- 1Department of Medicine, Mekelle University College of Health Sciences, Mekelle, Ethiopia
- 2Department of Global Health, Kenyan Medical Research Institute (KEMRI) – Wellcome Research Programme, Nairobi, Kenya
- 3Amsterdam Institute of Global Health and Development, Department of Global Health, Amsterdam University, Amsterdam, Netherlands
- 4Joep-Lange Institute, Amsterdam, Netherlands
Chronic immune activation has been considered as the driving force for CD4+ T cell depletion in people infected with HIV-1. Interestingly, the normal immune profile of adult HIV-negative individuals living in Africa also exhibit chronic immune activation, reminiscent of that observed in HIV-1 infected individuals. It is characterized by increased levels of soluble immune activation markers, such as the cytokines interleukin (IL)-4, IL-10, TNF-α, and cellular activation markers including HLA-DR, CD-38, CCR5, coupled with reduced naïve and increased memory cells in CD4+ and CD8+ subsets. In addition, it is accompanied by low CD4+ T cell counts when compared to Europeans. There is also evidence that mononuclear cells from African infants secrete less innate cytokines than South and North Americans and Europeans in vitro. Chronic immune activation in Africans is linked to environmental factors such as parasitic infections and could be responsible for previously observed immune hypo-responsiveness to infections and vaccines. It is unclear whether the immunogenicity and effectiveness of anti-SARS-CoV-2 vaccines will also be reduced by similar mechanisms. A review of studies investigating this phenomenon is urgently required as they should inform the design and delivery for vaccines to be used in African populations.
Introduction
CD4+ T cells play a pivotal role in the regulation of the immune system, protecting the host against various pathogens and autoimmunity (1). CD4+ T cells modulate the immune response by orchestrating responses of B-cells, CD8+ T cells and other components of the immune system. To do so, CD4+ T cells, also designated as T-helper (TH) cells, branch into five major subsets: TH1, TH2, TH17, follicular T helper (TFH) and regulatory T cells (Treg) CD4+ T cells (1). These different subsets of TH cells are differentiated by the expression of different lineage markers and transcription factors as well as the production of different cytokines. TH1 subsets express transcription factor T-bet, produce interferon (IFN)-γ, and they exert effector function against intracellular microorganisms, such as mycobacteria and viruses; TH2 subsets express transcription factor GATA-3, produce the cytokines interleukin (IL)-4, IL-5, IL-6, IL-13, and they exert effector function against extracellular organisms, such as helminths; TH17 subsets express transcription factor RORγT, produce IL-17, as well as IL-22, and they exert effector function against intracellular organisms, such as bacteria and fungi; TFH CD4+ T cells express transcription factor Bcl6, produce IL-21 and their effector function is to help B-cells produce immunoglobulins; Tregs express FoxP3 transcription factor, produce the cytokines IL-10 and transformation growth factor(TGF)-β, and their effector functions include immune homeostasis (1). Tregs propitiate an adequate immune response by helping in the recruitment of T cells, facilitating the removal of pathogens and preventing excessive tissue damage; hence they are critical in the prevention of autoimmunity and other forms of immune dysregulation (2). The differentiation of CD4+ T cells into the various subsets described above is highly dependent on the prevailing cytokine environment (1). Notably, dysregulation in CD4+ T cell immune responses may result in failure to protect the host from an infection or can lead to autoimmunity.
Previous reports showed that chronic immune activation is linked to the pathogenesis of CD4+ T cell lymphopenia in patients with HIV-1 infection (3). Moreover, the background immune profile of adult HIV-negative individuals living in Africa demonstrate a phenomenon of chronic immune activation, reminiscent of that observed in HIV-1 infected individuals. Here, we reviewed the potential influence of chronic immune activation or immune dysregulation and low CD4+ T cell count background against pathogens and vaccines on individuals residing in Africa. In addition, we discuss the potential role of altered immune responses that may impact on SARS-CoV-2 vaccine efficacy.
Chronic Immune Dysregulation in Africans
Systemic persistent immune activation has been considered as the driving force of CD4+ T cell depletion in people infected with HIV-1 (3). More importantly, effective antiretroviral therapy (ART) and suppressed HIV-1 viremia, appears not to reduce the accompanying chronic immune activation in these patients (3). One of the mechanisms leading to systemic chronic immune activation in HIV-1 infected patients has been ascribed to HIV-driven dysbiosis of the gut microbiota and microbial translocation into the blood stream (4–7). Interestingly, the immune profile of adult HIV-negative individuals living in Africa exhibit chronic immune activation, reminiscent of that observed in HIV-1 infected individuals (8–17). In addition, chronic immune activation in these apparently healthy individuals of African origin is accompanied by significantly lower CD4+ T cell counts and CD4/CD8 ratio when compared to Europeans (8–23).
Such a skewed immune background profile of the African population may have a “double-edged sword” outcomes when the host encounters another pathogen or when administered a vaccine. Whereas chronic immune activation with skewed TH2 and Treg background may increase risk of infections, in particular for responses that depend on TH1 immune responses, it may provide protection against chronic inflammatory conditions, such as allergy or autoimmunity. In the same token, chronic immune activation may reduce the potential efficacy of vaccines, including those targeted against SARS-CoV-2 infection. The immune profile of apparently healthy and HIV-negative Africans is characterized by increased levels of soluble and cellular markers for chronic immune activation. Soluble immune activation markers consisted of elevated levels of IgE, IgG, placental isoferritin and p75 soluble tumor necrosis factor (TNF) receptor (8). In addition to increased eosinophilia, the expressions of HLA-DR, CD38, CD28, CCR5, Ki67 in CD4+ as well as CD8+ T cell subsets among Africans is significantly higher when compared to Europeans (8–17). Reduction in CD4+ and CD8+ naïve T cells, whereas increase in CD4+ and CD8+ memory T cells, as well as increase in CCR5 expression were observed in these population. The background profile is also characterized by predominant TH2 and Treg immune response (9), and marked increase in lymphocyte apoptosis (10). In addition, chronic immune activation in apparently healthy individuals residing in Africa is accompanied by CD4+ T cell counts that are significantly lower when compared to Europeans (8–23). For example, absolute CD4+ T cell count among adult healthy Ethiopians is around 700 cells/µL on average as compared to around 1,100 cells/µL among the Dutch population or Israelis (9–17).
Underlying Causes of Immune Dysregulation in Africans
The reason for observed chronic immune activation, low CD4+ T cell count and inverted CD4/CD8 ratio in the African population remains poorly understood. Nonetheless, we noted that it is not genetic in origin, but something that is acquired during life (9, 15, 17). Earlier, we studied changes in T-cell receptor excision circles (TRECs), HLA-DR and CD31 expression on CD4+ T cells and T-cell telomere lengths with age in healthy Ethiopian and Dutch individuals. At birth, CD4+ naïve T cell numbers and TREC contents in Ethiopians were found to be comparable to those in Dutch neonates (17). At very young age, however, both CD4+ naïve T cell numbers and TREC contents fell dramatically in Ethiopians, but not in Dutch children. These differences between Ethiopian and Dutch individuals remained persistently into adulthood, because CD4+ naïve T cell numbers and TREC contents decreased at similar rates with age in Ethiopian and Dutch individuals (13, 17). In addition, telomere lengths in Ethiopian individuals tended to be shorter than the Dutch counterparts (17). Though the changes we observed in CD4+ T cell counts and their activation status occurred at earlier ages in life, as early as five years, adolescence and middle-aged adults, these changes appear to be reminiscent of immunosenescence (24). This state of dysregulated immune response associated with older age later during life is characterized by a chronic low-level inflammation (also known as “inflammaging”) and a decline of the immune system function (24).
These findings showing that immune activation increased with increasing age strengthen the notion that that external/environmental factors play significant role in inducing chronic immune activation (9). In addition, Ethiopian migrants to Israel who stayed longer period of time (> 5 years) indeed exhibited reversal in terms of increased CD4+ T cell counts and CD4/CD8 ratio as well as reduction in chronic immune activation (8, 10). Increased activation-induced lymphocyte apoptosis could be also one of the underlying mechanisms leading to the observed low CD4+ T cell among the Ethiopian population (10). In addition, tuberculosis (TB) patients have been shown to exhibit T cell immune activation (25), and hence latent TB, highly prevalent in Africa (26), may drive immune activation in this population. The role of viral infections, such as hepatitis C virus (HCV), cytomegalovirus and Epstein-Barr virus, in inducing immune activation in Africans remains unknown.
Among the environmental factors that drive immune activation in Africa is infection by parasites, in particular helminths. An estimated 2 billion of the world’s population is infected with helminth parasites (27). Chronic helminthic infections are often associated with the development of TH2-biased and alternatively activated macrophages (M2) and type 2 innate lymphoid cells (2, 28). These responses are accompanied with the induction of cytokines such as IL-4, IL-5, IL-13, and increased eosinophilia, IgE as well as goblet cell hyperplasia. Whereas the TH2-skewed immune responses are important in controlling helminthic infections, they are also considered to play an important role in the repair of tissue damage as a result of helminth infections (2, 28). Besides inducing TH2 immune responses, helminths also induce a strong regulatory networks, characterized by the induction of Tregs (2, 28). The induction of Tregs enhances survival and persistence of helminths within their host, and concomitantly may affect responses to heterologous infection, antigen, or vaccine. Indeed, several reports have demonstrated that infection by intestinal helminth is associated with chronic immune activation (8, 10, 12, 16, 29, 30). Interestingly, deworming resulted in reversal of immune activation, characterized by decreased eosinophilia, reduced expressions of HLA-DR, increased naïve cells and reduced memory cells (8, 10, 12, 16). Similar to infection with HIV-1, helminths also can cause microbial translocation (31–33), that may eventually lead to systemic chronic immune activation. Furthermore, profound CD4+ T lymphopenia and immune activation in the absence of HIV-1 infection among patients with malaria has been demonstrated (34, 35). The more severe form of P. falciparum resulted in significantly higher CD4+ T cell reduction or immune activation than the milder form of P. vivax (34, 35). Given the fact that CD4+ T cell count was determined from the peripheral blood, however, we assume that this was due to lymphocyte redistribution rather than direct CD4+ T cell death. We assume that macrophages engulf red blood cells laden with malarial parasites and migrate to the spleen or liver and TH1 cells are then redistributed from the periphery to these organs to activate the intracellular killing mechanism of the macrophage.
In addition to helminths, socio-demographic factors may influence CD4+ T cell counts. CD4+ T cell counts were independently and positively associated with female gender, cigarette smoking and khat (Catha Edulis) chewing (21). However, there is paucity of data with regard to the effect of diet on CD4+ T cell count differences among populations. We and others reported previously that body mass index was positively correlated with CD4+ T cell counts (21, 36). In addition, HIV-negative children with malnutrition from Uganda exhibited lower CD4% when compared to non-malnourished children (37). Increased exposure to ultraviolet light during summer has been reported to decrease CD4+ T cell counts among Europeans (38, 39). Overall, differences in CD4+ T cell counts between populations may be attributed to differences in diet, genetic, ethnicity, gender, residence, altitude, methods used to enumerate the cells and other yet unidentified factors.
Effects of Chronic Immune Activation on Immune Response to Other Infections or Vaccines
Systemic chronic immune activation may potentially be detrimental to the host. Notably, T cell hyporesponsiveness induced by helminths may result in increased susceptibility to infections, or reduced responses to vaccines (Table 1). Several studies have demonstrated that co-infection with helminths is associated with increased susceptibility to heterologous infections. For example, concomitant infection with helminths in patients with TB results in a plethora of immune responses with unfavorable clinical outcomes (40–42). Helminths-driven immune modulation in these conditions included reduced CD3+, CD4+, CD8+, natural killer (NK), CD4+CD25high and IFN-γ responses, but increased eosinophilia, Tregs, IL-4, IL-5 and IL-10 responses. Notably, individuals with latent TB infection co-infected with helminths had lower frequency of CD4+IFN-γ+ T cells and increased in CD4+FoxP3+ T cells (Tregs) compared to those without helminth co-infection (43). Although protective immunity against TB is considered to be dependent on cellular immune responses, mycobacteria-specific humoral immune responses have been proposed to play an important role in protection. However, co-infection with Strongloides stercoralis has been shown to reduce mycobacteria-specific B-cell responses (44). Unfavorable consequences include increased susceptibility to TB, persistence in Mycobacterium tuberculosis as well as more protracted TB disease course. In addition, studies conducting in experimental animal models demonstrated that helminth co-infection induced expression of arginase-1 by macrophages within the lung tissue resulting in enhanced inflammation and disease severity (45).
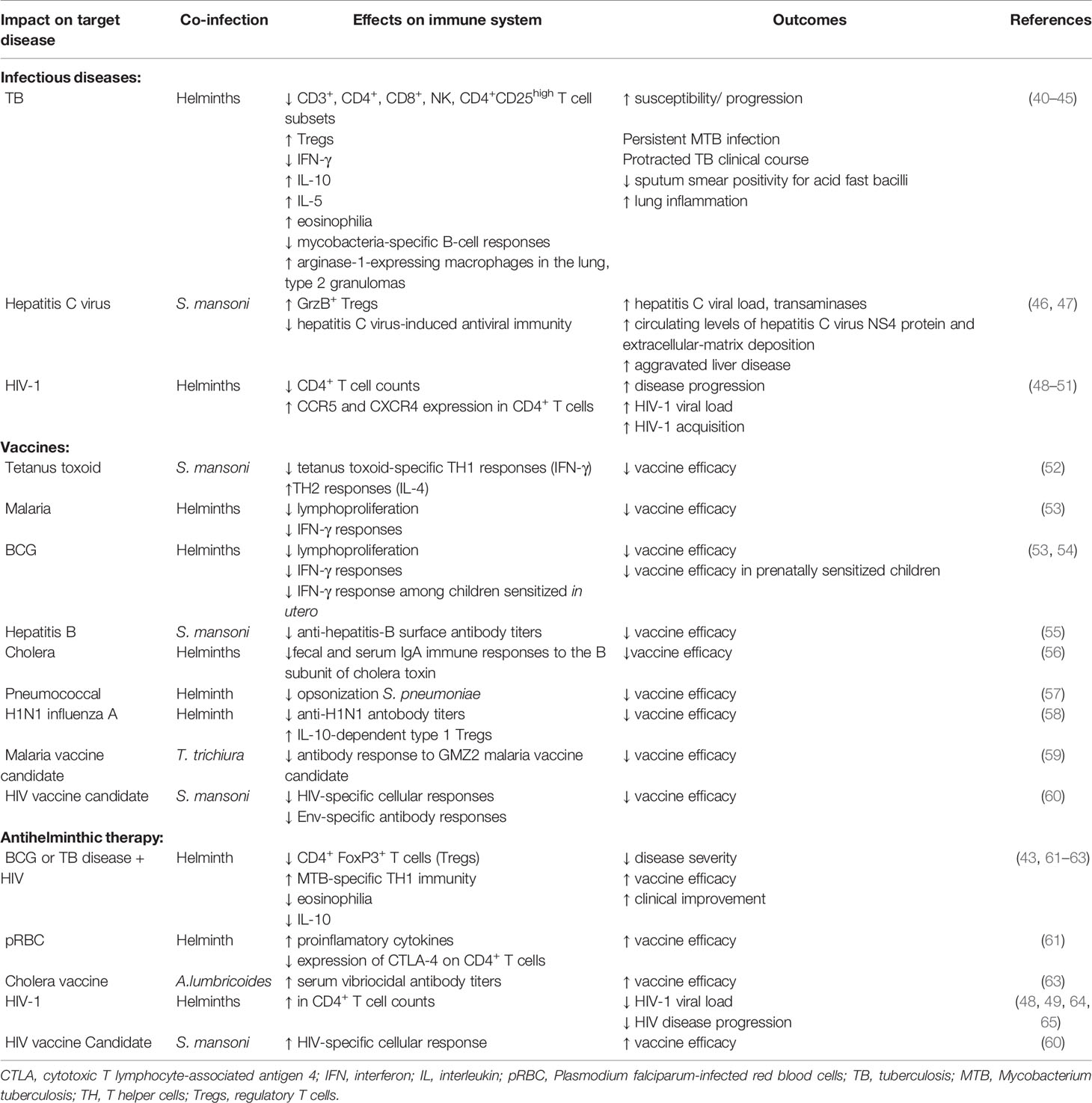
Table 1 Summary of the helminth-induced hyporesponsive immune responses to heterologous infections and vaccines.
Several reports have also showed that co-infection with helminths can attenuate immune response to important viruses with negative outcomes. For example, HCV patients co-infected with the helminth Schistosoma mansoni showed significantly increased GrzB+ Treg response, indicating reduced HCV-induced TH1 and attenuated antiviral immunity (46, 47). In these patients, helminth co-infection led to aggravated HCV-related liver disease characterized by significantly elevated HCV load and transaminases when compared to patients infected with HCV only. Previous studies undertaken by us and others demonstrated that co-infection with helminths correlated with much lower CD4+ T cell counts and significantly higher HIV-1 viral load compared to those without helminth coinfection (48–50), and plasma HIV-1 viral load strongly correlated to the intensity of helminth infection (48). In addition, a recent systemic review reported that co-infection with schistosomes increased the risk of HIV-1 acquisition by 4-fold, through mechanisms involving increased expression of CCR5 and CXCR4 HIV-1 co-receptors on CD4+ T cells and cervical mucosa lesions (51).
Likewise, several earlier reports showed that helminth-induced chronic immune activation leads to a significant negative effect on vaccine efficacy. Whereas tetanus toxoid-specific TH1 (IFN-γ) immune responses were significantly attenuated in individuals with S. mansoni infection, TH2 (IL-2) responses were significantly increased (52). Children infected with helminths showed significant reduction in lymphoproliferative responses to Plasmodium falciparum-infected red blood cells (pRBC) and BCG compared to those without helminths (53). Notably, both lymphoproliferative and IFN-γ responses were significantly increased upon CD4+CD425high T cell depletion, indicating that Tregs modulate helminth-driven immune responses to mycobacterial and malarial antigens. Children infected with the helminth S. mansoni when given hepatitis B vaccine showed significantly reduced hepatitis B virus surface antibody (anti-HBs) titers compared to helminth uninfected children (55). Similarly, patients with cholera co-infected with helminths had reduced fecal and serum IgA immune responses to the B subunit of cholera toxin (CTB) when compared to those without helminth co-infection (56). Interestingly, prenatal sensitization in utero has also been reported to reduce vaccine efficacy. Children of helminth-infected mothers showed significantly lower IFN-γ responses to the mycobacterial antigen – purified protein derivative (PPD) following vaccination with BCG compared to children of helminth uninfected mothers (54). Similarly, other investigators also demonstrated using animal experiments that helminths attenuated vaccine efficacy. Administration of pneumococcal vaccine to mice chronically infected with helminths was impaired due to failure to opsonize effectively S. pneumonia for killing by alveolar macrophages (57). In another recent study, helminth infection was reported to suppress the efficacy of vaccination against seasonal influenza (58). Helminth-infected mice had reduced quantity and neutralizing quality of antibody responses following vaccination with H1N1 influenza A virus, and attenuated vaccine efficacy was accompanied with increased levels of IL-10-dependent type 1 Tregs. Other investigators have reported similar reduced responses to vaccine candidates among individuals infected with helminths. A study (59) demonstrated that antibody responses to a blood stage malaria vaccine candidate GMZ2 was significantly lower in Trichuris trichiura infected children when compared to the antibody responses among parasite negative controls. Using an animal experimental model (60), it was shown that S. mansoni infected mice had significantly lower HIV-1-specific immune responses after prime-boost vaccination with DNA+MVA, or MVA+gp120 compared to uninfected control mice. In addition, gp140 Env-specific antibody responses were significantly in S. mansoni infected mice compared to controls.
Notably, anthelminthic treatment led to improved immune responses. For example, there was a decrease in the frequency of Treg cells accompanied with increased CD4+IFN-γ+ T cells after anthelmintic treatment of individuals with latent TB infection (43). In addition, cell-mediated immune responses to mycobacterial antigens following BCG immunization improved significantly among those who received anthelminthic therapy before vaccination when compared to controls (61–63). Deworming also can lead to improved antimalarial immune responses (61). Similarly, albendazole treatment of children infected with ascariasis enhanced the vibriocidal antibody response to live attenuated oral cholera vaccine (66). In addition, deworming resulted in increased CD4+ T cell counts, or reduced viral load in HIV-infected patients (48, 49, 64, 65), and increased HIV-1-specific cellular immune responses to HIV-1 vaccine candidate (60).
Exposure to specific microbial antigens has been shown to induce a sustained epigenetic alteration in innate immunity [reviewed in (67)]. Such alterations result in an enhanced immune response to a repeat challenge by the same antigen, or to heterologous one. This phenomenon is termed as “trained immunity”. The concept of trained immunity was originally discovered in studies involving BCG vaccine (67). The studies have provided evidence that BCG-induced trained immunity can protect against multitude of diseases, including respiratory infections and cancer. The concept of trained immunity is, however, in contrast to helminth-induced hyporesponsiveness described in the earlier sections of this review.
Chronic Immune Activation in Africans and COVID-19
COVID-19 patients, like those infected with HIV-1, exhibit also lymphopenia (68). Will low baseline CD4+ T cell counts among African individuals increase risk of COVID-19 severity? Though this remains to be elucidated, we hypothesize that this is not the case. We base this on the observations we made showing that 74% of all SARS-CoV-2 infections are asymptomatic and only 4.4% present with severe COVID-19 (69). Furthermore, parasites indeed appear to protect against severe COVID-19 (70), by counteracting against excessive TH1-induced hyperinflammation (71).
Similarly, despite low baseline CD4+ T cell counts and background chronic immune activation, earlier studies we conducted in Ethiopia showed that disease progression among HIV-1 infected patients was not accelerated (72). Indeed, it was similar when compared to their counterparts from Dutch who had relatively higher baseline CD4+ T cell count and very little immune activation. The rate of average CD4+ T cell count decline, in the absence of antiretroviral therapy, was around 36 and 66 cells/year in the Ethiopians and the Dutch, respectively (72). The findings suggested that the rate of CD4+ T cell decline was the most important factor associated with disease progression rather than baseline CD4+ T cell count. The proportion of proliferating Ki67+ cells within the naïve and memory CD4+ T cell subsets were lower in HIV-infected Ethiopians compared to Dutch HIV-infected patients matched for CD4+ T-cell count (17). Thus, the slower CD4+ T cell decline in HIV-infected Ethiopians might be explained by lower levels of proliferation.
Taken these observations, the important question is whether underlying chronic immune activation among Africans will impact on vaccine efficacies for SARS-CoV-2. Response to existing vaccines, such as BCG, yellow fever, rotavirus, polio, tetanus, influenza (73–76), as well as to candidate vaccines, such as for TB, malaria, Ebola and HIV-1, are lower when compared to responses in individuals in the northern hemisphere (77–80). Though the lower responses exhibited to the vaccines in the LMICs was observed irrespective of helminth status in the countries investigated, it is considered that co-infection with helminths is highly significant (81–83). Therefore, it is tempting to speculate that we might see similar reduced vaccine efficacies for SARS-COV-2 in LMICs (Figure 1). This notion is supported by a study that revealed Gabonese children living in rural areas with high incidence of helminth infection exhibited reduced anti-H1N1 and anti-B responses to a seasonal influenza vaccine compared to children living in semi-urban areas with lower incidence for helminth infection (83). Moreover, reduced vaccine efficacy has already been reported for various SARS-CoV-2 vaccines against the ‘South African variant’, B.1.351 (84). This reduction in efficacy was attributed to the specific B.1.351 mutations, particularly in the Spike-protein. However, the alternative explanation could be that the immune system of South African vaccine trial participants is skewed towards TH2, hyperactivated with low CD4+ T cells and therefore show less stimulation by SARS-CoV-2 vaccines.
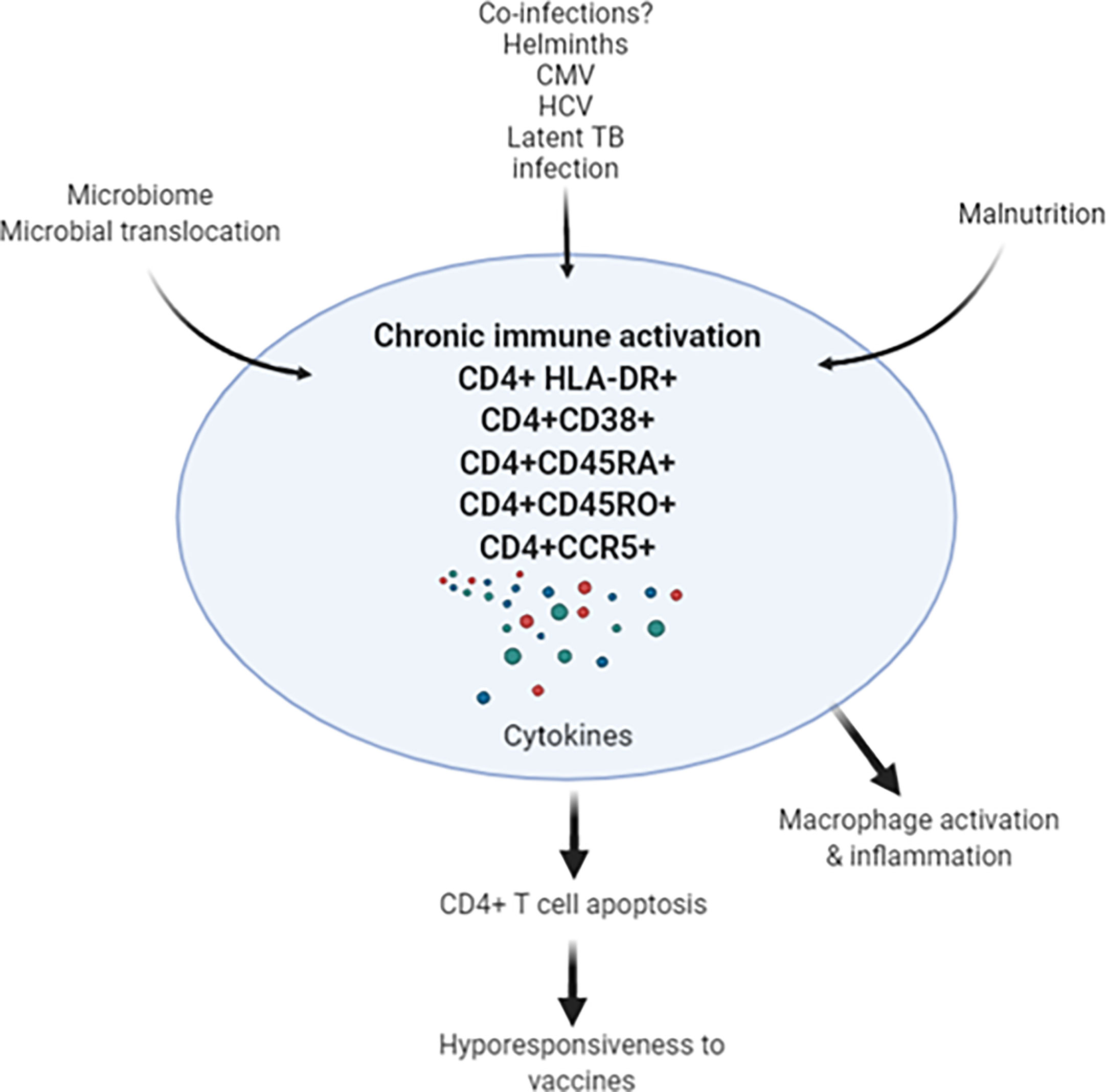
Figure 1 Chronic immune activation in apparently health Africans may be related to leaky gut, co-infections (such as helminths, CMV, HCV and latent TB infection) results in activation-induced CD4+ T cell apoptosis. In turn, depletion of CD4+ T cells may result in decreased response to SARS-CoV-2 vaccine in the African population. CMV, cytomegalovirus; HCV, hepatitis C virus; TB, tuberculosis.
In conclusion, studies are needed to evaluate the various factors that could potentially determine a reduced SARS-CoV-2 vaccine efficacy in Africa (85). These include both the effects of underlying immune activation status as described above as well as the influence of certain SARS-CoV-2 mutations. The current COVAX efforts in Africa will provide ample opportunities to evaluate these factors and findings should feed into COVAX vaccine distribution policies. Despite growing vaccine hesitancy in Africa, the roll-out of COVAX remains decisively important to avoid a situation of many years of persistence of significant SARS-CoV-2 outbreaks on the continent. Such events, particularly in immunocompromised individuals can lead to the emergence of new variants that could spread elsewhere in the world. The first evidence of such events is accumulating with a variant from Central Africa transmitting into Europe (86).
For all of the above reasons, we recommend that roll-out of COVAX and its potential reduced effectiveness due to different immune system backgrounds is proactively accompanied thorough information campaigns that involve African communities (87). Such campaigns should put into perspective the negative connotations that could be associated with the potential finding of generally reduced SARS-CoV-2 vaccine efficacy in African populations. Finally, we strongly recommend that highly prevalent infections, as the ones discussed in this manuscript, should be controlled for in future vaccine trials, not only for SARS-CoV-2 infection, to assess the real magnitude of the impact of these conditions on vaccine efficacy, to guide the design of African-tailored vaccines if needed. Eventually, an approach could emerge that involves the message: first deworm, then vaccinate.
Author Contributions
DW and TR conceived the idea and drafted the review. All authors contributed to the article and approved the submitted version.
Funding
This work was supported by the European and Developing Countries Clinical Trials Partnership (EDCTP) - European Commission (Project ID: RIA2020EF-2905), The Hague, The Netherlands and the Joep Lange Institute for Global Health and Development, Amsterdam, The Netherlands.
Conflict of Interest
The authors declare that the research was conducted in the absence of any commercial or financial relationships that could be construed as a potential conflict of interest.
References
1. Zhu X, Zhu J. Cd4 T Helper Cell Subsets and Related Human Immunological Disorders. Int J Mol Sci (2020) 21:8011–37. doi: 10.3390/ijms21218011
2. White MPJ, McManus CM, Maizels RM. Regulatory T-cells in Helminth Infection: Induction, Function and Therapeutic Potential. Immunol (2020) 160:248–60. doi: 10.1111/imm.13190
3. Paiardini M, Müller-Trutwin M. HIV-Associated Chronic Immune Activation. Immunol Rev (2014) 254:78–101. doi: 10.1111/imr.12079
4. Kroeze S, Ondoa P, Kityo CM, Siwale M, Akanmu S, Wellington M, et al. Suboptimal Immune Recovery During Antiretroviral Therapy With Sustained HIV Suppression in sub-Saharan Africa. AIDS (2018) 32):1043–51. doi: 10.1097/QAD.0000000000001801
5. Dillon SM, Lee EJ, Kotter CV, Austin GL, Dong Z, Hecht DK, et al. An Altered Intestinal Mucosal Microbiome in HIV-1 Infection is Associated With Mucosal and Systemic Immune Activation and Endotoxemia. Mucosal Immunol (2014) 7:983–94. doi: 10.1038/mi.2013.116
6. Gootenberg DB, Paer JM, Luevano JM, Kwon DS. HIV-Associated Changes in the Enteric Microbial Community: Potential Role in Loss of Homeostasis and Development of Systemic Inflammation. Cur Opin Infect Dis (2017) 30:31–43. doi: 10.1097/QCO.0000000000000341
7. Brenchley JM, Price DA, Schacker TW, Asher TE, Silvestri G, Rao S, et al. Microbial Translocation is a Cause of Systemic Immune Activation in Chronic HIV Infection. Nat Med (2006) 12:1365–71. doi: 10.1038/nm1511
8. Bentwich Z, weisman Z, Moroz C, Bar-Yehuda S, Kalinkovich A. Immune Dysregulation to Ethiopian Immigrants in Israel: Relevance to Helminth Infections? Clin Exp Immunol (1996) 103:239–43. doi: 10.1046/j.1365-2249.1996.d01-612.x
9. Clerici M, Butto S, Lukwiya M, Sarasella M. Immune Activation in Africa is Environmentally-Driven and is Associated With Upregulation of CCR5. AIDS (2000) 14:2083–92. doi: 10.1097/00002030-200009290-00003
10. Kalinkovich A, Weisman Z, Greenberg Z, Nahmias J, Eitan S, Stein M, et al. Decreased CD4 and Increased CD8 Counts With T Cell Activation is Associated With Chronic Helminth Infection. Clin Exp Immunol (1998) 114:414–21. doi: 10.1046/j.1365-2249.1998.00736.x
11. Messele T, Abdulkadir M, Fontanet A, Petros B, Hamann D, Koot M, et al. Reduced Naive and Increased Activated CD4 and CD8 Cells in Healthy Adult Ethiopians Compared With Their Dutch Counterparts. Clin Exp Immunol (1999) 115:443–50. doi: 10.1046/j.1365-2249.1999.00815.x
12. Borkow G, Leng Q, Weisman Z, Stein M, Galai N, Kalinkovich A, et al. Chronic Immune Activation Associated With Intestinal Helminth Infections Results in Impaired Signal Transduction and Anergy. J Clin Invest (2000) 106:1053–60. doi: 10.1172/JCI10182
13. Hazenberg MD, Otto SA, Cohen Stuart JW, Verschuren MC, Borleffs JC, Boucher CA, et al. Increased Cell Division But Not Thymic Dysfunction Rapidly Affects the T-cell Receptor Excision Circle Content of the Naive T Cell Population in HIV-1 Infection. Nat Med (2000) 6:1036–42. doi: 10.1038/79549
14. Kassu A, Tsegaye A, Petros B, Wolday D, Roos MTL, Hamann D, et al. Distribution of Lymphocyte Subsets in Healthy HIV-negative Adult Ethiopians From Different Geographical Locales. Clin Diagn Lab Immunol (2001) 8:1171–6. doi: 10.1128/CDLI.8.6.1171-1176.2001
15. Tsegaye A, Wolday D, Otto S, Petros B, Assefa T, Alebachew T, et al. Immunophenotyping of Blood Lymphocytes at Birth, During Childhood, and During Adulthood in HIV-1-uninfected Ethiopians. Clin Immunol (2003) 109:338–46. doi: 10.1016/j.clim.2003.08.008
16. Kassu A, Tsegaye A, Wolday D, Petros B, Aklilu M, Sanders EJ, et al. Role of Incident and/or Cured Intestinal Parasitic Infections on Profile of CD4+ and CD8+ T Cell Subsets and Activation Status in HIV-infected and Uninfected Adult Ethiopians. Clin Exp Immunol (2003) 132:113–9. doi: 10.1046/j.1365-2249.2003.02106.x
17. Vrisekoop N. Immune Abnormalities in Healthy Ethiopians are Induced by Chronic Immune Activation and Start at Early Age. PhD Thesis. The Netherlands: Utrecht University (2007) p. 48–73.
18. Pollack S, Fuad B, Etzioni A. Cd4 T-lymphopenia Without Opportunistic Infections in HIV-seronegative Ethiopian Immigrants to Israel. Lancet (1993) 342:50–1. doi: 10.1016/0140-6736(93)91912-6
19. Tsegaye A, Messele T, Tilahun T, Hailu E, Sahlu T, Doorly R, et al. Immunohematological Reference Ranges for Adult Ethiopians. Clin Diag Lab Immunol (1999) 6:410–4. doi: 10.1128/CDLI.6.3.410-414.1999
20. Kassa E, Rinke de Wit TF, Hailu E, Girma M, Messele T, Mariam HG, et al. Evaluation of the World Health Organization Staging System for HIV Infection and Disease in Ethiopia: Association Between Clinical Stages and Laboratory Markers. AIDS (1999) 13:381–9. doi: 10.1097/00002030-199902250-00011
21. Abuye C, Tsegaye A, West CE, Versloot P, Sanders EJ, Wolday D, et al. Determinants of CD4 Counts Among HIV-negative Ethiopians: Role of Body Mass Index, Gender, Cigarette Smoking, Khat (Catha Edulis) Chewing, and Possibly Altitude? J Clin Immunol (2005) 25:127–33. doi: 10.1007/s10875-005-2818-y
22. Karita E, Ketter N, Price MA, Kayitenkore K, kaleebu P, Nanvubya A, et al. CLSI-Derived Hematology and Biochemistry Reference Intervals for Healthy Adults in Eastern and Southern Africa. PLoS One (2009) 4:e4401. doi: 10.1371/journal.pone.0004401
23. Gize A, Mathewos B, Moges B, Workineh M, Gedefaw L. Establishment Normal Reference Intervals for CD3+, Cd4+, CD8+ and CD4/CD8 Ratio of T Lymphocytes in HIV-negative Adults From University of Gondar Hospital, North-west Ethiopia. AIDS Res Treat (2014) 2014:267450. doi: 10.1155/2014/267450
24. Herranz N, Gil J. Mechanisms and Functions of Cellular Senescence. J Clin Invest (2018) 128:1238–46. doi: 10.1172/JCI95148
25. Vanham G, Edmonds K, Qing L, Hom D, Toossi Z, Jones B, et al. Generalized Immune Activation in Pulmonary TB: Co-Activation With HIV Infection. Clin Exp Immunol (1996) 103:30–4. doi: 10.1046/j.1365-2249.1996.907600.x
26. Tegbaru B, Wolday D, Messele T, Legesse M, Mekonnen Y, Miedema F, et al. Tuberculin Skin Test Conversion and Reactivity Rates Among Adults With and Without Human Immunodeficiency Virus in Urban Settings in Ethiopia. Clin Vaccine Immunol (2006) 13:784–9. doi: 10.1128/CVI.00098-06
27. Herricks JR, Hotez PJ, Wanga V, Coffeng LE, Haagsma JA, Basáñez M-G, et al. The Global Burden of Disease Study 2013: What Does it Mean for the Ntds? PloS Negl Trop Dis (2017) 11:e0005424. doi: 10.1371/journal.pntd.0005424
28. Allen JE, Sutherland TE. Host Protective Roles of Type 2 Immunity: Parasite Killing and Tissue Repair, Flip Sides of the Same Coin. Semin Immunol (2014) 26:329–40. doi: 10.1016/j.smim.2014.06.003
29. Chachage M, Podola L, Clowes P, Nsojo A, bauer A, Mgaya O. Helminth-Associated Immune Activation and HIV Co-Receptor Expression. PLoS Negl Trop Med (2014) 8:e2755. doi: 10.1371/journal.pntd.0002755
30. Mkhize-Kwitshana ZL, Taylor MI, Jooste P, Walzl G. The Influence of Different Helminth Infection Phenotypes on Immune Responses Against HIV in Co-Infected Adults in South Africa. BMC Infect Dis (2011) 11:273–84. doi: 10.1186/1471-2334-11-273
31. Onguru D, Liang Y, Griffith Q, Nikolajczyk B, Mwinzi P, Ganley-Leal L. Human Schistosomiasis is Associated With Endotoxemia and Toll-like Receptor 2- and 4-Bearing B Cells. Am J Trop Med Hyg (2011) 84:321–4. doi: 10.4269/ajtmh.2011.10-0397
32. George PJ, Anuradha R, Kumar NP, Kumaraswani V, Nutman TB, Babu S. Evidence of Microbial Translocation Associated With Perturbations in T Cell and Antigen Presenting Cell Homeostasis in Hookworm Infections. PLoS Negl Trop Dis (2012) 6:e1830. doi: 10.1371/journal.pntd.0001830
33. Rajamanickam A, Munisankar S, Bhootra Y, Dolla C, Nutman TB, Babu S. Microbial Translocation Associated With an Acute-Phase Response and Elevations in MMP-1, Ho-1, and Proinflammatory Cytokines in Strongyloides Stercoralis Infection. Infect Immun (2017) 85:e00772–16. doi: 10.1128/IAI.00772-16
34. Kassa D, Petros B, Mesele T, Hailu E, Wolday D. Characterization of Peripheral Blood Lymphocyte Subsets in Patients With Acute Plasmodium Falciparum and P. Vivax Malaria Infections at Wonji Sugar Estate, Ethiopia. Clin Vaccine Immunol (2006) 13:376–9. doi: 10.1128/CVI.13.3.376-379.2006
35. Worku S, Bjorman A, Troye-Blomberg M, Jemaneh L, Farnert A, Christensson B. Lymphocyte Activation and Subset Redistribution in the Peripheral Blood in Acute Malaria Illness: Distinct γδ T Cell Patterns in Plasmodium Falciparum and P. Vivax Infections. Clin Exp Immunol (1997) 108:34–41. doi: 10.1046/j.1365-2249.1997.d01-981.x
36. Kam KM, Leung WL, Kwok MY, Hung MY, Lee SS, Mak WP. Lymphocyte Subpopulation Reference Ranges for Monitoring Human Immunodeficiency Virus-Infected Chinese Adults. Clin Diagn Lab Immunol (1996) 3:326–30. doi: 10.1128/cdli.3.3.326-330:1996
37. Bachou H, Tylleskär T, Downing R, Tumwine JK. Severe Malnutrition With and Without HIV-1 Infection in Hospitalised Children in Kampala, Uganda: Differences in Clinical Features, Haematological Findings and CD4+ Cell Counts. Nutr J (2006) 5:27–34. doi: 10.1186/1475-2891-5-27
38. Paglieroni TG, Holland PV. Circannual Variation in Lymphocyte Subsets, Revisited. Transfusion (1994) 34:512–624. doi: 10.1046/j.1537-2995.1994.34694295067.x
39. Falkenbach A, Sedlmeyer A. Travel to Sunny Countries is Associated With Changes in Immunological Parameters. Photodermatol Photoimmunol Photomed (1997) 13:139–42. doi: 10.1111/j.1600-0781.1997.tb00217.x
40. Resende Co T, Hirsch CS, Toossi Z, Dietze R, Ribeiro-Rodrigues R. Intestinal Helminth Co-Infection has a Negative Impact on Both Anti-Mycobacterium TB Immunity and Clinical Response to TB Therapy. Clin Exp Immunol (2007) 147:45–52. doi: 10.1111/j.1365-2249.2006.03247.x
41. Abate E, Belayneh M, Idh J, Diro E, Elias D, Britton S, et al. Asymptomatic Helminth Infection in Active TB is Associated With Increased Regulatory and Th-2 Responses and a Lower Sputum Smear Positivity. PLoS Negl Trop Dis (2015) 9:e0003994. doi: 10.1371/journal.pntd.0003994
42. Tristao-Sa R, Ribeiro-Rodrigues R, Johnson LT, Pereira FE, Dietze R. Intestinal Nematodes and Pulmonary TB. Rev Soc Bras Med Trop (2002) 35:533–5. doi: 10.1590/S0037-86822002000500020
43. Toulza F, Tsang L, Ottenhoff TH, Brown M, Dockrell HM. Mycobacterium Tuberculosis-Specific CD4+ T-Cell Response is Increased, and Treg Cells Decreased, in Anthelmintic-Treated Patients With Latent TB. Eur J Immunol (2016) 46:752–61. doi: 10.1002/eji.201545843
44. Anuradha R, Munisankar S, Bhootra Y, Dolla C, Kumaran P, Nutman TB, et al. Modulation of Mycobacterium Tuberculosis-Specific Humoral Immune Responses is Associated With Strongyloides Stercoralis Coinfection. PLoS Negl Trop Dis (2017) 11:e0005569. doi: 10.1371/journal.pntd.0005569
45. Monin L, Griffiths KL, Lam WY, Gopal R, Kang DD, Ahmed M, et al. Helminth-Induced Arginase-1 Exacerbates Lung Inflammation and Disease Severity in TB. J Clin Invest (2015) 125:4699–713. doi: 10.1172/JCI77378
46. Loffredo-Verde E, Abdel-Aziz I, Albrecht J, El-Guindy N, Yacob M, Solieman A, et al. Schistosome Infection Aggravates HCV-related Liver Disease and Induces Changes in the Regulatory T-cell Phenotype. Parasit Immunol (2015) 37:97–104. doi: 10.1111/pim.12171
47. Attallah AM, Abdallah SO, Albannan MS, Omran MM, Attallah AA, Farid K. Impact of Hepatitis C Virus/Schistosoma Mansoni Coinfection on the Circulating Levels of HCV-NS4 Protein and Extracellular-Matrix Deposition in Patients With Different Hepatic Fibrosis Stages. Am J Trop Med Hyg (2016) 95:1044–50. doi: 10.4269/ajtmh.16-0129
48. Wolday D, Gebremariam Z, Britton S, Galai N, Landay A, Bentwich Z. Treatment of Intestinal Worms is Associated With Decresed HIV Plasma Viral Load. J Acquir Immune Defic Syndr (2002) 31:56–62. doi: 10.1097/00126334-200209010-00008
49. Mulu A, Maier M, Liebert UG. Deworming of Intestinal Helminths Reduces HIV-1 Subtype C Viremia in Chronically Co-Infected Individuals. Int J Infect Dis (2013) 17:e897–901. doi: 10.1016/j/ijid.2013.03.022
50. Morawski BM, Yunus M, Kerukadho E, Turyasingura G, Barbra L, Ojok AM, et al. Hookworm Infection is Associated With Decreased CD4+ T Cell Counts in HIV-infected Adult Ugandans. PLoS Negl Trop Dis (2017) 11:e0005634. doi: 10.1371/journal.pntd.0005634
51. Furch BD, Koethe JR, Kayamba V, Heimburger DC, Kelly P. Interactions of Schistosoma and HIV in Sub-Saharan Africa: A Systematic Review. Am J Trop Med Hyg (2020) 102:711–8. doi: 10.4269/ajtmh.19-0494
52. Sabin EA, Araujo M, Carvalho EM, Pearce EJ. Impairment of Tetanus Toxoid-Specific Th1-like Immune Responses in Humans Infected With Schistosoma Mansoni. J Infect Dis (1996) 173:269–72. doi: 10.1093/infdis/173.1.269
53. Wammes LJ, Hamid F, Wiria AE, de Gier B, Sartono E, Maizels RM, et al. Regulatory T Cells in Human Geohelminth Infection Suppress Immune Responses to BCG and Plasmodium Falciparum. Eur J Immunol (2010) 40:437–42. doi: 10.1002/eji.200939699
54. Malhotra I, Mungai P, Wamachi A, Kioko J, Ouma JH, Kazura JW, et al. Helminth- and Bacillus Calmette-Guerin-induced Immunity in Children Sensitized In Utero to Filariasis and Schistosomiasis. J Immunol (1999) 162:6843–8.
55. Ghaffar YA, Kamel M, Abdel Wahab MF, Dorgham LS, Saleh MS, El Deeb AS. Hepatitis B Vaccination in Children Infected With Schistosoma Mansoni: Correlation With Ultrasonographic Data. Am J Trop Med Hyg (1990) 43:516–9. doi: 10.4269/ajtmh.1990.43.516
56. Harris JB, Podolsky MJ, Bhuiyan TR, Chowdhury F, Khan AI, Larocque RC, et al. Immunologic Responses to Vibrio Cholerae in Patients Co-Infected With Intestinal Parasites in Bangladesh. PLoS Negl Trop Dis (2009) 3:e403. doi: 10.1371/journal.pntd.0000403
57. Apiwattanakul N, Thomas PG, Iverson AR, McCullers JA. Chronic Helminth Infections Impair Pneumococcal Vaccine Responses. Vaccine (2014) 32:5405–10. doi: 10.1016/j.vaccine.2014.07.107
58. Hartmann W, Brunn ML, Stetter N, Gagliani N, Muscate F, Stanelle-Bertram S, et al. Helminth Infections Suppress the Efficacy of Vaccination Against Seasonal Influenza. Cell Rep (2019) 29:2243–56. doi: 10.1016/j.celrep.2019.10.051
59. Esen M, Mordmüller B, de Salazar PM, Adegnika AA, Agnandji ST, Schaumburg F, et al. Reduced Antibody Responses Against Plasmodium Falciparum Vaccine Candidate Antigens in the Presence of Trichuris Trichiura. Vaccine (2012) 30:7621–4. doi: 10.1016/j.vaccine.2012.10.026
60. Dzhivhuho GA, Rehrl SA, Ndlovu H, Horsnell WGC, Brombacher F, Williamson AL, et al. Chronic Schistosomiasis Suppresses HIV-specific Responses to DNA-MVA and MVA-gp140 Env Vaccine Regimens Despite Anti-Helminthic Treatment and Increases Helminth-Associated Pathology in a Mouse Model. PLoS Pathog (2018) 14:e1007182. doi: 10.1371/journal.ppat.1007182
61. Wammes LJ, Hamid F, Wiria AE, May L, Kaisar MMM, Prasetyani-Gieseler MA, et al. Community Deworming Alleviates Geohelminth-Induced Immune Hyporesponsiveness. Proc Natl Acad Sci U S A (2016) 113:12526–31. doi: 10.1073/pnas.1604570113
62. Abate E, Elias D, Getachew A, Alemu S, Diro E, Britton S, et al. Effects of Albendazole on the Clinical Outcome and Immunological Responses in Helminth Co-Infected TB Patients: A Double Blind Randomised Clinical Trial. Int J Parasitol (2015) 45:133–40. doi: 10.1016/j.ijpara.2014.09.006
63. Elias D, Wolday D, Akuffo H, Petros B, Britton S. Effect of Deworming on Human T Cell Responses to Mycobacterial Antigens in Helminth-Exposed Individuals Before and After Bacilli Calmette-Guérin (BCG) Vaccination. Clin Exp Immunol (2001) 123:219–25. doi: 10.1046/j.1365-2249.2001.01446.x
64. Ivan E, Crowther NJ, Mutimura E, Rucogoza A, Janssen S, Njunwa KK, et al. Effect of Deworming on Disease Progression Markers in HIV-1–infected Pregnant Women on Antiretroviral Therapy: A Longitudinal Observational Study From Rwanda. Clin Infect Dis (2015) 60:135–42. doi: 10.1093/cid/ciu715
65. Walson JL, Otieno PA, Mbuchi M, Richardson BA, Lohman-Payne B, Macharia SW, et al. Albendazole Treatment of HIV-1 and Helminth Co-Infection: A Randomized, Double-Blind, Placebo-Controlled Trial. AIDS (2008) 22:1601–9. doi: 10.1097/QAD.0b013e32830a502e
66. Cooper PJ, Chico ME, Losonsky G, Sandoval C, Espinel I, Sridhara R, et al. Albendazole Treatment of Children With Ascariasis Enhances the Vibriocidal Antibody Response to the Live-Attenuated Oral Cholera Vaccine CVD 103-Hgr. J Infect Dis (2000) 182:1199–206. doi: 10.1086/315837
67. Netea MG, Dominguez-Andres J, Barreiro LB, Chavakis T, Divangahi M, Fuchs E, et al. Defining Trained Immunity and its Role in Health and Disease. Nat Rev Immunol (2020) 20:375–88. doi: 10.1038/s41577-020-0285-6
68. Peng X, Ouyang J, Isnard S, Lin J, Fombuena B, Zhu B, et al. Sharing CD4+ T Cell Loss: When Covid-19 and HIV Collide on Immune System. Front Immunol (2021) 11:596631. doi: 10.3389/fimmunol.2020.596631
69. Abraha HE, Gessesse Z, Gebrecherkos T, Kebede Y, Weldegiorghis AW, Tequare MH, et al. Clinical Features and Risk Factors Associated With Morbidity and Mortality Among COVID-19 Patients in Northern Ethiopia. Int J Infect Dis (2021) 105:776–83. doi: 10.1016/j.ijid.2021.03.037
70. Gebrecherkos T, Gessesse Z, Kebede Y, Gebreegzabheer A, Tasew G, Abdulkader M, et al. Effect of Co-Infection With Parasites on Severity of COVID-19. medRxiv (2021). doi: 10.1101/2021.02.02.212.50995
71. Wolday D, Tasew G, Amogne W, Urban B, Schallig HD, Harris V, et al. Interogating the Impact of Intestinal-Microbiome on Pathogenesis of COVID-19 in Sub-Saharan Africa. Front Microbiol (2021) 12:614522. doi: 10.3389/fmicb.2021.614522
72. Mekonnen Y, Geskus RB, Hendriks JCM, Messele T, Borghans J, Miedema F, et al. Low CD4 T Cell Counts Before HIV-1 Seroconversion do Not Affect Disease Progression in Ethiopian Factory Workers. J Infect Dis (2005) 192:739–48. doi: 10.1086/432545
73. Muyanja E, Ssemaganda A, Ngauv P, Cubas R, Perrin H, Srinivasan D, et al. Immune Activation Alters Cellular and Humoral Responses to Yellow Fever 17D Vaccine. J Clin Invest (2014) 124:3147–58. doi: 10.1172/JCI75429
74. Black GF, Weir RE, Floyd S, Bliss L, Warndorff DK, Crampin AC, et al. BCG-induced Increase in Interferon-Gamma Response to Mycobacterial Antigens and Efficacy of BCG Vaccination in Malawi and the UK: Two Randomized Controlled Studies. Lancet (2002) 359:1393–401. doi: 10.1016/S01400-6736(02)08353-8
75. Kirkpatrick BD, Colgate ER, Mychaleckyj JC, Haque R, Dickson DM, Carmolli MP, et al. The “Performance of Rotavirus and Oral Polio Vaccines in Developing Countries” (PROVIDE) Study: Description of Methods of an Interventional Study Designed to Explore Complex Biologic Problems. Am J Trop Med Hyg (2015) 92:744–51. doi: 10.4269/ajtmh.14-0518
76. Kabagenyi J, Natukunda A, Nassuuna J, Sanya RE, Nampijja M, Webb EL, et al. Urban-Rural Differences in Immune Responses to Mycobacterial and Tetanus Vaccine Antigens in a Tropical Setting: A Role for Helminths? Parasitol Int (2020) 78:102132. doi: 10.1016/j.parint.2020.102132
77. Tanner R, Kakalacheva K, Miller E, Pathan AA, Chalk R, Sander CR, et al. Serum Indoleamine 2,3-Dioxygenase Activity is Associated With Reduced Immunogenicity Following Vaccination With MVA85A. BMC Infect Dis (2014) 14:660–72. doi: 10.1186/s12879-014-0660-7
78. Agnandji ST, Lell B, Fernandes JF, Abossolo BP, Methogo BG, Kabwende AL, et al. A Phase 3 Trial of RTS,S/AS01 Malaria Vaccine in African Infants. N Engl J Med (2012) 367:2284–95. doi: 10.1056/NEJMoa1208394
79. Jongo SA, Urbano V, Church LWP, Olotu A, Manock SR, Schindler T, et al. Immunogenicity and Protective Efficacy of Radiation-Attenuated and Chemo-Attenuated PfSPZ Vaccines in Equatoguinean Adults. Am J Trop Med Hyg (2021) 104:283–93. doi: 10.4269/ajtmh.20-0435
80. Venkatraman N, Ndiaye BP, Bowyer G, Wade D, Sridhar S, Wright D. Et al. Safety and Immunogenicity of a Heterologous Prime-Boost Ebola Virus Vaccine Regimen in Healthy Adults in the United Kingdom and Senegal. J Infect Dis (2019) 219:1187–97. doi: 10.1093/infdis/jiy639
81. de Ruiter K, Jochems SP, Tahapary DL, Stam KA, König M, van Unen V, et al. Helminth Infections Drive Heterogeneity in Human Type 2 and Regulatory Cells. Sci Transl Med (2020) 12:eaaw3703. doi: 10.1126/scitranslmed.aaw3703
82. Nkurunungi G, Zirimenya L, Natukunda A, Nassuuna J, Oduru G, Ninsiima C, et al. Population Differences in Vaccine Responses (POPVAC): Scientific Rationale and Cross-Cutting Analyses for Three Linked, Randomized Controlled Trials Assessing the Role, Reversibility and Mediators of Immunomodulation by Chronic Infections in the Tropics. BMJ Open (2021) 11:e040425. doi: 10.1136/bmjopen-2020-040425
83. van Riet E, Adegnika AA, Retra K, Vieira R, Tielens AG, Lell B, et al. Cellular and Humoral Responses to Influenza in Gabonese Children Living in Rural and Semi-Urban Areas. J Infect Dis (2007) 196:1671–8. doi: 10.1086/522010
84. Voysey M, Clemens SAC, Madhi SA, Weckx LY, Folegatti PM, Aley PK, et al. Single-Dose Administration and the Influence of the Timing of the Booster Dose on Immunogenicity and Efficacy of ChAdOx1 nCoV-19 (AZD1222) Vaccine: A Pooled Analysis of Four Randomized Trials. Lancet (2021) 397:881–91. doi: 10.1016/50140-6736(21)00432-3
85. Koff WC, Schenkelberg T, Williams T, Baric RS, McDermott A, Cameron CM, et al. Development and Deployment of COVID-19 Vaccines for Those Most Vulnerable. Sci Transl Med (2021) eabd1525. doi: 10.1126/scitranslmed.abd1525
86. Hong SL, Potter B, Calvignac-Spencer S, Niatou-Singa FS, Tombolomako TB, Fuh-Neba T, et al. Travel-Driven Emergence and Spread of SARS-CoV-2 Lineage B.1.620 With Multiple VOC-like Mutations and Deletions in Europe. medRxiv (2021). doi: 10.1101/2021.05.04.21256637. Available as preprint.
Keywords: Africa, chronic immune activation, SARS-CoV-2, vaccine, hyporesponsiveness, COVID-19, helminths
Citation: Wolday D, Ndungu FM, Gómez-Pérez GP and de Wit TFR (2021) Chronic Immune Activation and CD4+ T Cell Lymphopenia in Healthy African Individuals: Perspectives for SARS-CoV-2 Vaccine Efficacy. Front. Immunol. 12:693269. doi: 10.3389/fimmu.2021.693269
Received: 10 April 2021; Accepted: 04 June 2021;
Published: 17 June 2021.
Edited by:
Sara De Biasi, University of Modena and Reggio Emilia, ItalyReviewed by:
Henrique Borges da Silva, Mayo Clinic Arizona, United StatesFrancesco Nicoli, University of Ferrara, Italy
Copyright © 2021 Wolday, Ndungu, Gómez-Pérez and de Wit. This is an open-access article distributed under the terms of the Creative Commons Attribution License (CC BY). The use, distribution or reproduction in other forums is permitted, provided the original author(s) and the copyright owner(s) are credited and that the original publication in this journal is cited, in accordance with accepted academic practice. No use, distribution or reproduction is permitted which does not comply with these terms.
*Correspondence: Dawit Wolday, ZGF3d29sQGdtYWlsLmNvbQ==; ZGF3aXQud29sZGF5QG11LmVkdS5ldA==