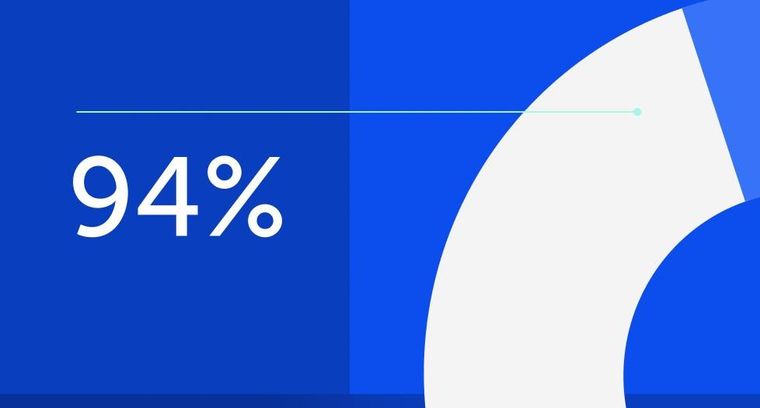
94% of researchers rate our articles as excellent or good
Learn more about the work of our research integrity team to safeguard the quality of each article we publish.
Find out more
ORIGINAL RESEARCH article
Front. Immunol., 14 May 2021
Sec. Multiple Sclerosis and Neuroimmunology
Volume 12 - 2021 | https://doi.org/10.3389/fimmu.2021.674189
This article is part of the Research TopicAssessing Microglial Function and IdentityView all 17 articles
G-protein-coupled receptors (GPCRs) are critical sensors affecting the state of eukaryotic cells. To get systematic insight into the GPCRome of microglia, we analyzed publicly available RNA-sequencing data of bulk and single cells obtained from human and mouse brains. We identified 17 rhodopsin and adhesion family GPCRs robustly expressed in microglia from human brains, including the homeostasis-associated genes CX3CR1, GPR34, GPR183, P2RY12, P2RY13, and ADGRG1. Expression of these microglial core genes was lost upon culture of isolated cells ex vivo but could be acquired by human induced pluripotent stem cell (iPSC)-derived microglial precursors transplanted into mouse brains. CXCR4 and PTGER4 were higher expressed in subcortical white matter compared to cortical grey matter microglia, and ADGRG1 was downregulated in microglia obtained from normal-appearing white and grey matter tissue of multiple sclerosis (MS) brains. Single-cell RNA sequencing of microglia from active lesions, obtained early during MS, revealed downregulation of homeostasis-associated GPCR genes and upregulation of CXCR4 expression in a small subset of MS-associated lesional microglia. Functional presence of low levels of CXCR4 on human microglia was confirmed using flow cytometry and transwell migration towards SDF-1. Microglia abundantly expressed the GPCR down-stream signaling mediator genes GNAI2 (αi2), GNAS (αs), and GNA13 (α13), the latter particularly in white matter. Drugs against several microglia GPCRs are available to target microglia in brain diseases. In conclusion, transcriptome profiling allowed us to identify expression of GPCRs that may contribute to brain (patho)physiology and have diagnostic and therapeutic potential in human microglia.
Microglia are brain-resident phagocytic cells that contribute to brain homeostasis as well as disease (1, 2). Populating the central nervous system (CNS) during embryonic development, microglia persist for the rest of life through local self-renewal. As a consequence, they possess a unique transcriptional signature that emerged only recently from RNA sequencing (RNAseq) of purified primary cells (3). Notably, G protein-coupled receptors (GPCRs) figure prominently in the microglia transcriptome, as exemplified by the characteristic surface expression of CX3CR1, GPR34, GPR183, P2Y12, P2Y13, and GPR56 (3, 4). GPCRs are the senses of our cells, comprising the largest and most diverse superfamily of membrane proteins in eukaryotic cells. Of particular interest is their widespread cellular distribution and the fact that ∼30% of all currently approved pharmaceuticals target them (5). GPCRs control cell and tissue physiology by regulating signaling pathways via heterotrimeric G proteins, which modulate cellular levels of second messengers and, in turn, a wide array of functional activities in all types of cells (6). Microglial GPCRs have been implicated in control of axon outgrowth and cortical laminar positioning during development as well as in support of survival of neurons (CX3CR1), in plasticity (P2Y12) and complement-mediated pruning (C3AR1 and C5AR1) of synapses, in microglial brain colonization (CXCR4) and chemotaxis of microglia to injury (P2Y12), and in neuropathic pain response (P2Y12) (7). Exploring bulk and single cell RNAseq studies of microglia from mice and human, we here describe the expression of GPCR and G protein genes in relation to microglia homeostasis, location, health, and disease.
Genome-wide gene expression data of microglia, macrophages, and non-phagocytic cells were derived from various publicly available RNAseq data sets (Table 1). Numbers indicating gene expression are provided either as absolute counts, presenting fragments per kilobase of transcript per million mapped reads (FPKM) or transcripts per kilobase million (TPM), or as relative counts, related to arbitrary chosen, fixed thresholds of all genes in the gene set (e.g., the top 50% = 0.50 percentile). Heatmaps show gene expression intensity, based on the average of all genes, with white indicating low expression and red indicating high expression. t-distributed stochastic neighbor embedding (t-SNE) plots of the clusters of microglia were generated as described (15). Relative RNA expression levels for CXCR4 and CXCL12 from laser-dissected tissue from mixed active/inactive and inactive demyelinated lesions were obtained from a microarray dataset (18).
Isolated human microglia from corpus callosum and subcortical white matter and occipital cortex grey matter were isolated and stained as described (4) with APC-conjugated anti-CD11b (clone ICRF44; eBioscience, San Diego, CA, USA), Alexa 700-conjugated anti-CD14 (clone MφP9; BD Biosciences, San Diego, CA, USA), PerCP-Cy5.5-conjugated anti-CD15 (clone HI98; BioLegend, San Diego, CA, USA), BB515-conjugated anti-CD45 (clone HI30; BioLegend), PE-Cy7-conjugated anti-CXCR4 (clone 12G5; BioLegend), and PE-conjugated anti-P2Y12 (clone S16007D; BioLegend). Dead cells were visualized by fixable viability dye eFluor 780 (eBioscience). Background staining was determined using fluorescence minus one control. Membrane protein expression was measured on a FACSCanto II (BD Biosciences), and median fluorescence intensity was determined with FlowJo software version 10.1 (Ashland, OR, USA).
Human brain microglia and paired peripheral blood monocytes were separately isolated by CD11b and CD14 microbeads (Miltenyi Biotec, Bergisch Gladbach, Germany) as described (19). 2 x 105 cells were loaded in a volume of 100 μl RPMI1640 medium containing 0.3% bovine serum albumin on transwell filters with a pore size of 5 μm (Corning, Corning, NY, USA). 100 ng/ml SDF-1 (stromal cell-derived factor-1, CXCL12) was added as chemoattractant to the lower compartment. After 4 h incubation at 37°C, cells in the lower compartment were harvested and quantified by flow cytometry at a fixed high speed for 120 sec.
All analyses were performed in GraphPad Prism 7 (GraphPad Software, San Diego, CA, USA). When data were not normally distributed, non-parametric tests, either Wilcoxon or Mann-Whitney U, were performed.
To explore the presence of GPCRs in microglia, we utilized the list of GPCRs not involved in olfaction, taste, light perception, and pheromone signaling as provided by the International Union of Basic and Clinical Pharmacology (IUPHAR)/British Pharmacological Society (BPS) Guide to Pharmacology (https://www.guidetopharmacology.org) (20). According to the GRAFS classification (21), the 384 receptors comprise 303 rhodopsin, 33 adhesion, 22 glutamate, 15 secretin, and 11 frizzled family members.
To identify GPCR genes that are reliably expressed in human microglia, we first tested bulk RNAseq expression data of homeostatic microglia from different vertebrate species we recently published (8). We found 83 GPCR genes in the top 50% (0.5 percentile) of all genes in the human and/or mouse gene sets (Figure 1A). 42 of these genes were lowly expressed (0.5–0.67 percentile), 12 genes were medium expressed (0.68–0.85 percentile), and 29 genes belonged to the highly expressed genes (0.86–1.0 percentile). While the homeostatic microglia marker genes CX3CR1, GPR34, GPR183, P2RY12, P2RY13, and ADGRG1 (encoding GPR56) were highly expressed in both species, expression of other GPCR genes was medium, low, or even absent in either human or mouse. For example, transcription of ADGRE1, encoding F4/80 in mouse, was lacking in human microglia, in line with its exclusive expression in human eosinophils (22). Other genes with a restricted, high expression in either mouse or human microglia were CCR6, GPR84, GPR146, and FPR1, respectively. Notably, ADGRE5 (encoding CD97), which is abundantly expressed by all types of bone marrow-derived leukocytes (23) was lowly expressed in mouse and human microglia, in line with previous findings (24). The previously disputed gene ADGRB1 (encoding BAI1) (25) was undetectable in mouse and human microglia.
Figure 1 Identification of GPCR and GPCR signaling molecule genes expressed in microglia. (A) Scatter plot providing GPCR gene expression in microglia isolated from human and mice as percentile of all human/mouse genes (8). (B) Scatter plot providing GPCR gene expression in brain and microglia of human as percentile of all genes (10). (C) Expression of GPCR and GPCR signaling molecule genes in mouse cortical tissue, neurons, astrocytes, oligodendrocytes, and microglia (11), and in mouse microglia, non-parenchymal brain and peripheral tissue macrophages (12). Expression is provided as FPKM (11) and gene count (12), respectively. mac, macrophages.
We confirmed the list of well-expressed human microglia GPCR genes in four other bulk RNAseq studies of primary human microglia [(4, 9, 10) and Mizee et al, unpublished data]. We found 15 genes abundantly expressed across the different data sets, which formed, together with two genes with a medium expression in grey matter microglia but high expression in white matter microglia (see below), the core microglia GPCR gene set for our further analyses (for all other genes, see the supplementary information). This gene set comprises rhodopsin (e.g., adenosine, chemokine, complement peptide, lysophospholipid, purinergic, and orphan receptors) as well as adhesion, but not secretin, glutamate, or frizzled family members. Among the 17 selected genes were the homeostatic microglia marker genes CX3CR1, GPR34, GPR183, P2RY12, P2RY13, and ADGRG1.
We next tested the expression of microglial GPCRs in other cell types of the CNS. The three most highly expressed genes, ADGRG1, P2RY12, and CX3CR1, also appeared top-abundant in whole brain tissue (10) (Figure 1B). All selected genes were more abundantly expressed in pure microglia as compared to the whole human cortex, which was further corroborated by data on gene expression in the major cell types of the mouse CNS (11) (Figure 1C and Supplementary Table 1). Except for Cxcr4, expression in microglia was higher as compared to neurons, astrocytes, and oligodendrocytes. Interestingly, high expression in microglia regularly correlated with gene activity in oligodendrocytes, albeit at a lower level.
Next to parenchymal microglia, the CNS harbors border-associated macrophages with distinct transcriptional signatures, residing in the dura mater, subdural meninges, and choroid plexus (12). Most microglia GPCRs were expressed also in non-parenchymal macrophages of the CNS, at comparable, higher, or lower level (Figure 1C and Supplementary Table 1). Inclusion of tissue-resident macrophages from peritoneum, lung, and liver unveiled similar patterns (12). The signature genes Cx3cr1, Gpr34, P2ry12, P2ry13, and Adgrg1 were particularly expressed in microglia.
Upon ligation, GPCRs diversify downstream signaling through four main classes of Gα subunit – Gαs, Gαi/o, Gαq/11, and Gα12/13 (6). Moreover, G protein-coupled receptor kinases (GRKs) phosphorylate intracellular domains of GPCRs and function together with β-arrestin to regulate the GPCR desensitization (6). Human and mouse microglia expressed GNAI2 (Gαi2), GNAS (Gαs), GNA13 (Gα13), GRK2, and ARRB2. In addition, mouse microglia also expressed Gna15 (Gα15) (Figure 1C and Supplementary Table 1). Gnai2, Gna15, Grk2, and Arrb2 were higher expressed in microglia as compared to neurons, astrocytes, and oligodendrocytes, while Gnas and Gna12 were expressed in different cell types. G protein, GRK, and β-arrestin gene expression levels in macrophages in- and out-side the CNS were mostly comparable.
Dissected from their natural microenvironment, microglia change their transcriptional program and alter morphological and functional characteristics (10, 19). Indeed, expression of ADORA3, ADRB2, CXCR4, CX3CR1, GPR34, LPAR5, LPAR6, P2RY12, P2RY13, and ADGRG1 was strongly downregulated or even lost in microglia cultured ex vivo for 1 or 7 days (Figure 2 and Supplementary Table 2).
Figure 2 Gene expression of selected GPCRs and GPCR signaling molecules in human microglia. Expression of GPCR and GPCR signaling molecule genes in microglia cultured ex vivo for 1 and 7 days (10), in iPSC-derived iMPs transplanted neonatal mouse brains or cultured in vitro for 10 and 60 days (13), and in white matter and grey matter microglia from control and MS brains (4). Expression is provided as TPM (10) and gene count (4, 13), respectively. CON, control; WM, white matter; GM, grey matter.
Human induced pluripotent stem cells (iPSCs) can be differentiated into induced microglial precursors cells (iMPs) showing the characteristic morphology and gene expression of primary human microglia (13). Notably, iMPs expressed the GPCRs typically found in microglia. When transplanted into the brains of NOD scid gamma (NSG) mice, carrying the human transgenes encoding IL-3, SCF, GM-CSF, and CSF1, expression of ADRB2, CX3CR1, GPR183, FPR1, LPAR5, LPAR6, P2RY12, P2RY13, and ADGRG1 was further enhanced at day 10 and/or 60 (Figure 2 and Supplementary Table 2). This induction was not seen in iMPs cultured for the same period in vitro, which rather resulted in a downregulation of the expression of several GPCRs.
Various studies have established regional differences in microglia gene expression (2). Using data from our laboratory, we compared GPCR expression between human microglia obtained from subcortical white and cortical grey matter (4) (Figure 2 and Supplementary Table 2). We found seven genes ≥2-fold higher expressed in either white or grey matter, respectively. In a second, independent dataset, we could confirm a higher gene expression in white matter microglia for CXCR4 and PTGER4 (Mizee et al, unpublished data). Single cell RNAseq has facilitated the investigation of microglial heterogeneity within brain regions. A study addressing this question showed, with the exception of C3AR1, a similar activity of GPCR genes in cortical microglia subsets (16) (Supplementary Figure 1).
Changes in gene expression in mouse models for Alzheimer’s disease and amyotrophic lateral sclerosis (ALS) have led to the description of a phenotype referred to as disease-associated microglia, associated with significantly reduced expression of several signature GPCR genes (14). When comparing gene expression in pure microglia from normal-appearing, non-lesional tissue of deceased multiple sclerosis (MS) autopsy cases and tissue of non-pathological brains (Figure 2 and Supplementary Table 2), we found downregulation of ADGRG1 in both, white and grey matter, while expression of other GPCR genes was not changed.
Single cell RNAseq data of (models of) Alzheimer’s disease, ALS, and MS revealed that pathological reprogramming only occurs in small subsets of disease-associated microglia that coexist with large subsets of homeostatic microglia and small subsets of infiltrating monocytes (14–16). Notably, disease-associated microglia in lesion biopsies of patients with histologically confirmed early active MS pathology showed reduced expression of the core signature genes CX3CR1, GPR34, GPR183, P2RY12, P2RY13, and ADGRG1 (15, 26) (Figures 3A, B). In contrast, both disease-associated microglia and infiltrating monocytes had upregulated expression of CXCR4. In disease-associated microglia in surgically resected material of Alzheimer’s disease patients, GPCR expression was not altered (16).
Figure 3 Gene expression of selected GPCRs and GPCR signaling molecules in MS lesions. (A) t-SNE plot of 1,602 individual cells isolated from five non-pathological brains (healthy) and five brains of patients with early active MS representing with indicated homeostatic microglia, MS- enriched/associated microglia, monocytes, and lymphocytes. (B, C) t-SNE plots of GPCR (B) and GPCR signaling molecule (C) genes (15). Color codes represent expression levels.
The late onset of neurodegenerative diseases, such as Alzheimer’s disease, Parkinson’s disease, and ALS, has triggered interest in the effect of aging on microglia gene expression. A study of aged microglia found a lower expression of the microglia signature genes GPR183, P2RY12, and P2RY13 (17).
Expression of GPCR signaling molecule genes in the data sets studied here was quite stable. Separation of microglia from their CNS microenvironment or transfer of iMPs into NSG mouse brains only moderately affected signaling molecule gene expression (Figure 2). However, white matter microglia more abundantly expressed GNA13 as compared to grey mater microglia. GPCR signaling molecule genes expression was not altered in normal-appearing or lesional MS microglia from either white or grey matter (Figure 3C).
The presence and MS-associated upregulation of CXCR4 expression in microglia is of particular interest since Werner et al. recently showed that CXCR4 distinguishes monocytes from microglia in mice (27). To test whether human microglia express CXCR4, we analyzed freshly isolated cells by flow cytometry, shown here for three donors with MS (Figure 4A). Expression was detectable, albeit levels were moderate on white matter microglia and low on grey matter microglia (Figures 4A, B). On lesional microglia, we noticed a slightly higher expression of CXCR4 as well as lower expression of P2Y12 compared to microglia from subcortical normal-appearing white matter, the latter in line with Zrzavy et al. (26) (Figures 4A, C). Whole tissue gene expression microarray analysis of laser-dissected control white matter and white matter MS lesions (18) further confirmed increased expression of CXCR4 in the rim of mixed active/inactive but not inactive lesions (Figure 4D).
Figure 4 Expression of functional CXCR4 in white and grey matter microglia in health and MS. (A) Representative dot plots of CXCR4 expression on paired peripheral CD14+ blood monocytes and P2Y12+ microglia from corpus callosum white matter and occipital cortex grey matter, as well as P2Y12+ microglia from peri-lesional and lesional subcortical WM of three MS brain donors measured by flow cytometry. (B) Quantification of CXCR4 expression (percentage of positive cells and geoMFI) on monocytes, WM microglia, and GM microglia of n=11 brain donors (3 MS, 8 non-MS; Wilcoxon-signed rank test; ***p < 0.0005). (C) Quantification of CXCR4 and P2Y12 expression on microglia from peri-lesional and lesional WM of three MS brain donors. (D) Quantification of tissue gene expression of CXCR4 in control as well as peri-lesional and lesional WM from mixed active/inactive and inactive MS lesions (18) (Wilcoxon-signed rank test; *p < 0.05). (E) Quantification of transwell migration of monocytes, white matter microglia, and grey matter microglia in response to the CXCR4 ligand SDF-1 of n=4 brain donors (left panel). Of note, SDF-1-stimulated transwell migration was higher as compared to spontaneous transwell migration in all three cell types (n=1 brain donor; right panel). A donor with a relatively low expression of CXCR4 on monocytes also showed low monocyte transmigration towards SDF-1 (grey arrowhead). mono, monocytes; CC, corpus callosum; WM, white matter; OC, occipital cortex; GM, grey matter; PL, peri-lesional; Les, lesional; mA/I, mixed active/inactive; geoMFI, geometric mean fluorescence intensity.
To test whether expression of CXCR4 on microglia is functional, we studied transwell migration in response to SDF-1 (CXCL12) (14). SDF-1 binds to CXCR4 and CXCR7, the latter however is not expressed by microglia (Supplementary Table 2). Of note SDF-1 stimulated chemotaxis of monocytes, white matter and grey matter microglia at levels corresponding with the presence of CXCR4 (Figure 4E). We conclude that CXCR4 expression on human microglia is functional.
GPCRs constitute an important share of the sensome of eukaryotic cells. By analyzing various RNAseq datasets, we here provide a comprehensive overview of their presence in microglia. We identified 17 GPCR genes that are robustly transcribed in adult human microglia, including the homeostatic core genes CX3CR1, GPR34, GPR183, P2RY12, P2RY13, and ADGRG1, but also ADORA3, ADRB2, CCR1, C3AR1, C5AR1, LPAR5, LPAR6, and PTAFR. GPCRs genes well expressed in human microglia but hardly found in mouse microglia were CXCR4, FPR1, and PTGER4.
Figure 5 summarizes the findings of this study. As expected, isolated microglia rapidly lost expression of several GPCRs when cultured ex vivo, which limits the value of in vitro-expanded primary cells for functional studies. In contrast, iPSC-derived iMPs had a GPCR expression remarkably similar to primary microglia, which further equalized upon transfer into NSG mouse brains, ectopically providing critical human growth factors building the microglia phenotype. Regional diversity of microglia has been suggested (2), and we indeed found more abundant expression of CXCR4 and PTGER4 in white matter as compared to grey matter microglia in two independent studies [(4) and Mizee et al., unpublished data].
Figure 5 Tabular summary of human microglia GPCR gene regulation, signaling, and drug availability. See Discussion for details. Arrows indicate the direction of regulation. Colored dots indicate prevalence of signaling of GPCRs through the respective G proteins (red = high; light red = medium) and abundance of gene expression of the G proteins in microglia (large dots = high; small dots = low). WM, white matter; GM, grey matter; NAGM, normal-appearing grey matter; NAWM, normal-appearing white matter.
Disease-associated microglia in mouse models of AD and ALS downregulate expression of various GPCR genes, including Cx3cr1, P2ry12, P2ry13, and Adgrg1 (14). The data presented here refer to MS, an inflammatory demyelinating and neurodegenerative disease. Bulk primary microglia collected post-mortem from normal-appearing tissue of MS donors showed downregulation of ADGRG1 (4) in grey and white matter. ADGRG1 encodes the adhesion family GPCR GPR56 (28), which is more widely expressed in the CNS and has been linked in neuronal precursors with cortical lamination and in oligodendrocyte precursors with proliferation (29). Abundant presence of GPR56 on human microglia (4) and regulation of synaptic refinement through a mouse GPR56 splicing isoform (30) have been reported only recently. Obviously, ADGRG1 expression not only distinguishes microglia from other macrophages but also fades out in response to minor changes in the microenvironment, stressing its value as indicator of microglia homeostasis. In biopsies of active lesions in early cases of MS, diseases-associated microglia subsets showed the expected general downregulation of GPCR genes associated with microglia homeostasis. Single-cell mass cytometry indeed confirmed the appearance of active MS lesion-enriched clusters with a down-regulated surface expression of P2Y12, CX3CR1, and GPR56 (31).
The distribution of the chemokine receptor CXCR4 is of particular interest. CXCR4 binds CXCL12 (SDF-1), which is expressed by the brain vasculature and upregulated in MS lesions (32). Werner et al. recently reported that CXCR4 distinguishes brain-infiltrating monocytes from resident microglia in mice (27). We here demonstrate that human microglia from white and – to a lesser extent – grey matter express CXCR4. Transmigration towards SDF-1 confirmed the relevance of the presence of CXCR4 on microglia. Expression of CXCR4 by both microglia and infiltrating monocytes and upregulation by small subset of MS-associated lesional microglia (15) makes this chemokine receptor, involved in immune cell homeostasis and margination (33), a potentially interesting target for therapeutic intervention. Indeed, Cxcr4 gene ablation reduced monocyte infiltration and response gene expression in experimental stroke in mice (27).
When testing the expression of genes encoding signaling molecules downstream of GPCRs, in particular Gα proteins, GRKs, and β-arrestin (6), we found robust expression of GNAI2, GNAS, GNA13, GRK2, and ARRB2. Further, mice, but not human, abundantly expressed Gna15. Expression of these genes did not depend on the specific microenvironment of microglia and was found at similar levels in other macrophages. Gαs-coupled receptors activate adenylate cyclase, leading to cAMP accumulation. While GNAS transcripts were abundant in microglia, only two highly expressed GPCRs primarily couple to this Gα subunit (ADRB2, PTGER4) suggesting rather limited augmentation of cAMP levels in microglia through GPCR signaling. Gαi-coupled receptors inhibit the cAMP-dependent pathway by inhibiting adenylyl cyclase activity. Our data indicate that Gαi, the preferred Gα subunit of 12 of the 17 abundant microglia GPCRs, is well expressed in microglia, implying inhibition of cAMP-dependent protein kinase (PKA). Gα12/13-coupled receptors activate the small GTPase RhoA. We found GNA13 abundantly transcribed in white but not grey matter, which explains why initial studies of cortical microglia reported dim expression (10). Finally, Gαq-coupled receptors activate phospholipase C to increase intracellular calcium concentration as well as activate protein kinase C, which results in Raf kinase activation of the MAPK pathway. GNAQ transcript levels were generally low, suggesting that LPAR5, LPAR6, and GPR56, which can engage Gα12/13 as well as Gαq, may rather control cell cytoskeleton remodeling and thus regulate microglia migration. Yet, in particular for GPR56, molecular mechanisms additional to G protein signaling may apply (28, 34).
GPCRs are known for their excellent drugability. A survey at DrugBank (https://www.drugbank.ca/) revealed approved drugs against at least five highly expressed microglia GPCRs with indications covering, amongst others, conditions of the lungs (ADORA3, ADRB2), eye (ADRB2), blood (CXCR4, P2Y12), heart (P2Y12), and uterus (PTGER4). Moreover, rodent models suggest efficacy of CXCR4 targeting in the treatment of stroke and glioma (35). Studying the effects of small molecules, penetrating the blood–brain barrier, on microglia in vitro and in vivo may disclose novel opportunities for the treatment of brain diseases in which microglia emerge as central players, including neurodevelopmental disorders (e.g., autism), neurodegenerative and -inflammatory conditions (e.g., Alzheimer’s disease and MS), and chronic pain (1).
In summary, we here describe the GPCR repertoire of human microglia based on publicly available bulk and single-cell RNAseq data. GPCRs that belong to the core signature of microglia are abundantly expressed under homeostatic and rapidly downregulated under non-homeostatic conditions, making them interesting models for studying microenvironmental factors that shape microglia identity during brain development and disease. Datasets of microglia from brain donors with neurological diseases only lately became available and require further investigation. This in particular holds true as drugs targeting different highly expressed microglia GPCRs have been developed, implying their potential application for CNS diseases in which microglia figurate.
The datasets presented in this study can be found in online repositories. The names of the repository/repositories and accession number(s) can be found below: https://www.ncbi.nlm.nih.gov/ (accessible under accession code GEO: GSE52564, GSE98969, GSE99074, GSE108000, GSE111972, GSE124335, GSE128855, GSE134707, GSE139194), https://www.synapse.org/#!Synapse:syn21438358 and http://shiny.maths.usyd.edu.au/Ellis/MicrogliaPlots/.
Written informed consent was obtained from the individual(s) for the publication of any potentially identifiable images or data included in this article.
C-CH and RS extracted and analyzed data. C-CH, RS, MP, JS, IH, and JH designed research and interpreted results. C-CH and JH wrote the paper. All authors contributed to the article and approved the submitted version.
The German Research Foundation (FOR 2149 – JH), the Berta-Ottenstein-Programme for Clinical Scientists (RS), the MS Research Foundation (MS 13-830 – IH/JH), and the Nationaal MS Fonds (OZ2018-003 – JS) funded this research.
The authors declare that the research was conducted in the absence of any commercial or financial relationships that could be construed as a potential conflict of interest.
The Supplementary Material for this article can be found online at: https://www.frontiersin.org/articles/10.3389/fimmu.2021.674189/full#supplementary-material
1. Salter MW, Stevens B. Microglia Emerge as Central Players in Brain Disease. Nat Med (2017) 23:1018–27. doi: 10.1038/nm.4397
2. Prinz M, Jung S, Priller J. Microglia Biology: One Century of Evolving Concepts. Cell (2019) 179:292–311. doi: 10.1016/j.cell.2019.08.053
3. Crotti A, Ransohoff RM. Microglial Physiology and Pathophysiology: Insights From Genome-wide Transcriptional Profiling. Immunity (2016) 44:505–15. doi: 10.1016/j.immuni.2016.02.013
4. van der Poel M, Ulas T, Mizee MR, Hsiao CC, Miedema SSM, Adelia, et al. Transcriptional Profiling of Human Microglia Reveals Grey–White Matter Heterogeneity and Multiple Sclerosis-Associated Changes. Nat Commun (2019) 10:1139. doi: 10.1038/s41467-019-08976-7
5. Hauser AS, Attwood MM, Rask-Andersen M, Schiöth HB, Gloriam DE. Trends in GPCR Drug Discovery: New Agents, Targets and Indications. Nat Rev Drug Discov (2017) 16:829–42. doi: 10.1038/nrd.2017.178
6. Pierce KL, Premont RT, Lefkowitz RJ. Seven-Transmembrane Receptors. Nat Rev Mol Cell Biol (2002) 3:639–50. doi: 10.1038/nrm908
7. Li Q, Barres BA. Microglia and Macrophages in Brain Homeostasis and Disease. Nat Rev Immunol (2018) 18:225–42. doi: 10.1038/nri.2017.125
8. Geirsdottir L, David E, Keren-Shaul H, Weiner A, Bohlen SC, Neuber J, et al. Cross-Species Single-Cell Analysis Reveals Divergence of the Primate Microglia Program. Cell (2019) 179:1609–22.e16. doi: 10.1016/j.cell.2019.11.010
9. Galatro TF, Holtman IR, Lerario AM, Vainchtein ID, Brouwer N, Sola PR, et al. Transcriptomic Analysis of Purified Human Cortical Microglia Reveals Age-Associated Changes. Nat Neurosci (2017) 20:1162–71. doi: 10.1038/nn.4597
10. Gosselin D, Skola D, Coufal NG, Holtman IR, Schlachetzki JCM, Sajti E, et al. An Environment-Dependent Transcriptional Network Specifies Human Microglia Identity. Science (2017) 356:1248–59. doi: 10.1126/science.aal3222
11. Zhang Y, Chen K, Sloan SA, Bennett ML, Scholze AR, O’Keeffe S, et al. An RNA-sequencing Transcriptome and Splicing Database of Glia, Neurons, and Vascular Cells of the Cerebral Cortex. J Neurosci (2014) 34:11929–47. doi: 10.1523/JNEUROSCI.1860-14.2014
12. Van Hove H, Martens L, Scheyltjens I, De Vlaminck K, Pombo Antunes AR, De Prijck S, et al. A Single-Cell Atlas of Mouse Brain Macrophages Reveals Unique Transcriptional Identities Shaped by Ontogeny and Tissue Environment. Nat Neurosci (2019) 22:1021–35. doi: 10.1038/s41593-019-0393-4
13. Svoboda DS, Barrasa MI, Shu J, Rietjens R, Zhang S, Mitalipova M, et al. Human iPSC-derived Microglia Assume a Primary Microglia-Like State After Transplantation Into the Neonatal Mouse Brain. Proc Natl Acad Sci USA (2019) 116:25293–303. doi: 10.1073/pnas.1913541116
14. Keren-Shaul H, Spinrad A, Weiner A, Matcovitch-Natan O, Dvir-Szternfeld R, Ulland TK, et al. A Unique Microglia Type Associated With Restricting Development of Alzheimer’s Disease. Cell (2017) 169:1276–1290.e17. doi: 10.1016/j.cell.2017.05.018
15. Masuda T, Sankowski R, Staszewski O, Böttcher C, Amann L, Sagar, et al. Spatial and Temporal Heterogeneity of Mouse and Human Microglia At Single-Cell Resolution. Nature (2019) 566:388–92. doi: 10.1038/s41586-019-0924-x
16. Olah M, Menon V, Habib N, Taga MF, Ma Y, Yung CJ, et al. Single Cell RNA Sequencing of Human Microglia Uncovers a Subset Associated With Alzheimer’s Disease. Nat Commun (2020) 11:6129. doi: 10.1038/s41467-020-19737-2
17. Olah M, Patrick E, Villani AC, Xu J, White CC, Ryan KJ, et al. A Transcriptomic Atlas of Aged Human Microglia. Nat Commun (2018) 9:539. doi: 10.1038/s41467-018-02926-5
18. Hendrickx DAE, van Scheppingen J, van der Poel M, Bossers K, Schuurman KG, van Eden CG, et al. Gene Expression Profiling of Multiple Sclerosis Pathology Identifies Early Patterns of Demyelination Surrounding Chronic Active Lesions. Front Immunol (2017) 8:1810. doi: 10.3389/fimmu.2017.01810
19. Mizee MR, Miedema SSM, van der Poel M, Adelia, Schuurman KG, van Strien ME, et al. Isolation of Primary Microglia From the Human Post-Mortem Brain: Effects of Ante- and Post-Mortem Variables. Acta Neuropathol Commun (2017) 5:16. doi: 10.1186/s40478-017-0418-8
20. Alexander SPH, Christopoulos A, Davenport AP, Kelly E, Mathie A, Peters JA, et al. The CONCISE Guide TO Pharmacology 2019/20: G Protein-Coupled Receptors. Br J Pharmacol (2019) 176:S21–141. doi: 10.1111/bph.14748
21. Schiöth HB, Lagerström MC. Structural Diversity of G Proteincoupled Receptors and Significance for Drug Discovery. Nat Rev Drug Discov (2008) 7:339–57. doi: 10.1038/nrd2518
22. Hamann J, Koning N, Pouwels W, Ulfman LH, van Eijk M, Stacey M, et al. EMR1, the Human Homolog of F4/80, is an Eosinophil-Specific Receptor. Eur J Immunol (2007) 37:2797–802. doi: 10.1002/eji.200737553
23. Lin HH, Hsiao CC, Pabst C, Hébert J, Schöneberg T, Hamann J. Adhesion GPCRs in Regulating Immune Responses and Inflammation. Adv Immunol (2017) 136:163–201. doi: 10.1016/bs.ai.2017.05.005
24. Visser L, De Vos AF, Hamann J, Melief MJ, Van Meurs M, Van Lier RAW, et al. Expression of the EGF-TM7 Receptor CD97 and its Ligand CD55 (DAF) in Multiple Sclerosis. J Neuroimmunol (2002) 132:156–63. doi: 10.1016/S0165-5728(02)00306-5
25. Hsiao CC, Van Der Poel M, Van Ham TJ, Hamann J. Macrophages do Not Express the Phagocytic Receptor BAI1/ADGRB1. Front Immunol (2019) 10:962. doi: 10.3389/fimmu.2019.00962
26. Zrzavy T, Hametner S, Wimmer I, Butovsky O, Weiner HL, Lassmann H. Loss of “Homeostatic” Microglia and Patterns of Their Activation in Active Multiple Sclerosis. Brain (2017) 140:1900–13. doi: 10.1093/brain/awx113
27. Werner Y, Mass E, Ashok Kumar P, Ulas T, Händler K, Horne A, et al. Cxcr4 Distinguishes HSC-derived Monocytes From Microglia and Reveals Monocyte Immune Responses to Experimental Stroke. Nat Neurosci (2020) 23:351–62. doi: 10.1038/s41593-020-0585-y
28. Hamann J, Aust G, Araç D, Engel FB, Formstone C, Fredriksson R, et al. International Union of Basic and Clinical Pharmacology. XCIV. Adhesion G Protein-Coupled Receptors. Pharmacol Rev (2015) 67:338–67. doi: 10.1124/pr.114.009647
29. Langenhan T, Piao X, Monk KR. Adhesion G Protein-Coupled Receptors in Nervous System Development and Disease. Nat Rev Neurosci (2016) 17:550–61. doi: 10.1038/nrn.2016.86
30. Li T, Chiou B, Gilman CK, Luo R, Koshi T, Yu D, et al. A Splicing Isoform of GPR56 Mediates Microglial Synaptic Refinement Via Phosphatidylserine Binding. EMBO J (2020) 39:e104136. doi: 10.15252/embj.2019104136
31. Böttcher C, Van Der Poel M, Fernández-Zapata C, Schlickeiser S, Leman JKH, Hsiao CC, et al. Single-Cell Mass Cytometry Reveals Complex Myeloid Cell Composition in Active Lesions of Progressive Multiple Sclerosis. Acta Neuropathol Commun (2020) 8:136. doi: 10.1186/s40478-020-01010-8
32. Krumbholz M, Theil D, Cepok S, Hemmer B, Kivisäkk P, Ransohoff RM, et al. Chemokines in Multiple Sclerosis: CXCL12 and CXCL13 Up-Regulation is Differentially Linked to CNS Immune Cell Recruitment. Brain (2006) 129:200–11. doi: 10.1093/brain/awh680
33. Nagasawa T. CXC Chemokine Ligand 12 (CXCL12) and its Receptor CXCR4. J Mol Med (2014) 92:433–9. doi: 10.1007/s00109-014-1123-8
34. Langenhan T, Aust G, Hamann J. Sticky Signaling - Adhesion Class G Protein-Coupled Receptors Take the Stage. Sci Signal (2013) 6:re3. doi: 10.1126/scisignal.2003825
Keywords: brain, microglia, GPCRs, G proteins, system biology, multiple sclerosis, CXCR4
Citation: Hsiao C-C, Sankowski R, Prinz M, Smolders J, Huitinga I and Hamann J (2021) GPCRomics of Homeostatic and Disease-Associated Human Microglia. Front. Immunol. 12:674189. doi: 10.3389/fimmu.2021.674189
Received: 28 February 2021; Accepted: 22 April 2021;
Published: 14 May 2021.
Edited by:
Amanda Sierra, Achucarro Basque Center for Neuroscience, SpainReviewed by:
Angela Schulz, Leipzig University, GermanyCopyright © 2021 Hsiao, Sankowski, Prinz, Smolders, Huitinga and Hamann. This is an open-access article distributed under the terms of the Creative Commons Attribution License (CC BY). The use, distribution or reproduction in other forums is permitted, provided the original author(s) and the copyright owner(s) are credited and that the original publication in this journal is cited, in accordance with accepted academic practice. No use, distribution or reproduction is permitted which does not comply with these terms.
*Correspondence: Cheng-Chih Hsiao, Yy5oc2lhb0BhbXN0ZXJkYW11bWMubmw= ; Jörg Hamann, ai5oYW1hbm5AYW1zdGVyZGFtdW1jLm5s
Disclaimer: All claims expressed in this article are solely those of the authors and do not necessarily represent those of their affiliated organizations, or those of the publisher, the editors and the reviewers. Any product that may be evaluated in this article or claim that may be made by its manufacturer is not guaranteed or endorsed by the publisher.
Research integrity at Frontiers
Learn more about the work of our research integrity team to safeguard the quality of each article we publish.