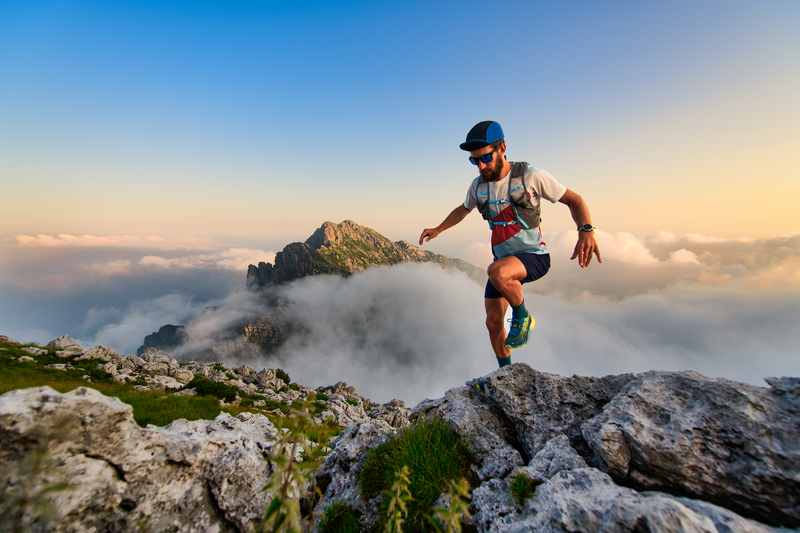
95% of researchers rate our articles as excellent or good
Learn more about the work of our research integrity team to safeguard the quality of each article we publish.
Find out more
REVIEW article
Front. Immunol. , 17 June 2021
Sec. Microbial Immunology
Volume 12 - 2021 | https://doi.org/10.3389/fimmu.2021.663328
This article is part of the Research Topic Humanized Mouse Models to Study Immune Responses to Human Infectious Organisms View all 11 articles
Periodontitis is an oral inflammatory disease in which the polymicrobial synergy and dysbiosis of the subgingival microbiota trigger a deregulated host immune response, that leads to the breakdown of tooth-supporting tissues and finally tooth loss. Periodontitis is characterized by the increased pathogenic activity of T helper type 17 (Th17) lymphocytes and defective immunoregulation mediated by phenotypically unstable T regulatory (Treg), lymphocytes, incapable of resolving the bone-resorbing inflammatory milieu. In this context, the complexity of the immune response orchestrated against the microbial challenge during periodontitis has made the study of its pathogenesis and therapy difficult and limited. Indeed, the ethical limitations that accompany human studies can lead to an insufficient etiopathogenic understanding of the disease and consequently, biased treatment decision-making. Alternatively, animal models allow us to manage these difficulties and give us the opportunity to partially emulate the etiopathogenesis of periodontitis by inoculating periodontopathogenic bacteria or by placing bacteria-accumulating ligatures around the teeth; however, these models still have limited translational application in humans. Accordingly, humanized animal models are able to emulate human-like complex networks of immune responses by engrafting human cells or tissues into specific strains of immunodeficient mice. Their characteristics enable a viable time window for the study of the establishment of a specific human immune response pattern in an in vivo setting and could be exploited for a wider study of the etiopathogenesis and/or treatment of periodontitis. For instance, the antigen-specific response of human dendritic cells against the periodontopathogen Porphyromonas gingivalis favoring the Th17/Treg response has already been tested in humanized mice models. Hypothetically, the proper emulation of periodontal dysbiosis in a humanized animal could give insights into the subtle molecular characteristics of a human-like local and systemic immune response during periodontitis and support the design of novel immunotherapeutic strategies. Therefore, the aims of this review are: To elucidate how the microbiota-elicited immunopathogenesis of periodontitis can be potentially emulated in humanized mouse models, to highlight their advantages and limitations in comparison with the already available experimental periodontitis non-humanized animal models, and to discuss the potential translational application of using these models for periodontitis immunotherapeutics.
The oral mucosa is a place of immense antigenic diversity that demands a tightly balanced immune surveillance, capable of maintaining the balance between host and microbial interactions. Indeed, a plethora of signals, including food antigens, airborne particles, commensal microbiota, and ongoing damage from mastication, finely tune the oral mucosal barrier immunity (1, 2). Moreover, the existence of an incredibly thin and highly-permeable epithelium located at the bottom of the gingival crevice, composed of 3-5 layers of cells and harboring diverse microbial communities between the tooth and the gingiva, allows the continuous transmigration of immune cells against the microbial challenge, thus making this scenario particularly challenging for a balanced immune response (1, 3).
Certainly, constant environmental stimuli given by oral anatomical features and their functions, as well as the salivary flow and composition, directly influence the tooth-adherent microbiota. Hence, the orchestrated stability of at least 700 different taxa colonizing these distinct oral meta-niches has been a permanent matter of attention for dentists (4). In a healthy periodontium, the symbiosis between the resident eubiotic microorganisms and the host’s immune response commands this equilibrium. However, ill-defined factors, such as plaque accumulation, diet, stress, smoking, genetic predisposition, chronic inflammation, among others, contribute to a disequilibrium within bacterial communities, favoring the emergence of highly virulent bacteria, including Porphyromonas gingivalis, Aggregatibacter actinomycetemcomitans, and Fusobacterium nucleatum, as well as the reduction of health-compatible commensal bacteria.
For instance, P. gingivalis pathogenicity relies on its capability of expressing a variety of virulence factors, such as lipopolysaccharide, extracellular capsule, gingipains, and fimbriae, which, despite its low relative abundance, can both over-activate and subvert the immune response in mice or human periodontium (5, 6). Furthermore, different strains of this keystone pathogen have been detected in active periodontal lesions of teeth with poor prognosis and have demonstrated the capacity of eliciting a differential osteo-destructive immune response (7–9). Otherwise, the pathobiont A. actinomycetemcomitans, associated with more severe forms of periodontitis, is also armed with a variety of virulence factors such as lipopolysaccharide, leukotoxin, and fimbriae, which induce leukocyte lysis, favor periodontal colonization and provoke a dysregulated immune response during periodontal inflammation (10–12). In this context, the polymicrobial synergy of a dysbiotic bacterial consortium, frequently including the interplay between P. gingivalis, A. actinomycetemcomitans, and/or F. nucleatum, leads to a cycle of pathogenic inflammation, which perpetuates a nutrient-rich environment that promotes their expansion and deeper invasion of periodontal tissues; thus, provoking inflammatory alveolar bone loss in a susceptible host (13, 14).
Apart from other mucosal barriers entirely dependent on microbial commensals, gingival immune homeostasis is distinctly influenced by physiological damage during mastication. In fact, chewing elicits the production of interleukin (IL)-6 by fibroblasts, which promotes oral barrier protection via T-helper type 17 (Th17)-mediated immunity (15). Indeed, Th17 lymphocytes are key players in the maintenance of oral integrity by actively recruiting neutrophils to the teeth/mucosa interface and controlling opportunistic fungal infections (16); however, they are also key drivers of osteolytic inflammation during periodontitis. Thus, the amplification and dysregulation of Th17 lymphocyte activity mediated by Th17-related cytokines, including IL-6, IL-17A, IL-21, IL-23, and the osteolytic factor termed receptor activator of nuclear factor κB ligand (RANKL), lead to the breakdown of soft tooth-supporting tissues and alveolar bone resorption in susceptible individuals (16–18).
Contrarily, the maintenance of tolerance, prevention of autoimmunity, and inhibition of chronic inflammation required in the healthy periodontium has been attributed to T regulatory (Treg) lymphocyte activity (19–21). Treg lymphocytes are mainly characterized by their sustained surface expression of the IL-2 receptor α chain, termed CD25, and their signature transcription factor forkhead box P3 (Foxp3), and by having an armament of molecular strategies for, as its name implies, regulate both the innate and adaptive immune response (22). Treg lymphocytes control, at least in part, periodontal inflammation via the production of immunoregulatory cytokines, such as transforming growth factor (TGF)-β1, IL-10, and IL-35, which inhibit the expansion and activity of effector T lymphocytes such as Th1 and Th17 cells (21, 23). Periodontitis-affected tissues show enrichment in Treg lymphocyte activity demonstrated by the increased expression of their associated immune-regulatory/suppressive molecules like cytotoxic T-lymphocyte antigen 4 (CTLA-4), glucocorticoid-induced TNF-related protein (GITR) and Foxp3 (24). On the other hand, systemic ablation of Treg lymphocytes, by inoculating anti-GITR, increases the periodontal levels of tumor necrosis factor (TNF)-α, RANKL, and alveolar bone loss (25). However, for these immune-regulatory properties to be effective, the maintenance of their regulatory phenotype, mediated by the expression of Foxp3, is mandatory (20, 26). During periodontitis, Treg lymphocytes show a reduced Foxp3 expression and, instead, produce IL-17A and RANKL (20, 27); thus, making the inflammatory milieu highly enriched in inflammatory cytokines like IL-6, pivotal in dictating Treg lymphocyte fate in periodontal lesions.
The antagonistic relationship between bone-resorptive Th17 lymphocytes and immunoregulatory Treg lymphocytes dictates the delicate balance of alveolar bone remodeling (17, 20). On the one hand, osteoclasts differentiate and activate in the presence of macrophage colony-stimulating factor (M-CSF) and RANKL, which increase during periodontitis mainly by Th17 activity; while on the other hand, osteoblasts produce osteoprotegerin (OPG), the RANKL soluble decoy, which is partly mediated by Treg lymphocyte activity during periodontitis (17, 23, 27–31). Therefore, the cooperative action between bone-resorbing osteoclasts and bone-forming osteoblasts, regulated by the immune response, defines the dynamic maintenance of alveolar bone homeostasis.
The complexity and diversity of factors that contribute to periodontitis pathogenesis, including the combination of polymicrobial synergy and dysbiosis, chronic inflammatory dysregulation, and genetic predisposition factors have done the search for an ideal animal model of periodontitis difficult and challenging. Nevertheless, several authors have managed to mimic how these factors influence the development of periodontal diseases by separating them into different phases, including the formation of biofilms, bacterial colonization and invasion of periodontal tissues, induction of a deregulated host immune response, and breakdown of soft tooth-supporting tissues and alveolar bone resorption (32). Table 1 summarizes the different methods that have been used to generate experimental periodontitis in mice. In general terms, the advantages of using mice models include a known microbiota and immune composition, diverse genetically engineered strains, and rapid availability of reagents for the investigation of recently described molecules (59). However, conditions such as substantially small mouths require highly skilled operators and a large number of animals (60).
Experimental mice models developed for the study of periodontitis have been substantially beneficial and important to examine diverse biologic hypotheses related to disease pathogenesis, host-bacteria interactions, and therapeutic approaches, being able to, at least in part, finely reproduce the clinical, molecular, and histologic features of human periodontitis. Besides, the animals used to generate periodontitis are relatively inexpensive, easy to handle, have a short gestation period, and are characterized by developing a highly reproducible periodontal inflammatory process (33, 61). Among the various methods employed to mimic periodontitis in mice, currently, the most commonly used are oral gavage, periodontal inoculation, and ligature.
The oral gavage model consists of the inoculation of human bacterial strains with an oral-esophageal gauge or a micropipette, usually using 109 colony-forming units in a viscous suspension, prepared with 2% carboxymethylcellulose (62). The bacteria that have been most frequently used to induce periodontitis by oral gavage are P. gingivalis, A. actinomycetemcomitans, and F. nucleatum, as well as combinations of different bacterial strains (63–66). This method has been used successfully to establish the relationship between periodontitis and systemic conditions. For instance, the inoculation of P. gingivalis in hyperlipidemic mice via oral gavage provoked the accelerated formation of atherosclerotic plaques (67).
However, this experimental design can last at least 4 and up to 8 weeks until clear and significant evidence of alveolar bone resorption is achieved. Moreover, the magnitude of bone loss is not always reproducible, and the frequent inconsistency of the results is attributable to various factors, being the systemic nature of the infection caused the main factor (33, 34, 61). In consequence, oral gavage has been established as not fully effective to induce periodontitis (34).
The periodontal inoculation model comprises the localized microinjection of bacteria or some isolated bacterial component, such as lipopolysaccharide, directly into the palatal interproximal gingiva between the first, second, and third maxillary molar (18, 35). This method promotes significant periodontal inflammation, characterized by an increased expression of pro-inflammatory cytokines, apical migration of the junctional epithelium, and activation of osteoclastogenesis, consistently resulting in alveolar bone resorption (36, 40). Regarding the injection regimen, evidence commonly shows that the injections are performed two or three times per week under general anesthesia, generally using isoflurane (61, 68). The experimental period for this methodology may vary according to the purpose of the study, being generally between 20 and 30 days, although significant evidence of alveolar bone loss can be verified 7 days after initiation of the injections (18, 69).
This model has been used to evaluate different hypotheses regarding the mechanisms of periodontal inflammation and alveolar bone loss, due to the fact that it allows a reliable characterization of the immune response induced in the periodontal tissues and the cervical lymph nodes that drain the infected periodontium (6, 40). Since the mono-infection with a known bacterium allows great experimental control over the pathogenic stimulus, this model has been shown to be useful for analyzing pathogenic differences between different periodontal bacteria, their different serotypes, or bacteria defective in a certain virulence factor (6, 7, 18, 40, 41).
Ligature-induced periodontitis is an efficient model capable of inducing predictable alveolar bone loss within few days in mice (70). Besides, the removal of ligatures allows the study of lesion healing and resolution of inflammation (53). The main procedure involves placing silk, nylon, or cotton ligatures around maxillary or mandibular molars under anesthesia so that the retentive ligatures facilitate the accumulation of bacteria and thus, provoke periodontal inflammation and alveolar bone loss (53, 61, 71). Indeed, the occurrence of these bacteria-host interactions is compatible with the current paradigm of periodontitis pathogenesis, in which the development of dysbiotic oral microbiota provokes a bone-destructive immune response (28, 72).
The use of ligatures to induce periodontitis in mice has permitted the kinetic analysis of the morphologic characteristics of bone resorption (53). Differences between control and ligated mice alveolar bone loss become evident after just 5 days and gradually increase their differences at day 10 and 15 (20). Apart from that, the characterization of bacterial accumulation in ligatures has revealed that mice oral commensal communities go through extensive structural and composition changes, leading to microbial dysbiosis (28). Meanwhile, differential immune responses can be appreciated at subsequent time points; for instance, myeloperoxidase-producing cells, such as neutrophils, can be seen since day 3, and genes related to the critical adaptive immune response in periodontitis have expression peaks in periodontal lesions after 9 days, including markers for Th1, Th2, Th17, and Treg lymphocyte activity (32).
The ligature-induced periodontitis model has been extensively reported in mice (20, 23, 29, 32–35, 53, 54, 60); however, the extremely small mice oral cavity and the minuscule interproximal space between the maxillary or mandibular molars represent a not minor technical difficulty in placing the ligatures (73). Moreover, this placement can get even harder when dealing with immunodeficient mice when working in sterile conditions; thus, reinforcing the idea that experienced and highly skilled operators are needed. In response to this, simpler ligature models have been proposed, including the use of a 0.2-mm orthodontic ligature wire, after filing the molar interproximal surfaces with a curved C+ nickel-titanium root canal file, and the use of already tied ligature knots and 3D-printed devices (32, 74). These preformed ligatures would allow their atraumatic positioning in any molar interdental space, accumulating plaque and provoking a local immune response followed by bone resorption, in a similar way to its predecessors (32).
Even though the above-described animal models have proven to be particularly useful to study the molecular mechanisms associated with the onset, progression, and recovery from periodontitis, different challenges also arise when we try to translate these results to human contexts. Despite the similarities between mice and human immune systems, including molecular mediators and cell subpopulations that interact during both innate and adaptive immune responses (59), there are still discrepancies that must be considered. Indeed, substantial molecular differences between murine and human growth factors and cytokines influence the course of their hematopoietic and immune system development (75). Among the most relevant differences, gingival microbiota, key molecular mediators, including immunoglobulins, cytokines, chemokines, co-stimulatory molecules, Toll-like receptors, and T-cell signaling pathways should be considered when a translational projection of results obtained in animal models of periodontitis is desired (28, 75, 76). For instance, human effector CD4+ T lymphocytes express human leukocyte antigen (HLA) molecules and regulate Foxp3 expression without necessarily acquiring an immunoregulatory phenotype, while mouse Foxp3+ T lymphocytes are mostly defined as regulatory (75, 76); thus, displaying variations that could be important in the study of periodontitis. Furthermore, the ethical and technical constraints that severely limit in vivo studies of human biology, constant evolution of human-specific diseases, resistance to microbial infections, and pharmacodynamic interactions have led to increasing demand for translationally enhanced animal models. In this context, the limitations that hinder the direct replication of outcomes predicted by murine studies into human diseases support the requirement for the generation of humanized mouse models for the study of periodontitis (77).
Humanized mice models, or mouse/human chimeras, have been defined as immuno-deficient mice engrafted with human primary hematopoietic cells and/or tissues capable of reconstituting a functional human immune system (HIS) (75); thus, providing an opportunity for the study of live human biological processes and affections. There are three main humanized mice models: the human peripheral blood lymphocytes (hu-PBL) model, the human stem cells (hu-HSC) model, and the human bone marrow/fetal liver/thymus (BLT) model (Figure 1), each with its distinctive advantages and limitations (Table 2).
Figure 1 Mice humanization strategies. Mice/human chimeras originate from immunodeficient mice engrafted with different sources of human cells or tissues able to reconstitute a human-like immune response, such as (A) hu-PBL: PBMCs are obtained from healthy donors and i.v. or i.p. inoculated, (B) hu-HSCs: HSCs may be obtained from bone marrow, umbilical cord blood, peripheral blood, or fetal liver, and i.v. inoculated into either adult or newborn mice, and (C) hu-BLT: Bone marrow stem cells and tissues from fetal liver and thymus are transplanted into previously irradiated mice, specifically under the renal capsule. hu-BLT, human bone marrow, liver, and thymus; hu-HSCs, human hematopoietic stem cells; hu-PBL, human peripheral blood lymphocytes; i.p., intraperitoneal; i.v., intravenous; PBMCs, peripheral blood mononuclear cells. Created with BioRender.com.
The hu-PBL model consists of the inoculation and engraftment of human leukocytes isolated from peripheral blood, also termed peripheral blood mononuclear cells (PBMCs), via intravenous (i.v.), intraperitoneal (i.p.), intrafemoral (i.f.), intracardiac (i.c.), or intrahepatic (i.h.) injection. This is the easiest and most cost-efficient method of animal humanization due to the large quantities of human leukocytes that can be isolated from peripheral blood. Also, this model has fast engraftment kinetics, as human leukocytes can be found circulating in murine peripheral blood within days and up to 4 to 6 weeks (75, 76). Human T lymphocytes with an activated effector and memory phenotype are the main population present in this model, whereas B lymphocytes and myeloid cells are present but at much lower quantities, probably due to the dominant expansion of T lymphocytes and the lack of human cytokines required for their survival (76, 85, 86).
Technically, mice may be preconditioned with a sublethal dose of irradiation, which has been reported to facilitate engraftment and colonization of human PBMCs. Nonetheless, this step is not completely necessary for all mice platforms because the PBMC inoculum contains already mature human leukocytes that do not need to undergo differentiation in the mouse environment. Moreover, irradiation accelerates the occurrence of xenogeneic graft-versus-host-disease (GvHD), which results in reduced animal survival (87). In this context, rapid onset of GvHD is the main disadvantage of the hu-PBL model, generated by the elevated levels of activated human T lymphocytes due to MHC I and II mismatch. However, new strains of mice deficient in MHC I and/or II delay GvHD development and increase animal survival, thus widening the available experimental window (88).
In the hu-HSC model, human hematopoietic stem cells (HSC) are injected and engrafted into either adult or newborn immunodeficient mice (89, 90). The CD34+ HSCs may be obtained from bone marrow, cord blood, fetal liver, or mobilized human HSCs and are injected i.v. or i.f. into adult mice or i.v. (facial vein), i.c., or i.h. into newborn mice (91, 92). Additionally, for this model to achieve effective levels of HSC engraftment, myelosuppression preconditioning with sublethal irradiation is necessary to deplete mouse HSCs. However, new mouse strains with mutations in c-Kit, a stem cell factor (SCF) receptor critical for HSC engraftment, or with transgenic expression of membrane-bound human SCF allow the successful engraftment of human HSCs without previous irradiation (93, 94). After preconditioning and inoculation, a diverse repertoire of cell populations differentiates into multiple lineages of human cells and engrafts the murine tissues. Those cells include erythrocytes, platelets, T lymphocytes, natural killer (NK) cells, dendritic cells, monocytes/macrophages, and granulocytes (89, 90, 95–97). Nevertheless, this model presents some important disadvantages, such as the lack of a functional B lymphocyte compartment, in part due to inadequate CD4+ T lymphocyte function and impaired antigen response. This is associated with the lack of HLA on the thymic epithelium and the absence of human primary lymphoid organs, and consequently, the limited differentiation of human cells inside the model.
The hu-BLT model consists of the surgical transplantation of human fetal liver and thymus fragments under the kidney capsule of sublethally irradiated immunocompromised mice, followed by an i.v. injection of autologous HSCs (98). This model has been described as superior to the others, as it promotes an enhanced reconstitution of secondary lymphoid organs, which contributes to HIS education and allows the systemic repopulation of multiple human immune cell lineages, including T and B lymphocytes, monocytes, macrophages, and dendritic cells. This model has been an important tool to study human T lymphocyte development, as these cells are educated in autologous thymic tissues (99, 100). Nevertheless, the hu-BLT model presents higher GvHD incidence compared with the hu-SRC model, sometimes earlier than 20 weeks after transplantation (101).
Besides the humanization strategy, successful engraftment largely relies on the features of the animal recipient host. In this context, the development of immunodeficient mice hosts, capable of engrafting human cells or tissues, has implied a progressive succession of genetic modifications in order to avoid xenogeneic graft rejection and enable a stable reconstitution of human cells (76, 102). In brief, one of the first groundbreaking achievements was the Prkdcscid (protein kinase, DNA activated, catalytic polypeptide) mutation on the CB17 mouse strain, commonly named as SCID (severe combined immunodeficiency) (103). This mutation results in a reduced number of functional T and B lymphocytes, enabling transient engraftment of human PBMCs, HSCs, or fetal hematopoietic tissues (92, 104). Nevertheless, with aging, these mice generate autologous T and B lymphocytes, an event termed leakiness. Moreover, this animal host still presents high levels of NK cells and other innate immune cells that hinder proper human cell engraftment (76, 102).
Another approach implied the targeted mutation of the recombination-activating genes 1 and 2 (Rag1 and Rag2), which impedes the development of functional T and B lymphocytes in mice and prevents leakiness (105, 106). However, Rag1/2-deficient mice maintain a high NK cell activity, allowing limited engraftment of HSCs (76). Afterward, NOD-SCID mice were developed by crossing NOD (for non-obese diabetic) mice with the SCID strains (107). This animal host presents additional defects in innate immunity, such as the lack of complement C5, and impaired macrophage cytokine production, antigen presentation, and NK function (76, 107, 108). Even though this approach provides enhanced human HSC and PBMC engraftments (109, 110), the model also has a limited life span due to the early development of lymphomas and innate immunity residual activity mice strain (76).
Fortunately, another immunodeficient mouse strain with a targeted mutation of the Il2rg gene, which encodes IL-2 receptor γ-chain (IL-2Rγ), has been developed (89, 90, 95, 111, 112) (Figure 2). IL-2Rγ is an essential component for IL-2, IL-4, IL-7, IL-9, IL-15, and IL-21 signaling and its absence in Il2rgnull mice leads to defective lymph nodes, deficient T and B lymphocyte development, affects innate immunity, and completely abolishes NK cell generation (113, 114). Besides, this mutation has promoted enhanced support of both human HSC and PBMC engraftments as compared with the previously described immunodeficient mice (76). After an extensive succession of mutations, the main immunodeficient mouse strains currently used are NOD-SCID Il2rgnull mice, which includes NOD.Cg-PrkdcscidIl2rgtm1Wjl (NSG mice), NODShi.Cg-PrkdcscidIl2rgtm1Sug (NOG mice), NOD-Rag1null Il2rgnull (NRG), and BALB/c-Rag2nullIl2rgnull or Rag2tm1FlvIl2rgtm1Flv (BRG mice). All these mice strains are able to support human tissue and cell engraftment (86, 90, 95). The characteristics, advantages, and disadvantages of each immunodeficient mouse model derived from Il2rgnull mice have been extensively reviewed in detail by Shultz et al. (75, 76).
Figure 2 Immunodeficient mice strains prone to humanization. Several genetic modifications have enabled that immunodeficient host mice be capable of engrafting human cells or tissues without immediate xenogeneic rejection and allow a stable reconstitution of human cells. These immunodeficient mice include NSG mice, NOG mice, and BRG mice, each one with its own advantages and disadvantages summarized in the Figure. il2rg, interleukin-2 receptor subunit gamma; MHC, major histocompatibility complex; NOD, non-obese diabetic. Created with BioRender.com.
Altogether, these animal models comprise an important opportunity for the study of multiple biological processes and diseases, including periodontitis. Importantly, the selection of a model or another depends on the scientific question and experimental settings; thus, it is highly recommended to consider that the levels of functional HIS engraftment differs depending on the recipient mouse strain and humanization strategy used.
Notwithstanding the accumulated successes achieved along with the development of new and more refined immunodeficient murine platforms, the development of a vigorous functional HIS following engraftment, necessary for transferable humanized models, has remained challenging. This is due to species specificity of MHC antigens, homing molecules that may impede appropriate trafficking of human immune cells, discrepancies between hematopoietic growth factors and cytokines, poor development of lymphoid architecture, and impaired class switching and affinity maturation of immunoglobulins (115).
In this context, mice humanization protocols that involve the transference of human cells into severely immunodeficient mice strains may initiate an alloreactive response in which T lymphocytes react against HLA disparities and minor antigens, resulting in the initiation of GvHD. This potentially life-threatening complication consists in the acute anti-host effector response of human T lymphocytes recognizing foreign murine MHC expressed by host recipient cells (88). While the rapid development of this disease enables preclinical testing of human immunosuppressive agents, the relatively short survival of engrafted animals given by GvHD could prevent the realization of long-term in vivo studies and the proper emulation of chronic diseases (101, 115).
In other words, as mature CD4+ T lymphocytes have been educated in the human thymic stroma, they are not tolerized to the murine antigenic environment, which leads to the rapid-onset of xenogeneic GvHD. In fact, during engraftment of human cells, GvHD onset and severity vary between donors, which seem to depend on the number of CD4+ T lymphocytes within the transferred human cells (116). Another important influencing factor is the mice strain, from which NSG mice provide a faster expansion of the human CD45+ compartment and higher engraftment levels of CD3+ T lymphocytes; however, they also have a faster rate of GvHD than, for example, BRG mice (117). Also, HSC-reconstituted mice might not be able to recognize antigens presented by HLA-DR human dendritic cells in the periphery because they are specific for murine MHC class II molecules. This negatively affects the induction of an efficient immune response, resulting in reduced Th lymphocyte activity and insufficient interactions between T and B lymphocytes, which are required for class-switch recombination (116). Thus, substantial considerations should be taken into account when selecting the ideal model to assess the immunopathogenesis and the efficacy of novel immunotherapies for periodontitis.
Despite these limitations, a significant improvement has been accomplished by the transgenic introduction of human HLA molecules into immunodeficient mice strains. For example, transgenic expression of HLA-DR4 in NRG mice has enabled the proper development of CD4+ T lymphocytes and completely functional B lymphocytes from infused HSCs of HLA-DR-matched donors (118). In addition, the complimentary removal of murine MHC class II molecules, the main target of human CD4+ T lymphocyte-mediated GvHD responses, further improved the generation of human antigen-specific immune responses in immunodeficient mice reconstituted with human cells while reducing the risk of xenogeneic GvHD development (119). Therefore, the diversity of humanized model systems represents an important set of tools for modeling pathogen interactions with human cells and tissues in vivo (120), so the consecutive overcome of their punctual limitations enlightens their potential application for the study of immunopathologies characterized by T lymphocyte-mediated aberrant responses, such as periodontitis.
Following the transplantation of human cells, an extensive engraftment characterization is often required. Indeed, clinical features of the recipient host, donor cell source, and engraftment technique have a great influence on the kinetics, extent, composition, and morphological aspects of the graft reconstitution in the different organs and, consequently, its consistency. For instance, NSG mice exhibit small, poorly developed primary and secondary lymphoid organs, which lack typical lymphoid structures and are solely composed of reticular stromal cells (75, 121). Consequently, grafted human cells, which form variably sized aggregates, are not capable of recreating the typical lymphoid tissue architecture of immunocompetent organisms (75, 121, 122). Therefore, extensive immune profiling across innate and adaptive immune cell subpopulations, including human T lymphocytes (CD3+, CD4+, and CD8+ cells), B lymphocytes (CD19+ cells), macrophages (CD68+ cells), and neutrophils (CD15+ cells), among others, should be characterized before studying the development periodontitis or any proposed therapeutic approach (123, 124).
To analyze human cell engraftment in immunosuppressed mice, flow cytometry is a cost-effective method for the characterization of cell populations through the identification of lineage-specific markers using fluorochrome-coupled antibodies (Figure 3A). Indeed, flow cytometry allows the analysis of isolated cells from different tissues or organs by quantifying the percentages and the absolute number of murine and human immune cells on each sample, starting with CD45+ cytometric gating strategy for immune cells subpopulations. Nowadays, flow cytometry technology has advanced to the capacity of measuring up to 50 parameters on a single cell; however, most flow cytometers are limited to 12-18 parameters per sample, and spectral overlap makes its analysis complex. Apart from that, immunohistochemistry analysis contributes with the tissue-specific spatial context to better understand engrafted human cell homing and distribution in mice tissues (Figure 3B), additionally helping to identify the GvHD inflammatory lesions dominated by T lymphocytes and macrophages (125–127).
Figure 3 Humanized mice model characterization. The diversity of graft sources and receptors for humanization often needs an extensive immune cell subpopulations’ profiling in order to discriminate the desired human-like immune response from the graft-versus-host-disease (GvHD) xenogeneic immune response. For this purpose, flow cytometry and immunohistochemistry have been used. (A) Flow cytometry: The use of multiple fluorochrome-labeled antibodies allows the identification of different immune cell lineages at the same time. The CD45+ peripheral blood mononuclear cells (PBMCs) can be further classified, for example, into CD3+CD4+/CD8+ T cells, CD19+ B cells, CD16+ natural killer cells, or CD68+ macrophages, which are characteristic of a human-like periodontal immune response. (B) Immunohistochemistry: Tissue labeling allows the spatial identification of human cells in mice tissues, the visualization of their interaction with periodontal tissue-specific morphology, and the identification of GvHD lesions. Created with BioRender.com.
Despite the important information that flow cytometry and immunohistochemistry provide for the characterization of humanized mice, new high-dimensional parameter analysis tools have been introduced to accurately describe and understand the complexity of these models (128). For instance, mass cytometry offers a single-cell analysis that couples flow cytometry with mass spectrometry and is able to evaluate up to 50 simultaneous parameters without spectral overlap using markers coupled with metal isotopes (129, 130). Another novel tool is imaging mass cytometry, which also uses metal-tagged antibodies, enhancing the imaging of up to 37 protein markers on fresh-frozen or formalin-fixed paraffin-embedded tissue sections (131). These and other complemental high throughput platforms could allow the performance of a multidimensional data analysis that could allow the identification of subtle changes in periodontal immune populations (132).
In the periodontal disease research arena, the use of humanized mice models has been increasingly gaining interest for their potential translational applicability. The first proposed model demonstrated that the engraftment of immunodeficient mice with hu-PBLs provided a conceivable animal model to study human immune responses towards periodontitis-associated antigens/pathogens (78–83).
Indeed, to characterize the human leukocyte response against the periodontopathogen A. actinomycetemcomitans, formerly termed Actinobacillus actinomycetemcomitans, a humanized animal model named Aa-hu-PBL-NOD/SCID was designed (83). NOD-SCID mice were reconstituted with periodontitis-affected patients-derived hu-PBLs and then orally inoculated with A. actinomycetemcomitans. From this experiment, relevant findings were obtained, including: i) The achievement of significant engraftment, between 30-60%, of human leukocytes (80); ii) After bacteria challenge, an increase in the number of B lymphocytes, CD4+ and CD8+ T lymphocytes, and monocytes/macrophages was observed in the periodontal compartment; however, without bacteria inoculation, human leukocytes were almost exclusively observed around bone marrow blood vessels in the proximity to the periodontium (80); iii) When CD4+ T lymphocytes were isolated from the mice oral mucosa, they revealed significantly higher activation and proliferation levels in response to bacterial infection, with a phenotype distribution similar to that observed in T-cells isolated from the periodontitis-affected donors (79); iv) An important A. actinomycetemcomitans-specific IgG antibody response was achieved and maintained over a 6 to 8 week period after bacteria inoculation (78); and v) A significant increment in the RANKL expression and alveolar bone resorption, as well as decreased OPG expression, was detected in response to A. actinomycetemcomitans infection, as compared with the absence of bacteria inoculation (82). Interestingly, when OPG was administered as a therapeutic strategy to protect mice against periodontitis, the levels of alveolar bone resorption were significantly reduced even after bacterial infection (78).
Afterward, Zhang et al. (83) assessed the role of suppressor of cytokine signaling (SOCS) molecules in A. actinomycetemcomitans-induced osteoclastogenesis by using a humanized model in which NOD/SCID mice were engrafted with hu-PBLs derived from periodontitis patients or age-matched healthy subjects. They achieved engraftment of ~30% of Hu-PBLs and concluded that the RANKL-mediated dendritic cell-related osteoclastogenesis was associated with an upregulation of SOCS and downregulation of SOCS3, the dominant-negative form of SOCS (83). Moreover, by using an HLA-DR1 humanized C57BL/6 mice model of periodontitis infected with P. gingivalis, the development of rheumatoid arthritis and its impact on bone density and systemic cytokine production were also analyzed (133). P. gingivalis gingival infection promoted a transient increase in the number of Th17 lymphocytes and higher systemic cytokine activity, femoral bone density loss, and production of anti-citrullinated protein antibodies, as compared with sham-infected mice controls (133). On the other hand, when human monocyte-derived dendritic cells were reconstituted in NSG mice to induce humanization, the exposure to a fimbriae-expressing mutant strain of P. gingivalis led to the formation of anti-apoptotic dendritic cells, which drove dampened Th1/Th17 responses and promoted a potent indoleamine-2,3-dioxygenase-dependent Treg response (84). In this context, the use of the humanized mice model accompanied by the in vivo tracking of pathogen-loaded dendritic cells contributed to validate the P. gingivalis-induced immunomodulation of dendritic cells and the intracellular bacteria persistence in distant organs. Particularly, P. gingivalis-primed dendritic cells promoted Treg activity to evade effector immune responses, by preventing the apoptosis of carrier dendritic cells and thus, favoring its systemic dissemination and survival (84).
Despite the huge progress achieved on revealing the pathogenesis of periodontitis using rodent models, the precise roles of human immune cells and molecular mediators have not been fully elucidated yet. Wild-type animal models have been extensively used to study the nature of immune response against periodontopathogens; nevertheless, oral mucosa colonizers in mice are not the same microorganisms found in human periodontal tissues (28, 134). In this context, human periodontopathogens inoculated in wild-type rodents or the induction of bacterial dysbiosis by the ligature placement around mice molars may not exactly reflect the host-microbiota interactions described in humans; thus, making the extrapolation of the findings to the human framework difficult and limited. Taking these antecedents into account, humanized mice models could provide robust physiological systems available to be exploited in the periodontal arena.
Indeed, the intricate inflammatory nature of periodontitis opens the possibility to propose the use of humanized mice models to specifically address scientific questions that might not be fully explained with conventional mice models. For instance, the first stages of the inflammatory response against periodontopathogens are enriched in mediators belonging to the innate arm of the immune response, which could be evaluated using the hu-HSCs model, as it allows the engraftment of hematopoietic precursors and further differentiation of a vast repertoire of immune cell populations (89, 90, 95–97). Nevertheless, if antigen presentation or dendritic cells are a matter of interest, this model may be inadequate as it lacks the HLA expression necessary for cell differentiation. Conversely, hu-PBLs mice models comprise a graft in which an important percentage of immune cells have already gone through a differentiation process (75, 76). Particularly, CD4+ T lymphocytes, the main cell population that settles into host tissues with this strategy, have an activated effector or memory phenotype, which means that they do not need to undergo cell differentiation into the mouse environment. In the case of periodontitis, CD4+ T lymphocytes have been identified as key cells that mediate the alveolar bone resorption (16–18); therefore, the hu-PBLs mice model would be useful to analyze committed immune-phenotype cells, not so for primary immune responses. In all these models, it is noteworthy that xenogeneic mismatch should be considered. Although it has been described from the first assays that an antigen-specific human immune response (both humoral and cell-mediated) can be achieved in SCID or NOD/SCID systems even in the presence of ongoing GvHD (135–137), the non-specific activation of human immune cells, detected, for example, as an IL-2 background production, could also occur (80). For this, MHC knockout and/or HLA transgenic mice platforms could represent a great opportunity to prevent or diminish any confounding factors given by GvHD development.
On the basis of the studies mentioned above, the use of humanized mice models for the study of the virulence of periodontal bacteria and the immunopathogenesis of periodontitis has proven to be feasible and particularly relevant (Figure 4). To ensure the success of this experimental strategy, it is highly recommended to adequately select both the murine platform and the humanization strategy, taking into account their advantages and limitations in the context of the study aims. In addition, a detailed characterization of the graft is necessary prior to any other experimental intervention, in order to ensure the standardization of humanization kinetics, adequate experimental work window, and reproducibility of the immunological findings developed in the host animal (115). Regarding therapeutic approaches, humanized animal models are an emerging topic in the field of periodontal research, so their development could be an important step in the search for innovative immunotherapeutic strategies applicable to periodontitis-affected patients.
Figure 4 Proposal for a humanized mouse model for the study of periodontitis. The placement of bilateral silk ligatures around the second maxillary molars of hu-PBL-NSG mice provokes local bacteria accumulation, periodontal microbiota dysbiosis, and bacteremia. Consequently, this bacterial insult results in systemic dissemination of antigens, bacterial debris, virulence factors, cytokines, and chemokines. In turn, they are capable of being recognized by the engrafted human peripheral blood mononuclear cells (PBMCs), which ultimately leads to the orchestration of a human-like immune response within the periodontal tissues and lymph nodes that drain them. The resulting local expansion and differentiation of the human PBMCs would lead to the formation of a periodontal lesion, characterized by the presence of a dense inflammatory infiltrate and alveolar bone resorption. Hu-PBLs, human peripheral blood lymphocytes. Created with BioRender.com.
The enhanced translational application and diversity of humanized mice models could innovate the way we study periodontal immune responses and consequently, prompt the designing of novel immunotherapeutics. However, there is an evolving need to overcome the complications related to the xenogeneic transfer of cells to immunodeficient hosts, which could compromise the study of this chronic disease and drug dynamics. Therefore, the proper characterization of the periodontitis humanized mice model should entail a high throughput analysis of the grafted human immune cells that repopulate the mice periodontium, potential antigen-presenting sites, and the remaining local and distal lymphoid organs. In this way, they could ensure staged profiling of the periodontitis-like immune response and avoid to the minimum its confusion with the GvHD-provoked immune response.
CR, EAC, and RV conceived the review. CR, EAC, and AP were involved in drafting the manuscript. MG designed and prepared the figures. LG-O, AS-C, and SM-R critically evaluated and supplemented the manuscript. EAC and RV revised and prepared the manuscript for submission. All authors contributed to the article and approved the submitted version.
This study was financially supported by grant FONDECYT 1181780 (RV) from the Agencia Nacional de Investigación y Desarrollo (ANID), Chile. EAC, AS-C, and SM-R were the recipients of Ph.D. scholarships from the Faculty of Dentistry, Universidad de Chile, Chile. CR and LG-O were the recipients of Ph.D. scholarships Fondecyt 21180841 and 21190087, respectively, from ANID.
The authors declare that the research was conducted in the absence of any commercial or financial relationships that could be construed as a potential conflict of interest.
1. Moutsopoulos NM, Konkel JE. Tissue-Specific Immunity at the Oral Mucosal Barrier. Trends Immunol (2018) 39(4):276–87. doi: 10.1016/j.it.2017.08.005
2. Moutsopoulos NM, Konkel JE. Healthy Mouth, Healthy Gut: A Dysbiotic Oral Microbiome Exacerbates Colitis. Mucosal Immunol (2020) 13(6):852–4. doi: 10.1038/s41385-020-00341-y
3. Acar B, Çağdaş D, Tan C, Özbek B, Yaz I, Yildirim YD, et al. Evaluation of Periodontal Status and Cytokine/Chemokine Profile of GCF in Patients With Severe Congenital Neutropenia. Odontology (2021) 109(2):474–82. doi: 10.1007/s10266-020-00565-1
4. Seidel CL, Gerlach RG, Wiedemann P, Weider M, Rodrian G, Hader M, et al. Defining Metaniches in the Oral Cavity According to Their Microbial Composition and Cytokine Profile. Int J Mol Sci (2020) 21(21):8218. doi: 10.3390/ijms21218218
5. Dashper SG, Mitchell HL, Seers CA, Gladman SL, Seemann T, Bulach DM, et al. Porphyromonas Gingivalis Uses Specific Domain Rearrangements and Allelic Exchange to Generate Diversity in Surface Virulence Factors. Front Microbiol (2017) 8:48. doi: 10.3389/fmicb.2017.00048
6. Monasterio G, Fernández B, Castillo F, Rojas C, Cafferata EA, Rojas L, et al. Capsular-Defective Porphyromonas Gingivalis Mutant Strains Induce Less Alveolar Bone Resorption Than W50 Wild-Type Strain Due to a Decreased Th1/Th17 Immune Response and Less Osteoclast Activity. J Periodontol (2019) 90(5):522–34. doi: 10.1002/JPER.18-0079
7. Boyer E, Leroyer P, Malherbe L, Fong SB, Loreal O, Bonnaure Mallet M, et al. Oral Dysbiosis Induced by Porphyromonas Gingivalis Is Strain-Dependent in Mice. J Oral Microbiol (2020) 12(1):1832837. doi: 10.1080/20002297.2020.1832837
8. Vernal R, Diaz-Guerra E, Silva A, Sanz M, Garcia-Sanz JA. Distinct Human T-Lymphocyte Responses Triggered by Porphyromonas Gingivalis Capsular Serotypes. J Clin Periodontol (2014) 41(1):19–30. doi: 10.1111/jcpe.12176
9. Vernal R, Díaz-Zúñiga J, Melgar-Rodríguez S, Pujol M, Díaz-Guerra E, Silva A, et al. Activation of RANKL-Induced Osteoclasts and Memory T Lymphocytes by Porphyromonas Gingivalis Is Serotype Dependant. J Clin Periodontol (2014) 41(5):451–9. doi: 10.1111/jcpe.12236
10. Díaz-Zúñiga J, Melgar-Rodríguez S, Alvarez C, Monasterio G, Benítez A, Ciuchi P, et al. T-Lymphocyte Phenotype and Function Triggered by Aggregatibacter Actinomycetemcomitans Is Serotype-Dependent. J Periodontal Res (2015) 50(6):824–35. doi: 10.1111/jre.12270
11. Fine DH, Karched M, Furgang D, Sampathkumar V, Velusamy S, Godboley D. Colonization and Persistence of Labeled and “Foreign” Strains of Aggregatibacter Actinomycetemcomitans Inoculated Into the Mouths of Rhesus Monkeys. J Oral Biol (2015) 2(1):10.13188/2377-987X.1000005. doi: 10.13188/2377-987X.1000005
12. Melgar-Rodríguez S, Díaz-Zúñiga J, Alvarez C, Rojas L, Monasterio G, Carvajal P, et al. Serotype B of Aggregatibacter Actinomycetemcomitans Increases Osteoclast and Memory T-Lymphocyte Activation. Mol Oral Microbiol (2015) 31(2):162–74. doi: 10.1111/omi.12112
13. Polak D, Shapira L, Weiss EI, Houri-Haddad Y. The Role of Coaggregation Between Porphyromonas Gingivalis and Fusobacterium Nucleatum on the Host Response to Mixed Infection. J Clin Periodontol (2012) 39(7):617–25. doi: 10.1111/j.1600-051X.2012.01889.x
14. Ebbers M, Lübcke PM, Volzke J, Kriebel K, Hieke C, Engelmann R, et al. Interplay Between P. Gingivalis, F. Nucleatum and A. Actinomycetemcomitans in Murine Alveolar Bone Loss, Arthritis Onset and Progression. Sci Rep (2018) 8(1):15129. doi: 10.1038/s41598-018-33129-z
15. Dutzan N, Abusleme L, Bridgeman H, Greenwell-Wild T, Zangerle-Murray T, Fife ME, et al. On-Going Mechanical Damage From Mastication Drives Homeostatic Th17 Cell Responses at the Oral Barrier. Immunity (2017) 46(1):133–47. doi: 10.1016/j.immuni.2016.12.010
16. Dutzan N, Abusleme L. T Helper 17 Cells as Pathogenic Drivers of Periodontitis. Adv Exp Med Biol (2019) 1197:107–17. doi: 10.1007/978-3-030-28524-1_9
17. Alvarez C, Monasterio G, Cavalla F, Córdova LA, Hernández M, Heymann D, et al. Osteoimmunology of Oral and Maxillofacial Diseases: Translational Applications Based on Biological Mechanisms. Front Immunol (2019) 10:1664. doi: 10.3389/fimmu.2019.01664
18. Monasterio G, Castillo F, Ibarra JP, Guevara J, Rojas L, Alvarez C, et al. Alveolar Bone Resorption and Th1/Th17-Associated Immune Response Triggered During Aggregatibacter Actinomycetemcomitans-Induced Experimental Periodontitis Are Serotype-Dependent. J Periodontol (2018) 89(10):1249–61. doi: 10.1002/JPER.17-0563
19. Alvarez C, Rojas C, Rojas L, Cafferata EA, Monasterio G, Vernal R. Regulatory T Lymphocytes in Periodontitis: A Translational View. Mediators Inflamm (2018) 2018:7806912. doi: 10.1155/2018/7806912
20. Alvarez C, Suliman S, Almarhoumi R, Vega ME, Rojas C, Monasterio G, et al. Regulatory T Cell Phenotype and Anti-Osteoclastogenic Function in Experimental Periodontitis. Sci Rep (2020) 10(1):19018. doi: 10.1038/s41598-020-76038-w
21. Cafferata EA, Jerez A, Vernal R, Monasterio G, Pandis N, Faggion CM Jr. The Therapeutic Potential of Regulatory T Lymphocytes in Periodontitis: A Systematic Review. J Periodontal Res (2019) 54(3):207–17. doi: 10.1111/jre.12629
22. Barbi J, Pardoll D, Pan F. Treg Functional Stability and Its Responsiveness to the Microenvironment. Immunol Rev (2014) 259(1):115–39. doi: 10.1111/imr.12172
23. Cafferata EA, Terraza-Aguirre C, Barrera R, Faúndez N, González N, Rojas C, et al. Interleukin-35 Inhibits Alveolar Bone Resorption by Modulating the Th17/Treg Imbalance During Periodontitis. J Clin Periodontol (2020) 47(6):676–88. doi: 10.1111/jcpe.13282
24. Nakajima T, Ueki-Maruyama K, Oda T, Ohsawa Y, Ito H, Seymour GJ, et al. Regulatory T-Cells Infiltrate Periodontal Disease Tissues. J Dent Res (2005) 84(7):639–43. doi: 10.1177/154405910508400711
25. Garlet GP, Cardoso CR, Mariano FS, Claudino M, de Assis GF, Campanelli AP, et al. Regulatory T Cells Attenuate Experimental Periodontitis Progression in Mice. J Clin Periodontol (2010) 37(7):591–600. doi: 10.1111/j.1600-051X.2010.01586.x
26. Dutzan N, Gamonal J, Silva A, Sanz M, Vernal R. Over-Expression of Forkhead Box P3 and Its Association With Receptor Activator of Nuclear Factor-Kappa B Ligand, Interleukin (IL)-17, IL-10 and Transforming Growth Factor-Beta During the Progression of Chronic Periodontitis. J Clin Periodontol (2009) 36(5):396–403. doi: 10.1111/j.1600-051X.2009.01390.x
27. Tsukasaki M, Komatsu N, Nagashima K, Nitta T, Pluemsakunthai W, Shukunami C, et al. Host Defense Against Oral Microbiota by Bone-Damaging T Cells. Nat Commun (2018) 9(1):701. doi: 10.1038/s41467-018-03147-6
28. Dutzan N, Kajikawa T, Abusleme L, Greenwell-Wild T, Zuazo CE, Ikeuchi T, et al. Dysbiotic Microbiome Triggers TH17 Cells to Mediate Oral Mucosal Immunopathology in Mice and Humans. Sci Transl Med (2018) 10(463):eaat0797. doi: 10.1126/scitranslmed.aat0797
29. Cafferata EA, Castro-Saavedra S, Fuentes-Barros G, Melgar-Rodríguez S, Rivera F, Carvajal P, et al. Boldine Inhibits the Alveolar Bone Resorption During Ligature-Induced Periodontitis by Modulating the Th17/Treg Imbalance. J Periodontol (2021) 92(1):123–36. doi: 10.1002/JPER.20-0055
30. Kikuta J, Wada Y, Kowada T, Wang Z, Sun-Wada GH, Nishiyama I, et al. Dynamic Visualization of RANKL and Th17-Mediated Osteoclast Function. J Clin Invest (2013) 123(2):866–73. doi: 10.1172/JCI65054
31. Bi CS, Wang J, Qu HL, Li X, Tian BM, Ge S, et al. Calcitriol Suppresses Lipopolysaccharide-Induced Alveolar Bone Damage in Rats by Regulating T Helper Cell Subset Polarization. J Periodontal Res (2019) 54(6):612–23. doi: 10.1111/jre.12661
32. Marchesan J, Girnary MS, Jing L, Miao MZ, Zhang S, Sun L, et al. An Experimental Murine Model to Study Periodontitis. Nat Protoc (2018) 13(10):2247–67. doi: 10.1038/s41596-018-0035-4
33. Saadi-Thiers K, Huck O, Simonis P, Tilly P, Fabre JE, Tenenbaum H, et al. Periodontal and Systemic Responses in Various Mice Models of Experimental Periodontitis: Respective Roles of Inflammation Duration and Porphyromonas Gingivalis Infection. J Periodontol (2013) 84(3):396–406. doi: 10.1902/jop.2012.110540
34. de Molon RS, Mascarenhas VI, de Avila ED, Finoti LS, Toffoli GB, Spolidorio DM, et al. Long-Term Evaluation of Oral Gavage With Periodontopathogens or Ligature Induction of Experimental Periodontal Disease in Mice. Clin Oral Investig (2016) 20(6):1203–16. doi: 10.1007/s00784-015-1607-0
35. de Molon RS, de Avila ED, Boas Nogueira AV, Chaves de Souza JA, Avila-Campos MJ, de Andrade CR, et al. Evaluation of the Host Response in Various Models of Induced Periodontal Disease in Mice. J Periodontol (2014) 85(3):465–77. doi: 10.1902/jop.2013.130225
36. Garlet GP, Cardoso CR, Silva TA, Ferreira BR, Avila-Campos MJ, Cunha FQ, et al. Cytokine Pattern Determines the Progression of Experimental Periodontal Disease Induced by Actinobacillus Actinomycetemcomitans Through the Modulation of MMPs, RANKL, and Their Physiological Inhibitors. Oral Microbiol Immunol (2006) 21(1):12–20. doi: 10.1111/j.1399-302X.2005.00245.x
37. Garlet GP, Cardoso CR, Campanelli AP, Garlet TP, Avila-Campos MJ, Cunha FQ, et al. The Essential Role of IFN-γ in the Control of Lethal Aggregatibacter Actinomycetemcomitans Infection in Mice. Microbes Infect (2008) 10(5):489–96. doi: 10.1016/j.micinf.2008.01.010
38. Palioto DB, Finoti LS, Kinane DF, Benakanakere M. Epigenetic and Inflammatory Events in Experimental Periodontitis Following Systemic Microbial Challenge. J Clin Periodontol (2019) 46(8):819–29. doi: 10.1111/jcpe.13151
39. Hamamoto Y, Ouhara K, Munenaga S, Shoji M, Ozawa T, Hisatsune J, et al. Effect of Porphyromonas Gingivalis Infection on Gut Dysbiosis and Resultant Arthritis Exacerbation in Mouse Model. Arthritis Res Ther (2020) 22(1):249. doi: 10.1186/s13075-020-02348-z
40. Monasterio G, Budini V, Fernández B, Castillo F, Rojas C, Alvarez C, et al. IL-22-Expressing CD4+Ahr+ T Lymphocytes Are Associated With RANKL-Mediated Alveolar Bone Resorption During Experimental Periodontitis. J Periodontal Res (2019) 54(5):513–24. doi: 10.1111/jre.12654
41. Monasterio G, Castillo F, Astorga J, Hoare A, Terraza-Aguirre C, Cafferata EA, et al. O-Polysaccharide Plays a Major Role on the Virulence and Immunostimulatory Potential of Aggregatibacter Actinomycetemcomitans During Periodontal Infection. Front Immunol (2020) 11:591240. doi: 10.3389/fimmu.2020.591240
42. Garlet GP, Avila-Campos MJ, Milanezi CM, Ferreira BR, Silva JS. Actinobacillus Actinomycetemcomitans-Induced Periodontal Disease in Mice: Patterns of Cytokine, Chemokine, and Chemokine Receptor Expression and Leukocyte Migration. Microbes Infect (2005) 7(4):738–47. doi: 10.1016/j.micinf.2005.01.012
43. Hao L, Chen J, Zhu Z, Reddy MS, Mountz JD, Chen W, et al. Odanacatib, A Cathepsin K-Specific Inhibitor, Inhibits Inflammation and Bone Loss Caused by Periodontal Diseases. J Periodontol (2015) 86(8):972–83. doi: 10.1902/jop.2015.140643
44. Wei W, Ren J, Yin W, Ding H, Lu Q, Tan L, et al. Inhibition of Ctsk Modulates Periodontitis With Arthritis Via Downregulation of TLR9 and Autophagy. Cell Prolif (2020) 53(1):e12722. doi: 10.1111/cpr.12722
45. Pan W, Yin W, Yang L, Xue L, Ren J, Wei W, et al. Inhibition of Ctsk Alleviates Periodontitis and Comorbid Rheumatoid Arthritis Via Downregulation of the TLR9 Signalling Pathway. J Clin Periodontol (2019) 46(3):286–96. doi: 10.1111/jcpe.13060
46. Wang X, Tong Y, Zhang J, Khan N, Zhang K, Bai H, et al. Neuroinflammation Changes With Periodontal Inflammation Status During Periodontitis in Wild-Type Mice. Oral Dis (2021) 27(4):1001–11. doi: 10.1111/odi.13618
47. Scanu A, Giraudo C, Galuppini F, Lazzarin V, Pennelli G, Sivolella S, et al. Periodontal Injection of Lipopolysaccharide Promotes Arthritis Development in Mice. Inflammation (2019) 42(3):1117–28. doi: 10.1007/s10753-019-00975-6
48. Chi L, Cheng X, He X, Sun J, Liang F, Pei Z, et al. Increased Cortical Infarction and Neuroinflammation in Ischemic Stroke Mice With Experimental Periodontitis. Neuroreport (2019) 30(6):428–33. doi: 10.1097/WNR.0000000000001220
49. Souza JAC, Magalhães FAC, Oliveira G, RS DEM, Zuanon JA, Souza PPC. Pam2CSK4 (TLR2 Agonist) Induces Periodontal Destruction in Mice. Braz Oral Res (2020) 34:e012. doi: 10.1590/1807-3107bor-2020.vol34.0012
50. Zhang J, Yu C, Zhang X, Chen H, Dong J, Lu W, et al. Porphyromonas Gingivalis Lipopolysaccharide Induces Cognitive Dysfunction, Mediated by Neuronal Inflammation Via Activation of the TLR4 Signaling Pathway in C57BL/6 Mice. J Neuroinflamm (2018) 15(1):37. doi: 10.1186/s12974-017-1052-x
51. Oz HS, Chen T, Ebersole JL. A Model for Chronic Mucosal Inflammation in IBD and Periodontitis. Dig Dis Sci (2010) 55(8):2194–202. doi: 10.1007/s10620-009-1031-x
52. Oz HS, Ebersole JL. A Novel Murine Model for Chronic Inflammatory Alveolar Bone Loss. J Periodontal Res (2010) 45(1):94–9. doi: 10.1111/j.1600-0765.2009.01207.x
53. Abe T, Hajishengallis G. Optimization of the Ligature-Induced Periodontitis Model in Mice. J Immunol Methods (2013) 394(1-2):49–54. doi: 10.1016/j.jim.2013.05.002
54. Alvarez C, Abdalla H, Suliman S, Rojas P, Wu Y-C, Almarhoumi R, et al. RvE1 Impacts the Gingival Inflammatory Infiltrate by the T Cell Response in Experimental Periodontitis. Front Immunol (2021) 12:664756. doi: 10.3389/fimmu.2021.664756
55. Huck O, Mulhall H, Rubin G, Kizelnik Z, Iyer R, Perpich JD, et al. Akkermansia Muciniphila Reduces Porphyromonas Gingivalis-Induced Inflammation and Periodontal Bone Destruction. J Clin Periodontol (2020) 47(2):202–12. doi: 10.1111/jcpe.13214
56. Batool F, Stutz C, Petit C, Benkirane-Jessel N, Delpy E, Zal F, et al. A Therapeutic Oxygen Carrier Isolated From Arenicola Marina Decreased P. Gingivalis Induced Inflammation and Tissue Destruction. Sci Rep (2020) 10(1):14745. doi: 10.1038/s41598-020-71593-8
57. Huck O, Al-Hashemi J, Poidevin L, Poch O, Davideau JL, Tenenbaum H, et al. Identification and Characterization of microRNA Differentially Expressed in Macrophages Exposed to Porphyromonas Gingivalis Infection. Infect Immun (2017) 85(3):e00771–16. doi: 10.1128/IAI.00771-16
58. Ishihara Y, Anan H, Yoneda M, Maeda K, Hirofuji T. Susceptibility of Type 2 Diabetic Mice to Low-Virulence Bacterial Infection: Induction of Abscess Formation by Gingipain-Deficient Porphyromonas Gingivalis. J Periodontal Res (2007) 42(3):253–8. doi: 10.1111/j.1600-0765.2006.00941.x
59. Rydell-Törmänen K, Johnson JR. The Applicability of Mouse Models to the Study of Human Disease. Methods Mol Biol (2019) 1940:3–22. doi: 10.1007/978-1-4939-9086-3_1
60. Graves DT, Kang J, Andriankaja O, Wada K, Rossa C Jr. Animal Models to Study Host-Bacteria Interactions Involved in Periodontitis. Front Oral Biol (2012) 15:117–32. doi: 10.1159/000329675
61. de Molon RS, de Avila ED, Cirelli JA. Host Responses Induced by Different Animal Models of Periodontal Disease: A Literature Review. J Investig Clin Dent (2013) 4(4):211–8. doi: 10.1111/jicd.12018
62. Wiebe CB, Adkins CA, Putnins EE, Hakkinen L, Larjava HS. Naturally Occurring Periodontal Bone Loss in the Wild Deer Mouse, Genus. Peromyscus J Periodontol (2001) 72(5):620–5. doi: 10.1902/jop.2001.72.5.620
63. Polak D, Wilensky A, Shapira L, Halabi A, Goldstein D, Weiss EI, et al. Mouse Model of Experimental Periodontitis Induced by Porphyromonas Gingivalis/Fusobacterium Nucleatum Infection: Bone Loss and Host Response. J Clin Periodontol (2009) 36(5):406–10. doi: 10.1111/j.1600-051X.2009.01393.x
64. Glowacki AJ, Yoshizawa S, Jhunjhunwala S, Vieira AE, Garlet GP, Sfeir C, et al. Prevention of Inflammation-Mediated Bone Loss in Murine and Canine Periodontal Disease Via Recruitment of Regulatory Lymphocytes. Proc Natl Acad Sci USA (2013) 110(46):18525–30. doi: 10.1073/pnas.1302829110
65. Barros SP, Arce RM, Galloway P, Lawter R, Offenbacher S. Therapeutic Effect of a Topical CCR2 Antagonist on Induced Alveolar Bone Loss in Mice. J Periodontal Res (2011) 46(2):246–51. doi: 10.1111/j.1600-0765.2010.01340.x
66. Nahid MA, Rivera M, Lucas A, Chan EK, Kesavalu L. Polymicrobial Infection With Periodontal Pathogens Specifically Enhances microRNA miR-146a in ApoE-/- Mice During Experimental Periodontal Disease. Infect Immun (2011) 79(4):1597–605. doi: 10.1128/IAI.01062-10
67. Lalla E, Lamster IB, Hofmann MA, Bucciarelli L, Jerud AP, Tucker S, et al. Oral Infection With a Periodontal Pathogen Accelerates Early Atherosclerosis in Apolipoprotein E-null Mice. Arterioscler Thromb Vasc Biol (2003) 23(8):1405–11. doi: 10.1161/01.ATV.0000082462.26258.FE
68. Garcia de Aquino S, Manzolli Leite FR, Stach-Machado DR, Francisco da Silva JA, Spolidorio LC, Rossa C Jr. Signaling Pathways Associated With the Expression of Inflammatory Mediators Activated During the Course of Two Models of Experimental Periodontitis. Life Sci (2009) 84(21-22):745–54. doi: 10.1016/j.lfs.2009.03.001
69. Nakamura H, Fukusaki Y, Yoshimura A, Shiraishi C, Kishimoto M, Kaneko T, et al. Lack of Toll-Like Receptor 4 Decreases Lipopolysaccharide-Induced Bone Resorption in C3H/HeJ Mice In Vivo. Oral Microbiol Immunol (2008) 23(3):190–5. doi: 10.1111/j.1399-302X.2007.00410.x
70. de Molon RS, Park CH, Jin Q, Sugai J, Cirelli JA. Characterization of Ligature-Induced Experimental Periodontitis. Microsc Res Tech (2018) 81(12):1412–21. doi: 10.1002/jemt.23101
71. Graves DT, Fine D, Teng YT, Van Dyke TE, Hajishengallis G. The Use of Rodent Models to Investigate Host-Bacteria Interactions Related to Periodontal Diseases. J Clin Periodontol (2008) 35(2):89–105. doi: 10.1111/j.1600-051X.2007.01172.x
72. Hajishengallis G. Periodontitis: From Microbial Immune Subversion to Systemic Inflammation. Nat Rev Immunol (2015) 15(1):30–44. doi: 10.1038/nri3785
73. Häärä O, Harjunmaa E, Lindfors PH, Huh SH, Fliniaux I, Äberg T, et al. Ectodysplasin Regulates Activator-Inhibitor Balance in Murine Tooth Development Through Fgf20 Signaling. Development (2012) 139(17):3189–99. doi: 10.1242/dev.079558
74. Li D, Feng Y, Tang H, Huang L, Tong Z, Hu C, et al. A Simplified and Effective Method for Generation of Experimental Murine Periodontitis Model. Front Bioeng Biotechnol (2020) 8:444. doi: 10.3389/fbioe.2020.00444
75. Shultz LD, Brehm MA, Garcia-Martinez JV, Greiner DL. Humanized Mice for Immune System Investigation: Progress, Promise and Challenges. Nat Rev Immunol (2012) 12(11):786–98. doi: 10.1038/nri3311
76. Shultz LD, Ishikawa F, Greiner DL. Humanized Mice in Translational Biomedical Research. Nat Rev Immunol (2007) 7(2):118–30. doi: 10.1038/nri2017
77. Walsh NC, Kenney LL, Jangalwe S, Aryee KE, Greiner DL, Brehm MA, et al. Humanized Mouse Models of Clinical Disease. Annu Rev Pathol (2017) 12:187–215. doi: 10.1146/annurev-pathol-052016-100332
78. Teng YT, Nguyen H, Gao X, Kong YY, Gorczynski RM, Singh B, et al. Functional Human T-Cell Immunity and Osteoprotegerin Ligand Control Alveolar Bone Destruction in Periodontal Infection. J Clin Invest (2000) 106(6):59–67. doi: 10.1172/jci10763
79. Gao X, Teng YT. T-Cell-Receptor Gene Usage of Actinobacillus Actinomycetemcomitans-Reactive Periodontal CD4+ T Cells From Localized Juvenile Periodontitis Patients and Human Peripheral Blood Leukocyte-Reconstituted NOD/SCID Mice. J Periodontal Res (2002) 37(5):399–404. doi: 10.1034/j.1600-0765.2002.01006.x
80. Teng YT, Nguyen H, Hassanloo A, Ellen RP, Hozumi N, Gorczynski RM. Periodontal Immune Responses of Human Lymphocytes in Actinobacillus Actinomycetemcomitans-Inoculated NOD/SCID Mice Engrafted With Peripheral Blood Leukocytes of Periodontitis Patients. J Periodontal Res (1999) 34(1):54–61. doi: 10.1111/j.1600-0765.1999.tb02222.x
81. Shenker BJ, Wannberg S, Vitale L, Kieba I, Lally ET. Reconstruction of Severe Combined Immunodeficient Mice With Lymphocytes From Patients With Localized Juvenile Periodontitis. J Periodontal Res (1993) 28(6 Pt 2):487–90. doi: 10.1111/j.1600-0765.1993.tb02109.x
82. Teng YT. Mixed Periodontal Th1-Th2 Cytokine Profile in Actinobacillus Actinomycetemcomitans-Specific Osteoprotegerin Ligand (or RANK-L)- Mediated Alveolar Bone Destruction In Vivo. Infect Immun (2002) 70(9):5269–73. doi: 10.1128/iai.70.9.5269-5273.2002
83. Zhang X, Alnaeeli M, Singh B, Teng YT. Involvement of SOCS3 in Regulation of CD11c+ Dendritic Cell-Derived Osteoclastogenesis and Severe Alveolar Bone Loss. Infect Immun (2009) 77(5):2000–9. doi: 10.1128/IAI.01070-08
84. Tyagi RK, Miles B, Parmar R, Garg NK, Dalai SK, Baban B, et al. Human IDO-Competent, Long-Lived Immunoregulatory Dendritic Cells Induced by Intracellular Pathogen, and Their Fate in Humanized Mice. Sci Rep (2017) 7:41083. doi: 10.1038/srep41083
85. Woodland RT, Schmidt MR. Homeostatic Proliferation of B Cells. Semin Immunol (2005) 17(3):209–17. doi: 10.1016/j.smim.2005.02.006
86. King M, Pearson T, Shultz LD, Leif J, Bottino R, Trucco M, et al. A New Hu-PBL Model for the Study of Human Islet Alloreactivity Based on NOD-scid Mice Bearing a Targeted Mutation in the IL-2 Receptor Gamma Chain Gene. Clin Immunol (2008) 126(3):303–14. doi: 10.1016/j.clim.2007.11.001
87. King MA, Covassin L, Brehm MA, Racki W, Pearson T, Leif J, et al. Human Peripheral Blood Leucocyte Non-Obese Diabetic-Severe Combined Immunodeficiency Interleukin-2 Receptor Gamma Chain Gene Mouse Model of Xenogeneic Graft-Versus-Host-Like Disease and the Role of Host Major Histocompatibility Complex. Clin Exp Immunol (2009) 157(1):104–18. doi: 10.1111/j.1365-2249.2009.03933.x
88. Brehm MA, Kenney LL, Wiles MV, Low BE, Tisch RM, Burzenski L, et al. Lack of Acute Xenogeneic Graft-Versus-Host Disease, But Retention of T-Cell Function Following Engraftment of Human Peripheral Blood Mononuclear Cells in NSG Mice Deficient in MHC Class I and II Expression. FASEB J (2019) 33(3):3137–51. doi: 10.1096/fj.201800636R
89. Ito M, Hiramatsu H, Kobayashi K, Suzue K, Kawahata M, Hioki K, et al. Nod/Scid/γcnull Mouse: An Excellent Recipient Mouse Model for Engraftment of Human Cells. Blood (2002) 100(9):3175–82. doi: 10.1182/blood-2001-12-0207
90. Ishikawa F, Yasukawa M, Lyons B, Yoshida S, Miyamoto T, Yoshimoto G, et al. Development of Functional Human Blood and Immune Systems in NOD/SCID/IL2 Receptor γ Chainnull Mice. Blood (2005) 106(5):1565–73. doi: 10.1182/blood-2005-02-0516
91. Holyoake TL, Nicolini FE, Eaves CJ. Functional Differences Between Transplantable Human Hematopoietic Stem Cells From Fetal Liver, Cord Blood, and Adult Marrow. Exp Hematol (1999) 27(9):1418–27. doi: 10.1016/s0301-472x(99)00078-8
92. Lapidot T, Pflumio F, Doedens M, Murdoch B, Williams DE, Dick JE. Cytokine Stimulation of Multilineage Hematopoiesis From Immature Human Cells Engrafted in SCID Mice. Science (1992) 255(5048):1137–41. doi: 10.1126/science.1372131
93. Brehm MA, Racki WJ, Leif J, Burzenski L, Hosur V, Wetmore A, et al. Engraftment of Human HSCs in Nonirradiated Newborn NOD-scid Il2rγnull Mice Is Enhanced by Transgenic Expression of Membrane-Bound Human SCF. Blood (2012) 119(12):2778–88. doi: 10.1182/blood-2011-05-353243
94. McIntosh BE, Brown ME, Duffin BM, Maufort JP, Vereide DT, Slukvin II, et al. Nonirradiated NOD,B6.SCID Il2rγ-/- KitW41/W41 (NBSGW) Mice Support Multilineage Engraftment of Human Hematopoietic Cells. Stem Cell Rep (2015) 4(2):171–80. doi: 10.1016/j.stemcr.2014.12.005
95. Shultz LD, Lyons BL, Burzenski LM, Gott B, Chen X, Chaleff S, et al. Human Lymphoid and Myeloid Cell Development in NOD/LtSz-scid Il2rγnull Mice Engrafted With Mobilized Human Hemopoietic Stem Cells. J Immunol (2005) 174(10):6477–89. doi: 10.4049/jimmunol.174.10.6477
96. Traggiai E, Chicha L, Mazzucchelli L, Bronz L, Piffaretti JC, Lanzavecchia A, et al. Development of a Human Adaptive Immune System in Cord Blood Cell-Transplanted Mice. Science (2004) 304(5667):104–7. doi: 10.1126/science.1093933
97. Hiramatsu H, Nishikomori R, Heike T, Ito M, Kobayashi K, Katamura K, et al. Complete Reconstitution of Human Lymphocytes From Cord Blood CD34+ Cells Using the Mice Model. Blood (2003) 102(3):873–80. doi: 10.1182/blood-2002-09-2755
98. Lan P, Tonomura N, Shimizu A, Wang S, Yang YG. Reconstitution of a Functional Human Immune System in Immunodeficient Mice Through Combined Human Fetal Thymus/Liver and CD34+ Cell Transplantation. Blood (2006) 108(2):487–92. doi: 10.1182/blood-2005-11-4388
99. Durost PA, Aryee KE, Manzoor F, Tisch RM, Mueller C, Jurczyk A, et al. Gene Therapy With an Adeno-Associated Viral Vector Expressing Human Interleukin-2 Alters Immune System Homeostasis in Humanized Mice. Hum Gene Ther (2018) 29(3):352–65. doi: 10.1089/hum.2017.072
100. Onoe T, Kalscheuer H, Danzl N, Chittenden M, Zhao G, Yang YG, et al. Human Natural Regulatory T Cell Development, Suppressive Function, and Postthymic Maturation in a Humanized Mouse Model. J Immunol (2011) 187(7):3895–903. doi: 10.4049/jimmunol.1100394
101. Greenblatt MB, Vrbanac V, Tivey T, Tsang K, Tager AM, Aliprantis AO. Graft Versus Host Disease in the Bone Marrow, Liver and Thymus Humanized Mouse Model. PLoS One (2012) 7(9):e44664. doi: 10.1371/journal.pone.0044664
102. Shultz LD, Pearson T, King M, Giassi L, Carney L, Gott B, et al. Humanized NOD/LtSz-scid IL2 Receptor Common Gamma Chain Knockout Mice in Diabetes Research. Ann N Y Acad Sci (2007) 1103:77–89. doi: 10.1196/annals.1394.002
103. Bosma GC, Custer RP, Bosma MJ. A Severe Combined Immunodeficiency Mutation in the Mouse. Nature (1983) 301(5900):527–30. doi: 10.1038/301527a0
104. Mosier DE, Gulizia RJ, Baird SM, Wilson DB. Transfer of a Functional Human Immune System to Mice With Severe Combined Immunodeficiency. Nature (1988) 335(6187):256–9. doi: 10.1038/335256a0
105. Shinkai Y, Rathbun G, Lam KP, Oltz EM, Stewart V, Mendelsohn M, et al. Rag-2-Deficient Mice Lack Mature Lymphocytes Owing to Inability to Initiate V(D)J Rearrangement. Cell (1992) 68(5):855–67. doi: 10.1016/0092-8674(92)90029-c
106. Mombaerts P, Iacomini J, Johnson RS, Herrup K, Tonegawa S, Papaioannou VE. Rag-1-Deficient Mice Have No Mature B and T Lymphocytes. Cell (1992) 68(5):869–77. doi: 10.1016/0092-8674(92)90030-g
107. Shultz LD, Schweitzer PA, Christianson SW, Gott B, Schweitzer IB, Tennent B, et al. Multiple Defects in Innate and Adaptive Immunologic Function in NOD/LtSz-scid Mice. J Immunol (1995) 154(1):180–91.
108. Serreze DV, Gaskins HR, Leiter EH. Defects in the Differentiation and Function of Antigen Presenting Cells in NOD/Lt Mice. J Immunol (1993) 150(6):2534–43.
109. Hesselton RM, Greiner DL, Mordes JP, Rajan TV, Sullivan JL, Shultz LD. High Levels of Human Peripheral Blood Mononuclear Cell Engraftment and Enhanced Susceptibility to Human Immunodeficiency Virus Type 1 Infection in NOD/LtSz-scid/scid Mice. J Infect Dis (1995) 172(4):974–82. doi: 10.1093/infdis/172.4.974
110. Lowry PA, Shultz LD, Greiner DL, Hesselton RM, Kittler EL, Tiarks CY, et al. Improved Engraftment of Human Cord Blood Stem Cells in NOD/LtSz-scid/scid Mice After Irradiation or Multiple-Day Injections Into Unirradiated Recipients. Biol Blood Marrow Transplant (1996) 2(1):15–23.
111. Liao W, Lin JX, Leonard WJ. IL-2 Family Cytokines: New Insights Into the Complex Roles of IL-2 as a Broad Regulator of T Helper Cell Differentiation. Curr Opin Immunol (2011) 23(5):598–604. doi: 10.1016/j.coi.2011.08.003
112. Yahata T, Ando K, Nakamura Y, Ueyama Y, Shimamura K, Tamaoki N, et al. Functional Human T Lymphocyte Development From Cord Blood CD34+ Cells in Nonobese Diabetic/Shi-Scid, IL-2 Receptor γ Null Mice. J Immunol (2002) 169(1):204–9. doi: 10.4049/jimmunol.169.1.204
113. DiSanto JP, Müller W, Guy-Grand D, Fischer A, Rajewsky K. Lymphoid Development in Mice With a Targeted Deletion of the Interleukin 2 Receptor γ Chain. Proc Natl Acad Sci USA (1995) 92(2):377–81. doi: 10.1073/pnas.92.2.377
114. Cao X, Shores EW, Hu-Li J, Anver MR, Kelsall BL, Russell SM, et al. Defective Lymphoid Development in Mice Lacking Expression of the Common Cytokine Receptor γ Chain. Immunity (1995) 2(3):223–38. doi: 10.1016/1074-7613(95)90047-0
115. Allen TM, Brehm MA, Bridges S, Ferguson S, Kumar P, Mirochnitchenko O, et al. Humanized Immune System Mouse Models: Progress, Challenges and Opportunities. Nat Immunol (2019) 20(7):770–4. doi: 10.1038/s41590-019-0416-z
116. Schinnerling K, Rosas C, Soto L, Thomas R, Aguillón JC. Humanized Mouse Models of Rheumatoid Arthritis for Studies on Immunopathogenesis and Preclinical Testing of Cell-Based Therapies. Front Immunol (2019) 10:203. doi: 10.3389/fimmu.2019.00203
117. Ali N, Flutter B, Sanchez Rodriguez R, Sharif-Paghaleh E, Barber LD, Lombardi G, et al. Xenogeneic Graft-Versus-Host-Disease in NOD-scid Il-2rγnull Mice Display a T-Effector Memory Phenotype. PLoS One (2012) 7(8):e44219. doi: 10.1371/journal.pone.0044219
118. Danner R, Chaudhari SN, Rosenberger J, Surls J, Richie TL, Brumeanu TD, et al. Expression of HLA Class II Molecules in Humanized NOD.Rag1KO.IL2RgcKO Mice Is Critical for Development and Function of Human T and B Cells. PLoS One (2011) 6(5):e19826. doi: 10.1371/journal.pone.0019826
119. Covassin L, Laning J, Abdi R, Langevin DL, Phillips NE, Shultz LD, et al. Human Peripheral Blood CD4 T Cell-Engrafted Non-Obese Diabetic-Scid IL2rγnullTg (Human Leucocyte Antigen D-Related 4) Mice: A Mouse Model of Human Allogeneic Graft-Versus-Host Disease. Clin Exp Immunol (2011) 166(2):269–80. doi: 10.1111/j.1365-2249.2011.04462.x
120. Sarkar S, Heise MT. Mouse Models as Resources for Studying Infectious Diseases. Clin Ther (2019) 41(10):1912–22. doi: 10.1016/j.clinthera.2019.08.010
121. Seymour R, Sundberg JP, Hogenesch H. Abnormal Lymphoid Organ Development in Immunodeficient Mutant Mice. Vet Pathol (2006) 43(4):401–23. doi: 10.1354/vp.43-4-401
122. Santagostino SF, Arbona RJR, Nashat MA, White JR, Monette S. Pathology of Aging in NOD Scid Gamma Female Mice. Vet Pathol (2017) 54(5):855–69. doi: 10.1177/0300985817698210
123. Gonzalez L, Strbo N, Podack ER. Humanized Mice: Novel Model for Studying Mechanisms of Human Immune-Based Therapies. Immunol Res (2013) 57(1-3):326–34. doi: 10.1007/s12026-013-8471-2
124. Misharin AV, Haines GK III, Rose S, Gierut AK, Hotchkiss RS, Perlman H. Development of a New Humanized Mouse Model to Study Acute Inflammatory Arthritis. J Transl Med (2012) 10:190. doi: 10.1186/1479-5876-10-190
125. Guo J, Li Y, Shan Y, Shu C, Wang F, Wang X, et al. Humanized Mice Reveal an Essential Role for Human Hepatocytes in the Development of the Liver Immune System. Cell Death Dis (2018) 9(6):667. doi: 10.1038/s41419-018-0720-9
126. Arrey F, Löwe D, Kuhlmann S, Kaiser P, Moura-Alves P, Krishnamoorthy G, et al. Humanized Mouse Model Mimicking Pathology of Human Tuberculosis for In Vivo Evaluation of Drug Regimens. Front Immunol (2019) 10:89. doi: 10.3389/fimmu.2019.00089
127. Vudattu NK, Waldron-Lynch F, Truman LA, Deng S, Preston-Hurlburt P, Torres R, et al. Humanized Mice as a Model for Aberrant Responses in Human T Cell Immunotherapy. J Immunol (2014) 193(2):587–96. doi: 10.4049/jimmunol.1302455
128. Herndler-Brandstetter D, Shan L, Yao Y, Stecher C, Plajer V, Lietzenmayer M, et al. Humanized Mouse Model Supports Development, Function, and Tissue Residency of Human Natural Killer Cells. Proc Natl Acad Sci USA (2017) 114(45):E9626–E34. doi: 10.1073/pnas.1705301114
129. Bendall SC, Nolan GP, Roederer M, Chattopadhyay PK. A Deep Profiler’s Guide to Cytometry. Trends Immunol (2012) 33(7):323–32. doi: 10.1016/j.it.2012.02.010
130. Bandura DR, Baranov VI, Ornatsky OI, Antonov A, Kinach R, Lou X, et al. Mass Cytometry: Technique for Real Time Single Cell Multitarget Immunoassay Based on Inductively Coupled Plasma Time-of-Flight Mass Spectrometry. Anal Chem (2009) 81(16):6813–22. doi: 10.1021/ac901049w
131. Chang Q, Ornatsky O, Hedley D. Staining of Frozen and Formalin-Fixed, Paraffin-Embedded Tissues With Metal-Labeled Antibodies for Imaging Mass Cytometry Analysis. Curr Protoc Cytom (2017) 82:12.47.1–12.47.8. doi: 10.1002/cpcy.29
132. Curran M, Mairesse M, Matas-Céspedes A, Bareham B, Pellegrini G, Liaunardy A, et al. Recent Advancements and Applications of Human Immune System Mice in Preclinical Immuno-Oncology. Toxicol Pathol (2020) 48(2):302–16. doi: 10.1177/0192623319886304
133. Sandal I, Karydis A, Luo J, Prislovsky A, Whittington KB, Rosloniec EF, et al. Bone Loss and Aggravated Autoimmune Arthritis in HLA-Drβ1-Bearing Humanized Mice Following Oral Challenge With Porphyromonas Gingivalis. Arthritis Res Ther (2016) 18(1):249. doi: 10.1186/s13075-016-1143-6
134. Abusleme L, Hong BY, Hoare A, Konkel JE, Diaz PI, Moutsopoulos NM. Oral Microbiome Characterization in Murine Models. Bio Protoc (2017) 7(24):e2655. doi: 10.21769/BioProtoc.2655
135. Sandhu J, Shpitz B, Gallinger S, Hozumi N. Human Primary Immune Response in SCID Mice Engrafted With Human Peripheral Blood Lymphocytes. J Immunol (1994) 152(8):3806–13.
136. Hozumi N, Gorczynski R, Peters W, Sandhu JS. A SCID Mouse Model for Human Immune Response and Disease. Res Immunol (1994) 145(5):370–9. doi: 10.1016/s0923-2494(94)80202-5
Keywords: periodontitis, animal model, humanized mice, immunopathogenesis, immunotherapy
Citation: Rojas C, García MP, Polanco AF, González-Osuna L, Sierra-Cristancho A, Melgar-Rodríguez S, Cafferata EA and Vernal R (2021) Humanized Mouse Models for the Study of Periodontitis: An Opportunity to Elucidate Unresolved Aspects of Its Immunopathogenesis and Analyze New Immunotherapeutic Strategies. Front. Immunol. 12:663328. doi: 10.3389/fimmu.2021.663328
Received: 02 February 2021; Accepted: 10 May 2021;
Published: 17 June 2021.
Edited by:
Qingfeng Chen, Institute of Molecular and Cell Biology (A*STAR), SingaporeReviewed by:
Christopher W. Cutler, Augusta University, United StatesCopyright © 2021 Rojas, García, Polanco, González-Osuna, Sierra-Cristancho, Melgar-Rodríguez, Cafferata and Vernal. This is an open-access article distributed under the terms of the Creative Commons Attribution License (CC BY). The use, distribution or reproduction in other forums is permitted, provided the original author(s) and the copyright owner(s) are credited and that the original publication in this journal is cited, in accordance with accepted academic practice. No use, distribution or reproduction is permitted which does not comply with these terms.
*Correspondence: Rolando Vernal, cnZlcm5hbEB1Y2hpbGUuY2w=; Emilio A. Cafferata, ZWNhZmZlcmF0YUBjaWVudGlmaWNhLmVkdS5wZQ==
†ORCID: Carolina Rojas, orcid.org/0000-0003-4004-3999
Michelle P. García, orcid.org/0000-0002-4283-7269
Alan F. Polanco, orcid.org/0000-0002-3883-7733
Luis González-Osuna, orcid.org/0000-0002-8456-6372
Alfredo Sierra-Cristancho, orcid.org/0000-0003-0952-9835
Samanta Melgar-Rodríguez, orcid.org/0000-0003-1480-5734
Emilio A. Cafferata, orcid.org/0000-0001-9243-1382
Rolando Vernal, orcid.org/0000-0002-1391-320X
Disclaimer: All claims expressed in this article are solely those of the authors and do not necessarily represent those of their affiliated organizations, or those of the publisher, the editors and the reviewers. Any product that may be evaluated in this article or claim that may be made by its manufacturer is not guaranteed or endorsed by the publisher.
Research integrity at Frontiers
Learn more about the work of our research integrity team to safeguard the quality of each article we publish.