- 1College of Veterinary Medicine, Southwest University, Chongqing, China
- 2Guangdong Laboratory of Lingnan Modern Agriculture, Guangdong Provincial Key Laboratory of Animal Nutrition Control, Institute of Subtropical Animal Nutrition and Feed, College of Animal Science, South China Agricultural University, Guangzhou, China
- 3Institute of Subtropical Agriculture, Chinese Academy of Sciences, Changsha, China
- 4Johann Friedrich Blumenbach Institute of Zoology and Anthropology, University of Göttingen, Göttingen, Germany
Diseases caused by pathogenic bacteria in animals (e.g., bacterial pneumonia, meningitis and sepsis) and plants (e.g., bacterial wilt, angular spot and canker) lead to high prevalence and mortality, and decomposition of plant leaves, respectively. Melatonin, an endogenous molecule, is highly pleiotropic, and accumulating evidence supports the notion that melatonin’s actions in bacterial infection deserve particular attention. Here, we summarize the antibacterial effects of melatonin in vitro, in animals as well as plants, and discuss the potential mechanisms. Melatonin exerts antibacterial activities not only on classic gram-negative and -positive bacteria, but also on members of other bacterial groups, such as Mycobacterium tuberculosis. Protective actions against bacterial infections can occur at different levels. Direct actions of melatonin may occur only at very high concentrations, which is at the borderline of practical applicability. However, various indirect functions comprise activation of hosts’ defense mechanisms or, in sepsis, attenuation of bacterially induced inflammation. In plants, its antibacterial functions involve the mitogen-activated protein kinase (MAPK) pathway; in animals, protection by melatonin against bacterially induced damage is associated with inhibition or activation of various signaling pathways, including key regulators such as NF-κB, STAT-1, Nrf2, NLRP3 inflammasome, MAPK and TLR-2/4. Moreover, melatonin can reduce formation of reactive oxygen and nitrogen species (ROS, RNS), promote detoxification and protect mitochondrial damage. Altogether, we propose that melatonin could be an effective approach against various pathogenic bacterial infections.
Introduction
Bacteria include both pathogenic and beneficial species and are universally present in all ecosystems as colonizers of hosts (1). Bacteria can be beneficial in multiple ways, for example, Lactobacillus and Bifidobacterium species are used for yoghurt production (2, 3), Lactococcus species and others in cheese production (4, 5), methane producing bacteria for methane production (6), Corynebacteria for monosodium glutamate formation (7) and Acetobacter for vinegar generation (8). Bacteria, being a normal flora can also benefit hosts, such as, Lactobacillus species can inhibit inflammation by competing with pathogens or stimulating skin barrier recovery (9). Most, but not all bacteria present in the gut microbiome, which contains slightly more cells than a human body, can be also classified as being beneficial and relevant to health, as far as it possesses a favorable compostion (10). Pathogenic bacteria may cause mild, severe and even life-threatening diseases [e.g., septicemia (11), pneumonia (12) and meningitis (13)]. In terms of accessibility to treatments, the bacterial surface structures are of great importance. These are partially detectable by gram staining (14), according to which they are categorized as gram positive bacteria (GPB), gram negative bacteria (GNB), and others (15, 16). Examples of pathogenic GPB (PGPB) comprise Staphylococcus aureus (S. aureus) (17), Streptococcus pneumoniae (S. pneumoniae) (18) and Bacillus anthracis (B. anthracis) (19). Pathogenic GNB (PGNB) include Escherichia coli (E. coli) (20), Helicobacter pylori (H. pylori) (21), Pseudomonas aeruginosa (P. aeruginosa) (22), Acinetobacter baumannii (A. baumannii) (23), and Klebsiella pneumoniae (K. pneumoniae) (24). Another important pathogen, Mycobacterium tuberculosis (M. tuberculosis) (25) possesses a special waxy coating of its cell wall by mycolic acid, which largely prevents gram staining, although the cell wall structure resembles that of other GPBs and is, thus, devoid of an outer membrane (26). Also in genetic terms, Mycobacterium species are GPB-like, but the different surface has consequences to treatment.
Bacterial infections caused by pathogenic bacteria severely threaten public health worldwide (27, 28). Historically, the various antibiotics (e.g., penicillin, tetracycline and their derivatives as well as numerous other similarly acting compounds) usually represent the preferred armory for fighting bacterial infections (29). However, new problems (i.e., antibiotic resistance and residues) occur since the overuse and misuse of antibiotics (30–32); therefore, the development of new antibiotics that can effectively kill pathogenic bacteria and do not give rise to problematic metabolites is a matter of highest urgency. Given that very few compounds are currently under development or approval in the clinical setting, hence, repurposing compounds for novel application may become a productive alternate strategy for the combat against bacterial pathogens.
Melatonin, which shows a wide distribution within phylogenetically distant organisms from bacteria to humans (33), is synthesized from tryptophan and produced in pineal gland and in numerous other organs of vertebrates (e.g., gut, skin and bone marrow) (34–36). Melatonin is involved in many physiological processes, as summarized comprehensively (37, 38). In particular, this includes growth modulation and reproduction (39), immune regulation (40–43), anti-inflammation (44–47), antioxidative protection (48–51) and antioncogenic action (52, 53). Meanwhile, increasing evidence has accumulated for remarkable antibacterial actions of melatonin, including protection against damage by bacterial infections. For instance, melatonin has been reported to exert antibacterial effects in PGPB and PGNB in vitro, concerning P. aeruginosa, A. baumannii and S. aureus (54). Melatonin has also been shown to protect Arabidopsis and tobacco against Pseudomonas syringae pv. tomato DC3000 (Pst DC3000) (55), suggesting that melatonin reduces biotic stress by bacterial infection in plants. Also in mammals, several reports have demonstrated substantial effects of melatonin in protecting against or alleviating bacterial infections. In mice infected with S. aureus and E. coli, symptoms were strongly attenuated by melatonin (56). More impressive evidence has been obtained in septic mice treated with cecal ligation and puncture, in which the decisive effects of melatonin were related to inhibition of NO-mediated inflammation in connection with mitochondrial protection (57–59). Efficacy of melatonin was also multiply demonstrated in human sepsis, including that of neonates, which again underlines the excellent tolerability of this agent (60–62). Despite the emerging role of melatonin in fighting against bacterial infections, the protection mechanisms are only evident in the above-mentioned murine studies, plus additional anti-inflammatory effects that have been recently summarized (46, 63). Concerning protection against bacterial infections in plants, promising data have been obtained on the effects of stress-related genes and phytohormones, but still require further elucidation. Of note, with regard to these numerous highly valuable and beneficial organisms, which also represent an immunological quasi-self, one should melatonin not expect to generally act as an anti-bacterial agent. Moreover, various bacteria tested in this regard have been shown to synthesize themselves melatonin, sometimes in relevant quantities (64, 65). Among intestinal bacteria, the formation of melatonin was first described in Escherichia coli (66). Bacteria are even regarded as the evolutionary source of melatonin in eukaryotes, in connection with the uptake of α-proteobacteria and cyanobacteria as ancestors of mitochondria and plastids, respectively (67, 68). This has an important consequence for the consideration of melatonin as a bacteria-controlling agent. As many bacteria synthesize this compound or live, at least, in close community with melatonin-producing microbes, one cannot expect anti-bacterial effects at low doses of melatonin to which these organisms are exposed anyway under physiological conditions. However, this does not hinder bacteriostatic actions at elevated concentrations and protection of hosts from damage by bacteria or by inflammatory responses induced by bacterial challenges.
In this review, we summarize the findings related to the antibacterial actions of melatonin in vitro and in vivo. Thereafter, we detailedly discuss the potential mechanisms whereby melatonin influences pathogenic bacteria as well as their deleterious effects on hosts. These concern: 1) in vitro: formation and detoxification of free radicals; regulation of bacterial replication by interference with the cell wall and exhaustion of intracellular substrates and micronutrients (e.g., iron); blockade of bacterial glucose and glutamate metabolism as related to bacterial cell division; 2) in plants: upregulation of genes related to phytohormone-dependent defense signaling as well as MAPK signaling; 3) in animals: involvement of multiple signaling pathways (e.g., NF-κB, STAT1 and Nrf2) related to anti-inflammatory and antioxidant control and mitochondrial protection. The review highlights the huge potential of melatonin in counteracting the growing threat of bacterial infections.
The Basic Physiology, Pathophysiology and Clinical Safety of Melatonin
Melatonin is a methoxyindole, mainly synthesized and secreted by the pineal gland at night under normal light and dark conditions, the main physiological functions of melatonin are related to hormonal properties (69). Melatonin transmits the information "darkness" and contributes to the synchronization of circadian oscillators (70), which is an important physiological sleep regulator in diurnal species including humans (71). In addition, melatonin is involved in numerous other physiological processes, such as regulation of blood pressure (72), glucose (73), and body temperature (74), suppression of oncogenesis (75), immune function (41), oxidative stress and inflammation (76). It is recognized that the "physiological" dose is the same as the peak plasma melatonin level at night, and the difference between the physiological and pharmacological effects of melatonin is not always clear, but is based on the consideration of the dosage rather than the duration of the hormone message (77). Indeed, the secretion of melatonin can be disturbed in the context of many pathophysiological conditions, which may increase the susceptibilities of the diseases (including infectious diseases) as well as increase the severity of symptoms or change the courses and outcomes of the diseases (78).
Of note, safety is important when melatonin is considered for clinical treatment. Clinically, 3mg, 6 mg and 10 mg melatonin showed satisfactory safety in patients (79–81). Moreover, there was no side effect of 1g/d melatonin for a month in humans (82). It has been shown that melatonin doses up to 800 mg/kg failed to cause any death in mice and it was impossible to obtain its LD50 (median lethal dose) in rats (83, 84). In study on Amyotrophic lateral sclerosis (ALS) patients, melatonin 300 mg/day was applied for 2 years and found to be safe (85). However, a trial of long-term controlled melatonin release for the treatment of sleep disorders in children with neurodevelopmental disabilities reported mild adverse effects, including seizures, cold/flu/infection, gastro-intestinal illness, agitation, anxiety and headache (86). In addition, the short- and intermediate-term administration of melatonin produced only minor adverse effects such as agitation, dizziness, headache, nausea and sleepiness in clinical studies on children; in clinical studies on adults, dizziness, paresthesias in the mouth, arms or legs, mild headaches, numbness and dyspnea aggravated; psychomotor impairment, sedation, disorientation, and amnesia in surgical patients; mild headache, increased sleepiness and skin rash in critically ill patients; daytime sleepiness in elderly (87, 88). Moreover, a recent systematic review showed that the most frequently reported melatonin adverse effects were daytime sleepiness (1.66%), dizziness (0.74%), headache (0.74%), other sleep-related adverse events (0.74%), and hypothermia (0.62%); but serious or clinical significance adverse events, including agitation, palpitations, nightmares, mood swings, fatigue, and skin irritation, were very few (89). Therefore, compared to other antibiotics, as a natural small molecule, melatonin is relatively safe with a low risk of side effects. However, the effect and safety of melatonin should be carefully monitored when melatonin is used clinically.
Bacteriostatic Actions of Melatonin In Vitro and Their Underlying Mechanisms
Historically, a great diversity of studies aimed to investigate whether melatonin does possess bacteriostatic actions. Initially, melatonin has only been shown to strongly reduce the lipid levels of the yeast Candida albicans (C. albicans) (90). Subsequently, one comparative study found that some pineal indoles that share a similar structure with melatonin possess antibacterial activities. For instance, 5-methoxytryptamine (5-MT), which is both a metabolite and precursor of melatonin (65), exhibits antibacterial actions against S. aureus and Bacillus subtilis (B. subtilis), and 6-methoxy-2-benzoxazolinone (6-MBOA) against Proteus vulgaris (P. vulgaris). However, no corresponding antibacterial effect was observed in the case of melatonin (91). Later, it has been reported that melatonin (31.25~125 mg/mL: 0.13-0.53 mM) is able to suppress the growth of PGPB (e.g., S. aureus) and PGNB (e.g., P. aeruginosa and A. baumannii) by reducing key intracellular substrates in vitro (54). Intriguingly, melatonin could also inhibit the growth of the atypical GPB M. tuberculosis, while the exact mechanism has not yet been identified (92). In this section, we present further evidence for antibacterial activities of melatonin against PGPBs and PGNBs in vitro, and we also discuss the potential mechanisms.
The antimicrobial resistance among GPBs (chiefly S. aureus, Enterococcus faecium, Enterococcus faecalis and Streptococcus pneumoniae) has become a serious threat to public health, spurring the development of new compounds against infections (93, 94). In an early study, melatonin had been shown to moderately inhibit the growth of S. aureus (95). This was later also reported for the methicillin-resistant S. aureus (MRSA), but this required concentrations in the millimolar range (54). However, another investigation showed that the antibacterial action against S. aureus and/or MRSA of flouroquinolones was substantially diminished by melatonin via reduction of oxidative stress in the bacterial cells (96). Additional bacteria had been also tested in some studies (54, 95, 96), but melatonin’s efficiency against other important PGPBs, such as Enterococcus faecium, Enterococcus faecalis and Streptococcus pneumoniae remains to be demonstrated.
PGNBs are also a major challenge in public health, such as P. aeruginosa (97) and A. baumannii (98). P. aeruginosa is one of the main opportunistic pathogenic bacteria in hospitals (99, 100), which often results in post-operative (101) or post-burn wound infections (102), bacteremia (103) and sepsis (104). Antimicrobial resistance also exists in P. aeruginosa, it has been discovered that melatonin has a direct suppressive role in carbapenem-resistant P. aeruginosa (54). CBR-4830 (a tricyclic indole analog which shows chemical similarity to melatonin), was supposed to suppress the growth of P. aeruginosa (105). A. baumannii, is a conditional pathogen (106), which often leads to pneumonia (107), meningitis (108) and bacteremia (109). It causes hospital infection frequently (110) and is often resistant to a variety of antimicrobial agents (111), bringing great difficulties to the clinical treatment. Fortunately, melatonin effectively inhibits the growth of A. baumannii (54), suggesting it may be suitable as adjunctive therapy in hospital infection. Similarly, we also found that melatonin could inhibit the growth of enterotoxigenic Escherichia coli (ETEC) and Pasteurella multocida serotype A strain CQ2 (PmCQ2) (unpublished data). Of note, melatonin was reported to moderately reduce surface hydrophobicity, a characteristic associated with the colonization of mammalian epithelia, inhibiting the adherence of E.coli; however, the reduction of surface hydrophobicity in E. coli remained at borderline and was only observed at high concentrations (0.2 mM) (112). In contrast, another study by the same group reported that melatonin instead increased cell surface hydrophobicity of Neisseria meningitidis (113). Thus, the influence of melatonin on surface hydrophobicity is obviously variable, which would require a convincing explanation, especially as the authors had tried to relate these effects to melatonin’s antioxidant properties (112).
M. tuberculosis, an atypical PGPB whose cell wall is coated by mycolic acid (26), is the main pathogen of tuberculosis (TB) in humans (25, 114) and various mammals (115, 116). In addition to dormancy and persistence, drug resistance is a major obstacle in the treatment of TB (117). Therefore, it is urgent to seek for sensitive anti-TB drugs or methods to enhance the sensibility of existing antimicrobial agents. In the earlier literatures, the protective function of melatonin against M. tuberculosis was evaluated with isoniazid in vitro, experiments that did not reveal significant growth inhibitions by either isoniazid (0.005 to 0.01 μg/mL) or melatonin (0.26 nM to 2.6 nM) alone, but by a combination of isoniazid (0.005 μg/mL) and melatonin (0.01 mg/mL) (61, 92). Furthermore, the bacteriostatic capacity of melatonin was also reported for M. tuberculosis (3×107 CFU/mL, 3×105 CFU/mL, 3×103 CFU/mL) treated with different concentrations of melatonin (0.05, 0.1, 0.2 and 0.4 mg/mL) in vitro (118). However, the extent of inhibition was not quantified in that study. Collectively, melatonin can affect pathogenic bacteria in some cases directly (e.g., inhibition of growth and adherence), which has been summarized in Table 1. It is likely concluded that the effectiveness of melatonin on pathogenic bacteria is obvioulsy variable, and may depend largely on its working concentrations. Current studies have centered on melatonin’s antibacterial activities on GNB, while the bacteriostatic capability of melatonin against other specific bacteria (especially antimicrobial resistant-GNB) is still poorly characterized.
As mentioned above, melatonin could exhibit bacteriostatic action against pathogenic bacteria; however, the potential mechanisms are not fully understood. Actually, there are two main hypotheses about bacteriostatic mechanisms of melatonin in vitro. It has been well documented that the growth of bacteria urgently requires metals, particularly free iron (121). Considering that melatonin has a high metal binding capacity, including iron, copper and zinc (122), thus, the addition of melatonin may reduce cytoplasmic availability of metal ions in bacteria to achieve the bacteriostatic effects (Figure 1A). Moreover, the membrane of bacteria is imbued with phospholipids (123, 124), and study has shown that melatonin can restrict the absorb of Linoleic acid (LA) used for facilitating cell proliferation (125). Therefore, melatonin may inhibit proliferation of bacteria by blocking the getting of bacterial growth factors (Figure 1B). These convincing findings indicate that melatonin inhibits the bacterial growth may through reducing intracellular substrates (54). Interestingly, a recent study declared that melatonin inhibits bacterial growth and proliferation by regulating the expression of genes associated with cell division (Figure 1C) and suppressing contents as well as activities of metabolism-related enzymes (Figure 1D) (119). These interesting results imply that melatonin could affect bacteria physiological condition by intrinsic molecular mechanisms; however, the experimental validation is still needed. Of note, our recent findings also demonstrated that melatonin exerts antibacterial activity against GNB (e.g., Klebsiella, Pasteurella multocida and Pseudomonas aeruginosa) through specifically inhibiting the activity of bacterial citrate synthase (Figure 1E), and the combination of colistin with melatonin enhances bacterial outer membrane permeability, oxidative damage and inhibits the effect of efflux pumps (Figure 1F) (126).
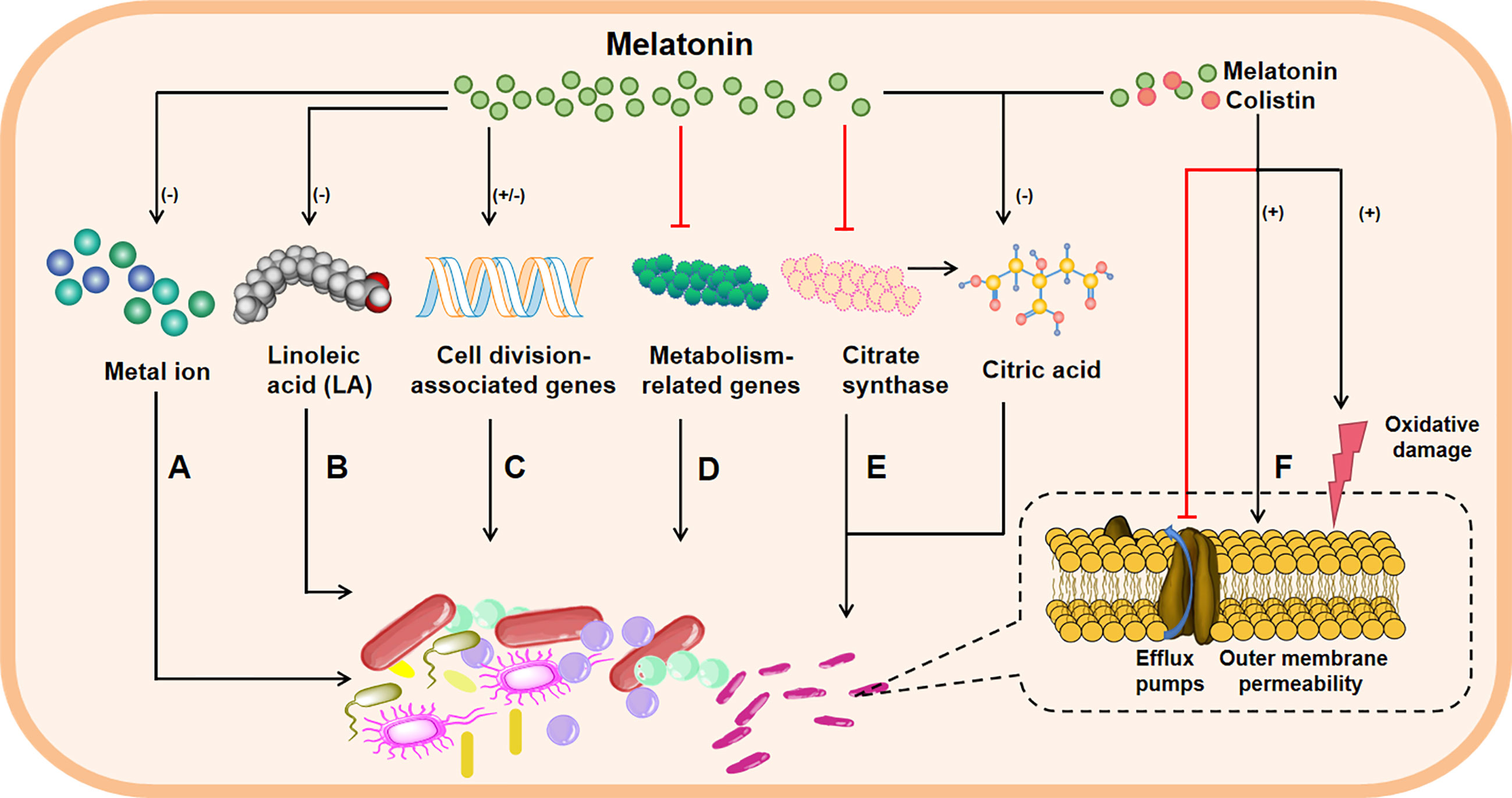
Figure 1 The possible bacteriostatic mechanisms of melatonin in vitro. Melatonin inhibits the growth of bacteria through these avenues: reducing cytoplasmic availability of metal ion (A); interfering cell wall formation, for example, melatonin restricts the absorb of Linoleic acid (LA), which makes up the cell wall, thus limiting cell proliferation (B); melatonin can also regulate the expressions of genes associated with cell division (C) or suppress the content and activity of metabolism-related enzymes (D) to inhibit bacterial growth and proliferation; melatonin resists against Gram-negative bacteria through inhibiting bacterial citrate synthase and reducing the synthesis of citric acid (E), besides, when melatonin is combination with colistin, bacterial outer membrane permeability and oxidative damage are enhanced, and the effect of efflux pumps is inhibited (F), leading to increased bacteria damage. Note: minus in parentheses represents decrease; plus in parentheses represents increase; and the red line represents inhibition; the black arrow indicates activation.
Bacteriostatic Actions of Melatonin In Vivo and Their Underlying Mechanisms
Melatonin not only has antibacterial effect in vitro, but also plays a significant role in the clinical prevention and treatment of bacterial infections in vivo (61). The inhibitory effects of melatonin in vivo includes both on PGPB [e.g., S. aureus (56) and S. pneumoniae (127)] and PGNB [e.g., E.coli (128), H. Pylori (129) and K. pneumonia (130)]. For example, in many GNB-infected animal models (e.g., sepsis), melatonin has a favorable effect in improving survival rates, ameliorating tissue damage and reducing levels of pro-inflammation mediators (e.g., TNF-α and IFN-γ), and increasing levels of anti-inflammation mediators (e.g., IL-10) (131, 132). Notably, various signaling have been described to shape bacterial infection in vivo by melatonin, including NF-κB, Nrf2, NLRP3 and ROS pathways. In this section, firstly, we present the evidence from studies that have used melatonin to resist bacterial infections in vivo. Subsequently, we illustrate the possible mechanisms.
S. aureus always causes many serious infections in human (133, 134). Virtually, melatonin not only inhibits the growth of S. aureus in vitro, but also has significant defensive effects against S. aureus-induced infection in vivo. For example, melatonin reduces lipid peroxidation (LPO), catalase (CAT), neutrophil recruitment, and TNF-α, IFN-γ, IL-6, iNOS, COX-2 and C-reactive protein (CRP), while increases superoxide dismutase (SOD) and glutathione (GSH) in animals infected by S. aureus (5 × 106 CFU/mL) (56). The pathogenicity of S. pneumoniae is second only to S. aureus in pyogenic cocci (135), which triggers major lobe pneumonia (136), meningitis (137), bronchitis (138). Fortunately, it has found that melatonin has a certain inhibitory effect on the infection of S. pneumonia (95). These aforementioned findings indicate that melatonin can target GPB to alleviate infections in vivo. However, it has been shown that melatonin therapy fails to reduce neuronal injury in S. pneumoniae-infected rabbit model, the possible reason may due to the 12 h delay in the administration of melatonin after the infection (139).
Historically, E. coli has been regarded as an integral part of the normal intestinal flora (140), and was considered to be non-pathogenic bacteria (141). Nevertheless, as the research moves along, some special serotypes of E. coli [e.g., ETEC (142), enterohemorrhagic E. coli (EHEC) (143) and enteropathogenic E. coli (EPEC) (144)] are shown to be pathogenic to humans and animals (especially infants and young animals) (145), causing severe diarrhea (146) and sepsis (147). Some studies have found that melatonin has a bacteriostatic effect in the animals infected by E. coli, for instance, melatonin reduces LPO, CAT, neutrophil recruitment, and TNF-α, IFN-γ, IL-6, iNOS, COX-2 and CRP, while increases SOD and GSH in animals infected by E. coli (2.5 × 107 CFU/mL) (56, 148). H. Pylori is the only microbial species known to survive in the human stomach (149) to induce gastritis (150), gastrointestinal hemorrhage (151) and gastric lymphoma (152). It has been revealed that H. Pylori infection inhibits gastric mucosal melatonin synthesizing enzymes [e.g., arylalkylamine-N-acetyltransferase (AANAT) and N-acetylserotonin O-methyltransferase (ASMT)] expression (153). Interestingly, melatonin facilitates H. pylori eradication in patients with gastroduodenal ulcer by omeprazole treatment (154–156), indicating melatonin can be used as an adjuvant clinical drug against H. Pylori infection. Furthermore, K. pneumonia is also a member of PGNB, isolated from the sputum of patients with pneumonia (157), mainly leads to pneumonia (158), septicemia (159) or bacteremia (160), meningitis (161) and peritonitis (162). An experimental study showed that the supply of 100 mg/kg of melatonin can reduce pro-inflammatory cytokines, inhibit microglial activation, and counteract neurocognitive damage in K. pneumoniae-infected rats (130). Likewise, we also found that melatonin can inhibit macrophage-mediated excessive inflammatory responses in Pasteurella multocida (PmCQ2)-infected mice (unpublished data). Of note, like GPB S. pneumoniae, the effect of melatonin on inactivated Pasteurella multocida (P52 strain) vaccine-mediated immune responses is time-dependent evidenced by exogenous melatonin administration at 4 h post vaccination augments immune responses in rats in comparison to 16 h post vaccination (163, 164).
Notably, melatonin has been demonstrated to increase survival rates and improve organ function in several sepsis models (165, 166). The oxidative imbalance is one of the characteristics of sepsis (167), and mitochondria play key roles in regulating sepsis-related redox dysregulation (168). Mechanistically, melatonin can alleviate sepsis symptoms by preventing mitochondria dysfunction via ROS/RNS scavenging (169) and many other pathways (e.g., intra-mitochondrial SIRT3 and MAPK/ERK pathway) (170–176). Inflammation is essential for the host to resist infection by pathogenic bacteria, however, excessive inflammation is another characteristic of the initial stage of sepsis, leading to organ dysfunction and eventually death (177, 178).
Melatonin as a signal molecule of stress can be induced by the pathogenic (but not the beneficial) bacteria invasion and the increased melatonin level in hosts can improve the protective effects or tolerance to the bacteria (179). In addition to inhibiting pathogenic bacteria, melatonin has a beneficial effect on intestinal flora (180, 181). For example, melatonin reprogramming of gut microbiota improves lipid dysmetabolism to prevent obesity in mice (182–185). Moreover, melatonin contributes to reshape gut microbiota to alleviate neuroinflammation and metabolic disorder in DSS-induced depression rats (186). Furthermore, melatonin ameliorates ochratoxin A-induced liver inflammation, oxidative stress and mitophagy involving in intestinal microbiota in mice (187). Altogether, as summarize in Table 2, melatonin may function as a novel compound to resist pathogenic bacterial infections in vivo. However, the defensive effects of melatonin against pathogenic bacteria are likely dose- and/or time-dependent.
Notably, the mechanisms whereby melatonin exerts bacteriostatic action in vivo are tightly associated with the immune responses, for example, during polymicrobial infection, melatonin treatment could promote the development of the neutrophil extracellular trap (NET), whereas inhibits the phagocytic activities of neutrophils (188). Indeed, mounting evidences suggest that melatonin always exerts its physiological effects via its receptors (e.g., MT1 and MT2). It has been discovered that melatonin is able to improve the survival rate of polymicrobial sepsis of mice through MT1 and MT2 receptor (Figure 2A) (189). Melatonin also alleviates acute lung injury induced by LPS via inhibiting the activation of NLRP3 inflammasome (Figure 2B) (190). Melatonin could dose-dependently reduce pro-inflammatory cytokine TNF-α, IL-6 and IL-8, increase anti-inflammatory cytokine IL-10 and improve survival, which are associated with p38MAPK and NF-κB signaling pathway (173, 191–195) (Figure 2C). Moreover, melatonin could block LPS (which is from P. intermedia)-induced activation of NF-κB signaling (Figure 2D) and STAT1 pathway (Figure 2E), thereby inhibiting the production of inflammatory mediators (e.g., NO and IL-6) (196, 197). Likewise, it has found that melatonin can reduce pro-inflammatory mediators [e.g., IL-1β, IL-6, NO and granulocyte-monocyte colony-stimulating factor (GM-CSF)], while increase anti-inflammatory cytokine (e.g., IL-10) by activating Nrf2 pathway (Figure 2F) (198). Actually, melatonin treatment can also alleviate H. pylori-induced gastritis through regulating TGF-β1 and Foxp3 expression via the suppression of TLR2 and activation of TLR4 (Figure 2G) (129), although the molecular target of melatonin in the TLR signaling warrants further investigation.
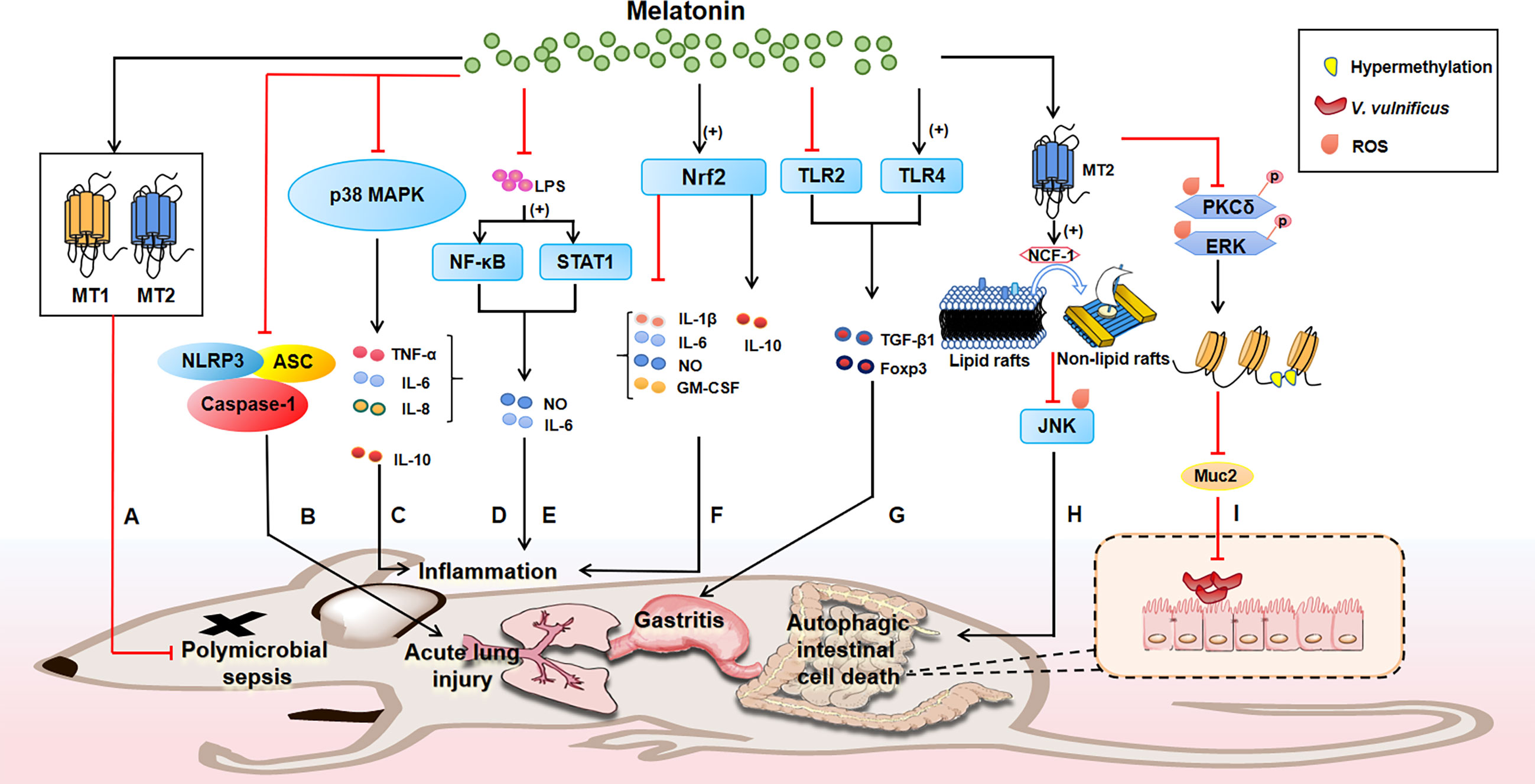
Figure 2 The bacteriostatic mechanisms of melatonin in animals. Melatonin receptors (e.g., MT1 and MT2) play a great role in mediating the functions of melatonin. Melatonin reduces polymicrobial sepsis through MT1 and MT2, thus improving the survival rate of mice (A); notably, the mechanisms whereby melatonin exerts bacteriostatic action in vivo are tightly associated with the immune responses, for instance, melatonin inhibits NLRP3 inflammasome to alleviate acute lung injury (B); melatonin reduces pro-inflammatory cytokines, increases anti-inflammatory cytokines and improves survival through p38MAPK signaling pathway (C); melatonin blocks LPS-induced activation of NF-κB (D) and STAT1 (E), thus inhibiting the production of inflammatory factors to modulate inflammation. Likewise, suppression of inflammation also occurs when melatonin activates Nrf2, evidenced by reduced pro-inflammatory mediators (e.g., IL-1β, IL-6, NO and GM-CSF) and increased anti-inflammatory cytokine (e.g., IL-10) (F); melatonin treatment can target TLR to alleviate H. pylori-induced gastritis by promoting TLR4 and inhibiting TLR2 to regulate TGF-β1 and Foxp3 expression (G); of note, melatonin could be a therapeutic alternative agent to fight bacterial infections due to its antioxidant function. For example, melatonin signaling via MT2 promotes NCF-1 recruitment from lipid rafts to non-lipid rafts to block the ROS-mediated JNK pathway, preventing autophagic intestinal cell death (H); moreover, MT2 signaling inhibits the ROS-mediated phosphorylation of PKCδ and ERK to reduce region-specific hypermethylation in the Muc2 promoter, combating V. vulnificus infection (I). Note: plus in parentheses represents increase; and the red line represents inhibition; the black arrow indicates activation.
Moreover, it should be noted that melatonin could be a therapeutic alternative agent to fight bacterial infections due to its antioxidant function. Intriguingly, melatonin inhibits apoptotic cell dealth in colonic epithelial cells induced by Vibrio vulnificus VvhA via MT2 (199). Mechanistically, melatonin signaling via MT2 stimulates NCF-1 recruitment into non-lipid rafts from lipid rafts to block the ROS-mediated JNK pathway, preventing rVvhA-induced apoptosis and autophagic intestinal cell death (Figure 2H). Similarly, melatonin treatment maintains the expression level of Muc2 in the intestine of V. vulnificus-infected mouse. Mechanistically, melatonin inhibits the ROS-mediated phosphorylation of PKCδ and ERK responsible for region-specific hypermethylation in the Muc2 promoter via MT2 receptor, restoring the level of Muc2 production in intestinal epithelial cells to resist V. vulnificus infection (Figure 2I) (200).
Collectively, melatonin could exert its bacteriostatic action in vivo by various potential mechanisms, including NF-κB, STAT1, Nrf2, TLR2/4, and ROS signaling (Figure 2). These aforementioned findings may provide promising strategies for controlling many diseases of public health importance. It should not be neglected that melatonin has a significant antibacterial effect in vitro; thus, whether the powerful effect of melatonin in vivo is also directly inhibiting bacterial growth remains further exploration.
Bacteriostatic Actions of Melatonin in Plants and Their Underlying Mechanisms
In addition to the significant bacteriostatic functions of melatonin in vitro and in animals, melatonin also exerts similar function against pathogenic bacterial infections in plants (201, 202). It has found that melatonin treatment inhibits the growth, motility and capsule formation of the bacterium Xanthomonas oryzae pv. oryzae (Xoo) (119), which leads to bacterial blight in rice (203, 204). Melatonin increases the resistance to Verticillium dahlia in cotton by regulating lignin and gossypol biosynthesis (205), although the molecular mechanisms are still not available. Authentically, it has been demonstrated that Serotonin N-acetyltransferase (SNAT)-deficient Arabidopsis shows lower melatonin levels and exhibits susceptibility to pathogen infection (206). Conformably, melatonin can trigger defense responses against Pst DC3000 infection in Arabidopsis and/or tobacco (55, 207, 208). Mechanistically, melatonin-induced rise of NO that favors in the expression of salicylic acid (SA)-related genes (e.g., AtEDS1, AtPAD4, AtPR1, AtPR2 and AtPR5), conferring improved disease resistance against Pst DC3000 infection in Arabidopsis (Figure 3); however, the beneficial effects of melatonin could be jeopardized by using a NO scavenger (cPTIO) and/or lost in NO-deficient mutants of Arabidopsis (209). Furthermore, it has been discovered that melatonin can active MPK3 and MPK6 signaling (members of MAPKs), which are independent of G-protein and Ca2+ signaling, and the inhibition of MPK3 and/or MPK6 induces reduced expression of defense and pathogen resistance-related genes (e.g. PR1, PR2, and PR5) (55). Indeed, melatonin activates MPK3 and MPK6 via four MKKs (i.e., MKK4/5/7/9) (210), and MAPKKK 3 and OXI 1 (oxidative signal-inducible 1) kinases are responsible for triggering melatonin-induced defense signaling pathways (211). Therefore, melatonin could mediate pathogen resistance in Arabidopsis and tobacco by activating MAPKs signaling via MKK4/5/7/9-MPK3/6 cascades through the activation of MAPKKK 3 and OXI 1 (Figure 3). In conclusion, melatonin plays a critical bacteriostatic role in Pst DC3000-infected Arabidopsis by MAPKs pathway. Moreover, melatonin could improve cell wall strengthening and callose-depositing factors (cellulose, xylose, and galactose) by increasing cell wall invertase (CWI) activity in Arabidopsis infected with Pst. DC3000 (Figure 3) (207). However, whether melatonin could function as a signaling molecule in modulating defense responses of other plants infected by various pathogenic bacteria are still poorly defined.
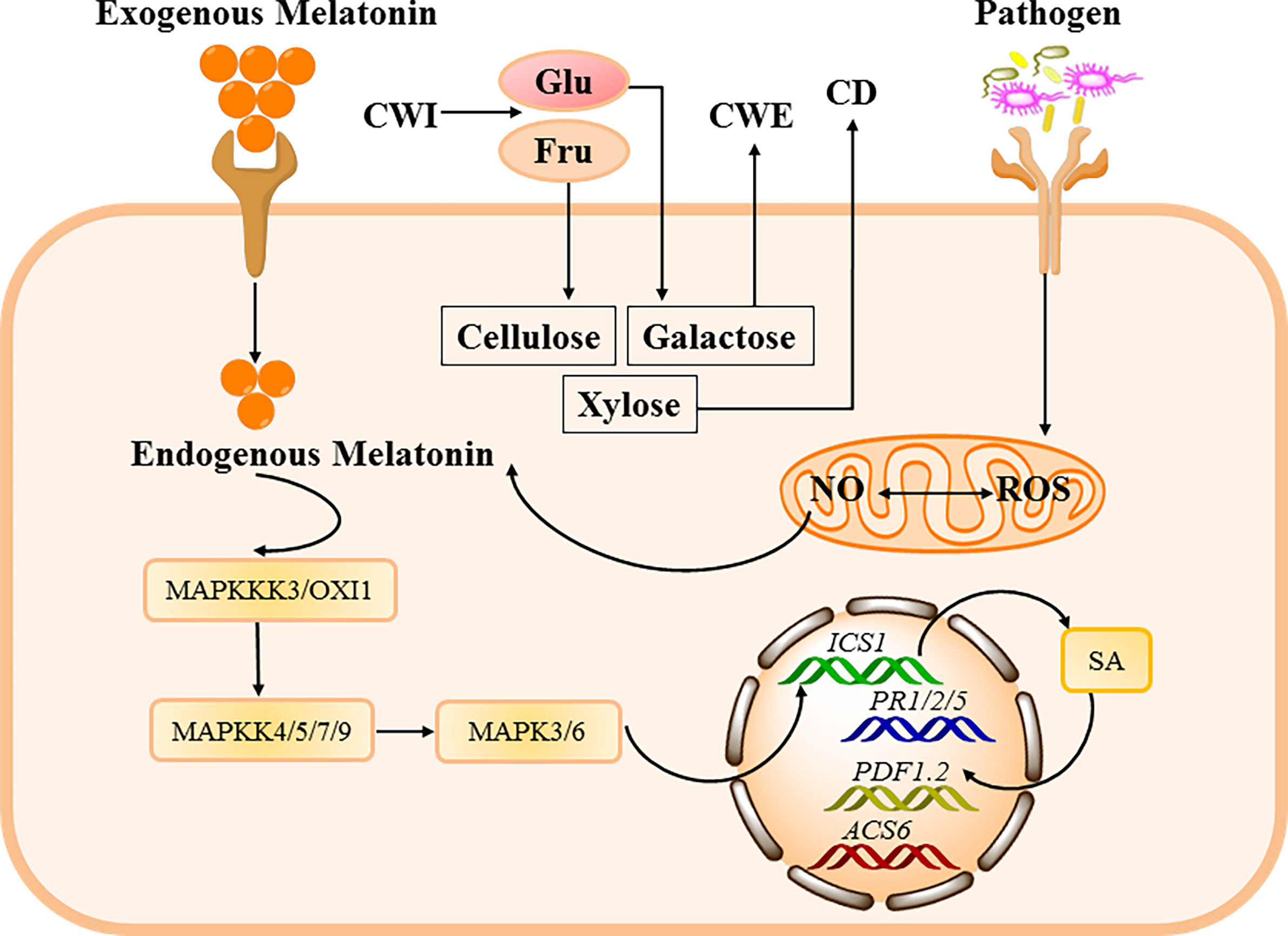
Figure 3 The bacteriostatic mechanisms of melatonin in plants. Antibacterial mechanisms against plant pathogenic bacteria are characterized by up-regulation of defense genes such as plant defensin1.2 (PDF1.2), plant resistance 1/2/5 (PR1/2/5), and 1-aminocyclopropane-1-carboxylate synthase 6 (ACS6) through several signal transduction pathways, including augmentation of NO levels in plants, which collaborates with melatonin in up-regulating SA. Moreover, melatonin can stimulate mitogen-activated protein kinases (MAPKs) cascades, which in turn up-regulate SA biosynthesis gene isochorismate synthase 1 (ICS1). Additionally, high cell wall invertase (CWI) activity within melatonin-treated Arabidopsis leads to improved cell wall strengthening and callose-depositing factors (cellulose, xylose, and galactose).
Concluding Remarks
Melatonin has multifarious functions, like circadian rhythm regulation (212, 213); anti-inflammatory/anti-tumor effects (214, 215); and, with particular relevance to this article, anti-bacterial function. Here, we summarize melatonin can directly influence bacteria in vitro (e.g., inhibition of growth) by reducing intracellular substrates. Considering that intestinal bacteria, Enterobacter aerogenes, responds to the melatonin by an increase in swarming activity, which is expressed rhythmically (216); thus, it is meaningful to further investigate the potential effects of melatonin in synchronizing bacterial rhythms in vitro. Notably, melatonin resists pathogenic bacterial infections in vivo by various pathways, such as NF-κB, TLR2/4, and ROS. Other pathways seem to get involved as well, but further experimental validation is needed. Furthermore, intestinal microbiota play a crucial role in the progress of different diseases, including bacterial infections in vivo (183, 217); therefore, it is necessary to further investigate the intestinal microbiota-mediated defensive roles of melatonin in attenuating bacterial infections in vivo. Melatonin serves as a signaling molecule to resist bacterial infections in Arabidopsis mainly through MAPK pathway, whether other specific pathways connecting to the bacteriostatic actions of melatonin in other plants or even other specific bacteria also remain an open question.
In addition to its significant antibacterial effect, it is thought that melatonin plays crucial roles in the fighting viral infections (61). Melatonin was found to effective against Ebola hemorrhagic shock syndrome by inhibiting Rho/ROCK signaling (218). Melatonin was also shown to protect mice infected with the Venezuelan equine encephalitis virus (VEEV) by reducing viral loads, brain apoptosis and oxidative stress (219, 220). Additionally, melatonin also was reported to exert protective and therapeutic effects against hemorrhagic disease virus (221), respiratory syncytial virus (RSV) (222), aleutian disease virus (223) and influenza virus (224). Recently, melatonin was proposed to be a potential candidate drug as an adjuvant treatment for patients with COVID-19 based on its antioxidant, anti-inflammatory and immunomodulatory properties (81, 225–227).
Overall, this review highlights melatonin as a novel and feasible preventive and therapy to tackle the increasing threat by bacterial infections. Based on the various physiological/pharmacological functions of melatonin and its significant anti-pathogenic effects, melatonin can be possibly used as clinical agent against pathogens and even viruses in the future.
Author Contributions
FH, XW, and YX designed and wrote the review article. PL, SC, RH, and YX revised the review article. FH, XW, QZ, YL, YY, PL, SC, and YP helped with designing figures and finding relevant literatures. YX approved the final manuscript. All authors contributed to the article and approved the submitted version.
Funding
This work was supported by Ministry of China Agriculture & Rural Affairs and Finance: the National Modern Agricultural Industry Technology System (Beef/Yak Cattle, CARS-37), and Chongqing Science & Technology Commission (cstc2017jcyjAX0288).
Conflict of Interest
The authors declare that the research was conducted in the absence of any commercial or financial relationships that could be construed as a potential conflict of interest.
Acknowledgments
Our profound admiration and respect go to researchers in this field and in our laboratories, for their dedication and hard work. We apologize to scientists whose work is in this field if their papers are not cited owing to space limitations.
Abbreviations
GPB, gram positive bacteria; GNB, gram negative bacteria; PGPB, pathogenic gram positive bacteria; PGNB, Pathogenic gram negative bacteria; S. aureus, Staphylococcus aureus; S. pneumoniae, Streptococcus pneumoniae; B. anthracis, Bacillus anthracis; E. coli, Escherichia coli; H. pylori, Helicobacter pylori; P. aeruginosa, Pseudomonas aeruginosa; A. baumannii, Acinetobacter baumannii; K. pneumoniae, Klebsiella pneumoniae; M. tuberculosis, Mycobacterium tuberculosis; C. albicans, Candida albicans; B. subtilis, Bacillus subtilis; P. vulgaris, Proteus vulgaris; MRSA, methicillin-resistant S. aureus; ALS, Amyotrophic lateral sclerosis; 5-MT, 5-methoxytryptamine; 6-MBOA, 6-methoxy-2-benzoxazolinone; ETEC, enterotoxigenic Escherichia coli; EHEC, enterohemorrhagic E. coli; EPEC, enteropathogenic E. coli; Xoo: Xanthomonas oryzae pv. Oryzae; AANAT, arylalkylamine-N-acetyltransferase; ASMT, N-acetylserotonin O-methyltransferase; PmCQ2, Pasteurella multocida serotype A strain CQ2; TB, tuberculosis; LA, Linoleic acid; LPO, peroxidation; CAT, catalase; CRP, C-reactive protein; SOD, superoxide dismutase; GSH, glutathione; NET, neutrophil extracellular trap; GM-CSF, granulocyte-monocyte colony-stimulating factor; OXI 1, oxidative signal-inducible.
References
1. Trdá L, Boutrot F, Claverie J, Brulé D, Dorey S, Poinssot B. Perception of Pathogenic or Beneficial Bacteria and Their Evasion of Host Immunity: Pattern Recognition Receptors in the Frontline. Front Plant Sci (2015) 6:219. doi: 10.3389/fpls.2015.00219
2. Jawad KI, Razavi SH. Characterization of Lactobacillus Plantarum as a Potential Probiotic In Vitro and Use of a Dairy Product (Yogurt) as Food Carrier. Appl Food Biotechnol (2017) 4:11–8. doi: 10.22037/afb.v4i1.13738
3. Kok CR, Hutkins R. Yogurt and Other Fermented Foods as Sources of Health-Promoting Bacteria. Nutr Rev (2018) 76:4–15. doi: 10.1093/nutrit/nuy056
4. Broadbent JR, Barnes M, Brennand C, Strickland M, Houck K, Johnson ME, et al. Contribution of Lactococcus Lactis Cell Envelope Proteinase Specificity to Peptide Accumulation and Bitterness in Reduced-Fat Cheddar Cheese. Appl Environ Microbiol (2002) 68:1778–85. doi: 10.1128/aem.68.4.1778-1785.2002
5. Abdelfatah EN, Mahboub HHH. Studies on the Effect of Lactococcus Garvieae of Dairy Origin on Both Cheese and Nile Tilapia (O. Niloticus). Int J Vet Sci Med (2018) 6:201–7. doi: 10.1016/j.ijvsm.2018.11.002
6. Mustapha NA, Hu A, Yu CP, Sharuddin SS, Ramli N, Shirai Y, et al. Seeking Key Microorganisms for Enhancing Methane Production in Anaerobic Digestion of Waste Sewage Sludge. Appl Microbiol Biotechnol (2018) 102:5323–34. doi: 10.1007/s00253-018-9003-8
7. Bampidis V, Azimonti G, Bastos ML, Christensen H, Dusemund B, Kos Durjava M, et al. Safety and Efficacy of Monosodium L-Glutamate Monohydrate Produced by Corynebacterium Glutamicum KCCM 80188 as a Feed Additive for All Animal Species. Efsa J (2020) 18:e06085. doi: 10.2903/j.efsa.2020.6085
8. Baek JH, Kim KH, Moon JY, Yeo SH, Jeon CO. Acetobacter Oryzoeni Sp. Nov., Isolated From Korean Rice Wine Vinegar. Int J Syst Evol Microbiol (2020) 70:2026–33. doi: 10.1099/ijsem.0.004008
9. Gueniche A, Benyacoub J, Philippe D, Bastien P, Kusy N, Breton L, et al. Lactobacillus Paracasei CNCM I-2116 (ST11) Inhibits Substance P-Induced Skin Inflammation and Accelerates Skin Barrier Function Recovery In Vitro. Eur J Dermatol (2010) 20:731–7. doi: 10.1684/ejd.2010.1108
10. Barko PC, McMichael MA, Swanson KS, Williams DA. The Gastrointestinal Microbiome: A Review. J Vet Intern Med (2018) 32:9–25. doi: 10.1111/jvim.14875
11. Huang M, Cai S, Su J. The Pathogenesis of Sepsis and Potential Therapeutic Targets. Int J Mol Sci (2019) 20:5376. doi: 10.3390/ijms20215376
12. Marangu D, Zar HJ. Childhood Pneumonia in Low-and-Middle-Income Countries: An Update. Paediatr Respir Rev (2019) 32:3–9. doi: 10.1016/j.prrv.2019.06.001
13. Koelman DLH, Brouwer MC, van de Beek D. Targeting the Complement System in Bacterial Meningitis. Brain (2019) 142:3325–37. doi: 10.1093/brain/awz222
14. Tripathi N, Sapra A. Gram Staining. In: Statpearls. Treasure Island (FL: StatPearls Publishing (2021). Copyright © 2021, StatPearls Publishing LLC.
15. Wang W, Wang Y, Lin L, Song Y, Yang CJ. A Tridecaptin-Based Fluorescent Probe for Differential Staining of Gram-Negative Bacteria. Anal Bioanal Chem (2019) 411:4017–23. doi: 10.1007/s00216-018-1465-0
16. Becerra SC, Roy DC, Sanchez CJ, Christy RJ, Burmeister DM. An Optimized Staining Technique for the Detection of Gram Positive and Gram Negative Bacteria Within Tissue. BMC Res Notes (2016) 9:216. doi: 10.1186/s13104-016-1902-0
17. Jenul C, Horswill AR. Regulation of Staphylococcus Aureus Virulence. Microbiol Spectr (2019) 7:1–21. doi: 10.1128/microbiolspec.GPP3-0031-2018
18. Kadioglu A, Weiser JN, Paton JC, Andrew PW. The Role of Streptococcus Pneumoniae Virulence Factors in Host Respiratory Colonization and Disease. Nat Rev Microbiol (2008) 6:288–301. doi: 10.1038/nrmicro1871
19. Norris MH, Kirpich A, Bluhm AP, Zincke D, Hadfield T, Ponciano JM. Convergent Evolution of Diverse Bacillus Anthracis Outbreak Strains Toward Altered Surface Oligosaccharides That Modulate Anthrax Pathogenesis. PLoS Biol (2020) 18:e3001052. doi: 10.1371/journal.pbio.3001052
20. Rueter C, Bielaszewska M. Secretion and Delivery of Intestinal Pathogenic Escherichia Coli Virulence Factors Via Outer Membrane Vesicles. Front Cell Infect Microbiol (2020) 10:91. doi: 10.3389/fcimb.2020.00091
21. Ji J, Yang H. Using Probiotics as Supplementation for Helicobacter Pylori Antibiotic Therapy. Int J Mol Sci (2020) 21:1136. doi: 10.3390/ijms21031136
22. Azam MW, Khan AU. Updates on the Pathogenicity Status of Pseudomonas Aeruginosa. Drug Discov Today (2019) 24:350–9. doi: 10.1016/j.drudis.2018.07.003
23. Harding CM, Hennon SW, Feldman MF. Uncovering the Mechanisms of Acinetobacter Baumannii Virulence. Nat Rev Microbiol (2018) 16:91–102. doi: 10.1038/nrmicro.2017.148
24. Martin RM, Bachman MA. Colonization, Infection, and the Accessory Genome of Klebsiella Pneumoniae. Front Cell Infect Microbiol (2018) 8:4. doi: 10.3389/fcimb.2018.00004
25. Le Chevalier F, Cascioferro A, Majlessi L, Herrmann JL, Brosch R. Mycobacterium Tuberculosis Evolutionary Pathogenesis and its Putative Impact on Drug Development. Future Microbiol (2014) 9:969–85. doi: 10.2217/fmb.14.70
26. Fu LM, Fu-Liu CS. Is Mycobacterium Tuberculosis a Closer Relative to Gram-Positive or Gram-Negative Bacterial Pathogens? Tuberculosis (Edinb) (2002) 82:85–90. doi: 10.1054/tube.2002.0328
27. Jones KE, Patel NG, Levy MA, Storeygard A, Balk D, Gittleman JL, et al. Global Trends in Emerging Infectious Diseases. Nature (2008) 451:990–3. doi: 10.1038/nature06536
28. Morens DM, Folkers GK, Fauci AS. The Challenge of Emerging and Re-Emerging Infectious Diseases. Nature (2004) 430:242–9. doi: 10.1038/nature02759
29. Lewis K. Platforms for Antibiotic Discovery. Nat Rev Drug Discov (2013) 12:371–87. doi: 10.1038/nrd3975
30. Neu HC. The Crisis in Antibiotic Resistance. Science (1992) 257:1064–73. doi: 10.1126/science.257.5073.1064
31. Martínez JL. Antibiotics and Antibiotic Resistance Genes in Natural Environments. Science (2008) 321:365–7. doi: 10.1126/science.1159483
32. Bush K, Courvalin P, Dantas G, Davies J, Eisenstein B, Huovinen P, et al. Tackling Antibiotic Resistance. Nat Rev Microbiol (2011) 9:894–6. doi: 10.1038/nrmicro2693
33. Cipolla-Neto J, Amaral FGD. Melatonin as a Hormone: New Physiological and Clinical Insights. Endocr Rev (2018) 39:990–1028. doi: 10.1210/er.2018-00084
34. Rusanova I, Martínez-Ruiz L, lorido J, Rodríguez-Santana C, Guerra-Librero A, Acuña-Castroviejo D, et al. Protective Effects of Melatonin on the Skin: Future Perspectives. Int J Mol Sci (2019) 20:4948. doi: 10.3390/ijms20194948
35. Tordjman S, Chokron S, Delorme R, Charrier A, Bellissant E, Jaafari N, et al. Melatonin: Pharmacology, Functions and Therapeutic Benefits. Curr Neuropharmacol (2017) 15:434–43. doi: 10.2174/1570159x14666161228122115
36. Wang B, Wen H, Smith W, Hao D, He B, Kong L. Regulation Effects of Melatonin on Bone Marrow Mesenchymal Stem Cell Differentiation. J Cell Physiol (2019) 234:1008–15. doi: 10.1002/jcp.27090
37. Hardeland R, Cardinali DP, Srinivasan V, Spence DW, Brown GM, Pandi-Perumal SR. Melatonin–a Pleiotropic, Orchestrating Regulator Molecule. Prog Neurobiol (2011) 93:350–84. doi: 10.1016/j.pneurobio.2010.12.004
38. Pandi-Perumal SR, Srinivasan V, Maestroni GJ, Cardinali DP, Poeggeler B, Hardeland R. Melatonin: Nature’s Most Versatile Biological Signal? FEBS J (2006) 273:2813–38. doi: 10.1111/j.1742-4658.2006.05322.x
39. Reiter RJ. The Pineal and its Hormones in the Control of Reproduction in Mammals. Endocr Rev (1980) 1:109–31. doi: 10.1210/edrv-1-2-109
40. Plaimee P, Khamphio M, Weerapreeyakul N, Barusrux S, Johns NP. Immunomodulatory Effect of Melatonin in SK-LU-1 Human Lung Adenocarcinoma Cells Co-Cultured With Peripheral Blood Mononuclear Cells. Cell Prolif (2014) 47:406–15. doi: 10.1111/cpr.12119
41. Xia Y, Chen S, Zeng S, Zhao Y, Zhu C, Deng B, et al. Melatonin in Macrophage Biology: Current Understanding and Future Perspectives. J Pineal Res (2019) 66:e12547. doi: 10.1111/jpi.12547
42. Ren W, Liu G, Chen S, Yin J, Wang J, Tan B, et al. Melatonin Signaling in T Cells: Functions and Applications. J Pineal Res (2017) 62:e12394. doi: 10.1111/jpi.12394
43. Tan D-X, Hardeland R. Potential Utility of Melatonin in Deadly Infectious Diseases Related to the Overreaction of Innate Immune Response and Destructive Inflammation: Focus on COVID-19. Melatonin Res (2020) 3:120–43. doi: 10.32794/mr11250052
44. Mauriz JL, Collado PS, Veneroso C, Reiter RJ, González-Gallego J. A Review of the Molecular Aspects of Melatonin’s Anti-Inflammatory Actions: Recent Insights and New Perspectives. J Pineal Res (2013) 54:1–14. doi: 10.1111/j.1600-079X.2012.01014.x
45. Álvarez-Sánchez N, Cruz-Chamorro I, Díaz-Sánchez M, Sarmiento-Soto H, Medrano-Campillo P, Martínez-López A, et al. Melatonin Reduces Inflammatory Response in Peripheral T Helper Lymphocytes From Relapsing-Remitting Multiple Sclerosis Patients. J Pineal Res (2017) 63:e12394. doi: 10.1111/jpi.12442
46. Hardeland R. Aging, Melatonin, and the Pro- and Anti-Inflammatory Networks. Int J Mol Sci (2019) 20:1223. doi: 10.3390/ijms20051223
47. Yawoot N, Govitrapong P, Tocharus C. Ischemic Stroke, Obesity, and the Anti-Inflammatory Role of Melatonin. Biofactors (2021) 47:41–58. doi: 10.1002/biof.1690
48. Reiter RJ, Tan DX, Burkhardt S. Reactive Oxygen and Nitrogen Species and Cellular and Organismal Decline: Amelioration With Melatonin. Mech Ageing Dev (2002) 123:1007–19. doi: 10.1016/s0047-6374(01)00384-0
49. Reiter RJ, Tan DX, Mayo JC, Sainz RM, Leon J, Czarnocki Z. Melatonin as an Antioxidant: Biochemical Mechanisms and Pathophysiological Implications in Humans. Acta Biochim Pol (2003) 50:1129–46. doi: 10.18388/abp.2003_3637
50. Hardeland R. Antioxidative Protection by Melatonin: Multiplicity of Mechanisms From Radical Detoxification to Radical Avoidance. Endocrine (2005) 27:119–30. doi: 10.1385/endo:27:2:119
51. Tian X, Wang F, Zhang L, Ji P, Wang J, Lv D, et al. Melatonin Promotes the In Vitro Development of Microinjected Pronuclear Mouse Embryos Via Its Anti-Oxidative and Anti-Apoptotic Effects. Int J Mol Sci (2017) 18:988. doi: 10.3390/ijms18050988
52. Hill SM, Frasch T, Xiang S, Yuan L, Duplessis T, Mao L. Molecular Mechanisms of Melatonin Anticancer Effects. Integr Cancer Ther (2009) 8:337–46. doi: 10.1177/1534735409353332
53. Samanta S. Melatonin: An Endogenous Miraculous Indolamine, Fights Against Cancer Progression. J Cancer Res Clin Oncol (2020) 146:1893–922. doi: 10.1007/s00432-020-03292-w
54. Tekbas OF, Ogur R, Korkmaz A, Kilic A, Reiter RJ. Melatonin as an Antibiotic: New Insights Into the Actions of This Ubiquitous Molecule. J Pineal Res (2008) 44:222–6. doi: 10.1111/j.1600-079X.2007.00516.x
55. Lee HY, Byeon Y, Back K. Melatonin as a Signal Molecule Triggering Defense Responses Against Pathogen Attack in Arabidopsis and Tobacco. J Pineal Res (2014) 57:262–8. doi: 10.1111/jpi.12165
56. Bishayi B, Adhikary R, Nandi A, Sultana S. Beneficial Effects of Exogenous Melatonin in Acute Staphylococcus Aureus and Escherichia Coli Infection-Induced Inflammation and Associated Behavioral Response in Mice After Exposure to Short Photoperiod. Inflammation (2016) 39:2072–93. doi: 10.1007/s10753-016-0445-9
57. López LC, Escames G, Tapias V, Utrilla P, León J, Acuña-Castroviejo D. Identification of an Inducible Nitric Oxide Synthase in Diaphragm Mitochondria From Septic Mice: its Relation With Mitochondrial Dysfunction and Prevention by Melatonin. Int J Biochem Cell Biol (2006) 38:267–78. doi: 10.1016/j.biocel.2005.09.008
58. Escames G, Acuña-Castroviejo D, López LC, Tan DX, Maldonado MD, Sánchez-Hidalgo M, et al. Pharmacological Utility of Melatonin in the Treatment of Septic Shock: Experimental and Clinical Evidence. J Pharm Pharmacol (2006) 58:1153–65. doi: 10.1211/jpp.58.9.0001
59. Ortiz F, García JA, Acuña-Castroviejo D, Doerrier C, López A, Venegas C, et al. The Beneficial Effects of Melatonin Against Heart Mitochondrial Impairment During Sepsis: Inhibition of iNOS and Preservation of Nnos. J Pineal Res (2014) 56:71–81. doi: 10.1111/jpi.12099
60. Henderson R, Kim S, Lee E. Use of Melatonin as Adjunctive Therapy in Neonatal Sepsis: A Systematic Review and Meta-Analysis. Complement Ther Med (2018) 39:131–6. doi: 10.1016/j.ctim.2018.06.002
61. Srinivasan V, Mohamed M, Kato H. Melatonin in Bacterial and Viral Infections With Focus on Sepsis: A Review. Recent Pat Endocr Metab Immune Drug Discov (2012) 6:30–9. doi: 10.2174/187221412799015317
62. Galley HF, Lowes DA, Allen L, Cameron G, Aucott LS, Webster NR. Melatonin as a Potential Therapy for Sepsis: A Phase I Dose Escalation Study and an Ex Vivo Whole Blood Model Under Conditions of Sepsis. J Pineal Res (2014) 56:427–38. doi: 10.1111/jpi.12134
63. Hardeland R. Melatonin and Inflammation-Story of a Double-Edged Blade. J Pineal Res (2018) 65:e12525. doi: 10.1111/jpi.12525
64. Tan DX, Zheng X, Kong J, Manchester LC, Hardeland R, Kim SJ, et al. Fundamental Issues Related to the Origin of Melatonin and Melatonin Isomers During Evolution: Relation to Their Biological Functions. Int J Mol Sci (2014) 15:15858–90. doi: 10.3390/ijms150915858
65. Tan DX, Hardeland R, Back K, Manchester LC, Alatorre-Jimenez MA, Reiter RJ. On the Significance of an Alternate Pathway of Melatonin Synthesis Via 5-Methoxytryptamine: Comparisons Across Species. J Pineal Res (2016) 61:27–40. doi: 10.1111/jpi.12336
66. Luo H, Schneider K, Christensen U, Lei Y, Herrgard M, Palsson B. Microbial Synthesis of Human-Hormone Melatonin at Gram Scales. ACS Synth Biol (2020) 9:1240–5. doi: 10.1021/acssynbio.0c00065
67. Tan DX, Manchester LC, Liu X, Rosales-Corral SA, Acuna-Castroviejo D, Reiter RJ. Mitochondria and Chloroplasts as the Original Sites of Melatonin Synthesis: A Hypothesis Related to Melatonin’s Primary Function and Evolution in Eukaryotes. J Pineal Res (2013) 54:127–38. doi: 10.1111/jpi.12026
68. Hardeland R. Melatonin in the Evolution of Plants and Other Phototrophs. Melatonin Res (2019) 2:10–36. doi: 10.32794/mr11250029
69. Claustrat B, Brun J, Chazot G. The Basic Physiology and Pathophysiology of Melatonin. Sleep Med Rev (2005) 9:11–24. doi: 10.1016/j.smrv.2004.08.001
70. Hardeland R, Madrid JA, Tan DX, Reiter RJ. Melatonin, the Circadian Multioscillator System and Health: The Need for Detailed Analyses of Peripheral Melatonin Signaling. J Pineal Res (2012) 52:139–66. doi: 10.1111/j.1600-079X.2011.00934.x
71. Zisapel N. New Perspectives on the Role of Melatonin in Human Sleep, Circadian Rhythms and Their Regulation. Br J Pharmacol (2018) 175:3190–9. doi: 10.1111/bph.14116
72. Scheer FA, Van Montfrans GA, van Someren EJ, Mairuhu G, Buijs RM. Daily Nighttime Melatonin Reduces Blood Pressure in Male Patients With Essential Hypertension. Hypertension (2004) 43:192–7. doi: 10.1161/01.HYP.0000113293.15186.3b
73. Rodríguez C, Puente-Moncada N. Regulation of Cancer Cell Glucose Metabolism is Determinant for Cancer Cell Fate After Melatonin Administration. J Cell Physiol (2021) 236:27–40. doi: 10.1002/jcp.29886
74. Dollins AB, Zhdanova IV, Wurtman RJ, Lynch HJ, Deng MH. Effect of Inducing Nocturnal Serum Melatonin Concentrations in Daytime on Sleep, Mood, Body Temperature, and Performance. Proc Natl Acad Sci U S A (1994) 91:1824–8. doi: 10.1073/pnas.91.5.1824
75. Reiter RJ, Rosales-Corral SA, Tan DX, Acuna-Castroviejo D, Qin L, Yang SF, et al. Melatonin, a Full Service Anti-Cancer Agent: Inhibition of Initiation, Progression and Metastasis. Int J Mol Sci (2017) 18:843. doi: 10.3390/ijms18040843
76. Chitimus DM, Popescu MR, Voiculescu SE, Panaitescu AM, Pavel B, Zagrean L, et al. Melatonin’s Impact on Antioxidative and Anti-Inflammatory Reprogramming in Homeostasis and Disease. Biomolecules (2020) 10:1211. doi: 10.3390/biom10091211
77. Ma Q, Reiter RJ, Chen Y. Role of Melatonin in Controlling Angiogenesis Under Physiological and Pathological Conditions. Angiogenesis (2020) 23:91–104. doi: 10.1007/s10456-019-09689-7
78. Claustrat B, Leston J. Melatonin: Physiological Effects in Humans. Neurochirurgie (2015) 61:77–84. doi: 10.1016/j.neuchi.2015.03.002
79. Mistraletti G, Umbrello M, Sabbatini G, Miori S, Taverna M, Cerri B, et al. Melatonin Reduces the Need for Sedation in ICU Patients: A Randomized Controlled Trial. Minerva Anestesiol (2015) 81:1298–310.
80. Mistraletti G, Sabbatini G, Taverna M, Figini MA, Umbrello M, Magni P, et al. Pharmacokinetics of Orally Administered Melatonin in Critically Ill Patients. J Pineal Res (2010) 48:142–7. doi: 10.1111/j.1600-079X.2009.00737.x
81. Zhang R, Wang X, Ni L, Di X, Ma B, Niu S, et al. Covid-19: Melatonin as a Potential Adjuvant Treatment. Life Sci (2020) 250:117583. doi: 10.1016/j.lfs.2020.117583
82. Nordlund JJ, Lerner AB. The Effects of Oral Melatonin on Skin Color and on the Release of Pituitary Hormones. J Clin Endocrinol Metab (1977) 45:768–74. doi: 10.1210/jcem-45-4-768
83. Barchas J, DaCosta F, Spector S. Acute Pharmacology of Melatonin. Nature (1967) 214:919–20. doi: 10.1038/214919a0
84. Sugden D. Psychopharmacological Effects of Melatonin in Mouse and Rat. J Pharmacol Exp Ther (1983) 227:587–91. doi: 10.1016/0160-5402(83)90069-4
85. Weishaupt JH, Bartels C, Pölking E, Dietrich J, Rohde G, Poeggeler B, et al. Reduced Oxidative Damage in ALS by High-Dose Enteral Melatonin Treatment. J Pineal Res (2006) 41:313–23. doi: 10.1111/j.1600-079X.2006.00377.x
86. Wasdell MB, Jan JE, Bomben MM, Freeman RD, Rietveld WJ, Tai J, et al. A Randomized, Placebo-Controlled Trial of Controlled Release Melatonin Treatment of Delayed Sleep Phase Syndrome and Impaired Sleep Maintenance in Children With Neurodevelopmental Disabilities. J Pineal Res (2008) 44:57–64. doi: 10.1111/j.1600-079X.2007.00528.x
87. Andersen LP, Gögenur I, Rosenberg J, Reiter RJ. The Safety of Melatonin in Humans. Clin Drug Investig (2016) 36:169–75. doi: 10.1007/s40261-015-0368-5
88. Ferlazzo N, Andolina G, Cannata A, Costanzo MG, Rizzo V, Currò M. Is Melatonin the Cornucopia of the 21st Century? Antioxidants (Basel) (2020) 9:1088. doi: 10.3390/antiox9111088
89. Besag FMC, Vasey MJ, Lao KSJ, Wong ICK. Adverse Events Associated With Melatonin for the Treatment of Primary or Secondary Sleep Disorders: A Systematic Review. CNS Drugs (2019) 33:1167–86. doi: 10.1007/s40263-019-00680-w
90. Ozturk AI, Yilmaz O, Kirbag S, Arslan M. Antimicrobial and Biological Effects of Ipemphos and Amphos on Bacterial and Yeast Strains. Cell Biochem Funct (2000) 18:117–26. doi: 10.1002/(sici)1099-0844(200006)18:2<117::aid-cbf863>3.0.co;2-1
91. Wang HX, Liu F, Ng TB. Examination of Pineal Indoles and 6-methoxy-2-benzoxazolinone for Antioxidant and Antimicrobial Effects. Comp Biochem Physiol C Toxicol Pharmacol (2001) 130:379–88. doi: 10.1016/S1532-0456(01)00264-2
92. Wiid I, Hoalvan HE, Hon D, Lombard C, Van HP. Potentiation of Isoniazid Activity Against Mycobacterium Tuberculosis by Melatonin. Antimicrob Agents Chemother (1999) 43:975–7. doi: 10.1128/AAC.43.4.975
93. Abbas M, Paul M, Huttner A. New and Improved? A Review of Novel Antibiotics for Gram-Positive Bacteria. Clin Microbiol Infect (2017) 23:697–703. doi: 10.1016/j.cmi.2017.06.010
94. Woodford N, Livermore DM. Infections Caused by Gram-Positive Bacteria: A Review of the Global Challenge. J Infect (2009) 59 Suppl 1:S4–16. doi: 10.1016/s0163-4453(09)60003-7
95. Atroshi F, Rizzo A, Westermarck T, Ali-vehmas T. Effects of Tamoxifen, Melatonin, Coenzyme Q10, and L-carnitine Supplementation on Bacterial Growth in the Presence of Mycotoxins. Pharmacol Res (1998) 38:289–95. doi: 10.1006/phrs.1998.0363
96. Masadeh MM, Alzoubi KH, Al-Azzam SI, Khabour OF, Al-Buhairan AM. Ciprofloxacin-Induced Antibacterial Activity Is Atteneuated by Pretreatment With Antioxidant Agents. Pathogens (2016) 5:28. doi: 10.3390/pathogens5010028
97. Thi MTT, Wibowo D, Rehm BHA. Pseudomonas Aeruginosa Biofilms. Int J Mol Sci (2020) 21:8671. doi: 10.3390/ijms21228671
98. Chen W. Host Innate Immune Responses to Acinetobacter Baumannii Infection. Front Cell Infect Microbiol (2020) 10:486. doi: 10.3389/fcimb.2020.00486
99. de Bentzmann S, Plésiat P. The Pseudomonas Aeruginosa Opportunistic Pathogen and Human Infections. Environ Microbiol (2011) 13:1655–65. doi: 10.1111/j.1462-2920.2011.02469.x
100. Redfern J, Enright MC. Further Understanding of Pseudomonas Aeruginosa’s Ability to Horizontally Acquire Virulence: Possible Intervention Strategies. Expert Rev Anti Infect Ther (2020) 18:539–49. doi: 10.1080/14787210.2020.1751610
101. Augustin P, Tran-Dinh A, Valin N, Desmard M, Crevecoeur MA, Muller-Serieys C, et al. Pseudomonas Aeruginosa Post-Operative Peritonitis: Clinical Features, Risk Factors, and Prognosis. Surg Infect (Larchmt) (2013) 14:297–303. doi: 10.1089/sur.2012.084
102. Brandenburg KS, Weaver AJ Jr., Qian L, You T, Chen P, Karna SLR, et al. Development of Pseudomonas Aeruginosa Biofilms in Partial-Thickness Burn Wounds Using a Sprague-Dawley Rat Model. J Burn Care Res (2019) 40:44–57. doi: 10.1093/jbcr/iry043
103. Yoshida S, Suzuki K, Suzuki A, Okada H, Niwa T, Kobayashi R, et al. Risk Factors for the Failure of Treatment of Pseudomonas Aeruginosa Bacteremia in Critically Ill Patients. Pharmazie (2017) 72:428–32. doi: 10.1691/ph.2017.7453
104. Britt NS, Ritchie DJ, Kollef MH, Burnham CA, Durkin MJ, Hampton NB, et al. Importance of Site of Infection and Antibiotic Selection in the Treatment of Carbapenem-Resistant Pseudomonas Aeruginosa Sepsis. Antimicrob Agents Chemother (2018) 62:e02400–17. doi: 10.1128/aac.02400-17
105. Robertson GT, Doyle TB, Du Q, Duncan L, Mdluli KE, Lynch AS. A Novel Indole Compound That Inhibits Pseudomonas Aeruginosa Growth by Targeting MreB is a Substrate for Mexab-Oprm. J Bacteriol (2007) 189:6870–81. doi: 10.1128/jb.00805-07
106. Wareth G, Neubauer H, Sprague LD. Acinetobacter Baumannii - a Neglected Pathogen in Veterinary and Environmental Health in Germany. Vet Res Commun (2019) 43:1–6. doi: 10.1007/s11259-018-9742-0
107. Amaya-Villar R, Garnacho-Montero J. How Should We Treat Acinetobacter Pneumonia? Curr Opin Crit Care (2019) 25:465–72. doi: 10.1097/mcc.0000000000000649
108. Xiao J, Zhang C, Ye S. Acinetobacter Baumannii Meningitis in Children: A Case Series and Literature Review. Infection (2019) 47:643–9. doi: 10.1007/s15010-018-1234-1
109. Chusri S, Chongsuvivatwong V, Silpapojakul K, Singkhamanan K, Hortiwakul T, Charernmak B, et al. Clinical Characteristics and Outcomes of Community and Hospital-Acquired Acinetobacter Baumannii Bacteremia. J Microbiol Immunol Infect (2019) 52:796–806. doi: 10.1016/j.jmii.2019.03.004
110. Shi J, Sun T, Cui Y, Wang C, Wang F, Zhou Y, et al. Multidrug Resistant and Extensively Drug Resistant Acinetobacter Baumannii Hospital Infection Associated With High Mortality: A Retrospective Study in the Pediatric Intensive Care Unit. BMC Infect Dis (2020) 20:597. doi: 10.1186/s12879-020-05321-y
111. Fernández-García L, Fernandez-Cuenca F, Blasco L, López-Rojas R, Ambroa A, Lopez M, et al. Relationship Between Tolerance and Persistence Mechanisms in Acinetobacter Baumannii Strains With AbkAB Toxin-Antitoxin System. Antimicrob Agents Chemother (2018) 62:e00250–00218. doi: 10.1128/aac.00250-18
112. Uberos J, Augustin C, Liébana J, Molina A, Muñoz-Hoyos A. Comparative Study of the Influence of Melatonin and Vitamin E on the Surface Characteristics of Escherichia Coli. Lett Appl Microbiol (2001) 32:303–6. doi: 10.1046/j.1472-765x.2001.00908.x
113. Uberos J, Molina A, Liébana J, Augustin MC, Muñoz A. The Influence of Different Concentrations of Melatonin on the Cell Surface Hydrophobic Characteristics of Neisseria Meningitidis. Lett Appl Microbiol (2000) 31:294–8. doi: 10.1046/j.1472-765x.2000.00813.x
114. Gagneux S. Ecology and Evolution of Mycobacterium Tuberculosis. Nat Rev Microbiol (2018) 16:202–13. doi: 10.1038/nrmicro.2018.8
115. Pavlik A, Yayo I, Parmova W, Melicharek I, Hanzlikova I, Svejcova M, et al. Mycobacterium Tuberculosis in Animal and Human Population in Six Central European Countries During 1990-1999. Vet Med (2003) 48:8–89. doi: 10.17221/5754-VETMED
116. Chen Y, Chao Y, Deng Q, Liu T, Xiang J, Chen J, et al. Potential Challenges to the Stop Tb Plan for Humans in China; Cattle Maintain M. Bovis, and M. Tuberculosis. Tuberculosis (Edinb) (2009) 89:95–100. doi: 10.1016/j.tube.2008.07.003
117. Sarathy JP, Via LE, Weiner D, Blanc L, Boshoff H, Eugenin EA, et al. Extreme Drug Tolerance of Mycobacterium Tuberculosis in Caseum. Antimicrob Agents Chemother (2018) 62:e02266–02217. doi: 10.1128/aac.02266-17
118. Jasim TM, Alabbassi MG, Almuqdadi SF, Kamel H, Kamel J. Anti-Bacterial Properties of Melatonin Against Mycobacterium Tuberculosis In Vitro. Iraqi J Pharm Sci (2010) 19:59–63.
119. Chen X, Sun C, Laborda P, Zhao Y, Palmer I, Fu ZQ, et al. Melatonin Treatment Inhibits the Growth of Xanthomonas Oryzae Pv. Oryzae. Front Microbiol (2018) 9:2280. doi: 10.3389/fmicb.2018.02280
120. Chen X, Sun C, Laborda P, He Y, Zhao Y, Li Z, et al. Melatonin Treatments Reduce the Pathogenicity and Inhibit the Growth of Xanthomonas Oryzae Pv. Oryzicola. Plant Pathol (2019) 68:288–96. doi: 10.1111/ppa.12954
121. Ward CG, Bullen JJ, Rogers HJ. Iron and Infection: New Developments and Their Implications. J Trauma (1996) 41:356–64. doi: 10.1097/00005373-199608000-00030
122. Gulcin I, Buyukokuroglu ME, Kufrevioglu OI. Metal Chelating and Hydrogen Peroxide Scavenging Effects of Melatonin. J Pineal Res (2003) 34:278–81. doi: 10.1034/j.1600-079X.2003.00042.x
123. Rothfield L, Horecker BL. The Role of Cell-Wall Lipid in the Biosynthesis of Bacterial Lipopolysaccharide. Proc Natl Acad Sci U S A (1964) 52:939–46. doi: 10.1073/pnas.52.4.939
124. Hebeler BH, Chatterjee AN, Young FE. Regulation of the Bacterial Cell Wall: Effect of Antibiotics on Lipid Biosynthesis. Antimicrob Agents Chemother (1973) 4:346–53. doi: 10.1128/AAC.4.3.346
125. Dauchy RT, Dauchy EM, Davidson LK, Krause JA, Lynch DT, Tirrell PC, et al. Inhibition of Fatty Acid Transport and Proliferative Activity in Tissue-Isolated Human Squamous Cell Cancer Xenografts Perfused in Situ With Melatonin or Eicosapentaenoic or Conjugated Linoleic Acids. Comp Med (2007) 57:377–82. doi: 10.1023/A:1010330504668
126. Liu Y, Jia Y, Yang K, Tong Z, Shi J, Li R, et al. Melatonin Overcomes MCR-Mediated Colistin Resistance in Gram-negative Pathogens. Theranostics (2020) 10:10697–711. doi: 10.7150/thno.45951
127. Gerber J, Lotz M, Ebert S, Kiel S, Huether G, Kuhnt U, et al. Melatonin is Neuroprotective in Experimental Streptococcus Pneumoniae Meningitis. J Infect Dis (2005) 191:783–90. doi: 10.1086/427816
128. Sener G, Tuğtepe H, Velioğlu-Oğünç A, Cetinel S, Gedik N, Yeğen BC. Melatonin Prevents Neutrophil-Mediated Oxidative Injury in Escherichia Coli-Induced Pyelonephritis in Rats. J Pineal Res (2006) 41:220–7. doi: 10.1111/j.1600-079X.2006.00357.x
129. Luo J, Song J, Zhang H, Zhang F, Liu H, Li L, et al. Melatonin Mediated Foxp3-Downregulation Decreases Cytokines Production Via the TLR2 and TLR4 Pathways in H. Pylori Infected Mice. Int Immunopharmacol (2018) 64:116–22. doi: 10.1016/j.intimp.2018.08.034
130. Wu UI, Mai FD, Sheu JN, Chen LY, Liu YT, Huang HC, et al. Melatonin Inhibits Microglial Activation, Reduces Pro-Inflammatory Cytokine Levels, and Rescues Hippocampal Neurons of Adult Rats With Acute Klebsiella Pneumoniae Meningitis. J Pineal Res (2011) 50:159–70. doi: 10.1111/j.1600-079X.2010.00825.x
131. Carrillo-Vico A, Lardone PJ, Naji L, Fernandez-Santos JM, Martin-Lacave I, Guerrero JM, et al. Beneficial Pleiotropic Actions of Melatonin in an Experimental Model of Septic Shock in Mice: Regulation of Pro-/Anti-Inflammatory Cytokine Network, Protection Against Oxidative Damage and Anti-Apoptotic Effects. J Pineal Res (2005) 39:400–8. doi: 10.1111/j.1600-079X.2005.00265.x
132. Maestroni GJ. Melatonin as a Therapeutic Agent in Experimental Endotoxic Shock. J Pineal Res (1996) 20:84–9. doi: 10.1111/j.1600-079X.1996.tb00244.x
133. de Jong NWM, van Kessel KPM, van Strijp JAG. Immune Evasion by Staphylococcus Aureus. Microbiol Spectr (2019) 7:GPP3-0061-201. doi: 10.1128/microbiolspec.GPP3-0061-2019
134. Smith TC, Wardyn SE. Human Infections With Staphylococcus Aureus CC398. Curr Environ Health Rep (2015) 2:41–51. doi: 10.1007/s40572-014-0034-8
135. Reddinger RM, Luke-Marshall NR, Sauberan SL, Hakansson AP. Streptococcus Pneumoniae Modulates Staphylococcus Aureus Biofilm Dispersion and the Transition From Colonization to Invasive Disease. mBio (2018) 9:e02089–02017. doi: 10.1128/mBio.02089-17
136. Zhao W, Pan F, Wang B, Wang C, Sun Y, Zhang T, et al. Epidemiology Characteristics of Streptococcus Pneumoniae From Children With Pneumonia in Shanghai: A Retrospective Study. Front Cell Infect Microbiol (2019) 9:258. doi: 10.3389/fcimb.2019.00258
137. Yau B, Hunt NH, Mitchell AJ, Too LK. Blood–Brain Barrier Pathology and CNS Outcomes in Streptococcus Pneumoniae Meningitis. Int J Mol Sci (2018) 19:3555. doi: 10.3390/ijms19113555
138. Priftis KN, Litt D, Manglani S, Anthracopoulos MB, Thickett K, Tzanakaki G, et al. Bacterial Bronchitis Caused by Streptococcus Pneumoniae and Nontypable Haemophilus Influenzae in Children: The Impact of Vaccination. Chest (2013) 143:152–7. doi: 10.1378/chest.12-0623
139. Spreer A, Gerber J, Baake D, Hanssen M, Huether G, Nau R. Antiinflammatory But No Neuroprotective Effects of Melatonin Under Clinical Treatment Conditions in Rabbit Models of Bacterial Meningitis. J Neurosci Res (2006) 84:1575–9. doi: 10.1002/jnr.21055
140. Mueller M, Tainter CR. Escherichia Coli. In: Statpearls. Treasure Island (FL: StatPearls Publishing (2020). Copyright © 2020, StatPearls Publishing LLC.
141. Köhler CD, Dobrindt U. What Defines Extraintestinal Pathogenic Escherichia Coli? Int J Med Microbiol (2011) 301:642–7. doi: 10.1016/j.ijmm.2011.09.006
142. Madhavan TP, Sakellaris H. Colonization Factors of Enterotoxigenic Escherichia Coli. Adv Appl Microbiol (2015) 90:155–97. doi: 10.1016/bs.aambs.2014.09.003
143. Bohnlein C, Kabisch J, Meske D, Franz CM, Pichner R. Fitness of Enterohemorrhagic Escherichia Coli (EHEC)/Enteroaggregative E. Coli O104:H4 in Comparison to That of EHEC O157: Survival Studies in Food and In Vitro. Appl Environ Microbiol (2016) 82:6326–34. doi: 10.1128/aem.01796-16
144. Hu J, Torres AG. Enteropathogenic Escherichia Coli: Foe or Innocent Bystander? Clin Microbiol Infect (2015) 21:729–34. doi: 10.1016/j.cmi.2015.01.015
145. Vijay D, Dhaka P, Vergis J, Negi M, Mohan V, Kumar M, et al. Characterization and Biofilm Forming Ability of Diarrhoeagenic Enteroaggregative Escherichia Coli Isolates Recovered From Human Infants and Young Animals. Comp Immunol Microbiol Infect Dis (2015) 38:21–31. doi: 10.1016/j.cimid.2014.11.004
146. Ramya Raghavan P, Purushothaman SA, Ramanathan T, Roy S. Diarrhegenic Escherichia Coli Replaces Shigella Spp. As the Predominant Bacteria Causing Childhood Diarrhea in Andaman and Nicobar Islands, India. Am J Trop Med Hyg (2018) 98:814–5. doi: 10.4269/ajtmh.17-0327
147. Khwaja J. Bilateral Adrenal Hemorrhage in the Background of Escherichia Coli Sepsis: A Case Report. J Med Case Rep (2017) 11:72. doi: 10.1186/s13256-017-1236-0
148. Paulose JK, Wright JM, Patel AG, Cassone VM. Human Gut Bacteria Are Sensitive to Melatonin and Express Endogenous Circadian Rhythmicity. PloS One (2016) 11:e0146643. doi: 10.1371/journal.pone.0146643
149. Dunne C, Dolan B, Clyne M. Factors That Mediate Colonization of the Human Stomach by Helicobacter Pylori. World J Gastroenterol (2014) 20:5610–24. doi: 10.3748/wjg.v20.i19.5610
150. Yang L, Zhang J, Xu J, Wei X, Yang J, Liu Y, et al. Helicobacter Pylori Infection Aggravates Dysbiosis of Gut Microbiome in Children With Gastritis. Front Cell Infect Microbiol (2019) 9:375. doi: 10.3389/fcimb.2019.00375
151. Lanas A, Dumonceau JM, Hunt RH, Fujishiro M, Scheiman JM, Gralnek IM, et al. Non-Variceal Upper Gastrointestinal Bleeding. Nat Rev Dis Primers (2018) 4:18020. doi: 10.1038/nrdp.2018.20
152. Kim SS, Ruiz VE, Carroll JD, Moss SF. Helicobacter Pylori in the Pathogenesis of Gastric Cancer and Gastric Lymphoma. Cancer Lett (2011) 305:228–38. doi: 10.1016/j.canlet.2010.07.014
153. Chojnacki C, Poplawski T, Blasiak J, Chojnacki J, Reiter RJ, Klupinska G. Expression of Melatonin Synthesizing Enzymes in Helicobacter Pylori Infected Gastric Mucosa. BioMed Res Int (2013) 2013:845032. doi: 10.1155/2013/845032
154. Malinovskaia NK, Rapoport SI, Zhernakova NI, Rybnikova SN, Postnikova LI, Parkhomenko IE. [Antihelicobacter Effects of Melatonin]. Klin Med (Mosk) (2007) 85:40–3.
155. Celinski K, Konturek PC, Konturek SJ, Slomka M, Cichoz-Lach H, Brzozowski T, et al. Effects of Melatonin and Tryptophan on Healing of Gastric and Duodenal Ulcers With Helicobacter Pylori Infection in Humans. J Physiol Pharmacol (2011) 62:521–6.
156. Osadchuk MA, Sibriaev AA, Kireeva NV, Kvetnoi IM. [The Influence of Melatonin Included in the Combined Treatment of Antichelicobaterial Therapy on Immunohistochemical Characteristics of Gastric Epitheliocytes From Patients With Duodenal Ulcer]. Klin Med (Mosk) (2012) 90:48–52.
157. Ikeda M, Mizoguchi M, Oshida Y, Tatsuno K, Saito R, Okazaki M, et al. Clinical and Microbiological Characteristics and Occurrence of Klebsiella Pneumoniae Infection in Japan. Int J Gen Med (2018) 11:293–9. doi: 10.2147/ijgm.s166940
158. Bueno MG, Iovine RO, Torres LN, Catao-Dias JL, Pissinatti A, Kierulff MC, et al. Pneumonia and Bacteremia in a Golden-Headed Lion Tamarin (Leontopithecus Chrysomelas) Caused by Klebsiella Pneumoniae Subsp. Pneumoniae During a Translocation Program of Free-Ranging Animals in Brazil. J Vet Diagn Invest (2015) 27:387–91. doi: 10.1177/1040638715584792
159. Bidewell CA, Williamson SM, Rogers J, Tang Y, Ellis RJ, Petrovska L, et al. Emergence of Klebsiella Pneumoniae Subspecies Pneumoniae as a Cause of Septicaemia in Pigs in England. PLoS One (2018) 13:e0191958. doi: 10.1371/journal.pone.0191958
160. Namikawa H, Yamada K, Sakiyama A, Imoto W, Yamairi K, Shibata W, et al. Clinical Characteristics of Bacteremia Caused by Hypermucoviscous Klebsiella Pneumoniae at a Tertiary Hospital. Diagn Microbiol Infect Dis (2019) 95:84–8. doi: 10.1016/j.diagmicrobio.2019.04.008
161. Carrie C, Walewski V, Levy C, Alexandre C, Baleine J, Charreton C, et al. Klebsiella Pneumoniae and Klebsiella Oxytoca Meningitis in Infants. Epidemiological and Clinical Features. Arch Pediatr (2019) 26:12–5. doi: 10.1016/j.arcped.2018.09.013
162. Dhruve MJ, Bargman JM. Klebsiella Pneumoniae Renal Abscess and Peritonitis in a Peritoneal Dialysis Patient: A Novel Route of Infection. Perit Dial Int (2017) 37:654–6. doi: 10.3747/pdi.2017.00094
163. Korde JP, Srivastava RS, Mishra SC, Sharma AK. Time Dependent Immunomodulatory Response of Exogenous Melatonin to Killed Pasteurella Multocida (P52 Strain) Vaccine in Albino Rats. Indian J Physiol Pharmacol (2005) 49:227–35.
164. Esquifino AI, Pandi-Perumal SR, Cardinali DP. Circadian Organization of the Immune Response: A Role for Melatonin. Clin Appl Immunol Rev (2004) 4:423–33. doi: 10.1016/j.cair.2004.08.002
165. Colunga Biancatelli RML, Berrill M, Mohammed YH, Marik PE. Melatonin for the Treatment of Sepsis: The Scientific Rationale. J Thorac Dis (2020) 12:S54–s65. doi: 10.21037/jtd.2019.12.85
166. Zhen G, Liang W, Jia H, Zheng X. Melatonin Relieves Sepsis-Induced Myocardial Injury Via Regulating JAK2/STAT3 Signaling Pathway. Minerva Med (2020). doi: 10.23736/s0026-4806.20.06626-4
167. Petronilho F, Giustina AD, Nascimento DZ, Zarbato GF, Vieira AA, Florentino D, et al. Obesity Exacerbates Sepsis-Induced Oxidative Damage in Organs. Inflammation (2016) 39:2062–71. doi: 10.1007/s10753-016-0444-x
168. Mantzarlis K, Tsolaki V. Role of Oxidative Stress and Mitochondrial Dysfunction in Sepsis and Potential Therapies. Oxid Med Cell Longev (2017) 2017:5985209. doi: 10.1155/2017/5985209
169. Tan DX, Manchester LC, Terron MP, Flores LJ, Reiter RJ. One Molecule, Many Derivatives: A Never-Ending Interaction of Melatonin With Reactive Oxygen and Nitrogen Species? J Pineal Res (2007) 42:28–42. doi: 10.1111/j.1600-079X.2006.00407.x
170. Chen Y, Qing W, Sun M, Lv L, Guo D, Jiang Y. Melatonin Protects Hepatocytes Against Bile Acid-Induced Mitochondrial Oxidative Stress Via the AMPK-SIRT3-SOD2 Pathway. Free Radic Res (2015) 49:1275–84. doi: 10.3109/10715762.2015.1067806
171. Pi H, Xu S, Reiter RJ, Guo P, Zhang L, Li Y, et al. Sirt3-SOD2-mROS-dependent Autophagy in Cadmium-Induced Hepatotoxicity and Salvage by Melatonin. Autophagy (2015) 11:1037–51. doi: 10.1080/15548627.2015.1052208
172. Zhai M, Li B, Duan W, Jing L, Zhang B, Zhang M, Jing L, Zhang B, Zhang M, et al. Melatonin Ameliorates Myocardial Ischemia Reperfusion Injury Through SIRT3-Dependent Regulation of Oxidative Stress and Apoptosis. (2017) 63:e12419. doi: 10.1111/jpi.12419
173. Zhang J, Wang L, Xie W, Hu S, Zhou H, Zhu P. Melatonin Attenuates ER Stress and Mitochondrial Damage in Septic Cardiomyopathy: A New Mechanism Involving BAP31 Upregulation and MAPK-ERK Pathway. J Cell Physiol (2020) 235:2847–56. doi: 10.1002/jcp.29190
174. Kirkebøen KA, Strand OA. The Role of Nitric Oxide in Sepsis–an Overview. Acta Anaesthesiol Scand (1999) 43:275–88. doi: 10.1034/j.1399-6576.1999.430307.x
175. Escames G, León J, Macías M, Khaldy H, Acuña-Castroviejo D. Melatonin Counteracts Lipopolysaccharide-Induced Expression and Activity of Mitochondrial Nitric Oxide Synthase in Rats. FASEB J (2003) 17:932–4. doi: 10.1096/fj.02-0692fje
176. Crespo E, Macías M, Pozo D, Escames G, Martín M, Vives F, et al. Melatonin Inhibits Expression of the Inducible NO Synthase II in Liver and Lung and Prevents Endotoxemia in Lipopolysaccharide-Induced Multiple Organ Dysfunction Syndrome in Rats. FASEB J (1999) 13:1537–46. doi: 10.1096/fasebj.13.12.1537
177. Srinivasan V, Pandi-Perumal SR, Spence DW, Kato H, Cardinali DP. Melatonin in Septic Shock: Some Recent Concepts. J Crit Care (2010) 25:656.e651–656. doi: 10.1016/j.jcrc.2010.03.006
178. Zhou L, Zhao D, An H, Zhang H, Jiang C, Yang B. Melatonin Prevents Lung Injury Induced by Hepatic Ischemia-Reperfusion Through Anti-Inflammatory and Anti-Apoptosis Effects. Int Immunopharmacol (2015) 29:462–7. doi: 10.1016/j.intimp.2015.10.012
179. Tan DX, Hardeland R. Targeting Host Defense System and Rescuing Compromised Mitochondria to Increase Tolerance Against Pathogens by Melatonin may Impact Outcome of Deadly Virus Infection Pertinent to COVID-19. Molecules (2020) 25:4410. doi: 10.3390/molecules25194410
180. Yasmin F, Sutradhar S, Das P, Mukherjee S. Gut Melatonin: A Potent Candidate in the Diversified Journey of Melatonin Research. Gen Comp Endocrinol (2020) 303:113693. doi: 10.1016/j.ygcen.2020.113693
181. Ma N, Zhang J, Reiter RJ, Ma X. Melatonin Mediates Mucosal Immune Cells, Microbial Metabolism, and Rhythm Crosstalk: A Therapeutic Target to Reduce Intestinal Inflammation. Med Res Rev (2020) 40:606–32. doi: 10.1002/med.21628
182. Xu P, Wang J, Hong F, Wang S, Jin X, Xue T, et al. Melatonin Prevents Obesity Through Modulation of Gut Microbiota in Mice. J Pineal Res (2017) 62:e12399. doi: 10.1111/jpi.12399
183. Yin J, Li Y, Han H, Chen S, Gao J, Liu G, et al. Melatonin Reprogramming of Gut Microbiota Improves Lipid Dysmetabolism in High-Fat Diet-Fed Mice. J Pineal Res (2018) 65:e12524. doi: 10.1111/jpi.12524
184. Yin J, Li Y, Han H, Ma J, Liu G, Wu X, et al. Administration of Exogenous Melatonin Improves the Diurnal Rhythms of the Gut Microbiota in Mice Fed a High-Fat Diet. mSystems (2020) 5:e00002–20. doi: 10.1128/mSystems.00002-20
185. Hong F, Pan S, Xu P, Xue T, Wang J, Guo Y, et al. Melatonin Orchestrates Lipid Homeostasis Through the Hepatointestinal Circadian Clock and Microbiota During Constant Light Exposure. Cells (2020) 9:489. doi: 10.3390/cells9020489
186. Lv WJ, Liu C. Melatonin Alleviates Neuroinflammation and Metabolic Disorder in DSS-Induced Depression Rats. Oxid Med Cell Longev (2020) 2020:1241894. doi: 10.1155/2020/1241894
187. Zhang H, Yan A, Liu X, Ma Y, Zhao F, Wang M, et al. Melatonin Ameliorates Ochratoxin A Induced Liver Inflammation, Oxidative Stress and Mitophagy in Mice Involving in Intestinal Microbiota and Restoring the Intestinal Barrier Function. J Hazard Mater (2020) 407:124489. doi: 10.1016/j.jhazmat.2020.124489
188. Xu L, Zhang W, Kwak M, Zhang L, Lee PCW, Jin JO. Protective Effect of Melatonin Against Polymicrobial Sepsis Is Mediated by the Anti-Bacterial Effect of Neutrophils. Front Immunol (2019) 10:1371. doi: 10.3389/fimmu.2019.01371
189. Fink T, Glas M, Wolf A, Kleber A, Reus E, Wolff M, et al. Melatonin Receptors Mediate Improvements of Survival in a Model of Polymicrobial Sepsis. Crit Care Med (2014) 42:e22–31. doi: 10.1097/CCM.0b013e3182a63e2b
190. Zhang Y, Li X, Grailer JJ, Wang N, Wang M, Yao J, et al. Melatonin Alleviates Acute Lung Injury Through Inhibiting the NLRP3 Inflammasome. J Pineal Res (2016) 60:405–14. doi: 10.1111/jpi.12322
191. Lowes DA, Almawash AM, Webster NR, Reid VL, Galley HF. Melatonin and Structurally Similar Compounds Have Differing Effects on Inflammation and Mitochondrial Function in Endothelial Cells Under Conditions Mimicking Sepsis. Br J Anaesth (2011) 107:193–201. doi: 10.1093/bja/aer149
192. Shang Y, Xu SP, Wu Y, Jiang YX, Wu ZY, Yuan SY, et al. Melatonin Reduces Acute Lung Injury in Endotoxemic Rats. Chin Med J (Engl) (2009) 122:1388–93. doi: 10.3760/cma.j.issn.0366-6999.2009.12.006
193. Titheradge MA. Nitric Oxide in Septic Shock. Biochim Biophys Acta (1999) 1411:437–55. doi: 10.1016/s0005-2728(99)00031-6
194. Acuña-Castroviejo D, Escames G, López LC, Hitos AB, León J. Melatonin and Nitric Oxide: Two Required Antagonists for Mitochondrial Homeostasis. Endocrine (2005) 27:159–68. doi: 10.1385/endo:27:2:159
195. Deng SL, Zhang BL, Reiter RJ, Liu YX. Melatonin Ameliorates Inflammation and Oxidative Stress by Suppressing the P38mapk Signaling Pathway in LPS-Induced Sheep Orchitis. Antioxidants (Basel) (2020) 9:1277. doi: 10.3390/antiox9121277
196. Choi EY, Jin JY, Lee JY, Choi JI, Choi IS, Kim SJ. Melatonin Inhibits Prevotella Intermedia Lipopolysaccharide-Induced Production of Nitric Oxide and Interleukin-6 in Murine Macrophages by Suppressing NF-kappaB and STAT1 Activity. J Pineal Res (2011) 50:197–206. doi: 10.1111/j.1600-079X.2010.00829.x
197. Tamura EK, Cecon E, Monteiro AW, Silva CL, Markus RP. Melatonin Inhibits LPS-Induced NO Production in Rat Endothelial Cells. J Pineal Res (2009) 46:268–74. doi: 10.1111/j.1600-079X.2008.00657.x
198. Yu GM, Kubota H, Okita M, Maeda T. The Anti-Inflammatory and Antioxidant Effects of Melatonin on LPS-Stimulated Bovine Mammary Epithelial Cells. PLoS One (2017) 12:e0178525. doi: 10.1371/journal.pone.0178525
199. Lee SJ, Lee HJ, Jung YH, Kim JS, Choi SH, Han HJ. Melatonin Inhibits Apoptotic Cell Death Induced by Vibrio Vulnificus VvhA Via Melatonin Receptor 2 Coupling With NCF-1. Cell Death Dis (2018) 9:48. doi: 10.1038/s41419-017-0083-7
200. Lee YM, Park JP, Jung YH, Lee HJ, Kim JS, Choi GE, et al. Melatonin Restores Muc2 Depletion Induced by V. vulnificus VvpM via melatonin receptor 2 coupling with Gαq. J Biomed Sci (2020) 27:21. doi: 10.1186/s12929-019-0606-x
201. Debnath B, Islam W, Li M, Sun Y, Lu X, Mitra S, et al. Melatonin Mediates Enhancement of Stress Tolerance in Plants. Int J Mol Sci (2019) 20:1040. doi: 10.3390/ijms20051040
202. Yin L, Wang P, Li M, Ke X, Li C, Liang D, et al. Exogenous Melatonin Improves Malus Resistance to Marssonina Apple Blotch. J Pineal Res (2013) 54:426–34. doi: 10.1111/jpi.12038
203. Mansfield J, Genin S, Magori S, Citovsky V, Sriariyanum M, Ronald P, et al. Top 10 Plant Pathogenic Bacteria in Molecular Plant Pathology. Mol Plant Pathol (2012) 13:614–29. doi: 10.1111/j.1364-3703.2012.00804.x
204. Mahmood T, Jan A, Kakishima M, Komatsu S. Proteomic Analysis of Bacterial-Blight Defense-Responsive Proteins in Rice Leaf Blades. Proteomics (2006) 6:6053–65. doi: 10.1002/pmic.200600470
205. Li C, He Q, Zhang F, Yu J, Li C, Zhao T, et al. Melatonin Enhances Cotton Immunity to Verticillium Wilt Via Manipulating Lignin and Gossypol Biosynthesis. Plant J (2019) 100:784–800. doi: 10.1111/tpj.14477
206. Lee HY, Byeon Y, Tan DX, Reiter RJ, Back K. Arabidopsis Serotonin N-acetyltransferase Knockout Mutant Plants Exhibit Decreased Melatonin and Salicylic Acid Levels Resulting in Susceptibility to an Avirulent Pathogen. J Pineal Res (2015) 58:291–9. doi: 10.1111/jpi.12214
207. Zhao H, Xu L, Su T, Jiang Y, Hu L, Ma F. Melatonin Regulates Carbohydrate Metabolism and Defenses Against Pseudomonas Syringae Pv. Tomato DC3000 Infection in Arabidopsis Thaliana. J Pineal Res (2015) 59:109–19. doi: 10.1111/jpi.12245
208. Qian Y, Tan DX, Reiter RJ, Shi H. Comparative Metabolomic Analysis Highlights the Involvement of Sugars and Glycerol in Melatonin-Mediated Innate Immunity Against Bacterial Pathogen in Arabidopsis. Sci Rep (2015) 5:15815. doi: 10.1038/srep15815
209. Shi H, Chen Y, Tan DX, Reiter RJ, Chan Z, He C. Melatonin Induces Nitric Oxide and the Potential Mechanisms Relate to Innate Immunity Against Bacterial Pathogen Infection in Arabidopsis. J Pineal Res (2015) 59:102–8. doi: 10.1111/jpi.12244
210. Lee HY, Back K. Mitogen-Activated Protein Kinase Pathways are Required for Melatonin-Mediated Defense Responses in Plants. J Pineal Res (2016) 60:327–35. doi: 10.1111/jpi.12314
211. Lee HY, Back K. Melatonin is Required for H2 O2 - and NO-Mediated Defense Signaling Through MAPKKK3 and OXI1 in Arabidopsis Thaliana. J Pineal Res (2017) 62:e12379. doi: 10.1111/jpi.12379
212. Wurtman RJ, Lieberman HR. Melatonin Secretion as a Mediator of Circadian Variations in Sleep and Sleepiness. J Pineal Res (1985) 2:301–3. doi: 10.1111/j.1600-079X.1985.tb00647.x
213. Li Y, Ma J, Yao K, Su W, Tan B, Wu X, et al. Circadian Rhythms and Obesity: Timekeeping Governs Lipid Metabolism. J Pineal Res (2020) 69:e12682. doi: 10.1111/jpi.12682
214. Fathizadeh H, Mirzaei H, Asemi Z. Melatonin: An Anti-Tumor Agent for Osteosarcoma. Cancer Cell Int (2019) 19:319. doi: 10.1186/s12935-019-1044-2
215. Nabavi SM, Nabavi SF, Sureda A, Xiao J, Dehpour AR, Shirooie S, et al. Anti-Inflammatory Effects of Melatonin: A Mechanistic Review. Crit Rev Food Sci Nutr (2019) 59:S4–s16. doi: 10.1080/10408398.2018.1487927
216. Paulose JK, Cassone VM. The Melatonin-Sensitive Circadian Clock of the Enteric Bacterium Enterobacter Aerogenes. Gut Microbes (2016) 7:424–7. doi: 10.1080/19490976.2016.1208892
217. Ren W, Wang P, Yan J, Liu G, Zeng B, Hussain T, et al. Melatonin Alleviates Weanling Stress in Mice: Involvement of Intestinal Microbiota. J Pineal Res (2018) 64:e12448. doi: 10.1111/jpi.12448
218. Junaid A, Tang H, van Reeuwijk A, Abouleila Y, Wuelfroth P, van Duinen V, et al. Ebola Hemorrhagic Shock Syndrome-on-a-Chip. iScience (2020) 23:100765. doi: 10.1016/j.isci.2019.100765
219. Bonilla E, Valero-Fuenmayor N, Pons H, Chacín-Bonilla L. Melatonin Protects Mice Infected With Venezuelan Equine Encephalomyelitis Virus. Cell Mol Life Sci (1997) 53:430–4. doi: 10.1007/s000180050051
220. Montiel M, Bonilla E, Valero N, Mosquera J, Espina LM, Quiroz Y, et al. Melatonin Decreases Brain Apoptosis, Oxidative Stress, and CD200 Expression and Increased Survival Rate in Mice Infected by Venezuelan Equine Encephalitis Virus. Antivir Chem Chemother (2015) 24:99–108. doi: 10.1177/2040206616660851
221. Crespo I, San-Miguel B, Sánchez DI, González-Fernández B, Álvarez M, González-Gallego J, et al. Melatonin Inhibits the Sphingosine Kinase 1/sphingosine-1-phosphate Signaling Pathway in Rabbits With Fulminant Hepatitis of Viral Origin. J Pineal Res (2016) 61:168–76. doi: 10.1111/jpi.12335
222. Huang SH, Cao XJ, Liu W, Shi XY, Wei W. Inhibitory Effect of Melatonin on Lung Oxidative Stress Induced by Respiratory Syncytial Virus Infection in Mice. J Pineal Res (2010) 48:109–16. doi: 10.1111/j.1600-079X.2009.00733.x
223. Ellis LC. Melatonin Reduces Mortality From Aleutian Disease in Mink (Mustela Vison). J Pineal Res (1996) 21:214–7. doi: 10.1111/j.1600-079x.1996.tb00288.x
224. Huang SH, Liao CL, Chen SJ, Shi LG, Lin GJ. Melatonin Possesses an Anti-Influenza Potential Through its Immune Modulatory Effect. J Funct Foods (2019) 58:189–98. doi: 10.1016/j.jff.2019.04.062
225. Shneider A, Kudriavtsev A. Can Melatonin Reduce the Severity of COVID-19 Pandemic? Int Rev Immunol (2020) 39:153–62. doi: 10.1080/08830185.2020.1756284
226. Zhou Y, Hou Y, Shen J, Huang Y, Martin W. Network-Based Drug Repurposing for Novel Coronavirus 2019-Ncov/SARS-Cov-2. Cell Discov (2020) 6:14. doi: 10.1038/s41421-020-0153-3
Keywords: melatonin, bacteriostasis, MAPKs, NF-κB, ROS, inflammasome, sepsis
Citation: He F, Wu X, Zhang Q, Li Y, Ye Y, Li P, Chen S, Peng Y, Hardeland R and Xia Y (2021) Bacteriostatic Potential of Melatonin: Therapeutic Standing and Mechanistic Insights. Front. Immunol. 12:683879. doi: 10.3389/fimmu.2021.683879
Received: 22 March 2021; Accepted: 13 May 2021;
Published: 31 May 2021.
Edited by:
Karl Kuchler, Medical University of Vienna, AustriaReviewed by:
Zhengkai Wei, Foshan University, ChinaShahzad Ali, University of Veterinary and Animal Sciences, Pakistan
Copyright © 2021 He, Wu, Zhang, Li, Ye, Li, Chen, Peng, Hardeland and Xia. This is an open-access article distributed under the terms of the Creative Commons Attribution License (CC BY). The use, distribution or reproduction in other forums is permitted, provided the original author(s) and the copyright owner(s) are credited and that the original publication in this journal is cited, in accordance with accepted academic practice. No use, distribution or reproduction is permitted which does not comply with these terms.
*Correspondence: Shuai Chen, Y2hlbnNodWFpQG1haWwuY29t; Yuanyi Peng, cHl5MjAwMkBzaW5hLmNvbQ==; Yaoyao Xia, eWFveWFveGlhMjAxOEAxMjYuY29t
†These authors have contributed equally to this work