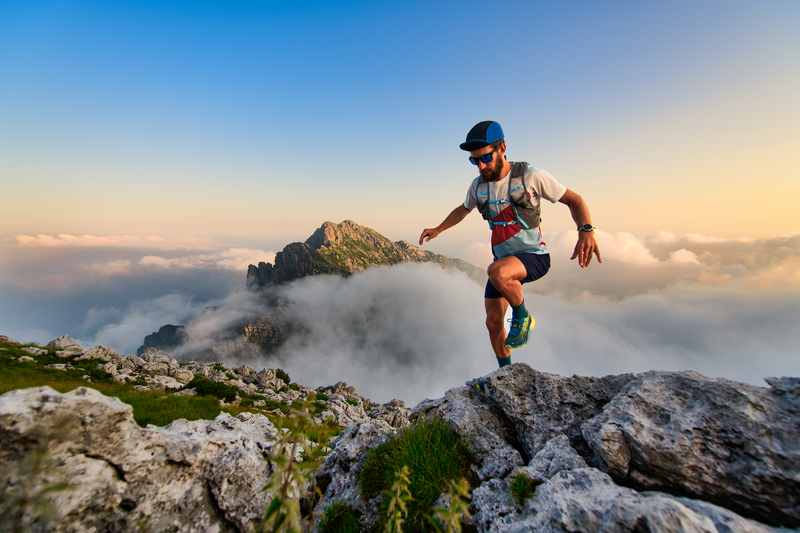
95% of researchers rate our articles as excellent or good
Learn more about the work of our research integrity team to safeguard the quality of each article we publish.
Find out more
SYSTEMATIC REVIEW article
Front. Immunol. , 08 July 2021
Sec. Viral Immunology
Volume 12 - 2021 | https://doi.org/10.3389/fimmu.2021.663912
The Spike (S) protein of the SARS-CoV-2 virus is critical for its ability to attach and fuse into the host cells, leading to infection, and transmission. In this review, we have initially performed a meta-analysis of keywords associated with the S protein to frame the outline of important research findings and directions related to it. Based on this outline, we have reviewed the structure, uniqueness, and origin of the S protein of SARS-CoV-2. Furthermore, the interactions of the Spike protein with host and its implications in COVID-19 pathogenesis, as well as drug and vaccine development, are discussed. We have also summarized the recent advances in detection methods using S protein-based RT-PCR, ELISA, point‐of‐care lateral flow immunoassay, and graphene-based field-effect transistor (FET) biosensors. Finally, we have also discussed the emerging Spike mutants and the efficacy of the Spike-based vaccines against those strains. Overall, we have covered most of the recent advances on the SARS-CoV-2 Spike protein and its possible implications in countering this virus.
The coronaviruses belong to the Nidovirales order, Coronaviridae family, which is divided into Alphacoronavirus, Betacoronavirus, Deltacoronavirus, and Gammacoronavirus genera (1). To date, the Betacoronavirus is the most studied genera, as it includes airborne viral species, such as severe acute respiratory syndrome–related coronaviruses (SARS-CoV) and Middle East respiratory syndrome coronaviruses (MERS-CoV), which can infect humans. In 2019, a new coronavirus, named as severe acute respiratory syndrome coronavirus (SARS-CoV-2), emerged in Wuhan, Hubei province, China (2, 3). Despite the high similarity (88%) of the viral genome with SARS-like viruses (4), the new species exhibit an increased transmission rate in human populations (5). In fact, the World Health Organization (WHO) has declared the syndrome associated with SARS-CoV-2 as a public health emergency due to its rapid global spread and declared as COVID-19 pandemic. Since then, several research groups have focused on characterizing the molecular mechanisms involved in the infection process to identify therapeutic and prophylactic targets (6). Among the main structural proteins encoded by the SARS-CoV-2 genome, the Spike (S) protein is considered fundamental in the pathogenesis, transmission, and virulence of the virus because it binds to the human host cell membrane by interacting with the host angiotensin-converting enzyme 2 (hACE2) receptor (7, 8).
Given the importance of the S protein in the context of the COVID-19 pandemic, the objective of this study is to conduct an in-depth review based on available literature on the novel coronavirus (2019-SARS-CoV-2) S protein. Additionally, we applied two different meta-analysis approaches based on keywords associated with the S protein to generate the outline of this article and introduce the covered topics.
For the meta-analysis, we selected all the publications available in the PubMed database that matched the search for keywords (chloroquine, diagnostic, nCoV, Spike, treatment, vaccine), and a file containing the publication dates was obtained for each keyword. Subsequently, a filter was applied to obtain the number of publications per month, which made it possible to analyze the average number of publications per year, before and during the pandemic period characterized from January 01, 2020, until April 20, 2021. Thus, we estimated the statistical difference before the pandemic period and during the ongoing SARS-CoV-2 outbreak. The quantitative analysis of the number of publications in each period has little chance of bias, as it considers the entire set of data available in the database. Because the goal of our study was not to observe any specific clinical event, exclusion criteria were not considered; therefore, all the studies that were related to the coronavirus were added. This makes the meta-analysis presented here representative to perform comparative analyses. To carry out the statistical analysis, the effect sizes were performed by Hedges test. Thus, the values of the mean and standard deviation of publications were initially calculated. After obtaining the values of the effect sizes, the summary of the results and the final plot were generated in R using the publicly available “meta” package. The random effects model was selected because of the large differences found when comparing the before and during pandemic periods.
The values found in the different periods considered in our study are displayed in Supplementary Tables S1 and S2. As shown in Figure 1, among the results from PubMed related to our six keywords (namely “Chloroquine,” “Diagnostic,” “nCoV,” “Spike,” “Treatment,” “Vaccine”), “Vaccine” and “Spike” obtained the highest values of estimated treatment effect (TE) (14.44 ± 0.44 and 11.64 ± 0.43, respectively). This showed that both of these terms would obtain accurate and reliable results. According to the scientific articles in the literature, these two terms were also the most studied (high weights 16.6%). The confidence interval (CI), represented by the diamond, showed that the analysis was very precise and summarized the results well. The CI values, representing the estimates of the effect, and the heterogeneity value showed us that the results between the studies are very divergent (I2 = 98%).
Figure 1 Brief meta-analysis study based on the six keywords used. The white lines represent a large dataset for the selected keywords.
Afterward, a second meta-analysis methodology was implemented to define the outline of this review article (Figure 2). For this analysis, each selected keyword-associated Spike protein publication was assembled, and then, a statistical test was performed on the monthly publications associated with the keywords in accordance with the data before (until December 31, 2019) and during the pandemic. In this case, the hypothesis was that there would be a difference between the publications associated with these terms before and during the pandemic. In order to obtain the effect size and its standard error for each sample, the Hedge’s g test was performed. Therefore, the R package Effect Size Computation for Meta-Analysis (esc) v0.5.1 was used based on average values, standard deviation, and sample size within each group. Finally, the data were obtained using the Meta v4.13 R package and plotted using the forest function.
Figure 2 Introductory meta-analysis based on the average number of publications before and during the pandemic period.
The main topics addressed in this review are to the left of Figure 2, which indicates the paucity of studies for the relationship between Spike and the respective terms. This motivated our study and indicated that new studies are required to achieve positive correlations and associations.
The Spike protein is a surface-anchored viral glycoprotein that comprises the second-largest open reading frame (ORF) in the Coronavirus (CoV) genome (8, 9), with approximately 200 kDa, belonging to class I viral fusion proteins (7, 10). It is the main regulator of viral attachment because of its penetration into the host cell membrane. Moreover, S protein plays a key role in defining the virulence of the virus, determining target tissues, and host diversity (11). The S protein mediates the entry of CoVs into the host cell by interacting with different receptors, depending on the CoV’s genus: Alphacoronavirus, Betacoronavirus, Gammacoronavirus, or Deltacoronavirus (Figure 3) (7, 12). The CoV Spike proteins from different genera can bind to the same host cell receptor. For instance, alphacoronavirus HCoV-NL63 and the betacoronavirus SARS-CoV interact with a zinc peptidase angiotensin-converting enzyme 2 (ACE2) (12, 13). On the other hand, CoVs from the same genera can also recognize different host receptors, such as MERS, which recognizes dipeptidyl peptidase 4 (DPP4) and is included in the Betacorornavirus genus (14–16). The CoV Spike has three main domains: a small cytoplasmic domain, an ectodomain that contains most of the elements involved in connection with the host cell, including fusion, and a transmembrane domain (7, 17). The two major domains, S1 N-terminal (S1-NTD) and S1 C-terminal (S1-CTD), are mainly involved as receptor binding domains (RBD) (7). The NTDs are responsible for sugar binding and the CTDs interact with protein receptors, such as ACE2, aminopeptidase N (APN), and DPP4 (18–20). An exception is the S1-NTD from MERS-CoV that recognizes the protein receptor carcinoembryonic antigen family (CEACAM1) (21). Furthermore, some CoV species use sugars for entry into the cell. This is the case of the transmissible gastroenteritis virus (TGEV) and porcine epidemic diarrhea virus (PEDV) under the Alphacoronavirus genus, bovine coronavirus (BCoV), and human coronavirus OC43 under the Betacoronavirus genus and infectious bronchitis virus (IBV) under Gammacoronavirus genus (22).
Figure 3 Classification of coronaviruses and their respective mechanisms of host cell adhesion and entry.
Coronavirus Spikes are generally subject to proteolytic cleavage at specific sites by host proteases both during and after the entry into the cell, that influences both the viral membrane fusion and its life cycle (23, 24). This cleavage process is universally required in retroviruses, orthomyxoviruses, paramyxoviruses, filoviruses, and arenaviruses (25). For such cleavage, protein convertases, such as (i) Furin, can act on the cleavage site between the S1 and S2 domains (26). Moreover, trypsin can activate S protein and generates the formation of syncytium in 293/hACE2 cells (11). Other enzymes include (ii) extracellular proteases, such as elastase that acts after viral copies leave the host cells and cell surface proteases, such as type II transmembrane serine protease (TMPRSS2), and (iii) lysosomal cathepsin L and cathepsin B (Figure 4A).
Figure 4 (A) Entry of coronavirus into host cell and the role of protease cleavage in viral replication. (B) Schematic description of coronavirus Spike. S1-NTD: N-terminal domain; S1-CTD: C-terminal domain; RBD: Receptor Binding Domain; S1/S2: furin/multibasic cleavage site; S2: proteolysis site; FP (fusion peptide); Heptad Repeat Regions N and C (HR-N and HR-C); Transmembrane Anchor (TM); and the Intracellular Tail (IC). S1, receptor-binding subunit; S2, membrane fusion subunit; TM, transmembrane anchor; IC, intracellular tail. (C) 3D representation of CoVs Spike with RBD, furin S1/S2, and S2 cleavage domains. (D) Sequence comparison of the SARS-CoV-2 and other SARS-CoV Spike proteins on the region at the S1/S2 boundary, indicating the RRAR motif for SARS-CoV-2.
In the functional state, the Spike protein is found in two distinct conformations, the pre-fusion and post-fusion conformationS (7). The SARS-CoV-2 Spike is divided into two functional units, called S1 and S2 (10, 27, 28): subunit 1 contains the receptor-binding domain (RBD), formed by 200 amino acid residues that strongly binds to hACE2 (29). Within the RBD, the receptor binding motif (RBM) is responsible for direct interaction with hACE2 (30) (Figures 4B, C). Subunit 2 (S2) has two repeat regions (HR-C and HR-N), which forms a spiral over the ectodomain of the protein, separated by an inter-helical region of ~140 amino acids (28). The S1/S2 site in SARS-CoV-2 forms an exposed multibasic loop (Figure 4C) composed of 77 arginine residues (31). Interestingly, this region has not been found in other SARS-CoV–related coronaviruses, but they are present in the human coronaviruses OC43, HKU1, and MERS-CoV (32). Fusion of SARS-CoV-2 with lung cells is critically dependent on the S1/S2 cleavage site by furin protease, and its motif RXXR is closely related to the furin consensus sequence RX (K/R) R (32). Furthermore, the addition of an arginine residue to this motif strongly increases the syncytium formation of the host cells, indicating that the RRAR insertion in this region could enhance cell spread and pathogenicity of SARS-CoV-2 (Figure 4D) (32, 33).
The RBD domain of S protein is a potential target for developing vaccines and drugs, as it could block the RBD-hACE2 interaction and has antigenic properties. Nonetheless, the use of S protein has difficulties. An epitope mapping using the SARS-CoV proteome shows that 70% of the peptides that elicited T-cell responses come from various structural proteins (Spike, envelope, membrane, and nucleocapsid) (34, 35). Furthermore, as compared with the SARS-CoV S protein sequence, the SARS-CoV-2 has 75% identity, but the RBD region has 73.7% identity, and the most divergent point is precisely the RBM has 50% identity (30). Interestingly, the SARS-CoV-2 RBD not only has 17.1% identity with HCoV-NL63 but also uses the receptor ACE2 (36). This lower identity makes SARS-CoV antibodies inefficient or incapable of completely neutralizing SARS-CoV-2 (30, 37, 38). SARS-CoV-2 also brings several challenges in the development of efficient antibodies or vaccines because of its dynamic mutations in RBM within the RBD (30). Therefore, the use of SARS-CoV–derived antigens with little specificity for SARS-CoV-2 may generate the “original antigenic sin” (OAS), making the immune system susceptible to emerging SARS-like viruses (37), but cannot provide SARS-CoV-2–specific immunity. However, despite the low similarities in the RBM, the similarity in the RBD may have partial protection against SARS-CoV-2 (39). Furthermore, a conserved proteolytic cleavage site in the sequence KRSFIEDLLFNKV has been found to be highly conserved in SARS-CoV-2, as well as several other coronaviruses that might be highly resistant to mutations. Therefore, it may be a strong candidate for both drug and vaccine development (40).
Phylogenetic analysis of S glycoproteins has shown that SARS-CoV-2 and SARS-CoV share a similar ancestor (41). Conversely, some studies have predicted that SARS-CoV-2 Spike is highly similar to pangolins, indicating that pangolins could be the intermediate host (42–44). Furthermore, metagenomic analysis of the pangolins’ lung, spleen, lymph, and fecal samples detected SARS-CoV-2–like viral sequences only in the lung samples (73% coverage; 91% identity) (45). The authors further identified that the RBD of CoV in pangolins were different from SARS-CoV-2 in only five residues while the CoV from bat (RaTG13) displayed differences in 19 residues. The authors hypothesized that the pangolins could be an intermediate host, and the bat or the pangolins themselves could be the natural host of SARS-CoV-2. Moreover, it is also possible that the SARS-CoV-2 could have originated by the recombination of RaTG13 and pangolins CoVs (44). The emergence of novel infectious viruses could be facilitated through gene reassortment that occurs during co-infection of a host cell with multiple viruses, which facilitates crossing over. Such mutations and gene reassortment could face positive selection and lead to evolutionary arms race (46). Of the nine recombinations identified in the SARS-CoV-2 genome, six of them were identified in the gene encoding S protein (47). Despite such evidences based on S protein, nucleotide sequence identity of whole genome between SARS-CoV-2 and pangolin CoV is comparatively lower (90.23%) compared with the SARS-CoV-2 and Bat-CoV-RaTG13 (96.12%) (48). The similarity between Bat-CoV-RaTG13 and pangolin CoV was also comparatively lower (90.15%). Hence, there could be a greater possibility of SARS-CoV-2 arising from bat (48). Nonetheless, so far, there is no conclusive evidence of zoonotic origin of SARS-CoV-2 and research to find the origin of this virus needs full attention of the scientific community. The SARS-CoV-2 Spike RBD is a hotspot for adaptive mutations that enhance the binding efficiency of the virus to its human host (49), leading to the emergence of more virulent strains with greater infectivity and transmission (50).
The RBD of most CoVs, including SARS-CoV-2, resides in the C terminal domain, whereas the RBD of other viruses, such as mouse hepatitis virus (MHV), is located in the N terminal domain (11). The S protein of SARS-CoV-2 also shares a mere ~76% similarity with that of SARS-CoV in the amino acid sequence (30, 51). As compared with other CoV Spike proteins, the RBD region of SARS-CoV-2 has 26% more mutations (52). The uniqueness of the SARS-CoV-2 Spike protein compared with SARS-CoV also lies in the lower (~55%) identity in the S1 domain and ~ 91% identity in the S2 domain region (53, 54). The mutations, particularly in the S protein, have significant implications in the 3D structure. The SARS-CoV-2 S protein is composed of a relatively higher number of helices (n=4) and sheets (n=10) as compared with SARS-CoV (2 helices and 5 sheets) (52, 55). Furthermore, binding of the SARS-CoV-2 to the hACE2 is also more rigid with a higher number of H bonds in two different receptor-accessible states as compared with the SARS-CoV (51, 52). The uniqueness of the SARS-CoV-2 Spike protein is summarized in Table 1.
The entry of SARS-CoV-2 is mediated mainly through the viral S glycoprotein, S1 in particular, by binding to the host cell surface receptor hACE2 (31, 60). Cleavage of the S1/S2 and S2 subunits, also known as priming, by the host proteases, is a crucial factor that determines the pathogenicity, structural flexibility, and tropism (23, 61). The cleavage site at the S1/S2 boundary also harbors a polybasic furin cleavage site, which is a hallmark of highly pathogenic viruses, such as avian influenza (62). The interaction of SARS-CoV-2-S1 with hACE2 has a low dissociation constant (Kd), indicating a stronger interaction with hACE2 compared with SARS-CoV-S (22). Interestingly, SARS-CoV-2 infection in the intestine with life-threatening cases is comparatively lower than that of lungs although the hACE2 is vastly over-expressed in small intestine enterocytes compared with lung. This could be explained by the interaction of the highly abundant human defensin 5 (HD5) (a 32-residue amphiphilic antimicrobial peptide secreted by Paneth cells) with hACE2, which have a fairly strong affinity (39.3 nM) that blocks the binding of S1 protein to hACE2 (63).
The crystal structure of the SARS-CoV-2 Spike and hACE2 complex shows that a ∼24 amino acid helix of hACE2 is associated with the RBD-hACE2 interaction (22, 55, 64). The precise structural basis of RBD-hACE2 interactions could provide valuable information to develop Spike-based vaccines or Spike targeting drugs (65). For instance, a 23-mer synthetic amino acid (SBP1) derived from the hACE2 α1 helix was shown to have a Kd of 47 nM comparable to hACE2 and SARS-CoV-2-RBD binding with Kd 14.7 nM (6, 66), indicating that SBP1 could inhibit the entry of the virus into the host cell if high concentration of SBP1 is used to outcompete the S protein. Because SBP1 is endogenous and derived from humans, it would be recognized as endogenous by the immune system. We have also designed peptides that may potentially block the RBD-hACE2 (67); however, the new mutations in the RBD domain is a challenge that may affect the efficacy of these designed peptides.
Apart from hACE2, other human proteins can also act as receptors for SARS-CoV-2 RBD-mediated attachment and entry into human cells. It has been recently reported that entry of SARS CoV-2 into the host cells is also mediated through the transmembrane receptor Neuropilin-1 (NRP1) (68). Because NRP1 is expressed largely in the CNS, it has been proposed as a route for SARS-CoV-2 into the host brain, which might explain the neurologic manifestations of COVID-19 (69). Other receptors involved in the entry of SARS-CoV-2 are olfactory receptor OR51E2 (70) and heparan sulfate (HS) (71). In addition, tumor marker CD147 (basigin) had been initially reported as a Spike receptor (59), which was later found not to have a direct interaction with SARS-CoV-2 Spike (59). Another receptor, AGTR2 (angiotensin II receptor type 2) that is highly expressed in the lung, has also been proposed to be a key receptor in the lungs for the entry of SARS-CoV-2 with higher binding affinity than hACE2 (72). The Spike protein also interacts with GOLGA7 and ZDHHC5 acyl-transferase complex that promotes cytosolic tail palmitoylation, which could be a potential drug target (73).
Although there are several variants emerging from the original Wuhan strain, the following three lineages have been identified to be more infectious: lineage B.1.1.7 (WHO label: Alpha variant) identified in the UK, the B.1.351 (WHO label: Beta variant) in South Africa, and the P.1 (WHO label: Alpha variant) from Brazil (3). The three lineages have unique and common mutations on the RBD that interacts with the hACE2. The N501Y mutation emerged in all three lineages. The B.1.1.7 shows key mutations N501Y, P681H, and H69-V70del. For B.1.351 and the P.1 lineages, the key mutations are N501Y and K417T, and E484K. These gain-of-function mutations increase the binding interaction of Spike RBD with hACE2 and also escape from important antibody classes (3, 74). These immune escape variants could also cause reinfections and reduce vaccine efficacy (75). The increased affinity of the S mutant protein with hACE2 was also identified in the cluster-five variant, initially identified in the farmed minks in Denmark, which have an Y453F residue mutation in the RBD. These mutations increased the binding to hACE2 ~4 folds higher than the Wuhan strain but did not decrease the immune response to previously infected individuals, indicating that the variants strived for increased transmissibility rather than immune escape (76).
Until April 2021, several mutations in the Spike protein have been reported from different countries as described in Table 2. These adaptive mutations are making the virus more virulent with higher transmission rate, posing a question on the efficacy of the currently available Spike protein-based vaccines (78, 79). Besides, the precise mechanism of vaccine-induced thrombotic thrombocytopenia found in Spike-based ChAdOx1 nCoV-19 (AZD1222)/Oxford–AstraZeneca (80, 81) and Janssen COVID-19/Ad.26.COV2.S (82) COVID-19 vaccines are yet to be identified.
Among the main structural proteins of SARS-CoV-2, Spike (S) and nucleocapsid (N) are highly immunogenic. While the S protein is involved in the attachment of the viral particle to the host cells, N protein is involved in the viral RNA replication, packaging, and transcription (83). The gold standard method for detection of SARS-CoV-2 is whole-genome sequencing (WGS) from viral blood culture (3, 84). However, application of WGS is limited because of its cost and turnaround time. Therefore, amplification of the viral nucleic acid through reverse transcription polymerase chain reaction (RT-PCR) is the current standard for diagnosis of SARS-CoV-2, using ORF1ab and N gene of SARS-CoV-2 (84). Primer selection and RT-PCR reaction optimization are of key importance to avoid false-negatives, false-positives, and primer dimer formation (85). A recent study has shown that two primer sets (CDC_N2 and CDC_N3) from CDC produce false positives (Ct<37) even in the absence of the cDNA template. In another report, in-house designed SARS-CoV-2 RNA-dependent RNA polymerase (RdRP), N, E, and Sgenes specific primers to amplify 100 to 120 bp amplicons show no false-positive results (86).
Detection methods that rely on non-invasive sampling are preferred for large-scale screening. Amplification of the SARS-CoV-2 Spike gene from saliva samples has shown promising results (87); therefore, saliva is a good choice for sampling because salivary glands, gingiva, oral mucosa, as well as the tongue, could serve as hosts for SARS-CoV-2 due to the expression of hACE2 receptor (88, 89). Furthermore, a recent study also suggests that RT-PCR diagnosis for SARS-CoV-2 using saliva samples is more sensitive than the nasopharyngeal or nasal swabs (90). However, there could be increased false-negative results due to the novel SARS-CoV-2 variants for which the primers are not designed properly. Mutation in the Spike gene, such as 69‐70del that is amplified by RT-PCR has shown to affect specificity and sensitivity of the assay (91). Similar results may also be observed for other genetic variation in the RBD sites if the 132 publicly available primers and probes are used to amplify the Spike gene of SARS-CoV-2 (92). Mass screening and sero-surveillance require rapid testing kits rather than RT-PCR (93). Spike protein can be used for such screening. Point of care lateral flow immunoassay (LFIA) can detect IgM and IgG antibodies against SARS-CoV-2 S protein within 15 minutes from blood samples with 88.66% sensitivity and 90.63% specificity (93). IgM, the first line of defense, against a viral infection is generated earlier than the long-term adaptive IgG that serves as immunological memory (94). IgM antibody against SARS-CoV-2 infection can be detected between 5 and 10 days, while IgG is detected between 14-21 days (95). Because SARS-CoV-2 and SARS belong to the same family, IgM detection could indicate recent exposure, whereas IgG could indicate otherwise (96). Hence, IgM- and IgG-based detection could provide brief information on the infection timeline. ELISA-based diagnosis for detection of IgM and IgG antibodies against nucleocapsid protein and S protein from confirmed that COVID-19 patients have shown that Spike protein-based ELISA has a significantly higher rate of positive results (97). In another approach, graphene-based field-effect transistor (FET) biosensing-coated antibodies against SARS-CoV-2 S protein exhibited high sensitivity (1 fg/ml) to nasopharyngeal swab (98). FET based biosensing has several advantages, such as instantaneous measurements at low concentrations, which make it ultrasensitive and a promising candidate for onsite detections.
Recent advances in the time resolved fluorescence (TRF) ELISA using monoclonal antibodies against different S1 subunit epitopes showed high specificity (99%) but with lower sensitivity (66%) when nasopharyngeal swab samples are used (99). Rapid detection methods for SARS-CoV-2 S1 subunit have also been developed using hACE2 that forms a highly specific matched antibody pair with S1-mAb in a lateral flow immunoassay (LFIA) (100). The pros of this method include the non-cross reactivity with MERS or SARS CoV S1 subunit. Label-free electrochemical sensor that works on interruption of redox conversion in the presence of SARS-CoV-2 antibodies is also developed. It is a paper-based sensor with immobilized S RBD on the hydrophilic ePAD that selectively binds to the IgG and IgM produced against SARS-CoV-2 (101).
The structural, functional, and antigenic characteristics of the S protein make it a good candidate for development of vaccines (11, 28, 31, 35), antibodies (102–106), and drugs (107–109) against SARS-CoV-2 (Figure 5).
Figure 5 Summary of Spike protein targeted therapeutics. Sequence variability increases in the sense of S2 (gray) and S1 (blue) domains, and reaches its apices on the RBD (dark pink). Regions used for drugs, peptides, antibodies, and vaccine development are indicated with dark gray arrows.
Although sequence conservation of S protein makes it a good candidate for broad spectrum vaccine development against various CoVs, its mutations among various CoV strains pose a challenge. In many cases, Spike RBD of different CoVs, including MERS and SARS-CoV strains, shows a great antigenic capacity and induces neutralizing activity in infected animals and in vitro experiments (30, 40, 110–113). The conserved subsequence “KRSFIEDLLFNKV” found in many CoVs may be used as a peptide vaccine candidate. However, because the Spike RBD is a hotspot for mutation, there is always a risk of developing escape mutations in such conserved sequences (40). Another strategy for SARS-CoV vaccine development could be the use of full-length S protein to induce T-cells neutralizing antibodies (110, 114, 115), but, on the other hand, it has been reported that it may cause harmful immune responses causing liver injuries (110, 116).
The development of specific monoclonal antibodies (mAbs) against specific CoV strains is another way of controlling CoV infections (117). The use of Spike RBD region has been described for this purpose by several authors, where a single intrasplenic injection of plasmid DNA encoding the Spike region of interest is used for generating the desired immunity response (31, 118). For SARS-CoV-2, novel immunoglobulin (corresponding to RBD) against the SARS-CoV-2 could be screened from the peripheral blood of recovered patients using enzyme-linked immunosorbent assay (ELISA) and molecular cloning techniques to produce the desired mAbs for therapeutic purpose (106, 119). Moreover, the IGHV3-53 antibodies that is generated during SARS-CoV-2 infection targets RBD through alternated binding site and may be engineered for more effective neutralizing therapeutic antibody against SARS-CoV-2 infection (120). Drug development that involves S protein is mostly related to blocking its interaction with cleavage proteases and host cell surface receptors. Several in vitro, in vivo, and in silico (67, 121) studies with drug-like peptides (122) focusing on the RBD interaction with hACE2 (108) and other surface proteases (123–125), surface host-cell receptors, and furin S1/S2 cleavage site have been reported with promising potentials (124, 125). However, except for a few cases, most of these studies that aim to block the interactions of RBD and S1/S2 with their respective host-cell receptor or protease using small molecules are mainly in silico (53, 126). In Table 3, we have summarized the important in vitro studies from the Coronavirus Antiviral Research Database (CoV-RDB) targeting S protein of SARS-CoV-2 for development of therapeutics.
Most of the vaccines currently under development, clinical trials, or in use, aim to prevent the uptake of viral particles via hACE2 receptor and also induction of neutralizing antibodies (nAbs) (129). Therefore, use of SARS-Cov-2 S protein in vaccine development could serve two purposes: inhibition of receptor binding as well as viral genome uncoating (130). This is because the Spike subunit is comprised of S1 and S2 with distinct functions, where S1 mediates the hACE2 receptor binding and S2 mediates the fusion and uncoating of the viral genome into the host cells (110). Furthermore, S protein with or without the presence of other structural proteins is the major inducer of nAbs and T-cell responses (110, 131). Thus, S protein is one of the most promising candidates for development of vaccines against SARS-CoV-2 (130). Additionally, self-assembling protein nanoparticles that block the Spike RBD and stabilize the Spike are also reported to be a potential vaccine candidate (116).
The broad-spectrum vaccine against SARS-CoV and MERS-CoV has been proposed based on the similarities in T-cell epitopes that could provide cross-reactivity (132). However, the S1 subunits of S protein of SARS-CoV-2 and SARS-CoV are variable, suggesting that the vaccines against SARS-CoV would be probably ineffective against SARS-CoV-2. Nevertheless, considering the high genetic similarity among the SARS-CoV and SARS-CoV-2, broad-spectrum vaccines against coronavirus infections could be explored (30, 130). The development of a universal vaccine against the coronavirus is essential to avoid future outbreaks from novel coronavirus species and strains (133). Variegated T-cell in subjects unexposed to the SARS-CoV-2 was found to be reactive to SARS-CoV-2 peptides (134–136). Such cross reactivity could be due to previous exposures to Human CoVs (hCoVs) that cause common cold with mild respiratory symptoms (136). The cross-reactivity could be based on the hCoV groups. hCoVs are grouped into group I (eg, hCoV-229E) and group II (eg, hKU1, MERS-Cov, SARS-CoV-1 and -2) (137). Immune responses to a recent infection by any of the group II CoVs could provide cross-protection or milder/asymptomatic infection upon subsequent exposure to the other CoVs within the group II (137). Between SARS-CoV-2 and SARS-CoV, there are similarities in phylogeny, genome sequence (~80%), binding to hACE2 as receptor for entry, and also exhibit false-positives in serology assay (137–139). In contrast, other studies have found weak cross-neutralizing despite the common cross-reactive response (138). Moreover, it is reported that only a minor fraction of the epitopes recognized in cross-reactive responses might be neutralizing epitopes (138). Currently, there are a variety of vaccines that have been approved and used in different countries that use the Spike protein as well as mRNA technology (Table 4). Additionally, with the emergence of novel SARS-CoV-2 variants, vaccination studies are still under investigation for a broad-spectrum coronavirus vaccine with high efficacy (78, 150).
Table 4 Currently approved Spike protein (S) -based important COVID-19 vaccines and their efficacy on Spike mutant strains.
The world is facing the third wave of SARS-CoV-2 infection. Spike is the key protein for the infection and transmission of this virus and the dynamic adaptive mutations in the Spike protein are making the virus more aggressive. Although rapidly developed Spike-based vaccines and therapeutics have been successful to some extent, the prevention and treatment of the infection are still major challenges. Deeper insights into the SARS-CoV-2 genome and proteome, especially its Spike protein structure, its uniqueness, function, and interactions with the host cell and immune system is essential to come up with innovative approaches to win the war against SARS-CoV-2 through the development of new vaccines and therapeutics. More importantly, one needs to better understand how and why the virus is evolving so rapidly and acquiring more aggressive characteristics. In this article, we have focused only on the Spike of SARS-CoV-2; however, other proteins of this virus should also be thoroughly investigated to understand these basic questions and to develop proper strategies to tackle this pandemic.
The original contributions presented in the study are included in the article/Supplementary Material. Further inquiries can be directed to the corresponding author.
Conceptualization, methodology, and design of the article: DB. Draft preparation: MI, RK, AC, BA, FA, ST, DR, KA, and DB. Technical inputs: VA, AG-N, MW, and PG. Editing and finalizing the paper: PG and DB. All authors contributed to the article and approved the submitted version.
The authors declare that the research was conducted in the absence of any commercial or financial relationships that could be construed as a potential conflict of interest
The Supplementary Material for this article can be found online at: https://www.frontiersin.org/articles/10.3389/fimmu.2021.663912/full#supplementary-material
1. Drexler JF, Corman VM, Drosten C. Ecology, Evolution and Classification of Bat Coronaviruses in the Aftermath of SARS. Antiviral Res (2014) 101:45–56. doi: 10.1016/j.antiviral.2013.10.013
2. Wu F, Zhao S, Yu B, Chen Y-M, Wang W, Song Z-G, et al. A New Coronavirus Associated With Human Respiratory Disease in China. Nature (2020) 579(7798):265–9. doi: 10.1038/s41586-020-2008-3
3. Zhou P, Yang X-L, Wang X-G, Hu B, Zhang L, Zhang W, et al. A Pneumonia Outbreak Associated With a New Coronavirus of Probable Bat Origin. Nature (2020) 579(7798):270–3. doi: 10.1038/s41586-020-2012-7
4. Lu R, Zhao X, Li J, Niu P, Yang B, Wu H, et al. Genomic Characterisation and Epidemiology of 2019 Novel Coronavirus: Implications for Virus Origins and Receptor Binding. Lancet (2020) 395(10224):565–74. doi: 10.1016/s0140-6736(20)30251-8
5. Vellingiri B, Jayaramayya K, Iyer M, Narayanasamy A, Govindasamy V, Giridharan B, et al. COVID-19: A Promising Cure for the Global Panic. Sci Total Environ (2020) 725. doi: 10.1016/j.scitotenv.2020.138277
6. Wrapp D, Wang N, Corbett KS, Goldsmith JA, Hsieh C-L, Abiona O, et al. Cryo-EM Structure of the 2019-Ncov Spike in the Prefusion Conformation. Science (2020) 367(6483):1260–3. doi: 10.1126/science.abb2507
7. Li F. Structure, Function, and Evolution of Coronavirus Spike Proteins. Annu Rev Virol (2016) 3(1):237–61. doi: 10.1146/annurev-virology-110615-042301
8. Wu A, Peng Y, Huang B, Ding X, Wang X, Niu P, et al. Genome Composition and Divergence of the Novel Coronavirus (2019-Ncov) Originating in China. Cell Host Microbe (2020) 27(3):325–8. doi: 10.1016/j.chom.2020.02.001
9. Shang J, Wan Y, Luo C, Ye G, Geng Q, Auerbach A, et al. Cell Entry Mechanisms of SARS-CoV-2. Proc Natl Acad Sci (2020) 117(21):11727–34. doi: 10.1073/pnas.2003138117
10. Jaimes JA, André NM, Chappie JS, Millet JK, Whittaker GR. Phylogenetic Analysis and Structural Modeling of SARS-CoV-2 Spike Protein Reveals an Evolutionary Distinct and Proteolytically Sensitive Activation Loop. J Mol Biol (2020) 432(10):3309–25. doi: 10.1016/j.jmb.2020.04.009
11. Ou X, Liu Y, Lei X, Li P, Mi D, Ren L, et al. Characterization of Spike Glycoprotein of SARS-CoV-2 on Virus Entry and Its Immune Cross-Reactivity With SARS-CoV. Nat Commun (2020) 11(1). doi: 10.1038/s41467-020-15562-9
12. Li F, Goff SP. Receptor Recognition Mechanisms of Coronaviruses: A Decade of Structural Studies. J Virol (2015) 89(4):1954–64. doi: 10.1128/jvi.02615-14
13. Li W, Moore MJ, Vasilieva N, Sui J, Wong SK, Berne MA, et al. Angiotensin-Converting Enzyme 2 Is a Functional Receptor for the SARS Coronavirus. Nature (2003) 426(6965):450–4. doi: 10.1038/nature02145
14. Du L, Zhao G, Kou Z, Ma C, Sun S, Poon VKM, et al. Identification of a Receptor-Binding Domain in the S Protein of the Novel Human Coronavirus Middle East Respiratory Syndrome Coronavirus as an Essential Target for Vaccine Development. J Virol (2013) 87(17):9939–42. doi: 10.1128/jvi.01048-13
15. Mou H, Raj VS, van Kuppeveld FJM, Rottier PJM, Haagmans BL, Bosch BJ. The Receptor Binding Domain of the New Middle East Respiratory Syndrome Coronavirus Maps to a 231-Residue Region in the Spike Protein That Efficiently Elicits Neutralizing Antibodies. J Virol (2013) 87(16):9379–83. doi: 10.1128/jvi.01277-13
16. Raj VS, Mou H, Smits SL, Dekkers DHW, Müller MA, Dijkman R, et al. Dipeptidyl Peptidase 4 Is a Functional Receptor for the Emerging Human Coronavirus-EMC. Nature (2013) 495(7440):251–4. doi: 10.1038/nature12005
17. Jaimes JA, Millet JK, Stout AE, André NM, Whittaker GR. A Tale of Two Viruses: The Distinct Spike Glycoproteins of Feline Coronaviruses. Viruses (2020) 12(1). doi: 10.3390/v12010083
18. Lin H-X, Feng Y, Wong G, Wang L, Li B, Zhao X, et al. Identification of Residues in the Receptor-Binding Domain (RBD) of the Spike Protein of Human Coronavirus NL63 That Are Critical for the RBD–ACE2 Receptor Interaction. J Gen Virol (2008) 89(4):1015–24. doi: 10.1099/vir.0.83331-0
19. Peng G, Xu L, Lin Y-L, Chen L, Pasquarella JR, Holmes KV, et al. Crystal Structure of Bovine Coronavirus Spike Protein Lectin Domain. J Biol Chem (2012) 287(50):41931–8. doi: 10.1074/jbc.M112.418210
20. Promkuntod N, van Eijndhoven REW, de Vrieze G, Gröne A, Verheije MH. Mapping of the Receptor-Binding Domain and Amino Acids Critical for Attachment in the Spike Protein of Avian Coronavirus Infectious Bronchitis Virus. Virology (2014) 448:26–32. doi: 10.1016/j.virol.2013.09.018
21. Wang N, Rosen O, Wang L, Turner HL, Stevens LJ, Corbett KS, et al. Structural Definition of a Neutralization-Sensitive Epitope on the MERS-CoV S1-NTD. Cell Rep (2019) 28(13):3395–405.e6. doi: 10.1016/j.celrep.2019.08.052
22. Wang Q, Zhang Y, Wu L, Niu S, Song C, Zhang Z, et al. Structural and Functional Basis of SARS-CoV-2 Entry by Using Human Ace2. Cell (2020) 181(4):894–904. doi: 10.1016/j.cell.2020.03.045
23. Millet JK, Whittaker GR. Host Cell Proteases: Critical Determinants of Coronavirus Tropism and Pathogenesis. Virus Res (2015) 202:120–34. doi: 10.1016/j.virusres.2014.11.021
24. Coutard B, Valle C, de Lamballerie X, Canard B, Seidah NG, Decroly E. The Spike Glycoprotein of the New Coronavirus 2019-Ncov Contains a Furin-Like Cleavage Site Absent in CoV of the Same Clade. Antiviral Res (2020) 176. doi: 10.1016/j.antiviral.2020.104742
25. Follis KE, York J, Nunberg JH. Furin Cleavage of the SARS Coronavirus Spike Glycoprotein Enhances Cell–Cell Fusion But Does Not Affect Virion Entry. Virology (2006) 350(2):358–69. doi: 10.1016/j.virol.2006.02.003
26. Belouzard S, Chu VC, Whittaker GR. Activation of the SARS Coronavirus Spike Protein via Sequential Proteolytic Cleavage at Two Distinct Sites. Proc Natl Acad Sci (2009) 106(14):5871–6. doi: 10.1073/pnas.0809524106
27. Yan L, Meng B, Xiang J, Wilson IA, Yang B. Crystal Structure of the Post-Fusion Core of the Human Coronavirus 229E Spike Protein at 1.86 Å Resolution. Acta Crystallogr Section D Struct Biol (2018) 74(9):841–51. doi: 10.1107/s2059798318008318
28. Kumar S, Maurya VK, Prasad AK, Bhatt MLB, Saxena SK. Structural, Glycosylation and Antigenic Variation Between 2019 Novel Coronavirus (2019-Ncov) and SARS Coronavirus (SARS-CoV). VirusDisease (2020) 31(1):13–21. doi: 10.1007/s13337-020-00571-5
29. Mycroft-West C, Su D, Elli S, Li Y, Guimond S, Miller G, et al. The 2019 Coronavirus (SARS-CoV-2) Surface Protein (Spike) S1 Receptor Binding Domain Undergoes Conformational Change Upon Heparin Binding. bioRxiv (2020). doi: 10.1101/2020.02.29.971093
30. Sun C, Chen L, Yang J, Luo C, Zhang Y, Li J, et al. SARS-CoV-2 and SARS-CoV Spike-RBD Structure and Receptor Binding Comparison and Potential Implications on Neutralizing Antibody and Vaccine Development. bioRxiv (2020). doi: 10.1101/2020.02.16.951723
31. Walls AC, Park Y-J, Tortorici MA, Wall A, McGuire AT, Veesler D. Structure, Function, and Antigenicity of the SARS-CoV-2 Spike Glycoprotein. Cell (2020) 181(2):281–92.e6. doi: 10.1016/j.cell.2020.02.058
32. Hoffmann M, Kleine-Weber H. Pöhlmann S. A Multibasic Cleavage Site in the Spike Protein of SARS-CoV-2 Is Essential for Infection of Human Lung Cells. Mol Cell (2020) 78(4):779–84.e5. doi: 10.1016/j.molcel.2020.04.022
33. Andersen KG, Rambaut A, Lipkin WI, Holmes EC, Garry RF. The Proximal Origin of SARS-CoV-2. Nat Med (2020) 26(4):450–2. doi: 10.1038/s41591-020-0820-9
34. Li CK-F, Wu H, Yan H, Ma S, Wang L, Zhang M, et al. T Cell Responses to Whole SARS Coronavirus in Humans. J Immunol (2008) 181(8):5490–500. doi: 10.4049/jimmunol.181.8.5490
35. Prompetchara E, Ketloy C, Palaga T. Immune Responses in COVID-19 and Potential Vaccines: Lessons Learned From SARS and MERS Epidemic. Asian Pac J Allergy Immunol (2020) 38(1):1–9. doi: 10.12932/ap-200220-0772
36. Brielle ES, Schneidman-Duhovny D, Linial M. The SARS-CoV-2 Exerts a Distinctive Strategy for Interacting With the ACE2 Human Receptor. bioRxiv (2020). doi: 10.1101/2020.03.10.986398
37. Wec AZ, Wrapp D, Herbert AS, Maurer D, Haslwanter D, Sakharkar M, et al. Broad Sarbecovirus Neutralizing Antibodies Define a Key Site of Vulnerability on the SARS-CoV-2 Spike Protein. bioRxiv (2020). doi: 10.1101/2020.05.15.096511
38. Hoffmann M, Kleine-Weber H, Schroeder S, Krüger N, Herrler T, Erichsen S, et al. SARS-CoV-2 Cell Entry Depends on ACE2 and TMPRSS2 and Is Blocked by a Clinically Proven Protease Inhibitor. Cell (2020) 181(2):271–80.e8. doi: 10.1016/j.cell.2020.02.052
39. Chen W-H, Hotez PJ, Bottazzi ME. Potential for Developing a SARS-CoV Receptor-Binding Domain (RBD) Recombinant Protein as a Heterologous Human Vaccine Against Coronavirus Infectious Disease (COVID)-19. Hum Vaccines Immunother (2020) 16(6):1239–42. doi: 10.1080/21645515.2020.1740560
40. Robson B. COVID-19 Coronavirus Spike Protein Analysis for Synthetic Vaccines, a Peptidomimetic Antagonist, and Therapeutic Drugs, and Analysis of a Proposed Achilles’ Heel Conserved Region to Minimize Probability of Escape Mutations and Drug Resistance. Comput Biol Med (2020) 121:103749. doi: 10.1016/j.compbiomed.2020.103749
41. Uddin MB, Hasan M, Harun-Al-Rashid A, Ahsan MI, Imran MAS, Ahmed SSU. Ancestral Origin, Antigenic Resemblance and Epidemiological Insights of Novel Coronavirus (SARS-CoV-2): Global Burden and Bangladesh Perspective. Infect Genet Evol (2020) 84:104440. doi: 10.1016/j.meegid.2020.104440
42. Wahba L, Jain N, Fire AZ, Shoura MJ, Artiles KL, McCoy MJ, et al. Identification of a Pangolin Niche for a 2019-Ncov-Like Coronavirus Through an Extensive Meta-Metagenomic Search. mSphere (2020) 5(3):e00160–20. doi: 10.1101/2020.02.08.939660
43. Wong MC, Javornik Cregeen SJ, Ajami NJ, Petrosino JF. Evidence of Recombination in Coronaviruses Implicating Pangolin Origins of Ncov-2019. bioRxiv (2020). doi: 10.1101/2020.02.07.939207
44. Xiao K, Zhai J, Feng Y, Zhou N, Zhang X, Zou J-J, et al. Isolation of SARS-CoV-2-Related Coronavirus From Malayan Pangolins. Nature (2020) 583(7815):286–9. doi: 10.1038/s41586-020-2313-x
45. Zhang C, Zheng W, Huang X, Bell EW, Zhou X, Zhang Y. Protein Structure and Sequence Reanalysis of 2019-Ncov Genome Refutes Snakes as Its Intermediate Host and the Unique Similarity Between Its Spike Protein Insertions and HIV-1. J Proteome Res (2020) 19(4):1351–60. doi: 10.1021/acs.jproteome.0c00129
46. Guo H, Hu B-J, Yang X-L, Zeng L-P, Li B, Ouyang S, et al. Evolutionary Arms Race Between Virus and Host Drives Genetic Diversity in Bat Severe Acute Respiratory Syndrome-Related Coronavirus Spike Genes. J Virol (2020) 94(20):e00902–20. doi: 10.1128/jvi.00902-20
47. Jackwood MW, Boynton TO, Hilt DA, McKinley ET, Kissinger JC, Paterson AH, et al. Emergence of a Group 3 Coronavirus Through Recombination. Virology (2010) 398(1):98–108. doi: 10.1016/j.virol.2009.11.044
48. Perlman S, Liu P, Jiang J-Z, Wan X-F, Hua Y, Li L, et al. Are Pangolins the Intermediate Host of the 2019 Novel Coronavirus (SARS-CoV-2)? PloS Pathog (2020) 16(5):e1008421. doi: 10.1371/journal.ppat.1008421
49. Ou J, Zhou Z, Dai R, Zhao S, Wu X, Zhang J, et al. Emergence of SARS-CoV-2 Spike RBD Mutants That Enhance Viral Infectivity Through Increased Human ACE2 Receptor Binding Affinity. bioRxiv (2020). doi: 10.1101/2020.03.15.991844
50. Chen J, Wang R, Wang M, Wei G-W. Mutations Strengthened SARS-CoV-2 Infectivity. J Mol Biol (2020) 432(19):5212–26. doi: 10.1016/j.jmb.2020.07.009
51. Peng C, Zhu Z, Shi Y, Wang X, Mu K, Yang Y, et al. Exploring the Binding Mechanism and Accessible Angle of SARS-CoV-2 Spike and ACE2 by Molecular Dynamics Simulation and Free Energy Calculation. chemrxiv (2020). doi: 10.26434/chemrxiv.11877492.v1
52. Veeramachaneni GK, Thunuguntla VBSC, Bobbillapati J, Bondili JS. Structural and Simulation Analysis of Hotspot Residues Interactions of SARS-CoV 2 With Human ACE2 Receptor. J Biomolec Struct Dynam (2020) 39:4015–25. doi: 10.1080/07391102.2020.1773318
53. Vankadari N, Wilce JA. Emerging COVID-19 Coronavirus: Glycan Shield and Structure Prediction of Spike Glycoprotein and Its Interaction With Human CD26. Emerg Microbes Infect (2020) 9(1):601–4. doi: 10.1080/22221751.2020.1739565
54. Vankadari N. Arbidol: A Potential Antiviral Drug for the Treatment of SARS-CoV-2 by Blocking Trimerization of the Spike Glycoprotein. Int J Antimicrob Agents (2020) 56(2):105998. doi: 10.1016/j.ijantimicag.2020.105998
55. Lan J, Ge J, Yu J, Shan S, Zhou H, Fan S, et al. Structure of the SARS-CoV-2 Spike Receptor-Binding Domain Bound to the ACE2 Receptor. Nature (2020) 581(7807):215–20. doi: 10.1038/s41586-020-2180-5
56. Xia S, Lan Q, Su S, Wang X, Xu W, Liu Z, et al. The Role of Furin Cleavage Site in SARS-CoV-2 Spike Protein-Mediated Membrane Fusion in the Presence or Absence of Trypsin. Signal Transduct Target Ther (2020) 5(1):92. doi: 10.1038/s41392-020-0184-0
57. Ali A, Vijayan R. Dynamics of the ACE2–SARS-CoV-2/SARS-CoV Spike Protein Interface Reveal Unique Mechanisms. Sci Rep (2020) 10(1):14214. doi: 10.1038/s41598-020-71188-3
58. Schuster O, Zvi A, Rosen O, Achdout H, Ben-Shmuel A, Shifman O, et al. Specific and Rapid SARS-CoV-2 Identification Based on LC-MS/MS Analysis. ACS Omega (2021) 6(5):3525–34. doi: 10.1021/acsomega.0c04691
59. Wang K, Chen W, Zhang Z, Deng Y, Lian JQ, Du P, et al. CD147-Spike Protein Is a Novel Route for SARS-CoV-2 Infection to Host Cells. Signal Transduct Target Ther (2020) 5(1):283. doi: 10.1038/s41392-020-00426-x
60. Cava C, Bertoli G, Castiglioni I. In Silico Discovery of Candidate Drugs Against Covid-19. Viruses (2020) 12(4):404. doi: 10.3390/v12040404
61. Hoffmann M, Hofmann-Winkler H, Pöhlmann S. Priming Time: How Cellular Proteases Arm Coronavirus Spike Proteins. In: Activation of Viruses by Host Proteases (2018). p. 71–98. doi: 10.1007/978-3-319-75474-1_4
62. Steinhauer DA. Role of Hemagglutinin Cleavage for the Pathogenicity of Influenza. Virus Virol (1999) 258(1):1–20. doi: 10.1006/viro.1999.9716
63. Wang C, Wang S, Li D, Zhao X, Han S, Wang T, et al. Lectin-Like Intestinal Defensin Inhibits 2019-Ncov Spike Binding to ACE2. bioRxiv (2020). doi: 10.1101/2020.03.29.013490
64. Shang J, Ye G, Shi K, Wan Y, Luo C, Aihara H, et al. Structural Basis of Receptor Recognition by SARS-CoV-2. Nature (2020) 581(7807):221–4. doi: 10.1038/s41586-020-2179-y
65. Whisenant J, Burgess K. Blocking Coronavirus 19 Infection via the SARS-CoV-2 Spike Protein: Initial Steps. ACS Med Chem Lett (2020) 11(6):1076–8. doi: 10.1021/acsmedchemlett.0c00233
66. Zhang G, Pomplun S, Loftis AR, Tan X, Loas A, Pentelute BL. The First-in-Class Peptide Binder to the SARS-CoV-2 Spike Protein. bioRxiv (2020). doi: 10.1101/2020.03.19.999318
67. Barh D, Tiwari S, Silva Andrade B, Giovanetti M, Almeida Costa E, Kumavath R, et al. Potential Chimeric Peptides to Block the SARS-CoV-2 Spike Receptor-Binding Domain. F1000Research (2020) 9:576. doi: 10.12688/f1000research.24074.1
68. Cantuti-Castelvetri L, Ojha R, Pedro LD, Djannatian M, Franz J, Kuivanen S, et al. Neuropilin-1 Facilitates SARS-CoV-2 Cell Entry and Infectivity. Science (2020) 370(6518):856–60. doi: 10.1126/science.abd2985
69. Davies J, Randeva HS, Chatha K, Hall M, Spandidos DA, Karteris E, et al. Neuropilin1 as a New Potential SARSCoV2 Infection Mediator Implicated in the Neurologic Features and Central Nervous System Involvement of COVID19. Mol Med Rep (2020) 22(5):4221–6. doi: 10.3892/mmr.2020.11510
70. Kerslake R, Hall M, Randeva HS, Spandidos DA, Chatha K, Kyrou I, et al. Coexpression of Peripheral Olfactory Receptors With SARSCoV2 Infection Mediators: Potential Implications Beyond Loss of Smell as a COVID19 Symptom. Int J Mol Med (2020) 46(3):949–56. doi: 10.3892/ijmm.2020.4646
71. Zamorano Cuervo N, Grandvaux N. ACE2: Evidence of Role as Entry Receptor for SARS-CoV-2 and Implications in Comorbidities. Elife (2020) 9:e61390. doi: 10.7554/eLife.61390
72. Cui C, Huang C, Zhou W, Ji X, Zhang F, Wang L, et al. AGTR2, One Possible Novel Key Gene for the Entry of SARS-CoV-2 Into Human Cells. IEEE/ACM Trans Comput Biol Bioinform (2020). doi: 10.1109/TCBB.2020.3009099
73. Gordon DE, Jang GM, Bouhaddou M, Xu J, Obernier K, O’Meara MJ, et al. A SARS-CoV-2-Human Protein-Protein Interaction Map Reveals Drug Targets and Potential Drug-Repurposing. bioRxiv (2020). doi: 10.1101/2020.03.22.002386
74. Ningombam SS, Kumar R, Tanwar P. Mutant Strains of SARS-CoV-2 Are More Prone to Infect Obese Patient: A Review. Wien Klin Wochenschr (2021) 133(7-8):383–92. doi: 10.1007/s00508-021-01819-w
75. Rani PR, Imran M, Lakshmi JV, Jolly B, Jain A, Surekha A, et al. Symptomatic Reinfection of SARS-CoV-2 With Spike Protein Variant N440K Associated With Immune Escape. J Med Virol (2021) 93(7):4163–5. doi: 10.1002/jmv.26997
76. Bayarri-Olmos R, Rosbjerg A, Johnsen LB, Helgstrand C, Bak-Thomsen T, Garred P, et al. The SARS-CoV-2 Y453F Mink Variant Displays a Pronounced Increase in ACE-2 Affinity But Does Not Challenge Antibody Neutralization. J Biol Chem (2021) 296:100536. doi: 10.1016/j.jbc.2021.100536
77. Rambaut A, Holmes EC, O’Toole A, Hill V, McCrone JT, Ruis C, et al. A Dynamic Nomenclature Proposal for SARS-CoV-2 Lineages to Assist Genomic Epidemiology. Nat Microbiol (2020) 5(11):1403–7. doi: 10.1038/s41564-020-0770-5
78. Gomez CE, Perdiguero B, Esteban M. Emerging SARS-CoV-2 Variants and Impact in Global Vaccination Programs Against SARS-CoV-2/COVID-19. Vaccines (Basel) (2021) 9(3):243. doi: 10.3390/vaccines9030243
79. Hossain MK, Hassanzadeganroudsari M, Apostolopoulos V. The Emergence of New Strains of SARS-CoV-2. What Does It Mean for COVID-19 Vaccines? Expert Rev Vaccines (2021), 1–4. doi: 10.1080/14760584.2021.1915140
80. Greinacher A, Thiele T, Warkentin TE, Weisser K, Kyrle PA, Eichinger S. Thrombotic Thrombocytopenia After ChAdOx1 Ncov-19 Vaccination. N Engl J Med (2021) 384:2092–101. doi: 10.1056/NEJMoa2104840
81. Ostergaard SD, Schmidt M, Horvath-Puho E, Thomsen RW, Sorensen HT. Thromboembolism and the Oxford-AstraZeneca COVID-19 Vaccine: Side-Effect or Coincidence? Lancet (2021) 397(10283):1441–3. doi: 10.1016/S0140-6736(21)00762-5
82. MacNeil JR, Su JR, Broder KR, Guh AY, Gargano JW, Wallace M, et al. Updated Recommendations From the Advisory Committee on Immunization Practices for Use of the Janssen (Johnson & Johnson) COVID-19 Vaccine After Reports of Thrombosis With Thrombocytopenia Syndrome Among Vaccine Recipients - United States, April 2021. MMWR Morb Mortal Wkly Rep (2021) 70(17):651–6. doi: 10.15585/mmwr.mm7017e4
83. McBride R, van Zyl M, Fielding B. The Coronavirus Nucleocapsid Is a Multifunctional. Protein Viruses (2014) 6(8):2991–3018. doi: 10.3390/v6082991
84. Li X, Geng M, Peng Y, Meng L, Lu S. Molecular Immune Pathogenesis and Diagnosis of COVID-19. J Pharm Anal (2020) 10(2):102–8. doi: 10.1016/j.jpha.2020.03.001
85. Jaeger LH, Nascimento TC, Rocha FD, Vilela FMP, Duque A, Silva LM, et al. Adjusting RT-qPCR Conditions to Avoid Unspecific Amplification in SARS-CoV-2 Diagnosis. Int J Infect Dis (2021) 102:437–9. doi: 10.1016/j.ijid.2020.10.079
86. Won J, Lee S, Park M, Kim TY, Park MG, Choi BY, et al. Development of a Laboratory-Safe and Low-Cost Detection Protocol for SARS-CoV-2 of the Coronavirus Disease 2019 (COVID-19). Exp Neurobiol (2020) 29(2):107–19. doi: 10.5607/en20009
87. To KK-W, Tsang OT-Y, Yip CC-Y, Chan K-H, Wu T-C, Chan JM-C, et al. Consistent Detection of 2019 Novel Coronavirus in Saliva. Clin Infect Dis (2020) 71(15):841–3. doi: 10.1093/cid/ciaa149
88. Xu H, Zhong L, Deng J, Peng J, Dan H, Zeng X, et al. High Expression of ACE2 Receptor of 2019-Ncov on the Epithelial Cells of Oral Mucosa. Int J Oral Sci (2020) 12(1):8. doi: 10.1038/s41368-020-0074-x
89. Xu R, Cui B, Duan X, Zhang P, Zhou X, Yuan Q. Saliva: Potential Diagnostic Value and Transmission of 2019-Ncov. Int J Oral Sci (2020) 12(1):11. doi: 10.1038/s41368-020-0080-z
90. Teo AKJ, Choudhury Y, Tan IB, Cher CY, Chew SH, Wan ZY, et al. Saliva Is More Sensitive Than Nasopharyngeal or Nasal Swabs for Diagnosis of Asymptomatic and Mild COVID-19 Infection. Sci Rep (2021) 11(1):3134. doi: 10.1038/s41598-021-82787-z
91. Ramirez JD, Munoz M, Patino LH, Ballesteros N, Paniz-Mondolfi A. Will the Emergent SARS-CoV2 B.1.1.7 Lineage Affect Molecular Diagnosis of COVID-19? J Med Virol (2021) 93(5):2566–8. doi: 10.1002/jmv.26823
92. Jain A, Rophina M, Mahajan S, Krishnan BB, Sharma M, Mandal S, et al. Analysis of the Potential Impact of Genomic Variants in Global SARS-CoV-2 Genomes on Molecular Diagnostic Assays. Int J Infect Dis (2021) 102:460–2. doi: 10.1016/j.ijid.2020.10.086
93. Li Z, Yi Y, Luo X, Xiong N, Liu Y, Li S, et al. Development and Clinical Application of a Rapid IgM-IgG Combined Antibody Test for SARS-CoV-2 Infection Diagnosis. J Med Virol (2020) 92(9):1518–24. doi: 10.1002/jmv.25727
94. Racine R, Winslow GM. IgM in Microbial Infections: Taken for Granted? Immunol Lett (2009) 125(2):79–85. doi: 10.1016/j.imlet.2009.06.003
95. Harrington WE, Trakhimets O, Andrade DV, Dambrauskas N, Raappana A, Jiang Y, et al. Rapid Decline of Neutralizing Antibodies Is Associated With Decay of IgM in Adults Recovered From Mild COVID-19. Cell Rep Med (2021) 2(4):100253. doi: 10.1016/j.xcrm.2021.100253
96. Al-Jighefee HT, Yassine HM, Nasrallah GK. Evaluation of Antibody Response in Symptomatic and Asymptomatic COVID-19 Patients and Diagnostic Assessment of New IgM/IgG ELISA Kits. Pathogens (2021) 10(2):161. doi: 10.3390/pathogens10020161
97. Liu W, Liu L, Kou G, Zheng Y, Ding Y, Ni W, et al. Evaluation of Nucleocapsid and Spike Protein-Based Enzyme-Linked Immunosorbent Assays for Detecting Antibodies Against SARS-CoV-2. J Clin Microbiol (2020) 58(6):e00461–20. doi: 10.1128/jcm.00461-20
98. Seo G, Lee G, Kim MJ, Baek S-H, Choi M, Ku KB, et al. Rapid Detection of COVID-19 Causative Virus (SARS-CoV-2) in Human Nasopharyngeal Swab Specimens Using Field-Effect Transistor-Based Biosensor. ACS Nano (2020) 14(4):5135–42. doi: 10.1021/acsnano.0c02823
99. Barlev-Gross M, Weiss S, Ben-Shmuel A, Sittner A, Eden K, Mazuz N, et al. Spike vs Nucleocapsid SARS-CoV-2 Antigen Detection: Application in Nasopharyngeal Swab Specimens. Anal Bioanal Chem (2021) 413(13):3501–10. doi: 10.1007/s00216-021-03298-4
100. Lee JH, Choi M, Jung Y, Lee SK, Lee CS, Kim J, et al. A Novel Rapid Detection for SARS-CoV-2 Spike 1 Antigens Using Human Angiotensin Converting Enzyme 2 (ACE2). Biosens Bioelectron (2021) 171:112715. doi: 10.1016/j.bios.2020.112715
101. Yakoh A, Pimpitak U, Rengpipat S, Hirankarn N, Chailapakul O, Chaiyo S. Paper-Based Electrochemical Biosensor for Diagnosing COVID-19: Detection of SARS-CoV-2 Antibodies and Antigen. Biosens Bioelectron (2021) 176:112912. doi: 10.1016/j.bios.2020.112912
102. Rockx B, Corti D, Donaldson E, Sheahan T, Stadler K, Lanzavecchia A, et al. Structural Basis for Potent Cross-Neutralizing Human Monoclonal Antibody Protection Against Lethal Human and Zoonotic Severe Acute Respiratory Syndrome Coronavirus Challenge. J Virol (2008) 82(7):3220–35. doi: 10.1128/jvi.02377-07
103. Coughlin MM, Babcook J, Prabhakar BS. Human Monoclonal Antibodies to SARS-Coronavirus Inhibit Infection by Different Mechanisms. Virology (2009) 394(1):39–46. doi: 10.1016/j.virol.2009.07.028
104. Ohnuma K, Haagmans BL, Hatano R, Raj VS, Mou H, Iwata S, et al. Inhibition of Middle East Respiratory Syndrome Coronavirus Infection by Anti-CD26 Monoclonal Antibody. J Virol (2013) 87(24):13892–9. doi: 10.1128/jvi.02448-13
105. Lei C, Qian K, Li T, Zhang S, Fu W, Ding M, et al. Neutralization of SARS-CoV-2 Spike Pseudotyped Virus by Recombinant ACE2-Ig. Nat Commun (2020) 11(1):2070. doi: 10.1038/s41467-020-16048-4
106. Chen X, Li R, Pan Z, Qian C, Yang Y, You R, et al. Human Monoclonal Antibodies Block the Binding of SARS-CoV-2 Spike Protein to Angiotensin Converting Enzyme 2 Receptor. Cell Mol Immunol (2020) 17(6):647–9. doi: 10.1038/s41423-020-0426-7
107. Millet JK, Séron K, Labitt RN, Danneels A, Palmer KE, Whittaker GR, et al. Middle East Respiratory Syndrome Coronavirus Infection Is Inhibited by Griffithsin. Antiviral Res (2016) 133:1–8. doi: 10.1016/j.antiviral.2016.07.011
108. Amin M, Abbas G. Docking Study of Chloroquine and Hydroxychloroquine Interaction With RNA Binding Domain of Nucleocapsid Phospho-Protein – An in Silico Insight Into the Comparative Efficacy of Repurposing Antiviral Drugs. J Biomolec Struct Dynam (2020) 2020:1–13. doi: 10.1080/07391102.2020.1775703
109. Wei T, Wang H, Wu X, Lu Y, Guan S, Dong F, et al. In Silico Screening of Potential Spike Glycoprotein Inhibitors of SARS-CoV-2 With Drug Repurposing Strategy. Res Sq (2020). doi: 10.21203/rs.3.rs-17720/v1
110. Du L, He Y, Zhou Y, Liu S, Zheng B-J, Jiang S. The Spike Protein of SARS-CoV — A Target for Vaccine and Therapeutic Development. Nat Rev Microbiol (2009) 7(3):226–36. doi: 10.1038/nrmicro2090
111. Zhang N, Jiang S, Du L. Current Advancements and Potential Strategies in the Development of MERS-CoV Vaccines. Expert Rev Vaccines (2014) 13(6):761–74. doi: 10.1586/14760584.2014.912134
112. Yuan M, Wu NC, Zhu X, Lee C-CD, So RTY, Lv H, et al. A Highly Conserved Cryptic Epitope in the Receptor Binding Domains of SARS-CoV-2 and SARS-CoV. Science (2020) 368(6491):630–3. doi: 10.1126/science.abb7269
113. Robson B. Computers and Viral Diseases. Preliminary Bioinformatics Studies on the Design of a Synthetic Vaccine and a Preventative Peptidomimetic Antagonist Against the SARS-CoV-2 (2019-Ncov, COVID-19) Coronavirus. Comput Biol Med (2020)119:103670. doi: 10.1016/j.compbiomed.2020.103670
114. Bisht H, Roberts A, Vogel L, Bukreyev A, Collins PL, Murphy BR, et al. Severe Acute Respiratory Syndrome Coronavirus Spike Protein Expressed by Attenuated Vaccinia Virus Protectively Immunizes Mice. Proc Natl Acad Sci (2004) 101(17):6641–6. doi: 10.1073/pnas.0401939101
115. Yang Z-Y, Kong W-P, Huang Y, Roberts A, Murphy BR, Subbarao K, et al. A DNA Vaccine Induces SARS Coronavirus Neutralization and Protective Immunity in Mice. Nature (2004) 428(6982):561–4. doi: 10.1038/nature02463
116. He Y, Li J, Li W, Lustigman S, Farzan M, Jiang S. Cross-Neutralization of Human and Palm Civet Severe Acute Respiratory Syndrome Coronaviruses by Antibodies Targeting the Receptor-Binding Domain of Spike Protein. J Immunol (2006) 176(10):6085–92. doi: 10.4049/jimmunol.176.10.6085
117. Yu XF, Liang LH, She M, Liao XL, Gu J, Li YH, et al. Production of a Monoclonal Antibody Against SARS-CoV Spike Protein With Single Intrasplenic Immunization of Plasmid DNA. Immunol Lett (2005) 100(2):177–81. doi: 10.1016/j.imlet.2005.03.015
118. Spitz M, Spitz L, Thorpe R, Eugui E. Intrasplenic Primary Immunization for the Production of Monoclonal Antibodies. J Immunol Methods (1984) 70(1):39–43. doi: 10.1016/0022-1759(84)90387-9
119. Liu J, Shao H, Tao Y, Yang B, Qian L, Yang X, et al. Production of an Anti-Severe Acute Respiratory Syndrome (SARS) Coronavirus Human Monoclonal Antibody Fab Fragment by Using a Combinatorial Immunoglobulin Gene Library Derived From Patients Who Recovered From SARS. Clin Vaccine Immunol (2006) 13(5):594–7. doi: 10.1128/cvi.13.5.594-597.2006
120. Wu NC, Yuan M, Liu H, Lee CD, Zhu X, Bangaru S, et al. An Alternative Binding Mode of IGHV3-53 Antibodies to the SARS-CoV-2 Receptor Binding Domain. Cell Rep (2020) 33(3):108274. doi: 10.1016/j.celrep.2020.108274
121. Ling R, Dai Y, Huang B, Huang W, Yu J, Lu X, et al. In Silico Design of Antiviral Peptides Targeting the Spike Protein of SARS-CoV-2. Peptides (2020) 130:170328. doi: 10.1016/j.peptides.2020.170328
122. Lu L, Liu Q, Zhu Y, Chan K-H, Qin L, Li Y, et al. Structure-Based Discovery of Middle East Respiratory Syndrome Coronavirus Fusion Inhibitor. Nat Commun (2014) 5:3067. doi: 10.1038/ncomms4067
123. Qian Z, Dominguez SR, Holmes KV. Role of the Spike Glycoprotein of Human Middle East Respiratory Syndrome Coronavirus (MERS-CoV) in Virus Entry and Syncytia Formation. PloS One (2013) 8(10):e76469. doi: 10.1371/journal.pone.0076469
124. Millet JK, Whittaker GR. Host Cell Entry of Middle East Respiratory Syndrome Coronavirus After Two-Step, Furin-Mediated Activation of the Spike Protein. Proc Natl Acad Sci (2014) 111(42):15214–9. doi: 10.1073/pnas.1407087111
125. Du L, Yang Y, Zhou Y, Lu L, Li F, Jiang S. MERS-CoV Spike Protein: A Key Target for Antivirals. Expert Opin Ther Targets (2016) 21(2):131–43. doi: 10.1080/14728222.2017.1271415
126. Silva Andrade B, Ghosh P, Barh D, Tiwari S, José Santana Silva R, Rodrigues de Assis Soares W, et al. Computational Screening for Potential Drug Candidates Against the SARS-CoV-2 Main Protease. F1000Research (2020) 9:514. doi: 10.12688/f1000research.23829.1
127. Han Y, Yang L, Duan X, Duan F, Nilsson-Payant BE, Yaron TM, et al. Identification of Candidate COVID-19 Therapeutics Using hPSC-Derived Lung Organoids. bioRxiv (2020). doi: 10.1101/2020.05.05.079095
128. Xiong H-L, Cao J-L, Shen C-G, Ma J, Qiao X-Y, Shi T-S, et al. Several FDA-Approved Drugs Effectively Inhibit SARS-CoV-2 Infection In Vitro. bioRxiv (2020). doi: 10.1101/2020.06.05.135996
129. Thanh Le T, Andreadakis Z, Kumar A, Gómez Román R, Tollefsen S, Saville M, et al. The COVID-19 Vaccine Development Landscape. Nat Rev Drug Discov (2020) 19(5):305–6. doi: 10.1038/d41573-020-00073-5
130. Dhama K, Sharun K, Tiwari R, Dadar M, Malik YS, Singh KP, et al. COVID-19, an Emerging Coronavirus Infection: Advances and Prospects in Designing and Developing Vaccines, Immunotherapeutics, and Therapeutics. Hum Vaccines Immunother (2020) 16(6):1232–8. doi: 10.1080/21645515.2020.1735227
131. Myung Hee Kim HJK. Jun Chang. Superior Immune Responses Induced by Intranasal Immunization With Recombinant Adenovirus-Based Vaccine Expressing Full-Length Spike Protein of Middle East Respiratory Syndrome Coronavirus. PloS One (2019) 14(7):e0220196. doi: 10.1371/journal.pone.0220196
132. Liu WJ, Zhao M, Liu K, Xu K, Wong G, Tan W, et al. T-Cell Immunity of SARS-CoV: Implications for Vaccine Development Against MERS-CoV. Antiviral Res (2017) 137:82–92. doi: 10.1016/j.antiviral.2016.11.006
133. Giurgea LT, Han A, Memoli MJ. Universal Coronavirus Vaccines: The Time to Start Is Now. NPJ Vaccines (2020) 5(1):43. doi: 10.1038/s41541-020-0198-1
134. Grifoni A, Weiskopf D, Ramirez SI, Mateus J, Dan JM, Moderbacher CR, et al. Targets of T Cell Responses to SARS-CoV-2 Coronavirus in Humans With COVID-19 Disease and Unexposed Individuals. Cell (2020) 181(7):1489–501.e15. doi: 10.1016/j.cell.2020.05.015
135. Le Bert N, Tan AT, Kunasegaran K, Tham CYL, Hafezi M, Chia A, et al. SARS-CoV-2-Specific T Cell Immunity in Cases of COVID-19 and SARS, and Uninfected Controls. Nature (2020) 584(7821):457–62. doi: 10.1038/s41586-020-2550-z
136. Mateus J, Grifoni A, Tarke A, Sidney J, Ramirez SI, Dan JM, et al. Selective and Cross-Reactive SARS-CoV-2 T Cell Epitopes in Unexposed Humans. Science (2020) 370(6512):89–94. doi: 10.1126/science.abd3871
137. Cohen SA, Kellogg C, Equils O. Neutralizing and Cross-Reacting Antibodies: Implications for Immunotherapy and SARS-CoV-2 Vaccine Development. Hum Vaccines Immunother 2021 17(1):84–87. doi: 10.1080/21645515.2020.1787074
138. Lv H, Wu NC, Tsang OT-Y, Yuan M, Perera RAPM, Leung WS, et al. Cross-Reactive Antibody Response Between SARS-CoV-2 and SARS-CoV Infections. Cell Rep (2020) 31(9):107725. doi: 10.1016/j.celrep.2020.107725
139. Wan WY, Lim SH, Seng EH. Cross-Reaction of Sera From COVID-19 Patients With SARS-CoV Assays. medRxiv (2020). doi: 10.1101/2020.03.17.20034454
140. Chagla Z. In High-Risk Adults, the Moderna Vaccine had 94% Efficacy Against COVID-19 >/=14 D After the 2nd Dose. Ann Intern Med (2021) 174(3):JC28. doi: 10.7326/ACPJ202103160-028
141. Abu-Raddad LJ, Chemaitelly H, Butt AA, National Study Group for C-V. Effectiveness of the BNT162b2 Covid-19 Vaccine Against the B.1.1.7 and B.1.351 Variants. N Engl J Med (2021) NEJMc2104974. doi: 10.1056/NEJMc2104974
142. Livingston EH, Malani PN, Creech CB. The Johnson & Johnson Vaccine for COVID-19. JAMA (2021) 325(15):1575. doi: 10.1001/jama.2021.2927
143. Sadoff J, Le Gars M, Shukarev G, Heerwegh D, Truyers C, de Groot AM, et al. Interim Results of a Phase 1–2a Trial of Ad26.COV2.S Covid-19 Vaccine. New Engl J Med (2021) 384(19):1824–35. doi: 10.1056/NEJMoa2034201
144. Baden LR, El Sahly HM, Essink B, Kotloff K, Frey S, Novak R, et al. Efficacy and Safety of the mRNA-1273 SARS-CoV-2 Vaccine. N Engl J Med (2021) 384(5):403–16. doi: 10.1056/NEJMoa2035389
145. Knoll MD, Wonodi C. Oxford-AstraZeneca COVID-19 Vaccine Efficacy. Lancet (2021) 397(10269):72–4. doi: 10.1016/S0140-6736(20)32623-4
146. Jones I, Roy P. Sputnik V COVID-19 Vaccine Candidate Appears Safe and Effective. Lancet (2021) 397(10275):642–3. doi: 10.1016/S0140-6736(21)00191-4
147. Baraniuk C. Covid-19: What Do We Know About Sputnik V and Other Russian Vaccines? BMJ (2021) 372:n743. doi: 10.1136/bmj.n743
148. Kumar A, Dowling WE, Roman RG, Chaudhari A, Gurry C, Le TT, et al. Status Report on COVID-19 Vaccines Development. Curr Infect Dis Rep (2021) 23(6):9. doi: 10.1007/s11908-021-00752-3
149. Kumar S, Tao Q, Weaver S, Sanderford M, Caraballo-Ortiz MA, Sharma S, et al. An Evolutionary Portrait of the Progenitor SARS-CoV-2 and Its Dominant Offshoots in COVID-19 Pandemic. Mol Biol Evol (2021) msab118. doi: 10.1093/molbev/msab118
Keywords: SARS-CoV-2, COVID-19, spike protein, mutations, diagnostics, drugs, vaccines
Citation: Kumavath R, Barh D, Andrade BS, Imchen M, Aburjaile FF, Ch A, Rodrigues DLN, Tiwari S, Alzahrani KJ, Góes-Neto A, Weener ME, Ghosh P and Azevedo V (2021) The Spike of SARS-CoV-2: Uniqueness and Applications. Front. Immunol. 12:663912. doi: 10.3389/fimmu.2021.663912
Received: 04 February 2021; Accepted: 16 June 2021;
Published: 08 July 2021.
Edited by:
Aurelio Cafaro, National Institute of Health (ISS), ItalyReviewed by:
Eleonora Cella, University of Central Florida, United StatesCopyright © 2021 Kumavath, Barh, Andrade, Imchen, Aburjaile, Ch, Rodrigues, Tiwari, Alzahrani, Góes-Neto, Weener, Ghosh and Azevedo. This is an open-access article distributed under the terms of the Creative Commons Attribution License (CC BY). The use, distribution or reproduction in other forums is permitted, provided the original author(s) and the copyright owner(s) are credited and that the original publication in this journal is cited, in accordance with accepted academic practice. No use, distribution or reproduction is permitted which does not comply with these terms.
*Correspondence: Debmalya Barh, ZHIuYmFyaEBnbWFpbC5jb20=
†These authors have contributed equally to this work
Disclaimer: All claims expressed in this article are solely those of the authors and do not necessarily represent those of their affiliated organizations, or those of the publisher, the editors and the reviewers. Any product that may be evaluated in this article or claim that may be made by its manufacturer is not guaranteed or endorsed by the publisher.
Research integrity at Frontiers
Learn more about the work of our research integrity team to safeguard the quality of each article we publish.