- 1Section of Immunology, Vetsuisse Faculty, University of Zürich, Zürich, Switzerland
- 2Institute of Experimental Immunology, University of Zürich, Zürich, Switzerland
Fungi are an integral part of the mammalian microbiota colonizing most if not all mucosal surfaces and the skin. Maintaining stable colonization on these surfaces is critical for preventing fungal dysbiosis and infection, which in some cases can lead to life threatening consequences. The epithelial barriers are protected by T cells and additional controlling immune mechanisms. Noncirculating memory T cells that reside stably in barrier tissues play an important role for host protection from commensals and recurrent pathogens due to their fast response and local activity, which provides them a strategic advantage. So far, only a few specific examples of tissue resident memory T cells (TRMs) that act against fungi have been reported. This review provides an overview of the characteristics and functional attributes of TRMs that have been established based on human and mouse studies with various microbes. It highlights what is currently known about fungi specific TRMs mediating immunosurveillance, how they have been targeted in preclinical vaccination approaches and how they can promote immunopathology, if not controlled. A better appreciation of the host protective and damaging roles of TRMs might accelerate the development of novel tissue specific preventive strategies against fungal infections and fungi-driven immunopathologies.
Introduction
Epithelial barriers cover the entire external and internal surfaces of our body to shield it from potentially harmful environmental influences, including toxic substances, radiation, and pathogens. Many of these barriers also fulfil essential physiological functions, such as nutrient uptake in the intestine or gas exchange in the lung. This entails challenging and partly opposing requirements for compartmentalization and permeability. One of the biggest challenges for barrier tissues is the maintenance of homeostasis with commensal microbes, while at the same time they have to prevent invasion and overgrowth of pathogenic microbes and the emergence of inflammatory disorders. Commensal microbes actively contribute to these processes, whereby the role of commensal fungi is increasingly being recognized (1–8). Stable maintenance of the microbiota in equilibrium with the host is strictly dependent on the immune system. Skin and mucosae harbor large numbers of myeloid and lymphoid immune cells that actively respond to commensal microbes, including commensal fungi (9–11), with T cells playing a predominant role in barrier tissue immunity against fungi. Thus, containment of the microbiota relies on active immunosurveillance and is not due to passive ignorance. In turn, the constant immune activation by the microbiota has to be tightly regulated to limit overt responses to innocuous microbes and to avoid tissue damaging inflammation. At the same time, the immune system must remain responsive against invading and disseminating pathogens.
The interest in barrier tissue immunity has steadily increased over the past decades, even though technical challenges have initially limited rapid advances in the field. Mucosal and cutaneous immune cells are scattered throughout the tissue parenchyma. They are often tightly associated with neighboring (non-immune) cells and their survival and function depends on the tissue environment, all of which hampers the isolation and ex vivo analysis of these cells (12). Access to human samples other than blood represents another limitation when studying human immunity in barrier tissues. Refined protocols for single cell isolation in combination with high-dimensional flow or mass cytometry have helped to define cellular subsets (13, 14). Single cell omics approaches, which can provide multidimensional high-resolution data (15–17), and advanced in situ imaging techniques, which provide spatial information for multiparameter settings (18) have further helped to promote the field. Moreover, refined genetic models allow selective targeting of specific cellular subsets in mice in a tissue- and/or time-restricted manner (19, 20). A novel humanized mouse model was recently reported to functionally study human cells in situ in the skin (21).
These approaches and combinations thereof have led to the discovery of various subsets of tissue-specific myeloid and lymphoid immune cells, including noncirculating memory T cells whose maintenance and function is regulated by tissue-specific environmental cues. These sessile memory T cells are referred to as tissue resident memory T cells (TRMs) (22). Seeding of the peripheral tissues with differentiated and long-lived T cells that are specialized in local microbe control is a major strategic advantage for both, the containment of commensal microbes and the rapid on-site control of (re)-appearing pathogens. To date, only a handful of papers have reported the existence of TRMs directed against fungi. In this review I discuss these studies in the context of the current general understanding of TRMs in antigen-specific protection from recurring pathogens and immunosurveillance of commensal microbes, but also of the pathological effects that they can exert. Moreover, I point out scenarios how TRMs might be harnessed for inducing vaccine immunity against fungi (Figure 1). Together, this review shall highlight the potential of this T cell subset in antifungal immunity in health and disease, and emphasize the gaps in knowledge that remain to be filled in the future.
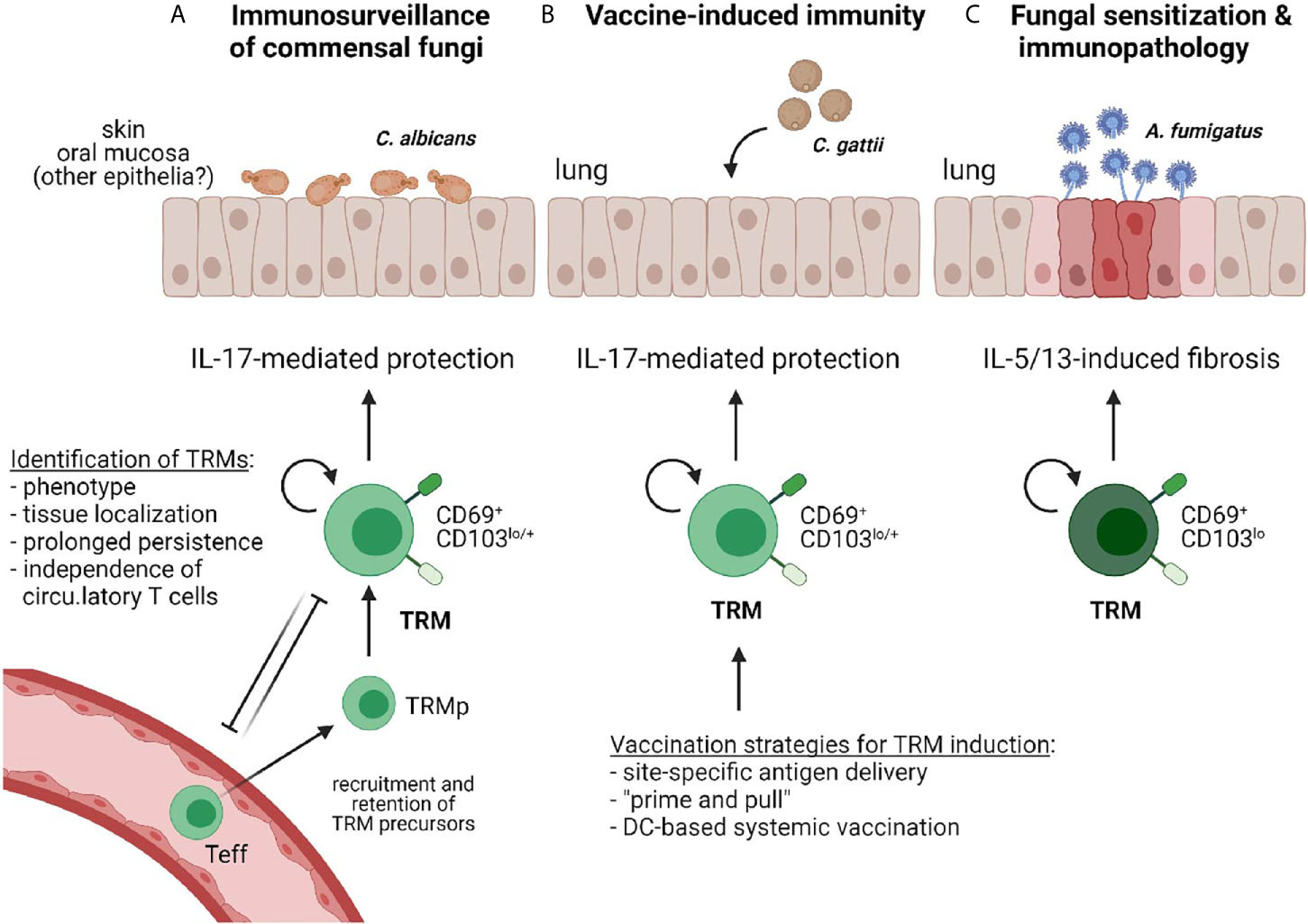
Figure 1 Reported examples of TRMs in antifungal immunity. (A) IL-17 producing CD4+ TRMs provide immunosurveillance against commensal fungi (e.g. C. albicans) that colonize barrier tissues such as the skin or the oral mucosa to prevent dysbiosis (23, 24). (B) Vaccine-induced immunity protects against fungal pathogens (e.g. C. gattii) via elicitation of IL-17-producing CD4+ TRMs (25). Different vaccination strategies for TRM induction in barrier tissues are indicated. (C) Antifungal TRMs can promote inflammation and immunopathology, such as those induced by A. fumigatus sensitization in the airways (26).
How Are TRMs Classified?
TRMs comprise CD4+ and CD8+ T cell subsets in mucosal tissues and the skin, but also in visceral organs, such as liver and lung. However, a T cell in any of these tissues is not automatically a TRM cell, even if it displays features of memory. TRM cells constitute a specific subset of memory T cells in non-lymphoid tissues, independent of circulating T cells. The following criteria define tissue-residency of T cells (27). First, TRMs can be identified by phenotyping: they express elevated levels of CD69, CD103, CD49a and CD11a when compared with circulating memory T cells, although none of these markers unequivocally denotes tissue residency and expression varies between CD4+ and CD8+ TRMs and between TRMs in different tissues. Second, the transcriptional signature can help distinguishing TRMs from other phenotypically similar cell subsets. Third, intravascular labelling allows identification of cells that are not in the circulation, at least at the time of analysis. Fourth, in vivo migration assays such as parabiotic surgery, in situ tissue labelling or tissue transplantation describe prolonged tissue persistence of T cells with limited recirculation potential. Finally, cutting the supply of circulatory T cells, for instance by blocking the migration of circulatory T cells or by selectively ablating them, disconnects the populations of TRM and circulatory T cells and thereby allows establishing autonomy of TRM survival and renewal. Each of these approaches has limitations, but together, they enabled the identification of TRMs as distinct non-recirculating long-lived sessile cells in various tissues (27).
In some tissues such as the epidermis of mice, CD8+ TRMs were found to display a slow crawling behavior and extend long protrusions in between their neighboring cells. This may help them to dynamically scan a large portion of their microenvironment for the presence of antigen (28, 29). Therefore, although tissue-residency follows strict criteria, it does not equal immobility within a given tissue. In contrast to CD8+ TRMs, their CD4+ counterparts were shown to form distinct microanatomical structures referred to as “memory lymphocyte clusters”, which are driven by TRM-derived cytokines (in particular IFN-γ) and chemokines derived from macrophages, both of which being central to cluster formation (30).
In compliance with all T cells TRMs possess a T cell receptor that determines their antigen-specificity. Until today, TRMs directed against diverse commensal and pathogenic microbes have been identified in the skin, the gastrointestinal tract, the reproductive tract, the nasal tissue, the lungs, and the liver. As such, fungi specific TRMs have also been reported: CD4+ TRMs responding to the commensal yeast C. albicans have been identified in healthy human skin based on phenotypic markers and cytokine production (23). In a murine model of C. albicans commensalism, the induction of fungus-specific Th17 cells with TRM features have been recapitulated (24). Beyond expression of CD69, CD103 and CD11a, C. albicans-specific TRMs also fulfil the criteria of TRMs to stay protected from in vivo labelling with an intravenously-injected CD4 antibody, and to be maintained independently of circulatory T cells (24). Another example of fungus-specific TRMs is provided by a study about Aspergillus sensitization in mice, where CD69hi CD103lo CD4+ TRMs were found induced in the lung, with separate subsets producing IL-5 and IL-17 (26). Their transcriptome profile and the finding that they were spared from the vasculature confirmed their identity. TRMs directed against other fungi await to be identified, especially those that respond to commensal and ubiquitous fungi, which are in constant contact with our barrier tissues and against which lasting T cell immunity is a prerequisite of homeostasis. The presence of circulatory memory CD4+ T cells reactive against diverse fungi in the healthy human blood (1) suggests that corresponding TRM subsets with the same specificities will likely be present in the epithelial tissues.
What Are the Functional Attributes of TRMs?
The raison d’être of memory T cells is to direct rapid and efficient protection against previously encountered antigens, which applies to TRMs as well. The first report on the function of TRMs in 2011 described the relevance of influenza-specific lung-resident memory CD4+ T cells for in situ protection against respiratory viral challenge, as they mediate enhanced viral clearance and survival to lethal influenza infection when compared to recirculating memory CD4+ T cells (31). Over the past ten years, the protective capabilities of CD4+ and CD8+ TRM cells have been demonstrated for members of all pathogen classes, including fungi, predominantly in murine infection models (23, 31–43). In humans, the protective effect of TRMs is evidenced by the correlation between presence of pathogen specific TRMs and enhanced protectivity (44–46).
The results from human studies emphasize that TRMs not only provide rapid infection control after re-exposure to a previously encountered and combatted pathogen, a setting modelled by many experimental mouse infection studies (N.B.: in SPF mice, most experimentally administered infectious agents are rapidly cleared), but that TRMs are also particularly critical for host protection during persistent infection (22). This in turn suggests that TRMs likely contribute to immunosurveillance of commensal microbes to which the host is constantly exposed. Indeed, TRMs with reactivity to intestinal microbes are abundant in the gut tissue of healthy individuals (47). An involvement of TRMs in maintaining homeostasis and preventing overgrowth of commensal fungi is evidenced by C. albicans-responsive CD4+ TRMs identified in healthy human skin (23) and in an experimental model of persistent C. albicans colonization in the oral mucosa of mice (24). In both species, these cells produce IL-17 in response to fungal re-stimulation, in line with the well-recognized host protective role of IL-17 against C. albicans in barrier tissues (48).
Overall, the population of TRMs in a given tissue reflects its history of exposures to pathogenic and commensal microbes. Analysis of T cell subsets in the intestinal mucosa of children revealed that naïve recent thymic emigrants and effector memory T cells predominate during early life, whereas TRMs progressively accumulate with increasing age (49). The continuous build-up thereby results in specific TRM pools that are geared to the need of the specific sites. By orchestrating regional immune responses to ongoing microbial exposure in a clinically silent manner, TRMs act as central players in maintaining tissue homeostasis and a stable host-microbe equilibrium.
How Do TRMs Exert Their Functions?
Like all T cells, TRMs respond to TCR-mediated stimulation and activate effector functions in an antigen-specific manner. The advantage of TRMs, if compared to circulatory T cell subsets, arises from their strategic positioning within tissues, which allows immediate local recognition of infected or antigen presenting cells and a rapid recall of canonical effector functions, such as cytokine secretion or perforin- and granzyme-mediated target cell killing (22). Thereby, TRMs can prevent invasion and dissemination of microbes that have crossed the physical barrier through which they are usually segregated, and thwart clinically apparent infection.
Upon re-encounter with cognate antigen, TRMs can profoundly alter the local tissue environment. Through production of cytokines, they can trigger rapid adaptive and innate immune responses, including local humoral responses, activation of local innate immune cells (dendritic cells, NK cells) and recruitment of circulating lymphocytes, resulting in a remarkable cross-over between innate and adaptive immunity. Thereby, the triggering of a small number of TRMs is amplified into an organ-wide response, which can provide protection against even antigenically unrelated organisms (50, 51). This may offer a particular advantage for controlling escape variants of pathogens that might emerge.
An explanation for the reactivity against unrelated antigens is provided by the broad cross-reactivity of the T cell repertoire (52). Widely cross-reactive T cells are abundant in the human T cell memory compartment comprising T cells that react against antigens to which the host has not been exposed previously (52, 53). Cross-reactivity has also been proposed for C. albicans-responsive memory T cells to explain the diverse and systemic effects that these cells can exert (54). In animal models, TRMs have further been found to respond to noncognate, bystander activation (55–57). The potential of microbiota-specific cross-reactive TRMs for host protection is illustrated by skin-resident TRMs induced in response to topically applied Staphylococcus epidermidis, which specifically reinforce the barrier function of the epithelium and prevents overgrowth of heterologous microbes, including C. albicans, in a CD8+ T cell- and IL-17-dependent manner (58). In addition to TCR cross-reactivity, TRMs can also provide heterologous protection by responding to bystander activation via TCR-independent mechanisms (59).
Besides their potential broad reactivity, TRMs may display some degree of functional plasticity. In fact, skin-resident commensal-specific memory Th17 cells were found to rapidly activate a type 2 effector program in response to tissue injury (60). This cell-intrinsic flexibility might allow them to swiftly adapt to environment insults, which might be caused by physical damage or invasive pathogens.
How Are TRMs Implicated in Immunopathological Responses?
As a consequence of their ability to rapidly respond to antigen or to react even in an antigen-unspecific manner, and to display functional plasticity, TRMs may entail severe consequences on tissue homeostasis. Indeed, commensal-specific responses in barrier tissues are characterized by the production of IL-17, a cytokine that promotes antimicrobial functions and barrier integrity of epithelia (48). IL-17 production in barrier tissues is particularly relevant for immunosurveillance of Candida, as genetic defects in genes of the IL-17 pathway drive the development of chronic mucocutaneous candidiasis (10). However, IL-17 can also contribute to the etiology and pathology of various inflammatory skin disorders, such as psoriasis (61).
Psoriasis is a T cell mediated disease (62). Although the specific antigens targeted by psoriatic T cells remain undefined (63), skin-colonizing microbes are likely candidates. Strikingly, psoriatic lesions are limited to characteristic sites, such as the flexor sides of extremities, the sacral region, and soles of the feet, and disease recurs at always the same predilection sites. An explanation for the site-specific manifestation of disease may be provided by tissue residency of the disease-mediating T cells. Indeed, a study looking into lesional and non-lesional skin samples from psoriasis patients found that the epidermis with active psoriasis was massively infiltrated by CD8+ TRMs when compared to non-lesional skin and healthy skin, with a 100-fold increase of TRMs in active psoriatic lesions (64, 65). The CD103+CD8+ T cells in psoriatic skin expressed IL-17 and IL-22 mRNA. Importantly, IL17A-expressing CD103+CD8+ T cells were found to be present in both active psoriatic plaques as well as in resolved skin lesions, but not in healthy control skin (65, 66), suggesting that they are poised for re-initiation of inflammation. Therefore, TRMs may act as drivers of disease flares in a site-specific manner.
TRMs were also implicated in other chronic and recurring inflammatory disorders that are characterized by localized manifestations in the skin, the oral-gastrointestinal tract, or the respiratory tract. For instance, in patients with chronic rhinosinusitis, TRMs accumulate in the nasal polyps (67). In periodontitis, expansion of Th17 TRMs drives immunopathology in response to local dysbiosis (68). In vitiligo, a pigmentation defect of the skin mediated by melanocyte eradicating cytotoxic T cells (69), the proportion of perforin and granzyme B expressing CD8+CD103+ TRMs is increased in lesional compared to non-lesional and healthy skin (70). Similarly, in atopic dermatitis (AD), CD69+CD103+ TRMs were significantly expanded in lesional compared to non-lesional skin and healthy controls (71). In AD, the chronic allergic skin inflammation is dominated by Th2 cells that react against environmental allergens such as house dust mite proteins, but also skin microbes such as S. aureus and the skin commensal yeast Malassezia (72). AD lesions occur primarily on the cubital and popliteal fossae, and in head and neck type AD on the upper trunk, shoulders, and scalp, with age-related variations (72). Certain subtypes of AD display overlapping characteristics with psoriasis, especially by the involvement of IL-17-producing T cells (73). However, AD and psoriasis are clearly separable diseases, not least based on the different skin regions that are affected (73, 74). A likely explanation for the site-specific recurrence of the lesions may once again be provided by the limited distribution of pathogenic TRMs across different skin sites, and by their diverging antigen specificity (63).
TRMs have also been linked to the pathogenesis of inflammatory bowel disease (IBD). CD4+CD69+ TRMs producing pro-inflammatory cytokines were found enriched in the intestinal mucosa of IBD compared to control patients, and the presence of these cells was predictive of the development of flares (19). Attenuation of disease in an experimental model of transfer colitis when Hobit- and Blimp-1-deficient CD4+ T cells were transferred, confirmed the disease-driving role of CD4+ TRMs in IBD (19). Finally, TRMs can also mediate immunopathology in the airways (75). In experimental allergy models, lung CD4+ TRMs generated in response to allergen exposure can promote reactive airway disease (76, 77). Similarly, in a model of fungal allergy, sensitization with Aspergillus fumigatus induced a population of CD103loCD69hi CD4+ TRMs that promoted pathology through enhanced production of IL-5 and IL-13 in the lung. Of interest, the fibrotic response was ameliorated by CD103hi tissue resident regulatory T cells (26).
The detrimental consequences that TRMs can have in chronic inflammatory diseases emphasize the need for tight regulation of their activity. Recent experimental evidence suggests that TRM intrinsic mechanisms control their reactivation to prevent damaging immunopathology. In a model of contact hypersensitivity to 2,4-dinitrofluorobenzene, inhibitory checkpoint receptor antagonists dramatically enhanced the magnitude and severity of eczema exacerbations (78). Similarly, the antiviral activity of TRMs against HSV-1 re-activation in latently infected rabbits was restored by antibodies directed against PD-1 and LAG-3 (79). This indicates that TRM responsiveness is restrained during steady state. In support of this hypothesis, TRMs express various inhibitory receptors including PD-1, CLTA-4, LAG-3 amongst others (80, 81). Moreover, tissue residency of T cells was associated with an immunoregulatory gene expression program, including IL-10 cytokine expression under the control of the transcriptional regulator c-MAF (82). Such cell-intrinsic regulatory mechanisms likely exist also for TRMs directed against fungi to ensure that under homeostatic conditions they remain clinically silent even if they are constantly exposed to stimulation by commensal and environmental fungi, although specific examples are not available yet.
How Can TRMs Be Harnessed for Achieving Vaccine Immunity?
The important role of TRMs in site-specific protection renders them an attractive target in vaccine development, particularly against tissue-tropic infections. This also applies to vaccines against fungal agents. While induction of long-lived immune memory against a vaccine antigen represents a challenge by itself, induction of residency adds another dimension of complexity to vaccine design. Site specific antigen delivery has produced promising results in preclinical studies with regards to the induction of TRM and vaccine immunity. As such, nasal immunization with certain antigen/adjuvant combinations results in effective induction of TRMs in the respiratory tract (83–85). Another example is provided by the intradermal injection of a synthetic DNA vaccine encoding a Leishmania antigen, which resulted in enhanced protection from experimental infection with Leishmania major in the skin if compared to intramuscular vaccination (86). Vaccine-induced TRMs have also proven effective in immunotherapy against melanoma in an experimental tumor model (87). Despite these promising proof of principle studies, TRM induction in barrier tissues by site-specific vaccine delivery remains a challenge due to the high tolerance threshold in these organs (88). Transient microbiota depletion has recently been explored for temporarily restraining colonization resistance in the gut and thereby allowing expansion of a pool of antigen specific functional TRMs directed against orally delivered Listeria monocytogenes, which ultimately enhance protection against infectious re-challenge (89).
An alternative vaccination strategy for generating TRM-mediated vaccine immunity at relevant tissue sites has been explored by a two-step approach, which consists of first generating a systemic immune response against a vaccine antigen, for instance by intramuscular immunization, and then applying a localized inflammatory stimulus to ‘pull’ the newly primed T cells towards a specific tissue. This “prime and pull” strategy relies on the notion that TRM precursors are primed in secondary lymphoid organs before they migrate to peripheral tissue, where micro-environmental cues then drive TRM maturation (90–93). In case of CD8+ TRMs, adoption of residency has been described to depend on local TGF-β and IL-15 (94–97). TGFβ appears to be required at multiple steps in TRM development (98). In the skin, exposure of CD8+ T cells to TGF-β efficiently induces expression of CD103, which anchors TRMs to E-cadherin expressing epithelial cells (43, 81, 95, 96). Accumulation and persistence of TRMs in the epithelial niche is further determined by the downregulation of pathways linked to T cell egress from peripheral tissues and the upregulation of tissue-retention and pro-survival molecules (22), which are under the control of the transcription factors Hobit and Runx3 (99, 100), albeit with species-specific variations (19, 99). Long-term persistence of TRMs is thought to depend on IL-15 (94) and was in addition recently found to depend on continuous availability of autocrine TGF-β (101).
Although inflammatory stimuli such as imiquimod have been shown sufficient to “pull” TRM precursors to peripheral tissues, conversion of recruited T cells into TRMs may be maximized by local antigen recognition, especially in the lung (102, 103). If more generally valid, this notion might be highly relevant for fungal vaccination strategies, considering that memory T cells directed against many different fungi exist in healthy human blood (1). “Pulling” these pre-existing fungus-specific memory T cells to a specific tissue site for local protection from fungal overgrowth may provide a suitable approach under certain conditions.
Induction of TRM immunity against antigens to which the host was not previously exposed and to which the host has therefore not yet mounted a T cell response, was also achieved by alternative approaches, for instance by antibody targeting. Selective delivery of antigen to respiratory dendritic cells resulted in the development of lung CD8+ TRMs that were highly protective against lethal influenza challenge (104). Similarly, CD4+ TRMs were efficiently induced by a dendritic cell-based vaccine in the context of a pulmonary mycosis. Systemically administered antigen-loaded dendritic cells were found to migrate with high efficiency to the lung, where they elicited a local and resident CD4+ T cell response. The vaccine suppressed fungal burden in the lungs and improved the survival of mice infected with the highly virulent fungus Cryptococcus gattii (25).
Although site-specific accumulation of TRMs has been associated with successful vaccine immunity, induction of TRMs does not always equal resistance to infection, as demonstrated by the following study, which explored a fungal vaccination strategy in a preclinical model of Blastomyces infection. Intranasal administration of a subunit vaccine composed of Blastomyces endoglucanase-2 (Bl-Eng2), which harbors both, a Dectin-2 agonistic ligand and a CD4+ T cell epitope, induced a large number of tetramer+ TRMs in the lung of mice. However, it failed to protect against lethal infection with Blastomyces (105). In contrast, systemic administration of the same vaccine induced a population of tetramer+ CD4+ migratory T cells enriched within the pulmonary vasculature that migrated to the lung tissue upon challenge and efficiently protected mice against infection (105). While the underlying cause of the mucosal vaccine failure remains to be established, one hypothesis concerns the localization of the pathogen to be combatted by intranasally induced vaccine immunity, which is intracellular in case of many of the examples provided above, but extracellular in case of Blastomyces. A lot remains to be learned about the signals favoring induction of functional TRMs capable of conveying enhanced local protection and how this knowledge can be translated to make it applicable in vaccine design.
Concluding Remarks
Over the past years the interest in TRMs has steadily grown and numerous studies have contributed to our current appreciation of the relevance of these cells residing in most, if not all non-lymphoid tissues where they contribute to tissue homeostasis and microbial control but can also drive immunopathology. In the field of fungal immunity, only very few reports are currently available on TRMs with antifungal functions. Fungus-specific TRMs follow the principles that were delineated for TRMs directed against other microbes and viruses. Fungi-specific TRMs are expected to populate all tissues that are naturally exposed to commensal or environmental fungi. Their exact features and their relevance in fungal control awaits to be identified, including similarities and differences between TRMs directed against distinct fungi and in different tissues. Besides promoting immunosurveillance, fungi specific TRMs will likely also be found to be involved in inflammatory pathologies of barrier tissues. More research on TRMs in fungal immunity using preclinical models and human tissues is warranted. The expected knowledge gain will help taking informed decisions for harnessing TRMs in antifungal vaccine development.
Author Contributions
The author confirms being the sole contributor of this work and has approved it for publication.
Funding
Work in the SL-L-lab is supported by the Swiss National Science Foundation (grant 310030_166206, 310030_189255 and CRSII5_173863).
Conflict of Interest
The author declares that the research was conducted in the absence of any commercial or financial relationships that could be construed as a potential conflict of interest.
Acknowledgments
The author would like to thank Julia Lagler for review of the manuscript and all members of the SL-L-lab for stimulating discussions. The figure was created with BioRender.com.
References
1. Bacher P, Hohnstein T, Beerbaum E, Rocker M, Blango MG, Kaufmann S, et al. Human Anti-fungal Th17 Immunity and Pathology Rely on Cross-Reactivity Against Candida Albicans. Cell (2019) 176:1340–55.e15. doi: 10.1016/j.cell.2019.01.041
2. Iliev ID, Funari VA, Taylor KD, Nguyen Q, Reyes CN, Strom SP, et al. Interactions Between Commensal Fungi and the C-type Lectin Receptor Dectin-1 Influence Colitis. Science (2012) 336:1314–7. doi: 10.1126/science.1221789
3. Jiang TT, Shao TY, Ang WXG, Kinder JM, Turner LH, Pham G, et al. Commensal Fungi Recapitulate the Protective Benefits of Intestinal Bacteria. Cell Host Microbe (2017) 22:809–16.e4. doi: 10.1016/j.chom.2017.10.013
4. Li X, Leonardi I, Semon A, Doron I, Gao IH, Putzel GG, et al. Response to Fungal Dysbiosis by Gut-Resident Cx3cr1(+) Mononuclear Phagocytes Aggravates Allergic Airway Disease. Cell Host Microbe (2018) 24:847–56.e4. doi: 10.1016/j.chom.2018.11.003
5. Limon JJ, Tang J, Li D, Wolf AJ, Michelsen KS, Funari V, et al. Malassezia Is Associated With Crohn’s Disease and Exacerbates Colitis in Mouse Models. Cell Host Microbe (2019) 25:377–388 e6. doi: 10.1016/j.chom.2019.01.007
6. Shao TY, Ang WXG, Jiang TT, Huang FS, Andersen H, Kinder JM, et al. Commensal Candida Albicans Positively Calibrates Systemic Th17 Immunological Responses. Cell Host Microbe (2019) 25:404–417 e6. doi: 10.1016/j.chom.2019.02.004
7. Skalski JH, Limon JJ, Sharma P, Gargus MD, Nguyen C, Tang J, et al. Expansion of Commensal Fungus Wallemia Mellicola in the Gastrointestinal Mycobiota Enhances the Severity of Allergic Airway Disease in Mice. PloS Pathog (2018) 14:e1007260. doi: 10.1371/journal.ppat.1007260
8. Wheeler ML, Limon JJ, Bar AS, Leal CA, Gargus M, Tang J, et al. Immunological Consequences of Intestinal Fungal Dysbiosis. Cell Host Microbe (2016) 19:865–73. doi: 10.1016/j.chom.2016.05.003
9. Leonardi I, Li X, Semon A, Li D, Doron I, Putzel G, et al. Cx3cr1(+) Mononuclear Phagocytes Control Immunity to Intestinal Fungi. Science (2018) 359:232–6. doi: 10.1126/science.aao1503
10. Li J, Vinh DC, Casanova JL, Puel A. Inborn Errors of Immunity Underlying Fungal Diseases in Otherwise Healthy Individuals. Curr Opin Microbiol (2017) 40:46–57. doi: 10.1016/j.mib.2017.10.016
11. Sparber F, De Gregorio C, Steckholzer S, Ferreira FM, Dolowschiak T, Ruchti F, et al. The Skin Commensal Yeast Malassezia Triggers a Type 17 Response That Coordinates Anti-Fungal Immunity and Exacerbates Skin Inflammation. Cell Host Microbe (2019) 25:389–403 e6. doi: 10.1016/j.chom.2019.02.002
12. Sparber F, LeibundGut-Landmann S. Assessment of Immune Responses to Fungal Infections: Identification and Characterization of Immune Cells in the Infected Tissue. Methods Mol Biol (2017) 1508:167–82. doi: 10.1007/978-1-4939-6515-1_8
13. Bandura DR, Baranov VI, Ornatsky OI, Antonov A, Kinach R, Lou X, et al. Mass Cytometry: Technique for Real Time Single Cell Multitarget Immunoassay Based on Inductively Coupled Plasma Time-of-Flight Mass Spectrometry. Anal Chem (2009) 81:6813–22. doi: 10.1021/ac901049w
14. Nolan JP, Condello D. Spectral Flow Cytometry. Curr Protoc Cytom (2013) Chapter 1:Unit1 27. doi: 10.1002/0471142956.cy0127s63
15. Tang F, Barbacioru C, Wang Y, Nordman E, Lee C, Xu N, et al. Mrna-Seq Whole-Transcriptome Analysis of a Single Cell. Nat Methods (2009) 6:377–82. doi: 10.1038/nmeth.1315
16. Budnik B, Levy E, Harmange G, Slavov N. Scope-MS: Mass Spectrometry of Single Mammalian Cells Quantifies Proteome Heterogeneity During Cell Differentiation. Genome Biol (2018) 19:161. doi: 10.1186/s13059-018-1547-5
17. Buenrostro JD, Giresi PG, Zaba LC, Chang HY, Greenleaf WJ. Transposition of Native Chromatin for Fast and Sensitive Epigenomic Profiling of Open Chromatin, DNA-binding Proteins and Nucleosome Position. Nat Methods (2013) 10:1213–8. doi: 10.1038/nmeth.2688
18. Bodenmiller B. Multiplexed Epitope-Based Tissue Imaging for Discovery and Healthcare Applications. Cell Syst (2016) 2:225–38. doi: 10.1016/j.cels.2016.03.008
19. Zundler S, Becker E, Spocinska M, Slawik M, Parga-Vidal L, Stark R, et al. Hobit- and Blimp-1-driven Cd4(+) Tissue-Resident Memory T Cells Control Chronic Intestinal Inflammation. Nat Immunol (2019) 20:288–300. doi: 10.1038/s41590-018-0298-5
20. Behr FM, Parga-Vidal L, Kragten NAM, van Dam TJP, Wesselink TH, Sheridan BS, et al. Tissue-Resident Memory Cd8(+) T Cells Shape Local and Systemic Secondary T Cell Responses. Nat Immunol (2020) 21:1070–81. doi: 10.1038/s41590-020-0723-4
21. Klicznik MM, Benedetti A, Gail LM, Varkhande SR, Holly R, Laimer M, et al. A Novel Humanized Mouse Model to Study the Function of Human Cutaneous Memory T Cells In Vivo in Human Skin. Sci Rep (2020) 10:11164. doi: 10.1038/s41598-020-67430-7
22. Gebhardt T, Palendira U, Tscharke DC, Bedoui S. Tissue-Resident Memory T Cells in Tissue Homeostasis, Persistent Infection, and Cancer Surveillance. Immunol Rev (2018) 283:54–76. doi: 10.1111/imr.12650
23. Park CO, Fu X, Jiang X, Pan Y, Teague JE, Collins N, et al. Staged Development of Long-Lived T-Cell Receptor Alphabeta TH17 Resident Memory T-Cell Population to Candida Albicans After Skin Infection. J Allergy Clin Immunol (2018) 142:647–62. doi: 10.1016/j.jaci.2017.09.042
24. Kirchner F, LeibundGut-Landmann S. Tissue-Resident Memory Th17 Cells Maintain Stable Fungal Commensalism in the Oral Mucosa. Mucosal Immunol under Rev (2020) 14:455–67. doi: 10.1038/s41385-020-0327-1
25. Ueno K, Urai M, Sadamoto S, Shinozaki M, Takatsuka S, Abe M, et al. A Dendritic Cell-Based Systemic Vaccine Induces Long-Lived Lung-Resident Memory Th17 Cells and Ameliorates Pulmonary Mycosis. Mucosal Immunol (2019) 12:265–76. doi: 10.1038/s41385-018-0094-4
26. Ichikawa T, Hirahara K, Kokubo K, Kiuchi M, Aoki A, Morimoto Y, et al. Cd103(Hi) Treg Cells Constrain Lung Fibrosis Induced by CD103(lo) Tissue-Resident Pathogenic Cd4 T Cells. Nat Immunol (2019) 20:1469–80. doi: 10.1038/s41590-019-0494-y
27. Szabo PA, Miron M, Farber DL. Location, Location, Location: Tissue Resident Memory T Cells in Mice and Humans. Sci Immunol (2019) 4:eaas9673. doi: 10.1126/sciimmunol.aas9673
28. Zaid A, Mackay LK, Rahimpour A, Braun A, Veldhoen M, Carbone FR, et al. Persistence of Skin-Resident Memory T Cells Within an Epidermal Niche. Proc Natl Acad Sci USA (2014) 111:5307–12. doi: 10.1073/pnas.1322292111
29. Ariotti S, Beltman JB, Chodaczek G, Hoekstra ME, van Beek AE, Gomez-Eerland R, et al. Tissue-Resident Memory Cd8+ T Cells Continuously Patrol Skin Epithelia to Quickly Recognize Local Antigen. Proc Natl Acad Sci USA (2012) 109:19739–44. doi: 10.1073/pnas.1208927109
30. Iijima N, Iwasaki A. T Cell Memory. A Local Macrophage Chemokine Network Sustains Protective Tissue-Resident Memory Cd4 T Cells. Science (2014) 346:93–8. doi: 10.1126/science.1257530
31. Teijaro JR, Turner D, Pham Q, Wherry EJ, Lefrancois L, Farber DL. Cutting Edge: Tissue-Retentive Lung Memory Cd4 T Cells Mediate Optimal Protection to Respiratory Virus Infection. J Immunol (2011) 187:5510–4. doi: 10.4049/jimmunol.1102243
32. Glennie ND, Yeramilli VA, Beiting DP, Volk SW, Weaver CT, Scott P. Skin-Resident Memory Cd4+ T Cells Enhance Protection Against Leishmania Major Infection. J Exp Med (2015) 212:1405–14. doi: 10.1084/jem.20142101
33. Jiang X, Clark RA, Liu L, Wagers AJ, Fuhlbrigge RC, Kupper TS. Skin Infection Generates non-Migratory Memory Cd8+ T(RM) Cells Providing Global Skin Immunity. Nature (2012) 483:227–31. doi: 10.1038/nature10851
34. Park SL, Zaid A, Hor JL, Christo SN, Prier JE, Davies B, et al. Local Proliferation Maintains a Stable Pool of Tissue-Resident Memory T Cells After Antiviral Recall Responses. Nat Immunol (2018) 19:183–91. doi: 10.1038/s41590-017-0027-5
35. Wilk MM, Misiak A, McManus RM, Allen AC, Lynch MA, Mills KHG. Lung CD4 Tissue-Resident Memory T Cells Mediate Adaptive Immunity Induced by Previous Infection of Mice With Bordetella Pertussis. J Immunol (2017) 199:233–43. doi: 10.4049/jimmunol.1602051
36. Bishu S, Hou G, El Zaatari M, Bishu SR, Popke D, Zhang M, et al. Citrobacter Rodentium Induces Tissue-Resident Memory Cd4(+) T Cells. Infect Immun (2019) 87:e00295-19. doi: 10.1128/IAI.00295-19
37. Shenoy AT, Wasserman GA, Arafa EI, Wooten AK, Smith NMS, Martin IMC, et al. Lung CD4(+) Resident Memory T Cells Remodel Epithelial Responses to Accelerate Neutrophil Recruitment During Pneumonia. Mucosal Immunol (2020) 13:334–43. doi: 10.1038/s41385-019-0229-2
38. Amezcua Vesely MC, Pallis P, Bielecki P, Low JS, Zhao J, Harman CCD, et al. Effector TH17 Cells Give Rise to Long-Lived Trm Cells That Are Essential for an Immediate Response Against Bacterial Infection. Cell (2019) 178:1176–88.e15. doi: 10.1016/j.cell.2019.07.032
39. Benoun JM, Peres NG, Wang N, Pham OH, Rudisill VL, Fogassy ZN, et al. Optimal Protection Against Salmonella Infection Requires Noncirculating Memory. Proc Natl Acad Sci USA (2018) 115:10416–21. doi: 10.1073/pnas.1808339115
40. Steinfelder S, Rausch S, Michael D, Kuhl AA, Hartmann S. Intestinal Helminth Infection Induces Highly Functional Resident Memory Cd4(+) T Cells in Mice. Eur J Immunol (2017) 47:353–63. doi: 10.1002/eji.201646575
41. Kinnear E, Lambert L, McDonald JU, Cheeseman HM, Caproni LJ, Tregoning JS. Airway T Cells Protect Against RSV Infection in the Absence of Antibody. Mucosal Immunol (2018) 11:249–56. doi: 10.1038/mi.2017.46
42. Tse SW, Cockburn IA, Zhang H, Scott AL, Zavala F. Unique Transcriptional Profile of Liver-Resident Memory Cd8+ T Cells Induced by Immunization With Malaria Sporozoites. Genes Immun (2013) 14:302–9. doi: 10.1038/gene.2013.20
43. Sheridan BS, Pham QM, Lee YT, Cauley LS, Puddington L, Lefrancois L. Oral Infection Drives a Distinct Population of Intestinal Resident Memory Cd8(+) T Cells With Enhanced Protective Function. Immunity (2014) 40:747–57. doi: 10.1016/j.immuni.2014.03.007
44. Jozwik A, Habibi MS, Paras A, Zhu J, Guvenel A, Dhariwal J, et al. Rsv-Specific Airway Resident Memory Cd8+ T Cells and Differential Disease Severity After Experimental Human Infection. Nat Commun (2015) 6:10224. doi: 10.1038/ncomms10224
45. Pallett LJ, Davies J, Colbeck EJ, Robertson F, Hansi N, Easom NJW, et al. Il-2(high) Tissue-Resident T Cells in the Human Liver: Sentinels for Hepatotropic Infection. J Exp Med (2017) 214:1567–80. doi: 10.1084/jem.20162115
46. Zhu J, Peng T, Johnston C, Phasouk K, Kask AS, Klock A, et al. Immune Surveillance by CD8alphaalpha+ Skin-Resident T Cells in Human Herpes Virus Infection. Nature (2013) 497:494–7. doi: 10.1038/nature12110
47. Hegazy AN, West NR, Stubbington MJT, Wendt E, Suijker KIM, Datsi A, et al. Circulating and Tissue-Resident Cd4(+) T Cells With Reactivity to Intestinal Microbiota Are Abundant in Healthy Individuals and Function Is Altered During Inflammation. Gastroenterology (2017) 153:20–1337.e16. doi: 10.1053/j.gastro.2017.07.047
48. McGeachy MJ, Cua DJ, Gaffen SL. The IL-17 Family of Cytokines in Health and Disease. Immunity (2019) 50:892–906. doi: 10.1016/j.immuni.2019.03.021
49. Thome JJ, Bickham KL, Ohmura Y, Kubota M, Matsuoka N, Gordon C, et al. Early-Life Compartmentalization of Human T Cell Differentiation and Regulatory Function in Mucosal and Lymphoid Tissues. Nat Med (2016) 22:72–7. doi: 10.1038/nm.4008
50. Ariotti S, Hogenbirk MA, Dijkgraaf FE, Visser LL, Hoekstra ME, Song JY, et al. T Cell Memory. Skin-resident Memory Cd8(+) T Cells Trigger a State of Tissue-Wide Pathogen Alert. Science (2014) 346:101–5. doi: 10.1126/science.1254803
51. Schenkel JM, Fraser KA, Beura LK, Pauken KE, Vezys V, Masopust D. T Cell Memory. Resident Memory Cd8 T Cells Trigger Protective Innate and Adaptive Immune Responses. Science (2014) 346:98–101. doi: 10.1126/science.1254536
52. Su LF, Kidd BA, Han A, Kotzin JJ, Davis MM. Virus-Specific CD4(+) Memory-Phenotype T Cells are Abundant in Unexposed Adults. Immunity (2013) 38:373–83. doi: 10.1016/j.immuni.2012.10.021
53. Campion SL, Brodie TM, Fischer W, Korber BT, Rossetti A, Goonetilleke N, et al. Proteome-Wide Analysis of HIV-specific Naive and Memory Cd4(+) T Cells in Unexposed Blood Donors. J Exp Med (2014) 211:1273–80. doi: 10.1084/jem.20130555
54. Scheffold A, Bacher P, LeibundGut-Landmann S. T Cell Immunity to Commensal Fungi. Curr Opin Microbiol (2020) 58:116–23. doi: 10.1016/j.mib.2020.09.008
55. Sckisel GD, Tietze JK, Zamora AE, Hsiao HH, Priest SO, Wilkins DE, et al. Influenza Infection Results in Local Expansion of Memory Cd8(+) T Cells With Antigen non-Specific Phenotype and Function. Clin Exp Immunol (2014) 175:79–91. doi: 10.1111/cei.12186
56. Ge C, Monk IR, Pizzolla A, Wang N, Bedford JG, Stinear TP, et al. Bystander Activation of Pulmonary Trm Cells Attenuates the Severity of Bacterial Pneumonia by Enhancing Neutrophil Recruitment. Cell Rep (2019) 29:4236–4244 e3. doi: 10.1016/j.celrep.2019.11.103
57. Low JS, Farsakoglu Y, Amezcua Vesely MC, Sefik E, Kelly JB, Harman CCD, et al. Tissue-Resident Memory T Cell Reactivation by Diverse Antigen-Presenting Cells Imparts Distinct Functional Responses. J Exp Med (2020) 217:e20192291. doi: 10.1084/jem.20192291
58. Naik S, Bouladoux N, Linehan JL, Han SJ, Harrison OJ, Wilhelm C, et al. Commensal-Dendritic-Cell Interaction Specifies a Unique Protective Skin Immune Signature. Nature (2015) 520:104–8. doi: 10.1038/nature14052
59. Lee HG, Lee JU, Kim DH, Lim S, Kang I, Choi JM. Pathogenic Function of Bystander-Activated Memory-Like Cd4(+) T Cells in Autoimmune Encephalomyelitis. Nat Commun (2019) 10:709. doi: 10.1038/s41467-019-08482-w
60. Harrison OJ, Linehan JL, Shih HY, Bouladoux N, Han SJ, Smelkinson M, et al. Commensal-Specific T Cell Plasticity Promotes Rapid Tissue Adaptation to Injury. Sci 363 (2019) 393:eaat6280. doi: 10.1126/science.aat6280
61. Martin DA, Towne JE, Kricorian G, Klekotka P, Gudjonsson JE, Krueger JG, et al. The Emerging Role of IL-17 in the Pathogenesis of Psoriasis: Preclinical and Clinical Findings. J Invest Dermatol (2013) 133:17–26. doi: 10.1038/jid.2012.194
63. Eyerich S, Onken AT, Weidinger S, Franke A, Nasorri F, Pennino D, et al. Mutual Antagonism of T Cells Causing Psoriasis and Atopic Eczema. N Engl J Med (2011) 365:231–8. doi: 10.1056/NEJMoa1104200
64. Vo S, Watanabe R, Koguchi-Yoshioka H, Matsumura Y, Ishitsuka Y, Nakamura Y, et al. Cd8 Resident Memory T Cells With Interleukin 17a-Producing Potential are Accumulated in Disease-Naive Nonlesional Sites of Psoriasis Possibly in Correlation With Disease Duration. Br J Dermatol (2019) 181:410–2. doi: 10.1111/bjd.17748
65. Cheuk S, Wiken M, Blomqvist L, Nylen S, Talme T, Stahle M, et al. Epidermal Th22 and Tc17 Cells Form a Localized Disease Memory in Clinically Healed Psoriasis. J Immunol (2014) 192:3111–20. doi: 10.4049/jimmunol.1302313
66. Matos TR, O’Malley JT, Lowry EL, Hamm D, Kirsch IR, Robins HS, et al. Clinically Resolved Psoriatic Lesions Contain Psoriasis-Specific Il-17-producing Alphabeta T Cell Clones. J Clin Invest (2017) 127:4031–41. doi: 10.1172/JCI93396
67. Ickrath P, Kleinsasser N, Ding X, Ginzkey C, Beyersdorf N, Hagen R, et al. Accumulation of CD69+ Tissueresident Memory T Cells in the Nasal Polyps of Patients With Chronic Rhinosinusitis. Int J Mol Med (2018) 42:1116–24. doi: 10.3892/ijmm.2018.3653
68. Dutzan N, Kajikawa T, Abusleme L, Greenwell-Wild T, Zuazo CE, Ikeuchi T, et al. A Dysbiotic Microbiome Triggers Th17 Cells to Mediate Oral Mucosal Immunopathology in Mice and Humans. Sci Transl Med (2018) 10:eaat0797. doi: 10.1126/scitranslmed.aat0797
69. Ezzedine K, Eleftheriadou V, Whitton M, van Geel N. Vitiligo. Lancet (2015) 386:74–84. doi: 10.1016/S0140-6736(14)60763-7
70. Cheuk S, Schlums H, Gallais Serezal I, Martini E, Chiang SC, Marquardt N, et al. Cd49a Expression Defines Tissue-Resident Cd8(+) T Cells Poised for Cytotoxic Function in Human Skin. Immunity (2017) 46:287–300. doi: 10.1016/j.immuni.2017.01.009
71. He H, Suryawanshi H, Morozov P, Gay-Mimbrera J, Del Duca E, Kim HJ, et al. Single-Cell Transcriptome Analysis of Human Skin Identifies Novel Fibroblast Subpopulation and Enrichment of Immune Subsets in Atopic Dermatitis. J Allergy Clin Immunol (2020) 145:1615–28. doi: 10.1016/j.jaci.2020.01.042
72. Weidinger S, Novak N. Atopic Dermatitis. Lancet (2016) 387:1109–22. doi: 10.1016/S0140-6736(15)00149-X
73. Guttman-Yassky E, Krueger JG. Atopic Dermatitis and Psoriasis: Two Different Immune Diseases or One Spectrum? Curr Opin Immunol (2017) 48:68–73. doi: 10.1016/j.coi.2017.08.008
74. Dainichi T, Kitoh A, Otsuka A, Nakajima S, Nomura T, Kaplan DH, et al. The Epithelial Immune Microenvironment (EIME) in Atopic Dermatitis and Psoriasis. Nat Immunol (2018) 19:1286–98. doi: 10.1038/s41590-018-0256-2
75. Snyder ME, Farber DL. Human Lung Tissue Resident Memory T Cells in Health and Disease. Curr Opin Immunol (2019) 59:101–8. doi: 10.1016/j.coi.2019.05.011
76. Hondowicz BD, An D, Schenkel JM, Kim KS, Steach HR, Krishnamurty AT, et al. Interleukin-2-Dependent Allergen-Specific Tissue-Resident Memory Cells Drive Asthma. Immunity (2016) 44:155–66. doi: 10.1016/j.immuni.2015.11.004
77. Turner DL, Goldklang M, Cvetkovski F, Paik D, Trischler J, Barahona J, et al. Biased Generation and In Situ Activation of Lung Tissue-Resident Memory Cd4 T Cells in the Pathogenesis of Allergic Asthma. J Immunol (2018) 200:1561–9. doi: 10.4049/jimmunol.1700257
78. Gamradt P, Laoubi L, Nosbaum A, Mutez V, Lenief V, Grande S, et al. Inhibitory Checkpoint Receptors Control Cd8(+) Resident Memory T Cells to Prevent Skin Allergy. J Allergy Clin Immunol (2019) 143:2147–2157 e9. doi: 10.1016/j.jaci.2018.11.048
79. Roy S, Coulon PG, Prakash S, Srivastava R, Geertsema R, Dhanushkodi N, et al. Blockade of PD-1 and LAG-3 Immune Checkpoints Combined With Vaccination Restores the Function of Antiviral Tissue-Resident CD8(+) Trm Cells and Reduces Ocular Herpes Simplex Infection and Disease in HLA Transgenic Rabbits. J Virol (2019) 93:e00827. doi: 10.1128/JVI.00827-19
80. Wakim LM, Woodward-Davis A, Liu R, Hu Y, Villadangos J, Smyth G, et al. The Molecular Signature of Tissue Resident Memory Cd8 T Cells Isolated From the Brain. J Immunol (2012) 189:3462–71. doi: 10.4049/jimmunol.1201305
81. Mackay LK, Rahimpour A, Ma JZ, Collins N, Stock AT, Hafon ML, et al. The Developmental Pathway for CD103(+)CD8+ Tissue-Resident Memory T Cells of Skin. Nat Immunol (2013) 14:1294–301. doi: 10.1038/ni.2744
82. Aschenbrenner D, Foglierini M, Jarrossay D, Hu D, Weiner HL, Kuchroo VK, et al. An Immunoregulatory and Tissue-Residency Program Modulated by c-MAF in Human Th17 Cells. Nat Immunol (2018) 19:1126–36. doi: 10.1038/s41590-018-0200-5
83. Gasper DJ, Neldner B, Plisch EH, Rustom H, Carrow E, Imai H, et al. Effective Respiratory Cd8 T-Cell Immunity to Influenza Virus Induced by Intranasal Carbomer-Lecithin-Adjuvanted Non-replicating Vaccines. PloS Pathog (2016) 12:e1006064. doi: 10.1371/journal.ppat.1006064
84. Allen AC, Wilk MM, Misiak A, Borkner L, Murphy D, Mills KHG. Sustained Protective Immunity Against Bordetella Pertussis Nasal Colonization by Intranasal Immunization With a Vaccine-Adjuvant Combination That Induces IL-17-secreting Trm Cells. Mucosal Immunol (2018) 11:1763–76. doi: 10.1038/s41385-018-0080-x
85. Bedford JG, Caminschi I, Wakim LM. Intranasal Delivery of a Chitosan-Hydrogel Vaccine Generates Nasal Tissue Resident Memory Cd8(+) T Cells That are Protective Against Influenza Virus Infection. Vaccines (Basel) (2020) 8:572. doi: 10.3390/vaccines8040572
86. Louis L, Clark M, Wise MC, Glennie N, Wong A, Broderick K, et al. Intradermal Synthetic Dna Vaccination Generates Leishmania-Specific T Cells in the Skin and Protection Against Leishmania Major. Infect Immun (2019) 87:e00227. doi: 10.1128/IAI.00227-19
87. Galvez-Cancino F, Lopez E, Menares E, Diaz X, Flores C, Caceres P, et al. Vaccination-Induced Skin-Resident Memory Cd8(+) T Cells Mediate Strong Protection Against Cutaneous Melanoma. Oncoimmunology (2018) 7:e1442163. doi: 10.1080/2162402X.2018.1442163
88. Vela Ramirez JE, Sharpe LA, Peppas NA. Current State and Challenges in Developing Oral Vaccines. Adv Drug Delivery Rev (2017) 114:116–31. doi: 10.1016/j.addr.2017.04.008
89. Becattini S, Littmann ER, Seok R, Amoretti L, Fontana E, Wright R, et al. Enhancing Mucosal Immunity by Transient Microbiota Depletion. Nat Commun (2020) 11:4475. doi: 10.1038/s41467-020-18248-4
90. Shin H, Iwasaki A. A Vaccine Strategy That Protects Against Genital Herpes by Establishing Local Memory T Cells. Nature (2012) 491:463–7. doi: 10.1038/nature11522
91. Mackay LK, Stock AT, Ma JZ, Jones CM, Kent SJ, Mueller SN, et al. Long-Lived Epithelial Immunity by Tissue-Resident Memory T (Trm) Cells in the Absence of Persisting Local Antigen Presentation. Proc Natl Acad Sci USA (2012) 109:7037–42. doi: 10.1073/pnas.1202288109
92. Santosuosso M, McCormick S, Roediger E, Zhang X, Zganiacz A, Lichty BD, et al. Mucosal Luminal Manipulation of T Cell Geography Switches on Protective Efficacy by Otherwise Ineffective Parenteral Genetic Immunization. J Immunol (2007) 178:2387–95. doi: 10.4049/jimmunol.178.4.2387
93. Bernstein DI, Cardin RD, Bravo FJ, Awasthi S, Lu P, Pullum DA, et al. Successful Application of Prime and Pull Strategy for a Therapeutic Hsv Vaccine. NPJ Vaccines (2019) 4:33. doi: 10.1038/s41541-019-0129-1
94. Mackay LK, Wynne-Jones E, Freestone D, Pellicci DG, Mielke LA, Newman DM, et al. T-Box Transcription Factors Combine With the Cytokines Tgf-Beta and IL-15 to Control Tissue-Resident Memory T Cell Fate. Immunity (2015) 43:1101–11. doi: 10.1016/j.immuni.2015.11.008
95. Casey KA, Fraser KA, Schenkel JM, Moran A, Abt MC, Beura LK, et al. Antigen-Independent Differentiation and Maintenance of Effector-Like Resident Memory T Cells in Tissues. J Immunol (2012) 188:4866–75. doi: 10.4049/jimmunol.1200402
96. Zhang N, Bevan MJ. Transforming Growth Factor-Beta Signaling Controls the Formation and Maintenance of Gut-Resident Memory T Cells by Regulating Migration and Retention. Immunity (2013) 39:687–96. doi: 10.1016/j.immuni.2013.08.019
97. Skon CN, Lee JY, Anderson KG, Masopust D, Hogquist KA, Jameson SC. Transcriptional Downregulation of S1pr1 is Required for the Establishment of Resident Memory Cd8+ T Cells. Nat Immunol (2013) 14:1285–93. doi: 10.1038/ni.2745
98. Mani V, Bromley SK, Aijo T, Mora-Buch R, Carrizosa E, Warner RD, et al. Migratory DCs Activate TGF-beta to Precondition Naive Cd8(+) T Cells for Tissue-Resident Memory Fate. Science (2019) 366:eaav5728. doi: 10.1126/science.aav5728
99. Mackay LK, Minnich M, Kragten NA, Liao Y, Nota B, Seillet C, et al. Hobit and Blimp1 Instruct a Universal Transcriptional Program of Tissue Residency in Lymphocytes. Science (2016) 352:459–63. doi: 10.1126/science.aad2035
100. Milner JJ, Toma C, Yu B, Zhang K, Omilusik K, Phan AT, et al. Runx3 Programs Cd8(+) T Cell Residency in non-Lymphoid Tissues and Tumours. Nature (2017) 552:253–7. doi: 10.1038/nature24993
101. Hirai T, Yang Y, Zenke Y, Li H, Chaudhri VK, De La Cruz Diaz JS, et al. Competition for Active Tgfbeta Cytokine Allows for Selective Retention of Antigen-Specific Tissue- Resident Memory T Cells in the Epidermal Niche. Immunity (2021) 54:84–98.e5. doi: 10.1016/j.immuni.2020.10.022
102. Davies B, Prier JE, Jones CM, Gebhardt T, Carbone FR, Mackay LK. Cutting Edge: Tissue-Resident Memory T Cells Generated by Multiple Immunizations or Localized Deposition Provide Enhanced Immunity. J Immunol (2017) 198:2233–7. doi: 10.4049/jimmunol.1601367
103. Haddadi S, Vaseghi-Shanjani M, Yao Y, Afkhami S, D’Agostino MR, Zganiacz A, et al. Mucosal-Pull Induction of Lung-Resident Memory Cd8 T Cells in Parenteral Tb Vaccine-Primed Hosts Requires Cognate Antigens and CD4 T Cells. Front Immunol (2019) 10:2075. doi: 10.3389/fimmu.2019.02075
104. Wakim LM, Smith J, Caminschi I, Lahoud MH, Villadangos JA. Antibody-Targeted Vaccination to Lung Dendritic Cells Generates Tissue-Resident Memory Cd8 T Cells That are Highly Protective Against Influenza Virus Infection. Mucosal Immunol (2015) 8:1060–71. doi: 10.1038/mi.2014.133
Keywords: tissue-resident memory T cells (TRM), antifungal immunity, fungal commensals, pathogenic fungi, immunopathology, vaccine immunity (Min.5-Max. 8)
Citation: LeibundGut-Landmann S (2021) Tissue-Resident Memory T Cells in Antifungal Immunity. Front. Immunol. 12:693055. doi: 10.3389/fimmu.2021.693055
Received: 09 April 2021; Accepted: 10 May 2021;
Published: 25 May 2021.
Edited by:
Marc Swidergall, UCLA Department of Medicine, United StatesReviewed by:
Karl Kai McKinstry, University of Central Florida, United StatesKingston Mills, Trinity College Dublin, Ireland
Marcel Wuethrich, University of Wisconsin-Madison, United States
Copyright © 2021 LeibundGut-Landmann. This is an open-access article distributed under the terms of the Creative Commons Attribution License (CC BY). The use, distribution or reproduction in other forums is permitted, provided the original author(s) and the copyright owner(s) are credited and that the original publication in this journal is cited, in accordance with accepted academic practice. No use, distribution or reproduction is permitted which does not comply with these terms.
*Correspondence: Salomé LeibundGut-Landmann, c2Fsb21lLmxlaWJ1bmRndXQtbGFuZG1hbm5AdXpoLmNo