine Storm. N Engl J Med (2020) 383:2255–73. doi: 10.1056/NEJMra2026131
24. Suntharalingam G, Perry MR, Ward S, Brett SJ, Castello-Cortes A, Brunner MD, et al. Cytokine Storm in a Phase 1 Trial of the Anti-CD28 Monoclonal Antibody TGN1412. N Engl J Med (2006) 355:1018–28. doi: 10.1056/NEJMoa063842
25. Topp MS, Gokbuget N, Stein AS, Zugmaier G, O’Brien S, Bargou RC, et al. Safety and Activity of Blinatumomab for Adult Patients With Relapsed or Refractory B-Precursor Acute Lymphoblastic Leukaemia: A Multicentre, Single-Arm, Phase 2 Study. Lancet Oncol (2015) 16:57–66. doi: 10.1016/S1470-2045(14)71170-2
26. La Gruta NL, Turner SJ. T Cell Mediated Immunity to Influenza: Mechanisms of Viral Control. Trends Immunol (2014) 35:396–402. doi: 10.1016/j.it.2014.06.004
27. McElhaney JE, Kuchel GA, Zhou X, Swain SL. And Haynes L. T-Cell Immunity to Influenza in Older Adults: A Pathophysiological Framework for Development of More Effective Vaccines. Front Immunol (2016) 7:41. doi: 10.3389/fimmu.2016.00041
28. Awate S, Babiuk LA, Mutwiri G. Mechanisms of Action of Adjuvants. Front Immunol (2013) 4:114. doi: 10.3389/fimmu.2013.00114
29. Glenny AT, Buttle GAH, Stevens MF. Rate of Disappearance of Diphtheria Toxoid Injected Into Rabbits and Guinea - Pigs: Toxoid Precipitated With Alum. J Pathol Bacteriol (1931) 34:267–75. doi: 10.1002/path.1700340214
30. Li H, Willingham SB, Ting JP, Re F. Cutting Edge: Inflammasome Activation by Alum and Alum’s Adjuvant Effect Are Mediated by NLRP3. J Immunol (2008) 181:17–21. doi: 10.4049/jimmunol.181.1.17
Keywords: vaccine, adjuvant, influenza virus, Toll-like receptor4, monophosphoryl lipid A, NF-κB
Citation: Saito T, Sako Y, Sato-Kaneko F, Hosoya T, Yao S, Lao FS, Shpigelman J, Messer K, Pu M, Shukla NM, Chan M, Chu PJ, Cottam HB, Hayashi T, Carson DA and Corr M (2021) Small Molecule Potentiator of Adjuvant Activity Enhancing Survival to Influenza Viral Challenge. Front. Immunol. 12:701445. doi: 10.3389/fimmu.2021.701445
Received: 28 April 2021; Accepted: 30 August 2021;
Published: 28 September 2021.
Edited by:
Julia Kzhyshkowska, Heidelberg University, GermanyReviewed by:
Francesco Peri, University of Milano-Bicocca, ItalyVyacheslav Ryabov, Russian Academy of Sciences, Russia
Copyright © 2021 Saito, Sako, Sato-Kaneko, Hosoya, Yao, Lao, Shpigelman, Messer, Pu, Shukla, Chan, Chu, Cottam, Hayashi, Carson and Corr. This is an open-access article distributed under the terms of the Creative Commons Attribution License (CC BY). The use, distribution or reproduction in other forums is permitted, provided the original author(s) and the copyright owner(s) are credited and that the original publication in this journal is cited, in accordance with accepted academic practice. No use, distribution or reproduction is permitted which does not comply with these terms.
*Correspondence: Dennis A. Carson, ZGNhcnNvbkBoZWFsdGgudWNzZC5lZHU=; Maripat Corr, bXBjb3JyQGhlYWx0aC51Y3NkLmVkdQ==